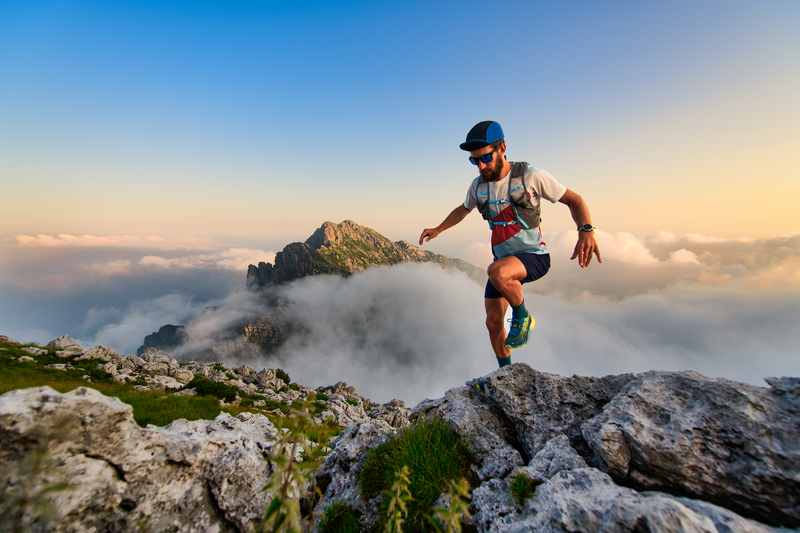
94% of researchers rate our articles as excellent or good
Learn more about the work of our research integrity team to safeguard the quality of each article we publish.
Find out more
REVIEW article
Front. Virol. , 06 April 2022
Sec. Translational Virology
Volume 2 - 2022 | https://doi.org/10.3389/fviro.2022.831754
This article is part of the Research Topic Translational Virology in Pregnancy View all 12 articles
Throughout gestation, the placenta is vital for proper development of the fetus. Disruptions in trophoblast, the main functional cell type of the placenta, stress the pregnancy, with potential adverse outcomes for both mother and baby. While the placenta typically functions as an effective pathogen barrier to protect the fetus, there are scenarios in which viral infections either cross the placenta or disturb its function. Here, we briefly review placental morphologic and functional changes across pregnancy and how these relate to routes for and protection from pathogens. We then explore the potential advantages and limitations of the current primary trophoblast models (primary cultures/explants, cell lines, trophoblast stem cells and trophoblast organoids) and stem cell-derived trophoblast models (naïve and primed embryonic stem cells [ESCs], and blastoids) and discuss these in the context of what is already known about (1) how viruses cross the placenta and the mechanisms that are used for its protection and (2) how these protective mechanisms change across gestation.
The success of human pregnancy relies on a healthy and functional placenta. The placenta is responsible for gas, nutrient, and waste exchange while concurrently functioning to protect the fetus from dangerous substances or microorganisms originating in the mother. While the placenta protects against many pathogens, there are multiple examples of maternal infections that cause adverse pregnancy outcomes or congenital deficits in the fetus. The most well-known are complications associated with the “TORCH” pathogens, which include Toxoplasma gondii, other [including Zika virus (ZIKV; Flaviviridae; Flavivirus) and human immunodeficiency virus (HIV; Retroviridae; Lentivirus)], rubella virus (RuV; Matonaviridae; Rubivirus), human cytomegalovirus (HCMV; Herpesviridae; Cytomegalovirus), and herpes simplex virus 1 and 2 (HSV-1, HSV-2; Herpesviridae; Simplexvirus) (1). Researchers are currently investigating whether severe acute respiratory syndrome coronavirus 2 (SARS-CoV-2; Coronaviridae; Betacoronavirus) can cross the placenta as well. Given the current pandemic and threats of future viral outbreaks, it is important to understand how the placenta can succeed or fail in protecting the fetus from maternal infections. In this review, we focus on how viruses can cross the placenta throughout gestation and envision how researchers can utilize newly derived human placental models to further our understanding of these processes.
The placenta originates from the trophectoderm, the outermost layer of the human blastocyst (Figure 1A). Initial implantation of the embryo into the maternal endometrium (decidua) begins with the formation of a highly invasive primary syncytiotrophoblast (SCT) layer migrating ahead of a zone of proliferating cytotrophoblast (Figure 1B). This early, pre-villous placenta has been defined as primitive trophoblast. During this stage of pregnancy, crossing the SCT is the only placental route for vertical (mother-to-fetus) transmission of viruses to the fetus. Little is known about the human primitive placenta and what is known stems from rare, archived hysterectomy samples and non-human primate research (2–4). At this early time in its development, the placenta is essentially nourished entirely by glandular secretions in the maternal decidua (5). While studying this peri-implantation phase in humans is nearly impossible, murine models suggest that the placenta can enable vertical virus transmission during this time window, i.e., prior to mature hemochorial placental formation (6–8). A human cohort study estimated congenital HCMV infection occurred at a rate of 45% after maternal periconceptional infection (defined as one week before and five weeks after the last menstrual period) providing further evidence of placental susceptibility during this time window (9).
Figure 1 Morphology of the human placenta across gestation. (A) Just prior to implantation, the blastocyst is comprised of an inner cell mass (yellow) that is surrounded by a single layer of trophoblast, termed the trophectoderm (blue). (B) Once the embryo contacts the maternal decidua, the trophectoderm forms a highly invasive primitive syncytium (purple). This multi-nucleated syncytium contacts maternal glands that provide histotroph to nourish the growing embryo. Beneath the primitive syncytium, the villous cytotrophoblast cells (VCT; blue) proliferate and eventually break through the primitive syncytium to generate tree-like primary and, ultimately, more mature villous structures. (C) Villi that reach and anchor to the maternal decidua are the source of the cytotrophoblast cells that will form the cytotrophoblastic shell (blue). Extravillous cytotrophoblast cells (EVT, green) invade into the decidua and interact with maternal cells and plug the maternal spiral arteries to prevent onset of maternal blood flow into the space between the placental villi, called the intervillous space. (D) Near the end of the first trimester, the EVT plugs dissolve and the EVT continue to remodel the spiral arteries to establish robust maternal blood flow into the intervillous space. (E) By the end of pregnancy, the VCT layer becomes sparse and the cytotrophoblast shell discontinuous.
As pregnancy progresses, the proliferative cytotrophoblast cells eventually break through the primitive syncytium and begin forming the primary chorionic villous structures. Villous cytotrophoblast cells (VCT) fuse to form a continuous layer of non-invasive multi-nucleated SCT (Figure 1C). At the distal ends of some of these villi (anchoring villi), the SCT layer is absent and instead, the VCT form cytotrophoblast cell columns (CCC) that anchor the placenta to the basal plate. In early pregnancy, this external layer of cytotrophoblast cells is known as the cytotrophoblastic shell, which spreads laterally to form a continuous layer between the anchoring villi and the decidua basalis (10, 11). The cytotrophoblast cells within the cell columns subsequently differentiate into extravillous trophoblast cells (EVT), which invade deeply into the decidua (Figure 1C). Initially, these EVT also form plugs in the maternal spiral arteries to prevent blood flow to the developing villous structures. The CCC and EVT are in contact with cells residing in the maternal decidua and serve as a potential route for viral transmission to the fetus via the villous placenta prior to onset of maternal blood flow into the intervillous space.
Around the 10th week of pregnancy, the EVT plugs dissipate and the EVT continue a process that remodels the spiral arteries by replacing the endothelial layer with endovascular trophoblast (EndoVT) to allow relatively unfettered maternal blood supply to the intervillous space (12) (Figure 1D). Once the plugs are dissolved, the SCT layer is bathed directly in maternal blood. This provides an additional route for viral particles present in maternal blood to infect the growing villous placenta (across the SCT layer). To infect a fetus, the virus must then traverse the underlying layer of VCT to enter the villous core that contains fetal blood vessels, fibroblasts, and fetal macrophages known as Hofbauer cells. These Hofbauer cells have the potential to provide an additional layer of defense through their response to Toll-like receptor stimulation (13, 14). Studies have indicated that Hofbauer cells are susceptible to viral infections, including TORCH pathogens, HIV (15) and ZIKV (16, 17), but respond differentially to these infections.
As the primary villi mature and pregnancy progresses, the number of migratory and spindle-like EVT decreases and the cell columns become more compact (18, 19) (Figure 1D). In addition, the VCT become sparse and infrequent underneath the continuous layer of SCT lining the villi (20, 21) (Figure 1E). While the focus of this review is on the placenta’s role in vertical transmission, the maternal decidua also contributes to infection risk and maternal response to infection during pregnancy (22). The fetal membranes, the decidua parietalis and the decidua basalis all have distinct immune cell phenotypes and may play roles in vertical transmission that differ based on the pathogen involved (23, 24). For instance, the best studied of these, the decidua basalis, contains multiple types of immune cells, including natural killer cells, dendritic cells, macrophages and T cells (24) indicating a robust potential to respond to viral insults. In contrast, there is also evidence that the decidual tissue can host active infections of TORCH pathogens, like HCMV and ZIKV, implying a role as a reservoir for infection during pregnancy (25–28). The balance among these decidual responses, and those of “para-placental” routes involving the parietal decidua and fetal membranes, likely differ by pathogen and level and timing of infectious load and, like the placenta, contribute to the ultimate risk for vertical transmission.
The SCT serves as the primary trophoblast barrier between fetal and maternal blood. The structure of the syncytial layer in and of itself serves as a protective boundary. The multinucleated and continuous layer of SCT lacks cell junctions, which are often hijacked by viral particles as entry points in other tissues (1). The dense actin structure underneath the brush boarder of the SCT surface may also play a role in preventing pathogen invasion and attachment (29, 30).
The chromosome 19 miRNA cluster (C19MC) has been demonstrated to have antiviral properties, specifically at term (31, 32). This miRNA cluster is located on a maternally imprinted chromosome and is predominantly expressed by trophoblast. However, less is known about the functional role and potential of C19MC miRNAs to provide viral protection during early gestation. One study assessed release of C19MC miRNAs into maternal circulation following successful in vitro fertilization (IVF) procedures (33). The investigators in this study were able to detect C19MCs in maternal blood as early as 2 weeks after blastocyst implantation, with a substantial increase during the first trimester of the resultant pregnancy. It is hard to deduce whether this initial low level of C19MC miRNA expression is due to the small size of the embryo (and thus the placenta) at this earliest stage of pregnancy or if there are indeed changes in C19MC miRNA expression across gestation that outpace placental growth and villous maturation. With the establishment of new first-trimester trophoblast models, discussed in the following sections, (34, 35), it is now possible for researchers to assess the role of these C19miRNAs in early gestation.
Generally speaking, trophoblast constitutively produces interferons (IFNs) which may aid in placental protection from viral insults (36–38). In mice, loss of type I IFN signaling leads to increased placental viral load upon exposure to murine herpesvirus-68 and increased vertical transmission to the fetus (39). Most viruses have developed a variety of strategies to avert immune recognition and allow for unencumbered propagation within the infected cell with subsequent transmission. For instance, many flaviviruses are capable of antagonizing type I IFN responses (40, 41). ZIKV NS5, the viral RNA-dependent RNA polymerase which is the most conserved protein amongst flaviviruses, binds to and subsequently targets signal transducer and activator of transcription 2 (STAT2) for degradation (42). STAT proteins are part of the signaling cascade that converts cytokine signals into immune cell responses, including proliferation and differentiation, to fight viral invasion (43). This same mechanism is conserved for another flavivirus, dengue virus (DENV), although here STAT2 degradation relies on successful binding of ubiquitin protein ligase E3 component N-recognin 4 (UBR) to the NS5 protein (44). NS5 has also been shown to bind cellular heat shock protein 90 leading to instability and subsequent degradation of janus kinase (JAK), another member of the signaling pathways involved in antiviral responses, (45). Type III interferon responses also rely on JAK/STAT signalling cascades and therefore may be similarly antagonized by flaviviruses (46). However, we expect that this possibility of antagonism would be reduced in trophoblast cells, considering that their expression and release of type III IFNs is constitutive and can therefore be independent of viral insults (47, 48).
Transport of maternal IgG across the SCT layer of the placenta occurs through the neonatal Fc receptor (FcRn) (49). The Fc region of IgG only binds to FcRn at acidic pHs and therefore must be taken up by endosomes within the SCT. The mechanism underlying the crossing of the stroma or fetal endothelium by IgG after SCT uptake, however, remains elusive (50). In humans, onset of transfer occurs around 13 weeks of gestation and continually rises during pregnancy (51). Maternal transfer of IgG can offer passive immunity to the developing fetus. This has been demonstrated in infants born to mothers who were vaccinated against Influenza virus (Orthomyxoviridae) and tetanus-diphtheria-pertussis (Tdap) during pregnancy (52–54). Recently, researchers discovered that in the third trimester neonates can acquire SARS-CoV-2 antibodies via placenta transfer (55). However, there is the potential for a virus to hijack FcRn to facilitate its own entry and vertical transmission across the SCT layer. Both HIV and HCMV have been shown to utilize this IgG-virion transcytosis mechanism to gain access to the placenta (56, 57).
The adverse fetal outcomes first reported among pregnant women in South America who were exposed to Asian strains of ZIKV were the first strong evidence for vertical transmission of this virus (58–60), which had caused previous outbreaks in Africa and Asia without reported fetal effects. Antibody-dependent enhancement (ADE) (61) is just one of the suggested mechanisms for this change in transmission dynamics. ADE occurs when a prior virus infection generates (cross-reacting/heterotypic) antibodies that are poorly neutralizing against a subsequent infection by a separate but similar virus (62). Instead, these antibodies can lead to increased pathogenicity for the subsequent infection. Sequence alignments indicate that various ZIKV isolates share approximately 99% amino acid sequence identity and that similar levels of homology can be found between the four DENV serotypes (DENV1-4, 98-99% amino acid sequence identity) (63). Of all Flavivirus species, DENV1-4 and ZIKV share the most similar amino acid sequences. This has led researchers to investigate whether the cross-reacting antibodies produced following a primary infection with DENV might influence disease outcome upon subsequent infection with ZIKV. One case report has suggested ADE in a converse combination of flavivirus infections. Here, prior ZIKV infection enhanced infection by DENV1, which led to severe and fatal consequences (64).
ADE of ZIKV transmission has been demonstrated in mice previously infected with DENV, suggesting that DENV-specific antibodies are able to increase the incidence of ZIKV vertical transmission and associated fetal microcephaly (65, 66). This process likely involves FcRn and transcytosis of ZIKV attached to a non-neutralizing, cross-reactive, DENV-recognizing antibody (65, 67, 68). The human populations exposed to ZIKV during the outbreaks in South America in 2015/2016 also exhibited high levels of DENV immunity (i.e., in DENV-endemic regions) (69), suggesting that ADE caused by subsequent ZIKV infection in DENV-exposed women might be involved. There are, however, conflicting observations. One study using type I interferon receptor-deficient mice indicated that anti-DENV monoclonal antibodies (mAbs) may act to neutralize ZIKV and thereby protect the fetus of a ZIKV-infected mother from vertical transmission of the virus (70). Another group reported that DENV immunity provided enhancement of ZIKV infection in vitro (as observed in a K562 human erythroleukemic cell line, an otherwise nonpermissive cell line but one that bears an Fcγ receptor) but not in vivo (i.e., AG129 mice), suggesting that the variation in results may be an artifact of the exploited in vitro model systems (71). Differences in stimulated antibody titers and variations in the pathogenicity of specific viral strains could also be responsible for these discrepant outcomes.
Understanding the potential and the limitations of existing and emerging in vitro model systems for early human placental development is of the upmost importance (72–74). Recent advancements in available human trophoblast models provide a platform to further investigate how viruses infect the placenta and to explore the possible consequences of such infection on placental function and fetal health. Animal models do provide some insight into placental susceptibility to infections; however, all have significant structural and functional differences that must be considered (75, 76). For this reason, we have chosen to only discuss human models here. For those interested in further exploration, we suggest extensive reviews of these models by others (77–79). While many studies have elucidated human trophoblast susceptibility or resistance to different viruses, the savvy reader must carefully account for discrepancies among the gestational ages represented by the selected trophoblast models, the specific trophoblast lineages assessed by these models, and the plausibility of vertical transmission via the route that is being mimicked in vitro.
Primary trophoblast cells and placental explant cultures have been used to demonstrate placental susceptibility to several viruses associated with adverse pregnancy outcomes (Figure 2A). Primary cultures are restricted by their relatively short lifespan, the limited range of gestational ages from which they can be obtained and the relative frequency of sample availability. Primary cultures from term pregnancies rapidly differentiate to SCT and lack a robust migratory set of EVTs (19, 80). This essentially limits the infectious disease researcher to the study of SCT susceptibility to pathogens, including viruses, at term. First trimester explants can capture all subtypes of trophoblast but are inaccessible to many researchers. Further, sample availability can be infrequent, making replication of studies challenging when using primary first trimester samples.
Figure 2 In vitro trophoblast models for placental infection studies. (A) Primary cells and explant cultures from the first, second and third trimesters of human pregnancy have been used to study placental susceptibility to infection. These explant cultures contain all the trophoblast lineages (VCT, blue; SCT, purple; EVT; green) albeit at different proportions depending on the gestational age of the sampled tissues. (B) Various immortalized and cancer-derived trophoblast cell lines have also been used to study placental susceptibility to infection. The cartoon is simplified to demonstrate that typically only a single cell type is represented in these models. (C) Trophoblast stem cells (TSC) provide a new model for the study of lineage-specific effects of viral infections during pregnancy since all trophoblast lineages can be induced. TSC can be generated from embryonic/induced pluripotent stem cells or isolated from blastocyst outgrowths and first trimester placental tissue. (D) Trophoblast organoids can be generated from first trimester placentas. Organoids more closely mimic the villous structures of the placenta than two dimensional models and can be induced to differentiate into EVT. Trophoblast organoids can be utilized to study vertical transmission of virus as the layers of the placental villi are present in three-dimensions (albeit “inside-out”). (E) Stem cell-derived trophoblast (BAP) have been used to study placental susceptibility to several viral infections. This model most closely represents early placentation and contains a heterogenous population of trophoblast cells. (F) Blastoids, generated from embryonic/induced pluripotent stem cells, provide a new model of early human embryo structures. Blastoids could be used to investigate placental susceptibility to infection during the peri-implantation phase of pregnancy.
Trophoblast lineage susceptibly to a multitude of viruses has been demonstrated using first-trimester placental explants. For example, it was shown that the specific site of HCMV replication resides in first-trimester VCT and that the overlaying SCT is relatively resistant to HCMV infection (81, 82). SCT express the Epidermal Growth Factor Receptor (EGFR) but lack integrin coreceptors, both of which are critical for efficient HCMV attachment and subsequent cell entry and intracellular replication (83). Using first trimester explants, integrin co-receptor expression in VCT led to HCMV entry and replication, whereas SCT and proximal cytotrophoblast columns, which do not exhibit such co-receptor expression, supported viral attachment but not cell entry or replication. Interestingly, HCMV may also interfere with syncytialization, as primary human term cytotrophoblast differentiation was suppressed following HCMV infection (84). Evidence regarding placental susceptibility to HCMV in early versus late pregnancy is conflicting, but it is generally observed that SCT are infected less frequently and do not allow for productive viral replication when compared with VCT (81, 82, 85, 86). These discrepancies may be in part due to the use of the high-passage HCMV strain’s, AD169 and Toledo, in most prior studies (87). Glycoprotein mutations, gained through viral propagation in fibroblast cells, are now characterized in the HCMV strains, AD169 (88–90) and Toledo (91), and contribute to the loss of tropism for epithelial and endothelial cells. One study found that SCT from first and third-trimester placentas can be infected with HCMV although the third-trimester samples required higher viral doses (85). Similar to HCMV, experiments using primary cells and explants from first and second trimester placental samples indicated that ZIKV infects VCT, proximal cell columns and EVT but SCT were once again spared (17, 92). There are conflicting observations concerning the susceptibility of human term SCT to ZIKV infection (28, 47, 93–95). Given the limitations of primary cell cultures, these discrepancies could be related to variation in cell culture methods and/or infection protocol variations and differences in the specific ZIKV-strains and viral titers used among studies. Human first, second, and third trimester placental explant cultures have also been used to demonstrate the role of DENV-related ADE in ZIKV vertical transmission (67, 96, 97). Exploitation of anti-DENV antibodies by ZIKV to aid transport across the placental barrier could explain how the latter could cross what is typically an otherwise non-permissive barrier for many viruses during the third trimester of human pregnancy (47). This same enhancement was not evident with sera from yellow fever virus (YFV; Flaviviridae; Flavivirus) or chikungunya virus (Togaviridae; Alphavirus) infected subjects (96). Notably, YFV is not as closely related to ZIKV as is DENV.
Primary cultures (of term SCT) have been used to investigate the packaging and release of C19MC miRNAs into exosomes. These exosomes have been demonstrated to confer resistance against numerous viral insults to recipient cells (31, 47, 98–100). These same studies also demonstrated that SCT were relatively resistant to infections caused by picornaviruses (Coxsackie B virus and Poliovirus), rhabdoviruses (Vesicular stomatitis virus), herpesviruses (HCMV, HSV-1), and flaviviruses (ZIKV, DENV) (31, 47). While primary human term SCT are relatively resistant to ZIKV, inhibition of JAK signaling increased viral replication in these cells (101). This inhibition of JAK-STAT signalling, i.e., interferon signalling, is likely necessary for productive infection as these term SCT are known to constitutively mount an interferon response (47, 102). SCT isolated and cultured from first-trimester placentas expresses the innate immune recognition receptor, toll-like receptor 3 and mounts an antiviral IFNβ response upon activation of this receptor (38). Mid-to-late gestation SCT constitutively releases type III IFNλ (47, 48), which has been reported to prevent viral transmission at skin and mucosal surfaces (103). It is notable that infection of primary human term SCT was minimal and only led to production of what the authors describe as “thin coated” virions when these term SCT were exposed to a clinical isolate of ZIKV (PRVABC59 strain from Puerto Rico, 2015), suggesting release of immature, infection-deficient viral particles (101). In contrast, upon interruption of JAK-STAT signaling, ZIKV infection of these same cells produced mature, “thick coated”, and likely more virulent virions. Interestingly, in human macrophages, ZIKV infection does not antagonize STAT2 phosphorylation and this leads to restricted viral replication (104).
Trophoblast-like cell lines have been frequently used to study placental infections. Unlike primary cultures, these cells are permissive to genetic manipulations and provide a better tool for mechanistic studies. These cell lines can be grouped into two main types: lines derived from choriocarcinomas (BeWo, JAR, JEG-3) and lines derived from first trimester placental cells that were subsequently immortalized (Swan71, ACH-3P, HTR8/SVneo) (Figure 2B). Since they are widely available, immortal, simple to propagate, and relatively stable in culture, these cell types are often chosen for initial experiments, which can be easily replicated and expanded in scale. Further, these cells can grow in simple media cocktails and on plastic culture dishes, making them a cost-effective choice. They have been used to assess treatments to prevent or limit infections. For example, treatment with palmitoleate reduced virus-induced apoptosis in ZIKV-infected HTR-8/SVneo, JEG-3 and JAR cells (105) and treatment with a non-nucleoside RNA polymerase inhibitor reduced virus replication in ZIKV-infected HTR8-SVneo cells (106). HTR8-SVneo cells were used to characterize pathogenicity of a ZIKV glycan-deficient mutant, which was shown to be less neuroinvasive in mice, yet the mutant virus displayed similar growth characteristics in this trophoblast cell line as did the wild-type (107).
These cell lines, however, have several limitations that can affect experimental design and interpretation. First, aside from BeWo cells, which are typically used to model SCT fusion, other immortalized trophoblast cell lines have a limited to non-existent capacity to differentiate into other trophoblast subtypes in culture. This characteristic restricts the utility of immortalized trophoblast cell lines in assessing viral effects on trophoblast differentiation. Further, there are crucial differences in the extent to which diverse trophoblast cell lines are able to form tight cellular junctions (108) and express and respond to Toll-like receptor activation (109, 110) when compared to primary trophoblast cells. Thus, experiments aiming at elucidating viral infection patterns across the placental barrier and immune responses to infections at this site become difficult to interpret when using immortalized or cancer-derived trophoblast-like cell lines. HTR8/SVneo cells (an extravillous-like trophoblast cell line) have the capacity to mount an antiviral response. Following infections with the flaviviruses ZIKV, DENV4, and YFV, infection patterns and immune responses were assessed in this cell line (111). All three viruses exhibited similar tropisms, yet ZIKV induced a stronger inflammatory response (involving IL-6, IL-8, CCL2, CLL3, and CCL5) but a lower IFN response when compared to DENV and YFV. HTR8/SVneo cells also responded to the presence of viral ssRNA in a TLR8-dependent manner (112). JEG-3 cells, which are typically cultured in two-dimensions, demonstrated an increased capacity to form syncytium, resist ZIKV infection, and basally expressed higher levels of IFN-stimulated genes when cultured in a three-dimensional system (48). Knocking down TLR7 and/or TLR8 by using siRNA also increased ZIKV replication in JEG-3 cells (113). Researchers often utilize one of these trophoblast cell lines alongside primary cultures to evaluate consistencies. For example, ZIKV-infected Swan71 and first-trimester primary cells (VCT) showed a similar induction of IFNβ and interferon stimulated genes (114).
Recently, new models of first-trimester trophoblast have been developed that will greatly enhance our knowledge of early pregnancy susceptibility to a variety of insults, including infection. Human trophoblast stem cells (TSC) can be cultured long-term without differentiation in two dimensions (2D) or stimulated to differentiate into SCT or EVT (34) (Figure 2C). These TSC can be established from blastocyst outgrowths or isolated from first trimester placental digests (34) and are permissive to genetic manipulations (115–117). Similarly, human trophoblast organoids have been derived from first trimester placentas and provide a three-dimensional (3D) representation of the villous structures of the placenta that contains both SCT and VCT (35, 118, 119) (Figure 2D). Upon stimulation, these organoids will differentiate to form outgrowths of EVT that are highly invasive and move through a Matrigel substratum (35, 119). One caveat, however, is that these organoids routinely grow “inside-out”, with the SCT structures forming cavities in the center of the organoid, when compared to the in vivo structure of the placental villi. This poses logistical hurdles when planning infection-based studies. To truly model vertical transmission during pregnancy, the SCT should be the first trophoblast subtype to contact virus, which would typically be introduced via maternal blood in vivo. It is therefore imperative that virus microinjection studies be optimized to allow direct exposure of “internal” SCT to virus using existing “inside-out” trophoblast organoids or that suspension and scaffold-based techniques be developed to invert the polarity of these organoids (120, 121).
Interestingly, even though both TSC and trophoblast organoids are derived from first-trimester placenta, each has a bias in terms of the specific in vivo trophoblast lineage counterpart it most closely resembles (122). Using distinct proliferation media, multiple groups have demonstrated that TSC’s are nearly all positive for ITGA2 (> 90%) (122, 123), a marker of the proliferative cells at the base of the cytotrophoblast cell columns (124), yet trophoblast organoids contain large areas of syncytium, numerous VCT (TP63+) and a small portion of ITGA2+ cells (< 23%) (35, 122). Further, there is also conflicting evidence about how well these TSC form multicellular organoid structures. When directly comparing TSC-derived organoids (TSC-organoids) and trophoblast organoids derived directly from first trimester tissue, the TSC-organoids formed significantly fewer SCT and secreted lower levels of HCG into the spent culture media (122). Other groups have demonstrated the ability of TSC-organoids to form SCT (115, 125–127) but it should be noted that none of these groups directly compared them to trophoblast organoids. It is still unclear why these results differ; however, we suspect that distinct methodologies might play a role. Sheridan et al. (122) passaged the TSC’s in organoid form for more than five passages, whereas the other groups analyzed only directly converted 3D cultures. Perhaps, once these TSC-organoids are passaged, they might lose the ability to spontaneously form SCT to the same degree as bonafide trophoblast organoids.
Due to ethical concerns and legal restrictions, not all investigators have access to early placental tissues. However, several research groups have recently described the ability to generate first trimester-like trophoblast stem cells from embryonic or induced pluripotent stem cells (123, 126, 128–130). These technologies increase world-wide access to approaches that model first trimester human placental development but do not require primary tissues. Most recently, it has been reported that trophoblast stem cells can be isolated from term placentas (131, 132; Preprint: 133), an accomplishment that was previously thought to be impossible. That said, the exact identity and function of these proliferative trophoblast cells needs to be further defined. Do these proliferative trophoblast cells represent the same proliferative cells found in first trimester human placental tissues or are they an entirely different stem cell population only found in later pregnancy? Such questions need further clarification, but the models may provide an exciting platform for disease-based studies given that some diseases of human pregnancy only manifest clinically in late pregnancy and the pathology of the placenta at the time of isolation of these term trophoblast stem cells is known. Lack of information on pregnancy outcomes remains a seemingly insurmountable limitation of stem cells isolated from many first trimester specimens.
Exposing pluripotent stem cells to bone morphogenetic protein 4 (BMP4) has also been employed as a useful model to study trophoblast differentiation (Figure 2E). The complete differentiation cocktail includes BMP4, an ACTIVIN/transforming growth factor (TGF)β inhibitor (A83-01) and a fibroblast growth factor (FGF)2 inhibitor (PD173074) and has been named BAP treatment and hence BAP-derived trophoblast (134). This model has been utilized by our group to investigate infections of the human placenta with ZIKV (102, 135) and SARS-CoV-2 (136), and by other groups with protocol modifications (137, 138). We found that these BAP-derived trophoblast were highly susceptible to ZIKV infection and that the African-lineage strains induced more severe cell lysis when compared to the contemporary Asian-lineage strains commonly associated with fetal microcephaly (102, 135). These findings led us to speculate that a maternal infection in early pregnancy with an African-lineage strain would actually result in a preclinical, early pregnancy loss which would abrogate the possibility of vertical transmission and potential fetal abnormalities. Interestingly, the BAP-derived trophoblast cultures also express reduced levels of C19MC miRNAs when compared to other trophoblast models, including trophoblast cell lines and term placenta (99, 102, 139) which could be a potential reason for their increased susceptibility to ZIKV. Another study suggests that stem-cell-derived trophoblast are equally lysed by Asian and African lineage-strains of ZIKV; however, in this study BMP4 was used in the absence of the other BAP cocktail ingredients and for only a single day to induce differentiation prior to infection (137), whereas our studies induced differentiation for four days with the complete BAP cocktail (102, 135). In fact, the ability of the African-lineage strains of ZIKV (in comparison to strains of the Asian-lineage) to induce massive cell death has been reported in other models, including stem cell-derived neural progenitor cells (140) and mouse embryos (141).
Important limitations of the BAP-derived trophoblast model are the short-lived nature of the cultures when grown in two dimensions and the heterogenous nature of the trophoblast generated during differentiation. Over the years, groups have questioned the validity of this model based upon the idea that stem cells from the epiblast might not have the ability to give rise to trophectoderm (126, 130, 139, 142). However, numerous studies and stringent comparisons with in vivo trophoblast indicate that these cells certainly represent trophoblast (143–146). A lingering question for BAP-derived trophoblast surrounds the precise trophoblast lineages and the developmental stage of placentation recapitulated by the model. It is clear that the transcriptome of SCT generated from BAP treatment is distinct from in vitro-derived SCT collected from term placentas (144). Recently, BAP-derived trophoblast were subjected to single nuclei RNA sequencing and demonstrated the presence of multiple transcriptomic cell clusters representing those seen for SCT in first-trimester placenta (24, 146). This same analysis revealed that only a few of the identified transcriptomic cell clusters exhibited cellular markers that were weakly associated with the EVT-like population isolated from primary first trimester placentas, suggesting the possibility that BAP treatment perhaps may not generate bona fide EVT (146). This finding would support the postulated identity of BAP-derived trophoblast as primitive syncytium, i.e., the invasive placenta prior to villous formation, since SCT would be highly represented at this early point in peri-implantation placentation whereas migratory EVT-like cells would be rare (102, 147, 148).
While it is impossible to validate the expression profile of the primary SCT (a.k.a., primitive trophoblast) formed in early pregnancy in vivo, advancements in extended embryo culture (149, 150) and in the culture of blastoids generated from pluripotent stem cells (151–154) could provide important insights into this stage of human placental development (Figure 2F). Blastoid structures can also be directly generated by reprogramming fibroblast cells (155) or through the use of extended-potential stem cells (156). Very little is known about blastocyst implantation and very early placentation in humans and these models offer a unique opportunity to study the impact of maternal infections during the peri-implantation phase of pregnancy. They also allow improved comparisons to previous works on peri-implantation rodent and non-human primate placentation. The establishment of reproducible and efficient blastoid protocols are highly important as work with human embryos is challenging due to the rarity of samples and their restricted access for many research groups.
The placenta’s many functions include nutrient exchange between mother and fetus, physical support for the fetus, immune protection, and maintenance of a maternal physiology that advances fetal development via production of placental hormones. The placenta must simultaneously respond to continuous changes in fetal growth and development, maternal physiology, as well as to pathogenic and environmental stressors across gestation (157–159). The available routes for viral breaching of the placental barrier and the ability of virus to infect and be transported across this barrier also change throughout gestation as does the degree of insult to the developing fetus infected by a pathogen via vertical transmission.
RuV, the causal agent of Rubella (German measles), was the first reported example of a teratogenic virus (160). Although no longer prevalent in the United States due to the development and widespread use of an effective vaccine (MMR, effective against measles, mumps, and rubella), there are still many Rubella cases worldwide in places where vaccination programs are not as common (161). The fetal effects of maternal RuV infection during pregnancy are closely linked to the timing of infection and thereby the risk for vertical transmission. The risk of congenital infection is significantly higher when the mother is infected with the virus within the first 12 weeks of pregnancy and this risk decreases dramatically by the third trimester (162). Very little is known about how RuV crosses the placenta. In one case study, viral antigen was detected in the basal plate and in the endothelial cells within the chorionic villi of the placenta at week 35 of gestation following primary maternal infection in the 13th week of pregnancy (163). This is indicative of a persistent mode of infection, which would allow for prolonged exposure of the placenta/fetus to the virus when infection occurs early in pregnancy.
Prospective cohort studies of primary HCMV infections indicate that the risk for intrauterine vertical transmission is the highest when maternal infection occurs in the third trimester of pregnancy (164, 165). This risk of transmission correlates with the proposed mechanism that HCMV traverses the SCT via FcRn-facilitated transcytosis, a potential transmission pathway that becomes increasingly robust throughout later pregnancy (56). Even so, the risk for congenital defects is highest when the mother is infected in her first trimester (166).
The risk for vertical transmission of ZIKV and associated fetal abnormalities is also strongly and positively associated with first trimester infections (167–171). Although several initial case studies reported fetal abnormalities associated with third-trimester infections (69, 172), larger cohort studies indicated that the risk for microcephaly and severe brain defects in the fetus is limited to pregnancies in which maternal infection occurred in the first trimester (171). In vitro models also suggest that ZIKV infection is enhanced in placental trophoblast from the first trimester when compared to that from term pregnancy. Our group demonstrated that the stem-cell derived trophoblast (BAP) that most closely resembles the primitive placenta of post-implantation human pregnancy are highly susceptible to ZIKV infection (102, 135). One study suggests that cross-talk between maternal decidual and fetal trophoblast cells may also help to determine susceptibility to ZIKV infection throughout gestation (28). To this point, decidual cells from first trimester pregnancies were infected by ZIKV at much higher rates than equivalent cells collected at term. Further, conditioned media from ZIKV-infected first trimester decidual cells enhanced ZIKV infection of primary term SCT, which had previously been shown to be resistant to ZIKV infection (28, 47). VCT isolated from term placentas also showed the presence of actively replicating ZIKV, suggesting that vertical transmission of the virus could occur in the third trimester if ZIKV has been able to cross the SCT barrier by then (16).
As discussed above, there are examples of stark, gestational-age-dependent differences in the risk for placental infection and vertical transmission after maternal exposure to viruses. Therefore, studies indicating that trophoblast generated/collected from term placentas are relatively resistant or susceptible to a particular virus may not directly translate to similar levels of susceptibility in the first trimester.
The placenta is critical for protecting and nourishing the developing fetus. While there are many mechanisms in place to prevent harmful substances and pathogens from entering and traversing the placenta, there are several examples of viruses that can do just that and thereby disrupt placental and fetal development. Advancements in in vitro trophoblast modeling and a burgeoning understanding of placental development and function will promote an improved capacity to study placental infections from a more critical viewpoint. A long history of experimental inconsistencies and a diverse set of in vitro placental models that may not recapitulate in vivo events have led to contradictions concerning how (and to what degree) the human placenta is infected with different viruses. Future studies using more robust and standardized model systems will hopefully generate more unified results. More consistent results, in turn, should allow for the development of new diagnostic tests and strategies to prevent and/or treat viral infections in pregnancy.
MS wrote the manuscript. JZ, AF, and DS provided critical feedback and review and editing of the manuscript. All authors contributed to the article and approved the submitted version.
This project was partially supported by grants R01HD094937 (DS), R21AI145071 (DS) from the National Institutes of Health.
The authors declare that the research was conducted in the absence of any commercial or financial relationships that could be construed as a potential conflict of interest.
All claims expressed in this article are solely those of the authors and do not necessarily represent those of their affiliated organizations, or those of the publisher, the editors and the reviewers. Any product that may be evaluated in this article, or claim that may be made by its manufacturer, is not guaranteed or endorsed by the publisher.
The authors would like to thank Stacy Cheavens for the illustrations and Dr. R. Michael Roberts for careful and critical review of the manuscript.
1. Megli CJ, Coyne CB. ‘Infections at the Maternal-Fetal Interface: An Overview of Pathogenesis and Defence’, Nature Reviews. Microbiology (2021) 20:67–82. doi: 10.1038/s41579-021-00610-y
3. Enders AC. Trophoblast Differentiation During the Transition From Trophoblastic Plate to Lacunar Stage of Implantation in the Rhesus Monkey and Human. Am J Anat (1989) 186(1):85–98. doi: 10.1002/aja.1001860107
4. Enders AC, King BF. Early Stages of Trophoblastic Invasion of the Maternal Vascular System During Implantation in the Macaque and Baboon. Am J Anat (1991) 192(4):329–46. doi: 10.1002/aja.1001920403
5. Burton GJ, Cindrova-Davies T, Turco MY. Review: Histotrophic Nutrition and the Placental-Endometrial Dialogue During Human Early Pregnancy. Placenta (2020) 102:21–6. doi: 10.1016/j.placenta.2020.02.008
6. Jagger BW, Miner JJ, Cao B, Arora N, Smith AM, Kovacs A, et al. Gestational Stage and IFN-λ Signaling Regulate ZIKV Infection In Utero. Cell Host Microbe (2017) 22(3):366–76.e3. doi: 10.1016/j.chom.2017.08.012
7. Vermillion MS, Lei J, Shabi Y, Baxter VK, Crilly NP, McLane M, et al. Intrauterine Zika Virus Infection of Pregnant Immunocompetent Mice Models Transplacental Transmission and Adverse Perinatal Outcomes. Nat Commun (2017) 8(1):14575. doi: 10.1038/ncomms14575
8. Xavier-Neto J, Carvalho M, Pascoalino BS, Cardoso AC, Costa AMS, Pereira AHM, et al. Hydrocephalus and Arthrogryposis in an Immunocompetent Mouse Model of ZIKA Teratogeny: A Developmental Study. PloS Neglect Trop Dis (2017) 11(2):e0005363. doi: 10.1371/journal.pntd.0005363
9. Daiminger A, Bäder U, Enders G. Pre- and Periconceptional Primary Cytomegalovirus Infection: Risk of Vertical Transmission and Congenital Disease. BJOG: Int J Obstet Gynaecol (2005) 112(2):166–72. doi: 10.1111/j.1471-0528.2004.00328.x
10. Hamilton WJ, Boyd JD. Development of the Human Placenta in the First Three Months of Gestation. J Anat (1960) 94(Pt 3):297–328.
11. Burton GJ, Jauniaux E. The Cytotrophoblastic Shell and Complications of Pregnancy. Placenta (2017) 60:134–9. doi: 10.1016/j.placenta.2017.06.007
12. Pollheimer J, Vondra S, Baltayeva J, Beristain AG, Knöfler M. Regulation of Placental Extravillous Trophoblasts by the Maternal Uterine Environment. Front Immunol (2018) 9:2597. doi: 10.3389/fimmu.2018.02597
13. Thomas JR, Appios A, Zhao X, Dutkiewicz R, Donde M, Lee CYC, et al. Phenotypic and Functional Characterization of First-Trimester Human Placental Macrophages, Hofbauer Cells. J Exp Med (2021) 218p(1):e20200891. doi: 10.1084/jem.20200891
14. Thomas JR, Naidu P, Appios A, McGovern N. The Ontogeny and Function of Placental Macrophages. Front Immunol (2021) 12:771054. doi: 10.3389/fimmu.2021.771054
15. Johnson EL, Chakraborty R. Placental Hofbauer Cells Limit HIV-1 Replication and Potentially Offset Mother to Child Transmission (MTCT) by Induction of Immunoregulatory Cytokines. Retrovirology (2012) 9(1):101. doi: 10.1186/1742-4690-9-101
16. Quicke KM, Bowen JR, Johnson EL, McDonald CE, Huailiang M, O'Neal JT, et al. Zika Virus Infects Human Placental Macrophages. Cell Host Microbe (2016) 20(1):83–90. doi: 10.1016/j.chom.2016.05.015
17. Tabata T, Petitt M, Puerta-Guardo H, Michlmayr D, Wang C, Fang-Hoover J, et al. Zika Virus Targets Different Primary Human Placental Cells, Suggesting Two Routes for Vertical Transmission. Cell Host Microbe (2016) 20(2):155–66. doi: 10.1016/j.chom.2016.07.002
18. Kemp B, Kertschanska S, Kadyrov M, Rath W, Kaufmann P, Huppertz B. Invasive Depth of Extravillous Trophoblast Correlates With Cellular Phenotype: A Comparison of Intra- and Extrauterine Implantation Sites. Histochem Cell Biol (2002) 117(5):401–14. doi: 10.1007/s00418-002-0396-0
19. Borbely AU, Sandri S, Fernandes IR, Prado KM, Cardoso EC, Correa-Silva S, et al. The Term Basal Plate of the Human Placenta as a Source of Functional Extravillous Trophoblast Cells. Reprod Biol Endocrinol (2014) 12(1):7. doi: 10.1186/1477-7827-12-7
20. Mayhew TM, Barker BL. Villous Trophoblast: Morphometric Perspectives on Growth, Differentiation, Turnover and Deposition of Fibrin-Type Fibrinoid During Gestation. Placenta (2001) 22(7):628–38. doi: 10.1053/plac.2001.0700
21. Jones CJP, Harris LK, Whittingham J, Aplin JD, Mayhew TM. A Re-Appraisal of the Morphophenotype and Basal Lamina Coverage of Cytotrophoblasts in Human Term Placenta. Placenta (2008) 29(2):215–9. doi: 10.1016/j.placenta.2007.11.004
22. Racicot K, Mor G. Risks Associated With Viral Infections During Pregnancy. J Clin Invest (2017) 127(5):1591–9. doi: 10.1172/JCI87490
23. Solders M, Gorchs L, Gidlöf S, Tiblad E, Lundell A-C, Kaipe H. Maternal Adaptive Immune Cells in Decidua Parietalis Display a More Activated and Coinhibitory Phenotype Compared to Decidua Basalis. Stem Cells Int (2017) 2017:8010961. doi: 10.1155/2017/8010961
24. Vento-Tormo R, Efremova M, Botting RA, Turco MY, Vento-Tormo M, Meyer KB, et al. Single-Cell Reconstruction of the Early Maternal–Fetal Interface in Humans. Nature (2018) 563(7731):347–53. doi: 10.1038/s41586-018-0698-6
25. Pereira L, Maidji E, McDonagh S, Genbacev O, Fisher S. Human Cytomegalovirus Transmission From the Uterus to the Placenta Correlates With the Presence of Pathogenic Bacteria and Maternal Immunity. J Virol (2003) 77(24):13301–14. doi: 10.1128/jvi.77.24.13301-13314.2003
26. Weisblum Y, Oiknine-Djian E, Vorontsov OM, Haimov-Kochman R, Zakay-Rones Z, Meir K, et al. Zika Virus Infects Early- and Midgestation Human Maternal Decidual Tissues, Inducing Distinct Innate Tissue Responses in the Maternal-Fetal Interface. J Virol (2017) 91(4):e01905–16. doi: 10.1128/JVI.01905-16
27. Tabata T, Petitt M, Fang-Hoover J, Pereira L. Survey of Cellular Immune Responses to Human Cytomegalovirus Infection in the Microenvironment of the Uterine–Placental Interface. Med Microbiol Immunol (2019) 208(3):475–85. doi: 10.1007/s00430-019-00613-w
28. Guzeloglu-Kayisli O, Guo X, Tang Z, Semerci N, Ozmen A, Larsen K, et al. Zika Virus–Infected Decidual Cells Elicit a Gestational Age–Dependent Innate Immune Response and Exaggerate Trophoblast Zika Permissiveness: Implication for Vertical Transmission. J Immunol (2020) 205(11):3083–94. doi: 10.4049/jimmunol.2000713
29. Spear M, Guo J, Wu Y. The Trinity of the Cortical Actin in the Initiation of HIV-1 Infection. Retrovirology (2012) 9(1):45. doi: 10.1186/1742-4690-9-45
30. Zeldovich VB, Clausen CH, Bradford E, Fletcher DA, Maltepe E, Robbins J, et al. Placental Syncytium Forms a Biophysical Barrier Against Pathogen Invasion. PloS Pathog (2013) 9(12):e1003821. doi: 10.1371/journal.ppat.1003821
31. Delorme-Axford E, Donker RB, Mouillet J-F, Chu T, Bayer A, Ouyang Y, et al. ‘Human Placental Trophoblasts Confer Viral Resistance to Recipient Cells’ Proc Natl Acad Sci USA (2013) 110(29):12048 LP–12053. doi: 10.1073/pnas.1304718110
32. Bayer A, Delorme-Axford E, Sleigher C, Frey TK, Trobaugh DW, Klimstra WB, et al. Human Trophoblasts Confer Resistance to Viruses Implicated in Perinatal Infection. Am J Obstet Gynecol (2015) 212(1):71.e1–8. doi: 10.1016/j.ajog.2014.07.060
33. Dumont TMF, Mouillet J-F, Bayer A, Gardner CL, Klimstra WB, Wolf DG, et al. The Expression Level of C19MC miRNAs in Early Pregnancy and in Response to Viral Infection. Placenta (2017) 53:23–9. doi: 10.1016/j.placenta.2017.03.011
34. Okae H, Toh H, Sato T, Hiura H, Takahashi S, Shirane K, et al. Derivation of Human Trophoblast Stem Cells. Cell Stem Cell (2018) 22(1):50–63.e6. doi: 10.1016/J.STEM.2017.11.004
35. Turco MY, Gardner L, Kay RG, Hamilton RS, Prater M, Hollinshead MS, et al. Trophoblast Organoids as a Model for Maternal–Fetal Interactions During Human Placentation. Nature (2018) 564(7735):263–7. doi: 10.1038/s41586-018-0753-3
36. Loke YW, King A. Current Topic: Interferon and Human Placental Development. Placenta (1990) 11(4):291–9. doi: 10.1016/S0143-4004(05)80220-6
37. Paulesu L, Romagnoli R, Fortino V, Cintorino M, Bischof P. Distribution of Type-I Interferon-Receptors in Human First Trimester and Term Placental Tissues and on Isolated Trophoblast Cells. Am J Reprod Immunol (1997) 37(6):443–8. doi: 10.1111/j.1600-0897.1997.tb00258.x
38. Abrahams VM, Schaefer TM, Fahey JV, Visintin I, Wright JA, Aldo PB, et al. Expression and Secretion of Antiviral Factors by Trophoblast Cells Following Stimulation by the TLR-3 Agonist, Poly(I : C). Hum Reprod (2006) 21(9):2432–9. doi: 10.1093/humrep/del178
39. Racicot K, Aldo P, El-Guindy A, Kwon J-Y, Romero R, Mor G. ‘Cutting Edge: Fetal/Placental Type I IFN Can Affect Maternal Survival and Fetal Viral Load During Viral Infection’. J Immunol (2017) 198(8):3029 LP–3032. doi: 10.4049/jimmunol.1601824
40. Diamond MS. Mechanisms of Evasion of the Type I Interferon Antiviral Response by Flaviviruses. J Interferon Cytokine Res (2009) 29(9):521–30. doi: 10.1089/jir.2009.0069
41. Cumberworth SL, Clark JJ, Kohl A, Donald CL. Inhibition of Type I Interferon Induction and Signalling by Mosquito-Borne Flaviviruses. Cell Microbiol (2017) 19(5):e12737. doi: 10.1111/cmi.12737
42. Grant A, Ponia SS, Tripathi S, Balasubramaniam V, Miorin L, Sourisseau M, et al. Zika Virus Targets Human STAT2 to Inhibit Type I Interferon Signaling. Cell Host Microbe (2016) 19(6):882–90. doi: 10.1016/j.chom.2016.05.009
43. Pfitzner E, Kliem S, Baus D, Litterst MC. The Role of STATs in Inflammation and Inflammatory Diseases. Curr Pharm Design (2004) 10(23):2839–50. doi: 10.2174/1381612043383638
44. Morrison J, Laurent-Rolle M, Maestre AM, Rajsbaum R, Pisanelli G, Simon V, et al. Dengue Virus Co-Opts UBR4 to Degrade STAT2 and Antagonize Type I Interferon Signaling. PloS Pathog (2013) 9(3):e1003265. doi: 10.1371/journal.ppat.1003265
45. Roby JA, Esser-Nobis K, Dewey-Verstelle EC, Fairgrieve MR, Schwerk J, Lu AY, et al. Flavivirus Nonstructural Protein NS5 Dysregulates HSP90 to Broadly Inhibit JAK/STAT Signaling’. Cells (2020) 9(4):899. doi: 10.3390/cells9040899
46. Stanifer ML, Pervolaraki K, Boulant S. Differential Regulation of Type I and Type III Interferon Signaling. Int J Mol Sci (2019) 20(6):1445. doi: 10.3390/ijms20061445
47. Bayer A, Lennemann NJ, Ouyang Y, Bramley JC, Morosky S, Torres De Azeved Margues E, et al. Type III Interferons Produced by Human Placental Trophoblasts Confer Protection Against Zika Virus Infection. Cell Host Microbe (2016) 19(5):705—712. doi: 10.1016/j.chom.2016.03.008
48. Corry J, Arora N, Good CA, Sadovsky Y, Coyne CB. ‘Organotypic Models of Type III Interferon-Mediated Protection From Zika Virus Infections at the Maternal–Fetal Interface’. Proc Natl Acad Sci (2017) 114(35):9433 LP–9438. doi: 10.1073/pnas.1707513114
49. Simister NE, Story CM, Chen H-L, Hunt JS. An IgG-Transporting Fc Receptor Expressed in the Syncytiotrophoblast of Human Placenta. Eur J Immunol (1996) 26(7):1527–31. doi: 10.1002/eji.1830260718
50. Clements T, Rice TF, Vamvakas G, Barnett S, Barnes M, Donaldson B, et al. Update on Transplacental Transfer of IgG Subclasses: Impact of Maternal and Fetal Factors. Front Immunol (2020) 11:1920. doi: 10.3389/fimmu.2020.01920
51. Saji F, Samejima Y, Kamiura S, Koyama M. Dynamics of Immunoglobulins at the Feto-Maternal Interface. Rev Reprod (1999) 4:81–9. doi: 10.1530/ror.0.0040081
52. Englund JA, Mbawuike IN, Hammill H, Holleman MC, Baxter BD, Glezen WP. Maternal Immunization With Influenza or Tetanus Toxoid Vaccine for Passive Antibody Protection in Young Infants. J Infect Dis (1993) 168(3):647–56. doi: 10.1093/infdis/168.3.647
53. Benowitz I, Esposito DB, Gracey KD, Shapiro ED, Vázquez M. Influenza Vaccine Given to Pregnant Women Reduces Hospitalization Due to Influenza in Their Infants. Clin Infect Dis (2010) 51(12):1355–61. doi: 10.1086/657309
54. Gall SA, Myers J, Pichichero M. Maternal Immunization With Tetanus–Diphtheria–Pertussis Vaccine: Effect on Maternal and Neonatal Serum Antibody Levels. Am J Obstet Gynecol (2011) 204(4):334.e1–5. doi: 10.1016/j.ajog.2010.11.024
55. Atyeo C, Pullen KM, Bordt EA, Fischinger S, Burke J, Michell A, et al. Compromised SARS-CoV-2-Specific Placental Antibody Transfer. Cell (2021) 184(3):628–42.e10. doi: 10.1016/j.cell.2020.12.027
56. Maidji E, McDonagh S, Genbacev O, Tabata T, Pereira L. Maternal Antibodies Enhance or Prevent Cytomegalovirus Infection in the Placenta by Neonatal Fc Receptor-Mediated Transcytosis. Am J Pathol (2006) 168(4):1210–26. doi: 10.2353/ajpath.2006.050482
57. Gupta S, Gach JS, Becerra JC, Phan TB, Pudney J, Moldoveanu Z, et al. The Neonatal Fc Receptor (FcRn) Enhances Human Immunodeficiency Virus Type 1 (HIV-1) Transcytosis Across Epithelial Cells. PloS Pathog (2013) 9(11):e1003776. doi: 10.1371/journal.ppat.1003776
58. Campos G, Bandeira A, Sardi S. Zika Virus Outbreak, Bahia, Brazil. Emerg Infect Dis J (2015) 21(10):1885. doi: 10.3201/eid2110.150847
59. Mlakar J, Korva M, Tul N, Popović M, Polijšak-Prijatelj M, Mraz J, et al. Zika Virus Associated With Microcephaly. New Engl J Med (2016) 374(10):951–8. doi: 10.1056/NEJMoa1600651
60. Oliveira Melo AS, Malinger G, Ximenes R, Szejnfeld PO, Alves Sampaio S, Bispo de Filippis AM. Zika Virus Intrauterine Infection Causes Fetal Brain Abnormality and Microcephaly: Tip of the Iceberg? Ultrasound Obstet Gynecol (2016) 47(1):6–7. doi: 10.1002/uog.15831
61. Langerak T, Mumtaz N, Tolk M VI, van Gorp EC, BE M, Rockx B, et al. The Possible Role of Cross-Reactive Dengue Virus Antibodies in Zika Virus Pathogenesis. PloS Pathog (2019) 15(4):e1007640. doi: 10.1371/journal.ppat.1007640
62. Khandia R, Munjal A, Dhama K, Karthik K, Tiwari R, Malik YS, et al. Modulation of Dengue/Zika Virus Pathogenicity by Antibody-Dependent Enhancement and Strategies to Protect Against Enhancement in Zika Virus Infection. Front Immunol (2018) 9:597. doi: 10.3389/fimmu.2018.00597
63. Xu X, Vaughan K, Weiskopf D, Grifoni A, Diamond MS, Sette A, et al. Identifying Candidate Targets of Immune Responses in Zika Virus Based on Homology to Epitopes in Other Flavivirus Species. PloS Currents (2016) 8:ecurrents.outbreaks.9aa2e1fb61b0f632f58a098773008c. doi: 10.1371/currents.outbreaks.9aa2e1fb61b0f632f58a098773008c4b
64. Bonheur AN, Thomas S, Soshnick SH, McGibbon E, Dupuis AP II, Hull R, et al. A Fatal Case Report of Antibody-Dependent Enhancement of Dengue Virus Type 1 Following Remote Zika Virus Infection. BMC Infect Dis (2021) 21(1):749. doi: 10.1186/s12879-021-06482-0
65. Bardina S, Bunduc P, Tripathi S, Duehr J, Frere JJ, Brown JA, et al. Enhancement of Zika Virus Pathogenesis by Preexisting Antiflavivirus Immunity. Science (2017) 356(6334):175–80. doi: 10.1126/science.aal4365
66. Miner JJ, Diamond MS. Dengue Antibodies, Then Zika: A Fatal Sequence in Mice. Immunity (2017) 46(5):771–3. doi: 10.1016/j.immuni.2017.04.023
67. Brown JA, Singh G, Acklin JA, Lee S, Duehr JE, Chokola AN, et al. Dengue Virus Immunity Increases Zika Virus-Induced Damage During Pregnancy. Immunity (2019) 50(3):751–62.e5. doi: 10.1016/j.immuni.2019.01.005
68. Rathore APS, Saron WAA, Lim T, Jahan N, St. John AL. Maternal Immunity and Antibodies to Dengue Virus Promote Infection and Zika Virus-Induced Microcephaly in Fetuses. Sci Adv (2019) 5(2):eaav3208–eaav3208. doi: 10.1126/sciadv.aav3208
69. Brasil P, Pereira JP, Moreira ME, Ribeiro Nogueira RM, Damasceno L, Wakimoto M, et al. Zika Virus Infection in Pregnant Women in Rio De Janeiro. N Engl J Med (2016) 375(24):2321–34. doi: 10.1056/NEJMoa1602412
70. Kam Y-W, Lee CY-P, Teo T-H, Howland SW, Amrun SN, Lum F-M, et al. Cross-Reactive Dengue Human Monoclonal Antibody Prevents Severe Pathologies and Death From Zika Virus Infections. JCI Insight (2017) 2(8):e92428. doi: 10.1172/jci.insight.92428
71. Stettler K, Beltramello M, Espinosa DA, Graham V, Cassotta A, Bianchi S, et al. Specificity, Cross-Reactivity, and Function of Antibodies Elicited by Zika Virus Infection. Science (2016) 353(6301):823–6. doi: 10.1126/science.aaf8505
72. Turco MY, Moffett A. Development of the Human Placenta. Development (2019) 146(22):dev163428. doi: 10.1242/dev.163428
73. Horii M, Touma O, Bui T, Parast MM. Modeling Human Trophoblast, the Placental Epithelium at the Maternal Fetal Interface. Reproduction (2020) 160(1):R1–R11. doi: 10.1530/REP-19-0428
74. Zhou J, West RC, Ehlers EL, Ezashi T, Schulz LC, Roberts RM, et al. Modeling Human Peri-Implantation Placental Development and Function†. Biol Reprod (2021) 105(1):40–51. doi: 10.1093/biolre/ioab080
75. Carter AM. Animal Models of Human Pregnancy and Placentation: Alternatives to the Mouse. Reproduction (2020) 160(6):R129–43. doi: 10.1530/REP-20-0354
76. Lee JK, Oh S-J, Park H, Shin OS. Recent Updates on Research Models and Tools to Study Virus–Host Interactions at the Placenta. Viruses (2020) 12(1):5. doi: 10.3390/v12010005
77. Caine EA, Jagger BW, Diamond MS. Animal Models of Zika Virus Infection During Pregnancy. Viruses (2018) 10(11):598. doi: 10.3390/v10110598
78. Ander SE, Diamond MS, Coyne CB. Immune Responses at the Maternal-Fetal Interface. Sci Immunol (2019) 4(31):eaat6114. doi: 10.1126/sciimmunol.aat6114
79. Roark HK, Jenks JA, Permar SR, Schleiss MR. Animal Models of Congenital Cytomegalovirus Transmission: Implications for Vaccine Development. J Infect Dis (2020) 221(Supplement_1):S60–73. doi: 10.1093/infdis/jiz484
80. Kliman HJ, Nestler JE, Sermasi E, Sanger JM, Strauss JF III. Purification, Characterization, and In Vitro Differentiation of Cytotrophoblasts From Human Term Placentae*. Endocrinology (1986) 118(4):1567–82. doi: 10.1210/endo-118-4-1567
81. Fisher S, Genbacev O, Maidji E, Pereira L. Human Cytomegalovirus Infection of Placental Cytotrophoblasts In Vitro and In Utero: Implications for Transmission and Pathogenesis. J Virol (2000) 74(15):6808–20. doi: 10.1128/jvi.74.15.6808-6820.2000
82. Gabrielli L, Losi L, Varani S, Lazzarotto T, Eusebi V, Landini MP. Complete Replication of Human Cytomegalovirus in Explants of First Trimester Human Placenta. J Med Virol (2001) 64(4):499–504. doi: 10.1002/jmv.1077
83. Maidji E, Genbacev O, Chang H-T, Pereira L. Developmental Regulation of Human Cytomegalovirus Receptors in Cytotrophoblasts Correlates With Distinct Replication Sites in the Placenta. J Virol (2007) 81(9):4701–12. doi: 10.1128/JVI.02748-06
84. Mimura N, Nagamatsu T, Morita K, Taguchi A, Toya T, Kumasawa K. Suppression of Human Trophoblast Syncytialization by Human Cytomegalovirus Infection. Placenta (2022) 117:200–8. doi: 10.1016/j.placenta.2021.12.011
85. Hemmings DG, Kilani R, Nykiforuk C, Preiksitis J, Guilbert LJ. Permissive Cytomegalovirus Infection of Primary Villous Term and First Trimester Trophoblasts. J Virol (1998) 72(6):4970–9. doi: 10.1128/JVI.72.6.4970-4979.1998
86. Schleiss MR, Aronow BJ, Handwerger S. Cytomegalovirus Infection of Human Syncytiotrophoblast Cells Strongly Interferes With Expression of Genes Involved in Placental Differentiation and Tissue Integrity. Pediatr Res (2007) 61(5):565–71. doi: 10.1203/pdr.0b013e318045be6d
87. Wilkinson GWG, Davison AJ, Tomasec P, Fielding CA, Aicheler R, Murrell I, et al. Human Cytomegalovirus: Taking the Strain. Med Microbiol Immunol (2015) 204(3):273–84. doi: 10.1007/s00430-015-0411-4
88. Hahn G, Revello MG, Patrone M, Percivalle E, Campanini G, Sarasini A, et al. Human Cytomegalovirus UL131-128 Genes Are Indispensable for Virus Growth in Endothelial Cells and Virus Transfer to Leukocytes. J Virol (2004) 78(18):10023–33. doi: 10.1128/JVI.78.18.10023-10033.2004
89. Wang D, Shenk T. ‘Human Cytomegalovirus Virion Protein Complex Required for Epithelial and Endothelial Cell Tropism’. Proc Natl Acad Sci USA (2005) 102(50):18153–58. doi: 10.1073/pnas.0509201102
90. Wang D, Shenk T. Human Cytomegalovirus UL131 Open Reading Frame Is Required for Epithelial Cell Tropism. J Virol (2005) 79(16):10330–8. doi: 10.1128/JVI.79.16.10330-10338.2005
91. Vashee S, Stockwell TB, Alperovich N, Denisova EA, Gibson DG, Cady KC, et al. Cloning, Assembly, and Modification of the Primary Human Cytomegalovirus Isolate Toledo by Yeast-Based Transformation-Associated Recombination. mSphere (2017) 2(5):e00331–17. doi: 10.1128/mSphereDirect.00331-17
92. Tabata T, Petitt M, Puerta-Guardo H, Michlmayr D, Harris E, Pereira L, et al. Zika Virus Replicates in Proliferating Cells in Explants From First-Trimester Human Placentas, Potential Sites for Dissemination of Infection. J Infect Dis (2018) 217(8):1202–13. doi: 10.1093/infdis/jix552
93. Jurado KA, Simoni MK, Tang Z, Uraki R, Hwang J, Householder S, et al. Zika Virus Productively Infects Primary Human Placenta-Specific Macrophages. JCI Insight (2016) 1(13):e88461. doi: 10.1172/jci.insight.88461
94. Aagaard KM, Lahon A, Suter MA, Arya RP, Seferovic MD, Vogt MB, et al. Primary Human Placental Trophoblasts Are Permissive for Zika Virus (ZIKV) Replication. Sci Rep (2017) 7:41389. doi: 10.1038/srep41389
95. Ribeiro MR, Moreli JB, Marques RE, Papa MP, Meuren LM, Rahal P, et al. Zika-Virus-Infected Human Full-Term Placental Explants Display Pro-Inflammatory Responses and Undergo Apoptosis. Arch Virol (2018) 163(10):2687–99. doi: 10.1007/s00705-018-3911-x
96. Hermanns K, Göhner C, Kopp A, Schmidt A, Merz WM, Markert UR, et al. Zika Virus Infection in Human Placental Tissue Explants Is Enhanced in the Presence of Dengue Virus Antibodies In-Vitro. Emerg Microbes Infect (2018) 7(1):198. doi: 10.1038/s41426-018-0199-6
97. Zimmerman MG, Quicke KM, O'Neal JT, Arora N, Machiah D, Priyamvada L, et al. Cross-Reactive Dengue Virus Antibodies Augment Zika Virus Infection of Human Placental Macrophages. Cell Host Microbe (2018) 24(5):731–742.e6. doi: 10.1016/j.chom.2018.10.008
98. Donker RB, Mouillet JF, Chu T, Hubel CA, Stolz DB, Morelli AE, et al. The Expression Profile of C19MC microRNAs in Primary Human Trophoblast Cells and Exosomes. Mol Hum Reprod (2012) 18(8):417–24. doi: 10.1093/molehr/gas013
99. Bayer A, Lennemann NJ, Ouyang Y, Sadovsky E, Sheridan MA, Roberts RM, et al. Chromosome 19 microRNAs Exert Antiviral Activity Independent From Type III Interferon Signaling. Placenta (2018) 61:33–38. doi: 10.1016/j.placenta.2017.11.004
100. Ouyang Y, Bayer A, Chu T, Tyurin VA, Kagan VE, Morelli AE, et al. Isolation of Human Trophoblastic Extracellular Vesicles and Characterization of Their Cargo and Antiviral Activity. Placenta (2016) 47:86–95. doi: 10.1016/j.placenta.2016.09.008
101. Gavegnano C, Bassit LC, Cox BD, Hsiao H-M, Johnson EL, Suthar M, et al. Jak Inhibitors Modulate Production of Replication-Competent Zika Virus in Human Hofbauer, Trophoblasts, and Neuroblastoma Cells. Pathog Immunity (2017) 2(2):199–218. doi: 10.20411/pai.v2i2.190
102. Sheridan MA, Yunusov D, Balaraman V, Alexenko AP, Yabe S, Verjovksi-Almeida S, et al. Vulnerability of Primitive Human Placental Trophoblast to Zika Virus. Proc Natl Acad Sci USA (2017) 114(9):E1587–96. doi: 10.1073/pnas.1616097114
103. Sommereyns C, Paul S, Steaheli P, Michiels T. IFN-Lambda (IFN-λ) Is Expressed in a Tissue-Dependent Fashion and Primarily Acts on Epithelial Cells In Vivo. PloS Pathog (2008) 4(3):e1000017. doi: 10.1371/journal.ppat.1000017
104. Yang D, Chu H, Lu G, Shuai H, Wang Y, Hou Y, et al. STAT2-Dependent Restriction of Zika Virus by Human Macrophages But Not Dendritic Cells. Emerg Microbes Infect (2021) 10(1):1024–37. doi: 10.1080/22221751.2021.1929503
105. Muthuraj PG, Pattnaik A, Sahoo PK, Islam MT, Pattnaik AK, Byrareddy SN. Palmitoleate Protects Against Zika Virus-Induced Placental Trophoblast Apoptosis. Biomedicines (2021) 9(6):643. doi: 10.3390/biomedicines9060643
106. Pattnaik A, Palermo N, Sahoo BR, Yuan Z, Hu D, Annamalai AS, et al. Discovery of a Non-Nucleoside RNA Polymerase Inhibitor for Blocking Zika Virus Replication Through In Silico Screening. Antiviral Res (2018) 151:78–86. doi: 10.1016/j.antiviral.2017.12.016
107. Annamalai AS, Pattnaik A, Sahoo BR, Muthukrishnan E, Natarajan SK, Steffen D, et al. Zika Virus Encoding Nonglycosylated Envelope Protein Is Attenuated and Defective in Neuroinvasion. J Virol (2017) 91(23):e01348–17. doi: 10.1128/JVI.01348-17
108. Rothbauer M, Patel N, Gondola H, Siwetz M, Huppertz B, Ertl P. A Comparative Study of Five Physiological Key Parameters Between Four Different Human Trophoblast-Derived Cell Lines. Sci Rep (2017) 7(1):5892. doi: 10.1038/s41598-017-06364-z
109. Gierman LM, Stodle GS, Tangeras LH, Austdal M, Olsen GD, Follestad T, et al. Toll-Like Receptor Profiling of Seven Trophoblast Cell Lines Warrants Caution for Translation to Primary Trophoblasts. Placenta (2015) 36(11):1246–53. doi: 10.1016/j.placenta.2015.09.004
110. Pudney J, He X, Masheeb Z, Kindelberger DW, Kuohung W, Ingalls RR. Differential Expression of Toll-Like Receptors in the Human Placenta Across Early Gestation. Placenta (2016) 46:1–10. doi: 10.1016/j.placenta.2016.07.005
111. Luo H, Winkelmann ER, Fernandez-Salas I, Li L, Mayer SV, Danis-Lozano R, et al. Zika, Dengue and Yellow Fever Viruses Induce Differential Anti-Viral Immune Responses in Human Monocytic and First Trimester Trophoblast Cells. Antiviral Res (2018) 151:55–62. doi: 10.1016/j.antiviral.2018.01.003
112. Potter JA, Garg M, Girard S, Abrahams VM. Viral Single Stranded RNA Induces a Trophoblast Pro-Inflammatory and Antiviral Response in a TLR8-Dependent and -Independent Manner1. Biol Reprod (2015) 92(1):1–17. doi: 10.1095/biolreprod.114.124032
113. Martinez Viedma MD, Pickett BE. Characterizing the Different Effects of Zika Virus Infection in Placenta and Microglia Cells. Viruses (2018) 10 (11):649. doi: 10.3390/v10110649
114. Ding J, Aldo P, Roberts CM, Stabach P, Liu H, You Y, et al. Placenta-Derived Interferon-Stimulated Gene 20 Controls ZIKA Virus Infection. EMBO Rep (2021) 22(10):e52450. doi: 10.15252/embr.202152450
115. Saha B, Ganguly A, Home P, Bhattacharya B, Ray S, Ghosh A, et al. TEAD4 Ensures Postimplantation Development by Promoting Trophoblast Self-Renewal: An Implication in Early Human Pregnancy Loss. Proc Natl Acad Sci (2020) 117(30):17864–17875. doi: 10.1073/pnas.2002449117
116. Hornbachner R, Lackner A, Papuchova H, Haider S, Knöfler M, Mechtler K, et al. MSX2 Safeguards Syncytiotrophoblast Fate of Human Trophoblast Stem Cells. Proc Natl Acad Sci (2021) 118(37):e2105130118. doi: 10.1073/pnas.2105130118
117. Perez-Garcia V, Lea G, Lopez-Jimenez P, Okkenhaug H, Burton GJ, Moffett A, et al. ‘BAP1/ASXL Complex Modulation Regulates Epithelial-Mesenchymal Transition During Trophoblast Differentiation and Invasion’. eLife (2021) 10:e63254. doi: 10.7554/eLife.63254
118. Haider S, Meinhardt G, Saleh L, Kunihs V, Gamperl M, Kaindl U, et al. Self-Renewing Trophoblast Organoids Recapitulate the Developmental Program of the Early Human Placenta. Stem Cell Rep (2018) 11(2):537–51. doi: 10.1016/J.STEMCR.2018.07.004
119. Sheridan MA, Fernando RC, Gardner L, Hollinshead MS, Burton GJ, Moffett A, et al. Establishment and Differentiation of Long-Term Trophoblast Organoid Cultures From the Human Placenta. Nat Protoc (2020) 15(10):3441–63. doi: 10.1038/s41596-020-0381-x
120. Co JY, Margalef-Català M, Li X, Mah AT, Kuo CJ, Monack DM, et al. Controlling Epithelial Polarity: A Human Enteroid Model for Host-Pathogen Interactions. Cell Rep (2019) 26(9):2509–2520.e4. doi: 10.1016/j.celrep.2019.01.108
121. Abbas Y, Brunel LG, Hollinshead MS, Fernando RC, Gardner L, Duncan I, et al. Generation of a Three-Dimensional Collagen Scaffold-Based Model of the Human Endometrium. Interface Focus (2020) 10(2):20190079. doi: 10.1098/rsfs.2019.0079
122. Sheridan MA, Zhao X, Fernando RC, Gardner L, Perez-Garcia V, Li Q, et al. Characterization of Primary Models of Human Trophoblast. Development (2021) 148(21). doi: 10.1242/dev.199749
123. Cinkornpumin JK, Kwon SY, Guo Y, Hossain I, Sirois J, Russett CS, et al. Naive Human Embryonic Stem Cells Can Give Rise to Cells With a Trophoblast-Like Transcriptome and Methylome. Stem Cell Rep (2020) 15(1):198–213. doi: 10.1016/j.stemcr.2020.06.003
124. Lee CQE, Turco MY, Gardner L, Simons BD, Hemberger M, Moffett A, et al. Integrin α2 Marks a Niche of Trophoblast Progenitor Cells in First Trimester Human Placenta. Dev (Cambridge England) (2018) 145(16):dev162305. doi: 10.1242/dev.162305
125. Castel G, Miestermann D, Bretin B, Firmin J, Blin J, Loubersac S, et al. Induction of Human Trophoblast Stem Cells From Somatic Cells and Pluripotent Stem Cells. Cell Rep (2020) 33(8):108419. doi: 10.1016/j.celrep.2020.108419
126. Io S, Kabata M, Iemura Y, Semi K, Morone N, Minagawa A, et al. Capturing Human Trophoblast Development With Naive Pluripotent Stem Cells In Vitro. Cell Stem Cell (2021) 28(6):1023–39. doi: 10.1016/j.stem.2021.03.013
127. Shannon MJ, Baltayeva J, Castellana B, Wächter J, McNeill GL, Yoon JS, et al. Cell Trajectory Modeling Identifies a Primitive Trophoblast State Defined by BCAM Enrichment. Development (2022) 149(1):dev199840. doi: 10.1242/dev.199840
128. Dong C, Beltcheva M, Gontarz P, Zhang B, Popli P, Fischer LA, et al. ‘Derivation of Trophoblast Stem Cells From Naïve Human Pluripotent Stem Cells. eLife (2020) 9:e52504. doi: 10.7554/eLife.52504
129. Liu X, Ouyang JF, Rossello FJ, Tan JP, Davidson KC, Valdes DS, et al. Reprogramming Roadmap Reveals Route to Human Induced Trophoblast Stem Cells. Nature (2020) 586(7827):101–7. doi: 10.1038/s41586-020-2734-6
130. Guo G, Stirparo GG, Strawbridge SE, Spindlow D, Yang J, Clarke J, et al. Human Naive Epiblast Cells Possess Unrestricted Lineage Potential. Cell Stem Cell (2021) 28(6):1040–56. doi: 10.1016/j.stem.2021.02.025
131. Gamage TKJB, Perry JJ, Fan V, Groom K, Chamley LW, James JL. Side-Population Trophoblasts Exhibit the Differentiation Potential of a Trophoblast Stem Cell Population, Persist to Term, and Are Reduced in Fetal Growth Restriction. Stem Cell Rev Rep (2020) 16(4):764–75. doi: 10.1007/s12015-020-09991-8
132. Bai T, Peng C-Y, Aneas I, Sakabe N, Requena DF, Billstrand C, et al. Establishment of Human Induced Trophoblast Stem-Like Cells From Term Villous Cytotrophoblasts. Stem Cell Res (2021) 56:102507. doi: 10.1016/j.scr.2021.102507
133. Yang L, Megli C, Coyne CB. Innate Immune Signaling in Trophoblast and Decidua Organoids Defines Differential Antiviral Defenses at the Maternal-Fetal Interface. bioRxiv (2021):2021.03.29.437467. doi: 10.1101/2021.03.29.437467
134. Amita M, Adachi K, Alexenko AP, Sinha S, Schust DJ, Schulz LC, et al. Complete and Unidirectional Conversion of Human Embryonic Stem Cells to Trophoblast by BMP4. Proc Natl Acad Sci (2013) 110(13):E1212 LP–E1221. doi: 10.1073/pnas.1303094110
135. Sheridan MA, Balaraman V, Schust DJ, Ezashi T, Roberts RM, Franz AWE. African and Asian Strains of Zika Virus Differ in Their Ability to Infect and Lyse Primitive Human Placental Trophoblast. PloS One (2018) 13(7). doi: 10.1371/journal.pone.0200086
136. Zhou J, Choi S, Liu H, Zhang J, Tian Y, Edlow AG, et al. Is SARS-CoV-2 Infection a Risk Factor for Early Pregnancy Loss? ACE2 and TMPRSS2 Co-Expression and Persistent Replicative Infection in Primitive Trophoblast. J Infect Dis (2021) 224(Supplement_6):S660–9. doi: 10.1093/infdis/jiab309
137. Tan L, Lacko LA, Zhou T, Tomoiaga D, Hurtado R, Zhang T, et al. Pre- and Peri-Implantation Zika Virus Infection Impairs Fetal Development by Targeting Trophectoderm Cells. Nat Commun (2019) 10(1):4155. doi: 10.1038/s41467-019-12063-2
138. Amaral MS, Goulart E, Caires-Junior L, Morales-Vicente DA, Soares-Schanoski A, Gomes RP, et al. Differential Gene Expression Elicited by ZIKV Infection in Trophoblasts From Congenital Zika Syndrome Discordant Twins. PloS Neglect Trop Dis (2020) 14(8):e0008424. doi: 10.1371/journal.pntd.0008424
139. Lee CQE, Gardner L, Turco M, Zhao N, Murray MJ, Coleman N, et al. What Is Trophoblast? A Combination of Criteria Define Human First-Trimester Trophoblast. Stem Cell Rep (2016) 6(2):257–72. doi: 10.1016/J.STEMCR.2016.01.006
140. Goodfellow FT, Willard KA, Wu X, Scoville S, Stice SL, Brindley MA. Strain-Dependent Consequences of Zika Virus Infection and Differential Impact on Neural Development. Viruses (2018) 10(10):550. doi: 10.3390/v10100550
141. Aubry F, Jacobs S, Darmuzey M, Lequime S, Delang L, Fontaine A, et al. Recent African Strains of Zika Virus Display Higher Transmissibility and Fetal Pathogenicity Than Asian Strains. Nat Commun (2021) 12(1):916. doi: 10.1038/s41467-021-21199-z
142. Bernardo AS, Faial T, Gardner L, Niakan KK, Ortmann D, Senner CE, et al. BRACHYURY and CDX2 Mediate BMP-Induced Differentiation of Human and Mouse Pluripotent Stem Cells Into Embryonic and Extraembryonic Lineages. Cell Stem Cell (2011) 9:144–55. doi: 10.1016/j.stem.2011.06.015
143. Horii M, Li Y, Wakeland AK, Pizzo DP, Nelson KK, Sabatini K, et al. Human Pluripotent Stem Cells as a Model of Trophoblast Differentiation in Both Normal Development and Disease. Proc Natl Acad Sci (2016) 113(27):E3882 LP–E3891. doi: 10.1073/pnas.1604747113
144. Yabe S, Alexenko AP, Amita M, Yang Y, Schust DJ, Sadovsky Y, et al. Comparison of Syncytiotrophoblast Generated From Human Embryonic Stem Cells and From Term Placentas. Proc Natl Acad Sci (2016) 113(19):E2598 LP–E2607. doi: 10.1073/pnas.1601630113
145. Jain A, Ezashi T, Roberts RM, Tuteja G. Deciphering Transcriptional Regulation in Human Embryonic Stem Cells Specified Towards a Trophoblast Fate. Sci Rep (2017) 7(1):17257. doi: 10.1038/s41598-017-17614-5
146. Khan T, Seetharam A, Zhou J, Bivens NJ, Schust DJ, Ezashi T, et al. Single Nucleus RNA Sequence (Snrnaseq) Analysis of the Spectrum of Trophoblast Lineages Generated From Human Pluripotent Stem Cells In Vitro. Front Cell Dev Biol (2021) 9:695248. doi: 10.3389/fcell.2021.695248
147. Roberts RM, Ezashi T, Sheridan MA, Yang Y. Specification of Trophoblast From Embryonic Stem Cells Exposed to BMP4. Biol Reprod (2018) 99:212–24. doi: 10.1093/biolre/ioy070
148. Karvas RM, McInturf S, Zhou J, Ezashi T, Schust DJ, Roberts RM, et al. Use of a Human Embryonic Stem Cell Model to Discover GABRP, WFDC2, VTCN1 and ACTC1 as Markers of Early First Trimester Human Trophoblast. Mol Hum Reprod (2020) 26(6):425–40. doi: 10.1093/molehr/gaaa029
149. Deglincerti A, Croft GF, Pietila LN, Zernicka-Goetz M, Siggia ED, Brivanlou AH. Self-Organization of the In Vitro Attached Human Embryo. Nature (2016) 533(7602):251–4. doi: 10.1038/nature17948
150. Shahbazi MN, Jedrusik A, Vuoristo S, Recher G, Hupalowska A, Bolton V. Self-Organization of the Human Embryo in the Absence of Maternal Tissues. Nat Cell Biol (2016) 18(6):700–8. doi: 10.1038/ncb3347
151. Kagawa H, Javali A, Khoei HH, Sommer TM, Sestini G, Novatchkova M, et al. Human Blastoids Model Blastocyst Development and Implantation. Nature (2021) 601:600–5. doi: 10.1038/s41586-021-04267-8
152. Sozen B, Jorgensen V, Weatherbee BAT, Chen S, Zhu M, Zernicka-Goetz M. Reconstructing Aspects of Human Embryogenesis With Pluripotent Stem Cells. Nat Commun (2021) 12(1):5550. doi: 10.1038/s41467-021-25853-4
153. Yanagida A, Spindlow D, Nichols J, Dattani A, Smith A, Guo G. Naive Stem Cell Blastocyst Model Captures Human Embryo Lineage Segregation. Cell Stem Cell (2021) 28(6):1016–22.e4. doi: 10.1016/j.stem.2021.04.031
154. Yu L, Wei Y, Duan J, Schmitz DA, Sakurai M, Wang L, et al. Blastocyst-Like Structures Generated From Human Pluripotent Stem Cells. Nature (2021) 591(7851):620–6. doi: 10.1038/s41586-021-03356-y
155. Liu X, Tan JP, Schröder J, Aberkane A, Ouyang JF, Mohenska M, et al. Modelling Human Blastocysts by Reprogramming Fibroblasts Into Iblastoids. Nature (2021) 591(7851):627–32. doi: 10.1038/s41586-021-03372-y
156. Fan Y, Min Z, Alsolami S, Ma Z, Zhang E, Chen W, et al. Generation of Human Blastocyst-Like Structures From Pluripotent Stem Cells. Cell Discovery (2021) 7(1):81. doi: 10.1038/s41421-021-00316-8
157. Burton GJ, Fowden AL. The Placenta: A Multifaceted, Transient Organ. Philos Trans R Soc B: Biol Sci (2015) 370(1663):20140066. doi: 10.1098/rstb.2014.0066
158. Armistead B, Johnson E, VanderKamp R, Kula-Eversole E, Kadam L, Drewlo S, et al. Placental Regulation of Energy Homeostasis During Human Pregnancy. Endocrinology (2020) 161(7):bqaa076. doi: 10.1210/endocr/bqaa076
159. Prater M, Hamilton RS, Yung HW, Sharkey AM, Robson P, Hamid NEA, et al. RNA-Seq Reveals Changes in Human Placental Metabolism, Transport and Endocrinology Across the First-Second Trimester Transition. Biol Open (2021) 10(6):bio058222. doi: 10.1242/bio.058222
160. Gregg NM. Congenital Cataract Following German Measles in the Mother BT - Problems of Birth Defects: From Hippocrates to Thalidomide and After. Persaud TVN, editor. Dordrecht: Springer Netherlands (1977) p. 170–80. doi: 10.1007/978-94-011-6621-8_22
161. Santis M, Cavaliere AF, Caruso SA. Rubella Infection in Pregnancy. Reprod Toxicol (2006) 21(4):390–8. doi: 10.1016/j.reprotox.2005.01.014
162. Dudgeon JA. Congenital Rubella. J Pediatr (1975) 87(6, Part 2):1078–86. doi: 10.1016/S0022-3476(75)80119-3
163. Lazar M, Perelygina L, Martines R, Greer P, Paddock CD, Peltecu G, et al. Immunolocalization and Distribution of Rubella Antigen in Fatal Congenital Rubella Syndrome. EBioMedicine (2016) 3:86–92. doi: 10.1016/j.ebiom.2015.11.050
164. Enders G, Daiminger A, Bäder U, Exler S, Enders M. Intrauterine Transmission and Clinical Outcome of 248 Pregnancies With Primary Cytomegalovirus Infection in Relation to Gestational Age. J Clin Virol (2011) 52(3):244–6. doi: 10.1016/j.jcv.2011.07.005
165. Picone O, Vauloup-Fellous C, Cordier AG, Guitton S, Senat MV, Fuchs F, et al. A Series of 238 Cytomegalovirus Primary Infections During Pregnancy: Description and Outcome. Prenatal Diagnosis (2013) 33(8):751–8. doi: 10.1002/pd.4118
166. Faure-Bardon V, Magny J-F, Parodi M, Couderc S, Garcia P, Maillotte A-M, et al. Sequelae of Congenital Cytomegalovirus Following Maternal Primary Infections Are Limited to Those Acquired in the First Trimester of Pregnancy. Clin Infect Dis (2019) 69(9):1526–32. doi: 10.1093/cid/ciy1128
167. Cauchemez S, Besnard M, Bompard P, Dub T, Guillemette-Artur P, Eyrolle-Guignot D, et al. Association Between Zika Virus and Microcephaly in French Polynesi–15: A Retrospective Study. Lancet (2016) 387(10033):2125–32. doi: 10.1016/S0140-6736(16)00651-6
168. De Carvalho NS, De Carvalho BF, Fugaca CA, Doris B, Biscaia ES. Zika Virus Infection During Pregnancy and Microcephaly Occurrence: A Review of Literature and Brazilian Data. Braz J Infect Dis (2016) 20(3):282–9. doi: 10.1016/j.bjid.2016.02.006
169. Coelho AV, Crovella S. ‘Microcephaly Prevalence in Infants Born to Zika Virus-Infected Women: A Systematic Review and Meta-Analysis’. Int J Mol Sci (2017) 18(8):1714. doi: 10.3390/ijms18081714
170. de Oliveira WK, de França GVA, Carmo EH, Duncan BB, de Souza Kuchenbecker R, Schmidt MI. Infection-Related Microcephaly After the 2015 and 2016 Zika Virus Outbreaks in Brazil: A Surveillance-Based Analysis. Lancet (2017) 390(10097):861–70. doi: 10.1016/S0140-6736(17)31368-5
171. Honein MA, Lee EH, Yazdy MM, Ahmad N, Macdonald J, Evert N, et al. Birth Defects Among Fetuses and Infants of US Women With Evidence of Possible Zika Virus Infection During Pregnancy. JAMA (2017) 317(1):59–68. doi: 10.1001/jama.2016.19006
Keywords: trophoblast, placenta, virus, maternal infections, models, stem cells, organoids
Citation: Sheridan MA, Zhou J, Franz AWE and Schust DJ (2022) Modeling the Human Placenta to Investigate Viral Infections During Pregnancy. Front.Virol. 2:831754. doi: 10.3389/fviro.2022.831754
Received: 08 December 2021; Accepted: 04 March 2022;
Published: 06 April 2022.
Edited by:
Kristina M. Adams Waldorf, University of Washington, United StatesReviewed by:
Craig John Bierle, University of Minnesota Twin Cities, United StatesCopyright © 2022 Sheridan, Zhou, Franz and Schust. This is an open-access article distributed under the terms of the Creative Commons Attribution License (CC BY). The use, distribution or reproduction in other forums is permitted, provided the original author(s) and the copyright owner(s) are credited and that the original publication in this journal is cited, in accordance with accepted academic practice. No use, distribution or reproduction is permitted which does not comply with these terms.
*Correspondence: Danny J. Schust, c2NodXN0ZEBoZWFsdGgubWlzc291cmkuZWR1
Disclaimer: All claims expressed in this article are solely those of the authors and do not necessarily represent those of their affiliated organizations, or those of the publisher, the editors and the reviewers. Any product that may be evaluated in this article or claim that may be made by its manufacturer is not guaranteed or endorsed by the publisher.
Research integrity at Frontiers
Learn more about the work of our research integrity team to safeguard the quality of each article we publish.