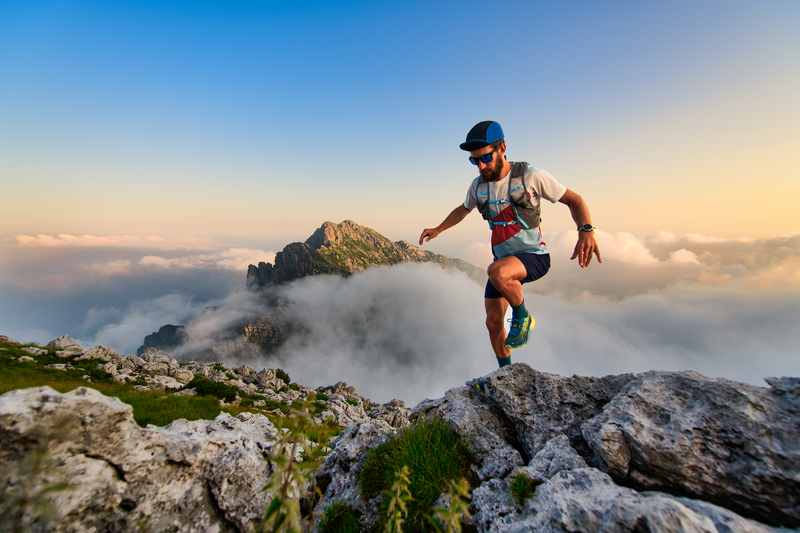
95% of researchers rate our articles as excellent or good
Learn more about the work of our research integrity team to safeguard the quality of each article we publish.
Find out more
REVIEW article
Front. Virol. , 24 March 2022
Sec. Translational Virology
Volume 2 - 2022 | https://doi.org/10.3389/fviro.2022.801778
This article is part of the Research Topic Translational Virology in Pregnancy View all 12 articles
Zika virus (ZIKV) is spread by mosquito bites or via sexual or vertical transmission. ZIKV-infected adults are generally asymptomatic, but can display mild symptoms including fever, joint pain, rash and conjunctivitis. However, during pregnancy, vertical ZIKV transmission can cause placental dysfunction and elicit severe fetal defects, including microcephaly, retinopathy, fetal growth restriction and/or stillbirth. Since no FDA-approved vaccine or anti-viral agents are currently available, ZIKV infection poses a global maternal-fetal health challenge. The maternal-fetal interface consists of maternal decidual and immune cells as well as fetal-derived trophoblasts. Compared to other cell types at the maternal-fetal interface, syncytiotrophoblasts, which form the outer layer of floating villi, are less-permissive to ZIKV, thereby preventing ZIKV transmission to the underlying cytotrophoblasts and/or other cells such as Hofbauer cells or fetal endothelium in the villi. However, anchoring villi are tightly attached to the decidua and their cytotrophoblastic cell columns are ZIKV-permissive, suggesting this location as the most likely site of ZIKV vertical transmission. Thus, at the maternal-fetal interface, maternal decidual cells likely serve as a reservoir of ZIKV persistence since they: 1) overexpress viral entry molecules compared to trophoblasts; 2) are highly permissive to ZIKV infection in a gestational age-dependent manner (more easily infected earlier in gestation); 3) augment ZIKV infection of weakly permissive primary cytotrophoblast cultures; and 4) display local maternal-immune tolerance, which prolongs ZIKV survival to facilitate fetal transmission. This review focuses on molecular mechanisms underlying ZIKV infection of cells at the human maternal-fetal interface, thus highlighting how decidual cells enhance propagation of ZIKV in extravillous cytotrophoblasts and why development of agents that eliminate ZIKV persistence in reproductive tissues before pregnancy is crucial to prevent perinatal ZIKV transmission.
Throughout pregnancy, the placenta is a specialized tissue that acts as a physical and immunological barrier against invading pathogens to protect the developing fetus from infectious agents. Unlike most viruses, which cannot cross the placental barrier, Zika virus (ZIKV), varicella zoster virus, rubella, and cytomegalovirus are transmitted from the mother to the fetus by infecting various placental cell types (1–3). Serving as major antecedents of infection-related global morbidity and mortality during pregnancy, these pathogens cause congenital anomalies and placental dysfunction resulting in adverse pregnancy outcomes such as preterm birth or fetal growth restriction and/or miscarriage (1–8). Therefore, pregnant women represent a vulnerable population for viral infections since pregnancy confers a unique immune status that facilitates maternal tolerance of the semi-allogenic fetus and enables viral infections (9, 10). Better understanding of the role and mechanism(s) responsible for viral infections during pregnancy has become increasingly relevant because of the risk of current pandemic.
ZIKV utilizes vertical route to cross the placenta and reach fetal neuronal tissues causing severe fetal defects (11, 12). Vertical transmission was confirmed by detection of ZIKV RNA in the cerebral tissues of the aborted fetuses and placental tissues as well as the increased numbers of neonatal neurodevelopmental defects during the ZIKV outbreak (13, 14). Identification of ZIKV-infected cell types as well as better understanding of the cellular and molecular mechanisms utilized by the ZIKV to cross the placenta are particularly important in preventing viral transmission to the fetus as well as ameliorating fetal prognosis and adverse pregnancy outcomes. Therefore, improved understanding ZIKV pathogenesis during pregnancy may guide the design and/or development of therapeutic agents against ZIKV.
This review focuses comprehensively on underlying molecular mechanisms of vertical ZIKV transmission at the maternal-fetal interface and highlights the trimester-dependent role of decidual stromal cells in promoting ZIKV replication as well as describes the potential use of Food and Drug Administration (FDA) approved drugs against ZIKV infection, thereby preventing perinatal ZIKV transmission.
The placenta is a unique, multifunctional organ that supplies oxygen and nutrients to the fetus to promote its development and functions as a physical barrier that protects the fetus against infections (9, 10). In humans, following implantation, collaboration between trophoblasts and extraembryonic mesodermal cells permits formation of the placenta’s floating and anchoring villi (15–17). Subsequently, the anchoring villi attach to the decidua basalis (17–19). Figure 1A represents schematized cells in the human maternal-fetal interface, which is composed of the maternally derived decidua and fetal-derived placenta. Both cytotrophoblasts (CTBs) and syncytiotrophoblasts (STBs) originate from the trophectoderm layer of the blastocyst (19, 20). CTBs are highly proliferative mononuclear cells that are attached to a basal membrane within the placental villi (Figure 1A). Villous CTBs are progenitor cells for STBs, which are multinucleated, terminally differentiated cells formed by fusion of CTBs. STBs cover the outer cell layer of placental floating villi and are in direct contact with the maternal circulation (Figure 1A) (3). Thus, STBs serve as the initial site of defense against pathogen(s) attempting to cross the placental barrier. In addition to their defensive role, STBs facilitate maternal-fetal oxygen exchange and nutrient transport and produce several growth factors and hormones that are critical for fetal development (3, 21). In the anchoring villi (Figure 1A), proliferation of CTBs forms extravillous trophoblasts (EVTs) of the cytotrophoblastic cell column, which differentiate into interstitial and endovascular CTBs that invade the maternal decidua and spiral arteries, respectively, thereby facilitating the spiral artery remodeling to markedly increase utero-placental blood flow required for fetal survival and growth (21–23).
Figure 1 Schematic presentation of the maternal-fetal interface and ZIKA virus infection. (A) The fetus-derived placental villi, which are bathed in maternal blood within intervillous space are composed of floating villi and anchoring villi that invade the maternal decidua. The maternal decidua consists of decidual cells that differentiate from uterine endometrial stromal cells, maternal blood vessels and maternal immune cells consisting of decidual natural killer (NK), dendritic cells (DC) and T cells. The placental villi are composed of fetal blood vessels, villous fibroblasts and Hofbauer cells in the villous core as well as proliferative cytotrophoblasts and multinucleated syncytiotrophoblasts, which cover the surface of the placental villous and direct contact to maternal blood. Extravillous trophoblasts are differentiated from cytotrophoblasts and invade the maternal decidua and maternal spiral arteries. Substantial crosstalk exists between maternal decidual cells and fetal trophoblasts that are essential for a successful pregnancy. (B) Representative presentation of decidual cell-mediated vertical transmission of the ZIKA virus. The enlarged inset represents the potential route of ZIKA virus (ZIKV) transmission from decidual cells to fetal cells. At the maternal-fetal interface, decidual cells exhibit higher viral entry/attachment factors AXL, GAS6 and PROS1 and they are more permissive to direct ZIKV infection in a gestational age-dependent manner compared to extravillous trophoblast or cytotrophoblast cells. Thus, ZIKV infects and spreads via decidual cells to fetal trophoblasts or Hofbauer cells or fetal endothelial cells. Therefore, decidual cells act as reservoirs for trimester-dependent placental transmission of ZIKV, thereby accounting for the higher ZIKV-infection susceptibility and more severe fetal sequelae observed in early versus late pregnancy.
In humans, the maternally derived decidua originates from the endometrium and promotes immunological tolerance of the semi-allogenic fetus as well as host defense against pathogens (24–28). The decidua is comprised of decidual stromal cells, glandular epithelial cells, maternal blood vessels and an immune cell population dominated by decidual natural killer (NK) cells and macrophages, with smaller percentages of dendritic cells and T lymphocytes (Figure 1A) (29, 30). Decidual cells are large, round, polyploid, epithelial-like cells derived from progesterone-induced decidualization of endometrial fibroblast-like stromal cells and are required for the establishment and maintenance of a normal pregnancy. Impaired decidualization is strongly associated with recurrent pregnancy loss or placental accreta or maternal hemorrhage confirming the importance of decidual cells in maintaining pregnancy (31–33). In the decidua, maternal immune cells play a crucial role in defending against infection with their numbers and subtypes changing dynamically during pregnancy. As the dominant lymphocyte population in the decidua, decidual NK cells participate in trophoblast invasion and spiral artery remodeling attaining maximum numbers during the first trimester then declining near term (34). Moreover, decidual NK cells play critical roles in promoting anti-viral innate immunity as well as placental development by producing several soluble factors (34–38). Conversely, lower T cell numbers are present in the first versus third trimester (39). In addition to decidual NK cells, decidual CD8+ T cells play a key role in balancing the paradoxical requirement for induction of maternal–fetal tolerance and anti-viral immunity (40).
ZIKV is a member of Flaviviridae that includes Dengue, West Nile, Japanese encephalitis, and yellow fever viruses and is primarily spread by the bite of the infected female Aedes mosquito (41, 42). The ZIKV was first isolated from a rhesus monkey in the Zika forest of Uganda in 1947 (43, 44). The first ZIKV infection outside of Africa occurred in Indonesia in 1981 (45), and then spread across the South Pacific islands in 2007, reaching Brazil in 2015 (43, 46). In 2016, a major ZIKV outbreak occurred in Central and South America and became a worldwide public health problem (46–48). ZIKV infections in adults are generally asymptomatic, but they can display mild symptoms including fever, joint pain, rash, and conjunctivitis (49, 50). However, during the 2015-16 epidemic in South America, ZIKV became a global health threat because of the dramatic increase in accompanying developmental defects e.g., microcephaly, ocular changes and retinopathy in up to 20% of children of affected mothers as well as a remarkable increase in adults with Guillain-Barré syndrome (46–48). The Centers for Disease Control and Prevention reported 42,750 symptomatic ZIKV cases in the U.S. and territories including 7,407 ZIKV-infected pregnant women delivering 283 live infants with ZIKV-associated birth defects and 17 pregnancy losses; among 1450 babies born to ZIKV infected mothers, 6% had ZIKV-associated birth defects such as small head size, eye damage etc., whereas 9% developed postpartum nervous system problems e.g., seizures, swallowing, movement problems, or developmental delays (51). In one large Brazilian series, Brasil et al. (11) reported that the rate of fetal death in ZIKV-infected pregnancies was 7% and overall adverse outcomes were 46% vs. 11.5% among newborns from ZIKV-noninfected women. Additionally, the recent findings from population-based birth defects surveillance data reported a four-fold increase in the prevalence of birth defects related to ZIKV infection during pregnancy (12). These observations suggest delayed postnatal sequalae and the need for long-term monitoring of children that were exposed to ZIKV in utero and born with a normal head circumference (52). Although no additional ZIKV infections have recently been reported in the Americas, ZIKV infections and outbreaks still occur in India (53) and China (54), indicating that ZIKV continues to pose a maternal-fetal health challenge.
The ZIKV genome consists of a single-strand positive-sense (ss) RNA with approximately 10.7 kilobases in length that contains a single open reading frame flanked with ~100 nucleotides at 5’ and ~400 nucleotides at 3’ untranslated region (UTR) (41, 55). As displayed in Figure 2A, the open reading frame of the ZIKV genome encodes three structural proteins (the capsid, precursor membrane, and envelope) and seven non-structural (NS) proteins (NS1, 2A, 2B, 3, 4A, 4B and 5). The encoded single polyprotein is subsequently cleaved by both viral and host proteases to yield structural and NS proteins. The structural proteins play a role in viral attachment, entry, assembly, and pathogenicity, whereas the NS proteins are involved in viral replication, polyprotein processing and attenuating host antiviral responses (55–58). The viral promoter is located at 5’ UTR with a cap site, and the terminal end of the 3’ UTR contains a conserved dinucleotide (CU) instead of polyadenylation (59–61). The mature capsid (C) protein protects the viral genome and is produced by cleavage of the immature C-protein by the viral protease. The membrane (M) protein, which is located on the viral surface, is generated by cleavage of precursor (pr) M protein by a host furin protease (62). The envelope (E) protein is a major viral surface protein that mediates viral entry by binding to host cell surface receptors (63, 64). Among NS proteins, central enzymatic activities are primarily encoded by NS3 and NS5 proteins. NS3 protein displays serine protease, RNA triphosphatase, and helicase activities, whereas NS5 possesses methyltransferase and RNA-dependent RNA polymerase (RdRP) activities (65, 66). The methyltransferase activity is required for methylation of the 5’-end caping of viral genomic RNA. Thus, viral RNA cannot be distinguished from host RNAs and consequently is translated into viral proteins by host ribosomes. RNA-dependent RNA polymerase generates a negative sense RNA using the viral positive sense ssRNA as a template, and thus is essential for viral genome replication and transcription (41, 67).
Figure 2 Detailed structure of the ZIKA virus genome, replication, and maturation into host cells. (A) The ZIKA virus (ZIKV) genome is single stranded positive sense RNA, which is approximately 10.7 kilobase in length and contains a single open reading frame (ORF) with two flanking untranslated regions on the capped 5′-end and the 3’-end lacking a polyA-tail. ORF encodes a single polyprotein comprised of 3423 amino acids, which is post-transcriptionally cleaved by viral and host cell proteases into 3 structural proteins (the capsid (C), precursor membrane (prM), and envelope E) and 7 non-structural proteins (NS; NS1-NS2A-NS2B-NS3-NS4A-NS4B-NS5). Structural proteins mediate viral attachment, entry, and fusion into host cells, whereas non-structural proteins are involved in viral replication and the host antiviral response. (B) Schematic presentation of ZIKV entry, replication, and maturation in host cells. The initial step in ZIKV infection is viral attachment via the viral envelop (E protein and phosphatidylserine; PS) to receptors (DC-SIGN, TIM, and TAM family members) on the host cell membrane. Subsequently, this binding mediates internalization into host cells via clathrin-mediated endocytosis. Then, the viral RNA is released into the host cytoplasm following fusion of viral and host endosomal membranes. The released RNA is immediately bound onto host ribosomes and translated into a polyprotein that is cleaved by viral and host cell proteases to form mature structural and nonstructural proteins. Viral replication is mediated by viral RNA-dependent RNA polymerase and methyltransferase on the surface of the endoplasmic reticulum (ER), resulting in a double stranded (ds) RNA. Transcription and replication of the dsRNA result in the formation of new viral mRNAs and ssRNA genomes, respectively. Following viral assembly of capsid proteins at the ER, the virion is transported to the Golgi apparatus where virion prM protein is cleaved to form the mature M protein. Finally, the host cell releases the mature virus by exocytosis.
As depicted in Figure 2B, the initial step in ZIKV infection is viral attachment to host cell surface receptors followed by endocytosis, enabling the virus to release ssRNA into the host cell cytoplasm (63, 65, 66). Subsequently, the ssRNA acts as a genome template that is translated into a viral polyprotein, which functions as a viral genome replicator by recruiting ssRNA to viral replication complexes. ZIKV replication occurs on the surface of the endoplasmic reticulum, resulting in a double stranded RNA genome synthesized from the genomic ssRNA by viral RdRP (68–70). The resultant double stranded RNA genome is then either transcribed to viral mRNAs for viral translation or replicated to produce new ssRNAs. Assembly of immature viral proteins and genome occurs in the endoplasmic reticulum, then transported to the Golgi apparatus for viral prM protein cleavage, which then fuse to form competent mature virions. These mature virions are then released by exocytosis (69, 70).
Like other viruses, ZIKV can infect and replicate in various cell types by attaching to several surface proteins that facilitate viral binding and entry into host cells (71). Since ZIKV displays a wide range of cellular tropisms, identification of ZIKV entry factor(s) is/are crucial to delineate details of ZIKV tropisms and pathogenesis. Several cellular receptors that contribute to ZIKV infection have been identified. These include C-type lectins aka dendritic cell-specific intercellular adhesion molecule-3-grabbing nonintegrin (DC-SIGN) and phosphatidylserine (PS) receptors (71), which serve as entry co-factors for ZIKV, include members of the T-cell immunoglobulin (TIM) (72) and the TYRO3, AXL, MERK (TAM) family (73, 74). TIM-family members (TIM1-4) bind viral PS in the viral membrane, whereas TAM members bind PS indirectly, through the soluble intermediates growth arrest-specific 6 (GAS6) and protein S1 (PROS1) that act as a bridge for ZIKV-TAM receptor binding, which induces viral endocytosis (Figure 2B) (73, 74). Among these putative ZIKV receptors, AXL is the most studied receptor for ZIKV infection. AXL is a transmembrane receptor that contains an extracellular and intracellular tyrosine kinase domain. The AXL extracellular domain acts a ZIKV entry factor by binding to its ligands GAS6 and PROS1, whereas the tyrosine kinase domain of AXL is involved in mediating an innate immune response as well as other biological processes including cell proliferation, differentiation, and survival (75, 76). Previous studies demonstrated that AXL-overexpressing human fetal neuronal stem and glial cells, astrocytes, endothelial and microglial cells are highly susceptible to ZIKV infection (74, 77), implicating the role of AXL in ZIKV-induced neuropathology. Later studies showed that the reduced AXL levels elicited by small interfering RNA or neutralizing antibodies dramatically diminished ZIKV infection in dermal fibroblast or glial cell cultures, thereby supporting the crucial role of AXL in ZIKV infection (74, 78). In contrast to in vitro studies, in situ results using Axl-deficient mice (79, 80) indicated that Axl may not be required for ZIKV infection in mice, suggesting that Axl expression and/or Axl-mediated signaling pathway(s) in mice are different than in human primary cells.
ZIKV is primarily spread to humans by the bite of infected female Aedes aegypti and albopictus mosquitoes (43, 81, 82), thereby, accepting the skin as the initial host target. Infected mosquitoes transmit ZIKV into the epidermis or directly into the circulation (78, 83, 84). Thus, epidermal cells serve as the initial site of ZIKV infection since epidermal keratinocytes, dermal fibroblasts, and immature dendritic cells are reported to be permissive to ZIKV infection (78).
Sexual transmission from male-to-female or vice-versa is also common and is responsible for about 32–54% of ZIKV infections (85, 86). Although the highest transmission occurs from male-to-female, female-to-male and male-to-male transmission are also reported (87–91). Detection of ZIKV RNA in semen (92), vaginal and cervical secretions (93, 94) supports the contribution of sexual ZIKV transmission in the increased numbers of infected people in high-risk areas as well as travelers to areas with risk of ZIKV. In the male reproductive tract, ZIKV infects testicular somatic and germ cells such as Sertoli cells, spermatozoa etc. (92, 95). In a mouse model, ZIKV persistence was reported in testicular tissues up to four weeks post-infection resulting in testicular inflammation, atrophy, and infertility (96, 97). Moreover, in humans, ZIKV RNA was detected in semen for 6 months (92) and in semen used for assisted reproductive technology up to 112 days (95), indicating that infected men serve as a potential long-term reservoir for sexual transmission.
Similarly, prolonged ZIKV shedding was initially reported in vaginal secretions up to 14 days after ZIKV infection (94). However, a later investigation by Reyes et al. reported prolonged viral shedding in vaginal secretions up to 6 months (98). This difference in ZIKV persistence in vaginal tissues is likely associated with the menstrual cycle phase during ZIKV infection. In support of this suggestion, vaginal ZIKV inoculation during diestrus in AG129 mice causes longer ZIKV survival (~10 days post-infection) and greater lethality compared with inoculation during estrus (~3 days post-infection) (99), indicating that ZIKV shedding is affected by estrous cycle stages. Additionally, vaginal inoculation of pregnant wild-type mice resulted in fetal brain infection and FGR (100), suggesting that ZIKV infection via sexual transmission route can amplify the severity of vertical transmission. ZIKV RNA was also detected in ovaries and uterus in animal models (101, 102), as well as in human oocytes (103). In vitro studies indicate that human endometrial stromal cells (HESCs) obtained from cycling endometrium are highly permissive to ZIKV infection (104, 105), and that viral replication is increased during decidualization (105). Taken together, these studies indicate that ZIKV persistence in both male and female reproductive tissues plays an important role in the management of ZIKV infection, since both male and female reproductive tissues serve as sanctuary and reservoir for prolonged ZIKV survival (72, 89). Thus, eradication of ZIKV persistence in the reproductive tissues is expected to reduce the risk of perinatal transmission yielding invaluable public health benefits.
Vertical transmission of ZIKV from the infected mother to the fetus causes severe fetal outcomes aka congenital ZIKA syndrome, which includes microcephaly, seizures, hypertonia, and other neurological problems as well as ocular and skeletal anomalies etc. (106–108),. During pregnancy, maternal ZIKV infection elicits placental dysfunction resulting in adverse pregnancy outcomes such as FGR, miscarriage and/or stillbirths. Araujo et al. (109) reported that 83% of neonates exposed to prenatal ZIKV infection were small for gestational age in Brazil during the 2015 epidemic, whereas in the United States, the rate of small for gestational age was reported to be 11.2% in women with antenatal ZIKV infection (110). Another study from Brazil reported that the rate of overall adverse outcomes was 46%, which included 7% of fetal death in ZIKV-infected pregnancies (11). Recently, Mercado-Reyes et al. (7) reported the pregnancy outcomes among pregnant women with ZIKV symptoms in Columbia between 2016-2018. Among the 1180 pregnancies, adverse pregnancy outcomes were found to be 22.4%, which included 1.4% pregnancy losses, 9.7% PTBs, 6.9% low birth weights and 4.6% small for gestational age. All these studies indicated that following placental transmission, ZIKV reaches the fetal brain where it causes severe detrimental defects by interrupting proliferation, migration, and differentiation as well as inducing apoptosis of neuronal progenitor cells (111–115). Recent studies demonstrated that ZIKV can infect a broad range of cells in the human placenta including cytotrophoblasts, endothelial cells, fibroblasts, amniotic epithelial, and/or Hofbauer cells (71, 115–118) as well as maternal decidual cells (104) and cause severe histopathological changes (119, 120). Initially, STBs were assumed to be the site of ZIKV entry. However, STBs are reported to resist ZIKV attachment and replication (121), therefore they are less-permissive to ZIKV infection, suggesting that maternal-fetal ZIKV transmission occurs at other placental site(s). The ZIKV resistance in STBs may be related to low expression of ZIKV attachment molecules such as TAM receptors (104) and/or high expression of interferon (IFN)-induced antiviral genes RIG-1, IFIH1, ISG15, etc. (121), as well as high production of type III IFNs, specifically IFNλ1, which protects host cells against ZIKV infection (122). Moreover, recently, Miranda et al. reported (123) the reduced expression of tight junction proteins, particularly claudin-4, and increased paracellular permeability of STBs obtained from ZIKV infected women, displayed an alternative mechanism of ZIKV transmission to other placental cells. On the other hand, compared to STBs, CTBs are ZIKV-permissive, suggesting that ZIKV replication in CTBs mediates its transmission to the fetus. However, ZIKV infection of CTBs in the floating villi is highly unlikely since the outer layer of the villi formed by STBs is in direct contact with maternal blood, thereby preventing ZIKV infection of CTBs in the floating villi. Thus, the anchoring villi are the most likely site of ZIKV infection of CTBs. Guzeloglu-Kayisli et al. (104) provided strong evidence that decidual cell-derived factors facilitate placental transmission by amplifying ZIKV replication in CTBs, the primary cell type in anchoring villi, which attach directly to the decidua. Collectively, these findings indicate that ZIKV persistence in immune privileged sites such as testis, brain and placenta potentiate sexual and vertical ZIKV transmission (77, 87, 89, 124). Of note, transmission of ZIKV via blood transfusion has also been documented, especially in Brazil (125, 126).
Fetal dissemination of any infectious agent requires transmission through the placenta attached to the immunologically active uterine decidua or via hematogenous transmission (1–3). As mentioned above, STBs are less permissive to ZIKV infection. Thus, maternal-fetal ZIKV transmission must occur at other potential placental site(s) at the maternal-fetal interface, suggesting that decidual cells or EVTs or both are the most likely site(s) of ZIKV transmission to the fetus. Ex vivo and in vitro decidual cell cultures demonstrated that decidual cells act as both a reservoir and source of ZIKV transmission to adjacent anchoring villi at the maternal-fetal interface, thereby representing a primary vertical transmission site (Figure 1B) (104, 118, 127). Guzeloglu-Kayisli et al. (104) reported that human decidual cell cultures are more permissive to ZIKV infection, replication, and viral release than human primary cultured CTBs. Similarly, cultured human decidual cells express significantly higher levels of viral entry and bridging molecules AXL, GAS6 and PROS1 (Figure 1B) than primary cultures of either CTBs or STBs. In addition, compared to cultured CTBs and STBs, decidual cells differentially express a set of genes that are involved in viral replication and/or infection (104). Similarly, Tabata et al. (116) found high AXL expression in decidual cells and EVTs obtained from second trimester placentas, but low levels of AXL expression in villous CTBs obtained from second and term trimester placentas, suggesting that AXL expression varies depending on the gestational age and placental cell types. This study also reported ZIKV infection in decidual cells, CTBs, EVTs, and Hofbauer cells, supporting ZIKV transmission from decidual cells to chronic villi and fetal circulation as the primary vertical transmission route (116). Moreover, Richard et al. (128) demonstrated that fetal endothelial cells are permissive to ZIKV infection and display higher AXL and GAS6 levels, indicating that the ZIKV infects fetal endothelial cells by using these entry molecules to cross the placental barrier. Similarly, Zheng et al. (129) reported higher AXL levels in decidual stromal cells and perivascular cells and lower levels in decidual dendritic cells and macrophages at the maternal-fetal interface using Single-Cell RNA sequencing. Additionally, Guzeloglu-Kayisli et al. (104) found that ZIKV replication and release is amplified in primary CTB cultures treated with ZIKV-infected decidual cell supernatants compared to direct ZIKV infection of these cells, indicating that decidual cells not only serve as a ZIKV reservoir, but also facilitate ZIKV infection of CTBs (Figure 1B). Furthermore, Wesblum et al. found that ZIKV induces expression of distinct innate immune response genes, particularly those related to anti-viral interferon signaling in decidua vs. chronic villi explant cultures, suggesting that this antiviral response paradoxically promotes a rapid and robust replication of ZIKV in decidual cells (127).
The risk of vertical transmission exists throughout pregnancy, whereas the greatest risk of severe fetal abnormalities is strongly associated with ZIKV infection in the 1st and 2nd trimester (46–48). Consistent with this clinical information, higher ZIKV replication is detected in decidual cells obtained from first trimester placentas compared to decidual cells from term placentas (104). However, the biological mechanism(s) utilized by ZIKV to cross to the placenta and the cause of the inverse relationship between the gestational age of ZIKV infection and its severity remain unclear. As potential mechanism(s) responsible for gestational age dependent ZIKV infection, we reported that: 1) decidual cells isolated from first trimester placentas exhibit higher viral entry/attachment molecules AXL, GAS6, and PROS1 than term decidual cells, indicating a higher risk of ZIKV infection in early pregnancy and greater subsequent detrimental ZIKV effects on the fetus (104); 2) decidual cells obtained from term placentas display a strong anti-viral response to ZIKV infection that correlates with lower ZIKV replication in term vs. first trimester decidual cell cultures (104); and 3) mid-gestation decidua expresses higher IFNα and IFNλ levels compared with early decidua, indicating an inverse correlation between IFN levels and ZIKV susceptibility during gestation (127). In addition to these mechanisms, dynamic changes in the immune cell populations at the maternal-fetal interface during pregnancy could alter ZIKV susceptivity (130, 131). For example, decidual NK cell numbers reach a maximum during the first trimester but decline near term. Conversely, lower T cell number are present in the first trimester than at term (39).
Unfortunately, no approved effective treatment or vaccine currently exist against ZIKV infection. Since recent ZIKV outbreaks resulted in significant adverse health effects, several investigations focused on development of drugs and vaccines to treat or prevent in utero ZIKV transmission. As examples, a plasmid-based DNA vaccine (132, 133) or modified recombinant measles virus vaccine backbone (NCT02996890) or mRNA-based vaccine (134) or inactivated whole ZIKV vaccine (135) candidates have been developed and tested to prevent ZIKV infection. In several vaccine studies, viral prM and E proteins have been intensively targeted since both prM and E in the ZIKV surface are accepted as the primary antigenic target (136). Moreover, many monoclonal antibodies targeting ZIKV proteins have been described in detail (137, 138). However, the main concern related to therapeutic potential for clinical applications is viral escape due to the high mutation rates of ZIKV genome.
To provide a potential treatment against the detrimental effects of ZIKV infection, several different strategies have been employed aimed at blocking viral replication or inhibiting viral protein synthesis. Several FDA-approved drugs have been investigated for their potential rapid response to the ZIKV outbreak since their mechanisms and safety as well as their pharmacokinetic and pharmacodynamic profiles of these drugs are well documented. Predictably, screening these drugs for their effectiveness against ZIKV infection should rapidly advance their approval for clinical use than newly identified drugs. Some FDA-approved candidate drugs against ZIKV infection are given in Table 1. As examples, Sofosbuvir, an FDA-approved drug used for the treatment of chronic hepatitis C virus infection, was investigated as a potential inhibitor of ZIKV RNA-dependent RNA polymerase (145). Although Sofosbuvir inhibited ZIKV infection in human hepatocellular carcinoma (Huh-7) and placental choriocarcinoma cells, no inhibitory effect was observed in Vero or A549 cells (139, 140), indicating cell type dependent anti-ZIKV activity. Recently, Mesci et al. (141) reported in vitro and in vivo protective effects of Sofosbuvir against ZIKV by demonstrating inhibition of ZIKV replication and ZIKV-induced apoptosis in human neuronal progenitor cell cultures as well as reduction of ZIKV titers in the serum of Sofosbuvir administered pregnant SCID immunodeficient mice.
Nitazoxanide was investigated as another potential treatment against ZIKV by inhibiting the viral protease complexes NS2B-NS3 that play essential roles during viral polyprotein processing (146). Nitazoxanide is a potent antiparasitic drug used to treat anaerobic bacterial and protozoal infections and possesses broad spectrum activity against many viruses (147). The FDA approved Nitazoxanide for treatment of diarrhea and enteritis in adults and in children ≥12-months (148–151). Nitazoxanide ingested with food is absorbed from the gastrointestinal tract and hydrolyzed in plasma to form its active metabolite, tizoxanide with serum levels attaining up to 10 μg/mL (152). Clinical trials revealed its efficacy against rotavirus and norovirus gastroenteritis in children and adults (153, 154). Moreover, Nitazoxanide therapy against influenza is currently a phase III clinical trial (NCT02612922). Its broad range of anti-viral activity (147, 154–157) suggests that Nitazoxanide induces a cell-specific effect rather than a viral-specific effect. However, the mechanism(s) mediating antiviral activity of Nitazoxanide and/or tizoxanide is/are not completely elucidated. The anti-viral activities of Nitazoxanide and its bioactive metabolite tizoxanide against ZIKV were first tested in Vero and A549 cells. Both agents significantly inhibited ZIKV infection in these cell types (158). Subsequently, Li et al. (146) demonstrated that Nitazoxanide inhibited ZIKV infection by decreasing viral replication and viral protein expression in human placental epithelial cells, human neuronal progenitor cells and human pluripotent stem cell line. Thereafter, De Souza et al. (142) found that Nitazoxanide reduced ZIKV viral loads up to 2 logs in primary cultured chorionic cells obtained from human term placentas and in a human cervical epithelial cell line. Recently, Guzeloglu-Kayisli et al. (104) evaluated the anti-ZIKV activity of tizoxanide in primary cultures of HESCs obtained from cycling endometrium, decidual cells obtained from first trimester and term placentas, as well as in primary cultures of CTBs from term placentas and found that tizoxanide significantly reduces ZIKV replication in all these cell types. Cao et al. (158) demonstrated that pre-treatment with either Nitazoxanide or tizoxanide failed to inhibit ZIKV replication in Vero cell line, but adding either drug post-infection exerted an anti-ZIKV effect, suggesting that both drugs inhibit infection after viral attachment. Taken together, these results provide solid evidence supporting the potential use of Nitazoxanide or tizoxanide to prevent ZIKV infection and associated fetal abnormalities.
Additionally, Yamamoto et al. (159) screened a library of 1017 FDA-approved drugs targeting ZIKV E protein and identified Atovaquone as an effective drug against ZIKV infection in both mammalian Vero and mosquito-derived C6/36 cells in vitro. Atovaquone is a well-known anti-malaria and anti-parasitic drug (143) and is a coenzyme Q analogue that inhibits the mitochondrial cytochrome complex III and pyrimidine synthesis (160). The anti-viral effect of Atovaquone against ZIKV infection was tested in JEG3 trophoblast cells as well as ex vivo chorionic villous explants, suggesting that Atovaquone may protect placental transmission of ZIKV and could be a potential candidate against ZIKV during pregnancy (160). Similarly, Stefanik et al. also (144) identified potential anti-ZIKV candidates by screening FDA-approved drugs that interact with ZIKV NS3 and NS5 proteins and found that only three drugs: Efavirenz, an antiretroviral drug against HIV, Tipranavir, a HIV protease inhibitor, and Dasabuvir, a RNA polymerase inhibitor against Hepatitis C virus, inhibited ZIKV titers in Vero cells as well as in primary human brain cortical astrocytes and a neuroblastoma cell line (144).
This review discusses in detail the putative mechanism(s) responsible for ZIKV infection at the maternal-fetal interface. Specifically, it reveals the role of immunologically active decidual cells, which are highly permissive to ZIKV infection and likely act as both a reservoir and source of ZIKV transmission to adjacent anchoring villi at the maternal-fetal interface. Moreover, the trimester-dependent responses of decidual cells to ZIKV infection could elucidate the clinically important questions such as why pregnant women are highly susceptible to ZIKV infection and why the subsequent effects are more detrimental in the first trimester than in late pregnancy. Finally, this review discusses the anti-ZIKV effects of FDA-approved candidate drugs that were demonstrated to inhibit ZIKV replication and dissemination. Accordingly, these drugs represent potential therapeutic candidate(s) that block perinatal ZIKV transmission, thereby averting its harmful effects on the fetus.
In conclusion, both current and previous pandemics demonstrated that viral infections pose a major risk during pregnancy because of their detrimental effects on the fetus and adverse pregnancy outcomes. Therefore, determination of viral tropisms and host factors at the maternal-fetal interface are crucial to improve understanding the mechanism(s) and/or route(s) employed by emerging viruses. Predictably, prevention of viral infections during pregnancy will be more rapidly accomplished by screening of anti-viral effects of FDA-approved drugs that were previously verified as to their modes of action, safety, and pharmacokinetic and pharmacodynamic profiles.
The authors OG-K, UK, FS, and CL made substantial contributions to this work and approved the final version of the manuscript.
This study was supported by the Florida Department of Health with ZIKA Research Grant Initiative (7ZK23) to OG-K and CJL.
The authors declare that the research was conducted in the absence of any commercial or financial relationships that could be construed as a potential conflict of interest.
All claims expressed in this article are solely those of the authors and do not necessarily represent those of their affiliated organizations, or those of the publisher, the editors and the reviewers. Any product that may be evaluated in this article, or claim that may be made by its manufacturer, is not guaranteed or endorsed by the publisher.
1. Pereira L. Congenital Viral Infection: Traversing the Uterine-Placental Interface. Annu Rev Virol (2018) 5(1):273–99. doi: 10.1146/annurev-virology-092917-043236
2. Leon-Juarez M, Martinez-Castillo M, Gonzalez-Garcia LD, Helguera-Repetto AC, Zaga-Clavellina V, Garcia-Cordero J, et al. Cellular and Molecular Mechanisms of Viral Infection in the Human Placenta. Pathog Dis (2017) 75(7):93–108. doi: 10.1093/femspd/ftx093
3. Delorme-Axford E, Sadovsky Y, Coyne CB. The Placenta as a Barrier to Viral Infections. Annu Rev Virol (2014) 1(1):133–46. doi: 10.1146/annurev-virology-031413-085524
4. Megli CJ, Coyne CB. Infections at the Maternal-Fetal Interface: An Overview of Pathogenesis and Defence. Nat Rev Microbiol (2021) 20(2):67–82. doi: 10.1038/s41579-021-00610-y
5. Ding ZY, Xu F, Chen DZ, Meng XN, Xu TS, Lu MD, et al. A Multifactorial Analysis of the Pregnancy Outcomes in Cytomegalovirus-Infected Women. Gynecol Obstet Invest (2015) 80(2):106–12. doi: 10.1159/000370333
6. Hoen B, Schaub B, Funk AL, Ardillon V, Boullard M, Cabie A, et al. Pregnancy Outcomes After ZIKV Infection in French Territories in the Americas. N Engl J Med (2018) 378(11):985–94. doi: 10.1056/NEJMoa1709481
7. Mercado-Reyes M, Gilboa SM, Valencia D, Daza M, Tong VT, Galang RR, et al. Pregnancy, Birth, Infant, and Early Childhood Neurodevelopmental Outcomes Among a Cohort of Women With Symptoms of Zika Virus Disease During Pregnancy in Three Surveillance Sites, Project Vigilancia De Embarazadas Con Zika (VEZ), Colombia, 2016-2018. Trop Med Infect Dis (2021) 6(4):183–99. doi: 10.3390/tropicalmed6040183
8. Trlifajova J, Benda R, Benes C. Effect of Maternal Varicella-Zoster Virus Infection on the Outcome of Pregnancy and the Analysis of Transplacental Virus Transmission. Acta Virol (1986) 30(3):249–55.
9. Abu-Raya B, Michalski C, Sadarangani M, Lavoie PM. Maternal Immunological Adaptation During Normal Pregnancy. Front Immunol (2020) 11:575197. doi: 10.3389/fimmu.2020.575197
10. Mor G, Aldo P, Alvero AB. The Unique Immunological and Microbial Aspects of Pregnancy. Nat Rev Immunol (2017) 17(8):469–82. doi: 10.1038/nri.2017.64
11. Brasil P, Pereira JP Jr., Raja Gabaglia C, Damasceno L, Wakimoto M, Ribeiro Nogueira RM, et al. Zika Virus Infection in Pregnant Women in Rio De Janeiro - Preliminary Report. N Engl J Med (2016) 375(24):2321–34. doi: 10.1056/NEJMoa1602412
12. Smoots AN, Olson SM, Cragan J, Delaney A, Roth NM, Godfred-Cato S, et al. Population-Based Surveillance for Birth Defects Potentially Related to Zika Virus Infection - 22 States and Territories, January 2016-June 2017. MMWR Morb Mortal Wkly Rep (2020) 69(3):67–71. doi: 10.15585/mmwr.mm6903a3
13. Bhatnagar J, Rabeneck DB, Martines RB, Reagan-Steiner S, Ermias Y, Estetter LB, et al. Zika Virus RNA Replication and Persistence in Brain and Placental Tissue. Emerg Infect Dis (2017) 23(3):405–14. doi: 10.3201/eid2303.161499
14. Reagan-Steiner S, Simeone R, Simon E, Bhatnagar J, Oduyebo T, Free R, et al. Evaluation of Placental and Fetal Tissue Specimens for Zika Virus Infection - 50 States and District of Columbia, January-December, 2016. MMWR Morb Mortal Wkly Rep (2017) 66(24):636–43. doi: 10.15585/mmwr.mm6624a3
15. Larsen JF, Knoth M. Ultrastructure of the Anchoring Villi and Trophoblastic Shell in the Second Week of Placentation. Acta Obstet Gynecol Scand (1971) 50(2):117–28. doi: 10.3109/00016347109157296
16. Turco MY, Gardner L, Kay RG, Hamilton RS, Prater M, Hollinshead MS, et al. Trophoblast Organoids as a Model for Maternal-Fetal Interactions During Human Placentation. Nature (2018) 564(7735):263–7. doi: 10.1038/s41586-018-0753-3
17. Tuttle SE, O’Toole RV, O’Shaughnessy RW, Zuspan FP. Immunohistochemical Evaluation of Human Placental Implantation: An Initial Study. Am J Obstet Gynecol (1985) 153(3):239–44. doi: 10.1016/s0002-9378(85)80104-6
18. Genbacev O, Schubach SA, Miller RK. Villous Culture of First Trimester Human Placenta–Model to Study Extravillous Trophoblast (EVT) Differentiation. Placenta (1992) 13(5):439–61. doi: 10.1016/0143-4004(92)90051-t
19. Knofler M, Haider S, Saleh L, Pollheimer J, Gamage T, James J. Human Placenta and Trophoblast Development: Key Molecular Mechanisms and Model Systems. Cell Mol Life Sci (2019) 76(18):3479–96. doi: 10.1007/s00018-019-03104-6
20. Vicovac L, Jones CJ, Aplin JD. Trophoblast Differentiation During Formation of Anchoring Villi in a Model of the Early Human Placenta In Vitro. Placenta (1995) 16(1):41–56. doi: 10.1016/0143-4004(95)90080-2
21. Cao B, Diamond MS, Mysorekar IU. Maternal-Fetal Transmission of Zika Virus: Routes and Signals for Infection. J Interferon Cytokine Res (2017) 37(7):287–94. doi: 10.1089/jir.2017.0011
22. Enders AC, King BF. Early Stages of Trophoblastic Invasion of the Maternal Vascular System During Implantation in the Macaque and Baboon. Am J Anat (1991) 192(4):329–46. doi: 10.1002/aja.1001920403
23. Multhaupt HA, Mazar A, Cines DB, Warhol MJ, McCrae KR. Expression of Urokinase Receptors by Human Trophoblast. A Histochemical and Ultrastructural Analysis. Lab Invest (1994) 71(3):392–400.
24. Griffith OW, Chavan AR, Protopapas S, Maziarz J, Romero R, Wagner GP. Embryo Implantation Evolved From an Ancestral Inflammatory Attachment Reaction. Proc Natl Acad Sci U.S.A. (2017) 114(32):E6566–E75. doi: 10.1073/pnas.1701129114
25. Hoo R, Nakimuli A, Vento-Tormo R. Innate Immune Mechanisms to Protect Against Infection at the Human Decidual-Placental Interface. Front Immunol (2020) 11:2070. doi: 10.3389/fimmu.2020.02070
26. Hsu P, Santner-Nanan B, Dahlstrom JE, Fadia M, Chandra A, Peek M, et al. Altered Decidual DC-SIGN+ Antigen-Presenting Cells and Impaired Regulatory T-Cell Induction in Preeclampsia. Am J Pathol (2012) 181(6):2149–60. doi: 10.1016/j.ajpath.2012.08.032
27. Schumacher A, Sharkey DJ, Robertson SA, Zenclussen AC. Immune Cells at the Fetomaternal Interface: How the Microenvironment Modulates Immune Cells To Foster Fetal Development. J Immunol (2018) 201(2):325–34. doi: 10.4049/jimmunol.1800058
28. Tilburgs T, Roelen DL, van der Mast BJ, van Schip JJ, Kleijburg C, de Groot-Swings GM, et al. Differential Distribution of CD4(+)CD25(bright) and CD8(+)CD28(-) T-Cells in Decidua and Maternal Blood During Human Pregnancy. Placenta (2006) 27 Suppl A:S47–53. doi: 10.1016/j.placenta.2005.11.008
29. Lockwood CJ, Basar M, Kayisli UA, Guzeloglu-Kayisli O, Murk W, Wang J, et al. Interferon-Gamma Protects First-Trimester Decidual Cells Against Aberrant Matrix Metalloproteinases 1, 3, and 9 Expression in Preeclampsia. Am J Pathol (2014) 184(9):2549–59. doi: 10.1016/j.ajpath.2014.05.025
30. Lockwood CJ, Huang SJ, Chen CP, Huang Y, Xu J, Faramarzi S, et al. Decidual Cell Regulation of Natural Killer Cell-Recruiting Chemokines: Implications for the Pathogenesis and Prediction of Preeclampsia. Am J Pathol (2013) 183(3):841–56. doi: 10.1016/j.ajpath.2013.05.029
31. Schatz F, Guzeloglu-Kayisli O, Arlier S, Kayisli UA, Lockwood CJ. The Role of Decidual Cells in Uterine Hemostasis, Menstruation, Inflammation, Adverse Pregnancy Outcomes and Abnormal Uterine Bleeding. Hum Reprod Update (2016) 22(4):497–515. doi: 10.1093/humupd/dmw004
32. Kaya HS, Hantak AM, Stubbs LJ, Taylor RN, Bagchi IC, Bagchi MK. Roles of Progesterone Receptor A and B Isoforms During Human Endometrial Decidualization. Mol Endocrinol (2015) 29(6):882–95. doi: 10.1210/me.2014-1363
33. Lockwood CJ, Krikun G, Hausknecht V, Wang EY, Schatz F. Decidual Cell Regulation of Hemostasis During Implantation and Menstruation. Ann N Y Acad Sci (1997) 828:188–93. doi: 10.1111/j.1749-6632.1997.tb48539.x
34. Le Bouteiller P. Human Decidual NK Cells: Unique and Tightly Regulated Effector Functions in Healthy and Pathogen-Infected Pregnancies. Front Immunol (2013) 4:404. doi: 10.3389/fimmu.2013.00404
35. Giugliano S, Petroff MG, Warren BD, Jasti S, Linscheid C, Ward A, et al. Hepatitis C Virus Sensing by Human Trophoblasts Induces Innate Immune Responses and Recruitment of Maternal NK Cells: Potential Implications for Limiting Vertical Transmission. J Immunol (2015) 195(8):3737–47. doi: 10.4049/jimmunol.1500409
36. Ophir Y, Duev-Cohen A, Yamin R, Tsukerman P, Bauman Y, Gamliel M, et al. PILRalpha Binds an Unknown Receptor Expressed Primarily on CD56bright and Decidual-NK Cells and Activates NK Cell Functions. Oncotarget (2016) 7(27):40953–64. doi: 10.18632/oncotarget.8397
37. Tilburgs T, Evans JH, Crespo AC, Strominger JL. The HLA-G Cycle Provides for Both NK Tolerance and Immunity at the Maternal-Fetal Interface. Proc Natl Acad Sci U.S.A. (2015) 112(43):13312–7. doi: 10.1073/pnas.1517724112
38. van der Meer A, Lukassen HG, van Lierop MJ, Wijnands F, Mosselman S, Braat DD, et al. Membrane-Bound HLA-G Activates Proliferation and Interferon-Gamma Production by Uterine Natural Killer Cells. Mol Hum Reprod (2004) 10(3):189–95. doi: 10.1093/molehr/gah032
39. Yang F, Zheng Q, Jin L. Dynamic Function and Composition Changes of Immune Cells During Normal and Pathological Pregnancy at the Maternal-Fetal Interface. Front Immunol (2019) 10:2317. doi: 10.3389/fimmu.2019.02317
40. van der Zwan A, Bi K, Norwitz ER, Crespo AC, Claas FHJ, Strominger JL, et al. Mixed Signature of Activation and Dysfunction Allows Human Decidual CD8(+) T Cells to Provide Both Tolerance and Immunity. Proc Natl Acad Sci U.S.A. (2018) 115(2):385–90. doi: 10.1073/pnas.1713957115
41. Phoo WW, Li Y, Zhang Z, Lee MY, Loh YR, Tan YB, et al. Structure of the NS2B-NS3 Protease From Zika Virus After Self-Cleavage. Nat Commun (2016) 7:13410. doi: 10.1038/ncomms13410
42. Mwaliko C, Nyaruaba R, Zhao L, Atoni E, Karungu S, Mwau M, et al. Zika Virus Pathogenesis and Current Therapeutic Advances. Pathog Glob Health (2021) 115(1):21–39. doi: 10.1080/20477724.2020.1845005
43. Duffy MR, Chen TH, Hancock WT, Powers AM, Kool JL, Lanciotti RS, et al. Zika Virus Outbreak on Yap Island, Federated States of Micronesia. N Engl J Med (2009) 360(24):2536–43. doi: 10.1056/NEJMoa0805715
44. Simpson DI. Zika Virus Infection in Man. Trans R Soc Trop Med Hyg (1964) 58:335–8. doi: 10.1016/0035-9203(64)90201-9
45. Olson JG, Ksiazek TG. Suhandiman, Triwibowo. Zika Virus, a Cause of Fever in Central Java, Indonesia. Trans R Soc Trop Med Hyg (1981) 75(3):389–93. doi: 10.1016/0035-9203(81)90100-0
46. Faria NR, Azevedo Rdo S, Kraemer MU, Souza R, Cunha MS, Hill SC, et al. Zika Virus in the Americas: Early Epidemiological and Genetic Findings. Science (2016) 352(6283):345–9. doi: 10.1126/science.aaf5036
47. Lupton K. Zika Virus Disease: A Public Health Emergency of International Concern. Br J Nurs (2016) 25(4):198. doi: 10.12968/bjon.2016.25.4.198
48. Petersen E, Wilson ME, Touch S, McCloskey B, Mwaba P, Bates M, et al. Rapid Spread of Zika Virus in The Americas–Implications for Public Health Preparedness for Mass Gatherings at the 2016 Brazil Olympic Games. Int J Infect Dis (2016) 44:11–5. doi: 10.1016/j.ijid.2016.02.001
49. Ioos S, Mallet HP, Leparc Goffart I, Gauthier V, Cardoso T, Herida M. Current Zika Virus Epidemiology and Recent Epidemics. Med Mal Infect (2014) 44(7):302–7. doi: 10.1016/j.medmal.2014.04.008
50. Oehler E, Watrin L, Larre P, Leparc-Goffart I, Lastere S, Valour F, et al. Zika Virus Infection Complicated by Guillain-Barre Syndrome–Case Report, French Polynesia, December 2013. Euro Surveill (2014) 19(9):20720–23. doi: 10.2807/1560-7917.ES2014.19.9.20720
51. Zika in Babies in US Territories (2018). Available at: https://www.cdc.gov/vitalsigns/zika-territories/index.html.
52. Adams Waldorf KM, Olson EM, Nelson BR, Little ME, Rajagopal L. The Aftermath of Zika: Need for Long-Term Monitoring of Exposed Children. Trends Microbiol (2018) 26(9):729–32. doi: 10.1016/j.tim.2018.05.011
53. Grubaugh ND, Ishtiaq F, Setoh YX, Ko AI. Misperceived Risks of Zika-Related Microcephaly in India. Trends Microbiol (2019) 27(5):381–3. doi: 10.1016/j.tim.2019.02.004
54. Zhou CM, Liu JW, Qi R, Fang LZ, Qin XR, Han HJ, et al. Emergence of Zika Virus Infection in China. PloS Negl Trop Dis (2020) 14(5):e0008300. doi: 10.1371/journal.pntd.0008300
55. Ojha CR, Rodriguez M, Lapierre J, Muthu Karuppan MK, Branscome H, Kashanchi F, et al. Complementary Mechanisms Potentially Involved in the Pathology of Zika Virus. Front Immunol (2018) 9:2340. doi: 10.3389/fimmu.2018.02340
56. Golubeva VA, Nepomuceno TC, Gregoriis G, Mesquita RD, Li X, Dash S, et al. Network of Interactions Between ZIKA Virus Non-Structural Proteins and Human Host Proteins. Cells (2020) 9(1):153–71. doi: 10.3390/cells9010153
57. Sironi M, Forni D, Clerici M, Cagliani R. Nonstructural Proteins Are Preferential Positive Selection Targets in Zika Virus and Related Flaviviruses. PloS Negl Trop Dis (2016) 10(9):e0004978. doi: 10.1371/journal.pntd.0004978
58. Wu Y, Liu Q, Zhou J, Xie W, Chen C, Wang Z, et al. Zika Virus Evades Interferon-Mediated Antiviral Response Through the Co-Operation of Multiple Nonstructural Proteins In Vitro. Cell Discov (2017) 3:17006. doi: 10.1038/celldisc.2017.6
59. Boukadida C, Torres-Flores JM, Yocupicio-Monroy M, Piten-Isidro E, Rivero-Arrieta AY, Luna-Villalobos YA, et al. Complete Genome Sequences, Before and After Mammalian Cell Culture, of Zika Virus Isolated From the Serum of a Symptomatic Male Patient From Oaxaca, Mexico. Genome Announc (2017) 5(12):e0072–73. doi: 10.1128/genomeA.00072-17
60. Bujalowski PJ, Bujalowski W, Choi KH. Identification of the Viral RNA Promoter Stem Loop A (SLA)-Binding Site on Zika Virus Polymerase NS5. Sci Rep (2020) 10(1):13306. doi: 10.1038/s41598-020-70094-y
61. Lee E, Bujalowski PJ, Teramoto T, Gottipati K, Scott SD, Padmanabhan R, et al. Structures of Flavivirus RNA Promoters Suggest Two Binding Modes With NS5 Polymerase. Nat Commun (2021) 12(1):2530. doi: 10.1038/s41467-021-22846-1
62. Imran M, Saleemi MK, Chen Z, Wang X, Zhou D, Li Y, et al. Decanoyl-Arg-Val-Lys-Arg-Chloromethylketone: An Antiviral Compound That Acts Against Flaviviruses Through the Inhibition of Furin-Mediated prM Cleavage. Viruses (2019) 11(11):1011–26. doi: 10.3390/v11111011
63. Wang A, Thurmond S, Islas L, Hui K, Hai R. Zika Virus Genome Biology and Molecular Pathogenesis. Emerg Microbes Infect (2017) 6(3):e13. doi: 10.1038/emi.2016.141
64. Carpio LE, Villalain J. Identification of the Phospholipid Binding Regions of the Envelope E Protein of Flaviviruses by Molecular Dynamics. J Biomol Struct Dyn (2020) 38(17):5136–47. doi: 10.1080/07391102.2019.1697368
65. Li Q, Kang C. Structure and Dynamics of Zika Virus Protease and Its Insights Into Inhibitor Design. Biomedicines (2021) 9(8):1044–60. doi: 10.3390/biomedicines9081044
66. Lee I, Bos S, Li G, Wang S, Gadea G, Despres P, et al. Probing Molecular Insights Into Zika Virus(-)Host Interactions. Viruses (2018) 10(5):233–59. doi: 10.3390/v10050233
67. Sebera J, Dubankova A, Sychrovsky V, Ruzek D, Boura E, Nencka R. The Structural Model of Zika Virus RNA-Dependent RNA Polymerase in Complex With RNA for Rational Design of Novel Nucleotide Inhibitors. Sci Rep (2018) 8(1):11132. doi: 10.1038/s41598-018-29459-7
68. Manokaran G, Finol E, Wang C, Gunaratne J, Bahl J, Ong EZ, et al. Dengue Subgenomic RNA Binds TRIM25 to Inhibit Interferon Expression for Epidemiological Fitness. Science (2015) 350(6257):217–21. doi: 10.1126/science.aab3369
69. Clarke BD, Roby JA, Slonchak A, Khromykh AA. Functional non-Coding RNAs Derived From the Flavivirus 3’ Untranslated Region. Virus Res (2015) 206:53–61. doi: 10.1016/j.virusres.2015.01.026
70. Nagy PD, Pogany J. The Dependence of Viral RNA Replication on Co-Opted Host Factors. Nat Rev Microbiol (2011) 10(2):137–49. doi: 10.1038/nrmicro2692
71. Aagaard KM, Lahon A, Suter MA, Arya RP, Seferovic MD, Vogt MB, et al. Primary Human Placental Trophoblasts are Permissive for Zika Virus (ZIKV) Replication. Sci Rep (2017) 7:41389. doi: 10.1038/srep41389
72. Ades AE, Brickley EB, Alexander N, Brown D, Jaenisch T, Miranda-Filho DB, et al. Zika Virus Infection in Pregnancy: A Protocol for the Joint Analysis of the Prospective Cohort Studies of the ZIKAlliance, ZikaPLAN and ZIKAction Consortia. BMJ Open (2020) 10(12):e035307. doi: 10.1136/bmjopen-2019-035307
73. Carnec X, Meertens L, Dejarnac O, Perera-Lecoin M, Hafirassou ML, Kitaura J, et al. The Phosphatidylserine and Phosphatidylethanolamine Receptor CD300a Binds Dengue Virus and Enhances Infection. J Virol (2015) 90(1):92–102. doi: 10.1128/JVI.01849-15
74. Meertens L, Labeau A, Dejarnac O, Cipriani S, Sinigaglia L, Bonnet-Madin L, et al. Axl Mediates ZIKA Virus Entry in Human Glial Cells and Modulates Innate Immune Responses. Cell Rep (2017) 18(2):324–33. doi: 10.1016/j.celrep.2016.12.045
75. Zaman A, Bivona TG. Targeting AXL in NSCLC. Lung Cancer (Auckl) (2021) 12:67–79. doi: 10.2147/LCTT.S305484
76. Rothlin CV, Ghosh S, Zuniga EI, Oldstone MB, Lemke G. TAM Receptors are Pleiotropic Inhibitors of the Innate Immune Response. Cell (2007) 131(6):1124–36. doi: 10.1016/j.cell.2007.10.034
77. Rosa-Fernandes L, Cugola FR, Russo FB, Kawahara R, de Melo Freire CC, Leite PEC, et al. Zika Virus Impairs Neurogenesis and Synaptogenesis Pathways in Human Neural Stem Cells and Neurons. Front Cell Neurosci (2019) 13:64. doi: 10.3389/fncel.2019.00064
78. Hamel R, Dejarnac O, Wichit S, Ekchariyawat P, Neyret A, Luplertlop N, et al. Biology of Zika Virus Infection in Human Skin Cells. J Virol (2015) 89(17):8880–96. doi: 10.1128/JVI.00354-15
79. Hastings AK, Yockey LJ, Jagger BW, Hwang J, Uraki R, Gaitsch HF, et al. TAM Receptors Are Not Required for Zika Virus Infection in Mice. Cell Rep (2017) 19(3):558–68. doi: 10.1016/j.celrep.2017.03.058
80. Li F, Wang PR, Qu LB, Yi CH, Zhang FC, Tang XP, et al. AXL is Not Essential for Zika Virus Infection in the Mouse Brain. Emerg Microbes Infect (2017) 6(3):e16. doi: 10.1038/emi.2017.10
81. Marchette NJ, Garcia R, Rudnick A. Isolation of Zika Virus From Aedes Aegypti Mosquitoes in Malaysia. Am J Trop Med Hyg (1969) 18(3):411–5. doi: 10.4269/ajtmh.1969.18.411
82. Dick GW. Zika Virus. II. Pathogenicity and Physical Properties. Trans R Soc Trop Med Hyg (1952) 46(5):521–34. doi: 10.1016/0035-9203(52)90043-6
83. Kim JA, Seong RK, Son SW, Shin OS. Insights Into ZIKV-Mediated Innate Immune Responses in Human Dermal Fibroblasts and Epidermal Keratinocytes. J Invest Dermatol (2019) 139(2):391–9. doi: 10.1016/j.jid.2018.07.038
84. Kwock JT, Handfield C, Suwanpradid J, Hoang P, McFadden MJ, Labagnara KF, et al. IL-27 Signaling Activates Skin Cells to Induce Innate Antiviral Proteins and Protects Against Zika Virus Infection. Sci Adv (2020) 6(14):eaay3245. doi: 10.1126/sciadv.aay3245
85. Coelho FC, Durovni B, Saraceni V, Lemos C, Codeco CT, Camargo S, et al. Higher Incidence of Zika in Adult Women Than Adult Men in Rio De Janeiro Suggests a Significant Contribution of Sexual Transmission From Men to Women. Int J Infect Dis (2016) 51:128–32. doi: 10.1016/j.ijid.2016.08.023
86. Denes A, Ibrahim MA, Oluoch L, Tekeli M, Tekeli T. Impact of Weather Seasonality and Sexual Transmission on the Spread of Zika Fever. Sci Rep (2019) 9(1):17055. doi: 10.1038/s41598-019-53062-z
87. Harrower J, Kiedrzynski T, Baker S, Upton A, Rahnama F, Sherwood J, et al. Sexual Transmission of Zika Virus and Persistence in Semen, New Zealand, 2016. Emerg Infect Dis (2016) 22(10):1855–7. doi: 10.3201/eid2210.160951
88. Davidson A, Slavinski S, Komoto K, Rakeman J, Weiss D. Suspected Female-To-Male Sexual Transmission of Zika Virus - New York City, 2016. MMWR Morb Mortal Wkly Rep (2016) 65(28):716–7. doi: 10.15585/mmwr.mm6528e2
89. D’Ortenzio E, Matheron S, Yazdanpanah Y, de Lamballerie X, Hubert B, Piorkowski G, et al. Evidence of Sexual Transmission of Zika Virus. N Engl J Med (2016) 374(22):2195–8. doi: 10.1056/NEJMc1604449
90. Oster AM, Russell K, Stryker JE, Friedman A, Kachur RE, Petersen EE, et al. Update: Interim Guidance for Prevention of Sexual Transmission of Zika Virus–United States, 2016. MMWR Morb Mortal Wkly Rep (2016) 65(12):323–5. doi: 10.15585/mmwr.mm6512e3
91. Foy BD, Kobylinski KC, Chilson Foy JL, Blitvich BJ, Travassos da Rosa A, Haddow AD, et al. Probable non-Vector-Borne Transmission of Zika Virus, Colorado, USA. Emerg Infect Dis (2011) 17(5):880–2. doi: 10.3201/eid1705.101939
92. Mead PS, Duggal NK, Hook SA, Delorey M, Fischer M, Olzenak McGuire D, et al. Zika Virus Shedding in Semen of Symptomatic Infected Men. N Engl J Med (2018) 378(15):1377–85. doi: 10.1056/NEJMoa1711038
93. Visseaux B, Mortier E, Houhou-Fidouh N, Brichler S, Collin G, Larrouy L, et al. Zika Virus in the Female Genital Tract. Lancet Infect Dis (2016) 16(11):1220. doi: 10.1016/S1473-3099(16)30387-5
94. Prisant N, Bujan L, Benichou H, Hayot PH, Pavili L, Lurel S, et al. Zika Virus in the Female Genital Tract. Lancet Infect Dis (2016) 16(9):1000–1. doi: 10.1016/S1473-3099(16)30193-1
95. Cassuto NG, Marras G, Jacomo V, Bouret D. Persistence of Zika Virus in Gradient Sperm Preparation. J Gynecol Obstet Hum Reprod (2018) 47(5):211–2. doi: 10.1016/j.jogoh.2018.02.004
96. Ma W, Li S, Ma S, Jia L, Zhang F, Zhang Y, et al. Zika Virus Causes Testis Damage and Leads to Male Infertility in Mice. Cell (2017) 168(3):542. doi: 10.1016/j.cell.2017.01.009
97. Govero J, Esakky P, Scheaffer SM, Fernandez E, Drury A, Platt DJ, et al. Zika Virus Infection Damages the Testes in Mice. Nature (2016) 540(7633):438–42. doi: 10.1038/nature20556
98. Reyes Y, Bowman NM, Becker-Dreps S, Centeno E, Collins MH, Liou GA, et al. Prolonged Shedding of Zika Virus RNA in Vaginal Secretions, Nicaragua. Emerg Infect Dis (2019) 25(4):808–10. doi: 10.3201/eid2504.180977
99. Tang WW, Young MP, Mamidi A, Regla-Nava JA, Kim K, Shresta S. A Mouse Model of Zika Virus Sexual Transmission and Vaginal Viral Replication. Cell Rep (2016) 17(12):3091–8. doi: 10.1016/j.celrep.2016.11.070
100. Yockey LJ, Varela L, Rakib T, Khoury-Hanold W, Fink SL, Stutz B, et al. Vaginal Exposure to Zika Virus During Pregnancy Leads to Fetal Brain Infection. Cell (2016) 166(5):1247–56 e4. doi: 10.1016/j.cell.2016.08.004
101. Duggal NK, McDonald EM, Ritter JM, Brault AC. Sexual Transmission of Zika Virus Enhances In Utero Transmission in a Mouse Model. Sci Rep (2018) 8(1):4510. doi: 10.1038/s41598-018-22840-6
102. Carroll T, Lo M, Lanteri M, Dutra J, Zarbock K, Silveira P, et al. Zika Virus Preferentially Replicates in the Female Reproductive Tract After Vaginal Inoculation of Rhesus Macaques. PloS Pathog (2017) 13(7):e1006537. doi: 10.1371/journal.ppat.1006537
103. Filho EA, Facio CL, Machado-Paula LA, Oliveira MA, Martinhago CD, Araujo LP, et al. Case Report of Zika Virus During Controlled Ovarian Hyperstimulation: Results From Follicular Fluid, Cumulus Cells and Oocytes. JBRA Assist Reprod (2019) 23(2):172–4. doi: 10.5935/1518-0557.20180081
104. Guzeloglu-Kayisli O, Guo X, Tang Z, Semerci N, Ozmen A, Larsen K, et al. Zika Virus-Infected Decidual Cells Elicit a Gestational Age-Dependent Innate Immune Response and Exaggerate Trophoblast Zika Permissiveness: Implication for Vertical Transmission. J Immunol (2020) 205(11):3083–94. doi: 10.4049/jimmunol.2000713
105. Pagani I, Ghezzi S, Ulisse A, Rubio A, Turrini F, Garavaglia E, et al. Human Endometrial Stromal Cells Are Highly Permissive To Productive Infection by Zika Virus. Sci Rep (2017) 7:44286. doi: 10.1038/srep44286
106. Freitas DA, Souza-Santos R, Carvalho LMA, Barros WB, Neves LM, Brasil P, et al. Congenital Zika Syndrome: A Systematic Review. PloS One (2020) 15(12):e0242367. doi: 10.1371/journal.pone.0242367
107. Nielsen-Saines K. Congenital Zika Virus Infection: Clinical Features, Evaluation, and Management of the Neonate (2019). Available at: https://www.uptodate.com/contents/congenital-zika-virus-infection-clinical-features-evaluation-and-management-of-the-neonate.
108. Liang B, Guida JP, Costa Do Nascimento ML, Mysorekar IU. Host and Viral Mechanisms of Congenital Zika Syndrome. Virulence (2019) 10(1):768–75. doi: 10.1080/21505594.2019.1656503
109. de Araujo TVB, Ximenes RAA, Miranda-Filho DB, Souza WV, Montarroyos UR, de Melo APL, et al. Association Between Microcephaly, Zika Virus Infection, and Other Risk Factors in Brazil: Final Report of a Case-Control Study. Lancet Infect Dis (2018) 18(3):328–36. doi: 10.1016/S1473-3099(17)30727-2
110. Cooper HJ, Iwamoto M, Lash M, Conners EE, Paladini M, Slavinski S, et al. Maternal Zika Virus Infection: Association With Small-For-Gestational-Age Neonates and Preterm Birth. Obstet Gynecol (2019) 134(6):1197–204. doi: 10.1097/AOG.0000000000003577
111. Calvet G, Aguiar RS, Melo AS, Sampaio SA, de Filippis I, Fabri A, et al. Detection and Sequencing of Zika Virus From Amniotic Fluid of Fetuses With Microcephaly in Brazil: A Case Study. Lancet Infect Dis (2016) 16(6):653–60. doi: 10.1016/S1473-3099(16)00095-5
112. Pena F, Pimentel R, Khosla S, Mehta SD, Brito MO. Zika Virus Epidemic in Pregnant Women, Dominican Republic, 2016-2017. Emerg Infect Dis (2019) 25(2):247–55. doi: 10.3201/eid2502.181054
113. Charlier C, Beaudoin MC, Couderc T, Lortholary O, Lecuit M. Arboviruses and Pregnancy: Maternal, Fetal, and Neonatal Effects. Lancet Child Adolesc Health (2017) 1(2):134–46. doi: 10.1016/S2352-4642(17)30021-4
114. Orofino DHG, Passos SRL, de Oliveira RVC, Farias CVB, Leite M, Pone SM, et al. Cardiac Findings in Infants With In Utero Exposure to Zika Virus- a Cross Sectional Study. PloS Negl Trop Dis (2018) 12(3):e0006362. doi: 10.1371/journal.pntd.0006362
115. Jurado KA, Simoni MK, Tang Z, Uraki R, Hwang J, Householder S, et al. Zika Virus Productively Infects Primary Human Placenta-Specific Macrophages. JCI Insight (2016) 1(13):e88461–67. doi: 10.1172/jci.insight.88461
116. Tabata T, Petitt M, Puerta-Guardo H, Michlmayr D, Wang C, Fang-Hoover J, et al. Zika Virus Targets Different Primary Human Placental Cells, Suggesting Two Routes for Vertical Transmission. Cell Host Microbe (2016) 20(2):155–66. doi: 10.1016/j.chom.2016.07.002
117. Quicke KM, Bowen JR, Johnson EL, McDonald CE, Ma H, O’Neal JT, et al. Zika Virus Infects Human Placental Macrophages. Cell Host Microbe (2016) 20(1):83–90. doi: 10.1016/j.chom.2016.05.015
118. El Costa H, Gouilly J, Mansuy JM, Chen Q, Levy C, Cartron G, et al. ZIKA Virus Reveals Broad Tissue and Cell Tropism During the First Trimester of Pregnancy. Sci Rep (2016) 6:35296. doi: 10.1038/srep35296
119. Rabelo K, de Souza LJ, Salomao NG, Machado LN, Pereira PG, Portari EA, et al. Zika Induces Human Placental Damage and Inflammation. Front Immunol (2020) 11:2146. doi: 10.3389/fimmu.2020.02146
120. Santos GR, Pinto CAL, Prudente RCS, Bevilacqua E, Witkin SS, Passos SD, et al. Histopathologic Changes in Placental Tissue Associated With Vertical Transmission of Zika Virus. Int J Gynecol Pathol (2020) 39(2):157–62. doi: 10.1097/PGP.0000000000000586
121. Sheridan MA, Yunusov D, Balaraman V, Alexenko AP, Yabe S, Verjovski-Almeida S, et al. Vulnerability of Primitive Human Placental Trophoblast to Zika Virus. Proc Natl Acad Sci USA (2017) 114(9):E1587–E96. doi: 10.1073/pnas.1616097114
122. Bayer A, Lennemann NJ, Ouyang Y, Bramley JC, Morosky S, Marques ET Jr., et al. Type III Interferons Produced by Human Placental Trophoblasts Confer Protection Against Zika Virus Infection. Cell Host Microbe (2016) 19(5):705–12. doi: 10.1016/j.chom.2016.03.008
123. Miranda J, Martin-Tapia D, Valdespino-Vazquez Y, Alarcon L, Espejel-Nunez A, Guzman-Huerta M, et al. Syncytiotrophoblast of Placentae From Women With Zika Virus Infection Has Altered Tight Junction Protein Expression and Increased Paracellular Permeability. Cells (2019) 8(10):1174–95. doi: 10.3390/cells8101174
124. Miner JJ, Sene A, Richner JM, Smith AM, Santeford A, Ban N, et al. Zika Virus Infection in Mice Causes Panuveitis With Shedding of Virus in Tears. Cell Rep (2016) 16(12):3208–18. doi: 10.1016/j.celrep.2016.08.079
125. Liu R, Wang X, Ma Y, Wu J, Mao C, Yuan L, et al. Prevalence of Zika Virus in Blood Donations: A Systematic Review and Meta-Analysis. BMC Infect Dis (2019) 19(1):590. doi: 10.1186/s12879-019-4226-6
126. Magnus MM, Esposito DLA, Costa VAD, Melo PS, Costa-Lima C, Fonseca B, et al. Risk of Zika Virus Transmission by Blood Donations in Brazil. Hematol Transfus Cell Ther (2018) 40(3):250–4. doi: 10.1016/j.htct.2018.01.011
127. Weisblum Y, Oiknine-Djian E, Vorontsov OM, Haimov-Kochman R, Zakay-Rones Z, Meir K, et al. Zika Virus Infects Early- and Midgestation Human Maternal Decidual Tissues, Inducing Distinct Innate Tissue Responses in the Maternal-Fetal Interface. J Virol (2017) 91(4):e01905–18. doi: 10.1128/JVI.01905-16
128. Richard AS, Shim BS, Kwon YC, Zhang R, Otsuka Y, Schmitt K, et al. AXL-Dependent Infection of Human Fetal Endothelial Cells Distinguishes Zika Virus From Other Pathogenic Flaviviruses. Proc Natl Acad Sci USA (2017) 114(8):2024–9. doi: 10.1073/pnas.1620558114
129. Qing-Liang Zheng TD. Li-Ping Jin. Single-Cell RNA Expression Profiling of ACE2 and AXL in the Human Maternal–Fetal Interface. Reprod Dev Med (2020) 4(1):7–10. doi: 10.4103/2096-2924.278679
130. Pique-Regi R, Romero R, Tarca AL, Sendler ED, Xu Y, Garcia-Flores V, et al. Single Cell Transcriptional Signatures of the Human Placenta in Term and Preterm Parturition. Elife (2019) 8:e52004–26. doi: 10.7554/eLife.52004
131. Rinaldi SF, Makieva S, Saunders PT, Rossi AG, Norman JE. Immune Cell and Transcriptomic Analysis of the Human Decidua in Term and Preterm Parturition. Mol Hum Reprod (2017) 23(10):708–24. doi: 10.1093/molehr/gax038
132. Kudchodkar SB, Choi H, Reuschel EL, Esquivel R, Jin-Ah Kwon J, Jeong M, et al. Rapid Response to an Emerging Infectious Disease - Lessons Learned From Development of a Synthetic DNA Vaccine Targeting Zika Virus. Microbes Infect (2018) 20(11-12):676–84. doi: 10.1016/j.micinf.2018.03.001
133. Dowd KA, Ko SY, Morabito KM, Yang ES, Pelc RS, DeMaso CR, et al. Rapid Development of a DNA Vaccine for Zika Virus. Science (2016) 354(6309):237–40. doi: 10.1126/science.aai9137
134. Richner JM, Himansu S, Dowd KA, Butler SL, Salazar V, Fox JM, et al. Modified mRNA Vaccines Protect Against Zika Virus Infection. Cell (2017) 169(1):176. doi: 10.1016/j.cell.2017.03.016
135. Larocca RA, Abbink P, Peron JP, Zanotto PM, Iampietro MJ, Badamchi-Zadeh A, et al. Vaccine Protection Against Zika Virus From Brazil. Nature (2016) 536(7617):474–8. doi: 10.1038/nature18952
136. Grubor-Bauk B, Wijesundara DK, Masavuli M, Abbink P, Peterson RL, Prow NA, et al. NS1 DNA Vaccination Protects Against Zika Infection Through T Cell-Mediated Immunity in Immunocompetent Mice. Sci Adv (2019) 5(12):eaax2388. doi: 10.1126/sciadv.aax2388
137. Dussupt V, Modjarrad K, Krebs SJ. Landscape of Monoclonal Antibodies Targeting Zika and Dengue: Therapeutic Solutions and Critical Insights for Vaccine Development. Front Immunol (2020) 11:621043. doi: 10.3389/fimmu.2020.621043
138. Keeffe JR, Van Rompay KKA, Olsen PC, Wang Q, Gazumyan A, Azzopardi SA, et al. A Combination of Two Human Monoclonal Antibodies Prevents Zika Virus Escape Mutations in Non-Human Primates. Cell Rep (2018) 25(6):1385–94 e7. doi: 10.1016/j.celrep.2018.10.031
139. Baz M, Boivin G. Antiviral Agents in Development for Zika Virus Infections. Pharmaceuticals (Basel) (2019) 12(3):101–16. doi: 10.3390/ph12030101
140. Mumtaz N, Jimmerson LC, Bushman LR, Kiser JJ, Aron G, Reusken C, et al. Cell-Line Dependent Antiviral Activity of Sofosbuvir Against Zika Virus. Antiviral Res (2017) 146:161–3. doi: 10.1016/j.antiviral.2017.09.004
141. Mesci P, Macia A, Moore SM, Shiryaev SA, Pinto A, Huang CT, et al. Blocking Zika Virus Vertical Transmission. Sci Rep (2018) 8(1):1218. doi: 10.1038/s41598-018-19526-4
142. de Souza AAA, Torres LR, Lima LRP, de Paula V, Barros JJ, Bonecini-Almeida MDG, et al. Inhibition of Brazilian ZIKV Strain Replication in Primary Human Placental Chorionic Cells and Cervical Cells Treated With Nitazoxanide. Braz J Infect Dis (2020) 24(6):505–16. doi: 10.1016/j.bjid.2020.09.001
143. Nixon GL, Moss DM, Shone AE, Lalloo DG, Fisher N, O’Neill PM, et al. Antimalarial Pharmacology and Therapeutics of Atovaquone. J Antimicrob Chemother (2013) 68(5):977–85. doi: 10.1093/jac/dks504
144. Stefanik M, Valdes JJ, Ezebuo FC, Haviernik J, Uzochukwu IC, Fojtikova M, et al. FDA-Approved Drugs Efavirenz, Tipranavir, and Dasabuvir Inhibit Replication of Multiple Flaviviruses in Vero Cells. Microorganisms (2020) 8(4):599–616. doi: 10.3390/microorganisms8040599
145. Bhatia HK, Singh H, Grewal N, Natt NK. Sofosbuvir: A Novel Treatment Option for Chronic Hepatitis C Infection. J Pharmacol Pharmacother (2014) 5(4):278–84. doi: 10.4103/0976-500X.142464
146. Li Z, Brecher M, Deng YQ, Zhang J, Sakamuru S, Liu B, et al. Existing Drugs as Broad-Spectrum and Potent Inhibitors for Zika Virus by Targeting NS2B-NS3 Interaction. Cell Res (2017) 27(8):1046–64. doi: 10.1038/cr.2017.88
147. Korba BE, Montero AB, Farrar K, Gaye K, Mukerjee S, Ayers MS, et al. Nitazoxanide, Tizoxanide and Other Thiazolides are Potent Inhibitors of Hepatitis B Virus and Hepatitis C Virus Replication. Antiviral Res (2008) 77(1):56–63. doi: 10.1016/j.antiviral.2007.08.005
148. Rossignol JF, El-Gohary YM. Nitazoxanide in the Treatment of Viral Gastroenteritis: A Randomized Double-Blind Placebo-Controlled Clinical Trial. Aliment Pharmacol Ther (2006) 24(10):1423–30. doi: 10.1111/j.1365-2036.2006.03128.x
149. Amadi B, Mwiya M, Musuku J, Watuka A, Sianongo S, Ayoub A, et al. Effect of Nitazoxanide on Morbidity and Mortality in Zambian Children With Cryptosporidiosis: A Randomised Controlled Trial. Lancet (2002) 360(9343):1375–80. doi: 10.1016/S0140-6736(02)11401-2
150. Ortiz JJ, Ayoub A, Gargala G, Chegne NL, Favennec L. Randomized Clinical Study of Nitazoxanide Compared to Metronidazole in the Treatment of Symptomatic Giardiasis in Children From Northern Peru. Aliment Pharmacol Ther (2001) 15(9):1409–15. doi: 10.1046/j.1365-2036.2001.01066.x
151. Rossignol JF, Ayoub A, Ayers MS. Treatment of Diarrhea Caused by Giardia Intestinalis and Entamoeba Histolytica or E. Dispar: A Randomized, Double-Blind, Placebo-Controlled Study of Nitazoxanide. J Infect Dis (2001) 184(3):381–4. doi: 10.1086/322038
152. Stockis A, De Bruyn S, Gengler C, Rosillon D. Nitazoxanide Pharmacokinetics and Tolerability in Man During 7 Days Dosing With 0.5 G and 1 G B.I.D. Int J Clin Pharmacol Ther (2002) 40(5):221–7. doi: 10.5414/CPP40221
153. Musher DM, Logan N, Hamill RJ, Dupont HL, Lentnek A, Gupta A, et al. Nitazoxanide for the Treatment of Clostridium Difficile Colitis. Clin Infect Dis (2006) 43(4):421–7. doi: 10.1086/506351
154. Piacentini S, La Frazia S, Riccio A, Pedersen JZ, Topai A, Nicolotti O, et al. Nitazoxanide Inhibits Paramyxovirus Replication by Targeting the Fusion Protein Folding: Role of Glycoprotein-Specific Thiol Oxidoreductase Erp57. Sci Rep (2018) 8(1):10425. doi: 10.1038/s41598-018-28172-9
155. Dang W, Xu L, Ma B, Chen S, Yin Y, Chang KO, et al. Nitazoxanide Inhibits Human Norovirus Replication and Synergizes With Ribavirin by Activation of Cellular Antiviral Response. Antimicrob Agents Chemother (2018) 62(11):e00707–24. doi: 10.1128/AAC.00707-18
156. Rossignol JF. Nitazoxanide: A First-in-Class Broad-Spectrum Antiviral Agent. Antiviral Res (2014) 110:94–103. doi: 10.1016/j.antiviral.2014.07.014
157. Rossignol JF, La Frazia S, Chiappa L, Ciucci A, Santoro MG. Thiazolides, a New Class of Anti-Influenza Molecules Targeting Viral Hemagglutinin at the Post-Translational Level. J Biol Chem (2009) 284(43):29798–808. doi: 10.1074/jbc.M109.029470
158. Cao RY, Xu YF, Zhang TH, Yang JJ, Yuan Y, Hao P, et al. Pediatric Drug Nitazoxanide: A Potential Choice for Control of Zika. Open Forum Infect Dis (2017) 4(1):ofx009–14. doi: 10.1093/ofid/ofx009
159. Yamamoto M, Ichinohe T, Watanabe A, Kobayashi A, Zhang R, Song J, et al. The Antimalarial Compound Atovaquone Inhibits Zika and Dengue Virus Infection by Blocking E Protein-Mediated Membrane Fusion. Viruses (2020) 12(12):1475–92. doi: 10.3390/v12121475
Keywords: Zika virus, decidual cells, trophoblasts, maternal-fetal interface, vertical transmission
Citation: Guzeloglu-Kayisli O, Kayisli UA, Schatz F and Lockwood CJ (2022) Vertical Zika Virus Transmission at the Maternal-Fetal Interface. Front.Virol. 2:801778. doi: 10.3389/fviro.2022.801778
Received: 25 October 2021; Accepted: 04 March 2022;
Published: 24 March 2022.
Edited by:
Kristina M. Adams Waldorf, University of Washington, United StatesReviewed by:
Julie Eggenberger, University of Washington, United StatesCopyright © 2022 Guzeloglu-Kayisli, Kayisli, Schatz and Lockwood. This is an open-access article distributed under the terms of the Creative Commons Attribution License (CC BY). The use, distribution or reproduction in other forums is permitted, provided the original author(s) and the copyright owner(s) are credited and that the original publication in this journal is cited, in accordance with accepted academic practice. No use, distribution or reproduction is permitted which does not comply with these terms.
*Correspondence: Ozlem Guzeloglu-Kayisli, b3psZW0yQHVzZi5lZHU=; Charles Joseph Lockwood, Y2psb2Nrd29vZEB1c2YuZWR1
Disclaimer: All claims expressed in this article are solely those of the authors and do not necessarily represent those of their affiliated organizations, or those of the publisher, the editors and the reviewers. Any product that may be evaluated in this article or claim that may be made by its manufacturer is not guaranteed or endorsed by the publisher.
Research integrity at Frontiers
Learn more about the work of our research integrity team to safeguard the quality of each article we publish.