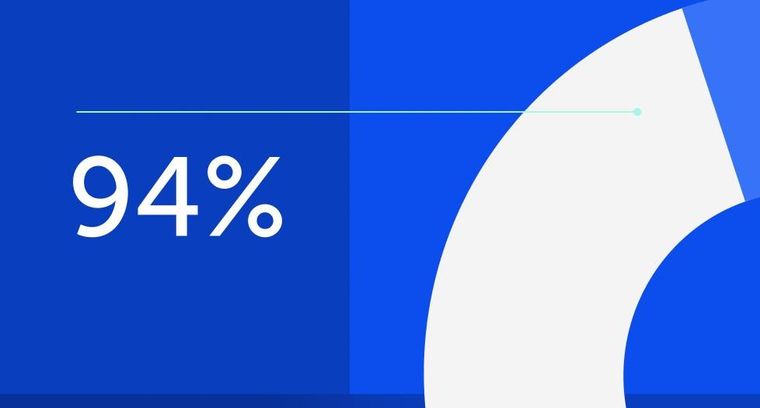
94% of researchers rate our articles as excellent or good
Learn more about the work of our research integrity team to safeguard the quality of each article we publish.
Find out more
REVIEW article
Front. Vet. Sci., 19 July 2024
Sec. Animal Nutrition and Metabolism
Volume 11 - 2024 | https://doi.org/10.3389/fvets.2024.1435788
Adipose tissue, both intricate and fundamental to physiological functions, comprises cell types, including adipocytes, pivotal in secreting bioactive peptides known as ‘adipokines.’ Apelin (APLN), Visfatin (VSFTN), and Irisin (IRSN) are novel adipokines involved in regulating energy, carbohydrate, protein, and lipid metabolism. APLN acts as an endogenous ligand for G-protein-coupled receptors, VSFTN is essential in nicotinamide adenine dinucleotide (NAD) biosynthesis, and IRSN is released from skeletal muscle and adipose tissues. Their influence spans various physiological domains, including insulin resistance and sensitivity, cardiovascular functions, angiogenesis, and reproductive systems. This review focuses on the potential roles of APLN, VSFTN, and IRSN in energy regulation mechanisms related to farm animal production. Despite accumulating evidence of their significance, comprehensive understanding is still emerging, with most studies based on model organisms. Thus, there’s a pressing need for targeted research on farm animals. Addressing these knowledge gaps could pave the way for improved health strategies, reproductive efficiency, and productivity in farm animals. Future research should focus on understanding the multifaceted interactions of these adipokines and their implications for promoting sustainable and effective animal production.
Adipose tissue (AT) is not merely a lipid-storage compartment but a complex endocrine organ with significant metabolic functionality (1). It mediates numerous physiological processes, including neuroendocrine modulation, energetic homeostasis, immunological activities, macrophage reconfiguration, and reproductive functions (2). Historically, AT has been classified into three principal categories: white AT (WAT), brown AT (BAT), and beige AT (BeAT) (3). WAT, which is widely distributed throughout the human body, includes mature adipocytes, macrophages, fibroblasts, endothelial cells, and preadipocytes, all playing integral roles in various energetic homeostasis pathways (1, 4). Conversely, BAT is specialized in energy expenditure, contrasting with WAT’s energy storage function. BAT’s distinguishing features include a high content of lipid droplets and an abundance of mitochondria, which facilitate its role in thermogenesis (5).
Notably, WAT secretes a wide array of bioactive peptides, including both anti-inflammatory and pro-inflammatory factors. These peptides, known as ‘adipokines’ or ‘cytokines,’ have diverse roles, such as modulating energetic homeostasis, regulating reproductive processes, adjusting metabolic balance, and being linked to various cardiovascular pathologies associated with adiposity (6). Key adipokines include leptin (LEP), Retinol Binding Protein 4 (RBP4), and adiponectin (ADIPOQ) (7).
While adiponectin and leptin are renowned for their involvement in energy regulation (8), emerging adipokines, notably APLN, VSFTN, and IRSN, reveal their significance in energy metabolism. APLN, an endogenous ligand of the APJ, a member of the orphan G-protein-coupled receptor (GPCR) family, closely resembles the angiotensin-II receptor (9). Fluctuations in the plasma levels of APLN are observed with weight variations, associating it directly with obesity (10). APLN also shows potential in modulating insulin sensitivity and cardiovascular functions (11).
VSFTN, also known as pre-B cell colony-enhancing factor 1 (PBEF1) or Nicotinamide phosphoribosyltransferase (NAMPT), is involved in B-cell maturation and neutrophil apoptosis (12). Predominantly found in visceral AT (13), several studies have explored its correlation with obesity and type 2 diabetes (14).
IRSN, a notable myokine and adipocytokine, functions across various tissues (15). It can upregulate the expression of uncoupling protein 1 (UCP1) in mitochondria, with its levels influenced by diet, exercise, obesity, and pharmacological agents (16, 17).
In farm animals, adipokines such as APLN, VSFTN, and IRSN are crucial for modulating nutrient intake, energy flux, and maintaining metabolic homeostasis (18). These mechanisms are especially important in livestock facing challenges related to negative energy balance (NEB) (19). The literature highlights the impact of these adipokines on various physiological processes, including metabolic dysregulations, angiogenesis, lipid homeostasis, and adiposity. Additionally, they have diverse effects on the mammalian reproductive system (20).
Despite their significance across multiple species, there is a notable gap in understanding their specific roles in the energy metabolism of farm animals. This review aims to investigate the structures, functions, and potential applications of APLN, VSFTN, and IRSN across different species, with a particular focus on farm animals. By comparing their known roles in other species, we emphasize the urgent need for targeted studies on these adipokines in livestock to enhance energy metabolism and production.
APLN, recognized as an endogenous ligand, uniquely binds to the APLN receptor (APJ) (21). It originates from APLN-77 or pre-pro-APLN. A spectrum of APLN isoforms emerge from it, including APLN-36, APLN-19, APLN-17, APLN-13, and APLN-12 (22–24). These isoforms are derived from the C-terminal of the preproprotein (25, 26). APLN-36 is considered the mature peptide due to its alignment with the 36\u00B0C-terminal amino acids (AAs) of the pre-pro-APLN protein (25). The conserved C-terminal 17 AA sequence, labeled as APLN-17 or K17F, includes APLN-13 (9, 25). Alterations in APLN-13’s N-terminal glutamine result in pyroglutamyl formations, highlighting significant APLN molecular structures (27).
The APLN gene is located on chromosome Xq25-26.1 in humans, marked by a single intron around 6 kb long (9, 28). In rats and mice, the gene is positioned at Xq35 and XA3.2, respectively (9). Identified primary promoter regions include-207/−1 for rats and-100/+74 base pairs for humans (29). A significant similarity of 76–95% is observed among bovine, human, rat, and mouse pre-pro-APLN precursors, with each species believed to possess a native dimeric APLN protein form (30).
APJ, also designated as APLNR, belongs to the seven-transmembrane G-protein coupled receptors (GPCRs) family, specifically within the Rhodopsin-like GPCRs class (31). Its sequence comprises 380 AAs, and its nomenclature is driven by the interaction with its ligand, APLN (32).
Although a definitive APLN structure is absent in the Protein Data Bank (PDB), available studies report that APLN-13 and APLN-36 adopt a “random conformation” at ambient temperatures (33). Circular dichroism spectroscopy (CD) studies indicate a random coil conformation for various APLN isoforms at 5°C and 35°C. APLN-17 is characterized by dominant β-turns and polyproline-II (PPII) structures spanning its peptide configuration (24, 26).
APLN mRNA is detected in stromal-vascular cells and adipocytes (34). However, its expression remains stable across intraabdominal and subcutaneous fat tissues (10). A key functional aspect of APLN is its activation of the Gi/Gq pathways, modulating intracellular AMP concentrations and inhibiting isoproterenol-induced lipolysis among other pathways (Figure 1). Exploring APLN’s structural and functional nuances can illuminate its potential implications in energy regulation within farm animals.
Figure 1. APLN, a GPCR ligand, activates Gi/Gq pathways to modulate intracellular AMPR concentration and inhibit isoproterenol-induced lipolysis, initiating other pathways.
APLN is ubiquitous in both humans’ and rats’ central nervous system (CNS) and peripheral tissues (35). It is detected in various organs, such as the testicles, intestines, and fetus, with variable expression levels across the placenta, heart, lungs, kidneys, and vascular endothelial cells (36–38). Distinctly, APLN expression is pronounced across adipose, connective, and vascular smooth muscle cells (36). Additionally, its presence spans various fish tissues, encompassing the brain, pituitary, spleen, kidney, and liver (39–41). In sheep, APLN is also expressed in peripheral organs such as the mammary gland, abomasum, and duodenum, in addition to the uterus (42–44).
Central APLN’s relevance is undeniable. Several CNS nuclei exhibit APLN mRNA, indicating its role in glucose metabolism (45). Specifically, APLN-positive nerve fibers in the hypothalamus point to APLN-producing neurons that modulate glucose homeostasis, influenced by circulating peptides and neurotransmitters (46). Numerous studies have solidified APLN’s critical role in insulin sensitivity and glucose metabolism. For instance, during conditions that inhibit hepatic glucose production, APLN fosters decreased blood glucose levels by enhancing glucose uptake in skeletal muscles and ATs, ultimately boosting insulin sensitivity (47, 48). Mechanistically, APLN promotes glucose transport in muscles and modulates pathways involving AMP-activated protein kinase (AMPK) and endothelial nitric oxide synthase (eNOS) (11, 48). AMPK activation in muscle cells is pivotal for APLN-induced glucose uptake (49). APLN also enhances glucose movement from the intestinal lumen to the bloodstream, influencing glucose levels in the portal vein and insulin production and sensitivity (36, 50).
Notably, studies on dairy cow reveal contrasting findings on APLN levels post-calving. One study observed decreased APLN levels after calving, with linked fluctuations in insulin and glucose concentrations (51). In contrast, another study reported consistent APLN levels during the transition from pregnancy to lactation (52).
APLN’s widespread distribution and its integral role in glucose metabolism and insulin sensitivity emphasize its potential importance in farm animal health, especially dairy cows. Monitoring APLN fluctuations might be key to optimizing these animals’ metabolic health and energy balance, particularly during pivotal stages such as pregnancy-to-lactation transition.
For a visual representation of the aforementioned mechanisms, Figure 2 offers a detailed schematic overview.
Figure 2. APLN enhances glucose uptake in skeletal muscles, ATs, and myocytes to maintain low blood glucose levels.
Energy homeostasis and nutrient metabolism are harmonized by a synergy of paracrine, endocrine, and autocrine regulators. Vital organs like the liver, pancreatic β-cells, AT, and skeletal muscle orchestrate this balance (53). Imbalances may usher in conditions like obesity, often linked with insulin resistance. Increased plasma APLN concentrations have been associated with obesity and type 2 diabetes (48).
APLN counteracts isoproterenol-induced lipolysis in isolated and differentiated 3 T3-L1 adipocytes (54). This modulation leverages the GQ, Gi, and AMPK signaling pathways, crucial for orchestrating cellular metabolic responses (55). By inhibiting lipid catabolism in adipocytes, APLN limits the release of free fatty acids. This is achieved through AMPK activation and the upsurge in perilipin surrounding lipid vacuoles, shielding stored lipids from lipase activity (54). APLN also fosters insulin secretion and enhances mitochondrial biogenesis in skeletal muscles and cardiomyocytes, increasing mitochondrial density and efficiency (56, 57).
APLN promotes endothelial cell proliferation, migration, and neovascularization (45). In the hypothalamus, APLN regulates the secretion of antidiuretic hormone (ADH), essential for fluid homeostasis (58). It also triggers α-MSH release, a neuropeptide known to reduce food consumption, potentially offsetting metabolic disparities in obese subjects despite receptor desensitization (59). APLN and its receptor, APJ, play roles in water metabolism, synergizing with ADH to maintain fluid balance (36).
APLN’s role in lipid and broader metabolic processes highlights its potential significance for livestock metabolic health. By influencing lipid metabolism, enhancing mitochondrial activity, and promoting angiogenesis, APLN could be pivotal in optimizing livestock productivity and overall health.
A summary of APLN’s metabolic impacts, as documented in reported findings from different species, is presented in Table 1.
APLN modulates a host of cellular activities through distinct signaling pathways, notably activating AMPK, which reduces the release of free fatty acids (FFA) from 3 T3-L1 adipocytes by enhancing lipoprotein stability (70). Isoforms of APLN, including APLN-36, APLN-13, APLN-17, and [pyr1]-APLN-13, inhibit forskolin-induced cAMP production via the Gαi/o protein in the APLN/APJ system, blocking specific PKA pathway effects (36, 71).
APLN also stimulates glucose transport in human AT explants through the AMPK pathway, while in 3 T3-L1 adipocytes, it enhances glucose transport via the PI3K/Akt signaling pathway (72, 73). Additionally, APLN boosts insulin-stimulated glucose transport in insulin-resistant 3 T3-L1 cells (73).
APLN regulates various physiological functions, including blood pressure, energy metabolism, blood flow, hydration, food intake, and immune function (28). It induces nitric oxide (NO) release, leading to vasorelaxation and increased heart muscle contractility (74). APLN also directs endothelial cell chemotaxis and provides anti-apoptotic protection (75). Its interaction with appetite-regulating hormones, such as orexin, highlights its role in complex metabolic networks (76, 77). In sheep, APLN expression has been identified in various peripheral organs, including the mammary gland, abomasum, and duodenum, in addition to the uterus. This widespread distribution suggests that APLN may play multiple roles in energy regulation and reproductive functions in sheep (42). For instance, the presence of APLN in the mammary gland could be associated with lactation and milk production processes. Similarly, its expression in the abomasum and duodenum indicates a potential role in digestive processes and nutrient absorption (43, 44). Further research is necessary to explore these roles and their implications for sheep health and productivity.
Understanding APLN’s multifaceted roles is crucial for energy regulation in farm animals. APLN’s effects on glucose and lipid metabolism, alongside its broader physiological impacts, suggest potential strategies for optimizing energy balance, health, and productivity in livestock. Further research on APLN’s signaling pathways could lead to innovative animal management and health applications.
VSFTN, initially identified as the pre-B cell colony-enhancing factor (PBEF), is characterized by its 52 kDa molecular weight and 491 amino acids (AAs), which are highly conserved across species (78). The porcine VSFTN gene is located on chromosome SSC9, sharing structural similarities with the human counterpart (13, 79). It plays a crucial role in the nicotinamide adenine dinucleotide (NAD) biosynthesis pathway and is also known as Nicotinamide Phosphoribosyltransferase (NAMPT) (80, 81).
NAMPT exists in two forms: intracellular (iNAMPT) and extracellular (eNAMPT) (82). iNAMPT is predominantly found in BAT and less in WAT, while eNAMPT is expressed in various cells, including adipocytes, myocytes, neurons, and immune cells (83, 84). iNAMPT is also present in the liver, kidneys, heart, skeletal muscle, and brain (20). eNAMPT, on the other hand, is expressed across a diverse array of cells ranging from adipocytes and myocytes to neurons and immune cells (83).
VSFTN’s monomer is organized into three domains: A, B, and C, each characterized by specific arrangements of α-helices and β-strands (7). Domain A features an antiparallel seven-stranded β-sheet/core flanked by five helices, domain B has another seven-stranded β-sheet/core, and domain C presents a simpler three-stranded β-sheet in an antiparallel configuration (7).
VSFTN is integral to NAD biosynthesis and influences pancreatic beta-cell functions (85). As NAMPT, it catalyzes the transformation of nicotinamide into nicotinamide mononucleotide (NMN), a crucial precursor for NAD synthesis. In pancreatic beta cells, this enhances glucose-stimulated insulin secretion through sirtuin-1 activation, a NAD-dependent deacetylase, regulating glucose-responsive insulin secretion (86).
VSFTN is detected in diverse human and animal tissues, such as AT, muscle, heart, and bone marrow (87). It has homologs in organisms ranging from mollusks and bacteria to mammals. In mice, VSFTN is located in the hypothalamus and certain pituitary lobes (88). Additionally, there are gender and tissue-specific variations in VSFTN mRNA expression among broiler chickens, indicating its responsiveness to energy balance-related determinants (89).
Given its central role in energy regulation, particularly in NAD synthesis and insulin secretion, VSFTN is pivotal for understanding energy balance in farm animals. Its widespread presence across multiple species and organs suggests that deeper exploration into VSFTN’s mechanisms could unveil strategies to enhance health, metabolic efficiency, and overall productivity in livestock.
VSFTN plays an indispensable role in various physiological processes, prominently influencing glucose and lipid metabolism. It significantly contributes to anti-inflammatory responses, glucose and lipid modulation, and behavioral aspects related to food intake (90). In adipocytes, VSFTN-induced NAD biosynthesis acts as a crucial physiological regulator, impacting metabolic activities within AT and exerting systemic influence (91). VSFTN has a metabolic effect analogous to insulin, stimulating glucose uptake in myocytes and adipocytes, augmenting lipogenesis, and suppressing glucose release from hepatocytes (92, 93). A visual representation of these multifarious roles can be found in Figure 3.
Figure 3. Key biological roles of VSFTN: insights into lipid and glucose metabolism and inflammation.
VSFTN’s influence on glucose and lipid metabolism spans endocrine, paracrine, and autocrine pathways, with its autocrine actions notably impacting insulin sensitivity in the liver (92). Research supports these findings; for instance, in a porcine cellular model, VSFTN and insulin elevated lipoprotein lipase and PPARγ mRNA expression in preadipocytes and enhanced fatty acid synthase mRNA expression in differentiated adipocytes (94). Uniquely, VSFTN also increased IL-6 mRNA expression, indicating its involvement in lipid metabolism.
VSFTN’s impact extends to behavioral modulation, particularly in regulating food intake and indirectly controlling energy balance (95). For example, an intracerebroventricular injection of VSFTN in chicks increased feed intake and altered brain activity, highlighting its role in modulating feeding behavior (96).
Dietary inputs can affect VSFTN gene expression. In Hanwoo beef calves, a reduction in concentrate intake increased VSFTN gene expression in the liver, indicating diet’s pivotal role in regulating this gene (97). Furthermore, an inverse correlation between VSFTN expression and body fat levels was observed in pigs, with leaner pigs exhibiting higher VSFTN concentrations, raising questions about its reliability as an indicator of fat storage (98).
The various biological implications of VSFTN across animal studies are systematically summarized in Table 2.
IRSN, identified as a significant myokine, is released from human muscle tissue following exercise (102). It arises from the precursor protein, fibronectin type III domain 5 (FNDC5), a type-I transmembrane protein initially described in 2002 (103, 104). Structurally, FNDC5 comprises an N-terminal peptide chain (AA sequence 1–28), a Fibronectin-III domain (AA sequence 33–124), a transmembrane domain (AA sequence 150–170), and a cytoplasmic tail (AA sequence 171–209) (105). The extracellular N-terminal tail undergoes proteolytic cleavage, releasing the 112 AA IRSN peptide that circulates across various tissues (102).
FNDC5 gene expression is modulated by pivotal proteins like peroxisome proliferator-activated receptor gamma (PPARγ) and coactivator PGC1α (106). PPARγ governs adipocyte differentiation and lipid metabolism, influencing processes such as anti-inflammatory responses, lipid storage in the liver, and glucose-stimulated insulin secretion in pancreatic beta cells (107–109). Conversely, PGC1α is renowned for overseeing mitochondrial biogenesis and regulating oxidative metabolism in diverse cell types (110).
Recent studies have further elucidated IRSN’s role in farm animals. One study established that delivery mode affects IRSN concentrations in Holstein calves. Dystocia and cesarean-born calves presented reduced IRSN levels post-colostrum intake, while those born vaginally showed a decline by day 15, highlighting the relationship between birth methods and metabolic adaptations via IRSN concentrations (111). Moreover, IRSN concentrations were notably heightened in cattle with subclinical ketosis compared to their healthy counterparts. This increase was accompanied by a pronounced positive correlation between IRSN and ghrelin levels, indicating IRSN’s potential as a ketosis biomarker (112). Additionally, IRSN has been shown to impact glucose metabolism in cattle by reducing mRNA levels of certain glucose transporters in granulosa cells and increasing lactate release (113).
A positive IRSN-leptin relationship, particularly in cows with subclinical ketosis, aligns with prior studies connecting insulin and IRSN in metabolic disorders (114–117). These findings underscore IRSN’s multifaceted role in farm animals, linking birth methods to IRSN levels and metabolic adjustments. Its elevated concentration in ketotic cattle and associations with ghrelin and leptin further indicate IRSN’s potential as a biomarker for metabolic disorders, specifically subclinical ketosis, and its influence on glucose metabolism.
Recent studies have begun exploring the role and expression of FNDC5 and IRSN in farm animals, revealing differences from humans and mice. For instance, the bovine genome shows higher transcript variability of FNDC5 compared to humans and mice. Although FNDC5 protein distribution remains consistent in bull skeletal muscles, mRNA transcript levels vary significantly in AT and the liver (118). However, detecting IRSN in cattle plasma remains challenging (119).
Further complicating the picture, Daudon et al. (15) posited that FNDC5 and IRSN play roles in lipid mobilization in AT of dairy cattle post-calving. The emerging picture indicates varying and sometimes conflicting views on IRSN’s expression and effects in cattle, warranting deeper investigations. Beyond cattle, the exploration of IRSN’s role in other farm animals has begun. For example, IRSN, FNDC5, and PGC1α were detected in skeletal muscles and WATs of dromedary camels, with correlations observed between their levels and metabolic responses to exercise (120). Further, the IRSN peptide was localized in swine ovaries, suggesting implications for ovarian function (121). Another study on Arabian horses linked serum IRSN levels to exercise regimes (122).
While the above findings shed some light, the overarching theme is clear: Our understanding of IRSN in farm animals, especially its implications on energy regulation, remains limited. Much of the IRSN research focus has historically been on humans and lab models, like mice and rats. This current state presents an extensive opportunity to investigate IRSN’s role in farm animals more comprehensively, aligning with the broader aim of deciphering its potential implications in energy regulation within this context.
IRSN is a prominent adipocytokine deeply involved in various metabolic pathways, influencing lipid homeostasis, cardiovascular health, CNS processes, and overall energy metabolism (123, 124). It plays a central role in converting WAT to BAT, thus amplifying energy expenditure (125). A detailed depiction of the signaling pathways activated by IRSN is provided in Figure 4.
IRSN stimulates glycolysis and oxidative metabolism, endorsing mitochondrial biogenesis via key genes like Glut4 and UCP3, enhancing energy output, insulin sensitivity, and a healthier metabolic profile (126). These effects are mediated through various pathways, including cAMP-PKA-HSL (126).
IRSN influences liver metabolism by stimulating key pathways and enzymes such as AMPK and LKB1, improving hepatic glucose and lipid metabolism (127, 128). The upregulation of UCP1 in BAT aids thermogenesis and energy expenditure, promoting weight loss and enhancing glucose metabolism (129).
Physical activity significantly affects IRSN expression, with exercise increasing its plasma and skeletal muscle levels, and extended durations enriching its presence in the brain, potentially reducing anxiety (130). IRSN levels are also modulated by dietary adjustments, obesity, and specific pharmacological exposures (16). For a comprehensive overview of how physical activity impacts the transcription of the FNDC5 gene and leads to the release of IRSN, refer to Figure 5.
Figure 5. Physical activity stimulates DNA transcription of the FNDC5 gene, leading to proteolytic cleavage and subsequent release of the IRSN.
In farm animals, IRSN shows intriguing responses. For example, camels exhibit a relationship between glucose and IRSN during exercise, suggesting regulatory roles of glucose, FFA, insulin, leptin, and cortisol on the PGC-1α/FNDC5/IRSN pathway (120). Postpartum dairy cows show correlations between FNDC5 plasma concentrations and various metabolic metrics, emphasizing IRSN’s importance during this period (15). In dairy cattle, IRSN plays a significant role in lipid metabolism, enhancing lipolysis and mobilizing lipid reserves post-parturition (15).
IRSN’s diverse roles in metabolic regulation and energy expenditure highlight its potential in improving health and productivity in farm animals. Understanding its pathways and effects can lead to innovative strategies for managing livestock metabolism.
Table 3 elucidates the biological effects of IRSN in laboratory animals and presents an all-encompassing summary of the observed results.
Farm animals, notably dairy cattle, undergo significant metabolic adjustments during early lactation. These shifts correlate with milk production and an observed disparity in feed intake, frequently leading to an NEB — a situation where energy demands outstrip consumption, with critical repercussions for post-calving health (137, 138). As they transition from late pregnancy to early lactation, there’s a marked decrease in insulin plasma levels, coupled with diminished insulin sensitivity in key tissues like skeletal muscle and AT (139). These alterations ensure glucose is adequately available for milk synthesis and fetal development, while heightened insulin resistance augments lipid mobilization, leading to elevated plasma NEFA and beta-hydroxybutyrate levels post-calving (140–144).
Adipokines, namely APLN, VSFTN, and IRSN, play instrumental roles in these metabolic transitions. Specifically, in dairy cows, APLN-36, a variant of APLN, exhibits elevated concentration and mRNA expression nearing parturition, showing an inverse relationship with serum NEFA levels. This suggests APLN’s potential to modulate lipid mobilization in dairy cows (51, 145, 146). VSFTN, with its critical function in insulin sensitivity and glucose regulation, gains prominence during the pregnancy-to-lactation transition, especially in high-producing dairy cows. Given its presence in mammary gland epithelial cells and milk, VSFTN might offer health benefits to calves (80, 147, 148). Conversely, IRSN is vital in lipid mobilization during post-partum NEB, with elevated plasma levels observed after calving (15).
In a study, diet-restricted calves displayed heightened VSFTN mRNA expression in liver biopsies versus controls, pointing to potential glucose metabolism irregularities and indicating the profound effect of diet on VSFTN expression and glucose regulation (97).
Furthermore, optimizing fat deposition in farm animals directly impacts meat quality. While some fats might be superfluous, intramuscular fat enhances flavor. The production of IRSN during physical activity could influence IM fat deposition, suggesting its potential to elevate meat quality (149).
The interplay between APLN, VSFTN, and IRSN in energy balance and metabolism, particularly in insulin resistance and obesity, is noteworthy. APLN levels are higher in obesity but drop with weight loss, while IRSN decreases in obesity but increases during exercise (150). Investigations have delved into the dynamics between these adipokines and metabolic parameters, revealing their fluctuating levels under normal, impaired glucose tolerance, and diabetic conditions (151). Unraveling these relationships and understanding their nuances is essential for the energy metabolism of farm animals and demands more in-depth exploration. The effects of the three adipokines on farm animals are summarized in Table 4.
Overall, APLN, VSFTN, and IRSN have pivotal roles in energy regulation in farm animals, especially during crucial metabolic transitions. Enhancing our understanding of these adipokines in farm animal contexts is fundamental for refining health and production strategies.
Farm animal fertility is intricately linked to energy metabolism. With the hypothalamus, anterior pituitary, and gonads collectively governing the reproductive system, energy status emerges as a determinant of fertility. In recent years, AT has evolved from being perceived merely as an energy storage site to an active endocrine organ, releasing a slew of adipokines. Notably, leptin and adiponectin have been pinpointed for their involvement in the hypothalamic–pituitary-gonadal axis and female reproductive tract. Yet, the adipokines APLN, VSTFN, and IRSN have garnered attention for their potential roles within various female reproductive systems of farm animals (20).
APLN and its receptor, APJ, are discernible in the ovaries across several farm animals, ranging from cattle to sheep (43, 152–155). Besides the ovaries, APLN is also detectable in sheep mammary glands (44). Furthermore, APLN has been identified in the uterus and uterine tubes as well as in the ovary (42). This adipokine has been found to augment steroidogenesis in several species, including cattle and porcine, and stimulate the proliferation of granulosa cells (152, 153, 156).
VSFTN’s presence is affirmed in the ovaries of animals like cattle, buffalo, chicken, and turkeys (89, 157–159). Its functional role varies across species; while it boosts steroidogenesis and granulosa cell proliferation in cattle, buffalo, and turkeys, its effect seems inverse in chickens (89).
IRSN’s landscape in farm animals is less explored. Though observed in porcine (121), its gene expression in cattle diverges from patterns seen in humans and mice (118). Preliminary data suggest the absence of IRSN expression in buffalo ovaries. IRSN is noted to influence steroidogenesis in porcine ovaries, but its broader implications for the reproductive systems of farm animals remain enigmatic.
Despite the evidence indicating adipokine involvement in certain species, data gaps exist, especially concerning their interactions with the hypothalamus-pituitary axis in farm animals. To realize the full scope of APLN, VSFTN, and IRSN’s roles in energy regulation and reproduction, targeted research in the context of farm animals is paramount.
In ruminants, such as sheep and goats, the role of APLN extends beyond mere energy regulation. It is intricately involved in postprandial responses and profoundly affects the secretion of growth hormone, arginine-vasopressin, and adrenocorticotropic hormones (160). Specifically, in sheep, APLN’s ability to modulate molecules is evident in its regulation of mammary gland activity. This regulation is characterized by the induction of hormonal activation and a biphasic hemodynamic response (60). During different physiological stages in ewes, such as lactation and pregnancy, serum APLN levels appear to be resilient to alterations in the body condition score (BCS). This consistent presence underlines its potential importance in energy regulation during these critical phases (161). Post-parturition, decreased levels of APLN are observed, suggesting its potential adaptive role during this period (146).
Dairy cows present another dimension to the understanding of APLN. Norvezh et al. (51) highlighted a decline in serum APLN-36 levels post-calving. This fluctuation is closely associated with blood biochemical parameters, indicative of the cow’s transition to an NEB state. Such alterations in APLN levels may be instrumental in helping dairy cows accommodate the increased energy requirements characteristic of lactation. Furthermore, cow’s milk represents a key dietary component for calves and humans. Rich in various adipokines, it offers both nutritional and physiological benefits. APLN, as a part of this adipokine profile, could be crucial for growth and overall health, emphasizing the milk’s value not just as a dietary component but as a potential modulator of energy regulation (162). Other findings in sheep showed that APLN levels in ewes varied significantly based on breed, gender, and their interactions with body condition score, but not by lactation or pregnancy status (161). These authors claimed that the variability in APLN levels during critical physiological phases and its potential role as a serum biomarker strongly suggest its potential application in diagnosing and understanding metabolic disorders (161). Similarly, APLN levels in dairy cows’ serum stayed stable between pregnancy and lactation, indicating slight fluctuation (52).
With the pivotal roles that APLN, VSFTN, and IRSN play in energy regulation across farm animals, it’s evident that understanding their dynamics can offer valuable insights. These insights can potentially drive advancements in farm animal production strategies, with health, growth, and reproductive efficiency implications.
Currently, there is a significant gap in knowledge regarding the specific roles of novel adipokines—APLN, VSFTN, and IRSN—in farm animals, particularly in terms of energy metabolism and production. Limited information is available on their impact, especially concerning VSFTN. Future research should aim to investigate the associations between these adipokines and their combined effects on energy regulation. Understanding these relationships will provide valuable insights into their physiological roles and potential applications for enhancing farm animal health and productivity.
Research priorities:
1. Interplay of adipokines: Investigate how changes in one adipokine influence the levels or functions of the others.
2. Impact on energy metabolism: Study the specific roles of APLN, VSFTN, and IRSN in energy metabolism in various farm animals.
3. Production outcomes: Assess the effects of these adipokines on farm animal production metrics, such as growth, milk yield, and meat quality.
4. Mechanistic studies: Conduct detailed mechanistic studies to understand the molecular pathways mediated by these adipokines.
5. Comparative analysis: Compare the roles and effects of these adipokines in farm animals with those observed in model animals to identify species-specific differences and similarities.
BS: Conceptualization, Data curation, Formal analysis, Funding acquisition, Investigation, Methodology, Project administration, Resources, Software, Supervision, Validation, Visualization, Writing – original draft, Writing – review & editing. S-SJ: Writing - Review & editing HL: Writing – review & editing. HA: Writing – review & editing. AS: Writing – review & editing. AG: Writing – review & editing. SM: Writing – review & editing. SA: Writing – review & editing.
The author(s) declare that no financial support was received for the research, authorship, and/or publication of this article.
The authors declare that the research was conducted in the absence of any commercial or financial relationships that could be construed as a potential conflict of interest.
All claims expressed in this article are solely those of the authors and do not necessarily represent those of their affiliated organizations, or those of the publisher, the editors and the reviewers. Any product that may be evaluated in this article, or claim that may be made by its manufacturer, is not guaranteed or endorsed by the publisher.
1. Ràfols, ME . Adipose tissue: cell heterogeneity and functional diversity. Endocrinol Nutr. (2014) 61:100–12. doi: 10.1016/j.endonu.2013.03.011
2. Sauerwein, H, Bendixen, E, Restelli, L, and Ceciliani, F. The adipose tissue in farm animals: a proteomic approach. Curr Protein Pept Sci. (2014) 15:146–55. doi: 10.2174/1389203715666140221123105
3. Chernukha, IM, Fedulova, LV, and Kotenkova, EA. White, beige and brown adipose tissue: structure, function, specific features and possibility formation and divergence in pigs. Foods Raw Mater. (2022) 10:10–8. doi: 10.21603/2308-4057-2022-1-10-18
4. Fain, JN, Madan, AK, Hiler, ML, Cheema, P, and Bahouth, SW. Comparison of the release of adipokines by adipose tissue, adipose tissue matrix, and adipocytes from visceral and subcutaneous abdominal adipose tissues of obese humans. Endocrinology. (2004) 145:2273–82. doi: 10.1210/en.2003-1336
5. Lee, JH, Park, A, Oh, K-J, Lee, SC, Kim, WK, and Bae, K-H. The role of adipose tissue mitochondria: regulation of mitochondrial function for the treatment of metabolic diseases. Int J Mol Sci. (2019) 20:4924. doi: 10.3390/ijms20194924
6. Radin, MJ, Sharkey, LC, and Holycross, BJ. Adipokines: a review of biological and analytical principles and an update in dogs, cats, and horses. Vet Clin Pathol. (2009) 38:136–56. doi: 10.1111/j.1939-165X.2009.00133.x
7. Raucci, R, Rusolo, F, Sharma, A, Colonna, G, Castello, G, and Costantini, S. Functional and structural features of adipokine family. Cytokine. (2013) 61:1–14. doi: 10.1016/j.cyto.2012.08.036
8. Palin, M-F, Farmer, C, and Duarte, C. Triennial lactation symposium/bolfa: Adipokines affect mammary growth and function in farm animals. J Anim Sci. (2017) 95:5689–700. doi: 10.2527/jas2017.1777
9. O’Carroll, A-M, Lolait, SJ, Harris, LE, and Pope, GR. The apelin receptor APJ: journey from an orphan to a multifaceted regulator of homeostasis. J Endocrinol. (2013) 219:R13–35. doi: 10.1530/JOE-13-0227
10. Castan-Laurell, I, Vítkova, M, Daviaud, D, Dray, C, Kováčiková, M, Kovacova, Z, et al. Effect of hypocaloric diet-induced weight loss in obese women on plasma apelin and adipose tissue expression of apelin and APJ. Eur J Endocrinol. (2008) 158:905–10. doi: 10.1530/EJE-08-0039
11. Dray, C, Knauf, C, Daviaud, D, Waget, A, Boucher, J, Buleon, M, et al. Apelin stimulates glucose utilization in normal and obese insulin-resistant mice. Cell Metab. (2008) 8:437–45. doi: 10.1016/j.cmet.2008.10.003
12. Kaczor, U, Jurkowska, M, Kucharski, M, and Kaczor, A. Protein function and polymorphism of the Visfatin gene in farm animals. Rocz Nauk Zoot. (2017) 44:133–9.
13. Chen, H, Xia, T, Zhou, L, Chen, X, Gan, L, Yao, W, et al. Gene organization, alternate splicing and expression pattern of porcine visfatin gene. Domest Anim Endocrinol. (2007) 32:235–45. doi: 10.1016/j.domaniend.2006.03.004
14. Tond, SB, Fallah, S, Salemi, Z, and Seifi, M. Influence of mulberry leaf extract on serum adiponectin, visfatin and lipid profile levels in type 2 diabetic rats. Braz Arch Biol Technol. (2016) 59:59. doi: 10.1590/1678-4324-2016160297
15. Daudon, M, Bigot, Y, Dupont, J, and Price, CA. Irisin and the fibronectin type III domain-containing family: structure, signaling and role in female reproduction. Reproduction. (2022) 164:R1–9. doi: 10.1530/REP-22-0037
16. Mahgoub, MO, D’Souza, C, Al Darmaki, RS, Baniyas, MM, and Adeghate, E. An update on the role of irisin in the regulation of endocrine and metabolic functions. Peptides. (2018) 104:15–23. doi: 10.1016/j.peptides.2018.03.018
17. Arhire, LI, Mihalache, L, and Covasa, M. Irisin: a hope in understanding and managing obesity and metabolic syndrome. Front Endocrinol. (2019) 10:524. doi: 10.3389/fendo.2019.00524
18. Polyzos, SA, Kountouras, J, Shields, K, and Mantzoros, CS. Irisin: a renaissance in metabolism? Metabolism. (2013) 62:1037–44. doi: 10.1016/j.metabol.2013.04.008
19. Block, S, Butler, W, Ehrhardt, R, Bell, A, Van Amburgh, M, and Boisclair, Y. Decreased concentration of plasma leptin in periparturient dairy cows is caused by negative energy balance. J Endocrinol. (2001) 171:339–48. doi: 10.1677/joe.0.1710339
20. Shokrollahi, B, Shang, JH, Saadati, N, Ahmad, HI, and Yang, CY. Reproductive roles of novel adipokines apelin, visfatin, and irisin in farm animals. Theriogenology. (2021) 172:178–86. doi: 10.1016/j.theriogenology.2021.06.011
21. Tatemoto, K, Hosoya, M, Habata, Y, Fujii, R, Kakegawa, T, Zou, M-X, et al. Isolation and characterization of a novel endogenous peptide ligand for the human APJ receptor. Biochem Biophys Res Commun. (1998) 251:471–6. doi: 10.1006/bbrc.1998.9489
22. Coquerel, D, Delile, E, Dumont, L, Chagnon, F, Murza, A, Sainsily, X, et al. Gαi-biased apelin analog protects against isoproterenol-induced myocardial dysfunction in rats. Am J Physiol Heart Circ Physiol. (2021) 320:H1646–56. doi: 10.1152/ajpheart.00688.2020
23. Shin, K, Chapman, NA, Sarker, M, Kenward, C, Huang, SK, Weatherbee-Martin, N, et al. Bioactivity of the putative apelin proprotein expands the repertoire of apelin receptor ligands. Biochim Biophys Acta Gen Subj. (2017) 1861:1901–12. doi: 10.1016/j.bbagen.2017.05.017
24. Shin, K, Kenward, C, and Rainey, JK. Apelinergic system structure and function. Compr Physiol. (2017) 8:407–50. doi: 10.1002/cphy.c170028
25. Ma, Y, Yue, Y, Ma, Y, Zhang, Q, Zhou, Q, Song, Y, et al. Structural basis for apelin control of the human apelin receptor. Structure. (2017) 25:858–866.e4. doi: 10.1016/j.str.2017.04.008
26. Langelaan, DN, Bebbington, EM, Reddy, T, and Rainey, JK. Structural insight into G-protein coupled receptor binding by apelin. Biochemistry. (2009) 48:537–48. doi: 10.1021/bi801864b
27. Zhen, EY, Higgs, RE, and Gutierrez, JA. Pyroglutamyl apelin-13 identified as the major apelin isoform in human plasma. Anal Biochem. (2013) 442:1–9. doi: 10.1016/j.ab.2013.07.006
28. Kurowska, P, Barbe, A, Różycka, M, Chmielińska, J, Dupont, J, and Rak, A. Apelin in reproductive physiology and pathology of different species: a critical review. Int J Endocrinol. (2018) 2018:1–12. doi: 10.1155/2018/9170480
29. Wang, G, Qi, X, Wei, W, Englander, EW, and Greeley, GH Jr. Characterization of the 5′-regulatory regions of the rat and human apelin genes and regulation of breast apelin by USF. FASEB J. (2006) 20:2639–41. doi: 10.1096/fj.06-6315fje
30. Salska, A, and Chiżyński, K. Apelin–a potential target in the diagnosis and treatment of the diseases of civilization. Acta Cardiol. (2016) 71:505–17. doi: 10.1080/AC.71.5.3167493
31. Gandham, R, Sumathi, M, Dayanand, C, Sheela, S, and Kiranmayee, P. Apelin and its receptor: an overview. J Clin Diagn Res. (2019) 13:EE01–5. doi: 10.7860/JCDR/2019/41074.12930
32. Lee, DK, Cheng, R, Nguyen, T, Fan, T, Kariyawasam, AP, Liu, Y, et al. Characterization of apelin, the ligand for the APJ receptor. J Neurochem. (2000) 74:34–41. doi: 10.1046/j.1471-4159.2000.0740034.x
33. Langelaan, DN. Structural studies of apelin and its receptor as well as the characteristics and causes of membrane protein helix kinks (Master’s thesis). Dalhousie University. (2013).
34. Wei, L, Hou, X, and Tatemoto, K. Regulation of apelin mRNA expression by insulin and glucocorticoids in mouse 3T3-L1 adipocytes. Regul Pept. (2005) 132:27–32. doi: 10.1016/j.regpep.2005.08.003
35. Lv, S-Y, Cui, B, Chen, W-D, and Wang, Y-D. Apelin/APJ system: a key therapeutic target for liver disease. Oncotarget. (2017) 8:112145:–112151. doi: 10.18632/oncotarget.22841
36. Hu, G, Wang, Z, Zhang, R, Sun, W, and Chen, X. The role of Apelin/Apelin receptor in energy metabolism and water homeostasis: a comprehensive narrative review. Front Physiol. (2021) 12:632886. doi: 10.3389/fphys.2021.632886
37. Pope, GR, Roberts, EM, Lolait, SJ, and O’Carroll, A-M. Central and peripheral apelin receptor distribution in the mouse: species differences with rat. Peptides. (2012) 33:139–48. doi: 10.1016/j.peptides.2011.12.005
38. Dawid, M, Mlyczyńska, E, Jurek, M, Respekta, N, Pich, K, Kurowska, P, et al. Apelin, APJ, and ELABELA: role in placental function, pregnancy, and foetal development—an overview. Cells. (2021) 11:99. doi: 10.3390/cells11010099
39. Volkoff, H, and Wyatt, JL. Apelin in goldfish (Carassius auratus): cloning, distribution and role in appetite regulation. Peptides. (2009) 30:1434–40. doi: 10.1016/j.peptides.2009.04.020
40. Lin, F, Wu, H, Chen, H, Xin, Z, Yuan, D, Wang, T, et al. Molecular and physiological evidences for the role in appetite regulation of apelin and its receptor APJ in Ya-fish (Schizothorax prenanti). Mol Cell Endocrinol. (2014) 396:46–57. doi: 10.1016/j.mce.2014.08.009
41. Hayes, J, and Volkoff, H. Characterization of the endocrine, digestive and morphological adjustments of the intestine in response to food deprivation and torpor in cunner, Tautogolabrus adspersus. Comp Biochem Physiol Part A Mol Integr Physiol. (2014) 170:46–59. doi: 10.1016/j.cbpa.2014.01.014
42. Palmioli, E, Dall’Aglio, C, Bellesi, M, Tardella, FM, Moscatelli, S, Scocco, P, et al. The apelinergic system immuno-detection in the abomasum and duodenum of sheep grazing on semi-natural pasture. Animals. (2021) 11:173. doi: 10.3390/ani11113173
43. Mercati, F, Scocco, P, Maranesi, M, Acuti, G, Petrucci, L, Cocci, P, et al. Apelin system detection in the reproductive apparatus of ewes grazing on semi-natural pasture. Theriogenology. (2019) 139:156–66. doi: 10.1016/j.theriogenology.2019.08.012
44. Mercati, F, Maranesi, M, Dall Aglio, C, Petrucci, L, Pasquariello, R, Tardella, FM, et al. Apelin system in mammary gland of sheep reared in semi-natural pastures of the central apennines. Animals. (2018) 8:223. doi: 10.3390/ani8120223
45. Chang, TK, Zhong, YH, Liu, SC, Huang, CC, Tsai, CH, Lee, HP, et al. Apelin promotes endothelial progenitor cell angiogenesis in rheumatoid arthritis disease via the miR-525-5p/angiopoietin-1 pathway. Front Immunol. (2021) 12:737990. doi: 10.3389/fimmu.2021.737990
46. Knauf, C, Drougard, A, Fournel, A, Duparc, T, and Valet, P. Hypothalamic actions of apelin on energy metabolism: new insight on glucose homeostasis and metabolic disorders. Horm Metab Res. (2013) 45:928–34. doi: 10.1055/s-0033-1351321
47. Banerjee, A, and Singh, J. Remodeling adipose tissue inflammasome for type 2 diabetes mellitus treatment: current perspective and translational strategies. Bioeng Transl Med. (2020) 5:e10150. doi: 10.1002/btm2.10150
48. Bertrand, C, Valet, P, and Castan-Laurell, I. Apelin and energy metabolism. Front Physiol. (2015) 6:115. doi: 10.3389/fphys.2015.00115
49. Afshounpour, MT, Habibi, A, and Ranjbar, R. Impact of combined exercise training on plasma concentration of Apelin, resistin and insulin resistance in patients with type 2 diabetics’ male. Hormozgan Med J. (2016) 20:158–69.
50. Ceranowicz, P, Warzecha, Z, and Dembinski, A. Peptidyl hormones of endocrine cells origin in the gut—their discovery and physiological relevance. J Physiol Pharmacol. (2015) 66:11–27. doi: 10.26402/jpp.2015.1.01
51. Norvezh, F, Jalali, MR, Tabandeh, MR, Hajikolaei, MRH, and Gooraninejad, S. Serum Apelin-36 alteration in late pregnancy and early lactation of dairy cows and its association with negative energy balance markers. Res Vet Sci. (2019) 125:285–9. doi: 10.1016/j.rvsc.2019.07.012
52. Weber, M, Locher, L, Huber, K, Kenéz, Á, Rehage, J, Tienken, R, et al. Longitudinal changes in adipose tissue of dairy cows from late pregnancy to lactation. Part 1: the adipokines apelin and resistin and their relationship to receptors linked with lipolysis. J Dairy Sci. (2016) 99:1549–59. doi: 10.3168/jds.2015-10131
53. Nadal, A, Quesada, I, Tuduri, E, Nogueiras, R, and Alonso-Magdalena, P. Endocrine-disrupting chemicals and the regulation of energy balance. Nat Rev Endocrinol. (2017) 13:536–46. doi: 10.1038/nrendo.2017.51
54. Than, A, Cheng, Y, Foh, L-C, Leow, MK-S, Lim, SC, Chuah, YJ, et al. Apelin inhibits adipogenesis and lipolysis through distinct molecular pathways. Mol Cell Endocrinol. (2012) 362:227–41. doi: 10.1016/j.mce.2012.07.002
55. Yue, P, Jin, H, Xu, S, Aillaud, M, Deng, AC, Azuma, J, et al. Apelin decreases lipolysis via Gq, Gi, and AMPK-dependent mechanisms. Endocrinology. (2011) 152:59–68. doi: 10.1210/en.2010-0576
56. Xu, S, Tsao, PS, and Yue, P. Apelin and insulin resistance: another arrow for the quiver? J Diabetes. (2011) 3:225–31. doi: 10.1111/j.1753-0407.2011.00132.x
57. Guo, R, Rogers, O, and Nair, S. Targeting Apelinergic system in Cardiometabolic disease. Curr Drug Targets. (2016) 17:1–7.
58. Girault-Sotias, P-E, Gerbier, R, Flahault, A, de Mota, N, and Llorens-Cortes, C. Apelin and vasopressin: the yin and yang of water balance. Front Endocrinol. (2021) 12:735515. doi: 10.3389/fendo.2021.735515
59. Reaux-Le Goazigo, A, Bodineau, L, De Mota, N, Jeandel, L, Chartrel, N, Knauf, C, et al. Apelin and the proopiomelanocortin system: a new regulatory pathway of hypothalamic α-MSH release. Am J Physiol Endocrinol Metab. (2011) 301:E955–66. doi: 10.1152/ajpendo.00090.2011
60. Charles, CJ, Rademaker, MT, and Richards, AM. Apelin-13 induces a biphasic haemodynamic response and hormonal activation in normal conscious sheep. J Endocrinol. (2006) 189:701–10. doi: 10.1677/joe.1.06804
61. Ohno, S, Yakabi, K, Ro, S, Ochiai, M, Onouchi, T, Sakurada, T, et al. Apelin-12 stimulates acid secretion through an increase of histamine release in rat stomachs. Reg Peptides. (2012) 174:71–8. doi: 10.1016/j.regpep.2011.12.002
62. Lv, S-Y, Yang, Y-J, Qin, Y-J, Xiong, W, and Chen, Q. Effect of centrally administered apelin-13 on gastric emptying and gastrointestinal transit in mice. Peptides. (2011) 32:978–82. doi: 10.1016/j.peptides.2011.01.023
63. Sunter, D, Hewson, AK, and Dickson, SL. Intracerebroventricular injection of apelin-13 reduces food intake in the rat. Neurosci Lett. (2003) 353:1–4. doi: 10.1016/S0304-3940(03)00351-3
64. Frier, BC, Williams, DB, and Wright, DC. The effects of apelin treatment on skeletal muscle mitochondrial content. Am J Physiol Regul Integr Comp Physiol. (2009) 297:R1761–8. doi: 10.1152/ajpregu.00422.2009
65. Gao, Z, Zhong, X, Tan, Y-X, and Liu, D. Apelin-13 alleviates diabetic nephropathy by enhancing nitric oxide production and suppressing kidney tissue fibrosis. Int J Mol Med. (2021) 48:1–9. doi: 10.3892/ijmm.2021.5008
66. Clarke, K, Whitaker, K, and Reyes, T. Diminished metabolic responses to centrally-administered apelin-13 in diet-induced obese rats fed a high-fat diet. J Neuroendocrinol. (2009) 21:83–9. doi: 10.1111/j.1365-2826.2008.01815.x
67. Xu, S, Han, P, Huang, M, Wu, JC, Chang, C, Tsao, PS, et al. In vivo, ex vivo, and in vitro studies on apelin's effect on myocardial glucose uptake. Peptides. (2012) 37:320–6. doi: 10.1016/j.peptides.2012.08.004
68. Fournel, A, Drougard, A, Duparc, T, Marlin, A, Brierley, SM, Castro, J, et al. Apelin targets gut contraction to control glucose metabolism via the brain. Gut. (2017) 66:258–69. doi: 10.1136/gutjnl-2015-310230
69. Than, A, He, HL, Chua, SH, Xu, D, Sun, L, Leow, MK-S, et al. Apelin enhances brown adipogenesis and browning of white adipocytes. J Biol Chem. (2015) 290:14679–91. doi: 10.1074/jbc.M115.643817
70. Liu, Q, Jiang, J, Shi, Y, Mo, Z, and Li, M. Apelin/Apelin receptor: a new therapeutic target in polycystic ovary syndrome. Life Sci. (2020) 260:118310. doi: 10.1016/j.lfs.2020.118310
71. Nyimanu, D, Kuc, RE, Williams, TL, Bednarek, M, Ambery, P, Jermutus, L, et al. Apelin-36-[L28A] and Apelin-36-[L28C (30kDa-PEG)] peptides that improve diet induced obesity are G protein biased ligands at the apelin receptor. Peptides. (2019) 121:170139. doi: 10.1016/j.peptides.2019.170139
72. Attané, C, Daviaud, D, Dray, C, Dusaulcy, R, Masseboeuf, M, Prévot, D, et al. Apelin stimulates glucose uptake but not lipolysis in human adipose tissue ex vivo. J Mol Endocrinol. (2011) 46:21–8. doi: 10.1677/JME-10-0105
73. Zhu, S, Sun, F, Li, W, Cao, Y, Wang, C, Wang, Y, et al. Apelin stimulates glucose uptake through the PI3K/Akt pathway and improves insulin resistance in 3T3-L1 adipocytes. Mol Cell Biochem. (2011) 353:305–13. doi: 10.1007/s11010-011-0799-0
74. Mughal, A, Sun, C, and O’Rourke, ST. Apelin reduces nitric oxide-induced relaxation of cerebral arteries by inhibiting activation of large conductance, calcium-activated K channels. J Cardiovasc Pharmacol. (2018) 71:223–32. doi: 10.1097/FJC.0000000000000563
75. Respekta, N, Pich, K, Dawid, M, Mlyczyńska, E, Kurowska, P, and Rak, A. The Apelinergic system: Apelin, ELABELA, and APJ action on cell apoptosis: anti-apoptotic or pro-apoptotic effect? Cells. (2022) 12:150. doi: 10.3390/cells12010150
76. Penney, CC, and Volkoff, H. Peripheral injections of cholecystokinin, apelin, ghrelin and orexin in cavefish (Astyanax fasciatus mexicanus): effects on feeding and on the brain expression levels of tyrosine hydroxylase, mechanistic target of rapamycin and appetite-related hormones. Gen Comp Endocrinol. (2014) 196:34–40. doi: 10.1016/j.ygcen.2013.11.015
77. Yan, X, Qin, C, Yang, G, Deng, D, Yang, L, Feng, J, et al. The regulatory role of apelin on the appetite and growth of common carp (Cyprinus carpio L.). Animals. (2020) 10:163. doi: 10.3390/ani10112163
78. Maresca, F, Palma, VD, Bevilacqua, M, Uccello, G, Taglialatela, V, Giaquinto, A, et al. Adipokines, vascular wall, and cardiovascular disease: a focused overview of the role of adipokines in the pathophysiology of cardiovascular disease. Angiology. (2015) 66:8–24. doi: 10.1177/0003319713520463
79. Karnuah, AB, Uenishi, H, Kiuchi, S, Kojima, M, Onishi, A, Yasue, H, et al. Assignment of 64 genes expressed in 28-day-old pig embryo to radiation hybrid map. Mamm Genome. (2001) 12:518–23. doi: 10.1007/s003350020035
80. Yonezawa, T, Haga, S, Kobayashi, Y, Takahashi, T, and Obara, Y. Visfatin is present in bovine mammary epithelial cells, lactating mammary gland and milk, and its expression is regulated by cAMP pathway. FEBS Lett. (2006) 580:6635–43. doi: 10.1016/j.febslet.2006.11.014
81. Dahl, TB, Holm, S, Aukrust, P, and Halvorsen, B. Visfatin/NAMPT: a multifaceted molecule with diverse roles in physiology and pathophysiology. Annu Rev Nutr. (2012) 32:229–43. doi: 10.1146/annurev-nutr-071811-150746
82. Butler, MJ, and Volkoff, H. The role of visfatin/NAMPT in the regulation of feeding in goldfish (Carassius auratus). Peptides. (2023) 160:170919. doi: 10.1016/j.peptides.2022.170919
83. Garten, A, Schuster, S, Penke, M, Gorski, T, De Giorgis, T, and Kiess, W. Physiological and pathophysiological roles of NAMPT and NAD metabolism. Nat Rev Endocrinol. (2015) 11:535–46. doi: 10.1038/nrendo.2015.117
84. Romacho, T, Elsen, M, Röhrborn, D, and Eckel, J. Adipose tissue and its role in organ crosstalk. Acta Physiol. (2014) 210:733–53. doi: 10.1111/apha.12246
85. Revollo, JR, Körner, A, Mills, KF, Satoh, A, Wang, T, Garten, A, et al. Nampt/PBEF/visfatin regulates insulin secretion in β cells as a systemic NAD biosynthetic enzyme. Cell Metab. (2007) 6:363–75. doi: 10.1016/j.cmet.2007.09.003
86. Revollo, JR, Grimm, AA, and Imai, S. The regulation of nicotinamide adenine dinucleotide biosynthesis by Nampt/PBEF/visfatin in mammals. Curr Opin Gastroenterol. (2007) 23:164–70. doi: 10.1097/MOG.0b013e32801b3c8f
87. Costford, SR, Bajpeyi, S, Pasarica, M, Albarado, DC, Thomas, SC, Xie, H, et al. Skeletal muscle NAMPT is induced by exercise in humans. Am J Physiol Endocrinol Metab. (2010) 298:E117–26. doi: 10.1152/ajpendo.00318.2009
88. Maillard, V, Elis, S, Desmarchais, A, Hivelin, C, Lardic, L, Lomet, D, et al. Visfatin and resistin in gonadotroph cells: expression, regulation of LH secretion and signalling pathways. Reprod Fertil Dev. (2017) 29:2479–95. doi: 10.1071/RD16301
89. Ons, E, Gertler, A, Buyse, J, Lebihan-Duval, E, Bordas, A, Goddeeris, B, et al. Visfatin gene expression in chickens is sex and tissue dependent. Domest Anim Endocrinol. (2010) 38:63–74. doi: 10.1016/j.domaniend.2009.08.007
90. Zhang, Y, Huo, Y, He, W, Liu, S, Li, H, and Li, L. Visfatin is regulated by interleukin 6 and affected by the PPAR γ pathway in BeWo cells. Mol Med Rep. (2019) 19:400–6. doi: 10.3892/mmr.2018.9671
91. Stromsdorfer, KL, Yamaguchi, S, Yoon, MJ, Moseley, AC, Franczyk, MP, Kelly, SC, et al. NAMPT-mediated NAD+ biosynthesis in adipocytes regulates adipose tissue function and multi-organ insulin sensitivity in mice. Cell Rep. (2016) 16:1851–60. doi: 10.1016/j.celrep.2016.07.027
92. Saddi-Rosa, P, Oliveira, CS, Giuffrida, F, and Reis, AF. Visfatin, glucose metabolism and vascular disease: a review of evidence. Diabetol Metab Syndr. (2010) 2:1–6. doi: 10.1186/1758-5996-2-21
93. Mayi, TH, Duhem, C, Copin, C, Bouhlel, MA, Rigamonti, E, Pattou, F, et al. Visfatin is induced by peroxisome proliferator-activated receptor gamma in human macrophages. FEBS J. (2010) 277:3308–20. doi: 10.1111/j.1742-4658.2010.07729.x
94. Yang, C, Deng, S, Hsu, C, Liu, B, Lin, E, Cheng, W, et al. Visfatin regulates genes related to lipid metabolism in porcine adipocytes. J Anim Sci. (2010) 88:3233–41. doi: 10.2527/jas.2010-2799
95. Pourvali-Talatappeh, P, and Alipoor, E. Visfatin; a potential novel mediator of brown adipose tissue. Obes Med. (2019) 15:100122. doi: 10.1016/j.obmed.2019.100122
96. Cline, MA, Nandar, W, Prall, BC, Bowden, CN, and Denbow, DM. Central visfatin causes orexigenic effects in chicks. Behav Brain Res. (2008) 186:293–7. doi: 10.1016/j.bbr.2007.08.016
97. Chang, S, Lohakare, J, Singh, N, Kwon, E, Nejad, J, Sung, K, et al. Limiting concentrate during growing period affect performance and gene expression of hepatic gluconeogenic enzymes and visfatin in Korean native beef calves. Asian Australas J Anim Sci. (2013) 26:202–10. doi: 10.5713/ajas.2012.12442
98. Palin, M-F, Labrecque, B, Beaudry, D, Mayhue, M, Bordignon, V, and Murphy, BD. Visfatin expression is not associated with adipose tissue abundance in the porcine model. Domest Anim Endocrinol. (2008) 35:58–73. doi: 10.1016/j.domaniend.2008.01.008
99. Sun, Q, Li, L, Li, R, Yang, M, Liu, H, Nowicki, MJ, et al. Overexpression of visfatin/PBEF/Nampt alters whole-body insulin sensitivity and lipid profile in rats. Annal Med. (2009) 41:311–20. doi: 10.1080/07853890902729760
100. Kang, YS, Lee, MH, Song, HK, Kim, JE, Ghee, JY, Cha, JJ, et al. Chronic administration of visfatin ameliorated diabetic nephropathy in type 2 diabetic mice. Kidney Blood Pres Res. (2016) 41:311–24. doi: 10.1159/000443433
101. Li, Z, Liu, X, Zhang, P, Han, R, Sun, G, Jiang, R, et al. Comparative transcriptome analysis of hypothalamus-regulated feed intake induced by exogenous visfatin in chicks. BMC Genomics. (2018) 19:1–17. doi: 10.1186/s12864-018-4644-7
102. Bostrom, P, Wu, J, Jedrychowski, MP, Korde, A, Ye, L, Lo, JC, et al. A PGC1-alpha-dependent myokine that drives brown-fat-like development of white fat and thermogenesis. Nature. (2012) 481:463–8. doi: 10.1038/nature10777
103. Ferrer-Martinez, A, Ruiz-Lozano, P, and Chien, KR. Mouse PeP: a novel peroxisomal protein linked to myoblast differentiation and development. Dev Dyn. (2002) 224:154–67. doi: 10.1002/dvdy.10099
104. Teufel, A, Malik, N, Mukhopadhyay, M, and Westphal, H. Frcp1 and Frcp2, two novel fibronectin type III repeat containing genes. Gene. (2002) 297:79–83. doi: 10.1016/S0378-1119(02)00828-4
105. Jodeiri Farshbaf, M, and Alviña, K. Multiple roles in neuroprotection for the exercise derived myokine irisin. Front Aging Neurosci. (2021) 13:649929. doi: 10.3389/fnagi.2021.649929
106. Jamwal, S, Blackburn, JK, and Elsworth, JD. PPARγ/PGC1α signaling as a potential therapeutic target for mitochondrial biogenesis in neurodegenerative disorders. Pharmacol Ther. (2021) 219:107705. doi: 10.1016/j.pharmthera.2020.107705
107. Ahmadian, M, Suh, JM, Hah, N, Liddle, C, Atkins, AR, Downes, M, et al. PPARγ signaling and metabolism: the good, the bad and the future. Nat Med. (2013) 19:557–66. doi: 10.1038/nm.3159
108. Han, L, Shen, W-J, Bittner, S, Kraemer, FB, and Azhar, S. PPARs: regulators of metabolism and as therapeutic targets in cardiovascular disease. Part II: PPAR-β/δ and PPAR-γ. Futur Cardiol. (2017) 13:279–96. doi: 10.2217/fca-2017-0019
109. Hong, F, Pan, S, Guo, Y, Xu, P, and Zhai, Y. PPARs as nuclear receptors for nutrient and energy metabolism. Molecules. (2019) 24:2545. doi: 10.3390/molecules24142545
110. Dabrowska, A, Venero, JL, Iwasawa, R, Hankir, M-k, Rahman, S, Boobis, A, et al. PGC-1α controls mitochondrial biogenesis and dynamics in lead-induced neurotoxicity. Aging (Albany NY). (2015) 7:629–43. doi: 10.18632/aging.100790
111. Kızıl, M, Rişvanli, A, Abay, M, Şafak, T, Kılınç, MA, Yılmaz, Ö, et al. Effect of calf delivery mode on İrisin, Asprosin, leptin, adiponectin, and İnsulin-like growth Factor-1 levels in dairy cattle and their calves. Turk J Vet Anim Sci. (2022) 55:1527. doi: 10.17582/journal.pjz/20221031081040
112. Eğritağ, HE, Merhan, O, Bozukluhan, K, Varol, K, and Atcali, T. Investigation of serum leptin, ghrelin, irisin, insulin levels and their correlations in cattle with subclinical ketosis. Vet J Mehmet Akif Ersoy Univ. (2022) 7:223–8. doi: 10.24880/maeuvfd.1202455
113. Daudon, M, Ramé, C, Price, C, and Dupont, J. Irisin modulates glucose metabolism and inhibits steroidogenesis in bovine granulosa cells. Reproduction. (2023) 165:533–42. doi: 10.1530/REP-22-0404
114. Sahin-Efe, A, Upadhyay, J, Ko, B-J, Dincer, F, Park, KH, Migdal, A, et al. Irisin and leptin concentrations in relation to obesity, and developing type 2 diabetes: a cross sectional and a prospective case-control study nested in the normative aging study. Metabolism. (2018) 79:24–32. doi: 10.1016/j.metabol.2017.10.011
115. Li, B, Yao, Q, Guo, S, Ma, S, Dong, Y, Xin, H, et al. Type 2 diabetes with hypertensive patients results in changes to features of adipocytokines: leptin, Irisin, LGR4, and Sfrp5. Clin Exp Hypertens. (2019) 41:645–50. doi: 10.1080/10641963.2018.1529779
116. Stengel, A, Hofmann, T, Goebel-Stengel, M, Elbelt, U, Kobelt, P, and Klapp, BF. Circulating levels of irisin in patients with anorexia nervosa and different stages of obesity–correlation with body mass index. Peptides. (2013) 39:125–30. doi: 10.1016/j.peptides.2012.11.014
117. De Meneck, F, de Souza, LV, Oliveira, V, and do Franco, M. High irisin levels in overweight/obese children and its positive correlation with metabolic profile, blood pressure, and endothelial progenitor cells. Nutr Metab Cardiovasc Dis. (2018) 28:756–64. doi: 10.1016/j.numecd.2018.04.009
118. Komolka, K, Albrecht, E, Schering, L, Brenmoehl, J, Hoeflich, A, and Maak, S. Locus characterization and gene expression of bovine FNDC5: is the myokine irisin relevant in cattle? PLoS One. (2014) 9:e88060. doi: 10.1371/journal.pone.0088060
119. Albrecht, E, Schering, L, Buck, F, Vlach, K, Schober, H, Drevon, C, et al. Irisin: still chasing shadows. Mol Metab. (2020) 34:124–35. doi: 10.1016/j.molmet.2020.01.016
120. Kirat, D, Hamada, M, Moustafa, A, and Miyasho, T. Irisin/FNDC5: A participant in camel metabolism. Saudi J Biol Sci. (2021) 28:693–706. doi: 10.1016/j.sjbs.2020.10.061
121. Basini, G, Bussolati, S, Iannarelli, M, Ragionieri, L, Grolli, S, Ramoni, R, et al. The myokine irisin: localization and effects in swine late medium and large antral ovarian follicle. Domest Anim Endocrinol. (2021) 74:106576. doi: 10.1016/j.domaniend.2020.106576
122. Kowalik, S, Wiśniewska, A, Kędzierski, W, and Janczarek, I. Concentrations of circulating Irisin and Myostatin in race and Endurace purebred Arabian horses—preliminary study. Animals. (2020) 10:2268. doi: 10.3390/ani10122268
123. Marrano, N, Biondi, G, Borrelli, A, Cignarelli, A, Perrini, S, Laviola, L, et al. Irisin and incretin hormones: similarities, differences, and implications in type 2 diabetes and obesity. Biomol Ther. (2021) 11:286. doi: 10.3390/biom11020286
124. Wang, S, and Pan, J. Irisin ameliorates depressive-like behaviors in rats by regulating energy metabolism. Biochem Biophys Res Commun. (2016) 474:22–8. doi: 10.1016/j.bbrc.2016.04.047
125. Aydin, S, Kuloglu, T, Aydin, S, Kalayci, M, Yilmaz, M, Cakmak, T, et al. A comprehensive immunohistochemical examination of the distribution of the fat-burning protein irisin in biological tissues. Peptides. (2014) 61:130–6. doi: 10.1016/j.peptides.2014.09.014
126. Xiong, XQ, Chen, D, Sun, HJ, Ding, L, Wang, JJ, Chen, Q, et al. FNDC5 overexpression and irisin ameliorate glucose/lipid metabolic derangements and enhance lipolysis in obesity. Biochim Biophys Acta. (2015) 1852:1867–75. doi: 10.1016/j.bbadis.2015.06.017
127. So, WY, and Leung, PS. Irisin ameliorates hepatic glucose/lipid metabolism and enhances cell survival in insulin-resistant human HepG2 cells through adenosine monophosphate-activated protein kinase signaling. Int J Biochem Cell Biol. (2016) 78:237–47. doi: 10.1016/j.biocel.2016.07.022
128. Xin, C, Liu, J, Zhang, J, Zhu, D, Wang, H, Xiong, L, et al. Irisin improves fatty acid oxidation and glucose utilization in type 2 diabetes by regulating the AMPK signaling pathway. Int J Obes. (2016) 40:443–51. doi: 10.1038/ijo.2015.199
129. Zhang, Y, Li, R, Meng, Y, Li, S, Donelan, W, Zhao, Y, et al. Irisin stimulates browning of white adipocytes through mitogen-activated protein kinase p38 MAP kinase and ERK MAP kinase signaling. Diabetes. (2014) 63:514–25. doi: 10.2337/db13-1106
130. Lee, TH, Formolo, DA, Kong, T, Lau, SW, Ho, CS, Leung, RYH, et al. Potential exerkines for physical exercise-elicited pro-cognitive effects: insight from clinical and animal research. Int Rev Neurobiol. (2019) 147:361–95. doi: 10.1016/bs.irn.2019.06.002
131. Ozcan, S, Ulker, N, Bulmus, O, Yardimci, A, Ozcan, M, and Canpolat, S. The modulatory effects of irisin on asprosin, leptin, glucose levels and lipid profile in healthy and obese male and female rats. Arch Phys Biochem. (2022) 128:724–31. doi: 10.1080/13813455.2020.1722706
132. Majeed, S, Shafi, R, Moin, H, Ashraf, I, Irshad, K, and Liaquat, A. Effects of recombinant irisin on body mass index, serum insulin, luteinizing hormone and testosterone levels in obese female BALB/c mice. J Coll Physicians Surg Pak. (2019) 29:736–40. doi: 10.29271/jcpsp.2019.08.736
133. Mansour, SW, Hasan, MM, Salah, HE, El-Deep, T, Hussein, S, and El-Malkey, NF. Effect of irisin on metabolic and platelet functions in type 2 diabetic rats: role of soluble receptor of advanced glycation end products (sRAGE). Beni-Suef univ J Basic Appl Sci. (2021) 10:1–7. doi: 10.1186/s43088-021-00148-1
134. Abozeid, M, and Abeer, A. Potential effect of Irisin and/or moderate intensity exercise on metabolic homeostasis in obesity in male albino rats. Med J Cairo Uni. (2019) 87:5255–62. doi: 10.21608/mjcu.2019.89764
135. Dehghan, F, Zamani, S, Barreiro, C, and Jami, MS. Irisin injection mimics exercise effects on the brain proteome. Eur J Neurosci. (2021) 54:7422–41. doi: 10.1111/ejn.15493
136. Huang, L, Yan, S, Luo, L, and Yang, L. Irisin regulates the expression of BDNF and glycometabolism in diabetic rats. Mol Med Rep. (2019) 19:1074–82. doi: 10.3892/mmr.2018.9743
137. Esposito, G, Irons, PC, Webb, EC, and Chapwanya, A. Interactions between negative energy balance, metabolic diseases, uterine health and immune response in transition dairy cows. Anim Reprod Sci. (2014) 144:60–71. doi: 10.1016/j.anireprosci.2013.11.007
138. Rumphorst, T, Scheu, T, Koch, C, and Sundrum, A. Balancing trade-offs in Milk production by making use of animal individual energy balancing. Dairy. (2022) 3:345–63. doi: 10.3390/dairy3020027
139. Ladyman, SR, and Brooks, VL. Central actions of insulin during pregnancy and lactation. J Neuroendocrinol. (2021) 33:e12946. doi: 10.1111/jne.12946
140. Accorsi, P, Govoni, N, Gaiani, R, Pezzi, C, Seren, E, and Tamanini, C. Leptin, GH, PRL, insulin and metabolic parameters throughout the dry period and lactation in dairy cows. Reprod Domest Anim. (2005) 40:217–23. doi: 10.1111/j.1439-0531.2005.00581.x
141. Piccione, G, Messina, V, Marafioti, S, Casella, S, Giannetto, C, and Fazio, F. Changes of some haematochemical parameters in dairy cows during late gestation, post partum, lactation and dry periods. Vet Med Zoot. (2012) 58:59–64.
142. Fiore, E, Piccione, G, Rizzo, M, Morgante, M, Barberio, A, Giudice, E, et al. Adaptation of some energetic parameters during transition period in dairy cows. J Appl Anim Res. (2018) 46:402–5. doi: 10.1080/09712119.2017.1313742
143. Pascottini, OB, Leroy, JL, and Opsomer, G. Metabolic stress in the transition period of dairy cows: focusing on the prepartum period. Animals. (2020) 10:1419. doi: 10.3390/ani10081419
144. Ospina, P, Nydam, D, Stokol, T, and Overton, T. Evaluation of nonesterified fatty acids and β-hydroxybutyrate in transition dairy cattle in the northeastern United States: critical thresholds for prediction of clinical diseases. J Dairy Sci. (2010) 93:546–54. doi: 10.3168/jds.2009-2277
145. Habata, Y, Fujii, R, Hosoya, M, Fukusumi, S, Kawamata, Y, Hinuma, S, et al. Apelin, the natural ligand of the orphan receptor APJ, is abundantly secreted in the colostrum. Biochim Biophys Acta, Mol Cell Res. (1999) 1452:25–35. doi: 10.1016/S0167-4889(99)00114-7
146. Mesmin, C, Fenaille, F, Becher, F, Tabet, J-C, and Ezan, E. Identification and characterization of apelin peptides in bovine colostrum and milk by liquid chromatography–mass spectrometry. J Proteome Res. (2011) 10:5222–31. doi: 10.1021/pr200725x
147. Moschen, AR, Kaser, A, Enrich, B, Mosheimer, B, Theurl, M, Niederegger, H, et al. Visfatin, an adipocytokine with proinflammatory and immunomodulating properties. J Immunol. (2007) 178:1748–58. doi: 10.4049/jimmunol.178.3.1748
148. Lemor, A, Hosseini, A, Sauerwein, H, and Mielenz, M. Transition period-related changes in the abundance of the mRNAs of adiponectin and its receptors, of visfatin, and of fatty acid binding receptors in adipose tissue of high-yielding dairy cows. Domest Anim Endocrinol. (2009) 37:37–44. doi: 10.1016/j.domaniend.2009.01.004
149. Li, X, Fu, X, Yang, G, and Du, M. Enhancing intramuscular fat development via targeting fibro-adipogenic progenitor cells in meat animals. Animal. (2020) 14:312–21. doi: 10.1017/S175173111900209X
150. Rashid, FA, Abbas, HJ, Naser, NA, and Ali, A. Effect of long-term moderate physical exercise on irisin between normal weight and obese men. Sci World J. (2020) 2020:1–7. doi: 10.1155/2020/1897027
151. Li, L, Yang, G, Li, Q, Tang, Y, Yang, M, Yang, H, et al. Changes and relations of circulating visfatin, apelin, and resistin levels in normal, impaired glucose tolerance, and type 2 diabetic subjects. Exp Clin Endocrinol Diabetes. (2006) 114:544–8. doi: 10.1055/s-2006-948309
152. Roche, J, Ramé, C, Reverchon, M, Mellouk, N, Rak, A, Froment, P, et al. Apelin (APLN) regulates progesterone secretion and oocyte maturation in bovine ovarian cells. Reproduction. (2017) 153:589–603. doi: 10.1530/REP-16-0677
153. Rak, A, Drwal, E, Rame, C, Knapczyk-Stwora, K, Słomczyńska, M, Dupont, J, et al. Expression of apelin and apelin receptor (APJ) in porcine ovarian follicles and in vitro effect of apelin on steroidogenesis and proliferation through APJ activation and different signaling pathways. Theriogenology. (2017) 96:126–35. doi: 10.1016/j.theriogenology.2017.04.014
154. Shokrollahi, B, Zheng, H-Y, Li, L-Y, Tang, L-P, Ma, X-Y, Lu, X-R, et al. Apelin and apelin receptor in follicular granulosa cells of buffalo ovaries: expression and regulation of steroidogenesis. Front Endocrinol. (2022) 13:844360. doi: 10.3389/fendo.2022.844360
155. Gupta, M, Korde, JP, Bahiram, K, Sardar, V, and Kurkure, NV. Expression and localization of apelin and apelin receptor (APJ) in buffalo ovarian follicles and corpus luteum and the in-vitro effect of apelin on steroidogenesis and survival of granulosa cells. Theriogenology. (2023) 197:240–51. doi: 10.1016/j.theriogenology.2022.12.013
156. Shokrollahi, B, Zheng, H-Y, Ma, X-Y, and Shang, J-H. The effects of apelin on IGF1/FSH-induced steroidogenesis, proliferation, Bax expression, and total antioxidant capacity in granulosa cells of buffalo ovarian follicles. Vet Res Commun. (2023) 47:1523–33. doi: 10.1007/s11259-021-09799-4
157. Reverchon, M, Rame, C, Bunel, A, Chen, W, Froment, P, and Dupont, J. VISFATIN (NAMPT) improves in vitro IGF1-induced steroidogenesis and IGF1 receptor signaling through SIRT1 in bovine granulosa cells. Biol Reprod. (2016) 94:54. doi: 10.1095/biolreprod.115.134650
158. Diot, M, Reverchon, M, Rame, C, Froment, P, Brillard, J-P, Brière, S, et al. Expression of adiponectin, chemerin and visfatin in plasma and different tissues during a laying season in turkeys. Reprod Biol Endocrinol. (2015) 13:1–14. doi: 10.1186/s12958-015-0081-5
159. Thakre, A, Gupta, M, Magar, S, Bahiram, K, Sardar, V, Korde, J, et al. Transcriptional and translational abundance of visfatin (NAMPT) in buffalo ovary during estrous cycle and its in vitro effect on steroidogenesis. Domest Anim Endocrinol. (2021) 75:106583. doi: 10.1016/j.domaniend.2020.106583
160. Sato, K, Takahashi, T, Kobayashi, Y, Hagino, A, Roh, S, and Katoh, K. Apelin is involved in postprandial responses and stimulates secretion of arginine-vasopressin, adrenocorticotropic hormone, and growth hormone in the ruminant. Domest Anim Endocrinol. (2012) 42:165–72. doi: 10.1016/j.domaniend.2011.11.006
161. Bayraktar, B, Tekce, E, Aksakal, V, Takma, Ç, Bayraktar, FG, and Şengül, B. Effects of race, gender, body condition score and pregnancy on serum Apelin levels in ewe. J Agric Sci. (2020) 26:363–72. doi: 10.15832/ankutbd.526907
Keywords: apelin, visfatin, irisin, energy regulation, farm animals
Citation: Shokrollahi B, Jang S-S, Lee HJ, Ahmad HI, Sesay AR, Ghazikhani Shad A, Morammazi S and Abdelnour SA (2024) Exploring the potential roles of apelin, visfatin, and irisin in energy regulation in farm animals: an overview. Front. Vet. Sci. 11:1435788. doi: 10.3389/fvets.2024.1435788
Received: 21 May 2024; Accepted: 05 July 2024;
Published: 19 July 2024.
Edited by:
Elena De Felice, University of Camerino, ItalyReviewed by:
Francesca Mercati, University of Perugia, ItalyCopyright © 2024 Shokrollahi, Jang, Lee, Ahmad, Sesay, Ghazikhani Shad, Morammazi and Abdelnour. This is an open-access article distributed under the terms of the Creative Commons Attribution License (CC BY). The use, distribution or reproduction in other forums is permitted, provided the original author(s) and the copyright owner(s) are credited and that the original publication in this journal is cited, in accordance with accepted academic practice. No use, distribution or reproduction is permitted which does not comply with these terms.
*Correspondence: Borhan Shokrollahi, Qm9yaGFuc2hAZ21haWwuY29t
†These authors have contributed equally to this work
Disclaimer: All claims expressed in this article are solely those of the authors and do not necessarily represent those of their affiliated organizations, or those of the publisher, the editors and the reviewers. Any product that may be evaluated in this article or claim that may be made by its manufacturer is not guaranteed or endorsed by the publisher.
Research integrity at Frontiers
Learn more about the work of our research integrity team to safeguard the quality of each article we publish.