- 1Department of Clinical Sciences, College of Veterinary Medicine, Auburn University, Auburn, AL, United States
- 2Department of Pathobiology, College of Veterinary Medicine, Auburn University, Auburn, AL, United States
- 3Roy J. Carver Biotechnology Center, Proteomics Core, University of Illinois, Urbana, IL, United States
- 4JF Peroni Laboratory, Department of Large Animal Medicine, College of Veterinary Medicine, University of Georgia, Athens, GA, United States
Introduction: Platelet lysate (PL) demonstrates antimicrobial and anti-inflammatory properties offering potential for treatment of bacterial pneumonia in horses. It remains unknown whether nebulization is suitable for PL administration in horses. This pilot study characterized particle size and flow rate of pooled equine PL (single preparation) nebulized using an equine-specific nebulizer (Flexivent®).
Methods: Protein composition and antimicrobial activity were compared before and after nebulization. Protein composition was evaluated according to growth factor, antimicrobial peptide and cytokine concentrations and proteomic analysis. To evaluate antimicrobial activity, bacterial growth inhibition [maximum growth (μmax); carrying capacity (K)] were determined for E. coli, Streptococcus equi subsp zooepidemicus and Rhodococcus equi (WT and MDR) using pre- and post-nebulized PL concentrations of 50%.
Results: Flow rate and median particle size were 0.8 ml/min and 4.991 μm with 52% of particles ≤ 5 μm. Differences in PL protein composition were detected with nebulization. For E. coli and S. zooepidemicus, nebulization did not alter effect of PL on growth parameters. PL treatments decreased K for S. zooepidemicus (p = 0.009) compared to BHI. For R. equi K was increased post- vs. pre-nebulization (WT and MDR) and μmax increased pre- vs, post-nebulization (MDR). PL treatments increased K and μmax for MDR R. equi and μmax for WT R. equi compared to BHI (p ≤ 0.05).
Conclusion: Nebulization of PL in vitro is technically feasible. The results of this study support further investigation to better characterize the effect of nebulization on PL and its suitability for nebulization in horses.
1 Introduction
Bacterial bronchopneumonia represents a significant cause of morbidity and loss of performance in horses of all ages. In adult horses and older foals, bronchopneumonia most often develops secondary to colonization by opportunistic bacteria from the upper respiratory tract and oral cavity into the lower airways with Streptococcus equi subsp zooepidemicus remaining the most frequently isolated bacterium (1). In nursing and weanling foals, bronchopneumonia caused by the primary respiratory pathogen Rhodococcus equi, is of worldwide significance (2–4). Antimicrobial medications represent the mainstay of treatment for bronchopneumonia in horses, regardless of the pathogen (1, 2). Unfortunately, antimicrobial resistance is an ever-growing global concern and resistance of bacterial pathogens associated with equine pneumonia, including Streptococcus equi subsp zooepidemicus and Rhodococcus equi, is reported (5–8). Concern of new and evolving antimicrobial resistance patterns, including multi-drug resistance, has also been raised for these pathogens (6). For example, antimicrobial and multi-drug resistance has markedly increased for Rhodococcus equi over the past decade (3, 4). Novel therapies are needed to reduce antimicrobial use and/or improve therapeutic potential of existing antimicrobials for treatment of bacterial bronchopneumonia in horses.
Traditionally, platelets have been recognized for their role in hemostasis and wound healing. More recently they have received greater recognition for their contribution to multiple and diverse regenerative, immunologic, and anti-inflammatory processes (9). Many of these non-hemostatic functions are derived from biologically active molecules stored and released in platelet α granules upon platelet activation. These bioactive molecules include growth factors as well as immunologic, anti-inflammatory, and antimicrobial molecules concentrated at supraphysiologic levels in platelet derived biotherapeutics, platelet lysate (PL) and platelet-rich-plasma (PRP) (10–13). Both PL and PRP have received increased recognition in human and veterinary medicine due to their anti-inflammatory, immunomodulatory, and antimicrobial properties (10–16). The composition, processing and disease modifying effects of PRP and PL in veterinary medicine have been reviewed recently (14, 17, 18). PL is prepared by activating platelets in PRP or concentrates obtained through plateletpheresis followed by filtration to remove platelet membranes (10, 19). Lysis of the platelets during preparation of PL releases the bioactive molecules and confers additional advantages over PRP, including preparation as an allogenic (vs. autologous), acellular product that can be frozen and stored for use on an as-needed basis (10, 12, 19). Furthermore, pooled PL (i.e., derived from platelet concentrates from multiple horses) demonstrates both reduced variability and increased concentrations of these bioactive molecules (10, 12).
Therapeutic investigation of PL, as well as PRP, in veterinary medicine has focused on their anti-inflammatory and regenerative potential for musculoskeletal injury as well as bacterial infections (10, 20). It is through its anti-inflammatory and antimicrobial potential that PL may offer promise for the treatment of respiratory disease in horses. Bioactive molecules considered important for anti-inflammatory and immunomodulatory activity of PL include various cytokines, chemokines, and growth factors important in mitigating inflammatory cell recruitment and dendritic cell response (9). Peptides and small proteins present in PL with potential antimicrobial activity include defensins, thrombocidins, thymocin β4, RANTES, complement precursors, and kinocidins, as well as oxygen metabolites (9, 11, 21). While the exact mechanisms directing antimicrobial activity of PL are ill-defined, it appears to demonstrate primarily bacteriostatic activity against a variety of gram negative and positive bacterial organisms including those of direct relevance to respiratory disease in horses, such as S. equi subsp zooepidemicus (10–12) and may also enhance activity of antimicrobials when administered concurrently (20).
Limited information is available regarding the therapeutic potential of PL or other platelet derived biotherapeutics for the treatment of respiratory disease (22). Experimental studies in mice administered intraperitoneal PL demonstrated improved lung vascular and alveolar regeneration post-pneumonectomy as well as reductions in vascular damage after LPS challenge and in a separate study, inhibition of airway hyperresponsiveness and airway eosinophilia in a murine asthma model (23, 24). Evaluation of PL for the treatment of musculoskeletal pathology demonstrates that local administration at the affected site is important for therapeutic efficacy (12, 14, 25–27). While intrabronchial instillation of platelet rich plasma (PRP) has been described in horses diagnosed with equine asthma (28) and exercise induced pulmonary hemorrhage (29), nebulized administration could provide a superior and non-invasive method to deliver PL directly to the lower airways in horses suffering from bronchopneumonia or other respiratory diseases. A single study demonstrated that nebulization of PRP was well-tolerated in a small group of humans suffering from inhalation lung injury (30). A separate ex vivo study suggests that growth factor concentrations and cell growth in culture containing human PL are maintained after nebulization (22).
The impact of nebulization on protein stability and bioactivity of protein molecules has been documented (31) but varies according to type of nebulizer and specific protein content of a solution. Given the complex protein composition of PL and unknown species related differences, evaluating species-specific and nebulizer-specific impacts on protein composition and bioactivity for PL is essential. Furthermore, given the viscous nature of PL (19) determining whether nebulization generates particles of suitable size to reach the lower airways is of direct clinical relevance. Thus, this pilot study serves as an important first step toward determining the potential clinical application of nebulization of equine PL for treatment of bacterial bronchopneumonia in horses. Our specific objectives were to characterize particle size and flow rate of a single pooled preparation of equine PL nebulized using a commercially available equine mesh nebulizer (Flexivent®), and to also evaluate and compare growth factor, cytokine, and protein composition of PL as well as antimicrobial activity against S. equi subsp zooepidemicus, R. equi, and E. coli, before and after nebulization. We predicted that clinically relevant flow rates (0.5–1 mL/min) and aerosolized particle sizes (< 10 μm) would be achieved, and that PL growth factor, cytokine and protein composition and antimicrobial activity would remain stable over the process of nebulization.
2 Materials and methods
2.1 Manufacture of pooled PL
Pooled equine PL was manufactured at the University of Georgia (JF Peroni laboratory, IACUC approval #A2018 01-013) as previously described (12). Briefly, one liter of platelet concentrates obtained via plateletpheresis from three mixed-breed, healthy adult university-owned horses (aged 4–14 years) were subject to two freeze-thaw cycles to lyse the platelets and release their contents (28). Each was then centrifuged three times, filtered through a Falcon cell strainer (40 μm) and Stericup sterile vacuum filter with cellulose acetate membrane (0.45 μm) to remove cellular debris and then pooled and centrifuged (30 min × 2,800 g) to insure thorough mixing. An aliquot of the pooled product was subjected to fungal and bacterial culture and the remainder stored at −80°C as 50 mL aliquots.
2.2 Nebulization of PL
For experimental purposes, 50 mL of PL was thawed in a 37°C water bath and then centrifuged for 30 min at 2,800 × g. A single 10 mL aliquot was separated and stored at −80°C for later measurement of flow rate and aerosol particle size. The remainder of the PL was divided into aliquots for pre- and post-nebulization comparisons (Figure 1). Aliquots that were not to be subjected to nebulization were stored at −80°C until analysis for comparison with post-nebulized samples. Aliquots allotted for nebulization were nebulized within 2 months of freezing and all further analysis was performed within a 9-month period. Nebulization of PL using a commercially available equine mesh nebulizer (Flexineb E3®, Union City, TN, USA) equipped with a standard (gray, Standard Flow Medication) cup was performed within a laminar flow hood and under sterile conditions. Nebulized PL condensate was collected into a sterile conical tube, aliquoted, and stored at −80°C for later analysis. A single aliquot underwent nebulization for cytokine, growth factor, and antimicrobial peptide measurement, three aliquots underwent separate nebulization for proteomic analysis, and a single aliquot underwent nebulization for in vitro bacterial growth curve analysis. A single medication cup was used for the study. According to manufacturer instructions (Nortev Limited, Claregalway, Galway, Ireland) the cup was cleaned between each nebulization and was additionally cold sterilized prior to nebulization of samples for growth curve analysis.
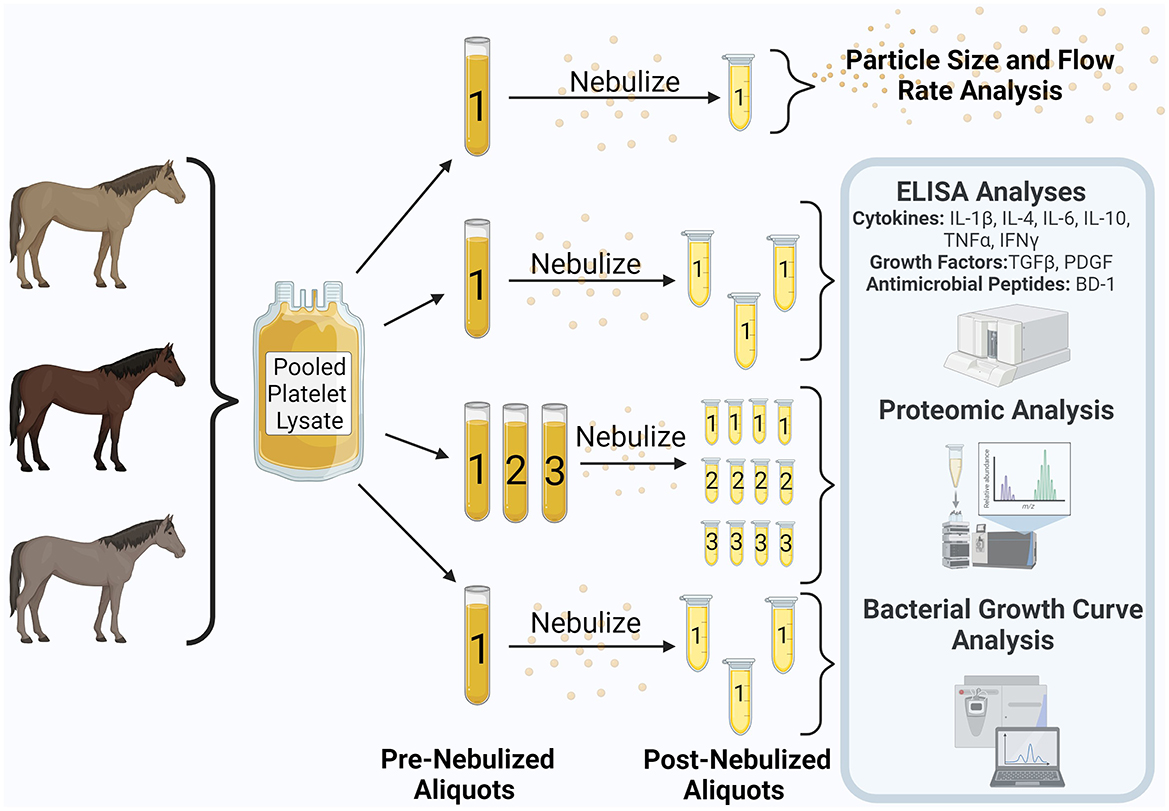
Figure 1. Study design. Equine pooled platelet lysate was aliquoted into pre-nebulization samples. Samples underwent nebulization and were collected into additional aliquots. Analyses contained within the blue shaded box were performed on both the pre- and post-nebulized samples. This figure was created using Biorender.com.
2.2.1 Flow rate and aerosol particle size measurement
Flow rates and aerosol particle size were measured by the manufacturer using a spray particle and spray droplet size analyzer (Spraytec; Malvern Panalytical Ltd, Malvern, UK) equipped with a standard medication cup (32, 33). For measurement of flow rate, saline was used as the standard for comparison. Particle size measurements were taken every 3 s over a 60 s interval and reported as minimum and maximum observed particle size, volume mean diameter 50 (DV50), and percent measured particles below 5 and 10 μm, respectively.
2.3 Measurement of cytokines, growth factors and Beta Defensin-1
Concentrations of IL-1β, IL-4, IL-6, IL-10, TNF-α, IFN-γ were measured on single pre- and single post-nebulized PL aliquots using a multiplex bead immunoassay (Equine Cytokine Magnetic Bead Panel, Millipore Sigma, Burlington, MA, USA) according to the manufacturer's instructions (34). All samples were analyzed in triplicate using a 96-well platform. A minimum bead count of 50 for each cytokine was acquired for data analysis. Data were analyzed using xPONENT® Immunoassay Curve Fitting Software from Luminex, using the MAGPIX® analyzer (Luminex Corporation, Austin, TX, USA). For each cytokine, the average concentration across triplicate measurements was calculated and used to calculate percent increase or decrease between pre- and post-nebulized PL samples.
ELISA analysis was performed on single pre- and single post-nebulized PL aliquots using commercially available kits previously validated in horses for TGF-β1 and PDGF (Human PDGF-BB DuoSet DY220 and Human TGF-β1 DuoSet, DY240E, R&D Systems, Minneapolis, MN) and for Beta Defensin-1 (BD-1) antimicrobial peptide (Nori® equine Beta-Defensin1, Genorise Scientific, INC, Glen Mills, PA, USA). Standards were provided for all kits and used to prepare standard curves according to manufacturer instructions (35–37). Samples were performed in triplicate (TGF-β1, BD-1) or duplicate (PDGF) and were diluted to 1:20. Average concentrations across replicate measurements were calculated and used to calculate percent increase or decrease between pre- and post-nebulized PL samples.
2.4 Proteomic analysis
Proteomic profiles on the single pre- and three post- PL samples were evaluated using high resolution LC-MS. The PL samples underwent protein digestion for protein identification or ultrafiltration for endogenous peptide identification prior to mass spectrometric analysis. For all methods for protein identification, unless otherwise specified, chemicals were purchased from Sigma. Optima grade LC-MS mobile phases were purchased from Fisher Scientific (Waltham, MA, USA). Ultrapure water (18.2 MΩ-cm) was obtained from a Barnstead Nanopure system.
2.4.1 Sample preparation with digestion for total protein identification
Platelet lysate samples were denatured in a buffer of 4 M GuHCl in 100 mM TEAB. Next, 10 mM TCEP and 40 mM CAA (TCI) were added, and the samples were heated to 95°C for 10 min to promote reduction of disulfide bonds and alkylation of cysteine residues. The proteins were then precipitated with chloroform and methanol, and the resulting pellets were briefly air dried. The protein pellets were dissolved in 2 M GuHCl in 50 mM TEAB and digested for 1 h with 2.5 μg LysC (Fujifilm Wako Chemicals, Richmond, VA, USA). After the pellets were fully dissolved, a BCA assay (Pierce, Fisher Scientific) was used to check protein concentration. Additional LysC was added to a final concentration of 1:100 w/w (enzyme:substrate), and samples were incubated at 25°C for 3 h. The samples were further diluted with 50 mM TEAB to GuHCl < 1M and digested overnight with trypsin (Pierce, Fisher Scientific) 1:50 w/w at 37°C. On the following day, the digested peptides were acidified with 10% TFA, desalted with StageTips (29), and dried in a vacuum centrifuge. The peptides were suspended in 5% ACN in 0.1% FA to a final concentration of 200 ng/μL, as determined by a colorimetric peptide BCA assay (Pierce).
2.4.2 Sample preparation with ultrafiltration for endogenous peptide identification
Platelet lysate samples were similarly denatured as described above for protein digestion, except that after alkylation of cysteine residues the samples were cooled and passed through Amicon 0.5 mL 30 K MWCO ultra centrifugal filters for a total of 20 min at 14,000 × g. The filters were washed with 200 μL of water and centrifuged for another 20 min at 14,000 × g; this wash was added to the initial flow-through. The peptides and small proteins that passed through the ultra-centrifugal filters were acidified with 10% TFA, desalted with StageTips (38), and dried in a vacuum centrifuge. The peptides were then suspended in 5% ACN in 0.1% FA to a final concentration of 500 ng/μL, as determined by a colorimetric peptide BCA assay (Pierce).
2.4.3 LC-MS analysis digested lysate
Each sample (400 ng) was analyzed by an UltiMate 3000 RSLCnano system coupled to an Orbitrap Fusion mass spectrometer (Thermo Fisher, Waltham, MA, USA). The LC was equipped with a μPAC 315 μm × 50 cm C18 column (Thermo Fisher) and operated at a flow rate of 300 nL/min with mobile phases of 0.1% FA (A) and 0.1% FA in 80% ACN (B). The peptides were separated with a gradient of 12–36% B over 120 min, which was followed by column cleaning and equilibration. The mass spectrometer was run in positive mode for data dependent acquisition. Precursor scans using the orbitrap analyzer were collected at 120 k resolution with a scan range of 300–2,000 m/z (max IT 100 ms, normalized AGC target 50%). Additional settings included exclusion of charge states ≥8 and a dynamic exclusion window of 60 s. MS2 spectra were collected in the ion trap in rapid mode after CID fragmentation (35% fixed energy). An isolation window of 1.6 m/z was used with a total DDA cycle time of 3 s.
2.4.4 LC-MS analysis filtered lysate
Each sample (500 ng) was then analyzed by an UltiMate 3000 RSLCnano system coupled to a Q Exactive HF-X mass spectrometer (Thermo Fisher). The LC was operated at a flow rate of 300 nL/min with mobile phases of 0.1% FA (A) and 0.1% FA in 80% ACN (B). The peptides were separated with a 25 cm Acclaim PepMap 100 C18 column (2 μm particle size, 75 μm ID) maintained at 50°C over the course of the run. The gradient was 5–35% B over 90 min and then 35–50% B over 5 min, followed by column washing and equilibration. The mass spectrometer was operated in positive polarity with MS1 scans from 350 to 2,000 m/z at 120 k resolution (50 ms max IT; 3e6 AGC) followed by HCD fragmentation (30 NCE) of the top 15 most abundant ions. MS2 scans were collected at 15 k resolution with an isolation window of 1.0 m/z, a maximum IT of 30 ms, and an AGC target of 5e4. Unassigned and singly charged ions were excluded from selection for MS2, and the dynamic exclusion window was 45 s.
2.4.5 Protein identification and quantification of digested and filtered lysate
The raw LC-MS data was processed with Byonic v5.3.5 (Protein Metrics, Cupertino, CA, USA) implemented in Proteome Discoverer v2.4.1.15 (Thermo Fisher) to identify the proteins; label-free quantitation (LFQ) was done with Minora feature finder and the precursor ion quantifier nodes in Proteome Discoverer. Settings for the Byonic search included a precursor mass tolerance of 10 ppm and a fragment mass tolerance of 0.5 Da for digested lysate and 20 ppm for filtered lysate. For digested lysate, tryptic digest with a maximum of two missed cleavages was specified along with a fixed modification for cysteine carbamidomethylation and variable modifications of protein N-terminal acetylation, methionine oxidation, and asparagine/glutamine deamidation. For filtered lysate, no enzyme was specified for digestion, and a fixed modification for cysteine carbamidomethylation and variable modifications of protein N-terminal acetylation and methionine oxidation were added to the searches. The LC-MS data were deposited with the ProteomeXchange Consortium via the jPOST partner repository (https://jpostdb.org) with the dataset identifier PXD055023.
For both digested and filtered lysate, searches were done against the Uniprot Equus caballus reference proteome (69,389 entries; downloaded October 2023). Using a reverse decoy database strategy, the false discovery rate (FDR) was set to 1%. For LFQ, a maximum RT shift of 10 min was allowed for mapping features across files for digested lysate and 5 min was permitted for chromatographic alignment. For digested and filtered lysate, both unique and razor peptide intensities were used for quantitation, but shared peptides were used for quantitation of only one protein—the protein with the highest number of other identified peptides. Protein abundances were calculated from the sum of their peptide abundances. For filtered lysate, normalization by total peptide amount was performed prior to calculation of protein abundances with the protein abundance ratios determined from the median of all possible pairwise peptide comparisons.
2.5 Bacterial growth curve assay to determine inhibition of bacterial growth
Bacterial growth curve analysis was performed on Rhodococcus equi, Escherichia coli (ATCC®25922TM, Manassas, VA, USA), and Streptococcus equi subsp zooepidemicus. Clinical isolates included a Rhodococcus equi strain susceptible to standard antimicrobials (WT-R. equi) and a multidrug resistant (MDR-R. equi) strain (both provided by L. Huber laboratory, Auburn University, Auburn, AL, USA) as well as an equine respiratory isolate for Streptococcus equi subsp zooepidemicus (provided by the Clinical Microbiology Laboratory, Auburn University College of Veterinary Medicine, Auburn, AL, USA). All bacteria were cultured in BBL Brain Heart Infusion broth (BHI, Becton Dickson, Franklin Lakes, NJ, USA) at 37°C with shaking (200 rpm). The optical density of the bacterial solution was measured at 600 nm (OD600) using a BioRad SmartSpec Plus spectrophometer. The OD600 was adjusted to 0.02, corresponding to ~107 CFU/mL (12). The required number of 1 mL aliquots were removed from each tube of bacterial solution and centrifuged at 3,000 rpm for 10 min to pellet the bacteria. Pellets were then washed with PBS and 500 μl of the appropriate treatment (50% pre-/post-nebulized PL diluted in BHI) or positive control (50% BHI diluted in PBS) was added. Corresponding negative controls (no bacteria added) for both treatment groups and 50% BHI were included for analysis. All treatments were prepared in duplicate and were transferred to a 48-well plate to be analyzed by an automated plate reader (Synergy HT, BioTek, Shoreline, WA, USA) measuring OD600 (corresponding to the number of bacteria in each well) every 10 min for 30 h. Blank no-bacteria were used to provide OD600 values for treatments only. Bacterial growth inhibitor parameters [maximum growth (μmax); carrying capacity (K)] were calculated for Streptococcus equi subsp zooepidemicus, Escherichia coli, and Rhodococcus equi (WT-R. equi and MDR-R. equi) using pre- and post-nebulized PL concentrations of 50% (PreN50, PostN50).
2.6 Statistical analysis
In accordance with the limited number of pre- and post-nebulized replicates used for analysis in this pilot study, descriptive statistics were primarily used to present the data. For cytokine, growth factor, and Beta Defensin-1 the average concentrations across duplicate or triplicate measurements are reported and were used to calculate the percent increase or decrease between pre- (n = 1) and post-nebulized (n = 1) PL samples. Descriptive statistics were also used to present proteomics data and for comparisons between pre- (n = 1) and post-nebulized (n = 3) lysate samples. A cutoff ratio of >1.5 or < 0.5 was used to suggest meaningful differences in protein abundance between pre- and post-nebulized platelet lysate. Gene ontology (GO) analysis was performed to categorize platelet lysate according to GO-biological processes terms, GO-cellular components terms, and GO-molecular functions terms using the DAVID (Database for Annotation, Visualization, and Integrated Discovery) functional annotation tool (https://david.ncifcrf.gov/). For analysis of the bacterial growth curve assay, the R package “growth-rates” was used to estimate the maximum growth rate during exponential growth (μmax) and carrying capacity (K) from OD600 values. Linear modeling was used to evaluate the effect of conditions on μmax and K and multipair wise comparisons between conditions were performed using post-hoc tests (Tukey HSD test). Statistical significance was set at p ≤ 0.05. Normality of data was assessed by plot visualization. Statistical analyses were performed using RStudio (version 2023.12.1+402).
3 Results
3.1 Platelet lysate nebulized flow rate and aerosol particle size
The measured flow rate of PL during nebulization was 0.8 ml/min compared to a flow rate of 1 ml/min of saline. Minimum and maximum aerosolized particle diameters were 0.10 μm and 2,500 μm. The DV 50 was 4.991 μm, with 52% of aerosolized particles ≤ 5 μm in size and 85% of particles ≤ 10 μm in size.
3.2 Cytokine, growth factor and antimicrobial peptide concentrations in pre- and post-nebulized platelet lysate
Measured concentrations in the pre- (n = 1) and post-nebulized (n = 1) PL samples for cytokines, TGF-β1, PDGF, and BD-1 along with percent change in concentrations between pre- and post-nebulized samples are reported in Table 1. Relative to pre-nebulization, all measured concentrations were decreased in the post-nebulized sample, except for IL-4. These differences were < 10% for all measured variables excluding IL-1β (22%), TGF-β1 (20%), and BD-1 (16%).
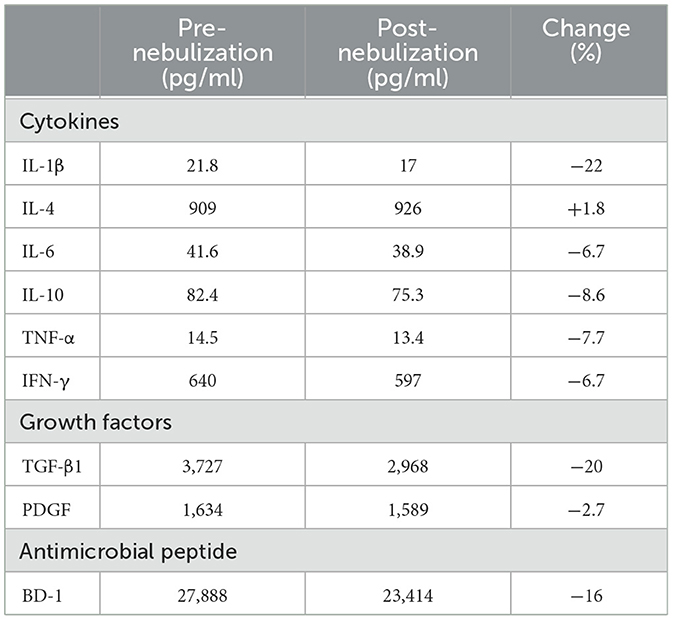
Table 1. Pre- (n = 1 aliquot) and post-nebulized (n = 1 aliquot) platelet lysate concentrations and respective percent difference between the two conditions for cytokines (IL-1β, IL-4, IL-6, IL-10, TNF-α, IFN-γ), growth factors (TGF-β1, PDGF), and Beta Defensin-1 (BD-1).
3.3 Proteomic analysis
3.3.1 Protein identification and characterization
A total of 456 proteins were identified in the pre- and post-nebulized platelet lysate pools using protein digestion and endogenous peptide identification through low molecular weight cut-off (MWCO) filtration. The number of proteins identified through digestion, MWCO filtration and those found common to both methods are presented in Figure 2 with a list of identified proteins available in Supplementary Table 1. Qualitatively, the most common groups of proteins (>20% of total proteins) identified across both methods include (in decreasing order of prevalence): complement system proteins, Ig like domain proteins, actin related proteins, tubulin related proteins, apolipoproteins, glycoproteins, coagulation factors, serpin family proteins, immunoglobulin component proteins, growth factors, and α-1 antitrypsin related proteins.
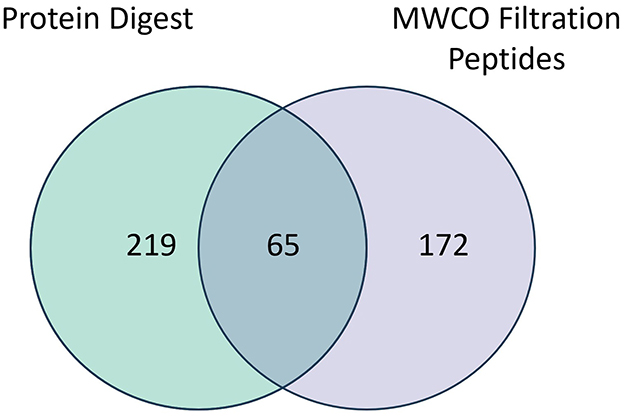
Figure 2. Venn diagram depicting overlap in proteins identified through digestion or filtration (MWCO) of pre-nebulized (n = 1) and post-nebulized (n = 3) platelet lysate samples.
Go Analysis on the digested lysate (Figure 3A) identified GO terms for 252/284 proteins categorized according to molecular function (MF; n = 72 terms), 255/284 proteins categorized according to biological processes (BP; n = 166 terms) and 257/284 proteins categorized according to cellular content CC; n = 75 terms). Similarly, Go Analysis on MWCO lysate (Figure 3B) identified GO terms for 216/237 proteins categorized according to molecular function (MF; n = 55 terms), 225/237 proteins categorized according to biological processes (BP; n = 155 terms) and 227/237 proteins categorized according to cellular content (CC; n = 74 terms). The top 10 terms for each category based on P-value are presented in Figures 3A, B. The most common biological processes identified for PL included complement activation, blood coagulation, acute phase response, fibrinolysis, and endopeptidase regulation among others.
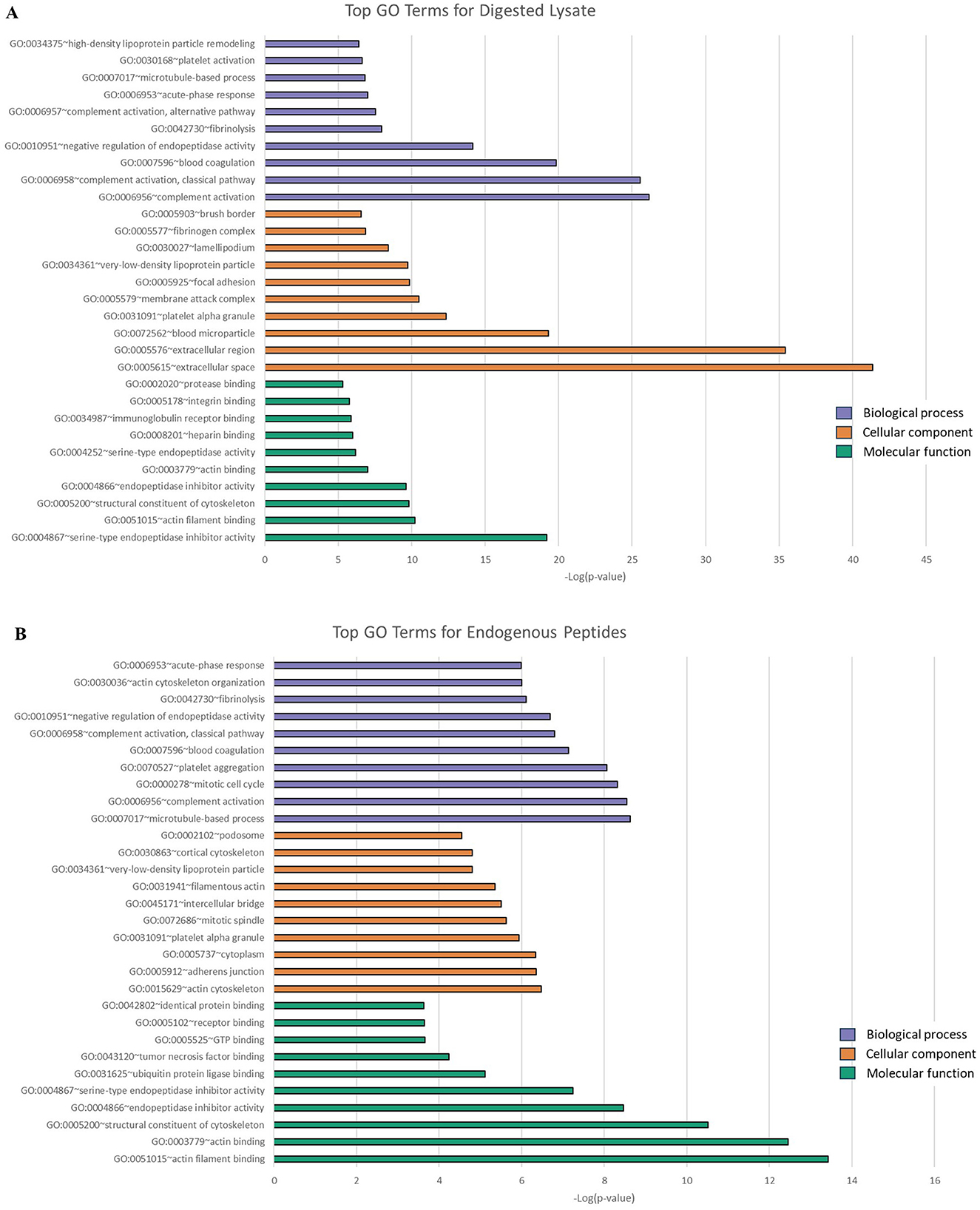
Figure 3. Categorization of digested (A) and filtered (B) equine platelet lysate by gene ontology (GO) according to the top ten GO terms for biological processes (purple), cellular components (orange), and molecular functions (green) using DAVID. The top ten terms chosen according to most significant P-values. Categorization based on pooled data from pre-nebulized (n = 1) and post-nebulized (n = 3) samples.
3.3.2 Minor differences in protein composition were identified between pre- and post-nebulized platelet lysate for both digested and filtered lysate
A total of 281/284 protein groups identified through digestion were common to the pre- and post-nebulized PL samples. No proteins were unique to the pre-nebulized lysate. Three proteins (Ig-like domain containing protein and two Actin related proteins) were exclusive to post-nebulized samples but were not uniformly expressed across all samples. A total of 2,890 quantifiable peptides were identified through filtration representing 237 protein groups found in both the pre- and post- nebulized PL samples. Fourteen peptides were unique to the pre-nebulized sample. One of these, beta defensin 3, was found only in the pre-nebulized sample with the remaining peptides corresponding to proteins found in both pre- and post-nebulization samples. Thirty-three peptides were unique to the post-nebulized samples. These peptides corresponded to seven proteins exclusive to post-nebulized samples, with none uniformly expressed across all samples.
3.3.3 Minor differences in protein and peptide abundance were identified between pre- and post-nebulized platelet lysate for both digested and filtered lysate
For the 281 proteins common to pre- and post-nebulized samples as identified through digestion, abundance was qualitatively similar between the pre- and post-nebulized samples. The median (IQR) abundance ratio for pre-nebulized sample/pooled post-nebulized samples was 1.034 (5.31–0.05). Using cutoff ratios of ≥1.5 or ≤ 0.5 to suggest differences in protein abundance, 7/281 proteins demonstrated increased abundance in the pre-nebulized sample and 12 proteins demonstrated increased abundance in the post-nebulized samples (Table 2). Differences in peptide abundances were detected between the pre- and post-nebulized samples with 54/2,890 quantifiable peptides significantly increased in abundance in the pre-nebulized sample (relative to post-nebulized) and 142/2,890 quantifiable peptides significantly decreased in the post-nebulized samples (relative to pre-nebulized; Supplementary Figure 1).
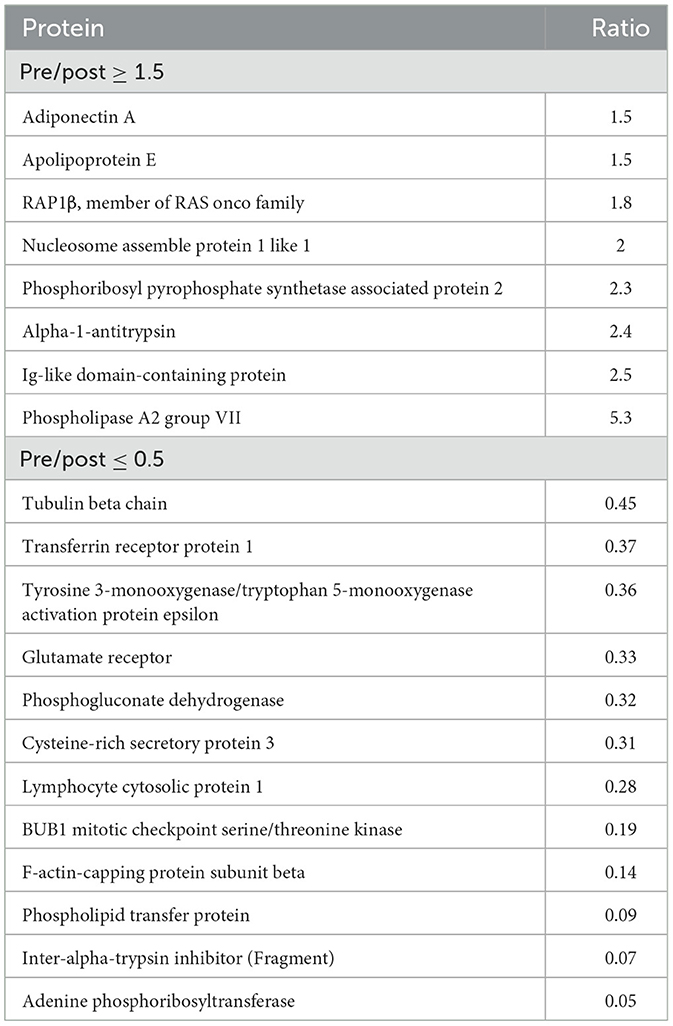
Table 2. Proteins identified through digestion of platelet lysate that are increased (≥1.5) or decreased (≤0.5) in abundance in the pre-nebulized sample as compared to post nebulized samples (n = 3).
3.4 Bacterial growth curve assay
Growth curves and associated carrying capacity (K) and maximum growth rate during exponential growth (μmax) for E. coli, S. zooepidemicus, and R. equi (WT, MDR) are depicted in Figures 4–6.
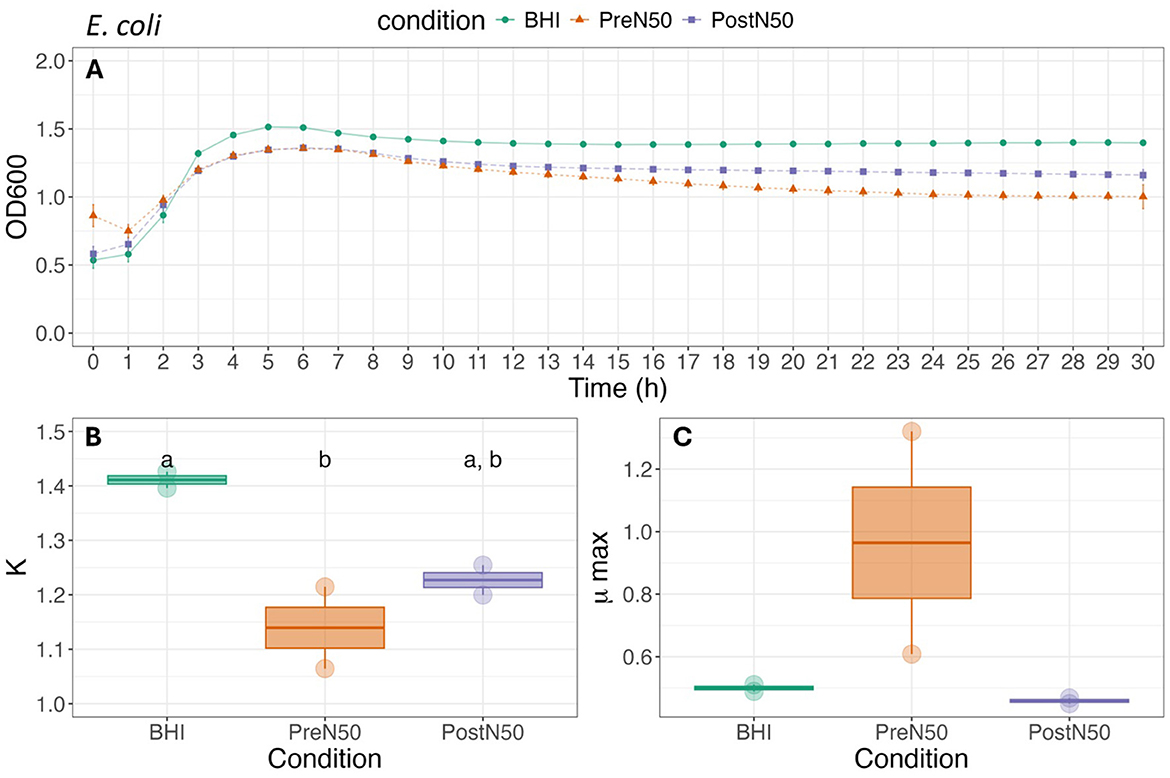
Figure 4. Growth curve (A), carrying capacity (K, h−1) (B) and maximum growth rate during exponential growth (μmax, OD600) (C) of E. coli under different conditions. For (B, C), different lower-case letters represent statistically significant effect (with BHI as the reference group) or difference among groups at P ≤ 0.05 using linear modeling and post-hoc tests (Tukey HSD test). Single pre- and post-nebulized aliquots used for analysis.
3.4.1 Nebulization does not change the effects of PL on bacterial growth rate parameters of E. coli and S. zooepidemicus
As illustrated in Figure 4, differences in K and μmax of E. coli were not observed between the pre- (PreN50) and post-nebulization (PostN50) treatments. Addition of PreN50 decreased carrying capacity (K) when compared with the BHI control (p = 0.026). However, post-hoc tests revealed that carrying capacity did not differ among groups. No effect of condition (treatment or control) was observed on μmax of E. coli.
As illustrated in Figure 5, differences between the PL treatments were not observed for K or μmax of S. zooepidemicus. The addition of PL decreased carrying capacity (K) of S. zooepidemicus when compared to the BHI control (p = 0.009) for both the PostN50 (p = 0.046) and PreN50 (p = 0.043) treatments. No effect of PL treatment was observed on the μmax of S. zooepidemicus.
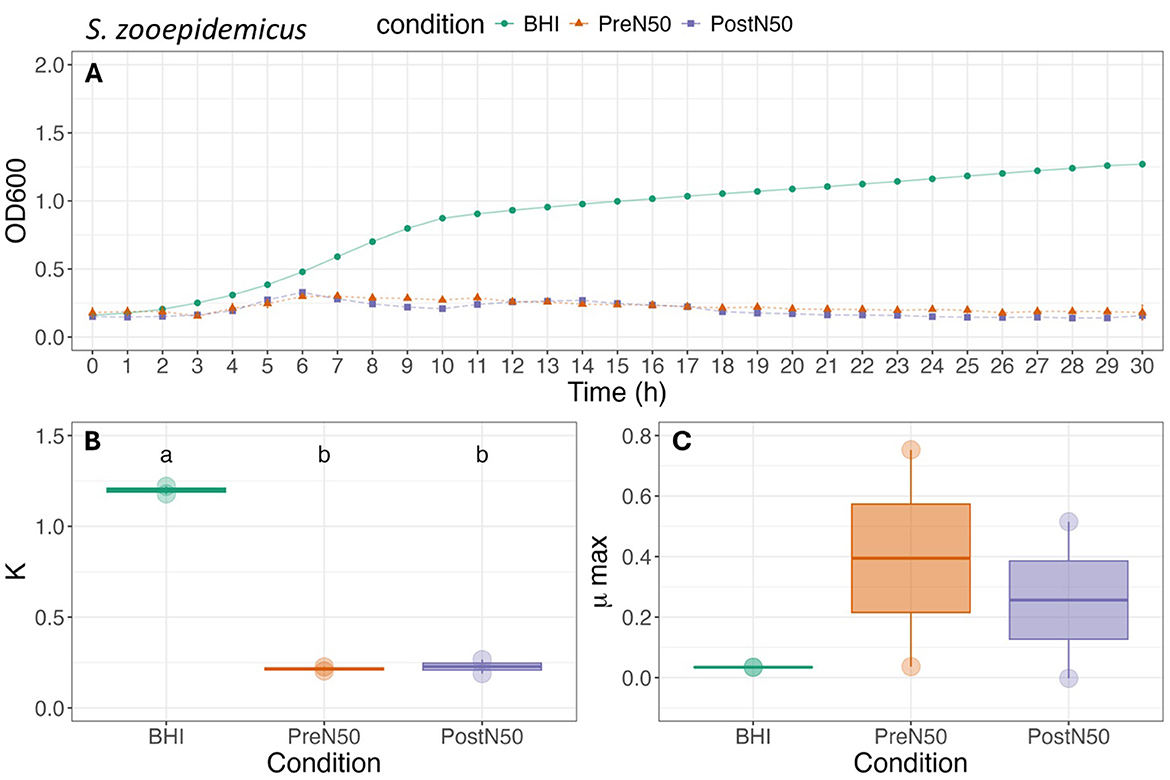
Figure 5. Growth curve (A), carrying capacity (K, h−1) (B) and maximum growth rate during exponential growth (μmax, OD600) (C) of S. zooepidemicus under different conditions. For (B, C), different lower-case letters represent statistically significant effect (with BHI as the reference group) or difference among groups at P ≤ 0.05 using linear modeling and post-hoc tests (Tukey HSD test). Single pre- and post-nebulized aliquots used for analysis.
3.4.2 Pre- and post-nebulized PL effect bacterial growth rate parameters for both strains of R. equi (WT and MDR)
As illustrated in Figure 6, K of WT-R. equi was higher for PostN50 when compared to both the PreN50 treatment and BHI control (p ≤ 0.001). There was no effect of treatment when PreN50 and BHI were compared. For MDR-R. equi, K was higher for both PL treatments when compared to the BHI control (p < 0.001). K was higher in PostN50 compared with PreN50 (p < 0.001).
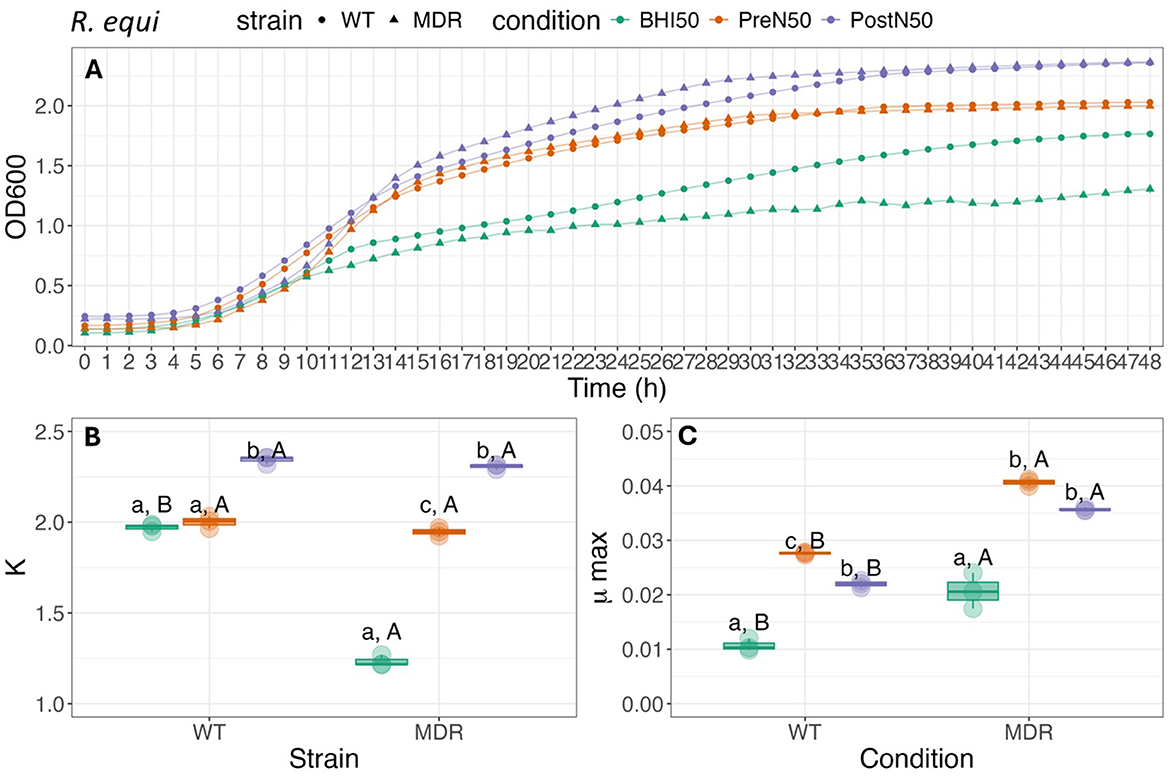
Figure 6. Growth curve (A), carrying capacity (K, h−1) (B) and maximum growth rate (C) during exponential growth (μmax, OD600) of WT-R. equi and MDR-R. equi under different conditions. For (B, C), different lower-case letters represent statistically significant difference among conditions within the same strain; different capital letters represent statistically significant difference between strains for the same condition at P ≤ 0.05 using Tukey HSD test. Single pre- and post-nebulized aliquots used for analysis.
Maximum growth (μmax) of WT-R. equi was higher when grown in PreN50 compared to PostN50 (p = 0.036) and for both PL treatments when compared with BHI (p < 0.001). Maximum growth (μmax) of MDR- R. equi did not differ between PL treatments (p = 0.38). The μmax of both PreN50 and PostN50 was higher compared with the BHI control (p < 0.001).
When the WT and MDR R. equi strains were compared, K was higher for WT-R. equi (p < 0.001) when grown in BHI. Differences between strains in K were not observed for WT-R. equi and MDR-R. equi grown in PostN50 (p = 0.75) or PreN50 (p = 0.25). When comparing between strains, MDR-R. equi had higher μmax compared with WT-R. equi in all media types (p = 0.035, p < 0.001, p < 0.001, for BHI, PreN50, and PostN50, respectively).
4 Discussion
In this present pilot study we evaluated in vitro the feasibility of administering equine platelet lysate using an equine specific vibrating mesh nebulizer. To the best of our knowledge, similar studies have not been performed. Additionally, while not a primary objective, this study is also unique in describing the proteomic profile of pooled equine platelet lysate manufactured via plateletpheresis. In agreement with our study hypotheses, clinically relevant flow rates and particle size distribution were achieved. A limited number of growth factor and cytokines demonstrated a change in concentration >10% and some differences in protein expression were identified in response to nebulization in the proteomic profile. Assessment of antimicrobial activity against E. coli, S. zooepidemicus, and R. equi demonstrated similar effects of equine PL on several bacterial growth inhibition parameters before and after nebulization; however the effect of PL and nebulization on these parameters differed by bacterial species.
Inhalation therapy, including via nebulization, is used with increasing frequency in horses for treatment of equine asthma and pneumonia (39, 40). Achievable flow rates and particle size distribution are important factors to consider when determining the suitability of a medication for nebulization. Considering patient tolerance and compliance, nebulization time for horses should be as short as possible, ideally not exceeding 20–25 min (40). At our measured flow rate of 0.8 ml/min using the Flexineb® nebulizer system equipped with the Standard Flow medication cup, this would allow for delivery of 16–20 mL of lysate. While predicting a therapeutic dose for any inhaled medication can be challenging and remains to be determined for nebulization of PL, these volumes fall within the range of 10–20 ml reported for direct intrabronchial instillation of PRP in adult horses with EIPH (29) or equine asthma (28). Aerosolized particle size distribution is a key factor determining appropriate deposition of an aerosolized medication within the airways and thus its' relevance for use in treating infectious or inflammatory conditions of the lung (39, 40). In this study, 52% of aerosolized PL particles were measured to be ≤ 5 μm and 85% of particles to be ≤ 10 μm in size. Aerosolized particles 1–5 μm in size are deposited in the smaller bronchioles and alveoli and are generally considered therapeutic aerosols (31, 41). Particles 6–10 μm may still hold relevance for treatment of conditions such as pneumonia because they are deposited in the larger bronchioles and bronchi (41). Thus, although factors beyond particle size can influence aerosol penetration in a live animal, our results suggest that nebulized PL could reach targeted areas for treatment of pneumonia.
Aerosol generation for mesh nebulizers such as Flexineb®, occurs when high frequency vibrations drive liquid through small pores of a metallic mesh membrane located within a medication cup (31, 39, 42). The flow rate and distribution of particle sizes are primarily determined by pore size and the penetration of solution through the pores (31, 39). Alternative nebulizer systems used in horses include jet (compressor) and ultrasonic nebulizers. The flow rate and particle size distribution reported in the present study should not be assumed to be the same for other types of nebulizers due to differences in function or performance among these systems (31, 39, 42). Regardless of nebulizer type, consideration must be given to the viscosity of the solution undergoing nebulization. Subjectively, equine PL is viscous. This is not unexpected given that the viscosity of equine plasma is greater than other species and is approximately 1.5 times that of saline (19, 43). For mesh nebulizers, viscous solutions can clog pores in the mesh of the medication cup, reducing flow rate and potentially altering particle size distribution (31, 33, 39). The flow rates reported in the present study were achieved using a Standard Medication cup which is most often utilized in clinical practice. Delivery of larger volumes of PL in a clinical setting could potentially reduce effectiveness of nebulization due to viscosity. A medication cup with increased pore size (Fast Delivery, Flexineb®) is available to accommodate more viscous solutions. Unfortunately, while flow rate is improved, aerosolized particle size is increased (33, 39), potentially impacting deposition within the lung. Lyophilization of equine platelet extracts appears to preserve biological activity while allowing for reconstitution in water (44) and could be evaluated in future studies for suitability for nebulization.
For this study, we chose to evaluate concentration changes in response to nebulization for select growth factors (PDGF, TGF-β1), pro- (IL-1β, IL-6, TNF-α, IFN-γ) and anti-inflammatory (IL-4, IL-10) cytokines, and the antimicrobial peptide (Beta Defensin-1), that could hold potential relevance for the use of PL in treating bacterial pneumonia. Variability across studies in reported composition and activity of these bioactive molecules within equine PL is most likely due to differences in experimental design as well as inherent differences among donor populations (10, 13, 45, 46) and in methods of processing and preparation of PL (10, 12, 47–49). Additionally, while the impact of storage time at −80°C on concentrations of growth factors or other proteins has not been described for equine PL, variable changes over time in concentration of some growth factors have been reported for equine and human PRP stored at −80°C (50, 51). Overall, growth factor and cytokine concentrations in this study were slightly lower or comparable to those previously reported for equine PL products (13, 48, 49, 52, 53). Concentrations of all measured effector molecules except IL-4 were decreased after nebulization. It is unknown whether these changes, if repeatable, would carry clinical significance. The pro-inflammatory cytokine, IL-1β, demonstrated a 20% reduction post-nebulization. Increases in IL-1β gene expression have been detected in BAL fluid in response to inflammatory conditions, including in horses with severe equine asthma (54, 55) and humans with community-acquired pneumonia (56). Interestingly, nebulization of human platelet lysate via jet nebulizer (22), resulted in mild increases in PDGF and TGF-β1 concentrations and this was attributed to water evaporation during the nebulization process. Nebulization of a larger number of replicates or from different sources of PL would have provided a more robust assessment regarding whether the concentration changes observed in this study represent true degradation of PL in response to nebulization. Additionally, cell culture studies could have allowed for evaluation of potential changes in cytokine or growth-factor mediated bioactivity after nebulization.
Proteomic analysis was performed on PL before and after nebulization to provide a more comprehensive assessment regarding the impact of nebulization on protein composition. Thermal and mechanical stresses that occur during the process of nebulization can potentially alter the stability of macromolecules and lead to protein degradation particularly within high protein solutions, such as PL (31). While these stresses are of greatest concern with ultrasonic nebulization, they may still be of relevance to mesh nebulization (31). Complementary methods of protein digestion and low molecular weight filtration were chosen for proteomic analysis in this study with the goal to improve detection of smaller proteins and peptides that could be missed with digestion alone. Proteomic profiles are lacking for equine PL but have been utilized to highlight differences in human PL according to methods of preparation (57–61). Variability in the methodological approach to proteomic analysis makes it difficult to draw comparisons not only with the proteomic profile described in this study, but also among the referenced human PL studies. Nevertheless, some overlap in the most common biological processes identified through GO analysis exists between this study and what was reported by Le et al. (57) for human PL.
Observed differences in abundance and expression of proteins and peptides between pre- and post-nebulized PL samples were quite small. The peptide, beta defensin 3, was the only peptide (or protein) uniquely expressed in pre-nebulized platelet lysate. Interestingly, this peptide could be of relevance for treatment of bacterial pneumonia. Beta defensin 3 is one of a group of low molecular weight (< 10 kDa) cationic peptides with constitutive or induced expression and that demonstrate antimicrobial and immunomodulatory activity in a variety species (60–62). While it is possible this peptide was lost or degraded during the process of nebulization, given the small size (5 kDa), it is also possible that lack of detection in the post nebulization samples is secondary to sample processing prior to digestion or LC/MS analysis. This may be a more reasonable explanation as thymosin β-4, another antimicrobial peptide of similar molecular weight, was identified in pre- and post-nebulized samples, and the related peptide, beta defensin 1, was identified using ELISA in similar concentrations in both pre- and post-nebulized samples.
The impact of nebulization on the antimicrobial bioactivity of equine PL was evaluated in this study through use of a bacterial growth curve assay. E. coli, S. zooepidemicus and R. equi (WT and MDR) strains were chosen because of their relevance to bacterial pneumonia in adult and juvenile horses (1, 2, 4). Although 100% platelet lysate would most likely be used in a clinical setting (12, 63–65), for this study PL was diluted to 50% in BHI to allow distinction of growth inhibition due to direct effects of PL vs. due to a lack of nutrients, as has been demonstrated when >60% PL is used in growth inhibition assays (12, 16). Overall, any differences in antimicrobial activity of PL observed in this study and associated with nebulization were small and largely limited to R. equi. For both the WT and MDR R. equi strains, growth rate parameters increased with addition of PL irrespective of nebulization. This effect was more prominent for maximum growth rate (μmax) with pre-nebulized PL and for carrying capacity (K) with post-nebulized PL.
The antimicrobial activity of PL and other platelet derived products is a complex process that has been demonstrated for a variety of Gram negative and positive bacteria (10, 12, 20, 21, 46, 66–70). The effects of PL on in vitro bacterial growth for S. zooepidemicus observed in this study is consistent with a previous study that demonstrated equine PL antimicrobial activity against S. zooepidemicus biofilms (10). In our study, growth inhibition of E. coli when exposed to PL was minimal. The antimicrobial activity of equine PL against E. coli is inconsistently reported across studies (10, 12, 16). Using PL prepared as described in this study, Gordon et al. (12) demonstrated reduced growth rate, delayed growth, and lower bacterial yield for E. coli when exposed to similar concentrations of PL. Explanations for the discrepancy between our results and those reported by Gordon et al. (12) could include inherent differences in susceptibility between strains of E. coli or in expression of specific bioactive molecules between PL batches, or potentially in differences in experimental protocols.
To the best of our knowledge, this study is the first to investigate antimicrobial activity of equine PL against R. equi. The overall effect of PL increased R. equi growth relative to BHI in this study were unexpected but should be interpreted with caution as they do not necessarily indicate that PL supports growth of R. equi. Detailed in vitro characterization of the antimicrobial effects of PL on R. equi was beyond the scope of this pilot study but should be performed to determine whether PL is contraindicated with R. equi pneumonia. As demonstrated for other bacteria, the specific antimicrobial effects of PL can be unique to bacterial strain both within and across studies and can potentially vary according to methods used for preparation of PL and evaluation antimicrobial activity or potentially other experimental factors (46). For example, the concentrations and activity of cytokines, growth factors and peptides expressed in PL and important for antimicrobial and related immunomodulatory activity can be influenced by donor pool (13), number of freeze thaw cycles (47, 57–59), and other methods of preparation such as fractionation to increase concentration of low molecular weight cationic peptides (20). Improved antimicrobial activity has also been demonstrated through co-administration of PL and an antibiotic (20). Furthermore, in vitro evaluation does not account for potential indirect effects of growth factors, cytokines, or antimicrobial peptides within PL on cellular and immunomodulatory actions that could play critical roles in influencing antimicrobial activity against R. equi through promoting phagocytosis, T-cell stimulation, or activation and recruitment of other immune cells (62, 71, 72).
There are limitations to this pilot study. The nature of this study is in vitro, and our findings may not reflect deposition, anti-inflammatory and/or antimicrobial activity of PL in live horses with respiratory disease where changes in breathing pattern, increased respiratory secretions, and damage to the airways can all impact delivery and activity of aerosolized medications (40). Aerosolized delivery of medications can potentially cause irritation to the airways and thus nebulization of PL to healthy horses followed by cytological evaluation of bronchoalveolar lavage fluid would provide assessment of tolerance to nebulization and would also add valuable information regarding any pro-inflammatory or other undesirable affects within the airways. Assessment of antimicrobial activity was limited to growth curve analysis with OD600 readings used to approximate the number of bacteria present in the sample. Over estimation of the number of bacteria can occur with OD readings since they do not distinguish between live and killed bacteria (12). Moreover, experimental error is possible with this kind of approach, demonstrated by the overestimation of E. coli concentration in the beginning of the experiment. Because the concentration was adjusted in the first hour after that and followed the expected concentration for each experimental time, repeating the measurements was not justified. Additional approaches that may have provided a more thorough characterization of the antimicrobial effects of PL could have included evaluation using killing assays, broth microdilution or disk diffusion methods both with or without co-culture with relevant antimicrobials. Finally, although PL used in the study was pooled, only three horses were used for preparation and evaluation of the effects of nebulization relied on technical replicates from a single batch. Increasing the number of biologic replicates by including additional batches of PL as well as increasing the number of horses included in the pooled lysate may have provided a more robust characterization of cytokine, growth factor, and protein composition and antimicrobial activity before and after nebulization.
5 Conclusion
In conclusion, the results of this pilot study demonstrate that nebulization of equine PL is technically feasible using a commercially available equine mesh nebulizer based on determination of particle size and flow rate. In addition, in vitro evaluation of nebulized PL resulted in some changes in protein composition and antimicrobial properties with the antimicrobial effects of PL being organism specific. Further investigation is warranted to better define in vitro the potential antimicrobial and anti-inflammatory properties of nebulized equine PL with the goal to ultimately determine its therapeutic potential in horses with bacterial pneumonia or other inflammatory airway conditions such as equine asthma.
Data availability statement
The datasets presented in this study can be found in online repositories. The names of the repository/repositories and accession number(s) can be found in the article/Supplementary material.
Ethics statement
The animal study was approved by Institutional Animal Care and Use Committee University of Georgia. The study was conducted in accordance with the local legislation and institutional requirements.
Author contributions
PE: Conceptualization, Investigation, Methodology, Writing – original draft, Writing – review & editing. LB: Conceptualization, Funding acquisition, Investigation, Methodology, Supervision, Visualization, Writing – original draft, Writing – review & editing. LH: Conceptualization, Investigation, Methodology, Writing – original draft, Writing – review & editing. CH: Investigation, Writing – original draft. PG: Investigation, Writing – original draft. JA: Formal analysis, Methodology, Writing – original draft, Writing – review & editing. MN: Writing – original draft, Writing – review & editing. JP: Resources, Writing – original draft, Writing – review & editing. JG: Resources, Methodology, Writing – original draft, Writing – review & editing. KL: Conceptualization, Funding acquisition, Investigation, Methodology, Project administration, Supervision, Writing – original draft, Writing – review & editing.
Funding
The author(s) declare financial support was received for the research, authorship, and/or publication of this article. This study was performed with the financial support of the Veterinary Comparative Respiratory Society (VCRS).
Acknowledgments
The authors would like to thank Fletcher North and Qiao Zhong (from AU), as well as Matt Walsh from Nortev Ltd/Flexineb for technical assistance. Material support (nebulizer unit) and particle size analysis was provided by Nortev Ltd/Flexineb.
Conflict of interest
The authors declare that the research was conducted in the absence of any commercial or financial relationships that could be construed as a potential conflict of interest.
Publisher's note
All claims expressed in this article are solely those of the authors and do not necessarily represent those of their affiliated organizations, or those of the publisher, the editors and the reviewers. Any product that may be evaluated in this article, or claim that may be made by its manufacturer, is not guaranteed or endorsed by the publisher.
Supplementary material
The Supplementary Material for this article can be found online at: https://www.frontiersin.org/articles/10.3389/fvets.2024.1488942/full#supplementary-material
Abbreviations
BD-1, Beta defensin 1; BHI, Brain Heart Infusion; IFN-γ, Interferon-γ; IL, Interleukin; K, Carrying capacity; MDR, Multi drug resistant; PDGF, Platelet derived growth factor; PL, Platelet lysate; PRP, Platelet rich plasma; TNF-α, Tumor necrosis factor-α; μmax, Maximum growth; WT, Wild Type.
References
1. Reuss SM, Giguère S. Update on bacterial pneumonia and pleuropneumonia in the adult horse. Vet Clin North Am Equine Pract. (2015) 31:105–20. doi: 10.1016/j.cveq.2014.11.002
2. Reuss SM, Cohen ND. Update on bacterial pneumonia in the foal and weanling. Vet Clin North Am Equine Pract. (2015) 31:121–35. doi: 10.1016/j.cveq.2014.11.004
3. Higgins C, Huber L. Rhodococcus equi: challenges to treat infections and to mitigate antimicrobial resistance. J Equine Vet Sci. (2023) 127:104845. doi: 10.1016/j.jevs.2023.104845
4. Bordin AI, Huber L, Sanz MG, Cohen ND. Rhodococcus equi foal pneumonia: update on epidemiology, immunity, treatment and prevention. Equine Vet J. (2022) 54:481–94. doi: 10.1111/evj.13567
5. Ferri M, Ranucci E, Romagnoli P, Giaccone V. Antimicrobial resistance: a global emerging threat to public health systems. Crit Rev Food Sci Nutr. (2017) 57:2857–76. doi: 10.1080/10408398.2015.1077192
6. Lord J, Carter C, Smith J, Locke S, Phillips E, Odoi A. Antimicrobial resistance among Streptococcus equi subspecies zooepidemicus and Rhodococcus equi isolated from equine specimens submitted to a diagnostic laboratory in Kentucky, USA. PeerJ. (2022) 10:e13682. doi: 10.7717/peerj.13682
7. Palma E, Tilocca B, Roncada P. Antimicrobial resistance in veterinary medicine: an overview. Int J Mol Sci. (2020) 21:1914. doi: 10.3390/ijms21061914
8. Steinman A, Navon-Venezia S. Antimicrobial resistance in horses. Animals. (2020) 10:1161. doi: 10.3390/ani10071161
9. Semple JW, Italiano JE Jr, Freedman J. Platelets and the immune continuum. Nat Rev Immunol. (2011) 11:264–74. doi: 10.1038/nri2956
10. Gilbertie JM, Schaer TP, Schubert AG, Jacob ME, Menegatti S, Ashton Lavoie R, et al. Platelet-rich plasma lysate displays antibiofilm properties and restores antimicrobial activity against synovial fluid biofilms in vitro. J Orthop Res. (2020) 38:1365–74. doi: 10.1002/jor.24584
11. Zamani M, Yaghoubi Y, Movassaghpour A, Shakouri K, Mehdizadeh A, Pishgahi A, et al. Novel therapeutic approaches in utilizing platelet lysate in regenerative medicine: are we ready for clinical use? J Cell Physiol. (2019) 234:17172–86. doi: 10.1002/jcp.28496
12. Gordon J, Álvarez-Narváez S, Peroni JF. Antimicrobial effects of equine platelet lysate. Front Vet Sci. (2021) 8:703414. doi: 10.3389/fvets.2021.703414
13. Moellerberndt J, Hagen A, Niebert S, Büttner K, Burk J. Cytokines in equine platelet lysate and related blood products. Front Vet Sci. (2023) 10:1117829. doi: 10.3389/fvets.2023.1117829
14. Camargo Garbin L, Lopez C, Carmona JU. A critical overview of the use of platelet-rich plasma in equine medicine over the last decade. Front Vet Sci. (2021) 8:641818. doi: 10.3389/fvets.2021.641818
15. Ríos DL, López C, Carmona JU. Evaluation of the anti-inflammatory effects of two platelet-rich gel supernatants in an in vitro system of cartilage inflammation. Cytokine. (2015) 76:505–13. doi: 10.1016/j.cyto.2015.07.008
16. Avellar HK, Lutter JD, Ganta CK, Beard W, Smith JR, Jonnalagadda N, et al. In vitro antimicrobial activity of equine platelet lysate and mesenchymal stromal cells against common clinical pathogens. Can J Vet Res. (2022) 86:59–64.
17. McCarrell TM. Equine platelet-rich plasma. Vet Clin North Amer: Eq Pract. (2023) 39:429–42. doi: 10.1016/j.cveq.2023.06.007
18. Soares CS, Babo PS, Reis RL, Carvalho PP, Gomes ME. Platelet-derived products in veterinary medicine: a new trend or an effective therapy? Trends Biotechnol. (2021) 39:225–43. doi: 10.1016/j.tibtech.2020.07.011
19. Sumner SM, Naskou MC, Thoresen M, Copland I, Peroni JF. Platelet lysate obtained via plateletpheresis performed in standing and awake equine donors. Transfusion. (2017) 57:1755–62. doi: 10.1111/trf.14124
20. Gilbertie JM, Schaer TP, Engiles JB, Seiler GS, Deddens BL, Schubert AG, et al. A platelet-rich plasma-derived biologic clears Staphylococcus aureus biofilms while mitigating cartilage degeneration and joint inflammation in a clinically relevant large animal infectious arthritis model. Front Cell Infect Microbiol. (2022) 12:895022. doi: 10.3389/fcimb.2022.895022
21. Tang YQ, Yeaman MR, Selsted ME. Antimicrobial peptides from human platelets. Infect Immun. (2002) 70:6524–33. doi: 10.1128/IAI.70.12.6524-6533.2002
22. Beitia M, Delgado D, Sánchez P, Vallejo de la Cueva A, Cugat JR, Sánchez M. Platelet lysate nebulization protocol for the treatment of COVID-19 and its sequels: proof of concept and scientific rationale. Int J Mol Sci. (2021) 22:1856. doi: 10.3390/ijms22041856
23. Jeyaraman M, Muthu S, Khanna M, Jain R, Anudeep TC, Muthukanagaraj P, et al. Platelet lysate for COVID-19 pneumonia-a newer adjunctive therapeutic avenue. Stem Cell Investig. (2021) 8:11. doi: 10.21037/sci-2020-042
24. Lee YL, Lee LW, Su CY, Hsiao G, Yang YY, Leu SJ, et al. Virally inactivated human platelet concentrate lysate induces regulatory T cells and immunosuppressive effect in a murine asthma model. Transfusion. (2013) 53:1918–28. doi: 10.1111/trf.12068
25. Brossi PM, Moreira JJ, Machado TS, Baccarin RY. Platelet-rich plasma in orthopedic therapy: a comparative systematic review of clinical and experimental data in equine and human musculoskeletal lesions. BMC Vet Res. (2015) 11:98. doi: 10.1186/s12917-015-0403-z
26. Sampson S, Gerhardt M, Mandelbaum B. Platelet rich plasma injection grafts for musculoskeletal injuries: a review. Curr Rev Musculoskelet Med. (2008) 1:165–74. doi: 10.1007/s12178-008-9032-5
27. Monteiro SO, Lepage OM, Theoret CL. Effects of platelet-rich plasma on the repair of wounds on the distal aspect of the forelimb in horses. Am J Vet Res. (2009) 70:277–82. doi: 10.2460/ajvr.70.2.277
28. Dzyekanski B, Rocha D, Lopes A, Kunz J, Rebelatto C, Pimpão C., et al. Intrabronchial instillation of platelet-rich plasma in equids with inflammatory airway disease. Estudos Biol. (2012) 34:82. doi: 10.7213/estud.biol.6120
29. Pires NR, Miranda S, Costa MFM, Ramos MT, Bernardes C, Alencar NX et al. Effect of intrabronchial platelet rich plasma on the exercise-induced pulmonary hemorrhage endoscopic score in thoroughbred racehorses using furosemide: a preliminary study. Arq Bras Med Vet Zoot. (2021) 73:605–12. doi: 10.1590/1678-4162-12212
30. Salama SM, Kamel IH, Ghanim M, Elsherif AF. The Efficacy of autologous nebulized platelet rich plasma (PRP) as an early adjuvant therapeutic and prognostic treatment modality in the management of inhalation lung injury. Egypt J Plast Reconstruct Surg. (2019) 43:203–8. doi: 10.21608/ejprs.2019.65115
31. Carvalho TC, McConville JT. The function and performance of aqueous aerosol devices for inhalation therapy. J Pharm Pharmacol. (2016) 68:556–78. doi: 10.1111/jphp.12541
32. Fultz L, Giguère S, Berghaus LJ, Grover GS, Merritt DA. Pulmonary pharmacokinetics of desfuroylceftiofur acetamide after nebulisation or intramuscular administration of ceftiofur sodium to weanling foals. Equine Vet J. (2015) 47:473–7. doi: 10.1111/evj.12316
33. Sierra-Rodriguez T, Groover ES, Lascola KM, Mora-Pereira M, Lee YH, Duran SH, et al. Clinical feasibility and airway deposition of nebulized voriconazole in healthy horses. J Equine Vet Sci. (2020) 94:103246. doi: 10.1016/j.jevs.2020.103246
34. Kimura S, McCoy AM, Boothe DM, Wooldridge AA, Graff E, Hammack SM, et al. Effects of a single dose of orally and rectally administered misoprostol in an in vivo endotoxemia model in healthy adult horses. Am J Vet Res. (2022) 83:ajvr.21.12.0206. doi: 10.2460/ajvr.21.12.0206cx
35. Boger BL, Manfredie J, Yob C, Weber PSD, Jacobs C. Beta defensins as biomarkers: detectable in LPS-stimulated equine peripheral blood mononuclear cells and normal, aseptic, and probable septic equine synovial fluid. Am J Vet Res. (2022) 83:1–7. doi: 10.2460/ajvr.21.12.0204
36. Rios Dl, Lopez C, Carmona JU. Platelet-rich gel supernatants stimulate the release of anti-inflammatory proteins on culture media of normal equine synovial membrane explants. Vet Med Int. (2015) 2015:547052. doi: 10.1155/2015/547052
37. Zucca E, Corsini E, Galbiati V, Lange-Consiglio A, Ferrucci F. Evaluation of amniotic mesenchymal cell derivatives on cytokine production in equine alveolar macrophages: an in vitro approach to lung inflammation. Stem Cell Res Ther. (2016) 7:137. doi: 10.1186/s13287-016-0398-9
38. Rappsilber J, Mann M, Ishihama Y. Protocol for micro-purification, enrichment, pre-fractionation and storage of peptides for proteomics using StageTips. Nat Protoc. (2007) 2:1896–906. doi: 10.1038/nprot.2007.261
39. Pirie RS, McGorum BC. Inhalation therapy for equine lower respiratory tract disease. In Pract. (2017) 39:317–27. doi: 10.1136/inp.j2879
40. Cha ML, Costa LR. Inhalation therapy in horses. Vet Clin North Am Equine Pract. (2017) 33:29–46. doi: 10.1016/j.cveq.2016.11.007
41. Carvalho TC, Peters JI, Williams RO 3rd. Influence of particle size on regional lung deposition–what evidence is there? Int J Pharm. (2011) 406:1–10. doi: 10.1016/j.ijpharm.2010.12.040
42. Ari A. Jet, ultrasonic, and mesh nebulizers: an evaluation of nebulizers for better clinical outcomes. Eur J Pulmonol. (2014) 16:1–7. doi: 10.5152/ejp.2014.00087
43. Windberger UBA, Plasenzotti R, Korak KJ. Whole blood viscosity, plasma viscosity and erythrocyte aggregation in nine mammalian species: reference values and comparison of data. Exp Physiol. (2003) 88:431–40. doi: 10.1113/eph8802496
44. Pennati A, Apfelbeck T, Brounts S, Galipeau J. Washed equine platelet extract as an anti-inflammatory biologic pharmaceutical. Tissue Eng Part A. (2021) 27:582–92. doi: 10.1089/ten.tea.2020.0160
45. Giraldo CE, López C, Álvarez ME, Samudio IJ, Prades M, Carmona JU. Effects of the breed, sex and age on cellular content and growth factor release from equine pure-platelet rich plasma and pure-platelet rich gel. BMC Vet Res. (2013) 9:29. doi: 10.1186/1746-6148-9-29
46. Pezzanite LM, Chow L, Dow SW, Goodrich LR, Gilbertie JM, Schnabel LV. Antimicrobial properties of equine stromal cells and platelets and future directions. Vet Clin Equine. (2023) 39:565–78. doi: 10.1016/j.cveq.2023.06.005
47. Strandberg G, Sellberg F, Sommar P, Ronaghi M, Lubenow N, Knutson F, et al. Standardizing the freeze-thaw preparation of growth factors from platelet lysate. Transfusion. (2017) 57:1058–65. doi: 10.1111/trf.13998
48. Hagen A, Lehmann H, Aurich S, Bauer N, Melzer M, Moellerberndt J, et al. Scalable production of equine platelet lysate for multipotent mesenchymal stromal cell culture. Front Bioeng Biotechnol. (2021) 8:613621. doi: 10.3389/fbioe.2020.613621
49. Naskou MC, Sumner S, Berezny A, Copland IB, Peroni JF. Fibrinogen-depleted equine platelet lysate affects the characteristics and functionality of mesenchymal stem cells. Stem Cells Dev. (2019) 28:1572–80. doi: 10.1089/scd.2019.0070
50. McClain AK, McCarrel TM. The effect of four different freezing conditions and time in frozen storage on the concentration of commonly measured growth factors and enzymes in equine platelet-rich plasma over six months. BMC Vet Res. (2019) 15:292. doi: 10.1186/s12917-019-2040-4
51. Beitia M, Delgado D, Mercader J, Gimeno I, Espregueira-Mendes J, Aizpurua B, et al. The effect of short-term cryopreservation on the properties and functionality of platelet-rich plasma. Platelets. (2023) 34:2210243. doi: 10.1080/09537104.2023.2210243
52. Naskou MC, Tyma JF, Gordon J, Berezny A, Kemelmakher H, Richey AC, et al. Equine platelet lysate gel: a matrix for mesenchymal stem cell delivery. Stem Cells Dev. (2022) 31:569–78. doi: 10.1089/scd.2022.0097
53. Ionita CR, Troillet AR, Vahlenkamp TW, Winter K, Brehm W, Ionita JC. Comparison of humoral insulin-like growth factor-1, platelet-derived growth factor-BB, transforming growth factor-β1, and interleukin-1 receptor antagonist concentrations among equine autologous blood-derived preparations. Am J Vet Res. (2016) 77:898–905. doi: 10.2460/ajvr.77.8.898
54. Sad EP, Hess TM, Santos HA, Lessa DAB, Botteon PTL. Molecular and cellular evaluation of horses with summer pasture associated asthma syndrome. J Equine Vet Sci. (2023) 131:104928. doi: 10.1016/j.jevs.2023.104928
55. Giguère S, Viel L, Lee E, MacKay RJ, Hernandez J, Franchini M. Cytokine induction in pulmonary airways of horses with heaves and effect of therapy with inhaled fluticasone propionate. Vet Immunol Immunopathol. (2002) 85:147–58. doi: 10.1016/S0165-2427(01)00420-2
56. Kolsuz M, Erginel S, Alataş O, Alataş F, Metintaş M, Uçgun I, et al. Acute phase reactants and cytokine levels in unilateral community-acquired pneumonia. Respiration. (2003) 70:615–22. doi: 10.1159/000075208
57. Le NTN, Han CL, Delila L, Nebie O, Chien HT, Wu YW, et al. Proteomics of human platelet lysates and insight from animal studies on platelet protein diffusion to hippocampus upon intranasal administration. APL Bioeng. (2024) 8:026111. doi: 10.1063/5.0196553
58. Rodrigues RM, Valim VS, Berger M, da Silva APM, Fachel FNS, Wilke II, et al. The proteomic and particle composition of human platelet lysate for cell therapy products. J Cell Biochem. (2022) 123:1495–505. doi: 10.1002/jcb.30310
59. Bianchetti A, Chinello C, Guindani M, Braga S, Neva A, Verardi R, et al. A blood bank standardized production of human platelet lysate for mesenchymal stromal cell expansion: proteomic characterization and biological effects. Front Cell Dev Biol. (2021) 9:650490. doi: 10.3389/fcell.2021.650490
60. Dhople V, Krukemeyer A, Ramamoorthy A. The human beta-defensin-3, an antibacterial peptide with multiple biological functions. Biochim Biophys Acta. (2006) 1758:1499–512. doi: 10.1016/j.bbamem.2006.07.007
61. Fu J, Zong X, Jin M, Min J, Wang F, Wang Y. Mechanisms and regulation of defensins in host defense. Transduct Target Ther. (2023) 8:300. doi: 10.1038/s41392-023-01553-x
62. Meade KG, O'Farrelly C. β-Defensins: farming the microbiome for homeostasis and health. Front Immunol. (2019) 9:3072. doi: 10.3389/fimmu.2018.03072
63. Shariati A, Moradabadi A, Ghaznavi-Rad E, Dadmanesh M, Komijani M, Nojoomi F. Investigation into antibacterial and wound healing properties of platelets lysate against Acinetobacter baumannii and Klebsiella pneumoniae burn wound infections. Ann Clin Microbiol Antimicrob. (2021) 20:40. doi: 10.1186/s12941-021-00442-x
64. Valtetsiotis K, Di Martino A, Brunello M, D'Agostino C, Poluzzi R, Ferri R, et al. Platelet lysate for the treatment of osteoarthritis: a systematic review of preclinical and clinical studies. Musculoskelet Surg. (2024) 108:275–88. doi: 10.1007/s12306-024-00827-z
65. Franini A, Entani MG, Colosio E, Melotti L, Patruno M. Case report: Flexor carpi ulnaris tendinopathy in a lure-coursing dog treated with three platelet-rich plasma and platelet lysate injections. Front Vet Sci. (2023) 10:1003993. doi: 10.3389/fvets.2023.1003993
66. Burnouf T, Chou ML, Wu YW, Su CY, Lee LW. Antimicrobial activity of platelet (PLT)-poor plasma, PLT-rich plasma, PLT gel, and solvent/detergent-treated PLT lysate biomaterials against wound bacteria. Transfusion. (2013) 53:138–46. doi: 10.1111/j.1537-2995.2012.03668.x
67. López C, Carmona JU, Giraldo CE, Alvarez ME. Bacteriostatic effect of equine pure platelet-rich plasma and other blood products against methicillin-sensitive Staphylococcus aureus. An in vitro study. Vet Comp Orthop Traumatol. (2014) 27:372–8. doi: 10.3415/VCOT-14-04-0054
68. Mariani E, Filardo G, Canella V, Berlingeri A, Bielli A, Cattini L, et al. Platelet-rich plasma affects bacterial growth in vitro. Cytotherapy. (2014) 16:1294–304. doi: 10.1016/j.jcyt.2014.06.003
69. Moojen DJ, Everts PA, Schure RM, Overdevest EP, van Zundert A, Knape JT, et al. Antimicrobial activity of platelet-leukocyte gel against Staphylococcus aureus. J Orthop Res. (2008) 26:404–10. doi: 10.1002/jor.20519
70. Drago L, Bortolin M, Vassena C, Romanò CL, Taschieri S, Del Fabbro M. Plasma components and platelet activation are essential for the antimicrobial properties of autologous platelet-rich plasma: an in vitro study. PLoS ONE. (2014) 9:e107813. doi: 10.1371/journal.pone.0107813
71. Deppermann C, Kubes P. Platelets and infection. Semin Immunol. (2016) 28:536–45. doi: 10.1016/j.smim.2016.10.005
Keywords: equine, platelet lysate, respiratory, nebulization, antibiotic alternative
Citation: Egli P, Boone L, Huber L, Higgins C, Gaonkar PP, Arrington J, Naskou MC, Peroni J, Gordon J and Lascola KM (2024) Pilot study characterizing a single pooled preparation of equine platelet lysate for nebulization in the horse. Front. Vet. Sci. 11:1488942. doi: 10.3389/fvets.2024.1488942
Received: 30 August 2024; Accepted: 25 November 2024;
Published: 12 December 2024.
Edited by:
Fausto Cremonesi, University of Milan, ItalyReviewed by:
Luca Melotti, University of Padua, ItalyTaralyn M. McCarrel, University of Guelph, Canada
Copyright © 2024 Egli, Boone, Huber, Higgins, Gaonkar, Arrington, Naskou, Peroni, Gordon and Lascola. This is an open-access article distributed under the terms of the Creative Commons Attribution License (CC BY). The use, distribution or reproduction in other forums is permitted, provided the original author(s) and the copyright owner(s) are credited and that the original publication in this journal is cited, in accordance with accepted academic practice. No use, distribution or reproduction is permitted which does not comply with these terms.
*Correspondence: Kara M. Lascola, a21sMDA2OEBhdWJ1cm4uZWR1