- 1Department of Veterinary Medicine and Animal Sciences, University of Milan, Lodi, Italy
- 2Department of Chemistry, Sapienza University of Rome, Rome, Italy
- 3Department of Chemical Sciences, University of Naples Federico II, Napoli, Italy
- 4I.N.B.B., Istituto Nazionale Biostrutture e Biosistemi, Rome, Italy
- 5Proteomics and Metabolomics Unit, San Raffaele Scientific Institute, Milan, Italy
- 6UNIRELab, Settimo Milanese, Italy
Background: Recently, environmental pollution has become a significant concern for human, animal, and environmental health, fitting within the “One Health” framework. Among the various environmental contaminants, per- and polyfluoroalkyl substances (PFASs) have gathered substantial attention due to their persistence, bioaccumulation, and adverse health effects. This study aimed to compare the levels of 12 PFASs in the fur, liver, and muscle of wild roe deer to evaluate the feasibility of using fur as a non-invasive biomonitoring matrix.
Methods: A total of 20 male and 20 female roe deer aged between 12 and 24 months were randomly sampled from a hunting area in Northern Italy. Samples of fur, muscle, and liver were collected post-mortem, and PFAS concentrations were measured using a validated UHPLC-HRMS method.
Results and discussion: The results indicated significant differences in PFAS concentrations among the three matrices. Fur, although easier to sample and store, showed highly variable PFAS levels, with different detection frequencies compared to the muscle and liver. PFASs such as PFHxA were more frequently detected in fur than in the liver and muscle, while compounds such as PFBA, PFPeA, PFHpA, PFDA, PFHxS, 6-2 FTS, and 8-2 FTS were less frequently detected in fur. In conclusion, while fur presents many practical advantages for biomonitoring, such as non-invasive sampling and stability, its use is complicated by varying detection frequencies and concentration levels. These aspects, together with the use of a single sampling technique, can be considered a limitation of the study. Notably, compounds such as PFOA, PFNA, and PFOS showed partially similar detection frequencies across the matrices, suggesting potential interest for further research. This study offers new perspectives on the use of fur for environmental monitoring, highlighting the need for more extensive research to understand the relationship between PFAS concentrations in fur and other biological matrices. Future studies should focus on methodological improvements in extraction and quantification techniques for PFASs in fur to enhance their reliability as a biomonitoring tool.
1 Introduction
Recently, environmental pollution has become a concern for human, animal, and environmental health within the context now defined as “One Health” (1). Environmental contaminants are defined as substances introduced into the environment due to human activity or natural processes that can harm ecosystems, organisms, or human health. These include pollutants such as metals, pesticides, polychlorinated biphenyls (PCBs), dioxins, plastics, per- and polyfluoroalkyl substances (PFASs), and hydrocarbons (2). They often originate from industrial processes, agricultural runoff, waste disposal, or atmospheric deposition and can accumulate in water, soil, air, and biological systems (3). Once released into the environment and comes into contact with living organisms, if the rate of absorption exceeds the rate of elimination, bioaccumulation occurs (4). Another characteristic of environmental pollutants is their entry into the food chain, leading to the phenomenon known as biomagnification, which describes the increasing concentration of these substances as they move up the food chain. Predators at higher trophic levels accumulate higher concentrations of contaminants because they consume multiple prey, each containing accumulated pollutants (4). Environmental contaminants that can bioaccumulate and biomagnify, and thus have a long half-life, are known as persistent organic pollutants (POPs). These substances remain stable in the environment, with extended half-lives in soil, sediments, air, or living organisms (5). Although there is no universally agreed-upon threshold for the half-life duration required for a pollutant to be deemed persistent, in practical terms, a POP may have a half-life ranging from years to decades in soil or sediments and lasting several days in the atmosphere (6). The bioaccumulation and biomagnification of environmental pollutants in living organisms often result in adverse health effects (7).
In general, exposure to xenobiotics is influenced by numerous factors related to both the living organism and the compound; hence, environmental monitoring may not be sufficient to determine the real exposure at the biota level, as stated by Rendon Lugo et al. and Zhou et al. (8, 9). Indeed, standard environmental monitoring can detect the presence of contaminants, but does not provide information on the biological effects these contaminants have on living organisms. Wild mammals can show signs of physiological stress, behavioral changes, reduced fertility, and other biological responses that may indicate the real impact of the contaminants (10). Moreover, since wild mammals often share the same ecosystems and food resources with humans, they can serve as indicators for understanding the potential health effects on humans (10). Finally, another particularly useful aspect is the possibility of obtaining a more comprehensive picture of the distribution and concentration of contaminants in the environment (11, 12). Wild mammals, through their movements and behaviors, can accumulate contaminants from various sources and geographical areas (13). Over the past few decades, numerous wild mammals have been identified as potential sentinels for monitoring the presence of xenobiotics and have been used to conduct biomonitoring studies. Some examples include herbivorous and omnivorous macromammals such as the wild boar (14), otter (15), deer (16), and roe deer (17) and herbivorous and omnivorous micromammals such as shrews (18), hedgehogs (19), and bats (20). The European roe deer, among wild mammals, has been recognized as a suitable bioindicator due to its unique behavioral traits. These include small home ranges (16–80 ha) and high behavioral adaptability, enabling it to thrive in diverse habitats, including those heavily influenced by human activities (21). Roe deer primarily consume leaves, young shoots, berries, and grass, favoring easily digestible forage. Studies have shown that pollutant levels in their muscles correlate with their dietary intake (22, 23). In the past, this animal has already been used as a bioindicator for pesticides (24), fluoride, lead (25), and toxic trace elements (26).
Among environmental contaminants, PFASs are xenobiotics receiving considerable attention recently from the scientific and public communities and also frequently considered in biomonitoring programs. Per- and polyfluoroalkyl substances are emerging POPs of anthropogenic origin (27). They contain between 5,000 and 10,000 compounds (28), regularly used in various industrial processes such as plasticizers, polymerizing acids, and flame retardants (29). Their worldwide use is attributed to their chemical–physical characteristics, ensuring high thermal, chemical, and biological stability (30, 31). These same characteristics prevent their degradation when dispersed into the environment, leading to their entry into the food chain and subsequent bioaccumulation in biota (32). Over the decades, their bioaccumulation in living organisms has been found to have numerous serious adverse health effects, both in humans and animals. A growing number of studies on humans and animals, and mammals in general, indicate that exposure to PFASs leads to the disruption of endocrine functions and metabolism (33), impairment of liver and thyroid hormones (34), impact on renal physiology (35) and bones (36), together with their immunotoxicity with immunosuppressive effects (37, 38).
Till date, the identification and quantification of PFASs, both in humans and animals, have been mainly carried out in tissues and body fluids such as muscle, liver (39) blood, urine, and feces (40). All these matrices allow for precise quantification but have ethical and practical implications. They are difficult to obtain as sampling requires invasive procedures with physical or chemical restraint of animals or must occur post-mortem, during necropsy, or at the slaughterhouse (20, 41). The biological matrices traditionally used also present practical problems of transportation and preservation since they degrade very easily (41). In recent decades, to overcome many of these inconveniences, many new matrices collected non-invasively have been used to identify the presence of PFASs in living organisms. Non-invasive sampling, as the name suggests, involves the collection of tissues without sacrificing the animal and with minimal pain or stress induction (20, 42). The matrices mainly used have been hair in humans and fur in animals; however, other matrices have also been identified in animals, for example, feathers in birds (43). These matrices are much simpler to sample as they do not require any invasive procedure (44). Fur, if stored away from light, humidity, and heat sources, is a stable matrix (12). Both fur and hair have been frequently used for identifying exposure to xenobiotics despite the lack of pharmacokinetic or toxicokinetic studies (45). Indeed, substances circulating in the blood are incorporated into the fur through the follicle and distributed along its length as it grows. Finally, but fundamentally, fur allows for the identification of exposure to a xenobiotic over a broader time window, unlike the immediate timeframe of other regularly used matrices (44).
Hair and fur have been used to identify exposure to various xenobiotics and drugs, but to the best of our knowledge, only one study has quantified the presence of PFASs in the fur of dogs (46). For this reason, the objective of this study was to quantify 12 PFASs in the fur, liver, and muscle of wild roe deer to understand whether the fur reflects the PFAS content in the organism and, therefore, whether the fur of wild animals can be used as a biomonitoring tool for the environmental presence of PFASs.
2 Materials and methods
2.1 Animals and sample collection
This study included 40 roe deer (20 males and 20 females), aged between 12 and 24 months. A specific area of Northern Italy, which presented a well-structured hunting activity, was chosen, and the sampling procedures were conducted during the hunting season from June to August. The hunting area is characterized by an altitude ranging from 500 to 900 m above sea level. There are cultivated areas, sporadically inhabited centers, wooded areas with oaks and beeches, and pastures in the higher altitude zones. The animal culling area was georeferenced. The authors, before starting the procedure, requested and obtained the study approval as a non-experimental project by the Institutional Animal Care and Use Committee of Università degli Studi di Milano (Permission OPBA_26_2022). Tissues from the 40 roe deer were collected at the hunting meat processing plants after the culling during the regular hunting activities. From each animal, before starting the slaughtering procedures, an area of 10 cm2 was shaved close to the skin with an electric shaver, behind the costal arch, on the left side of the animal. Fur was preserved and wrapped in aluminum foil in a dry and shaded place until the analysis. During the slaughtering procedures, 100 g of muscle, from the longissimus lumborum et thoracis on the left side of the carcass, and 100 g of liver were collected. The tissue samples were stored in 50-mL glass conical centrifuge tubes (Corning Incorporated, Corning, NY, USA) at −20°C.
2.2 Chemicals and reagents
Acetic acid, acetone, sodium dodecyl sulfate (SDS), and methanol of analytical grade were purchased from Merck (Merck KGaA, Darmstadt, Germany). The internal standards (IS), C-labeled, MPFOS (sodium perfluoro-1-[1,2,3,4-13C4]octanesulfonate) and MPFNA (perfluoro-n-[1,2,3,4,5-13C5]nonanoic acid) and the ISOmix (ISO21675:2019 native stock solution) were purchased from Wellington Laboratories INC (345 Southgate Drive, Guelph, Ontario N1G3M5, Canada). Individual stock standard solutions of IS were prepared at a concentration of 1 ppm in MeOH and stored at −20°C. Working solutions were prepared daily by diluting the stock standard solutions in methanol.
2.3 Extraction procedure and UPLC-HRMS
The protocol used for the sample preparation for the analysis of fur was previously described by Makowska et al. and Martin et al. (46, 47), with a few modifications. An aliquot of 0.2 g of the sample was weighed and placed into a glass tube with a conical bottom to perform a fourfold washing to remove exogenous substances: first, 10 ml of ultrapure water was added, gently vortexed, and sonicated for 5 min. Then, 10 ml of SDS 0.1% was added. The samples were gently vortexed and sonicated for 5 min. In the end, two subsequent washing steps were carried out with 10 ml of ultrapure water, each one followed by a 5-min sonication. After the washing, the fur was dried at room temperature on blotting paper. After complete drying, 0.1 g of fur from each sample was weighed, cut into small fragments approximately 2–3 mm in length, and placed into a new glass tube. To the aliquots of fur, 1 ng of IS and 2 ml of a mixture of methanol and acetic acid (MeOH/HAc 85:15 v/v) were added, gently vortexed, and incubated at 38°C for 12 h. The samples were cooled at room temperature and then 3 mL of acetone was added. After a 15-min sonication step, the samples were centrifuged for 10 min at 2,900 × g. fThe supernatant was transferred into a clean glass tube using a glass Pasteur pipette and evaporated until dry under vacuum in a centrifugal evaporator at a temperature of 55°C. In the end, the extract was reconstituted by adding 100 μl of MeOH and 100 μl of the mobile phase (90% water with 20 mM ammonium formate and 10% MeOH), vortexed, and transferred into vials for UPLC-HRMS. For the extraction of PFASs from muscle and liver samples, a method already validated by the authors in other biological matrices was used (39, 48, 49). The instrumental method, based on the recent study by Draghi et al. (49), used a Vanquish system equipped with a binary pump, auto-sampler, and a thermostatted compartment for two columns (Thermo Fisher Scientific, Waltham, MA, USA), coupled to a Thermo Q Exactive Orbitrap™ (Thermo Fisher Scientific, Waltham, MA, USA) with a heated electrospray ionization source. Instrumental parameters for UPLC-HRMS are detailed in the Supplementary material. Post-run chromatograms and spectra were processed using Xcalibur™ 4.3 software (Thermo Fisher Scientific, Waltham, MA, USA) for data interpretation.
2.4 Method validation
Validation was conducted for perfluorobutanoic acid (PFBA), perfluoropentanoic acid (PFPeA), perfluorohexanoic acid (PFHxA), perfluoroheptanoic acid (PFHpA), perfluorooctanoic acid (PFOA), perfluorononanoic acid (PFNA), perfluorodecanoic acid (PFDA), perfluorobutanesulfonic acid (PFBS), perfluorohexanesulfonic acid (PFHxS), perfluorooctanesulfonic acid (PFOS), 6:2-fluorotelomersulfonic acid (6-2 FTS), and 8:2-fluorotelomersulfonic acid (8-2 FTS), in accordance with the updated SANTE guidelines 11,312/2021 (75), by assessing selectivity, limit of detection (LOD), limit of quantification (LOQ), and matrix effect. The method’s selectivity was confirmed by evaluating interference peaks in blank samples near the expected PFAS retention times. To prevent analytical misinterpretation due to potential PFAS contamination in extraction and purification materials, eight procedural blanks without matrix were prepared for each extraction session. Quality assurance/control (QA/QC) was carried out by analyzing five matrix blank samples to determine PFAS contributions in unfortified matrices, and adjusting final concentrations if necessary. Matrix-matched calibration curves (10–100 pg g−1) were constructed by spiking blank samples with standard mixtures. LOD and LOQ were calculated using the equations LOD = 3.3 SD/b and LOQ = 10 SD/b, where SD is the standard deviation of the intercept for low concentration levels and b is the slope of the regression line from the principal calibration curve. The matrix effect was quantified by comparing peak areas of PFASs spiked after blank sample extraction to those of standards in solution, expressed as a percentage. The validation procedures for the liver and muscle were carried out in a previous work by this research group (39). The validation parameters for PFASs, considered in this study, in the liver and muscle are shown in Supplementary Table S1. The validation parameters for fur are shown in Table 1.
2.5 Statistical analysis
At first, a preliminary statistical evaluation was performed using the Shapiro–Wilk test, which revealed that data were not normally distributed; thus, a non-parametric statistical evaluation was applied. In particular, the Kruskal–Wallis one-way (non-parametric ANOVA) analysis was used to check differences between the three datasets (fur, muscle, and liver), followed by all pairwise multiple comparison processes (Dwass–Steel–Critchlow–Fligner method). Statistical analyses were performed using GraphPad Prism 8 ® software (La Jolla, CA, USA). A p-value of 0.05 was set as significant, while a p-value of ≤0.1 was considered a tendency.
3 Results
Mean, median, minimum, and maximum levels of PFAS concentration and statistically significant differences between matrices are reported in Table 2. The differences are reported graphically in Figure 1.
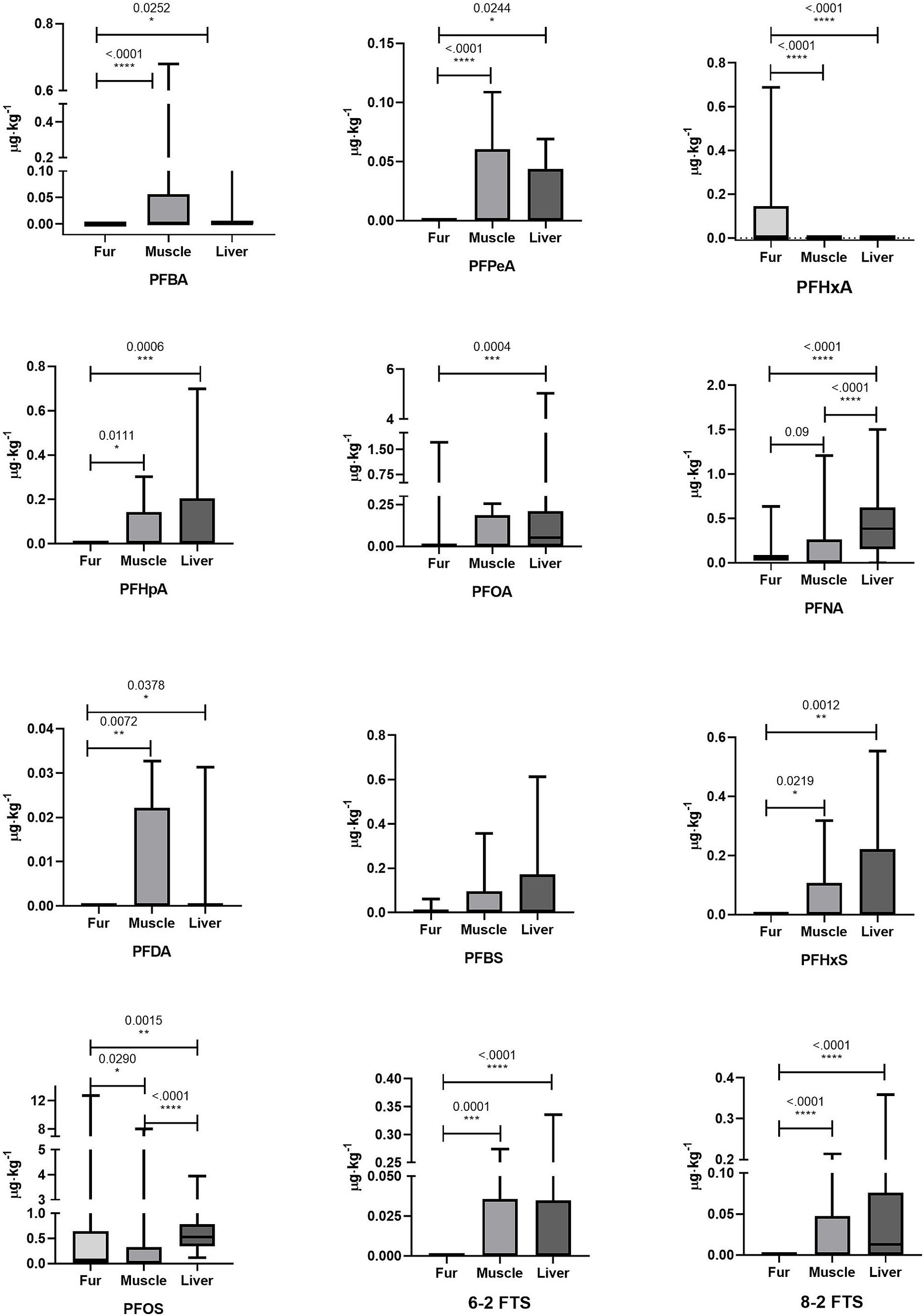
Figure 1. Graphical representation comparing the concentrations of PFASs in the fur, muscle, and liver of roe deer.
In this study, PFNA and PFOS resulted in significantly higher levels in the liver than in muscle and significantly lower levels in the fur than in the other matrices. Specifically, PFNA concentration detected in fur was 0.080 ± 0.103 μg⋅kg−1, in muscle was 0.112 ± 0.227 μg⋅kg−1, and in liver was 0.414 ± 0.322 μg⋅kg−1. PFOS concentration detected in fur was 0.912 ± 2.258 μg⋅kg−1, in muscle was 0.296 ± 1.263 μg⋅kg−1, and in liver was 0.666 ± 0.636 μg⋅kg−1. All the other compounds showed only tendencies to be higher in the liver than in the muscle. In fur, PFBA, PFPeA, PFHpA, PFDA, PFHxS, 6–2 FTS, and 8–2 FTS were not detected and thus significantly lower than in the liver and muscle. The compound PFBS was quantified in fur but did not show any statistical difference between the evaluated matrices (fur concentration: 0.004 ± 0.013 μg⋅kg−1; muscle concentration: 0.065 ± 0.118 μg⋅kg−1; liver concentration: 0.082 ± 0.152 μg⋅kg−1). PFHxA was detected in fur (0.071 ± 0.134 μg⋅kg−1) and in one sample of muscle (0.002 μg⋅kg−1), even though no statistically significant differences were identified in comparison with the liver and muscle. The compound with the most similar detection frequencies among the three matrices was PFOS, although it showed a significantly lower concentration in fur than in the muscle and liver.
4 Discussion
To the best of our knowledge, this is the first study on wildlife that compares the concentration of 12 different PFASs in different matrices (fur, liver, and muscle) from the same animal. This work interprets the One Health perspective by using the roe deer as a biomonitoring species to identify the presence of PFASs in an environment where humans and animals live in close contact, thus proposing this wild species as a sentinel of PFAS pollution.
In this study, we identified PFBA, PFPeA, PFHxA, PFHpA, PFOA, PFNA, PFDA, PFBS, PFHxS, PFOS, 6-2 FTS, and 8-2 FTS in the three analyzed matrices (liver, muscle, and fur). The concentrations of PFNA and PFOS were found to be significantly higher in the liver than in the muscle, consistent with those reported by the authors for liver and muscle roe deer samples collected in a neighboring area (39). The mean and median concentrations in the liver and muscle were also similar. Differently, in the present study, FOSA was not identified in any of the three considered matrices, while PFHxA was identified in the fur and in one muscle sample. The concentrations of PFASs in this study were also compared to those reported in other species. For example, in wild boar liver, the mean concentrations of PFOS and PFOA were 215 and 8.18 μg·kg−1, respectively (50). These differences could be explained by interspecies variations, particularly concerning feeding habits, digestive physiology features, and home ranges. Moreover, the concentrations of PFASs found in roe deer tissues in our study were lower than those reported by Falk et al. for roe deer in Germany (17). A study by Falk covered a larger and more diverse sampling area, encompassing forested, agricultural, and suburban habitats, and was conducted during a historical period (1989–2010) when stricter PFAS regulations were not yet in place. Conversely, our sampling focused on a more restricted area and a single hunting period. Interestingly, PFAS concentrations in roe deer muscle reported in our study were lower than those reported in other species, such as duck (51), cattle (52), and pig (53). This suggests potential interspecies differences that may stem from varying ecological behaviors, exposure routes, and physiological mechanisms of PFAS metabolism (39). In general, PFASs are primarily distributed to protein-rich tissues such as the liver, kidneys, and serum (54, 55), unlike similar hydrophobic contaminants such as PAHs and PCBs (56), which accumulate in adipose tissue. This distribution kinetics has been attributed to their high affinity for proteins, particularly plasma albumins synthesized in the liver (57).In addition, their chemical type causes the tissue-serum partition coefficient to vary from compound to compound. For example, PFOS, PFOA, and PFBS accumulate more in protein-rich tissues such as the liver, whereas PFBA and PFHxS are more distributed in the serum (57, 58). The physicochemical properties of PFASs and their affinities for the aforementioned protein matrices have made blood, serum, muscle, and liver the preferred matrices for quantifying PFASs in both humans and animals over the past decades (59). Obtaining these types of samples in vivo is impossible without sometimes extremely invasive interventions, which carry significant ethical implications. For this reason, alternative matrices are now being explored to identify and quantify PFASs (46).
In humans, many studies have reported the use of hair (60); conversely, to the best of our knowledge, and at the time of writing this article, a single veterinary study has been conducted by Makowska et al. (46) on domestic animals, specifically on dog fur. In the study by Makowska et al. (46), dogs were identified as sentinels for PFAS exposure of their owners as they share the same living conditions and are consequently exposed to similar environmental contaminants (61). As described by many authors, one of the peculiar characteristics of PFASs is their widespread environmental distribution, even far from their production and usage sites (62, 63). This is why wild animals are widely used as sentinels for their environmental presence (64). In this study, roe deer were evaluated as they are free-living wild mammals, which are widely distributed across various environments, including those heavily influenced by anthropogenic activities, making them easily exposed to PFASs (39). In the context of this study, the typical anthropogenic activities exploited within the evaluated geographical area that can contribute to the release of substances such as pesticides, pharmaceutical residues, and trace elements in the environment, include agriculture and livestock farming.
Regarding the identified concentration ranges, when compared with the study by Makowska et al. (46) in dogs, our results indicate lower concentrations in roe deer fur. Moreover, Makowska et al. (45) identified PFBuA, PFPeA, PFHxA, PFHpA, PFOA, and PFOS; in this study, we also identified PFNA, PFDA, and PFBS, obtaining a more comprehensive panel of compounds, including shorter-chain perfluoroalkyl substances and sulfonated compounds. Certainly, comparing different species is complex because the existing toxicokinetic data on PFASs show significant interspecies differences in some toxicokinetic parameters among different compounds (65). The first difference in absorption concerns the digestive system, a physiological feature able to affect the bioavailability of xenobiotics. The roe deer is a ruminant, a selective browser of concentrated forage (66, 67), whereas the dog has a monogastric digestive system and can currently be considered omnivorous (67). The roe deer, being herbivorous, is at the second level of the food chain, whereas the dog is at the third level of the food chain (64). This implies differences in the biomagnification of PFASs. The differences in feeding behavior, food chain position, and digestive physiology can be considered primary factors influencing the absorption of PFASs (58). Another factor to consider is the living environment. The dogs sampled in the study by Makowska et al. (46) are from urban areas, whereas the roe deer analyzed in this study are free-living animals from sparsely populated rural areas, likely resulting in lower exposure to PFASs. Finally, the average lifespan; the dogs analyzed were aged between 3 and over 10 years, while the roe deer used in this study (to minimize variability and focus on matrix comparison) were aged between 12 and 24 months. As reported in other studies, age is an influencing factor of exposure; older animals are exposed to xenobiotics for a longer time and consequently present higher concentrations of contaminants in their tissues (21, 67). All these factors could explain the wide difference observed.
In many studies, fur has been considered a highly accessible matrix for monitoring wild animals, both in terms of environmental toxicology and for different studies related to the general well-being of free-living animals (68). For wildlife, other biological matrices such as blood and muscle are difficult to sample in vivo, whereas in many studies, fur has been considered for non-invasive biomonitoring. An example is the method proposed by Henry et al. (68) for elusive small mammals, which involves placing strips of packing tape arranged in a web-like fashion along travel routes in the pikas’ habitat (68).
For other compounds, such as trace elements, hair has been identified as an excellent matrix for their quantification (69, 70). The availability of studies related to PFASs is very scarce and mainly related to studies conducted on human hair (61). The main problem with using hair as a monitoring tool for PFAS exposure is understanding whether it accurately reflects concentrations in the more commonly used matrices. Alves et al. (60) reported a high variability in PFAS concentrations in hair (ranging from <LOQ to 46 ng/g). As shown in Table 2, also in our case, the concentrations and standard deviations of the compounds quantified in hair are very broad. A study by Li et al. (71) showed that many PFASs identified in hair have a lower concentration and a much lower detection frequency (DF%) than those in urine or nails (60, 71). Our data are also consistent with this, as shown in Table 2 for PFBA, PFPeA, PFHpA, PFDA, PFHxS, 6-2FTS, and 8-2FTS. However, surprisingly, PFHxA was identified much more frequently in hair compared to liver and muscle. Only PFOA, PFNA, and PFOS have partially similar detection frequencies (DF%) in the three analyzed matrices, again in agreement with other studies (60, 71).
These differences are likely due to the so-called matrix effect and the type of hair, which can significantly influence quantification (60). It is widely recognized that the structure of hair (and fur) is a crucial parameter for the incorporation of both drugs and environmental contaminants (72). Despite this, as previously mentioned, PFASs bind to serum proteins and are not lipophilic like other POPs (57), making their quantification in hair reasonable and straightforward (73, 74). Another factor to consider is that hair is a complex matrix with multiple compartments, such as the skin above the hair bulb from which pollutants can access, diffusion from blood to the actively growing follicle, from secretions of the apocrine and sebaceous glands (sweat and sebum), or from the external environment during the hair growth cycle (74). Another factor that could be considered involves the deposition of PFASs on the outer surface of the hair/fur. However, in the case of this study, it can be excluded because the procedure involves multiple washes coupled to sonication before the extraction, as described in the Materials and Methods section. In fact, the wash protocol is always necessary to eliminate the contamination of the fur’s outer face and to guarantee the detected compounds derived from the inside of hair/fur. Conversely, a factor that could have influenced the concentration of PFASs is the absence of the hair bulb. Indeed, our hair samples were shaved close to the skin and not plucked. The hair bulb is directly supplied by the bloodstream and could therefore present a higher concentration (45). Sampling hair with the bulb requires a slightly more invasive sampling procedure that would cause pain and stress to the sampled subject, thus making this type of sampling more complex in the case of wild animals and those raised in captivity. In addition, the collection of fur samples from wild animals in their natural habitat without direct contact, with tools such as hair traps, would result in any case in the sampling of hair without the bulb. For these reasons, the proposed method and the considerations raised in this study can be considered applicable to other non-invasive sampling situations of fur matrix.
Finally, neither in this study nor, to the best of our knowledge, in other studies, have the differences in accumulation in hair based on the carbon chain length of PFASs been considered. This type of study has been done on other matrices such as milk (49) and blood (61, 65). The factors described above may explain the variability in identified concentrations and the difference between the liver and muscle, which have a single route of exposure, namely blood perfusion.
5 Conclusion
This study described a successful method validation for identifying 12 PFASs in roe deer fur, and detection frequencies and concentrations in fur for most substances were generally lower than those found in liver and muscle. Nevertheless, this biological matrix demonstrates many positive aspects for biomonitoring purposes, such as ease of sampling and simplicity of storage, but the determination of how and to what extent PFASs can distribute to fur is yet unknown. Given the results obtained for PFOA, PFNA, and PFOS, this study offers new perspectives, requiring a greater amount of research of this type to understand the relationship between concentrations in fur compared to other matrices such as blood, urine, and feces, and studies aimed at methodological improvement of extraction and quantification techniques for PFASs in this promising matrix, which might include a larger sample aliquot of fur for analysis or the use of different solvents for the washing steps. Finally, further investigation will be necessary to compare the outcomes of different fur sampling techniques (e.g., shaving and plucking with the bulb) and to explore the spatial–temporal trends of PFAS presence in this matrix.
Data availability statement
The original contributions presented in the study are included in the article/Supplementary material, further inquiries can be directed to the corresponding author.
Ethics statement
The animal study was approved by Organismo Preposto al Benessere Animale—University of Milan. The study was conducted in accordance with the local legislation and institutional requirements.
Author contributions
SD: Conceptualization, Formal analysis, Investigation, Methodology, Visualization, Writing – original draft, Writing – review & editing, Data curation. GC: Conceptualization, Investigation, Visualization, Writing – original draft, Writing – review & editing, Resources, Supervision. RR: Writing – review & editing. SM: Writing – review & editing, Funding acquisition. GG: Writing – review & editing, Data curation. AA: Writing – review & editing, Funding acquisition. MS: Writing – review & editing, Data curation. CF: Data curation, Writing – review & editing. RP: Writing – review & editing, Formal analysis, Methodology, Software, Validation. AP: Writing – review & editing, Software, Validation. MF: Writing – review & editing, Resources. PC: Writing – review & editing, Resources. FDC: Writing – review & editing, Conceptualization, Formal analysis, Investigation, Methodology, Supervision, Visualization, Writing – original draft. FA: Writing – review & editing, Funding acquisition, Project administration, Supervision.
Funding
The author(s) declare that financial support was received for the research, authorship, and/or publication of this article. This research was funded by European Union’s NextGenerationEU action and by the Italian Ministry of University and Research, PRIN Project 2022 Grant No. 2022K5TRCZ.
Conflict of interest
The authors declare that the research was conducted in the absence of any commercial or financial relationships that could be construed as a potential conflict of interest.
The author(s) declared that they were an editorial board member of Frontiers, at the time of submission. This had no impact on the peer review process and the final decision.
Publisher’s note
All claims expressed in this article are solely those of the authors and do not necessarily represent those of their affiliated organizations, or those of the publisher, the editors and the reviewers. Any product that may be evaluated in this article, or claim that may be made by its manufacturer, is not guaranteed or endorsed by the publisher.
Supplementary material
The Supplementary material for this article can be found online at: https://www.frontiersin.org/articles/10.3389/fvets.2024.1500651/full#supplementary-material
References
1. Prata, JC, Rocha-Santos, T, and Ribeiro, AI. An introduction to the concept of one health. One Health. (2022) 2022:1–31. doi: 10.1016/B978-0-12-822794-7.00004-6
2. Wexler, P. Encyclopedia of toxicology. J Anal Toxicol. (2005) 38:464–2005. doi: 10.1093/jat/bku065
4. Popek, E. Sampling and analysis of environmental chemical pollutants: a complete guide. Elsevier (2017). 1–414.
5. Jones, KC, and De Voogt, P. Persistent organic pollutants (POPs): state of the science. Environ Pollut. (1999) 100:209–21. doi: 10.1016/S0269-7491(99)00098-6
6. Ashraf, MA. Persistent organic pollutants (POPs): a global issue, a global challenge. Environ Sci Pollut Res. (2017) 24:4223–7. doi: 10.1007/s11356-015-5225-9
7. Prata, JC, da Costa, JP, Lopes, I, Andrady, AL, Duarte, AC, and Rocha-Santos, T. A one health perspective of the impacts of microplastics on animal, human and environmental health. Sci Total Environ. (2021) 777:146094. doi: 10.1016/J.SCITOTENV.2021.146094
8. Rendón-Lugo, AN, Santiago, P, Puente-Lee, I, and León-Paniagua, L. Permeability of hair to cadmium, copper and lead in five species of terrestrial mammals and implications in biomonitoring. Environ Monit Assess. (2017) 189:640. doi: 10.1007/S10661-017-6338-Z
9. Zhou, Q, Zhang, J, Fu, J, Shi, J, and Jiang, G. Biomonitoring: an appealing tool for assessment of metal pollution in the aquatic ecosystem. Anal Chim Acta. (2008) 606:135–50. doi: 10.1016/j.aca.2007.11.018
10. Burger, J, and Gochfeld, M. On developing bioindicators for human and ecological health. Environ Monit Assess. (2001) 66:23–46. doi: 10.1023/A:1026476030728
11. Draghi, S, Spinelli, M, Fontanarosa, C, Curone, G, Amoresano, A, Pignoli, E, et al. Evaluation of the difference in the content of essential and non-essential elements in wild boar and swine tissues sampled in the same area of northern Italy. Animals (Basel). (2024) 14:827. doi: 10.3390/ani14060827
12. Agradi, S, Munga, A, Barbato, O, Palme, R, Tarhan, D, Bilgiç, B, et al. Goat hair as a bioindicator of environmental contaminants and adrenal activation during vertical transhumance. Front Vet Sci. (2023) 10:1274081. doi: 10.3389/fvets.2023.1274081
13. Beyer, WN, and Meador, JP. Environmental Contaminants in Biota: interpreting tissue concentrations In: Beyer WN, Meador JP, editors. Environmental contaminants in biota: Interpreting tissue concentrations, second edition. Second ed. CRC Press (2011). 1–733.
14. Tavoloni, T, Stramenga, A, Stecconi, T, Gavaudan, S, Moscati, L, Sagratini, G, et al. Brominated flame retardants (PBDEs and HBCDs) and perfluoroalkyl substances (PFASs) in wild boars (Sus scrofa) from Central Italy. Sci Total Environ. (2023) 858:159745. doi: 10.1016/J.SCITOTENV.2022.159745
15. O’Rourke, E, Hynes, J, Losada, S, Barber, JL, Pereira, MG, Kean, EF, et al. Anthropogenic drivers of variation in concentrations of Perfluoroalkyl substances in otters (Lutra lutra) from England and Wales. Environ Sci Technol. (2022) 56:1675–87. doi: 10.1021/acs.est.1c05410
16. Mikolajczyk, S, Warenik-Bany, M, Pajurek, M, and Marchand, P. Perfluoroalkyl substances in the meat of polish farm animals and game – occurrence, profiles and dietary intake. Sci Total Environ. (2024) 945:174071. doi: 10.1016/J.SCITOTENV.2024.174071
17. Falk, S, Brunn, H, Schröter-Kermani, C, Failing, K, Georgii, S, Tarricone, K, et al. Temporal and spatial trends of perfluoroalkyl substances in liver of roe deer (Capreolus capreolus). Environ Pollut. (2012) 171:1–8. doi: 10.1016/j.envpol.2012.07.022
18. Sánchez-Chardi, A, López-Fuster, MJ, and Nadal, J. Bioaccumulation of lead, mercury, and cadmium in the greater white-toothed shrew, Crocidura russula, from the Ebro Delta (NE Spain): sex- and age-dependent variation. Environ Pollut. (2007) 145:7–14. doi: 10.1016/j.envpol.2006.02.033
19. Jota Baptista, C, Seixas, F, Gonzalo-Orden, JM, Patinha, C, Pato, P, Ferreira da Silva, E, et al. High levels of heavy metal(loid)s related to biliary hyperplasia in hedgehogs (Erinaceus europaeus). Animals. (2023) 13:1359. doi: 10.3390/ani13081359
20. Jota Baptista, C, Seixas, F, Gonzalo-Orden, JM, and Oliveira, PA. Biomonitoring metals and metalloids in wild mammals: invasive versus non-invasive sampling. Environ Sci Pollut Res. (2022) 29:18398–407. doi: 10.1007/s11356-022-18658-5
21. Cygan-Szczegielniak, D, and Stasiak, K. Correction to: effects of age and sex on the content of heavy metals in the hair, liver and the longissimus lumborum muscle of roe deer Capreolus capreolus L. Environ Sci Pollut Res. (2022) 29:10782–90. doi: 10.1007/s11356-021-16425-6
22. Tixier, H, Duncan, P, Scehovic, J, Yani, A, Gleizes, M, and Lila, M. Food selection by European roe deer (Capreolus capreolus): effects of plant chemistry, and consequences for the nutritional value of their diets. J Zool. (2009) 242:229–45. doi: 10.1111/J.1469-7998.1997.TB05799.X
23. Lehel, J, Laczay, P, Gyurcsó, A, Jánoska, F, Majoros, S, Lányi, K, et al. Toxic heavy metals in the muscle of roe deer (Capreolus capreolus)--food toxicological significance. Environ Sci Pollut Res Int. (2016) 23:4465–72. doi: 10.1007/s11356-015-5658-1
24. Collas, C, Helder, R, Guillon, E, Sayen, S, Quintaine, T, Feidt, C, et al. Roe deer exposure to trace metals and pesticides in forests and agricultural plains of North-Eastern France. Environ Sci Pollut Res Int (2024) doi: 10.1007/s11356-024-34926-y [Epub ahead of print].
25. Kierdorf, H, and Kierdorf, U. Roe deer antlers as monitoring units for assessing temporal changes in environmental pollution by fluoride and lead in a german forest area over a 67-year period. Arch Environ Contam Toxicol. (2000) 39:1–6. doi: 10.1007/s002440010072
26. Draghi, S, Agradi, S, Riva, F, Tarhan, D, Bilgiç, B, Dokuzeylül, B, et al. Roe deer (Capreolus capreolus) hair as a bioindicator for the environmental presence of toxic and trace elements. Toxics. (2023) 11:49. doi: 10.3390/toxics11010049
27. Sunderland, EM, Hu, XC, Dassuncao, C, Tokranov, AK, Wagner, CC, and Allen, JG. A review of the pathways of human exposure to poly- and perfluoroalkyl substances (PFASs) and present understanding of health effects. J Expo Sci Environ Epidemiol. (2018) 29:131–47. doi: 10.1038/s41370-018-0094-1
28. Death, C, Bell, C, Champness, D, Milne, C, Reichman, S, and Hagen, T. Per- and polyfluoroalkyl substances (PFAS) in livestock and game species: a review. Sci Total Environ. (2021) 774:144795. doi: 10.1016/j.scitotenv.2020.144795
29. Jha, G, Kankarla, V, McLennon, E, Pal, S, Sihi, D, Dari, B, et al. Per- and Polyfluoroalkyl substances (PFAS) in integrated crop-livestock systems: environmental exposure and human health risks. Int J Environ Res Public Health. (2021) 18:2550. doi: 10.3390/IJERPH182312550
30. Rayne, S, and Forest, K. Perfluoroalkyl sulfonic and carboxylic acids: a critical review of physicochemical properties, levels and patterns in waters and wastewaters, and treatment methods. J Environ Sci Health A Tox Hazard Subst Environ Eng. (2009) 44:1145–99. doi: 10.1080/10934520903139811
31. Hammer, J, and Endo, S. Volatility and nonspecific van der Waals interaction properties of per- and Polyfluoroalkyl substances (PFAS): evaluation using hexadecane/air partition coefficients. Environ Sci Technol. (2022) 56:15737–45. doi: 10.1021/acs.est.2c05804
32. Sznajder-Katarzyńska, K, Surma, M, Wiczkowski, W, and Cieślik, E. The perfluoroalkyl substance (PFAS) contamination level in milk and milk products in Poland. Int Dairy J. (2019) 96:73–84. doi: 10.1016/j.idairyj.2019.04.008
33. Christensen, KY, Raymond, M, and Meiman, J. Perfluoroalkyl substances and metabolic syndrome. Int J Hyg Environ Health. (2019) 222:147–53. doi: 10.1016/j.ijheh.2018.08.014
34. Coperchini, F, Croce, L, Ricci, G, Magri, F, Rotondi, M, Imbriani, M, et al. Thyroid disrupting effects of old and new generation PFAS. Front Endocrinol (Lausanne). (2021) 11:612320. doi: 10.3389/FENDO.2020.612320
35. Stanifer, JW, Stapleton, HM, Souma, T, Wittmer, A, Zhao, X, and Boulware, LE. Perfluorinated chemicals as emerging environmental threats to kidney health: a scoping review. Clin J Am Soc Nephrol. (2018) 13:1479–92. doi: 10.2215/CJN.04670418
36. Jeddy, Z, Tobias, JH, Taylor, EV, Northstone, K, Flanders, WD, and Hartman, TJ. Prenatal concentrations of perfluoroalkyl substances and bone health in British girls at age 17. Arch Osteoporos. (2018) 13:84. doi: 10.1007/S11657-018-0498-5
37. Ehrlich, V, Bil, W, Vandebriel, R, Granum, B, Luijten, M, Lindeman, B, et al. Consideration of pathways for immunotoxicity of per- and polyfluoroalkyl substances (PFAS). Environ Health. (2023) 22:19. doi: 10.1186/S12940-022-00958-5
38. Liang, L, Pan, Y, Bin, L, Liu, Y, Huang, W, Li, R, et al. Immunotoxicity mechanisms of perfluorinated compounds PFOA and PFOS. Chemosphere. (2022) 291:132892. doi: 10.1016/j.chemosphere.2021.132892
39. Draghi, S, Curone, G, Pavlovic, R, Di Cesare, F, Cagnardi, P, Fornesi Silva, C, et al. Influence of area, age and sex on per- and Polyfluorinated alkyl substances detected in roe deer muscle and liver from selected areas of northern Italy. Animals. (2024) 14:529. doi: 10.3390/ani14040529
40. Ma, J, Zhu, H, and Kannan, K. Fecal excretion of Perfluoroalkyl and Polyfluoroalkyl substances in pets from New York state, United States. Environ Sci Technol Lett. (2020) 7:135–42. doi: 10.1021/acs.estlett.9b00786
41. Richards, NL. Merging wildlife and environmental monitoring approaches with forensic principles: application of unconventional and non-invasive sampling in eco- pharmacovigilance. J For Res. (2014) 5:228. doi: 10.4172/2157-7145.1000228
42. D’Havé, H, Scheirs, J, Mubiana, VK, Verhagen, R, Blust, R, and De Coen, W. Nondestructive pollution exposure assessment in the European hedgehog (Erinaceus europaeus): I. Relationships between concentrations of metals and arsenic in hair, spines, and soil. Environ Toxicol Chem. (2005) 24:2356–64. doi: 10.1897/04-597R.1
43. Groffen, T, Bervoets, L, Jeong, Y, Willems, T, Eens, M, and Prinsen, E. A rapid method for the detection and quantification of legacy and emerging per- and polyfluoroalkyl substances (PFAS) in bird feathers using UPLC-MS/MS. J Chromatogr B Anal Technol Biomed Life Sci. (2021) 1172:122653. doi: 10.1016/j.jchromb.2021.122653
44. Gil-Jiménez, E, Mateo, R, de Lucas, M, and Ferrer, M. Feathers and hair as tools for non-destructive pollution exposure assessment in a mining site of the Iberian Pyrite Belt. Environ Pollut. (2020) 263:114523. doi: 10.1016/j.envpol.2020.114523
45. Jang, WJ, Choi, JY, Park, B, Seo, JH, Seo, YH, Lee, S, et al. Hair metabolomics in animal studies and clinical settings. Molecules. (2019) 24:2195. doi: 10.3390/molecules24122195
46. Makowska, K, Martín, J, Rychlik, A, Aparicio, I, Santos, JL, Alonso, E, et al. Assessment of exposure to perfluoroalkyl substances (PFASs) in dogs by fur analysis. Environ Pollut. (2021) 286:117435. doi: 10.1016/j.envpol.2021.117435
47. Martín, J, Santos, JL, Aparicio, I, and Alonso, E. Analytical method for biomonitoring of endocrine-disrupting compounds (bisphenol a, parabens, perfluoroalkyl compounds and a brominated flame retardant) in human hair by liquid chromatography-tandem mass spectrometry. Anal Chim Acta. (2016) 945:95–101. doi: 10.1016/j.aca.2016.10.004
48. Chiesa, LM, Pavlovic, R, Arioli, F, Nobile, M, Di Cesare, F, Mosconi, G, et al. Presence of perfluoroalkyl substances in Mediterranean Sea and north Italian lake fish addressed to Italian consumer. Int J Food Sci Technol. (2022) 57:1303–16. doi: 10.1111/ijfs.15532
49. Draghi, S, Pavlovic, R, Pellegrini, A, Fidani, M, Riva, F, Brecchia, G, et al. First investigation of the physiological distribution of legacy and emerging Perfluoroalkyl substances in raw bovine Milk according to the component fraction. Food Secur. (2023) 12:2449. doi: 10.3390/FOODS12132449
50. Knutsen, HK, Alexander, J, Barregård, L, Bignami, M, Brüschweiler, B, Ceccatelli, S, et al. Risk to human health related to the presence of perfluorooctane sulfonic acid and perfluorooctanoic acid in food. EFSA J. (2018) 16:e05194. doi: 10.2903/j.efsa.2018.5194
51. Sharp, S, Sardiña, P, Metzeling, L, McKenzie, R, Leahy, P, Menkhorst, P, et al. Per- and Polyfluoroalkyl substances in ducks and the relationship with concentrations in water, sediment, and soil. Environ Toxicol Chem. (2021) 40:846–58. doi: 10.1002/etc.4818
52. Wang, G, Lu, J, Xing, Z, Li, S, and Liu, Z, Tong Y. Occurrence, Distribution, and risk assessment of Perfluoroalkyl acids (PFAAs) in muscle and liver of cattle in Xinjiang, China. Int J Environ Res Public Health (2017) 14:970. doi: 10.3390/ijerph14090970
53. Chen, WL, Bai, FY, Chang, YC, Chen, PC, and Chen, CY. Concentrations of perfluoroalkyl substances in foods and the dietary exposure among Taiwan general population and pregnant women. J Food Drug Anal. (2018) 26:994–1004. doi: 10.1016/j.jfda.2017.12.011
54. Pérez, F, Nadal, M, Navarro-Ortega, A, Fàbrega, F, Domingo, JL, Barceló, D, et al. Accumulation of perfluoroalkyl substances in human tissues. Environ Int. (2013) 59:354–62. doi: 10.1016/j.envint.2013.06.004
55. Blake, BE, and Fenton, SE. Early life exposure to per- and polyfluoroalkyl substances (PFAS) and latent health outcomes: a review including the placenta as a target tissue and possible driver of peri- and postnatal effects. Toxicology. (2020) 443:152565. doi: 10.1016/j.tox.2020.152565
56. La Merrill, M, Emond, C, Kim, MJ, Antignac, JP, Le Bizec, B, Clément, K, et al. Toxicological function of adipose tissue: focus on persistent organic pollutants. Environ Health Perspect. (2013) 121:162–9. doi: 10.1289/ehp.1205485
57. Kelly, BC, Ikonomou, MG, Blair, JD, Surridge, B, Hoover, D, Grace, R, et al. Perfluoroalkyl contaminants in an Arctic marine food web: trophic magnification and wildlife exposure. Environ Sci Technol. (2009) 43:4037–43. doi: 10.1021/es9003894
58. Pizzurro, DM, Seeley, M, Kerper, LE, and Beck, BD. Interspecies differences in perfluoroalkyl substances (PFAS) toxicokinetics and application to health-based criteria. Regul Toxicol Pharmacol. (2019) 106:239–50. doi: 10.1016/j.yrtph.2019.05.008
59. Lau, C. Perfluorinated compounds: an overview. Mol Integrat Toxicol. (2015) 1:1–21. doi: 10.1007/978-3-319-15518-0_1
60. Alves, A, Jacobs, G, Vanermen, G, Covaci, A, and Voorspoels, S. New approach for assessing human perfluoroalkyl exposure via hair. Talanta. (2015) 144:574–83. doi: 10.1016/j.talanta.2015.07.009
61. Karthikraj, R, Lee, S, and Kannan, K. Biomonitoring of exposure to bisphenols, benzophenones, triclosan, and triclocarban in pet dogs and cats. Environ Res. (2020) 180:108821. doi: 10.1016/j.envres.2019.108821
62. Xie, Z, and Kallenborn, R. Legacy and emerging per- and poly-fluoroalkyl substances in polar regions. Curr Opin Green Sustain Chem. (2023) 42:100840. doi: 10.1016/j.cogsc.2023.100840
63. Taniyasu, S, Senthilkumar, K, Yamazaki, E, Yeung, LWY, Guruge, KS, Kannan, K, et al. Perfluoroalkyl substances in the blood of wild rats and mice from 47 prefectures in Japan: use of samples from nationwide specimen bank. Arch Environ Contam Toxicol. (2013) 65:149–70. doi: 10.1007/s00244-013-9878-4
64. Freschi, P, Fascetti, S, Riga, F, Rizzardini, G, Musto, M, and Cosentino, C. Feeding preferences of the Italian roe deer (Capreolus Capreolus Italicus festa, 1925) in a coastal mediterranean environment. Animals. (2021) 11:308. doi: 10.3390/ani11020308
65. Piva, E, Fais, P, Cecchetto, G, Montisci, M, Viel, G, and Pascali, JP. Determination of perfluoroalkyl substances (PFAS) in human hair by liquid chromatography-high accurate mass spectrometry (LC-QTOF). J Chromatogr B Anal Technol Biomed Life Sci. (2021) 1172:122651. doi: 10.1016/j.jchromb.2021.122651
66. Li, P, and Wu, G. Characteristics of nutrition and metabolism in dogs and cats. Adv Exp Med Biol. (2024) 1446:55–98. doi: 10.1007/978-3-031-54192-6_4
67. Squadrone, S, Robetto, S, Orusa, R, Griglione, A, Falsetti, S, Paola, B, et al. Wildlife hair as bioindicators of metal exposure. Biol Trace Elem Res. (2022) 200:5073–80. doi: 10.1007/s12011-021-03074-6
68. Henry, P, Henry, A, and Russello, MA. A noninvasive hair sampling technique to obtain high quality DNA from elusive small mammals. J Vis Exp. (2011) 13:2791. doi: 10.3791/2791-v
69. Draghi, S, Fehri, NE, Ateş, F, Özsobacı, NP, Tarhan, D, Bilgiç, B, et al. Use of hair as matrix for trace elements biomonitoring in cattle and roe deer sharing pastures in northern Italy. Animals. (2024) 14:2209. doi: 10.3390/ani14152209
70. Croose, E, Hanniffy, R, Harrington, A, Põdra, M, Gómez, A, Bolton, PL, et al. Mink on the brink: comparing survey methods for detecting a critically endangered carnivore, the European mink Mustela lutreola. Eur J Wildl Res. (2023) 69. doi: 10.1007/s10344-023-01657-3
71. Li, J, Guo, F, Wang, Y, Liu, J, Cai, Z, Zhang, J, et al. Development of extraction methods for the analysis of perfluorinated compounds in human hair and nail by high performance liquid chromatography tandem mass spectrometry. J Chromatogr A. (2012) 1219:54–60. doi: 10.1016/j.chroma.2011.11.015
72. D’Havé, H, Covaci, A, Scheirs, J, Schepens, P, Verhagen, R, and De Coen, W. Hair as an indicator of endogenous tissue levels of brominated flame retardants in mammals. Environ Sci Technol. (2005) 39:6016–20. doi: 10.1021/es0507259
73. Zhang, H, Chai, Z, and Sun, H. Human hair as a potential biomonitor for assessing persistent organic pollutants. Environ Int. (2007) 33:685–93. doi: 10.1016/j.envint.2007.02.003
74. Henderson, GL. Mechanisms of drug incorporation into hair. Forensic Sci Int. (1993) 63:19–29. doi: 10.1016/0379-0738(93)90256-A
75. SANTE. Guidance document on analytical quality control and method validation procedures for pesticides. Residues analysis in food and feed SANTE 11312/2021. European commission directorate-general for health and food safety, SANTE/11312/2021 (2021). Available at: https://www.eurl-pesticides.eu/userfiles/file/EurlALL/SANTE_11312_2021.pdf
Keywords: biomonitoring, perfluoroalkyl substances, high-resolution mass spectrometry, ecotoxicology, wildlife, endocrine disruptors, roe deer, environmental pollution
Citation: Draghi S, Curone G, Risoluti R, Materazzi S, Gullifa G, Amoresano A, Spinelli M, Fontanarosa C, Pavlovic R, Pellegrini A, Fidani M, Cagnardi P, Di Cesare F and Arioli F (2024) Comparative analysis of PFASs concentrations in fur, muscle, and liver of wild roe deer as biomonitoring matrices. Front. Vet. Sci. 11:1500651. doi: 10.3389/fvets.2024.1500651
Edited by:
Valentina Meucci, University of Pisa, ItalyReviewed by:
Enes Atmaca, Ondokuz Mayis University, TürkiyeCatarina Jota Baptista, Egas Moniz Center for Interdisciplinary Research (CiiEM), Portugal
Copyright © 2024 Draghi, Curone, Risoluti, Materazzi, Gullifa, Amoresano, Spinelli, Fontanarosa, Pavlovic, Pellegrini, Fidani, Cagnardi, Di Cesare and Arioli. This is an open-access article distributed under the terms of the Creative Commons Attribution License (CC BY). The use, distribution or reproduction in other forums is permitted, provided the original author(s) and the copyright owner(s) are credited and that the original publication in this journal is cited, in accordance with accepted academic practice. No use, distribution or reproduction is permitted which does not comply with these terms.
*Correspondence: Federica Di Cesare, ZmVkZXJpY2EuZGljZXNhcmVAdW5pbWkuaXQ=