- 1Artemis Bio-Support, Delft, Netherlands
- 2Department of Viroscience, Erasmus Medical Center, Rotterdam, Netherlands
Following the disruptive epidemics throughout the Indian Ocean, Southeast Asia and the Americas, efforts have been deployed to develop an effective vaccine against chikungunya virus (CHIKV). The continuous threat of CHIKV (re-)emergence and the huge public health and economic impact of the epidemics, makes the development of a safe and effective vaccine a priority. Several platforms have been used to develop candidate vaccines, but there is no consensus about how to translate results from preclinical models to predict efficacy in humans. This paper outlines a concept of what constitutes an effective vaccine against CHIKV, which may be applied to other viral vaccines as well. Defining endpoints for an effective vaccine is dependent on a proper understanding of the pathogenesis and immune response triggered during infection. The preclinical model adopted to evaluate experimental vaccines is imperative for the translation of preclinical efficacy data to humans. Several CHIKV animal models exist; however, not all provide suitable endpoints for measuring vaccine efficacy. This review summarizes the current knowledge related to CHIKV pathogenesis and the correlates of protection. We then define what would constitute an effective CHIKV vaccine in humans using four key endpoints, namely: (i) prevention of chronic disease, (ii) prevention of acute disease, (iii) prevention of transmission to mosquitoes, and (iv) complete prevention of infection. Lastly, we address some of the gaps that prevent translation of immunogenicity and efficacy findings from preclinical models to humans, and we propose to use the combination of virus–cytokine–ferritin levels as a read-out for measuring vaccine-induced protection.
Introduction
Chikungunya is an arthrogenic disease caused by chikungunya virus (CHIKV), an arbovirus from the family Togaviridae, genus Alphavirus. The virus is spread by Aedes (Ae) aegypti and Ae albopictus mosquitoes. Before 2004, outbreaks caused by CHIKV were mostly restricted to several countries in Africa and Asia. In 2004, a large epidemic started on the coast of Kenya, from where the virus subsequently spread to several islands in the Indian Ocean, including La Réunion, but also to India and Southeast Asia (1). Following these outbreaks, the virus spread from Asia to the Americas and Europe, resulting in millions of cases of severe, debilitating and often chronic arthralgia/arthritis. Due to high morbidity and substantial economic loss, CHIKV is, in addition to dengue virus, an important arbovirus in Asia, Latin America and the Caribbean. In addition, the virus currently also poses a real threat to Europe following an outbreak in France in 2014 and in Italy in 2007 and 2017 (2–4). During CHIKV outbreaks, a high case/infection ratio has been reported, with up to 70–80% of infected individuals developing a febrile disease marked by a sudden onset of high fever, rash, and polyarthritis/polyarthralgia. Symptoms of polyarthritis or polyarthralgia may last for months to years after infection, causing significant financial as well as public health burdens (5). Following the disruptive epidemics throughout the Indian Ocean, Southeast Asia, and the Americas, considerable efforts have been deployed to develop an effective prophylactic vaccine against CHIKV. Several platforms have been used to develop candidate vaccines, such as live attenuated vaccines, vectored vaccines, subunit vaccines, virus-like particles (VLPs), and nucleic acid vaccines. Although some vaccine candidates have reached clinical trials, conducting trials is becoming a challenging task. After the first wave of CHIKV epidemics, the incidence of new outbreaks in the succeeding years usually declines due to development of herd immunity, which makes evaluation of candidate vaccines extremely difficult. As a result, defining clinical endpoints and correlates of protection in relevant preclinical models is an important endeavor. When done carefully, the “Animal Rule” pathway can be used to support the licensure procedure (1). The Animal Rule indicates that vaccine efficacy studies based on well-controlled animal studies are enough to allow the vaccine to enter clinical trials, provided that the results of those studies demonstrate reasonable evidence that the vaccine will provide clinical benefit in humans. However, what are the relevant endpoints and markers of protective immunity in CHIKV preclinical models? Here we provide a definition of vaccine efficacy that may help in the evaluation of candidate vaccines and the decision-making process of whether to bring a candidate vaccine further in the development pipeline.
Characteristics of Chikungunya Virus Infection in Humans
It is not clear what the cellular receptor for CHIKV entry of target cells is, but it is likely be ubiquitous given the large tropism of CHIKV for different species and cell types (6). Recently, Mxra8, an adhesion molecule found in mammals, birds, and amphibians, has been identified as a potential receptor (7). In humans, CHIKV spreads rapidly in the body after initial infection, targeting various cell types, including fibroblasts, keratinocytes, melanocytes, dendritic cells, macrophages, myotubes, satellite cells, osteoblasts, osteoclasts, chondrocytes, and neurons (6). CHIKV tropism and disease severity in humans might vary with age (8), genetic background and presence of co-morbidities (9). In addition, severe neurological disease has been frequently reported in neonates whose mothers were viremic at the time of delivery (10). In the mouse and non-human primate models, the virus has been detected in the same cell types in the joint, muscle, skin, liver, and to a lesser extent in the kidneys, eye and the central nervous system (CNS) (11, 12). In general, the main target cells in affected organs are fibroblasts, epithelial cells and monocytes/macrophages.
The clinical signs of acute infection include high fever (>38.5°C) and shivers, skin rash, weakness, headache, and severe joint and muscle pain. In most cases, the symptoms remain for about 4–7 days as a self-limiting disease and are followed by a complete patient recovery (13). Frequently observed laboratory abnormalities include pancytopenia (anemia, moderate thrombocytopenia and leukopenia with marked lymphopenia), increased prothrombin time, hyperglycemia, hypoalbuminemia, hypocalcemia, and increased liver enzymes, LDH, and creatine kinase. Mononuclear cell infiltration and viral replication in the muscles (particularly skeletal muscle satellite (progenitor) cells, not muscle fibers) and joints (in fibroblasts of the joint capsule and presumably in osteoblasts) are associated with debilitating arthralgia, myalgia, and in some cases, arthritis (14). The acute stage can last up to one month. The post-acute stage of CHIKV is defined as the period from one month to the third month after the onset of acute disease. This stage is characterized by very polymorphous manifestations, including periarticular involvement, slowly regressive enthesitis, tenosynovitis and bursitis, together with nonrheumatic and systemic symptoms (15). This stage of the disease is marked by production of several inflammatory mediators. Approximately 40–60% of cases may progress to the chronic phase (>3 months) (16, 17) that may last up to several years. The CHIKV outbreak on Reunion Island in 2006 indicated that persistence of myalgia, arthralgia and asthenia may last up to 36 months post-infection in 60–67% of chronic cases.
Chikungunya Virus Lineages
The high neutral mutation and evolutionary rates characteristic of RNA viruses, including CHIKV, play a role in the potential adaptation to new vectors or are the cause of antigenic drifts, which could allow evasion of the host immune system (18). In addition, extensive traveling results in infection of large populations with diverse genetic backgrounds, which further impacts CHIKV diversity. Previous evolutionary studies grouped CHIKV into three geographically-associated lineages based on their origin: West African (WA) (isolates from Senegal and Nigeria), East/Central/South African (ECSA) (isolates from Uganda, Tanzania, Democratic Republic of Congo) and Asian (isolates from India, Malaysia, Indonesia, Philippines, Thailand) (8, 19). However, phylogenetic analyses of strains from 2005 and later, recovered from the Indian Ocean, Indian sub-continent and the Americas, revealed new insights that resulted in additional clusters of CHIKV, dubbed according to the geographical origin. All strains characterized after 2005 shared a common ancestor with the ECSA genotype. A recent study conducted by Casal et al. based on Bayesian phylogenetic analysis showed further subdivision of the ECSA clade into three sub-groups: ECSA-I, ECSA-II, and ECSA-III [previously reported as the Indian Ocean Lineage (IOL)], all of which have been reported to share a common ancestor (20) (Figure 1).
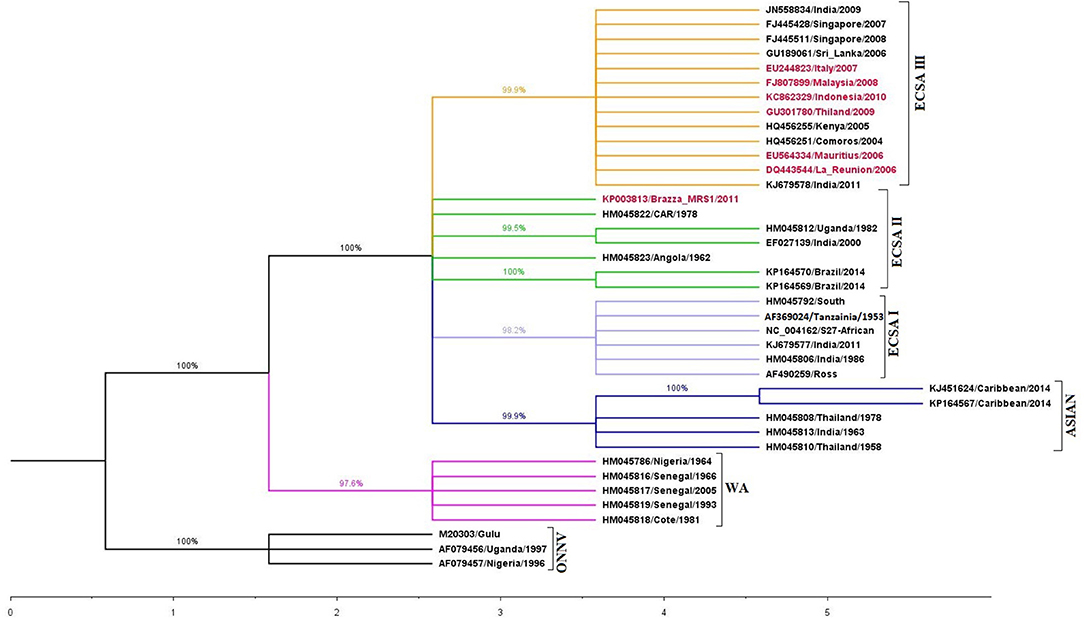
Figure 1. Phylogenetic analysis based on E1 gene sequence of different Chikungunya virus strains. The tree was constructed using maximum likelihood (ML) method with 1,000 bootstrap resampling, using Kimura 2-parameter model + Gamma Distributed (K2+G) in MEGA 5.2.2. The accession numbers highlighted in red contains the Ala226Val mutation. Different clades are marked with different color (ECSA-I: yellow; ECSA-II: green; ECSA-III: light blue; Asian: dark blue; West African (WA): pink and O'nyong'nyong virus (ONNV): black).
Antigenic Structure, Genotypes and Vaccine Development
CHIKV consists of two glycoproteins (E1 and E2) that are involved in infection of cells. The E1 glycoprotein of CHIKV consists of 442 amino acids that form three β-barrel domains (4), namely domains I, II, and III. The CHIKV E2 glycoprotein consists of 423 amino acids that form domains A, B, and C. Domain A has been shown to contain the receptor binding site and to be involved in cell-to-cell transmission (21). Domain B functions more as a mask for the fusion loop on domain II of E1, and domain C is proximal to the viral membrane with an as yet unknown function (Figure 2).
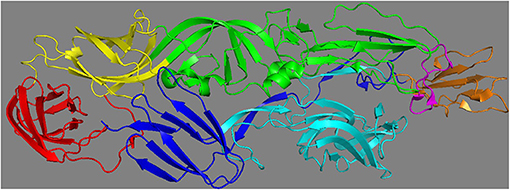
Figure 2. Different domains of Chikungunya virus E1 and E2 glycoprotein (PDB: 3N42). The E1 glycoprotein consists of three domains: I (in yellow), II (in green), III (in red) and the fusion loop (in pink). E2 glycoprotein consists of three domains: A (in cyan), B (in orange) and C (in blue).
The majority of neutralizing antibodies induced during CHIKV infection has been shown to target E2 (22, 23), which can be explained by the fact that the E2 glycoprotein is the more exposed of the two. Few studies have also identified neutralizing antibodies directed to E1 (24). Several mechanisms of antibody neutralization of CHIKV have been described. The most relevant mechanisms of neutralization are believed to be (1) prevention of CHIKV domain A and B binding to and entry into target cells, (2) prevention of fusion by E1, and (3) prevention of budding from infected cells by targeting E1 and E2. Since domain B plays an important role in protecting the fusion loop in E1, antibodies targeting domain B may also have a neutralization effect by preventing fusion (25). Potent vaccines are able to induce antibodies that neutralize at all three levels (multi-target neutralization) and therefore prevent infection of cells or spread from the initial site of infection. Developing an assay that allows for a better understanding of the mechanisms of neutralization may be important in the rational design of vaccines and understanding the correlates of protection. It is important to realize that there is currently no consensus of what constitutes an effective CHIKV vaccine.
Several lines of evidence indicate that antibodies, both neutralizing and nonneutralizing, correlate with protection. First, asymptomatic cases have been associated with high levels of neutralizing antibodies (26). Second, B cell-deficient mice (μMT) infected with CHIKV exhibited a long-lasting persistent viremia and more severe joint disease compared to wild-type mice, suggesting an important role for B cells in the resolution of the disease (27). Furthermore, studies involving the transfer of antibodies significantly altered the course of disease as passive transfer of IgG antibodies from convalescent patients was able to prevent and cure CHIKV infection in two mouse models (28). Other studies indicated that antibodies against both E1 and E2 may provide protection against acute and chronic chikungunya (29–31). In addition to protection, antibodies have also been shown to be involved in recovery (30). It is less clear however, how much neutralizing antibodies are needed to provide protection from infection or disease. In a prospective cohort study from the Philippines it was estimated that an anti-CHIKV PRNT (full form) titer ≥10 was associated with protection from development of symptomatic CHIKV infection (26).
With regard to vaccine development, it is important that a vaccine induces cross-neutralization between the CHIKV genotypes. The available data suggests that there is cross-neutralization between lineages, a conclusion mainly based on evaluation of the neutralizing antibody responses in vitro (32, 33) using only a few strains from each genotype. Several antibody epitopes have been identified in E1, E2, and E3 (27, 34, 35), of which some are known to be conserved among different strains of both the ECSA and Asian genotypes. In addition, it has been shown that a CHIKV vaccine could cross-protect against the closely related (85% homology) O'nyong'nyong virus, an African alphavirus (36). Furthermore, CHIKV vaccination followed by a heterologous CHIKV challenge in vivo also supported the conclusion of cross-protection, demonstrating that CHIKV neutralizing antibodies to one genotype are highly effective against the other genotypes (37).
Since viruses belonging to the ECSA and Asian lineages are major causes of recent outbreaks, it is important to develop safe and effective vaccines that would provide protection against all members of these two major lineages. It is still not clear whether differences in virulence and pathogenesis exist among lineages and sublineages. Although there is no unified definition of the word virulence (pathogenic), it may be defined as the ability of one CHIKV strain to cause more severe disease over another CHIKV variant. There is some evidence that strains that belong to the ECSA lineage cause more joint inflammation, higher levels of inflammatory cytokines and feet swelling in mice (37–39). In addition, it has been suggested from an observational study that the Asian lineage may be less virulent since strains of the Asian lineage have been associated with a higher rate of subclinical infections and less persistent arthralgia than the ECSA strains (26). This theory was recently supported by a meta-analysis of publications about chronic chikungunya (9). However, since only a few isolates from each lineage have been evaluated in experimental settings, and virulence is the outcome of the interaction between the immune system and the virus, it is difficult to conclude whether differences in clinical manifestations are strain- or lineage-dependent.
Correlates of Pathogenesis
Viral Load
High viral load in the acute phase of CHIKV infection represents a significant risk factor for developing chronic disease (40, 41). Although both newborns and older patients develop high viral load during CHIKV infection, the predictive value of viremia is especially high in older patients. During the acute phase, viral loads ranging from 104 to 1012 copies/ml have been reported (42). Analysis of the literature suggests that patients with CHIKV viremia titers of 104-106 copies/ml were more likely to develop acute disease (42), whereas patients with titers >107 copies/ml were more likely to develop chronic disease (40, 41). Interestingly, high viral loads were also associated with higher levels of pro-inflammatory cytokines, such as IL-6 (43). Although several studies have described an association between viral load and age with disease severity, high viral loads have also been measured in some asymptomatic individuals (44), indicating that other factors such as genetic background are also involved in the development of chronic disease.
The biggest public health impact of chikungunya is without any doubt the development of debilitating polyarthralgia/arthritis in the acute and chronic phase, which has been associated with levels of viremia early in the disease (41). Chronic arthralgia has been linked to persistent virus replication in the target cells and/or the establishment of a self-sustained inflammatory response that leads to tissue damage (11, 45). CHIKV RNA has been detected in synovial macrophages, and satellite muscle cells contain viral RNA or protein even months after infection (40). In agreement with these observations, long-term persistence of CHIKV infection and chronic joint disease has occurred in experimentally infected nonhuman primates and mice (11, 46), suggesting that residual virus or viral products in tissues may promote chronic inflammation (40). In contrast, Chang et al. did not detect CHIKV RNA in synovial fluid of chronic patients, suggesting that CHIKV may not always persist in synovial fluid or the virus was below the detection limit of the assays used (47). Although the mechanisms leading to chronic chikungunya have not been completely elucidated, a very likely hypothesis is that extensive virus replication early during infection, and subsequently persistence of CHIKV and chronic inflammation, lead to the symptoms of chronic joint disease.
Cytokines
There is evidence that activation of the innate immune response by alphavirus infection results in osteoblast and osteoclast proliferation and dysfunction, which may contribute to the effects of chronic CHIKV. In this respect, high levels of TNF-α, IL-6, and IL-1 may promote osteoclast activity, which has been associated with osteoclastogenesis (48, 49). Although a number of inflammatory cytokines, such as IL-6, IL-7, IL-12p40, IL-16, IL-17, IP-10, MCP-1, MIF, SDF-1α, IL-1Rα, IL-2Rα, G-CSF, GM-CSF, VEGF, and IFN-α2, have been measured during acute CHIKV infection (50), high levels of IL-6 were identified as a biomarker for severe and persistent arthralgia (43, 51, 52). IL-6 has also been identified as a marker for rheumatoid arthritis (53), suggesting a potential immunopathologic association of IL-6 with chronic chikungunya. Specifically, for IL-6, elevated levels were found to be associated with acute CHIKV disease in humans (51, 54) and in monkeys (11), or with the development of CHIKV chronic disease in humans (43, 48, 51, 55, 56). Furthermore, in a study using the CHIKV arthritis mouse model, serum IL-6 levels correlated with peak viremia (38).
Ferritin
Elevated serum levels of ferritin have been found in individuals infected with bacteria (57), but also with protozoa, such as Plasmodium vivaxin (58), the malaria parasite. So far, only a few studies have investigated the association between cytokines and hyperferritinemia during severe viral infections in humans (59–64). High concentrations of ferritin have also been found to be associated with CHIKV load and severe disease (16, 65). Therefore, given the positive correlation between CHIKV load and IL-6 (43), as well as between viral load and ferritin (16), these three molecules can also be useful as markers for disease severity. However, as little is known about the role of serum ferritin and its relation to CHIKV pathogenesis and disease outcomes, more efforts should be employed to understand the kinetics of ferritin during CHIKV infection in different animal models.
The mechanisms that regulate iron homeostasis involve two molecules, hepcidin and ferroportin. Hepcidin is produced mainly in the liver and its expression and release follows the pattern of acute phase proteins. It is regulated by several stimuli, including tissue iron levels, anemia, hypoxia, and pro-inflammatory cytokines, such as IL-6. Hepcidin decreases circulating iron levels by binding and promoting the internalization and degradation of the iron exporter, ferroportin. Accumulation of iron in cells leads to increased translation of ferritin (66) aimed to store the iron. Ferritin is therefore mainly considered to be the intracellular storage protein of iron. As small amounts of ferritin are usually secreted by cells into the blood, the concentration of serum ferritin is, under normal circumstances, positively correlated with the amount of iron stored in the body.
The source of circulating ferritin during CHIKV infection remains unclear and studies should be designed to investigate this.
An Integrated Model of Pathogenesis: the Virus—Cytokine—Ferritin (VCF) Model
Conceptually, viral pathogenesis may manifest as the result of cellular dysfunction and/or cell death. Several cell death pathways have been described that may occur independently (66, 67), sequentially, as well as simultaneously. CHIKV infection has been shown to induce apoptosis and autophagy both in vitro and in vivo (68–72). Although less is known about the contribution of other cell death pathways to the pathogenesis of chikungunya, there is evidence for ferroptosis as an important effector pathway for Mayaro virus (MAYV), another alphavirus. Infection of two different cell types with MAYV led to increased levels of reactive oxygen species (ROS), lipid peroxidation products and protein carbonyl (73), which are important participants of ferroptosis. Ferroptosis is an iron-dependent form of cell death characterized by a disrupted iron homeostasis, increased lipid peroxidation and an accompanying insufficient capacity to eliminate lipid peroxides. Here, we propose a theory that integrates viral load, IL-6 and ferroptosis, resulting in hyperferritinemia (Figure 3).
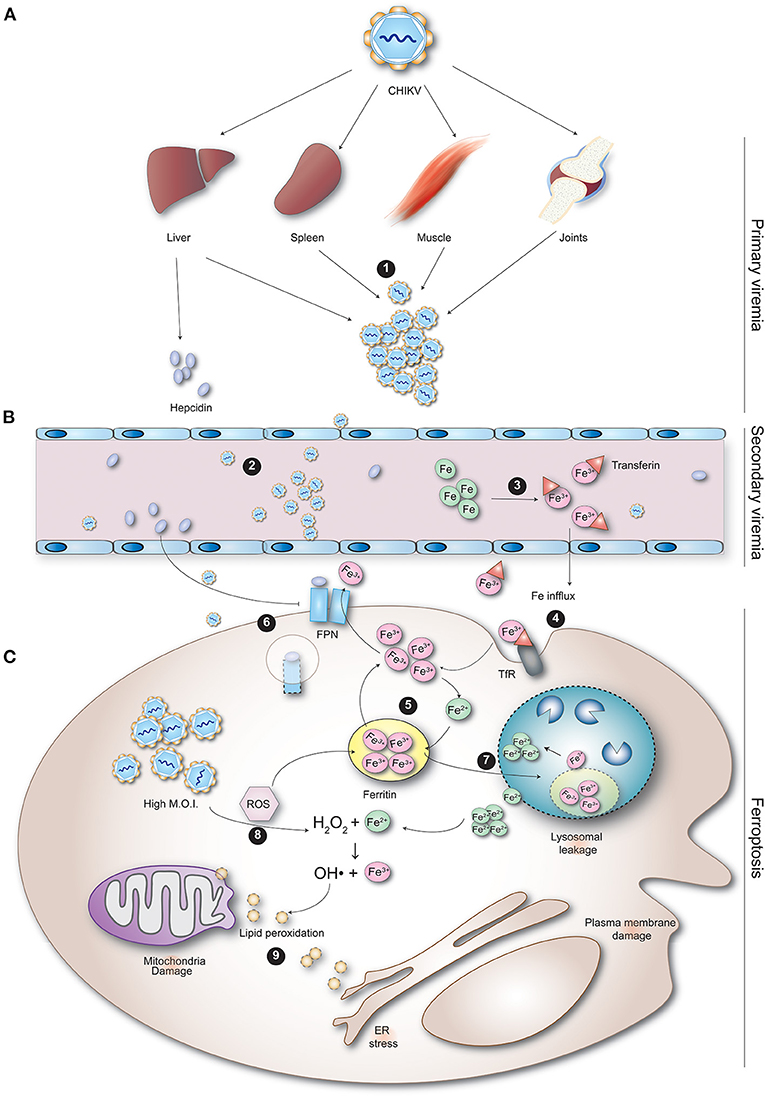
Figure 3. CHIKV-induced ferroptosis as a potential cause of hyperferritinemia observed during infection. (A) During primary viremia, CHIKV spreads from regional lymph nodes via the blood to infect cells in visceral organs, including the spleen, liver, joints and muscles. (1) After replication in these organs, (B) CHIKV enters the blood again (secondary viremia) to spread to other tissues. (2) Replication of the virus inside the organs and immune cells causes cytokinemia. (3) Cytokines such as IL-1β, IL-6, and IFN-α cause the upregulation of hepcidin. (C) (4) Hepcidin binds to ferroportin, blocking release of iron from the cell decreasing the iron in circulation. (5) In addition, the regular influx of iron into the cell via TfR increases the intracellular iron. (6) Iron overload in the cell leads to increased production of ferritin, which may also be stimulated by IL-6 directly. (7) The cytokine response and replication of CHIKV inside the infected cell trigger both oxidative and endoplasmic reticulum stress resulting in increased production of reactive oxygen species (ROS) and reactive nitrogen species (RNS). ROS further up-regulates release of hepcidin from infected cells. The free oxidative agents produced during infection damage ferritin. (8) Ferritin aggregates stimulate autophagy (ferritinophagy). The damaged ferritin taken up into autophagosomes eventually fuse with lysosomes (forming autolysosomes). (9) The lysosomal degradation of ferritin causes release of the ferrous iron into the cytosol. The reductive milieu reduces ferrous iron into ferric iron. This reactive iron damages the lysosomal membrane, causing lysosomal leakage. The lysosomal leakage enhances autophagy resulting in a self-perpetuating cycle of lysosomal damage. (10) The cytosolic ferrous iron is then oxidized by hydrogen peroxide (H2O2) to ferric iron, forming toxic hydroxyl radicals (•OH). (11) The free radicals and H2O2 mediate oxygenation of phospholipid and polyunsaturated fatty acids, resulting in lipid peroxides. Lipid peroxidation alters the physical properties of lipid bilayers leading to ferroptosis.
Following inoculation of CHIKV into the skin, the virus infects susceptible cells in the dermis. The virus is then transported to the local draining lymph nodes where it is further amplified and transported into the blood, where it subsequently infects cells in different organs, including the spleen, liver, joints, and muscles. CHIKV infection induces a pro-inflammatory response with the up-regulation of multiple inflammatory mediators (40, 43, 50, 51, 55, 74). The inflammatory cytokines IL-1β, IL-6, and IFN-α regulate the expression of hepcidin (75, 76). Hepcidin down-regulates iron release by enterocytes and macrophages by binding to ferroportin and inducing its internalization and degradation (77), increasing the intracellular iron load and decreasing the amount of iron in circulation. The iron overload in the cell leads to the increased production of ferritin. In addition, IL-6 may stimulate the production of ferritin directly. This has, for example, been seen in rats who were injected intraperitoneally with IL-6, which led to enhanced hepatic transferrin uptake and ferritin expression (78). Concomitantly, the cytokine response together with CHIKV replication in infected cells trigger both oxidative and endoplasmic reticulum stress resulting in increased production of ROS and reactive nitrogen species (RNS). This is in agreement with a study that found higher intracellular ROS in peripheral blood mononuclear cells of patients suffering from persistent polyarthralgia post-CHIKV infection compared to healthy subjects (79). ROS further up-regulates release of hepcidin from infected cells (80). ROS are molecules that contain radical oxygen species formed by the partial reduction of oxygen, such as superoxide anion (O2-), hydrogen peroxide (H2O2), and hydroxyl radical (•OH). ROS are by-products of normal or stress-induced metabolic reactions and may have both beneficial and harmful effects. However, the damaging effect of ROS is dependent on the concentration and failure of the antioxidant system to restore the oxidative stress (imbalance between oxidants and antioxidants present in the cell resulting in dysfunctional redox signaling). It is conceivable that excess ROS production is triggered by a high cellular multiplicity of infection in vivo caused by the high viral load (81, 82).
The free oxidative agents produced during infection damage ferritin, resulting in aggregates that stimulate autophagy. This selective form of autophagy is referred to as ferritinophagy, which requires the cargo receptor NCOA4 for delivery of ferritin to the lysosome (83). Following autophagic uptake of oxidatively damaged ferritin, iron is released from ferritin by lysosomal degradation of the protein. This process involves the formation of autophagosomes that contain damaged ferritin and other cell materials, which will subsequently fuse with lysosomes to form autolysosomes. The acidic pH in autolysosomes results in degradation of ferritin and release of iron, and due to the reductive milieu, ferric iron is reduced into ferrous iron. This reactive iron causes damage to lysosomal membranes, resulting in lysosomal leakage. The cytosolic ferrous iron is then oxidized by hydrogen peroxide (H2O2) to ferric iron, forming a hydroxyl radical (•OH), in a reaction known as the Fenton reaction. •OH is one of the most toxic species that can be formed in biological systems. In addition, the lysosomal leakage enhances autophagy resulting in a self-perpetuating cycle of lysosomal damage. Moreover, the free radicals produced during the Fenton reaction, as well as high concentrations of H2O2, mediate oxygenation of phospholipid and polyunsaturated fatty acids, resulting in lipid peroxides. It is unclear where lipid peroxidation takes place, but the plasma membrane, mitochondria, ER, and lysosomes are the potential sites of lipid peroxidation (84). Ultimately, lipid peroxidation is believed to alter the physical properties of lipid bilayers in terms of disrupted ion gradients, decreased membrane fluidity, slower lateral diffusion, and increased membrane permeability (84). This process leads to cell death known as iron-dependent cell death (ferroptosis). Leakage of cell content may therefore explain the increased ferritin levels in circulation and may therefore be considered a marker of cell damage. Splenic macrophages and hepatocytes represent the cells damaged by CHIKV infection, resulting in increased circulating ferritin levels.
In addition, it is conceivable that inflammation induced during the acute phase of CHIKV infection decreases the half-life of erythrocytes (RBCs). This is supported by clinical observations showing that anemia is frequently detected in patients with severe chikungunya (85–87). The decreased RBC lifespan in inflammation is probably due to an altered morphology of the RBCs, resulting in increased adherence to the splenic endothelium and clearance from the circulation by splenic macrophages. As RBCs are an important reservoir of ferritin, and splenic macrophages are known to recycle iron, damage of splenic macrophages is possibly the most important source of ferritin.
Relevant Preclinical Animal Models in Chikv Vaccine Evaluation
In order to use preclinical animal models to infer vaccine efficacy, similar endpoints must be defined in humans and animal models. The use of relevant animal models is therefore crucial; i.e., similar tropism, correlates of disease and correlates of protection. Several animal models have been described for studying CHIKV pathogenesis and evaluating candidate vaccines (12, 88). Mice have been used in the majority of cases and despite the many advantages of the mouse model, mice lack signs of polyarthralgia and chronic inflammation, which are typical clinical symptoms observed in chikungunya patients. The macaque model (old cynomolgus macaques or rhesus macaques) has been shown to recapitulate the chronic disease seen in patients, but is not a model that can be used routinely. Therefore, more efforts should be deployed to develop better mouse models. So far three mouse models of CHIKV have been described, each for a different purpose (12): the (1) neuroinvasive model, (2) immunocompromised model and (3) acute arthritis model. In most experiments, mice were challenged by either the intranasal (i.n), subcutaneous (s.c) or intraperitoneal (i.p) route (Table 1).
• The neurologic model is based on the use of neonatal or adult mice (see below). Neonatal mice <12 days old are extremely susceptible to CHIKV infection, and are known to develop lethal encephalitis (110). The tropism of CHIKV for the CNS and the multi-system involvement observed in neonatal mice is similar as described in neonatal humans (111). Therefore, this model is suitable for studying human neonatal infection and it provides a system for studying viral and host factors that contribute to severe disease in neonates. In adult mice, only neurological symptoms are decribed and is therefore more appropriate for studying CHIKV neuroinvasive disease. Although time-consuming, this both models may be used to evaluate the protective capacity of neutralizing antibodies, using a vaccination/adoptive transfer/challenge design.
• The immunocompetent viremic model has been developed using both inbred (C57BL/6, BALB/c) (93) and outbred mice (NIH Swiss) (94). In this model, mice are often infected with the neuroadapted CHIKV Ross strain via the intranasal route, and depending on their age, this can lead to fatal encephalitis. Typically, in BALB/c mice exceeding 6 weeks of age, challenge usually does not lead to fatality (105–108). As a result, immunocompetent mice (C57BL/6, NIH Swiss, BALB/c) of 6 weeks or younger are usually used when survival is a desired outcome for determining vaccine efficacy (93, 94). Nonetheless, one study has demonstrated mortality in BALB/c mice at 14 weeks of age (95), while another study showed that also C57BL/6 mice of 13–14 weeks-old died as a result of i.n. CHIKV infection (92). This outcome, however, may be explained by the fact that both studies used the CHIKV PC-08 strain, which apparently can lead to fatal encephalitis in older mice. Although this model usually measures entry of virus into the brain, also low viremia of short duration has been reported. Therefore, in immunocompetent mice in which infection does not lead to mortality, viremia can also be considered as a useful endpoint parameter.
• The acute arthritis model is based on the use of immunocompetent inbred (112) or outbred (94) adult mice. Mice are inoculated subcutaneously in the footpad with CHIKV, which results in viremia and arthrogenic disease with maximum swelling in the infected foot within 7 days after infection. Interestingly, severe arthritis, necrotizing myositis, tendonitis and fasciitis, develop in the infected foot (38, 113). This model has been useful for testing vaccines for their ability to protect against CHIKV-induced arthritis and persistent infection (113).
• The immunocompromised lethal model constitutes mice deficient in the type I interferon (IFN) system, which is essential for protection from systemic CHIKV (33, 93). Immunocompromised mice frequently used in the chikungunya field include: A129, AG129, and IRF3/IRF7 knock-out (12). Several studies have shown that these mice can develop neutralizing antibodies after vaccination, which, together with the fact that they die rapidly from CHIKV infection due to their high susceptibility, make them good models for studying sterilizing immunity. Additionally, the AG129 mouse model can also be used as an arthritis model as mice inoculated in the footpad develop arthritis as well (114).
Translating CHIKV Vaccine Efficacy From Preclinical Models to Humans
In general, vaccine efficacy can be measured using three different parameters: (a) protection against local and/or systemic virus replication, (b) protection from development of clinical disease, and (c) development of an anemnestic response following challenge. For CHIKV, there is no consensus about what would constitute an effective response to a vaccine in humans. In preclinical mouse models, efficacy can mainly be defined as the ability of the vaccine to induce sterilizing immunity and prevent against lethal disease or prevent the development of arthritis. It is important to define clinical endpoints and correlates of protection in preclinical models due to the increasing challenge of performing phase-III trials in humans in endemic areas as a result of herd immunity.
The process of translating preclinical data to predict vaccine effectiveness is similar to the process known as “forecasting by analogy” (115). As outlined above, viral load, IL-6 and ferritin are the hallmarks of the VCF model, as determined from human studies. If relevant studies are conducted in animal models to fill the gap between human and animal responses, the VCF model may be used to predict vaccine efficacy by analogy (Figure 4A).
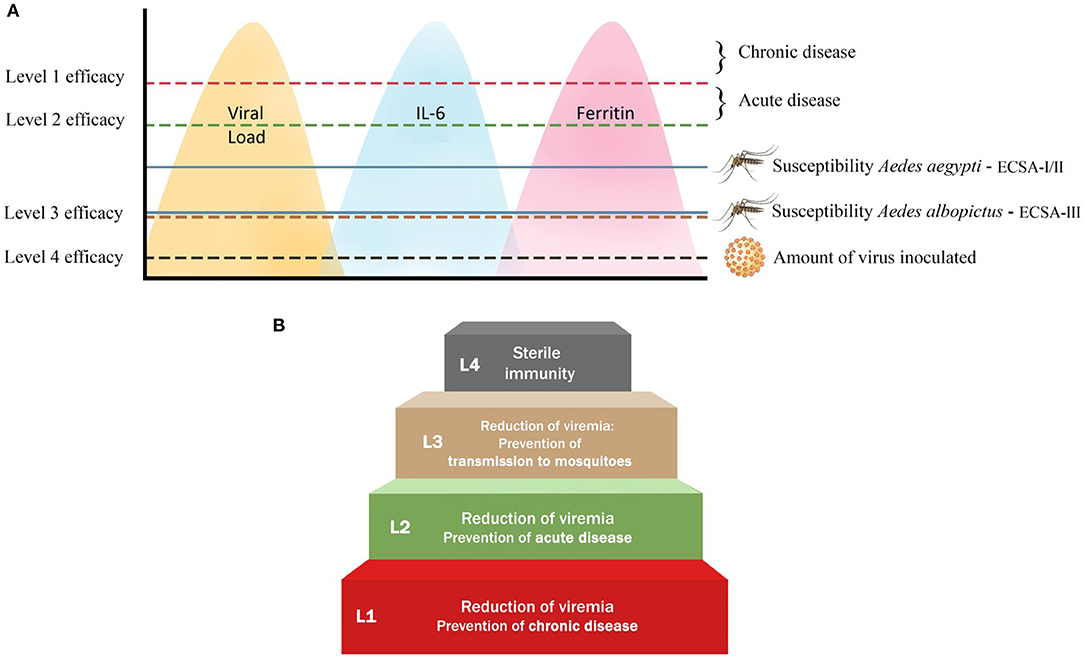
Figure 4. The four efficacy levels that a vaccine against CHIKV can attain in humans. (A) The four different levels of vaccine efficacy are defined in terms of the ability of a CHIKV vaccine to reduce the level of viremia, and consequently the level of IL-6 and ferritin (indicated by the yellow, blue and pink peaks, respectively). Level 1: A vaccine that can lower the amount of virus produced in order to prevent the development of chronic disease (indicated by red line). Level 2: The reduction of viremia to a level that prevents the development of both chronic and acute disease, but transmission to mosquitoes may still occur (indicated by green line). Level 3: A vaccine that is able to reduce viremia to the level below the threshold required to infect Ae aegypti and Ae albopictus (indicated by brown line). Level 4: A vaccine that can provide sterilizing immunity (indicated by black line). The blue lines indicate the viremia level required for Ae aegypti and Ae albopictus to become infected. Level (L) 1 is the minimum requirement for a CHIKV vaccine, while L4 is the ideal requirement that is not easily obtained by a vaccine. (B) The step-wise attainability of CHIKV vaccine efficacy comprising four different levels. L1 vaccines reduce viremia below the threshold that leads to chronic disease; L2 reduces viremia below the level that leads to acute disease; L3 reduces it below the threshold that allows transmission of CHIKV to both A. aegypti and A. albopictus; L4 leads to sterile immunity by lowering the amount of virus to the level found at the inoculation site.
We have determined four key outcomes (efficacy levels) that may identify an effective CHIKV vaccine candidate in humans (Figure 4B). The VCF model may be used for prediction of efficacy from the preclinical animal models.
Level 1: Prevention of Chronic Disease
A vaccine that can lower the amount of virus produced is likely to prevent the development of chronic disease. To this end, the best animal model, which may be translated to humans, is the A129 or AG129 mouse model, where high viremia (>107 copies/ml) is measured after intradermal infection (116, 117). Based on clinical observations in humans, patients with peak virus titers of >107 copies/ml appear to have a higher risk for developing chronic disease (40, 41). As a result, a vaccine that can reduce viremia to below this level may be able to prevent the development of chronic disease. In this scenario, however, acute disease and transmission to mosquitoes can still occur. Furthermore, there is evidence that immunocompromised mice (A129 and AG129) infected with CHIKV also develop high levels of IL-6, which may be predictive of severe disease. Although the association between the concentration of IL-6 and chronic disease has not been established, it is clear that in humans, IL-6 is associated with severe disease (43, 51). Taken together, an effective vaccine would significantly reduce the viral load in blood and target organs, as well as prevent the production of high levels of IL-6, and consequently ferritin.
Level 2: Prevention of Acute Disease
Similar to the evaluation of efficacy level 1, level 2 can also be evaluated in the A129 or AG129 models, as high viremia and IL-6 can be measured in these interferon-deficient mice. At this level of efficacy, viremia is reduced to a level that should prevent the development of both chronic and acute disease, but transmission to mosquitoes may still occur. This definition is based on studies that suggest that patients with viremia titers of 104-106 copies/ml were more likely to develop acute disease (42). As a result, a vaccine able to reduce viremia to <104 copies/ml may be able to prevent acute disease. We propose to evaluate IL-6 and ferritin as potential endpoint markers of vaccine efficacy in the arthritis (immunocompetent and immunosuppressed) mouse model and in non-human primates. In addition, these endpoint markers may be validated during clinical trials.
Level 3: Prevention of Transmission to Mosquitoes
Interfering with the transmission cycle of CHIKV is an attractive approach to reduce or prevent epidemics with the virus. Theoretically, a vaccine that can prevent infection of susceptible mosquitoes could also be considered effective. In other words, the vaccine is able to reduce viremia to the level below the threshold required to infect Ae aegypti and Ae albopictus. Although the infectivity of various CHIKV strains varies widely for both Ae aegypti and Ae albopictus, it has been shown that the susceptibility between them differs significantly. Virus could be detected in the saliva of at least one Ae albopictus mosquito that fed on a blood meal with a minimum dose of 103.9 PFU/ml, while 105.3 PFU/ml of virus was needed for the salivary glands of Ae aegypti to become positive (118). These results suggest that infected individuals (titers are usually >104 copies/ml) may be infectious for Ae albopictus almost the entire period of their viremia. Furthermore, an effective CHIKV candidate vaccine should break the transmission cycle between humans and mosquitoes and, according to these results, must therefore suppress viremia in the host below approximately 104 PFU/ml. However, CHIKV reservoir competence studies still need to be conducted in animal models like macaques and mice in order to confirm the generalization of these results.
Level 4: Prevention of Infection
Sterilizing immunity can be defined as the ability of the immune response to completely neutralize the virus inoculum and prevent infection or inhibit further spread of virus from the site of infection. It is different from the immunity that allows infection to spread, but virus is successfully eradicated afterwards (such as seen for levels 1, 2, and 3). Sterilizing immunity has been observed in a few viral infection studies in preclinical models (119–121). However, what still remains to be determined is the longevity of such a level of immunity. It is also unclear how sterilizing immunity in preclinical models translates to the immunity induced in humans. Protection of immunodeficient mice against infection is the best way to study sterilizing immunity against CHIKV. Absence of viremia and virus in the spleen, as well as lack of an anemnestic response after challenge, can provide evidence for sterilizing immunity. While sterilizing immunity might be an ideal level of vaccine efficacy, the reality is that most vaccines do not elicit durable sterilizing immunity and, as a result, obtaining this endpoint may not be clinically relevant or feasible.
Discussion
Although the model that we have proposed above for humans may consist of plausible parameters for determining vaccine efficacy, practically it cannot be applied in phase III clinical trials as the window of viremia in CHIKV infected individuals is almost impossible to capture. As a result, it is much more practical to determine the levels of vaccine efficacy in animal models. However, a major drawback of animal models is the absence of CHIKV-induced chronic disease as observed in humans. Nonetheless, we categorized the abovementioned animal models for CHIKV infection in the following order of relevance:
(1) lethal-arthritis, (2) lethal, (3) arthritis, and (4) viremic, which are based on the main endpoint parameters that can be measured (as displayed in Table 1) and translated to humans. The lethal-arthritis model involves s.c. or intradermal inoculation of virus in the foot of immunocompromised A129 mice (and possibly AG129), after which swelling can be measured up to 5–8 days post-infection, before the mice succumb to the infection. The main parameter measured in the lethal model is mortality, for which immunocompromised or neonatal mice are generally used. The arthritis model uses immunocompetent mice to measure foot swelling. In both models, viremia can also be measured. Lastly, the viremic model comprises older mice in which challenge does not lead to mortality or foot swelling, but viremia does still occur. Even though mortality and/or arthritis cannot be measured in this model, it is a useful model for identifying vaccine candidates that do not provide any kind of protection. However, its main disadvantage is that it does not provide good translation to humans. In addition to the mouse models, macaques can also be used to measure viremia and/or arthritis. However, due to availability, costs, and ethical arguments, macaques are usually not preferred.
Taken together, the lethal-arthritis model is the most appropriate for prediction of vaccine efficacy in humans because it utilizes the extreme susceptibility of the immunodeficient mouse while allowing the simultaneous testing of the largest number of efficacy parameters, namely mortality, foot swelling, viremia, viral persistence in target organs, levels of IL-6 (and other cytokines) and ferritin. It is unclear, however, which threshold levels of IL-6 and ferritin are associated with acute or chronic disease in mice. Therefore, in order to validate the proposed VCF model, more studies are warranted where all three efficacy endpoints are measured simultaneously.
Author Contributions
BM and SL wrote and edited the manuscript. SD conducted the phylogenetic analysis and protein modeling and contributed to the writing of the related sections. All authors contributed to the article and approved the submitted version.
Conflict of Interest
SL, SD, and BM were employed by company Artemis Bio-Support.
Publisher's Note
All claims expressed in this article are solely those of the authors and do not necessarily represent those of their affiliated organizations, or those of the publisher, the editors and the reviewers. Any product that may be evaluated in this article, or claim that may be made by its manufacturer, is not guaranteed or endorsed by the publisher.
Acknowledgments
We would like to thank Jingshu Zhang for her design of Figure 4.
References
1. Petersen LR, Powers AM, Chikungunya: epidemiology. F1000Res. (2016) 5. doi: 10.12688/f1000research.7171.1
2. Venturi G, Di Luca M, Fortuna C, Remoli ME, Riccardo F, Severini F, et al. Detection of a chikungunya outbreak in Central Italy, August to September 2017. Euro Surveill. (2017) 22. doi: 10.2807/1560-7917.ES.2017.22.39.17-00646
3. Sambri V, Cavrini F, Rossini G, Pierro A, Landini MP. The 2007 epidemic outbreak of Chikungunya virus infection in the Romagna region of Italy: a new perspective for the possible diffusion of tropical diseases in temperate areas? New Microbiol. (2008) 31:303–4.
4. Rezza G, Nicoletti L, Angelini R, Romi R, Finarelli AC, Panning M. et al. Infection with chikungunya virus in Italy: an outbreak in a temperate region. Lancet. (2007) 370:1840–6. doi: 10.1016/S0140-6736(07)61779-6
5. Elsinga J, Gerstenbluth I, van der Ploeg S, Halabi Y, Lourents NT, Burgerhof JG. et al. Long-term chikungunya sequelae in curacao: burden, determinants, and a novel classification tool. J Infect Dis. (2017) 216:573–81. doi: 10.1093/infdis/jix312
6. Matusali G, Colavita F, Bordi L, Lalle E, Ippolito G, Capobianchi MR, et al. Tropism of the Chikungunya Virus. Viruses. (2019) 11. doi: 10.3390/v11020175
7. Zhang R, Kim AS, Fox JM, Nair S, Basore K, Klimstra WB, et al. Mxra8 is a receptor for multiple arthritogenic alphaviruses. Nature. (2018) 557 570–4. doi: 10.1038/s41586-018-0121-3
8. Volk SM, Chen R, Tsetsarkin KA, Adams AP, Garcia TI, Sall AA, et al. Genome-scale phylogenetic analyses of chikungunya virus reveal independent emergences of recent epidemics and various evolutionary rates. J Virol. (2010) 84:6497–504. doi: 10.1128/JVI.01603-09
9. Paixao ES, Rodrigues LC, Costa M, Itaparica M, Barreto F, Gerardin P, et al. Teixeira, Chikungunya chronic disease: a systematic review and meta-analysis. Trans R Soc Trop Med Hyg. (2018) 112:301–16. doi: 10.1093/trstmh/try063
10. Gerardin P, Barau G, Michault A, Bintner M, Randrianaivo H, Choker G, et al. Multidisciplinary prospective study of mother-to-child chikungunya virus infections on the island of La Reunion. PLoS Med. (2008) 5:e60. doi: 10.1371/journal.pmed.0050060
11. Labadie K, Larcher T, Joubert C, Mannioui A, Delache B, Brochard P L, et al. Chikungunya disease in nonhuman primates involves long-term viral persistence in macrophages. J Clin Invest. (2010) 120:894–906. doi: 10.1172/JCI40104
12. Haese NN, Broeckel RM, Hawman DW, Heise MT, Morrison TE, Streblow DN. Animal models of chikungunya virus infection and disease. J Infect Dis. (2016) 214:S482–7. doi: 10.1093/infdis/jiw284
13. Silva LA, Dermody TS. Chikungunya virus: epidemiology, replication, disease mechanisms, and prospective intervention strategies. J Clin Invest. (2017) 127:737–49. doi: 10.1172/JCI84417
14. van Duijl-Richter MK, Hoornweg TE, Rodenhuis-Zybert IA, Smit JM. Early events in chikungunya virus infection-from virus cell binding to membrane fusion. Viruses. (2015) 7:3647–74. doi: 10.3390/v7072792
15. Zaid A, Gerardin P, Taylor A, Mostafavi H, Malvy D, Mahalingam S. Chikungunya Arthritis: implications of acute and chronic inflammation mechanisms on disease management. Arthritis Rheumatol. (2018) 70:484–95. doi: 10.1002/art.40403
16. Anfasa F, Provacia L, GeurtsvanKessel C, Wever R, Gerstenbluth I, Osterhaus AD, et al. Hyperferritinemia is a potential marker of chronic chikungunya: A retrospective study on the Island of Curacao during the 2014-2015 outbreak. J Clin Virol. (2017) 86 31–8. doi: 10.1016/j.jcv.2016.11.003
17. Ramon-Pardo P, Cibrelus L, Yactayo S. Chikungunya: case definitions for acute, atypical and chronic cases. Genève: World Health Organization. (2015) p. 409–20.
18. Pybus OG, Rambaut A. Evolutionary analysis of the dynamics of viral infectious disease. Nat Rev Genet. (2009) 10:540–50. doi: 10.1038/nrg2583
19. Powers AM, Brault AC, Tesh RB, Weaver SC. Re-emergence of Chikungunya and O'nyong-nyong viruses: evidence for distinct geographical lineages and distant evolutionary relationships. J Gen Virol. (2000) 81:471–9. doi: 10.1099/0022-1317-81-2-471
20. Casal PE, Chouhy D, Bolatti EM, Perez GR, Stella EJ, Giri AA. Evidence for homologous recombination in Chikungunya Virus. Mol Phylogenet Evol. (2015) 85:68–75. doi: 10.1016/j.ympev.2015.01.016
21. Lee CY, Kam YW, Fric J, Malleret B, Koh EG, Prakash C, et al. Chikungunya virus neutralization antigens and direct cell-to-cell transmission are revealed by human antibody-escape mutants. PLoS Pathog. (2011) 7:e1002390. doi: 10.1371/journal.ppat.1002390
22. Kam YW, Lee WW, Simarmata D, Harjanto S, Teng TS, Tolou H, et al. Longitudinal analysis of the human antibody response to Chikungunya virus infection: implications for serodiagnosis and vaccine development. J Virol. (2012) 86:13005–15. doi: 10.1128/JVI.01780-12
23. Weger-Lucarelli J, Aliota MT, Kamlangdee A, Osorio JE. Identifying the role of E2 domains on alphavirus neutralization and protective immune responses. PLoS Negl Trop Dis. (2015) 9:e0004163. doi: 10.1371/journal.pntd.0004163
24. Masrinoul P, Puiprom O, Tanaka A, Kuwahara M, Chaichana P, Ikuta K, et al. Monoclonal antibody targeting chikungunya virus envelope 1 protein inhibits virus release. Virology. (2014) 464–465:111–7. doi: 10.1016/j.virol.2014.05.038
25. Sun S, Xiang Y, Akahata W, Holdaway H, Pal P, Zhang X, et al. Structural analyses at pseudo atomic resolution of Chikungunya virus and antibodies show mechanisms of neutralization. Elife. (2013) 2:e00435. doi: 10.7554/eLife.00435
26. Yoon IK, Alera MT, Lago CB, Tac-An IA, Villa D, Fernandez S, et al. High rate of subclinical chikungunya virus infection and association of neutralizing antibody with protection in a prospective cohort in the Philippines. PLoS Negl Trop Dis. (2015) 9:e0003764. doi: 10.1371/journal.pntd.0003764
27. Lum FM, Teo TH, Lee WW, Kam YW, Renia L, Ng LF. An essential role of antibodies in the control of Chikungunya virus infection. J Immunol. (2013) 190:6295–302. doi: 10.4049/jimmunol.1300304
28. Couderc T, Khandoudi N, Grandadam M, Visse C, Gangneux N, Bagot S, et al. Prophylaxis and therapy for Chikungunya virus infection. J Infect Dis. (2009) 200:516–23. doi: 10.1086/600381
29. Pal P, Dowd KA, Brien JD, Edeling MA, Gorlatov S, Johnson S, et al. Development of a highly protective combination monoclonal antibody therapy against Chikungunya virus. PLoS Pathog. (2013) 9:e1003312. doi: 10.1371/journal.ppat.1003312
30. Hawman DW, Stoermer KA, Montgomery SA, Pal P, Oko L, Diamond MS, et al. Chronic joint disease caused by persistent Chikungunya virus infection is controlled by the adaptive immune response J Virol. (2013) 87:13878–88. doi: 10.1128/JVI.02666-13
31. Selvarajah S, Sexton NR, Kahle KM, Fong RH, Mattia KA, Gardner J, et al. A neutralizing monoclonal antibody targeting the acid-sensitive region in chikungunya virus E2 protects from disease. PLoS Negl Trop Dis. (2013) 7:e2423. doi: 10.1371/journal.pntd.0002423
32. Sam IC, Chan YF, Chan SY, Loong SK, Chin HK, Hooi PS, et al. Chikungunya virus of Asian and Central/East African genotypes in Malaysia. J Clin Virol. (2009) 46 180–3. doi: 10.1016/j.jcv.2009.07.016
33. van den Doel P, Volz A, Roose JM, Sewbalaksing VD, Pijlman GP, van Middelkoop I, et al. Recombinant modified vaccinia virus Ankara expressing glycoprotein E2 of Chikungunya virus protects AG129 mice against lethal challenge. PLoS Negl Trop Dis. (2014) 8:e3101. doi: 10.1371/journal.pntd.0003101
34. Kam YW, Lum FM, Teo TH, Lee WW, Simarmata D, Harjanto S, et al. Early neutralizing IgG response to Chikungunya virus in infected patients targets a dominant linear epitope on the E2 glycoprotein. EMBO Mol Med. (2012) 4:330–43. doi: 10.1002/emmm.201200213
35. Goyal M, Chauhan A, Goyal V, Jaiswal N, Singh S, Singh M. Recent development in the strategies projected for chikungunya vaccine in humans. Drug Des Devel Ther. (2018) 12:4195–206. doi: 10.2147/DDDT.S181574
36. Partidos CD, Paykel J, Weger J, Borland EM, Powers AM, Seymour R, et al. Cross-protective immunity against o'nyong-nyong virus afforded by a novel recombinant chikungunya vaccine. Vaccine. (2012) 30:4638–43. doi: 10.1016/j.vaccine.2012.04.099
37. Langsjoen RM, Haller SL, Roy CJ, Vinet-Oliphant H, Bergren NA, Erasmus JH, et al. Chikungunya virus strains show lineage-specific variations in virulence and cross-protective ability in murine and nonhuman primate models. MBio. (2018) 9. doi: 10.1128/mBio.02449-17
38. Gardner J, Anraku I, Le TT, Larcher T, Major L, Roques P, et al. Chikungunya virus arthritis in adult wild-type mice. J Virol. (2010) 84:8021–32. doi: 10.1128/JVI.02603-09
39. Teo TH, Her Z, JTan JJ, Lum FM, Lee WW, Chan YH, et al. Caribbean and La Reunion Chikungunya Virus isolates differ in their capacity to induce proinflammatory Th1 and NK cell responses and acute joint pathology. J Virol. (2015) 89:7955–69. doi: 10.1128/JVI.00909-15
40. Hoarau JJ, Jaffar Bandjee MC, Krejbich Trotot P, Das T, Li-Pat-Yuen G, Dassa B, et al. Persistent chronic inflammation and infection by Chikungunya arthritogenic alphavirus in spite of a robust host immune response. J Immunol. (2010) 184:5914–27. doi: 10.4049/jimmunol.0900255
41. Dupuis-Maguiraga L, Noret M, Brun S, Le Grand R, Gras G, Roques P. Chikungunya disease: infection-associated markers from the acute to the chronic phase of arbovirus-induced arthralgia. PLoS Negl Trop Dis. (2012) 6:e1446. doi: 10.1371/journal.pntd.0001446
42. Dutta SK, Pal T, Saha B, Mandal S, Tripathi A. Copy number variation of Chikungunya ECSA virus with disease symptoms among Indian patients. J Med Virol. (2014) 86:1386–92. doi: 10.1002/jmv.23794
43. Chow A, Her Z, Ong EK, Chen JM, Dimatatac F, Kwek DJ, et al. Persistent arthralgia induced by Chikungunya virus infection is associated with interleukin-6 and granulocyte macrophage colony-stimulating factor. J Infect Dis. (2011) 203:149–57. doi: 10.1093/infdis/jiq042
44. Appassakij H, Khuntikij P, Kemapunmanus M, Wutthanarungsan R, Silpapojakul K. Viremic profiles in asymptomatic and symptomatic chikungunya fever: a blood transfusion threat? Transfusion. (2013) 53:2567–74. doi: 10.1111/j.1537-2995.2012.03960.x
45. Poo YS, Rudd PA, Gardner J, Wilson JA, Larcher T, Colle MA, et al. Multiple immune factors are involved in controlling acute and chronic chikungunya virus infection. PLoS Negl Trop Dis. (2014) 8:e3354. doi: 10.1371/journal.pntd.0003354
46. Hawman DW, Carpentier KS, Fox JM, May NA, Sanders W, Montgomery SA, et al. Mutations in the E2 glycoprotein and the 3′ Untranslated region enhance chikungunya virus virulence in mice. J Virol. (2017) 91. doi: 10.1128/JVI.00816-17
47. Chang AY, Martins KAO, Encinales L, Reid SP, Acuna M, Encinales C, et al. Chikungunya arthritis mechanisms in the Americas: a cross-sectional analysis of chikungunya arthritis patients twenty-two months after infection demonstrating no detectable viral persistence in synovial fluid. Arthritis Rheumatol. (2018) 70:585–93. doi: 10.1002/art.40383
48. Chaaitanya IK, Muruganandam N, Sundaram SG, Kawalekar O, Sugunan AP, Manimunda SP, et al. Role of proinflammatory cytokines and chemokines in chronic arthropathy in CHIKV infection. Viral Immunol. (2011) 24:265–71. doi: 10.1089/vim.2010.0123
49. Chen W, Foo SS, Sims NA, Herrero LJ, Walsh NC, Mahalingam S. Arthritogenic alphaviruses: new insights into arthritis and bone pathology. Trends Microbiol. (2015) 23:35–43. doi: 10.1016/j.tim.2014.09.005
50. Wauquier N, Becquart P, Nkoghe D, Padilla C, Ndjoyi-Mbiguino A, Leroy EM. The acute phase of Chikungunya virus infection in humans is associated with strong innate immunity and T CD8 cell activation. J Infect Dis. (2011) 204:115–23. doi: 10.1093/infdis/jiq006
51. Ng LF, Chow A, Sun YJ, Kwek DJ, Lim PL, Dimatatac F, et al. IL-1beta, IL-6, and RANTES as biomarkers of Chikungunya severity. PLoS ONE. (2009) 4:e4261. doi: 10.1371/journal.pone.0004261
52. Chopra A, Anuradha V, Ghorpade R, Saluja M. Acute Chikungunya and persistent musculoskeletal pain following the 2006 Indian epidemic: a 2-year prospective rural community study. Epidemiol Infect. (2012) 140:842–50. doi: 10.1017/S0950268811001300
53. Srirangan S, Choy EH. The role of interleukin 6 in the pathophysiology of rheumatoid arthritis. Ther Adv Musculoskelet Dis. (2010) 2:247–56. doi: 10.1177/1759720X10378372
54. Kelvin AA, Banner D, Silvi G, Moro ML, Spataro N, Gaibani P, et al. Inflammatory cytokine expression is associated with chikungunya virus resolution and symptom severity. PLoS Negl Trop Dis. (2011) 5:e1279. doi: 10.1371/journal.pntd.0001279
55. Teng TS, Kam YW, Lee B, Hapuarachchi HC, Wimal A, Ng LC, et al. A systematic meta-analysis of immune signatures in patients with acute Chikungunya virus infection. J Infect Dis. (2015) 211:1925–35. doi: 10.1093/infdis/jiv049
56. Ninla-Aesong P, Mitarnun W, Noipha K. Proinflammatory cytokines and chemokines as biomarkers of persistent arthralgia and severe disease after Chikungunya virus infection: A 5-year follow-up study in Southern Thailand. Viral Immunol. (2019) 32:442–52. doi: 10.1089/vim.2019.0064
57. Birgegard G, Hallgren R, Killander A, Stromberg A, Venge P, Wide L. Serum ferritin during infection. Scand J Haematol. (1978) 21:333–40. doi: 10.1111/j.1600-0609.1978.tb00374.x
58. Gozzelino R, Andrade BB, Larsen R, Luz NF, Vanoaica L, Seixas E, et al. Metabolic adaptation to tissue iron overload confers tolerance to malaria. Cell Host Microbe. (2012) 12:693–704. doi: 10.1016/j.chom.2012.10.011
59. Chaiyaratana W, Chuansumrit A, Atamasirikul K, Tangnararatchakit K. Serum ferritin levels in children with dengue infection. Southeast Asian J Trop Med Public Health. (2008) 39:832–6.
60. Tan LH, Lum LC, Omar SF, Kan FK. Hemophagocytosis in dengue: comprehensive report of six cases. J Clin Virol. (2012) 55:79–82. doi: 10.1016/j.jcv.2012.06.005
61. van de Weg CA, Huits RM, Pannuti CS, Brouns RM, van den Berg RW, van den Ham HJ, et al. Hyperferritinaemia in dengue virus infected patients is associated with immune activation and coagulation disturbances. PLoS Negl Trop Dis. (2014) 8:e3214. doi: 10.1371/journal.pntd.0003214
62. van de Veerdonk FL, Wever PC, Hermans MH, Fijnheer R, Joosten LA, van der Meer JW, et al. IL-18 serum concentration is markedly elevated in acute EBV infection and can serve as a marker for disease severity. J Infect Dis. (2012) 206:197-201. doi: 10.1093/infdis/jis335
63. Vecchiet J, Falasca K, Cacciatore P, Zingariello P, Dalessandro M, Marinopiccoli M, et al. Association between plasma interleukin-18 levels and liver injury in chronic hepatitis C virus infection and non-alcoholic fatty liver disease. Ann Clin Lab Sci. (2005) 35:415–22.
64. Wu J, Chen L, Chen Y, Yang J, Wu D. Serum ferritin concentration predicts mortality in patients with hepatitis B virus-related acute on chronic liver failure. Arch Med Res. (2014) 45:251–6. doi: 10.1016/j.arcmed.2014.03.004
65. Betancur JF, Navarro EP, Echeverry A, Moncada PA, Canas CA, Tobon GJ. Hyperferritinemic syndrome: Still's disease and catastrophic antiphospholipid syndrome triggered by fulminant Chikungunya infection: a case report of two patients. Clin Rheumatol. (2015) 34:1989–92. doi: 10.1007/s10067-015-3040-9
66. Duprez L, Wirawan E, Vanden Berghe T, Vandenabeele P. Major cell death pathways at a glance. Microbes Infect. (2009) 11:1050–62. doi: 10.1016/j.micinf.2009.08.013
67. Galluzzi L, Vitale I, Aaronson SA, Abrams JM, Adam D, Agostinis P, et al. Molecular mechanisms of cell death: recommendations of the Nomenclature Committee on Cell Death 2018. Cell Death Differ. (2018) 25:486–541. doi: 10.1038/s41418-017-0012-4
68. Nayak TK, Mamidi P, Kumar A, Singh LP, Sahoo SS, Chattopadhyay S, et al. Regulation of Viral Replication, Apoptosis and Pro-Inflammatory Responses by 17-AAG during Chikungunya Virus Infection in Macrophages. Viruses. (2017) 9. doi: 10.3390/v9010003
69. Dhanwani R, Khan M, Bhaskar AS, Singh R, Patro IK, Rao PV, et al. Characterization of Chikungunya virus infection in human neuroblastoma SH-SY5Y cells: role of apoptosis in neuronal cell death. Virus Res. (2012) 163:563–72. doi: 10.1016/j.virusres.2011.12.009
70. Joubert PE, Werneke SW, de la Calle C, Guivel-Benhassine F, Giodini A, Peduto L, et al. Chikungunya virus-induced autophagy delays caspase-dependent cell death. J Exp Med. (2012) 209:1029–47. doi: 10.1084/jem.20110996
71. Krejbich-Trotot P, Denizot M, Hoarau JJ, Jaffar-Bandjee MC, Das T, Gasque P. Chikungunya virus mobilizes the apoptotic machinery to invade host cell defenses. FASEB J. (2011) 25:314–25. doi: 10.1096/fj.10-164178
72. Joubert PE, Werneke SW, de la Calle C, Guivel-Benhassine F, Giodini A, Peduto L, et al. Chikungunya-induced cell death is limited by ER and oxidative stress-induced autophagy. Autophagy. (2012) 8:1261–3. doi: 10.4161/auto.20751
73. Camini FC, da Silva Caetano CC, Almeida LT, da Costa Guerra JF, de Mello Silva B, de Queiroz Silva S, et al. Oxidative stress in Mayaro virus infection. Virus Res. (2017) 236:1–8. doi: 10.1016/j.virusres.2017.04.017
74. Chopra A, Saluja M, Venugopalan A. Effectiveness of chloroquine and inflammatory cytokine response in patients with early persistent musculoskeletal pain and arthritis following chikungunya virus infection. Arthritis Rheumatol. (2014) 66:319–26. doi: 10.1002/art.38221
75. Lee P, Peng H, Gelbart T, Wang L, Beutler E. Regulation of hepcidin transcription by interleukin-1 and interleukin-6. Proc Natl Acad Sci U S A. (2005) 102:1906–10. doi: 10.1073/pnas.0409808102
76. Ryan JD, Altamura S, Devitt E, Mullins S, Lawless MW, Muckenthaler MU, et al. Pegylated interferon-alpha induced hypoferremia is associated with the immediate response to treatment in hepatitis C. Hepatology. (2012) 56:492–500. doi: 10.1002/hep.25666
77. Nemeth E, Tuttle MS, Powelson J, Vaughn MB, Donovan A, Ward DM, et al. Hepcidin regulates cellular iron efflux by binding to ferroportin and inducing its internalization. Science. (2004) 306:2090–3. doi: 10.1126/science.1104742
78. Kobune M, Kohgo Y, Kato J, Miyazaki E, Niitsu Y. Interleukin-6 enhances hepatic transferrin uptake and ferritin expression in rats. Hepatology. (1994) 19:1468–75. doi: 10.1002/hep.1840190623
79. Banerjee N, Saha B, Mukhopadhyay S. Intracellular ROS generated in chikungunya patients with persisting polyarthralgia can be reduced by Tinospora cordifolia leaf extract. Virus Dis. (2018) 29:375–9. doi: 10.1007/s13337-018-0465-1
80. Silva I, Rausch V, Peccerella T, Millonig G, Seitz HK, Mueller S. Hypoxia enhances H2O2-mediated upregulation of hepcidin: Evidence for NOX4-mediated iron regulation. Redox Biol. (2018) 16:1–10. doi: 10.1016/j.redox.2018.02.005
81. Smith JM, Heese A. Rapid bioassay to measure early reactive oxygen species production in Arabidopsis leave tissue in response to living Pseudomonas syringae. Plant Methods. (2014) 10:6. doi: 10.1186/1746-4811-10-6
82. McCormick AL, Roback L, Wynn G, Mocarski ES. Multiplicity-dependent activation of a serine protease-dependent cytomegalovirus-associated programmed cell death pathway. Virology. (2013) 435:250–7. doi: 10.1016/j.virol.2012.08.042
83. Gatica D, Lahiri V, Klionsky DJ. Cargo recognition and degradation by selective autophagy. Nat Cell Biol. (2018) 20:233–42. doi: 10.1038/s41556-018-0037-z
84. Feng H, Stockwell BR. Unsolved mysteries: How does lipid peroxidation cause ferroptosis? PLoS Biol. (2018) 16:e2006203. doi: 10.1371/journal.pbio.2006203
85. Da Rocha Araujo FAG. Chikungunya arthritis: an emerging rheumatologic problem. Int J Clin Rheumatol. (2018) 13. doi: 10.4172/1758-4272.1000190
86. Epelboin L, Bidaud B, Mosnier E, Le Turnier P, Vesin G, Walter G, et al. Fatal case of chikungunya and concomitant thrombotic thrombocytopenic purpura in French Guiana during air flight medical evacuation. J Travel Med. (2017) 24. doi: 10.1093/jtm/tax028
87. Torres JR, Leopoldo Codova G, Castro JS, Rodriguez L, Saravia V, Arvelaez J, et al. Chikungunya fever: Atypical and lethal cases in the Western hemisphere: A Venezuelan experience. IDCases. (2015) 2:6–10. doi: 10.1016/j.idcr.2014.12.002
88. Couderc T, Lecuit M. Focus on Chikungunya pathophysiology in human and animal models. Microbes Infect. (2009) 11:1197–205. doi: 10.1016/j.micinf.2009.09.002
89. Metz SW, Martina BE, van den Doel P, Geertsema C, Osterhaus AD, Vlak JM, et al. Chikungunya virus-like particles are more immunogenic in a lethal AG129 mouse model compared to glycoprotein E1 or E2 subunits. Vaccine. (2013) 31:6092–6. doi: 10.1016/j.vaccine.2013.09.045
90. Chattopadhyay A, Aguilar PV, Bopp NE, Yarovinsky TO, Weaver SC, Rose JK. A recombinant virus vaccine that protects against both Chikungunya and Zika virus infections. Vaccine. (2018) 36:3894–900. doi: 10.1016/j.vaccine.2018.05.095
91. Wang E, Kim DY, Weaver SC, Frolov I. Chimeric Chikungunya viruses are nonpathogenic in highly sensitive mouse models but efficiently induce a protective immune response. J Virol. (2011) 85:9249–52. doi: 10.1128/JVI.00844-11
92. Bao H, Ramanathan AA, Kawalakar O, Sundaram SG, Tingey C, Bian CB, et al. Nonstructural protein 2 (nsP2) of Chikungunya virus (CHIKV) enhances protective immunity mediated by a CHIKV envelope protein expressing DNA Vaccine. Viral Immunol. (2013) 26:75–83. doi: 10.1089/vim.2012.0061
93. Plante K, Wang E, Partidos CD, Weger J, Gorchakov R, Tsetsarkin K, et al. Novel chikungunya vaccine candidate with an IRES-based attenuation and host range alteration mechanism. PLoS Pathog. (2011) 7:e1002142. doi: 10.1371/journal.ppat.1002142
94. Wang E, Volkova E, Adams AP, Forrester N, Xiao SY, Frolov I, et al. Chimeric alphavirus vaccine candidates for chikungunya. Vaccine. (2008) 26:5030–9. doi: 10.1016/j.vaccine.2008.07.054
95. Mallilankaraman K, Shedlock DJ, Bao H, Kawalekar OU, Fagone P, Ramanathan AA, et al. A DNA vaccine against chikungunya virus is protective in mice and induces neutralizing antibodies in mice and nonhuman primates. PLoS Negl Trop Dis. (2011) 5:e928. doi: 10.1371/journal.pntd.0000928
96. Saraswat S, Athmaram TN, Parida M, Agarwal A, Saha A, Dash PK. Expression and characterization of yeast derived Chikungunya virus like particles (CHIK-VLPs) and its evaluation as a potential vaccine candidate. PLoS Negl Trop Dis. (2016) 10:e0004782. doi: 10.1371/journal.pntd.0004782
97. Brandler S, Ruffie C, Combredet C, Brault JB, Najburg V, Prevost MC, et al. A recombinant measles vaccine expressing chikungunya virus-like particles is strongly immunogenic and protects mice from lethal challenge with chikungunya virus. Vaccine. (2013) 31:3718–25. doi: 10.1016/j.vaccine.2013.05.086
98. Piper A, Ribeiro M, Smith KM, Briggs CM, Huitt E, Nanda K, et al. Chikungunya virus host range E2 transmembrane deletion mutants induce protective immunity against challenge in C57BL/6J mice. J Virol. (2013) 87:6748–57. doi: 10.1128/JVI.03357-12
99. Chattopadhyay A, Wang E, Seymour R, Weaver SC, Rose JK. A chimeric vesiculo/alphavirus is an effective alphavirus vaccine. J Virol. (2013) 87:395–402. doi: 10.1128/JVI.01860-12
100. Garcia-Arriaza J, Cepeda V, Hallengard D, Sorzano CO, Kummerer BM, Liljestrom P, et al. A novel poxvirus-based vaccine, MVA-CHIKV, is highly immunogenic and protects mice against chikungunya infection. J Virol. (2014) 88:3527–47. doi: 10.1128/JVI.03418-13
101. Hallengard D, Kakoulidou M, Lulla A, Kummerer BM, Johansson DX, Mutso M, et al. Novel attenuated Chikungunya vaccine candidates elicit protective immunity in C57BL/6 mice. J Virol. (2014) 88:2858–66. doi: 10.1128/JVI.03453-13
102. Prow NA, Liu L, Nakayama E, Cooper TH, Yan K, Eldi P, et al. A vaccinia-based single vector construct multi-pathogen vaccine protects against both Zika and chikungunya viruses. Nat Commun. (2018) 9:1230. doi: 10.1038/s41467-018-03662-6
103. Wang D, Suhrbier A, Penn-Nicholson A, Woraratanadharm J, Gardner J, Luo M, et al. A complex adenovirus vaccine against chikungunya virus provides complete protection against viraemia and arthritis. Vaccine. (2011) 29:2803–9. doi: 10.1016/j.vaccine.2011.01.108
104. Erasmus JH, Auguste AJ, Kaelber JT, Luo H, Rossi SL, Fenton K, et al. A chikungunya fever vaccine utilizing an insect-specific virus platform. Nat Med. (2017) 23:192–9. doi: 10.1038/nm.4253
105. Weger-Lucarelli J, Chu H, Aliota MT, Partidos CD, Osorio JE. A novel MVA vectored Chikungunya virus vaccine elicits protective immunity in mice. PLoS Negl Trop Dis. (2014) 8:e2970. doi: 10.1371/journal.pntd.0002970
106. Kumar M, Sudeep AB, Arankalle VA. Evaluation of recombinant E2 protein-based and whole-virus inactivated candidate vaccines against chikungunya virus. Vaccine. (2012) 30:6142–9. doi: 10.1016/j.vaccine.2012.07.072
107. Weber C, Buchner SM, Schnierle BS. A small antigenic determinant of the Chikungunya virus E2 protein is sufficient to induce neutralizing antibodies which are partially protective in mice. PLoS Negl Trop Dis. (2015) 9:e0003684. doi: 10.1371/journal.pntd.0003684
108. Tretyakova I, Hearn J, Wang E, Weaver S, Pushko P. DNA vaccine initiates replication of live attenuated chikungunya virus in vitro and elicits protective immune response in mice. J Infect Dis. (2014) 209:1882–90. doi: 10.1093/infdis/jiu114
109. Akahata W, Yang ZY, Andersen H, Sun S, Holdaway HA, Kong WP, et al. A virus-like particle vaccine for epidemic Chikungunya virus protects nonhuman primates against infection. Nat Med. (2010) 16:334–8. doi: 10.1038/nm.2105
110. Fraisier C, Koraka P, Belghazi M, Bakli M, Granjeaud S, Pophillat M, et al. Kinetic analysis of mouse brain proteome alterations following Chikungunya virus infection before and after appearance of clinical symptoms. PLoS ONE. (2014) 9:e91397. doi: 10.1371/journal.pone.0091397
111. Couderc T, Chretien F, Schilte C, Disson O, Brigitte M, Guivel-Benhassine F, et al. A mouse model for Chikungunya: young age and inefficient type-I interferon signaling are risk factors for severe disease. PLoS Pathog. (2008) 4:e29. doi: 10.1371/journal.ppat.0040029
112. Taylor A, Liu X, Zaid A, Goh LY, Hobson-Peters J, Hall RA, et al. Mutation of the N-terminal region of Chikungunya virus capsid protein: implications for vaccine design. MBio. (2017) 8. doi: 10.1128/mBio.01970-16
113. Morrison TE, Oko L, Montgomery SA, Whitmore AC, Lotstein AR, Gunn BM, et al. A mouse model of chikungunya virus-induced musculoskeletal inflammatory disease: evidence of arthritis, tenosynovitis, myositis, and persistence. Am J Pathol. (2011) 178:32–40. doi: 10.1016/j.ajpath.2010.11.018
114. Dagley A, Ennis J, Turner JD, Rood KA, Van Wettere AJ, Gowen BB, et al. Protection against Chikungunya virus induced arthralgia following prophylactic treatment with adenovirus vectored interferon (mDEF201). Antiviral Res. (2014) 108:1–9. doi: 10.1016/j.antiviral.2014.05.004
115. Green K, Armstrong JS. Structured analogies for forecasting. Int J Forecasting. (2007) 23:365–76. doi: 10.1016/j.ijforecast.2007.05.005
116. Partidos CD, Weger J, Brewoo J R, Seymour EM, Borland JP, Ledermann AM, et al. Probing the attenuation and protective efficacy of a candidate chikungunya virus vaccine in mice with compromised interferon (IFN) signaling. Vaccine. (2011) 29:3067–73. doi: 10.1016/j.vaccine.2011.01.076
117. Ngwe Tun MM, Muthugala R, Kyaw Kyaw A, Shimada S, Morita K, Hayasaka D. Pathogenetic potential relating to metabolic activity in a mouse model of infection with the Chikungunya virus East/Central/South African Genotype. Viruses. (2020) 12. doi: 10.3390/v12020169
118. Ledermann JP, Borland EM, Powers AM. Minimum infectious dose for chikungunya virus in Aedes aegypti and Ae. albopictus mosquitoes. Rev Panam Salud Publica. (2017) 41:e65. doi: 10.26633/RPSP.2017.65
119. Dittmer U, Brooks DM, Hasenkrug KJ. Protection against establishment of retroviral persistence by vaccination with a live attenuated virus. J Virol. (1999) 73:3753–7. doi: 10.1128/JVI.73.5.3753-3757.1999
120. Dutta A, Huang CT, Lin CY, Chen TC, Lin YC, Chang CS, et al. Sterilizing immunity to influenza virus infection requires local antigen-specific T cell response in the lungs. Sci Rep. (2016) 6:32973. doi: 10.1038/srep32973
Keywords: chikungunya virus, vaccine, efficacy, IL-6 (Interleukin 6), ferritin, preclinical model, viremia
Citation: Lim SM, Dutta SK and Martina BEE (2021) Defining Efficacy of Chikungunya Virus Candidate Vaccines: Different Endpoints Derived From the Virus—Cytokine—Ferritin (VCF) Model. Front. Virol. 1:693439. doi: 10.3389/fviro.2021.693439
Received: 10 April 2021; Accepted: 26 July 2021;
Published: 23 August 2021.
Edited by:
Siew Pheng Lim, Denka Life Innovation Research (DLIR), SingaporeReviewed by:
Mariah Hassert, Saint Louis University, United StatesBo Zhang, Wuhan Institute of Virology (CAS), China
Copyright © 2021 Lim, Dutta and Martina. This is an open-access article distributed under the terms of the Creative Commons Attribution License (CC BY). The use, distribution or reproduction in other forums is permitted, provided the original author(s) and the copyright owner(s) are credited and that the original publication in this journal is cited, in accordance with accepted academic practice. No use, distribution or reproduction is permitted which does not comply with these terms.
*Correspondence: Byron E. E. Martina, Yi5tYXJ0aW5hQGFydGVtaXMtYmlvc3VwcG9ydC5jb20=