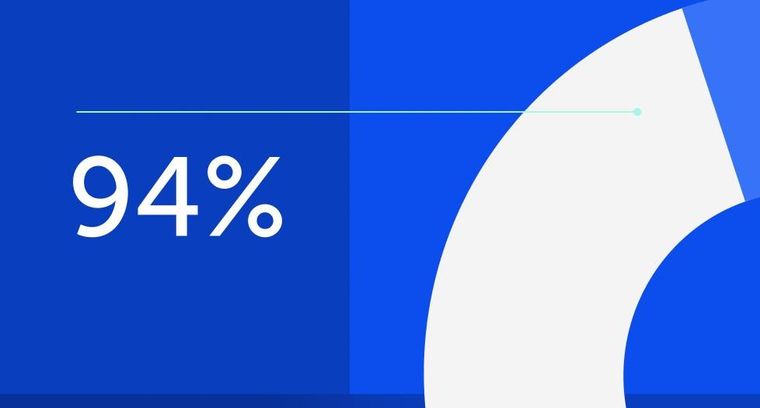
94% of researchers rate our articles as excellent or good
Learn more about the work of our research integrity team to safeguard the quality of each article we publish.
Find out more
ORIGINAL RESEARCH article
Front. Vet. Sci., 06 January 2025
Sec. Animal Nutrition and Metabolism
Volume 11 - 2024 | https://doi.org/10.3389/fvets.2024.1514952
This article is part of the Research TopicNatural Compounds/Products and Livestock Productivity: Enhancing Antioxidant Levels, Gut Health, Mitigating Greenhouse Gas Emissions, and Disease ControlView all 28 articles
Introduction: In ruminants, a symbiotic rumen microbiota is responsible for supporting the digestion of dietary fiber and contributes to health traits closely associated with meat and milk quality. A holistic view of the physicochemical profiles of mixed rumen microbiota (MRM) is not well-illustrated.
Methods: The experiment was performed with a 3 × 4 factorial arrangement of the specific surface area (SSA: 3.37, 3.73, and 4.44 m2/g) of NDF extracted from rice straw and the surface tension (ST: 54, 46, 43, and 36 dyn/cm) of a fermented medium in a fermentation time series of 6, 12, 24, 48 h with three experimental units. Here, we used three rumen-fistulated adult Liuyang black goats as the rumen liquid donors for this experiment.
Results: It was found that increasing SSA decreased the average acetate/propionate ratio (A/P, p < 0.05) and increased the molarity of propionate (p < 0.05). Increasing ST decreased total volatile fatty acid (tVFA) concentration (p < 0.01). Greater SSA increased (p < 0.01) MRM hydrophobicity, whereas increasing ST increased MRM cell membrane permeability (p < 0.01). The neutral detergent fiber digestibility (NDFD, r = 0.937) and tVFA (r = 0.809) were positively correlated with the membrane permeability of MRM.
Discussion: The surface tension of the artificial medium and substrate-specific surface area had a significant influence on MRM's fermentation profiles, hydrophobicity, and permeability. The results suggest that physical environmental properties are key in regulating rumen fermentation function and homeostasis in the gastrointestinal tract ecosystem.
Enzyme activity and microbes' adhesion to the substrate are directly related to the physical characteristics of the substrate and rumen fluid. We measured the in vitro fermentation of neutral detergent fiber and the surface physical properties of microbes under the different specific surface areas of the neutral detergent fiber and surface tension of the culture medium. This findings indicate that rumen fermentation and animal production can be improved by modifying the physical characteristics of the substrate and rumen fluid.
For ruminants, the rumen microbiota is responsible for degrading indigestible fibers into energy and nutrients (1). The ability of the microbiota to digest fiber is enhanced when they transform from planktonic to irreversible adhesion model (2). Microbial biofilms are the prerequisite for fiber digestion and microbial adhesion (3). Microbial biofilms are multicellular microbial communities formed after planktonic cells adhere to solid surfaces (4). The transition from a free-living planktonic lifestyle to a sessile, attached state, forming biofilms, is a multifactorial process governed by biological, chemical, and physical properties of the environment, surface, and bacterial cell (5). Bacterial biofilm is a matrix of extracellular polymeric substances (EPS) (6) that provide mechanical stability and protection against environmental adversities (7). The biofilm is influenced by the availability of environmental nutrients and some nucleotide second messengers, such as cyclic adenosine monophosphate (cAMP) and cyclic di-guanosine monophosphate (c-di-GMP) through cAMP receptor protein (CRP) signaling (8).
The initial bacterial adhesion process is influenced by the properties of the material surface, including surface roughness, topography, wettability, and stiffness (7, 9, 10). The structured channels within the biofilm (3) facilitate the exchange of nutrients between the embedded microbes and the external environments, which contribute to microbial colonization (11) and quorum sensing (12). Specific surface area (SSA) is a crucial parameter in quantifying interactive functions at liquid-solid/gas interfaces, especially in the case of adsorption, heterogeneous catalysis (13), and reaction at the surface of materials (14, 15). Feed particles with greater SSA provide more adsorption sites, resulting in higher fiber digestion efficiency (7). Bacterial attachment and biofilm formation on substrate surfaces highly depend on the available surface area (16, 17). However, comprehensive data linking SSA with microbial adhesion and biofilm formation are still lacking, representing a significant knowledge gap.
Surface tension (ST) of the media also plays a critical role in microbial attachment abilities and the adhesion process, such as its superhydrophobic or super-hydrophilic properties (7, 18). This study is novel in its approach to systematically evaluating the combined effects of SSA and ST on the physical properties of the rumen microbiota and fermentation profiles, an area that has not been extensively explored. The interaction between these factors and their influence on microbial cell membrane permeability, hydrophobicity, and fermentation efficiency remain poorly understood, despite their potential to optimize microbial activity and feed digestibility.
From an economic perspective, improving the efficiency of rumen fermentation is crucial for sustainable livestock production (19). Feed costs account for up to 70% of total expenses in ruminant farming (20). Enhancing fiber digestibility through physicochemical modifications to feed properties offers a cost-effective strategy to maximize feed utilization and minimize waste (21). Additionally, optimizing fermentation conditions, such as reducing ST, can further improve microbial efficiency, leading to higher productivity and lower feed costs (22, 23). These strategies are particularly valuable in the context of increasing global demand for livestock products and the need for sustainable agricultural practices.
This study aims to address these gaps by employing a factorial experimental design to evaluate how varying SSA and ST levels influence the physical and chemical properties of rumen microbes, as well as their fermentation capabilities. By integrating these physicochemical parameters, our findings offer actionable strategies for improving feed efficiency, livestock productivity, and environmental sustainability (24, 25). Additionally, the strong correlations observed between microbial cell membrane permeability and key fermentation metrics, such as fiber digestibility and volatile fatty acid (VFA) production, provide a mechanistic understanding that could guide future advancements in ruminant nutrition and biotechnology.
An in-depth understanding of the physical properties of the environmental surface on fermentation kinetics and physicochemical properties of the rumen microbiota could be conducive to regulating the fiber degradation process. Therefore, this study was conducted to investigate the effects of ST of the fermentation inoculum and SSA of the substrate on the physical properties of the rumen microbes and the consequences of fiber degradation.
The experiment consisted of a series of in vitro batch cultures performed as a completely randomized experimental design with 12 treatments in a 3 × 4 factorial arrangement, each comprising three individual runs. The present work used three SSAs of neutral detergent fiber (NDF) (3.37, 3.73, and 4.44 m2/g) and four STs (36, 43, 46, and 54 dyn/cm) of the incubation medium. Another run was performed on separate days using the same SSA preparations. The new ST was similar to the previous run and was prepared with fresh rumen inoculum collected from three experimental goats at a similar ratio. The experiment was approved by the Animal Care Committee of the Institute of Subtropical Agriculture, Chinese Academy of Sciences, Changsha, Hunan (No. ISA-2012-018).
In the present work, animal welfare and management strategies of rumen inoculum donors were described in our previous work (23, 26). Briefly, three rumen-fistulated adult black Liuyang goats of similar age, weight, and good health status were used to provide equal rumen fluids of about 150 ml rumen liquid of each donor for in vitro fermentation to reveal the physical characteristics of ST and SSA. The in vitro fermentation procedure and chemical compositions of the buffer medium were explained in our previous publications (15, 27). About 50 mL of fermentation medium at a ratio of 1:2 (v/v, rumen fluid: buffer medium) with 4 ST properties, combined with 500 ± 50 mg neutral detergent fiber (NDF) of rice straw (3 SSA levels) at 39°C under continuous flushing with CO2, was performed in a time series of 6, 12, 24, and 48 h to collect fermented microbial samples. The fermented samples were used to determine volatile fatty acid (VFA) concentrations and physical properties (zeta potential, also called electrokinetic potential, hydrophobicity, and cell membrane permeability) of the microbiota in the rumen. The diet for goats consisted of a concentrate (corn 47%, soybean meal 24%, wheat bran 22%, salt 0.77%, limestone powder 2.23%, and premix 4%) and forage (corn stover) in a ratio of 40:60. The goats were provided free access to water and were fed twice daily at 08:00 and 18:00, receiving 200 g of concentrate and 300 g of forage per day.
The NDF resource was derived from rice straw, while the surface tension system was constructed as described in our previous work (23). Briefly, 3 SSAs (3.37, 3.73, and 4.44 m2/g) of NDF were ground, sieved through a mill with different sieve pore sizes, and measured using a surface area analyzer (Quadrasorb-SI, Quantachrome Inc. Florida, CA, USA). Four STs (36, 43, 46, and 54 dynes/cm) of inoculum medium were prepared by adding alkyl polyglucoside (APG, 28.7 dynes/cm) and immediately measured using a model K100 tensiometer (KRÜSS GmbH, Hamburg, Germany).
The mixed fermentation samples, consisting of a mixture of fermentation substrate and microbes, were collected at a fermentation time series of 6, 12, 24, and 48 h. The mixed fermentation samples of 2, 3, and 2 mL of supernatant were collected by centrifugation (5,000 × g at 4°C for 10 min to remove the substrate) to analyze the zeta potential (ξ), cell membrane permeability, and hydrophobicity of the mixed rumen microbes, respectively. To measure the mixed rumen microbiota's physical properties, we followed our previous work's detailed descriptions (15, 23, 28).
The microbial pellet was prepared by rinsing and resuspending the collected residue in 5 mL of 1 mM KNO3 (pH 6.6) as described by Pelletier et al. (29) and determined using a zeta potential analyzer (Brookhaven Instruments Corp., Holtsville, NY, USA) equipped with a He-Ne laser (658.0 nm) as the light source in a high-precision system (30 cycles) at 39°C.
Three milliliter of supernatant was mixed with 1 mL of 100 mg/L fluorescein isothiocyanate-dextran (FITC-dextran, Sigma, molecular weight ≈ 38 kDa) solution and incubated for 1 h at 37°C and then centrifuged to collect 2 mL of sub-supernatant for fluorescence intensity determination (15, 30). The blank negative control was prepared using 3 mL supernatant and 1 mL distilled water.
The microbiota pellet from each fermented flask was centrifuged, collected, rinsed, homogenized, and resuspended in 6 mL of 0.1 M KNO3 phosphate-buffered saline (PBS) solution (pH 6.6) as described in previous reports (23, 31). The hydrophobicity and permeability of the microbial cell membrane were calculated according to Equations 1, 2 (23), respectively.
Hydrophobicity was defined as the difference between the percentage of cells retained by the hydrocarbon and aqueous layers. Ha0 represents the absorbance value of optical density (OD) value at 400 nm, OD400, before the addition of hexadecane, and Ha1 represents the absorbance value of OD400 after hexadecane addition. Pa0 represents the absorbance value of 25 mg/L FITC-dextran rumen microbe-free liquid, and Pa1 represents the incubated microbe-free liquid absorbance value.
The concentration of VFA in the fermentation liquid was determined according to our previous report (28). Two milliliter of fermented liquid was centrifuged, collected, immediately mixed, and homogenized with 0.15 mL metaphosphoric acid (conc. 25%). The sub-supernatant was then centrifuged and collected for analysis of VFA concentrations at incubation time series of 6, 12, 24, and 48 h by gas chromatography (HP5890, Agilent 5890; Agilent Technologies Co. Ltd, USA).
Statistical analysis of a completely randomized experimental design was performed using the MIXED procedure of SAS software (32). For VFA and surface physical variables, the SSA, ST, time series, and interactions were included in the model as fixed effects, while the run was used as a random effect and incubation time as a repeated effect. The SLICE statement of SAS software was used to detect differences between means at specific SSA levels, and the pairwise difference (PDIFF) statement of SAS software was used to compare ST effects within each SSA level. Pearson correlation analysis was performed using the Proc Corr procedure of SAS (32), and r > 0.5 was considered biologically significant. Least-squares means are given throughout the text, and significance was reported as P ≤ 0.05.
The propionate was affected by SSA, which increased with increasing SSA (P < 0.05) (Table 1). In addition, the ratio of acetate to propionate decreased with increasing SSA (P < 0.05). The tVFA and isovalerate decreased (P < 0.01), but propionate increased (P < 0.01) with increasing ST. Interactive effects for NDFD, tVFA, and individual VFA were not observed between SSA and ST. A similar interaction for tVFA (Figure 1A), butyrate (Figure 1B), and isobutyrate (Figure 1C) was observed between time and SSA. Valerate and isovalerate increased with increasing time (SSA = 4.44 m2/g), whereas they decreased after a fermentation time longer than 24 h (SSA = 3.37 and 3.73 m2/g, respectively) (SSA × time interaction, P < 0.05; Figures 1D, E). In addition, isobutyrate and valerate increased with fermentation time as a function of ST of the fermentation liquid (ST × time interaction, P < 0.05 for isobutyrate, Figure 1D, and ST × time interaction, P < 0.01 for isobutyrate, Figure 1F).
Figure 1. The SSA or ST interacted with fermentation time on VFA profiles, tVFA (A), butyrate (B), isobutyrate (C), isovalerate (D), and valerate (E, F).
Zeta potential (ξ) changed with increasing incubation time (P < 0.01), and the effects of incubation time on ξ depended on the SSA of the substrate (SSA × time interaction, P < 0.05) (Table 2). At SSA = 3.37 m2/g, the increasing and decreasing magnitude of ξ from 12 to 24 h and 24 to 48 h, respectively, was greater than that of SSA = 3.73 m2/g (Figure 2A).
Table 2. Combinatorial interactions of SSA, ST, and incubation time on the physicochemical properties of rumen microbes in vitro.
Figure 2. The SSA or ST interacted with fermentation time on cell membrane permeability of rumen microbes, Zeta potential (A), cell surface hydrophobicity (B), and membrane permeability (C, D).
The hydrophobicity of the cell membrane of mixed rumen bacteria increased (P < 0.01) with increasing SSA of NDF. The effect of incubation time on hydrophobicity depended on the SSA of the substrate (SSA × time interaction, P < 0.05). The hydrophobicity of the cell surface of rumen bacteria decreased with increasing fermentation time (SSA = 3.37 and 3.73 m2/g), whereas it increased at 24 h (SSA = 4.44 m2/g) (Figure 2B).
Cell membrane permeability was higher (P < 0.01) for ST = 43 and 46 dynes/cm compared with ST = 36 dynes/cm. The effects of incubation time on cell membrane permeability were dependent on the SSA (SSA × time interactions, P < 0.05) and ST (ST × time interactions, P < 0.001). The increase in cell membrane permeability was greater for SSA = 4.44 m2/g (P < 0.05) than for SSA = 3.37 m2/g when the incubation time increased from 12 to 24 hours (Figure 2C). For ST = 36 dynes/cm, cell membrane permeability decreased with incubation time from 6 to 12 h, whereas it increased numerically for ST = 43 dynes/cm, and increased (P < 0.01) sharply with increasing fermentation time from 12 to 24 h than for ST = 36 dynes/cm (Figure 2D).
The correlation between cell membrane permeability of the microbiota was calculated, and the important fermentation value indices, NDFD, and tVFA (Figure 3). A high correlation (r = 0.937, P < 0.001) was found between permeability and NDFD. In addition, a high correlation (r = 0.809, P < 0.001) was established between microbiota permeability and tVFA concentration between all treatments and incubation times.
Figure 3. Correlations between bacteria cell membrane permeability and (A) neutral detergent fiber digestibility (NDFD, r = 0.937, P < 0.001), and (B) total volatile fatty acids (tVFA, r = 0.809, P < 0.001) among all the treatments and incubation times.
Volatile fatty acids (VFAs) are not only the primary end-products of carbohydrate metabolism in the rumen but also play critical roles in host energy metabolism and microbial homeostasis (33). The observed increase in tVFA with reduced surface tension suggests that the physicochemical properties of the fermentation medium can significantly influence microbial metabolic efficiency (17, 19). Reduced ST with nonionic surfactants or biosurfactants may enhance microbial interaction with substrates, promoting enzymatic action (34, 35). This finding aligns with studies demonstrating that microbial surfactants lower interfacial tension to improve fermentation efficiency (22, 36).
The increased cell membrane permeability observed with lower ST values is consistent with findings that nonionic surfactants enhance microbial membrane fluidity, facilitating metabolite exchange and enzymatic leakage (15, 37). This mechanism allows extracellular enzymes to act more effectively on substrates, supporting the breakdown of complex carbohydrates (38, 39). The extracellular enzymatic activity of laccase, lignin peroxidase, and manganese peroxidase of Phanerochaete chrysosporium was increased with increasing permeability under the electronic field (30). Gram-negative bacteria are surrounded by an outer membrane consisting of a complex arrangement of glycerolphospholipids (GPL) (40). Their main function is to serve as a permeability barrier to allow the influx of essential nutrients while excluding harmful compounds, such as antibiotics and antimicrobial peptides (41, 42). There are strong positive correlations between cell membrane permeability and NDFD or tVFA. The high linear co-relationship between cell membrane permeability and fermentation parameters, such as NDFD (r = 0.937) and tVFA production (r = 0.809), underscore the critical role of permeability in microbial fermentation dynamics (15). Theoretical models suggest that optimal membrane permeability supports both nutrient uptake and the secretion of secondary metabolites, such as VFAs. This dual role highlights the centrality of permeability in microbial ecosystem stability and efficiency (43, 44). In general, the functions of signal transduction, secretion of active substances, nutrient absorption, metabolite excretion, and biological membrane function of the microbiota are influenced by cell membrane permeability (45), which in turn is related to the zeta potential and hydrophobicity properties of the membrane (46).
SSA significantly influenced microbial hydrophobicity, which is essential for microbial adhesion to feed particles and biofilm formation. Increased SSA provides more adsorption sites, enhancing microbial colonization and fiber degradation efficiency. These results are consistent with earlier findings showing that hydrophobicity promotes microbial adhesion, an essential precursor to effective fermentation.
Cell adhesion of microbes to the substrate, which is a prerequisite for bacterial colonization and proliferation, is influenced by cell surface hydrophobicity (47, 48). Higher hydrophobic strains have stronger adhesion ability (49, 50). We reported that hydrophobicity increased with increasing SSA, and higher hydrophobicity occurred in the first fermentation phage. We hypothesize that higher SSA increases the activity and adhesiveness of the microbiota in the rumen by altering its surface hydrophobicity. Interestingly, while SSA had a pronounced effect on microbial hydrophobicity, ST did not significantly alter this property. This observation underscores the dominant role of substrate characteristics in influencing microbial adhesion, emphasizing the importance of feed preparation in optimizing ruminal fermentation. Surface hydrophobicity was not affected by the ST of the medium. The lower hydrophobicity of the cell surface was evident during the incubation period. In previous studies, rumen microbes' hydrophobicity was related to bacterial aging, and a high value was found in freshly isolated strains of Staphylococcus aureus or Serratia spp. (51, 52). Given the complexity of the rumen microecosystem, further studies are needed to explain the relationships between the surface properties of the rumen microbiota and the adhesion process, microbial proliferation, and microbial enzyme secretion.
Zeta potential (ξ), a measure of surface charge, reflects the electrostatic interactions governing microbial and dispersion. In this study, ξ remained unaffected by ST or SSA, except for temporal changes. The reduction in ξ after 24 h may result from microbial aggregation and the accumulation of dead cells, which alter surface charge distribution (53). Although ξ showed a minimal direct correlation with membrane permeability, its role in maintaining microbial dispersion and biofilm integrity is essential (54, 55). In addition, the surface charge of bacteria is also influenced by the growth medium, bacterial phages, and bacterial surface structure (56). Membrane permeability can be directly affected by membrane zeta potential through a class of special channels known as voltage-gated ion channels or voltage-dependent ion channels, such as sodium/calcium/channel proteins (α-helical transmembrane segments, S1-S6) with a particular ion selectivity and voltage dependence (57, 58). The cell surface of the rumen microbiota was negatively charged, referred to as a net negative charge (56). The negative charges of the membranes increased with increasing fermentation time up to 24 h and decreased the resistance of the microbe attached to the substrate (59, 60). This indicates that the main adhesion of rumen microbes to the substrate occurred before 24 h.
The interplay between hydrophobicity, membrane permeability, and zeta potential provides a comprehensive understanding of microbial responses to environmental changes (48). Manipulating SSA and ST can selectively enhance desirable microbial properties, such as adhesion (61, 62) and enzyme secretion while mitigating inhibitory factors like excessive aggregation (63, 64). These findings align with current research strategies to tailor fermentation environments for optimized microbial activity (10, 65).
This study highlights actionable strategies for improving feed formulation in livestock nutrition. Increasing SSA through particle processing or additive application enhances microbial adhesion and fiber degradation, while moderate reductions in ST using bio-/nonionic- surfactants or other agents optimize enzymatic activity. These approaches can significantly enhance feed efficiency, reduce waste, and promote sustainable livestock production. Future studies should explore the molecular mechanisms underlying the observed effects using advanced omics technologies. Metagenomics and metabolomics can provide deeper insights into how SSA and ST influence microbial community composition and metabolic pathways, paving the way for precision management of ruminal fermentation.
In the rumen, both the large specific surface area of the fiber and the low surface tension of the inoculum result in increased propionate production and decreased acetate-to-propionate ratios by disrupting the microbiome ecosystem. Cell surface properties of rumen microbes, including hydrophobicity and permeability, change with substrate properties and interfacial properties of the medium. In addition, neutral detergent fiber digestibility and total volatile fatty acid correlate strongly with the cell membrane permeability of the rumen microbiota. These results suggest that physical environmental properties may be critical in regulating the physical properties of the rumen microbiota and the balanced ecosystem.
The raw data supporting the conclusions of this article will be made available by the authors, without undue reservation.
The animal studies were approved by the trial was conducted at the experimental farm of the Institute of Subtropical Agriculture (ISA), the Chinese Academy of Sciences. The protocol and experiment procedures were approved by the Animal Care Committee of ISA (Approval ISA-2012-018). The studies were conducted in accordance with the local legislation and institutional requirements. Written informed consent was obtained from the owners for the participation of their animals in this study.
YL: Conceptualization, Formal analysis, Methodology, Writing – original draft. JL: Conceptualization, Software, Validation, Writing – original draft. ST: Resources, Visualization, Writing – original draft. CZ: Project administration, Validation, Writing – original draft. ZT: Supervision, Validation, Writing – original draft. AS: Writing – original draft.
The author(s) declare financial support was received for the research, authorship, and/or publication of this article. The present project was supported by the Strategic Priority Research Program of the Chinese Academy of Sciences (No. XDA26010301), the National Natural Science Foundation of China (32372828), the Natural Science Foundation of Guangxi Zhuang Autonomous Region (2022GXNSFAA035517), the Natural Science Foundation of Hunan Province (2023JJ30613), and Technical system of herbivore industry in Hunan Province.
The authors declare that the research was conducted in the absence of any commercial or financial relationships that could be construed as a potential conflict of interest.
The author(s) declare that no Gen AI was used in the creation of this manuscript.
All claims expressed in this article are solely those of the authors and do not necessarily represent those of their affiliated organizations, or those of the publisher, the editors and the reviewers. Any product that may be evaluated in this article, or claim that may be made by its manufacturer, is not guaranteed or endorsed by the publisher.
APG, alkyl polyglucoside; A/P, acetate/propionate ratio; cAMP, cyclic adenosine monophosphate; c-di-GMP, cyclic di-guanosine monophosphate; CRP, cAMP receptor protein (CRP); EPS, extracellular polymeric substances; FITC-dextran, fluorescein isothiocyanate-dextran; GPL, glycerolphospholipids; LOS, lipooligosaccharide; LPS, lipopolysaccharide; MRM, mixed rumen microbiota; NDF, neutral detergent fiber; NDFD, neutral detergent fiber digestibility; OD, optical density; PBP1A, penicillin-binding protein; PBS, phosphate-buffered saline; SSA, specific surface area; ST, surface tension; tVFA, total volatile fatty acid; VFA, volatile fatty acid; ξ, Zeta potential.
1. Liu K, Zhang Y, Yu Z, Xu Q, Zheng N, Zhao S, et al. Ruminal microbiota-host interaction and its effect on nutrient metabolism. Anim Nutr. (2021) 7:49–55. doi: 10.1016/j.aninu.2020.12.001
2. Zhao A, Sun J, Liu Y. Understanding bacterial biofilms: from definition to treatment strategies. Front Cell Infect Microbiol. (2023) 13:1137947. doi: 10.3389/fcimb.2023.1137947
3. Sauer K, Stoodley P, Goeres DM, Hall-Stoodley L, Burmølle M, Stewart PS, et al. The biofilm life cycle: expanding the conceptual model of biofilm formation. Nat Rev Microbiol. (2022) 20:608–20. doi: 10.1038/s41579-022-00767-0
4. Karygianni L, Ren Z, Koo H, Thurnheer T. Biofilm matrixome: extracellular components in structured microbial communities. Trends Microbiol. (2020) 28:668–81. doi: 10.1016/j.tim.2020.03.016
5. Hong SH, Gorce JB, Punzmann H, Francois N, Shats M, Xia H. Surface waves control bacterial attachment and formation of biofilms in thin layers. Sci Adv. (2020) 6:eaaz9386. doi: 10.1126/sciadv.aaz9386
6. Flemming H-C, van Hullebusch ED, Little BJ, Neu TR, Nielsen PH, Seviour T, et al. Microbial extracellular polymeric substances in the environment, technology and medicine. Nat Rev Microbiol. (2024). doi: 10.1038/s41579-024-01098-y
7. Zheng S, Bawazir M, Dhall A, Kim HE, He L, Heo J, et al. Implication of surface properties, bacterial motility, and hydrodynamic conditions on bacterial surface sensing and their initial adhesion. Front Bioeng Biotechnol. (2021) 9:643722. doi: 10.3389/fbioe.2021.643722
8. Liu C, Sun D, Liu J, Chen Y, Zhou X, Ru Y, et al. Camp and C-Di-Gmp synergistically support biofilm maintenance through the direct interaction of their effectors. Nat Commun. (2022) 13:1493. doi: 10.1038/s41467-022-29240-5
9. Mu M, Liu S, DeFlorio W, Hao L, Wang X, Salazar KS, et al. Influence of surface roughness, nanostructure, and wetting on bacterial adhesion. Langmuir. (2023) 39:5426–39. doi: 10.1021/acs.langmuir.3c00091
10. Huang N, Ruan L, Zhang J, Wang Y, Shen Q, Deng Y, et al. Improved physicochemical and functional properties of dietary fiber from matcha fermented by trichoderma viride. Food Chem. (2024) 460:140784. doi: 10.1016/j.foodchem.2024.140784
11. Flemming H-C, Wingender J, Szewzyk U, Steinberg P, Rice SA, Kjelleberg S. Biofilms: an emergent form of bacterial life. Nat Rev Microbiol. (2016) 14:563–75. doi: 10.1038/nrmicro.2016.94
12. Li S, Liu SY, Chan SY, Chua SL. Biofilm matrix cloaks bacterial quorum sensing chemoattractants from predator detection. ISME J. (2022) 16:1388–96. doi: 10.1038/s41396-022-01190-2
13. Jo J, Price-Whelan A, Dietrich LEP. Gradients and consequences of heterogeneity in biofilms. Nat Rev Microbiol. (2022) 20:593–607. doi: 10.1038/s41579-022-00692-2
14. Bernard P, Stelmachowski P, Broś P, Makowski W, Kotarba A. Demonstration of the influence of specific surface area on reaction rate in heterogeneous catalysis. J Chem Educ. (2021) 98:935–40. doi: 10.1021/acs.jchemed.0c01101
15. Liu Y, Ran T, Tenorio-Borroto E, Tang S, Pazos A, Tan Z, et al. Experimental and chemometric studies of cell membrane permeability. Chemomet Intell Lab Syst. (2016) 154:1–6. doi: 10.1016/j.chemolab.2016.03.010
16. Al-Amshawee S, Yunus MYBM, Lynam JG, Lee WH Dai F, Dakhil IH. Roughness and wettability of biofilm carriers: a systematic review. Environ Technol Innovat. (2021) 21:101233. doi: 10.1016/j.eti.2020.101233
17. Raj Deena S, Kumar G, Vickram AS, Rani Singhania R, Dong CD, Rohini K, et al. Efficiency of various biofilm carriers and microbial interactions with substrate in moving bed-biofilm reactor for environmental wastewater treatment. Bioresour Technol. (2022) 359:127421. doi: 10.1016/j.biortech.2022.127421
18. Naderizadeh S, Dante S, Picone P, Di Carlo M, Carzino R, Athanassiou A, et al. Bioresin-based superhydrophobic coatings with reduced bacterial adhesion. J Colloid Interface Sci. (2020) 574:20–32. doi: 10.1016/j.jcis.2020.04.031
19. Matthews C, Crispie F, Lewis E, Reid M, O'Toole PW, Cotter PD. The rumen microbiome: a crucial consideration when optimising milk and meat production and nitrogen utilisation efficiency. Gut Microbes. (2019) 10:115–32. doi: 10.1080/19490976.2018.1505176
20. McGrath J, Duval SM, Tamassia LFM, Kindermann M, Stemmler RT, de Gouvea VN, et al. Nutritional strategies in ruminants: a lifetime approach. Res Vet Sci. (2018) 116:28–39. doi: 10.1016/j.rvsc.2017.09.011
21. Cheng L, Zhang X, Reis S, Ren C, Xu J, Gu B, et al. 12% switch from monogastric to ruminant livestock production can reduce emissions and boost crop production for 525 million people. Nature Food. (2022) 3:1040–51. doi: 10.1038/s43016-022-00661-1
22. Abdi A, Ranjbar B, Kazemzadeh Y, Aram F, Riazi M. Investigating the mechanism of interfacial tension reduction through the combination of low-salinity water and bacteria. Sci Rep. (2024) 14:11408. doi: 10.1038/s41598-024-62255-0
23. Liu Y, Tang S, Fernandez-Lozano C, Munteanu CR, Pazos A, Yu Y-z, et al. Experimental study and random forest prediction model of microbiome cell surface hydrophobicity. Exp Syst Appl. (2017) 72:306–16. doi: 10.1016/j.eswa.2016.10.058
24. Tullo E, Finzi A, Guarino M. Review: environmental impact of livestock farming and precision livestock farming as a mitigation strategy. Sci Total Environ. (2019) 650:2751–60. doi: 10.1016/j.scitotenv.2018.10.018
25. Moojen FG, Ryschawy J, Wulfhorst JD, Archer DW, de Faccio Carvalho PC, Hendrickson JR. Case study analysis of innovative producers toward sustainable integrated crop-livestock systems: trajectory, achievements, and thought process. Agron Sustain Dev. (2024) 44:26. doi: 10.1007/s13593-024-00953-9
26. Liu Y, Ran T, Tan ZL, Tang SX, Wang PP. Effects of surface tension and specific surface areas on in vitro fermentation of fiber. Chin J Anim Vet Sci. (2013) 44:901–10.
27. Tang S, Tan Z, Zhou C, Jiang H, Jiang Y, Sheng L, et al. Comparison of in vitro fermentation characteristics of different botanical fractions of mature maize stover. J Anim Feed Sci. (2006) 15:505–15. doi: 10.22358/jafs/66920/2006
28. Liu Y, Munteanu CR, Fernandez-Lozano C, Pazos A, Ran T, Tan Z, et al. Experimental study and ann dual-time scale perturbation model of electrokinetic properties of microbiota. Front Microbiol. (2017) 8:1216. doi: 10.3389/fmicb.2017.01216
29. Pelletier C, Bouley C, Cayuela C, Bouttier S, Bourlioux P, Bellon-fontaine M-N. Cell surface characteristics of Lactobacillus casei Subsp Casei, Lactobacillus paracasei Subsp Paracasei, and Lactobacillus rhamnosus strains. Appl Environ Microbiol. (1997) 63:1725–31. doi: 10.1128/aem.63.5.1725-1731.1997
30. Wang FY, Miao CC, Han HL, Jin WB, Liu Z. Effect of electric field on cell growth, permeability and extracellular enzymatic reactions of phanerochaete chrysosporium. Chin J Process Eng. (2007) 7:385–9.
31. Li J, McLandsborough LA. The effects of the surface charge and hydrophobicity of Escherichia coli on its adhesion to beef muscle. Int J Food Microbiol. (1999) 53:185–93. doi: 10.1016/S0168-1605(99)00159-2
32. SAS Institute, editor. The Sas System for Microsoft Windows, Release 8.2. Cary, NC: SAS Institute Inc. (2001).
33. De Vadder F, Kovatcheva-Datchary P, Goncalves D, Vinera J, Zitoun C, Duchampt A, et al. Microbiota-generated metabolites promote metabolic benefits via gut-brain neural circuits. Cell. (2014) 156:84–96. doi: 10.1016/j.cell.2013.12.016
34. Xu Z, Munyaneza NE, Zhang Q, Sun M, Posada C, Venturo P, et al. Chemical upcycling of polyethylene, polypropylene, and mixtures to high-value surfactants. Science. (2023) 381:666–71. doi: 10.1126/science.adh0993
35. Dini S, Bekhit AE-DA, Roohinejad S, Vale JM, Agyei D. The physicochemical and functional properties of biosurfactants: a review. Molecules. (2024) 29:2544. doi: 10.3390/molecules29112544
36. Pardhi DS, Panchal RR, Raval VH, Joshi RG, Poczai P, Almalki WH, et al. Microbial surfactants: a journey from fundamentals to recent advances. Front Microbiol. (2022) 13:982603. doi: 10.3389/fmicb.2022.982603
37. Kaczorek E, Pacholak A, Zdarta A, Smułek W. The impact of biosurfactants on microbial cell properties leading to hydrocarbon bioavailability increase. Colloids Int. (2018) 2:35. doi: 10.3390/colloids2030035
38. Guseva K, Mohrlok M, Alteio L, Schmidt H, Pollak S, Kaiser C. bacteria face trade-offs in the decomposition of complex biopolymers. PLoS Comput Biol. (2024) 20:e1012320. doi: 10.1371/journal.pcbi.1012320
39. Nakamura T, Fujii T, Ichihara A. Enzyme leakage due to change of membrane permeability of primary cultured rat hepatocytes treated with various hepatotoxins and its prevention by glycyrrhizin. Cell Biol Toxicol. (1985) 1:285–95. doi: 10.1007/BF00118193
40. Kamischke C, Fan J, Bergeron J, Kulasekara HD, Dalebroux ZD, Burrell A, et al. The Acinetobacter baumannii Mla system and glycerophospholipid transport to the outer membrane. Elife. (2019) 8:e40171. doi: 10.7554/eLife.40171
41. Ebbensgaard A, Mordhorst H, Aarestrup FM, Hansen EB. The role of outer membrane proteins and lipopolysaccharides for the sensitivity of Escherichia coli to antimicrobial peptides. Front Microbiol. (2018) 9:2153. doi: 10.3389/fmicb.2018.02153
42. Delcour AH. Outer membrane permeability and antibiotic resistance. Biochim Biophys Acta. (2009) 1794:808–16. doi: 10.1016/j.bbapap.2008.11.005
43. Di Vincenzo F, Del Gaudio A, Petito V, Lopetuso LR, Scaldaferri F. Gut microbiota, intestinal permeability, and systemic inflammation: a narrative review. Int Emerg Med. (2024) 19:275–93. doi: 10.1007/s11739-023-03374-w
44. Chen Y-J, Leung PM, Wood JL, Bay SK, Hugenholtz P, Kessler AJ, et al. Metabolic flexibility allows bacterial habitat generalists to become dominant in a frequently disturbed ecosystem. ISME J. (2021) 15:2986–3004. doi: 10.1038/s41396-021-00988-w
45. Rojas M, Donahue JP, Tan ZJ, Lin YZ. Genetic engineering of proteins with cell membrane permeability. Nat Biotechnol. (1998) 16:370–5. doi: 10.1038/nbt0498-370
46. Yuan Y, Hays MP, Hardwidge PR, Kim J. Surface characteristics influencing bacterial adhesion to polymeric substrates. RSC Adv. (2017) 7:14254–61. doi: 10.1039/C7RA01571B
47. Balazs DJ, Triandafillu K, Chevolot Y, Aronsson BO, Harms H, Descouts P, et al. Surface modification of Pvc endotracheal tubes by oxygen glow discharge to reduce bacterial adhesion. Surf Interf Anal. (2003) 35:301–9. doi: 10.1002/sia.1533
48. Oh JK, Yegin Y, Yang F, Zhang M, Li J, Huang S, et al. The influence of surface chemistry on the kinetics and thermodynamics of bacterial adhesion. Sci Rep. (2018) 8:17247. doi: 10.1038/s41598-018-35343-1
49. Moser I, Schröder W. Hydrophobic characterization of thermophilic campylobacter species and adhesion to Int 407 cell membranes and fibronectin. Microb Pathog. (1997) 22:155–64. doi: 10.1006/mpat.1996.0104
50. Nguyen VT, Turner MS, Dykes GA. Influence of cell surface hydrophobicity on attachment of campylobacter to abiotic surfaces. Food Microbiol. (2011) 28:942–50. doi: 10.1016/j.fm.2011.01.004
51. Baselga R, Albizu I, Penadés JR, Aguilar B, Iturralde M, Amorena B. Hydrophobicity of ruminant mastitis Staphylococcus aureus in relation to bacterial aging and slime production. Curr Microbiol. (1992) 25:173–9. doi: 10.1007/BF01571026
52. Zhang C, Jia L, Wang SH, Qu J, Li K, Xu LL, et al. Biodegradation of beta-cypermethrin by two Serratia spp. with different cell surface hydrophobicity. Bioresour Technol. (2010) 101:3423–9. doi: 10.1016/j.biortech.2009.12.083
53. Soni K, Balasubramanian A, Beskok A, Pillai S. Zeta potential of selected bacteria in drinking water when dead, starved, or exposed to minimal and rich culture media. Curr Microbiol. (2008) 56:93–7. doi: 10.1007/s00284-007-9046-z
54. Wang S, Zhao Y, Breslawec AP, Liang T, Deng Z, Kuperman LL, et al. Strategy to combat biofilms: a focus on biofilm dispersal enzymes. npj Biofilms Microb. (2023) 9:63. doi: 10.1038/s41522-023-00427-y
55. Ferreyra Maillard APV, Espeche JC, Maturana P, Cutro AC, Hollmann A. Zeta potential beyond materials science: applications to bacterial systems and to the development of novel antimicrobials. Biochim Biophys Acta Biomembr. (2021) 1863:183597. doi: 10.1016/j.bbamem.2021.183597
56. Delgado AV, González-Caballero F, Hunter RJ, Koopal LK, Lyklema J. Measurement and interpretation of electrokinetic phenomena. Pure Appl Chem. (2005) 77:1753–805. doi: 10.1351/pac200577101753
57. Catterall WA. From ionic currents to molecular mechanisms: the structure and function of voltage-gated sodium channels. Neuron. (2000) 26:13–25. doi: 10.1016/S0896-6273(00)81133-2
58. Clairfeuille T, Xu H, Koth CM, Payandeh J. Voltage-gated sodium channels viewed through a structural biology lens. Curr Opin Struct Biol. (2017) 45:74–84. doi: 10.1016/j.sbi.2016.11.022
59. Hua ZZ, Chen J, Lun SY, Wang XR. Influence of biosurfactants produced by Candida antarctica on surface properties of microorganism and biodegradation of N-alkanes. Water Res. (2003) 37:4143–50. doi: 10.1016/S0043-1354(03)00380-4
60. Zhang QZ, He GF, Wang J, Cai WM, Xu YT. Two-Stage co-hydrolysis of rice straw by Trichoderma reesei Zm4-F3 and Pseudomonas aeruginosa Bsz-07. Biomass Bioenergy. (2009) 33:1464–8. doi: 10.1016/j.biombioe.2009.06.012
61. Schluter J, Nadell CD, Bassler BL, Foster KR. Adhesion as a weapon in microbial competition. ISME J. (2015) 9:139–49. doi: 10.1038/ismej.2014.174
62. Pizarro-Cerdá J, Cossart P. Bacterial adhesion and entry into host cells. Cell. (2006) 124:715–27. doi: 10.1016/j.cell.2006.02.012
63. Doloman A, Sousa DZ. Mechanisms of microbial co-aggregation in mixed anaerobic cultures. Appl Microbiol Biotechnol. (2024) 108:407. doi: 10.1007/s00253-024-13246-8
64. D'Souza G, Ebrahimi A, Stubbusch A, Daniels M, Keegstra J, Stocker R, et al. Cell aggregation is associated with enzyme secretion strategies in marine polysaccharide-degrading bacteria. ISME J. (2023) 17:703–11. doi: 10.1038/s41396-023-01385-1
Keywords: physicochemical properties, rumen microbes, specific surface area, surface tension, physical properties
Citation: Liu Y, Liao J, Tang S, Zhou C, Tan Z and Salem AZM (2025) Physicochemical profiles of mixed ruminal microbes in response to surface tension and specific surface area. Front. Vet. Sci. 11:1514952. doi: 10.3389/fvets.2024.1514952
Received: 21 October 2024; Accepted: 05 December 2024;
Published: 06 January 2025.
Edited by:
Yosra Ahmed Soltan, Alexandria University, EgyptReviewed by:
Hani M. El-Zaiat, Sultan Qaboos University, OmanCopyright © 2025 Liu, Liao, Tang, Zhou, Tan and Salem. This is an open-access article distributed under the terms of the Creative Commons Attribution License (CC BY). The use, distribution or reproduction in other forums is permitted, provided the original author(s) and the copyright owner(s) are credited and that the original publication in this journal is cited, in accordance with accepted academic practice. No use, distribution or reproduction is permitted which does not comply with these terms.
*Correspondence: Shaoxun Tang, c3h0YW5nQGlzYS5hYy5jbg==; Abdelfattah Z. M. Salem, c2FsZW1AdWFlbWV4Lm14
Disclaimer: All claims expressed in this article are solely those of the authors and do not necessarily represent those of their affiliated organizations, or those of the publisher, the editors and the reviewers. Any product that may be evaluated in this article or claim that may be made by its manufacturer is not guaranteed or endorsed by the publisher.
Research integrity at Frontiers
Learn more about the work of our research integrity team to safeguard the quality of each article we publish.