- 1Chongqing Academy of Animal Sciences, Chongqing, China
- 2College of Veterinary Medicine, Southwest University, Chongqing, China
- 3College of Animal Science and Technology, Southwest University, Chongqing, China
- 4Mammalian NutriPhysioGenomics, Department of Animal Sciences and Division of Nutritional Sciences, University of Illinois, Urbana, IL, United States
Introduction: The ratio of lysine (Lys) to methionine (Met) with 3.0: 1 is confirmed as the “ideal” profile for milk protein synthesis, but whether this ratio is suitable for milk protein synthesis under HS needs to be further studied.
Methods: To evaluate the molecular mechanism by which HS and Lys to Met ratios affect mammary cell functional capacity, an immortalized bovine mammary epithelial cell line (MAC-T) is incubated with 5 doses of Met while maintaining a constant concentration of Lys. The MAC-T cells was treated for 6 h as follow: Lys: Met 3.0: 1 (control 37°C and IPAA 42°C) or treatments under HS (42°C) with different ratios of Lys: Met at 2.0: 1 (LM20), 2.5: 1 (LM25), 3.5: 1 (LM35) and 4.0: 1 (LM40). RNA sequencing was used to assess transcriptome-wide alterations in mRNA abundance.
Results: The significant difference between control and other groups was observed base on PCA analysis. A total of 2048 differentially expressed genes (DEGs) were identified in the IPAA group relative to the control group. Similarly, 226, 306, 148, 157 DEGs were detected in the LM20, LM25, LM35 and LM40 groups, respectively, relative to the IPAA group. The relative mRNA abundance of HSPA1A was upregulated and anti-apoptotic genes (BCL2L1 and BCL2) was down-regulated in the IPAA group, compared to the control group (p < 0.05). Compared with the IPAA group, the relative mRNA abundance of anti-apoptotic genes and casein genes (CSN1S2 and CSN2) was up-regulated in the LM25 group (p < 0.05). The DEGs between LM25 and IPAA groups were associated with the negative regulation of transcription RNA polymerase II promoter in response to stress (GO: 0051085, DEGs of BAG3, DNAJB1, HSPA1A) as well as the mTOR signaling pathway (ko04150, DEGs of ATP6V1C2, WNT11, WNT3A, and WNT9A). Several DEGs involved in amino acids metabolism (AFMID, HYKK, NOS3, RIMKLB) and glycolysis/gluconeogenesis (AFMID and MGAT5B) were up-regulated while DEGs involved in lipolysis and beta-oxidation catabolic processes (ALOX12 and ALOX12B) were down-regulated.
Conclusion: These results suggested that increasing Met supply (Lys: Met at 2.5: 1) may help mammary gland cells resist HS-induced cell damage, while possibly maintaining lactation capacity through regulation of gene expression.
1 Introduction
In a high temperature and humidity environment, the imbalance between heat accumulation and dissipation in dairy cows can induce heat stress (HS) (1). Once the ambient temperature rises above the threshold and the body is unable to dissipate heat effectively, the cow will be under HS due to the disruption of internal homeostasis (2). Due to the innate self-protection mechanisms of animals, several biological processes are initiated, such as reducing dry matter intake and rumination time, increasing respiratory rate and hormone secretion, to alleviate the negative effects of HS (3). The lactation performance of dairy cows is severely negatively affected by HS, which seriously undermines the economic efficiency of dairy farming.
Mammary gland is the most important organ for milk synthesis and secretion. In addition to the decline in milk production, the protein content of milk also decreases during the hot summer months (4, 5), partly due to the direct negative impact on milk protein synthesis in the mammary gland (6). Milk synthesis and secretion are considered system processes incredibly sensitive to both physiological and environmental factors (7–9). Previous study suggested that inadequate feed intake, changes in postabsorptive metabolism and nutrient partitioning may contribute to discordant changes in mammary protein synthesizing capacity in heat-stressed cows (10). In addition, the apoptosis rate of mammary epithelial cells in dairy cows was increased under HS conditions, and cytoskeletal and cell transport functions were disturbed (11–13). In modern dairy farms, managers adopt a variety of approaches to alleviate or prevent the occurrence of HS in dairy cows, and some feed additives [betaine (14), choline (15), taurine (16) and methionine (17)] seem to be effective in alleviating negative effects of HS.
Methionine (Met) and lysine (Lys) are the most-limiting amino acids in a large range of diets for dairy cows (18). Previous studies indicated that an approximately 3.0: 1 ratio of Lys to Met in dietary metabolic proteins can increase the yield of milk protein to an optimal level (19, 20), which is considered as the “ideal” amino acid profile (IPAA) for milk protein synthesis. However, during HS, the uptake of amino acids (including Met) of dairy cows is altered, resulting in inhibition of the synthesis of milk protein content (21). Increasing the supply of Met in bovine mammary epithelial cells (BMECs) reduced apoptosis and necrosis, decreased lipid peroxidation, and increased the activities of superoxide dismutase, catalase, and glutathione peroxidase, resulting in comprehensive cytoprotective effects under high temperature conditions (17, 22, 23). Thus, whether the 3.0: 1 ratio of Lys: Met is ideal to promote milk protein synthesis under HS conditions and the regulatory mechanisms are not well known.
Our hypothesis was that changing the ratio of Lys: Met by increasing or decreasing Met supplementation could be a way to help mitigate the negative impact of HS on BMECs. To address this hypothesis, an immortalized bovine mammary epithelial cell line (MAC-T) was cultured with different ambient temperature conditions: thermo-neutral (37°C) and HS (42°C), and 5 media contains 175 mM Lys and varying Met concentrations (58 mM, 44 mM, 50 mM, 70 mM, and 87 mM). The RNA sequencing (RNA-Seq) approach was used to identify the molecular mechanisms regulated by changes in Met supplementation.
2 Materials and methods
2.1 Cell culture and treatments
An immortalized bovine mammary cell line (MAC-T) was chosen as the model. The MAC-T cells were derived from our laboratory, and the cell culture protocol followed our previous similar study with minor modifications (24). Briefly, the thawed bovine MAC-T cells were cultured in 75cm2 flasks using an incubator at 37°C and 5% CO2 until sufficient cells were obtained for subsequent experiments. The basal medium was prepared with minimum essential medium with Earle’s balanced salts (GE Healthcare Life Sciences, Logan, UT) and fetal bovine serum at a ratio of 9.0: 1, and supplemented with 5 mg/L insulin, 1 mg/L hydrocortisone, 5 mg/L transferrin, 5 μM ascorbic acid, 5 mM sodium acetate, 100 U/mL penicillin, 100 μg/mL streptomycin, 0.25 μg/mL antimycotic, 1 mg/L progesterone, 0.05% lactalbumin, and 0.05% α-lactose. When confluency reached 80–90%, the cells were digested with trypsin–EDTA solution and re-inoculated in 6-well plates. The basal medium was replaced by the lactogenic medium when the cell confluency reached 80–90% again, followed by the plates were incubated overnight at 37°C. The lactogenic medium was changed minimum essential medium with Earle’s balanced salts in the basal medium to high-glucose Dulbecco’s modified Eagle’s medium (Hyclone, GE Healthcare Life Sciences), and supplemented with 1 g/L bovine serum albumin and 2.5 mg/L prolactin. Subsequently, the lactogenic medium was changed to the special lactogenic medium containing different ratios of amino acids (as presented in Table 1), and the cells were further at cultured 37°C or 42°C for 6 h (27). Accordingly, there were 6 treatments as follow: 37°C treatment with Lys: Met 3.0: 1 (control), 42°C treatments with Lys: Met at 2.0: 1 (LM20), 2.5: 1 (LM25), 3.0: 1 (IPAA), 3.5: 1 (LM35) and 4.0: 1 (LM40). After incubation for 6 h, cell samples were collected and stored at −80°C until RNA extraction. The reagents and chemicals were purchased from Sigma-Aldrich (St. Louis, MO) unless otherwise stated.
2.2 RNA extraction and RT-qPCR analysis
Total RNA was extracted from MAC-T cells using TRIzol reagent (#15596026, Invitrogen, United States) and RNA quality determined using a NanoDrop 1,000 ND-2000 spectrophotometer (Thermo Scientific, USA). The cDNA synthesis was performed using the PrimeScript RT reagent Kit with gDNA Eraser (Takara Biotechnology, Dalian, China) according to the manufacturer’s instructions. The RT-PCR was performed according to the manufacturer’s instructions using SYBR Premix Ex Taq (Takara Biotechnology, Dalian, China). The cDNA was diluted to 50 ng with RNase-free water and 2 μL of diluted cDNA was combined with the 20 μL reaction mixture. The 20 μL system contained 10 μL of 2 × SYBR Premix Ex Taq (Tli RNsesH Plus), 0.4 μL each of 10 μM forward and reverse primers, 0.4 μL of 50x ROX Reference Dye II and 4.8 μL of RNase-free water. All RT-PCR was performed in a QuantStudio 6 Flex System (Applied Biosystems, Foster City, CA, United States) with the following program: 95°C for 30 s, 40 cycles at 95°C for 5 s, and 60°C for 34 s. The detailed list of primer sequence is presented in Table 2. All primers were commercially manufactured by Sangon Biotech Co., Ltd. (Shanghai, China). Three reference genes (GADPH, UXT, and RPS9) were used to normalize the expression of target genes. The comparative cycle threshold (2−∆∆Ct) method was used to determine the mRNA abundance of target genes (28, 29).
2.3 RNA sequencing
Total RNA was extracted using Trizol reagent (Invitrogen, Carlsbad, CA, United States) according to the manufacturer’s protocols. RNA quality was assessed on an Agilent 2,100 Bioanalyzer (Agilent Technologies, Palo Alto, CA, United States) and checked using RNase free agarose gel electrophoresis. After total RNA was extracted, eukaryotic mRNA was enriched by Oligo (dT) beads, while prokaryotic mRNA was enriched by removing rRNA by Ribo-ZeroTM Magnetic Kit (Epicentre, Madison, WI, USA). Then the enriched mRNA was fragmented into short fragments using fragmentation buffer and reverse-transcribed into cDNA with random primers. Second-strand cDNA was synthesized with DNA polymerase I, RNase H, and dNTP. Then, the cDNA fragments were purified with QiaQuick PCR extraction kit (Qiagen, Venlo, The Netherlands), end repaired, A base added, and ligated to Illumina sequencing adapters. The ligation products were size selected by agarose gel electrophoresis, PCR amplified, and sequenced using Illumina Novaseq6000 by Gene Denovo Biotechnology Co., Ltd. (Guangzhou, China). OD260/OD280 values of all samples were ≥ 1.9, and RNA Integrity Number (RIN) values were ≥ 8.0. The cDNA library was constructed using 3 μg total RNA for each sample. Before the library was constructed, the ribosomal RNA was removed by Epicentre Ribo-zero™ rRNA removal kit (Epicentre, United States), and the total RNA removed by rRNA was cleaned by precipitation with ethanol. The NEB Next®Ultra™ Directional RNA Library Prep Kit for Illumina® (NEB, USA) was then used for library construction using RNA with the rRNA removed. Library sequencing was performed using Illumina HiSeq4000 at Guangzhou Gidio Biotechnology Co., Ltd. The short-read alignment tool Bowtie2 (30) (version 2.2.8, https://bowtie-bio.sourceforge.net/bowtie2/index.shtml) was used for mapping reads to the ribosomal RNA (rRNA) database. An index of the reference genome was built and paired-end clean reads mapped to the reference genome using HISAT 2.2.4 (31) with “-rna-strandness RF” and other parameters set as default. The mapped reads for each sample were assembled with StringTie v1.3.1 (32, 33) in a reference-based approach. Principal component analysis (PCA) was performed with R package gmodels (http://www.rproject.org/). RNA differential expression analysis between two different groups was assessed via DESeq2 (34, 35). The genes/transcripts with a false discovery rate (FDR) below 0.05 and absolute fold change ≥1.5 were considered as differentially expressed genes (DEGs). The DEGs were annotated by Gene ontology (GO) functional enrichment and Kyoto Encyclopedia of Genes and Genomes (KEGG) pathway enrichment using the R programming language (3.5 version, http://www.r-project.org/), based on the hypergeometric distribution.
2.4 Statistical analysis
The mRNA abundance data of each gene were log2 transformed to obtain a normal distribution before statistical analysis. The statistical analysis was performed using the MIXED model in SAS (version 9.3; SAS Institute Inc., Cary, NC, United States) with Lys to Met ratios as the main fixed effect and individual cell culture well as random effect. Treatment means were generated using the LSMEANS option and separated when they were significant with the PDIFF option. Statistical significance was considered at p < 0.05.
3 Results
3.1 Heat shock response
The effects of HS on the mRNA expression of heat shock response genes are shown in Figure 1. HS up-regulated the gene expression of HSPA5, HSP90AB1, HspA1A, and HSPB8 (p < 0.05). However, under HS, the gene expression of HSPA5, HSP90AB1, HSPA1A and HSPB8 was down-regulated in the LM25 and LM20 group (p < 0.05), the gene expression of HSP90AB1 and HSPA1A was down-regulated in the LM35 group (p < 0.05), the gene expression of HSPA1A and HSP8 was down-regulated in the LM35 group (p < 0.05), compared to the IPAA group.
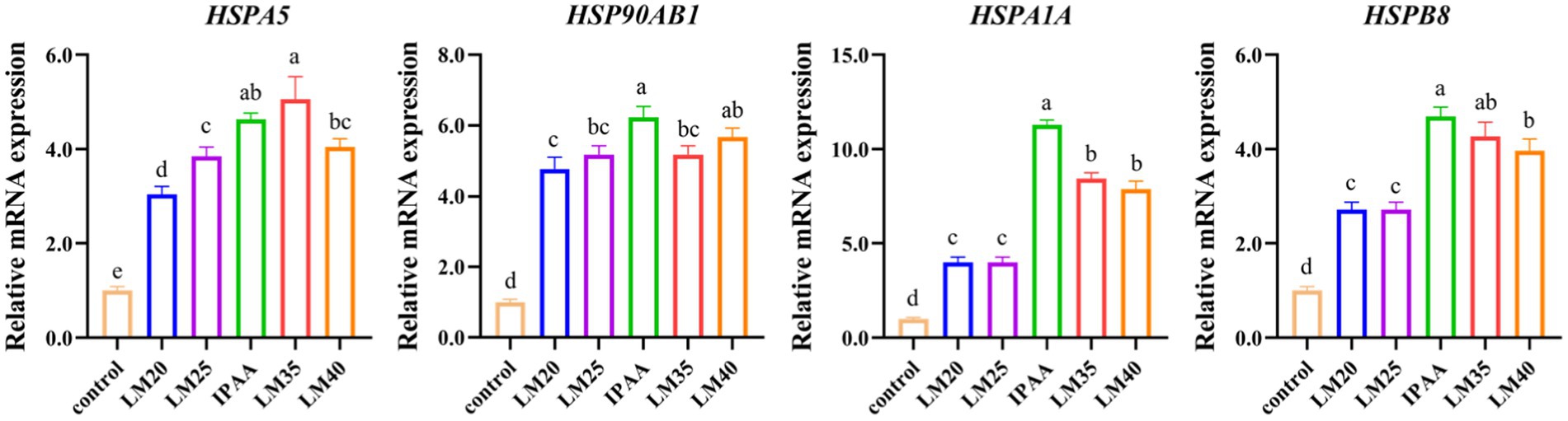
Figure 1. The relative mRNA expression of heat shock response genes in MAC-T cells with the different treatments. Control (37°C) and IPAA (42°C) treatments containing Lys: Met at 3.0, LM40, LM35, LM25 and LM20 containing Lys: Met at 2.0: 1, 2.5: 1, 3.5: 1 and 4.0: 1, respectively. Asterisks indicated significant differences between different groups: * p < 0.05.
3.2 Abundant of apoptosis-related genes
Compared with the control group, the gene expression of BCL2 was down-regulated in the IPAA group (p < 0.05, Figure 2). Under HS, compared with the IPAA group, the gene expression of BCL2 was up-regulated in the LM20, LM25 and LM40 groups (p < 0.05), the gene expression of BCL2L1 was up-regulated in the LM25 group (p < 0.05).
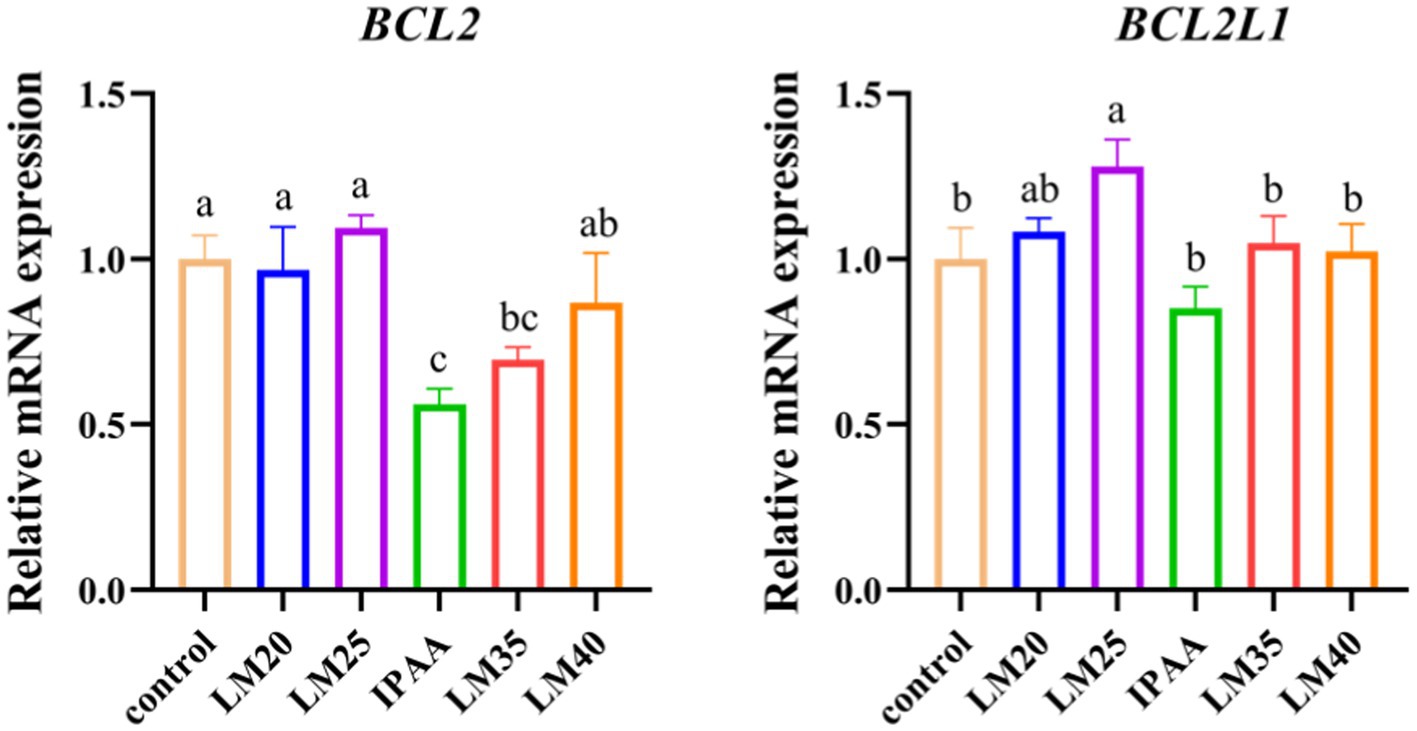
Figure 2. The relative mRNA expression of apoptosis-related genes in MAC-T cells with the different treatments. Control (37°C) and IPAA (42°C) treatments containing Lys: Met at 3.0: 1, LM40, LM35, LM25 and LM20 containing Lys: Met at 2.0: 1, 2.5: 1, 3.5: 1 and 4.0: 1, respectively. Asterisks indicated significant differences between different groups: * p < 0.05.
3.3 mRNA expression of casein genes
The expression of CSN1S2 and CSN2 in the LM25 group was up-regulated compared with the control group (p < 0.05, Figure 3).
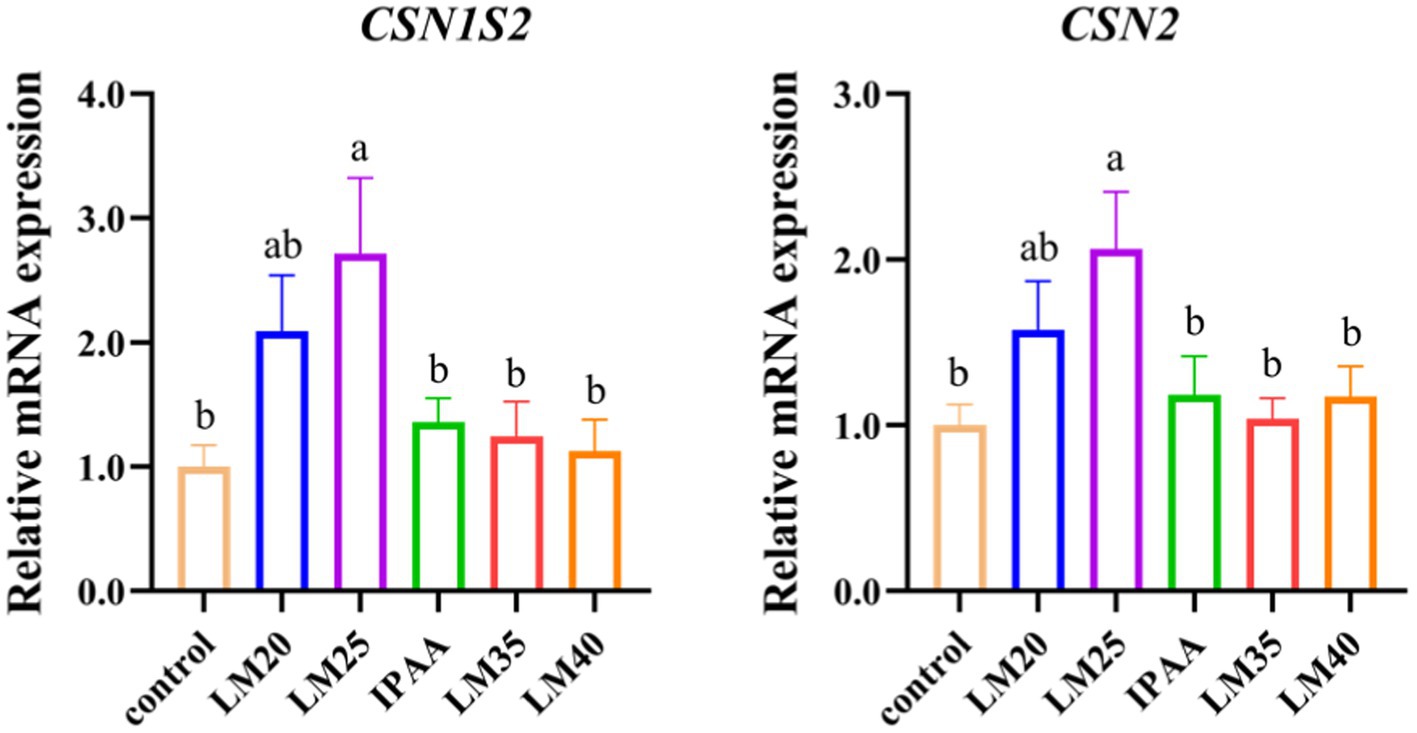
Figure 3. The relative mRNA expression of casein genes in MAC-T cells with the different treatments. Control (37°C) and IPAA (42°C) treatments containing Lys: Met at 3.0: 1, LM40, LM35, LM25 and LM20 containing Lys: Met at 2.0: 1, 2.5: 1, 3.5: 1 and 4.0: 1, respectively. Asterisks indicated significant differences between different groups: * p < 0.05.
3.4 RNA sequencing results
A total of 3.8–5.1 million raw sequencing reads were generated in each group. The high-quality (HQ) clean reads obtained accounted for more than 99% of all the raw reads (Figure 4A) and were mapped to the bovine reference genome (Bos Taurus, assembly ARS-UCD1.2). The mean mapping ratio was greater than 96% in each group. A total of 13,773, 13,390, 13,552, 13,623, 13,528 and 13,456 known genes and 591, 591, 585, 592, 593 and 592 new genes were identified in the control, IPAA, LM20, LM25, LM35 and LM40 groups, respectively (Figure 4B). The cumulative variance contribution rate (65.2%, PC1 + PC2) of the principal component analysis (PCA) for the gene expression profiles was lower than the standard of 85% (Figure 4C). The principal component analysis illustrated that a significant difference existed between the control and other groups under HS. At the same time, except for the IPAA group, the three repeats in each HS groups tended to cluster closely, indicating that adding different concentrations of Met resulted in a high similarity in the overall expression levels of core genes during HS (Figure 4C). The sample clustering analyses further confirmed the PCA results (Figure 4D).
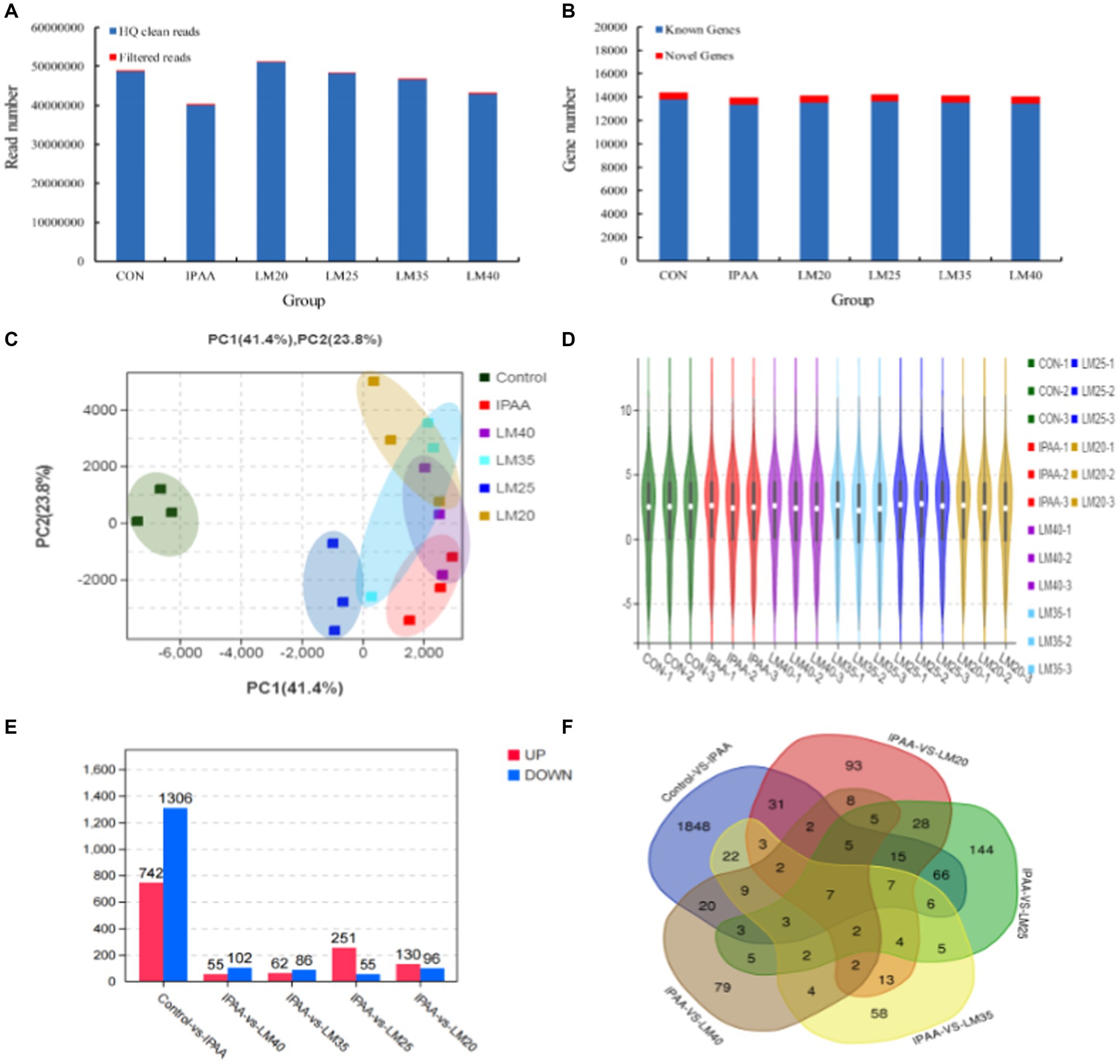
Figure 4. Regulation of gene transcription expression in MAC-T cells with the different treatments. Control (37°C) and IPAA (42°C) treatments containing Lys: Met at 3.0: 1, LM40, LM35, LM25 and LM20 containing Lys: Met at 2.0: 1, 2.5: 1, 3.5: 1 and 4.0: 1, respectively. Read number obtained by RNA sequencing (A); genes number identified by RNA sequencing (B); PCA analysis (C); sample cluster analysis (D); number of differentially expressed genes (E); Venn diagram (F).
To investigate the regulatory mechanisms for the effects of Met on lactation performance, gene function analysis was performed. Genes with a p-value <0.05 and an absolute value of log2 fold-change (|log2 FC|) > 2 were considered as DEGs. A total of 2048 DEGs were screened resulting in 742 upregulated and 1,306 downregulated in the IPAA group relative to the control group. A total of 306 DEGs with 251 upregulated and 55 downregulated were in the LM25 group relative to the IPAA group, and 130, 62, 55 upregulated and 96, 86, 102 downregulated were detected in the LM20, LM35 and LM40 group, respectively, relative to the IPAA group (Figure 4E).
Increasing Met supplementation significantly affected the expression of 28 DEGs under HS (Figure 4F; Supplementary Table S1). These included CCN1 (cellular communication network factor 1, CCN1) and ZNF182 (zinc finger protein 182, ZNF182), which play a role in cell proliferation, differentiation, and apoptosis. In addition, FGF2 1 (fibroblast growth factor 21, FGF21) and AFMID (arylformamidase, AFMID) function as metabolic regulators. In contrast, decreasing Met supplementation significantly affected the expression of 4 DEGs under HS (Figure 4F; Supplementary Table S1) including SELPLG (selectin P ligand, SELPLG), NR1H4 (nuclear receptor subfamily 1, group H, member 4, NR1H4).
The top 10 DEGs encoding heat shock proteins (HSPA5, HSPA1A, HSPA6, HSPH1, HSPA8, DNAJA4, HYOU1 and HSP90AA1) were highly up-regulated during HS in the IPAA group relative to the control group (Figure 5A). BAG3 (BAG cochaperone 3, BAG3), associated with apoptosis, was also upregulated. For more in-depth biological function information, KEGG pathway annotation analysis was performed using the DEGs list with the assistance of KEGG database. The enriched pathways with p value <0.05 are reported in Figure 5B. Eight pathways in the Control-vs-IPAA condition had a close relationship to the autoimmune disorders including cushing syndrome, breast cancer, endocrine resistance, AGE-RAGE signaling pathway in diabetic complications, endometrial cancer, basal cell carcinoma, prostate cancer, and MicroRNAs in cancer. Some of the pathways are associated with cellular responses by HS including sphingolipid signaling pathway, protein processing in the endoplasmic reticulum, Wnt signaling pathway, IL-17 signaling pathway, GnRH signaling pathway, ubiquitin mediated and proteolysis, and peroxisome. Two pathways are related to metabolism including Selenocompound metabolism and nicotinate and nicotinamide metabolism. Nearly all these top-affected pathways are related to immune and heat shock response. The responsiveness of bovine MAC-T cells to HS in this study, clearly suggested its suitability as a model to understand the modulation of cow mammary gland expression signatures in response to HS.
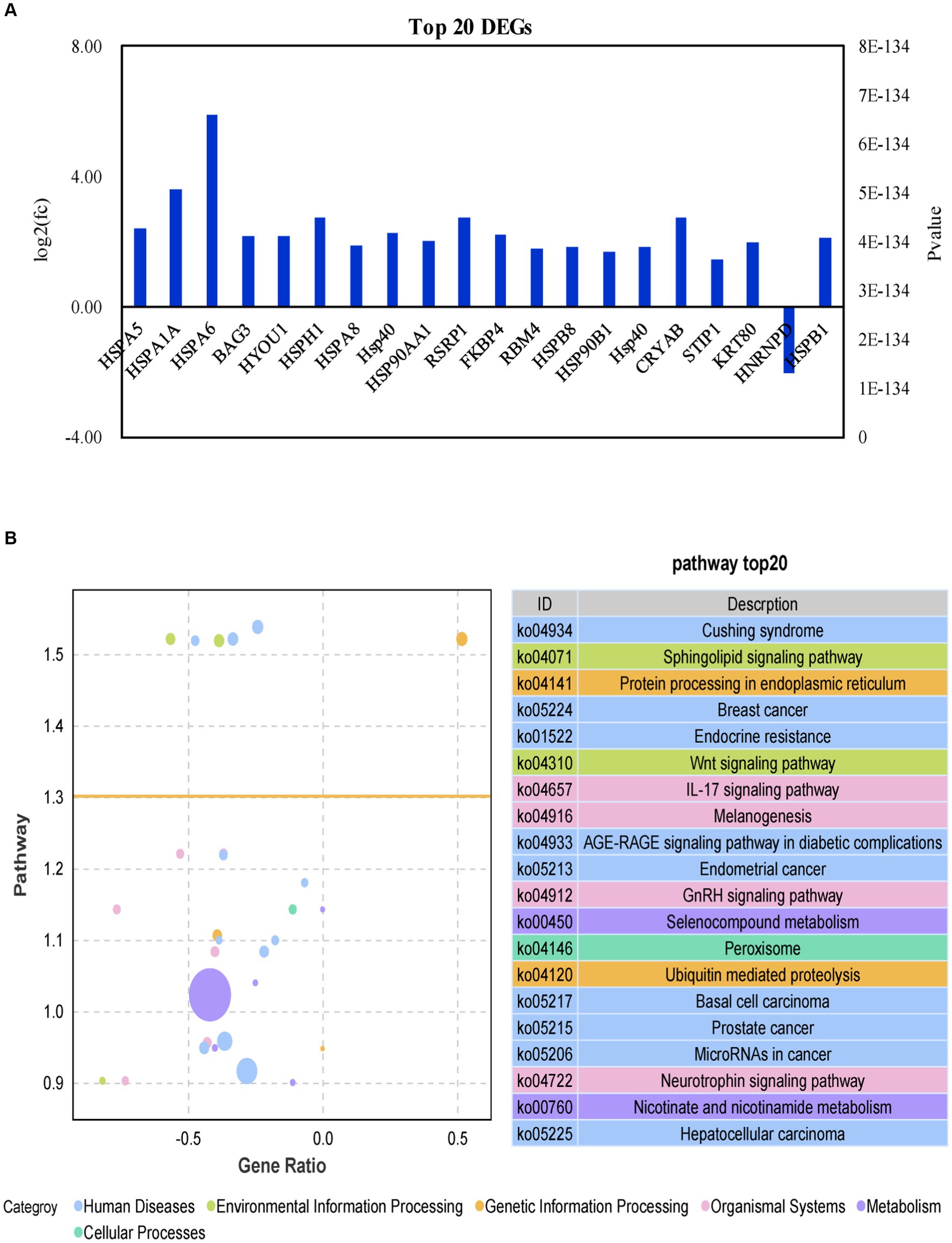
Figure 5. Analysis of differential genes function in MAC-T cells with the the different treatments. Control (37°C) and IPAA (42°C) treatments containing Lys: Met at 3.0: 1, LM40, LM35, LM25 and LM20 containing Lys: Met at 2.0: 1, 2.5: 1, 3.5: 1 and 4.0: 1, respectively. Top 20 differential genes (A); KEGG analysis (B).
The KEGG pathway annotation analysis revealed that DEGs were mainly involved in amino acid metabolism, immune system, infectious diseases, and signal transduction (Figure 6A). In addition, most DEGs (DNAJB1, EEF1A2, EGR1, EGR2, FGF21, HSPA1A, MAPK12, and WNT3A) among the four groups were all significantly enriched in immunology-related pathways involved in the heat stock response (Figure 6B; Supplementary Table S2). In addition, the DEGs of the LM25 group were also significantly enriched in glyoxylate and dicarboxylate metabolism and mTOR signaling pathway (Figure 6B). The DEGs of the LM35 group were significantly enriched in nicotinate and nicotinamide metabolism, glycine, serine and threonine metabolism (Figure 6B). The DEGs of the LM40 group were markedly enriched in the MAPK signaling pathway and nicotinate and nicotinamide metabolism (Figure 6B).
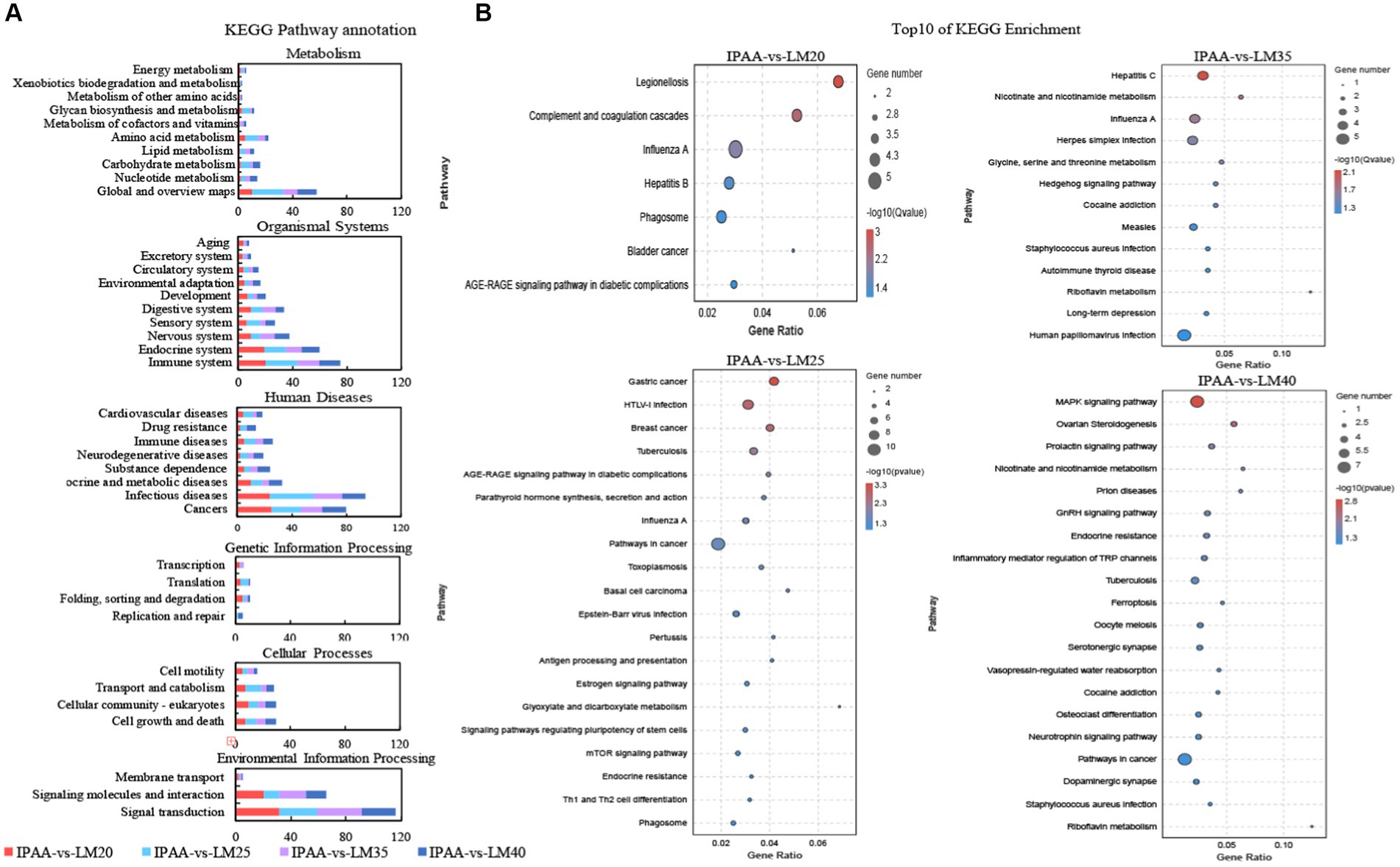
Figure 6. KEGG analysis of differential genes in MAC-T cells with the different treatments. Control (37°C) and IPAA (42°C) treatments containing Lys: Met at 3.0: 1, LM40, LM35, LM25 and LM20 containing Lys: Met at 2.0: 1, 2.5: 1, 3.5: 1 and 4.0: 1, respectively. KEGG Pathway annotation (A); Top10 of KEGG Enrichment (B).
The effects of Met supplementation on mRNA abundance of milk fat, lactose, and mTOR signaling pathway genes is reported in Table 3. Compared with the IPAA group, the mRNA abundance of LPL, ACACA, SCD, FADS1, GPAM, PGM2, LPIN1, SPTLC1, SPTLC2, INSIG1, INSIG2 and PPARG was up-regulated in the LM25 group, as well as the lactose synthesis genes of UGP2, B4GALT1 and GALE. In addition, an upregulation in the expression of EIF4E, EEF2K, and RHEB, and a downregulation in expression of EIF4EBP1 and eEF2 were also detected in the LM25 group. It was observed that the Met concentration at 70 mM in the LM25 group resulted in a higher expression of genes coding for milk fat, lactose and mTOR signaling-responsive genes.
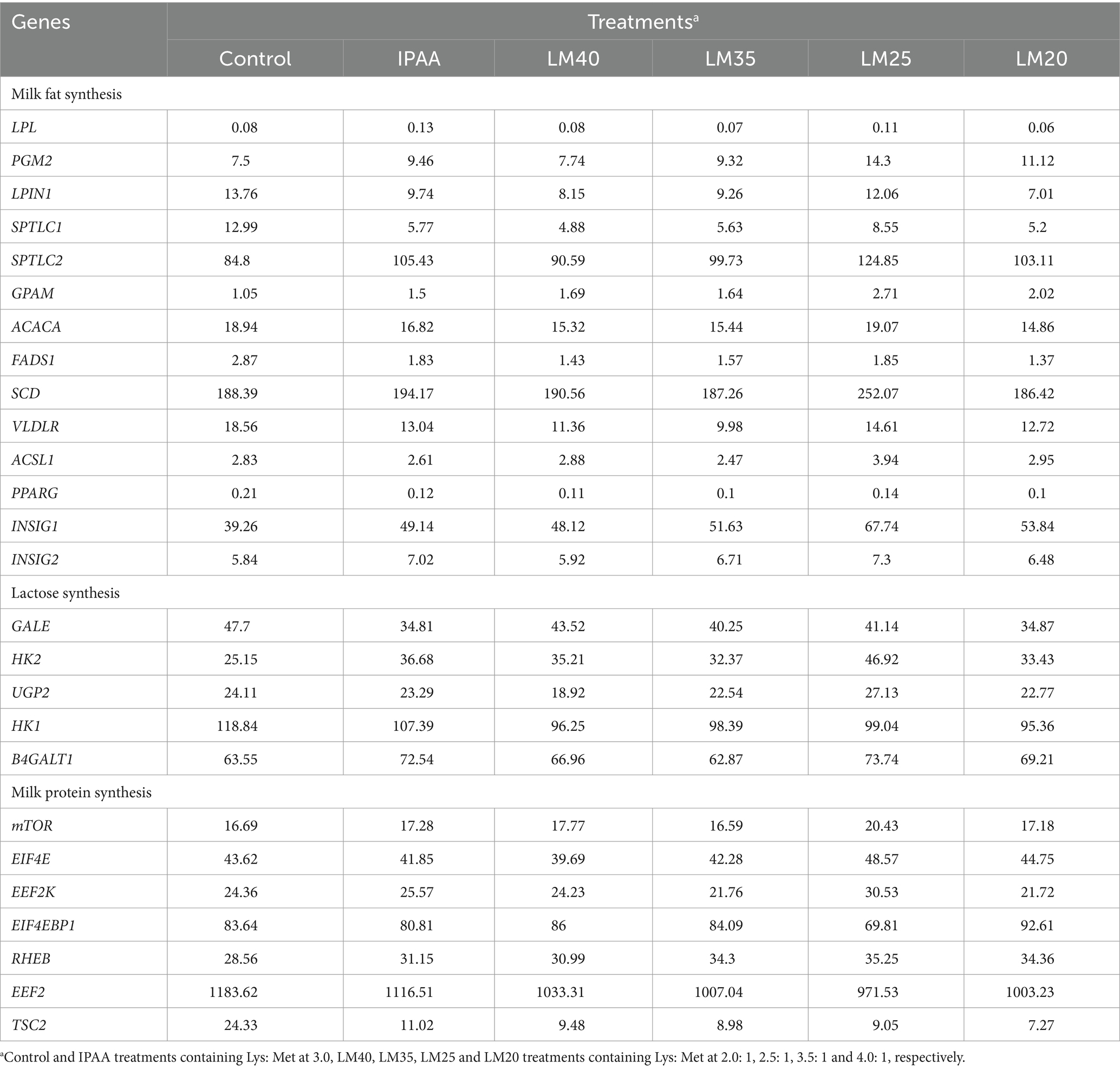
Table 3. The mRNA abundance (log2FC) of milk fat, lactose, and mTOR singal pathway genes in MAC-T cells with the different treatments.
As presented in Figure 7, the RT-qPCR results were consistent with the RNA-Seq data. The relative mRNA expression of eIF4E in the LM25 group was significantly higher than that in the IPAA, LM35 and LM40 groups (p < 0.05). Compared with the control group, the relative expression of TSC2 in all other groups was significantly down-regulated (p < 0.05).
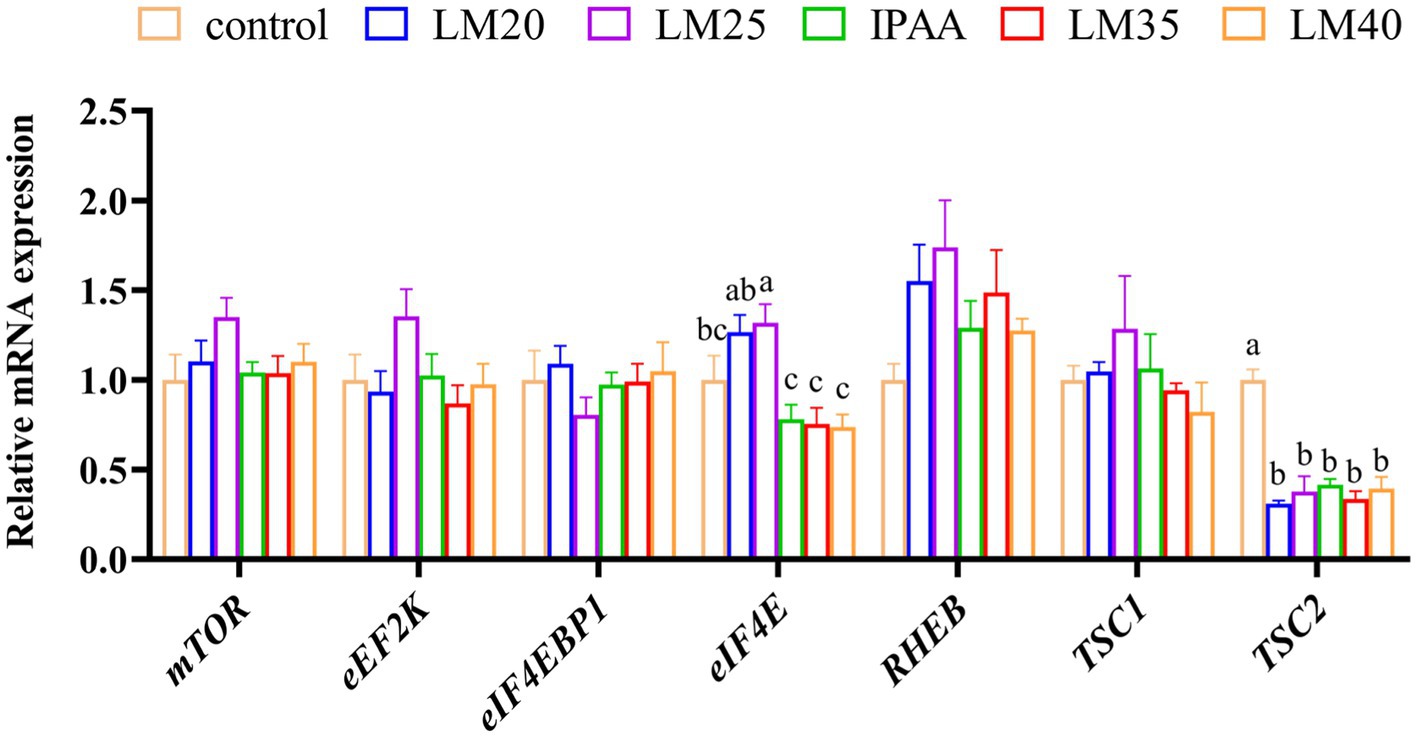
Figure 7. The relative mRNA expression of mTOR signaling pathway related genes in MAC-T cells with the different treatments. Control (37°C) and IPAA (42°C) treatments containing Lys: Met at 3.0: 1, LM40, LM35, LM25 and LM20 containing Lys: Met at 2.0: 1, 2.5: 1, 3.5: 1 and 4.0: 1, respectively. Asterisks indicated significant differences between different groups: * p < 0.05.
4 Discussion
The heat shock protein (HSPs) family members constitute a group of chaperone proteins that exhibit rapid up-regulation in response to HS, thereby ensuring cellular homeostasis through the regulation of protein folding and maturation within cells (36). In accordance with previous findings (37–39), the expression of genes encoding Hsps (HSPA5, HSPA1A, HSPA6, HSPH1, HSPA8, DNAJA4, HYOU1 and HSP90AA1) was significantly up-regulated in MAC-T cells upon exposure to HS in this study. It has been demonstrated that the 70 kDa heat shock protein (HSP70) is a reliable biomarker for monitoring changes in body temperature in mammals. These proteins contribute significantly to the heat tolerance of cells by up-regulating protein expression to help restore homeostasis in heat-exposed cells (40–43). The supplementation of Met (70 mg/L) decreased the protein level of HSP70 compared with the control group (60 mg/L Met) (44). Improving the supply of Met has also been reported to prevent heat-induced oxidative stress and significantly reduce mortality of BMECs in vitro (23). In this study, the relative mRNA abundance of the genes encoding HSP70 (HSPA5, HSPA1A, HSPA6 and HSPA8) was down-regulated with the increase of Met addition under HS, indicating that enhancing Met supply has a potential role in increasing the tolerance of MAC-T cells to heat.
Apoptosis is the ultimate outcome of mammalian cells undergoing sustained HS. In this process, cell death occurs due to the programmed control of genes and the stepwise activation of the apoptotic pathway. B-cell lymphoma 2 (BCL-2)-associated athanogene 3 (BAG3) protein is a co-chaperone of HSP70, acts by binding to the ATPase domain to help the chaperone release ADP and nucleotide cycle (45), and responds to HS with elevated expression. It also has the binding site for BCL-2, an intrinsic (mitochondria-dependent) pathway leading to apoptosis, as well as activation of macrophage phagocytosis through co-infection with HSPs (46). The level of the anti-apoptotic BCL-2 family protein Bcl-xL decreased with the knockdown of BAG3 (47). In this study, compared to the control group, the gene expression of BAG3 was higher in the IPAA group, while the mRNA level of the anti-apoptotic gene (BCL2) was lower in the IPAA group. Moreover, the mRNA level of the anti-apoptotic genes (BCL2 and BCL2L1) was higher in the LM25 group than that in the IPAA group. This result is consistent with previous reports (17), which may be related to the fact that Met effectively triggers the anti-apoptotic response in cells during HS (48). Thus, enhancing Met supply up-regulated the expression of anti-apoptotic genes in MAC-T cells, which may help alleviate heat-induced apoptosis.
There could be a direct link between the decline in milk production and the down-regulation of gene expression associated with milk protein synthesis caused by hyperthermia (49). The ratio of Lys to Met has been demonstrated to alter the expression of casein genes in BMECs (20, 50, 51). In the current study, the mRNA level of casein genes (CSN1S1 and CSN2) was the highest in the LM25 group. There was a dose-dependent relationship between the synthesis of milk fat and the supply of Met, and the secretion of triglycerides and lipid droplets was greatest in BMECs at a dose of 0.6 mM (52). Similarly, in this study, the transcriptional abundance of genes related to de novo synthesis of fatty acids (ACACA, SCD and FADS1), triacylglycerol synthesis (GPAM, PGM2 and LPIN1), sphingolipid synthesis (SPTLC1 and SPTLC2), and transcription regulation (INSIG1, INSIG2 and PPARG) were up-regulated in the LM25 group compared to the IPAA group. Following mammary cell uptake, glucose is converted to uridine diphosphate (UDP-) glucose and UDP-galactose in the cytoplasm under the action of UDP glucose pyrophosphorylase (UGP2) and galactose epimerase (GALE). Finally, one molecule of UDP-galactose and one molecule of glucose are combined by β-1,4-galactosyltransferase 1 (B4GALT1) in the Golgi apparatus to produce lactose (53). The transcript abundance of UGP2, B4GALT1 and GALE was upregulated in the LM25 group compared to the IPAA group. The differences in the expression levels of these genes (related to casein, milk fat and lactose synthesis) indicated that the ratio of Lys to Met at 2.5: 1 may be more conducive to the synthesis of milk components in mammary cells under HS conditions.
There is growing evidence that the mammalian target of rapamycin (mTOR) signaling pathway is the central node of the amino acid regulatory pathway that controls the synthesis of milk protein, milk fat and lactose (54–57). Previous studies have demonstrated that increased availability of Met and arginine (54), tryptophan (58) and Lys (59) could affect milk protein synthesis by changing the mTOR signaling pathway. Additionally, our earlier study has also indicated that modifications in the intracellular metabolism of glutamate, arginine and proline, alanine, aspartate, and tryptophan can provide sufficient substrates and energy for milk protein synthesis during HS (24). Thus, the upregulation of genes involved in amino acid metabolism (AFMID, HYKK, NOS3, and RIMKLB) in the LM25 group confirmed the biological correlation between Met supply and the mTOR signaling pathway compared to the IPAA group during HS. The mTOR signaling pathway also regulates the metabolism of lipids and carbohydrates by up-regulating the expression of related genes to control enzyme synthesis (60). Compared with the control group, the transcriptional abundance of AFMID and MGAT5B (involved in glyoxylate and dicarboxylate metabolism as well as mannose type O-glycan biosynthesis) was up-regulated in the LM25 group, indicating that the increased supply of Met may also regulate carbohydrate and lipid metabolism in MAC-T cells through the mTOR signaling pathway.
Taken together, the data from this study indicated that an increased supply of Met (Lys: Met at 2.5: 1) had the ability to attenuate cellular damage (e.g., apoptosis) during HS. In addition, increasing the supply of Met may help increase the synthesis of casein, milk fat, and lactose in mammary cells, in part by altering the expression of genes involved in intracellular metabolism of amino acids, lipids, and carbohydrates, as well as the mTOR signaling pathway. The limitation of this study is that only the MAC-T cell model was used to investigate the increase in Met supply to mitigate the negative effects of HS on milk secretion ability, rather than in vivo. Thus, more studies should be conducted on dairy cows to validate the results of this study.
5 Conclusion
Heat stress causes changes at the level of gene transcription in MAC-T cells. These changes are partially reversed by the addition of Met supply (ratio of Lys to Met of 2.5:1). The potential mechanism is related to the mRNA expression regulation of HSPs, anti-apoptosis and milk component synthesis genes explored by whole transcription sequencing technology. The findings of this study raise the possibility supplementation with Met might have a positive effect on mammary cells during HS.
Data availability statement
The original contributions presented in the study are publicly available. The data presented in the study are deposited in the NCBI repository, accession number PRJNA1119483. https://www.ncbi.nlm.nih.gov/sra/?term=PRJNA1119483.
Ethics statement
Ethical approval was not required for the studies on animals in accordance with the local legislation and institutional requirements because only commercially available established cell lines were used.
Author contributions
LF: Formal analysis, Methodology, Software, Writing – original draft, Writing – review & editing. YY: Software, Validation, Writing – review & editing. YuZ: Methodology, Resources, Writing – review & editing. QR: Project administration, Supervision, Writing – review & editing. YaZ: Project administration, Supervision, Writing – review & editing. RL: Methodology, Resources, Writing – review & editing. HY: Investigation, Methodology, Software, Writing – review & editing. JC: Data curation, Software, Writing – review & editing. JL: Investigation, Validation, Writing – review & editing. GW: Funding acquisition, Writing – review & editing. LZ: Data curation, Formal analysis, Funding acquisition, Investigation, Methodology, Writing – review & editing. XD: Data curation, Investigation, Methodology, Software, Writing – review & editing.
Funding
The author(s) declare that financial support was received for the research, authorship, and/or publication of this article. This work was supported by the Chongqing performance incentive guide special project (22526 J), Chongqing Special Postdoctoral Science Foundation (XmT2018001) and Chongqing Financial Fund Projects (24516C, 24519C).
Conflict of interest
The authors declare that the research was conducted in the absence of any commercial or financial relationships that could be construed as a potential conflict of interest.
Publisher’s note
All claims expressed in this article are solely those of the authors and do not necessarily represent those of their affiliated organizations, or those of the publisher, the editors and the reviewers. Any product that may be evaluated in this article, or claim that may be made by its manufacturer, is not guaranteed or endorsed by the publisher.
Supplementary material
The Supplementary material for this article can be found online at: https://www.frontiersin.org/articles/10.3389/fvets.2024.1393372/full#supplementary-material
References
1. Tao, S, Orellana, RM, Weng, X, Marins, TN, Dahl, GE, and Bernard, JK. Symposium review: the influences of heat stress on bovine mammary gland function. J Dairy Sci. (2018) 101:5642–54. doi: 10.3168/jds.2017-13727
2. Cartwright, SL, Schmied, J, Karrow, N, and Mallard, BA. Impact of heat stress on dairy cattle and selection strategies for thermotolerance: a review. Front Vet Sci. (2023) 10:1198697. doi: 10.3389/fvets.2023.1198697
3. Heinrichs, AJ, Heinrichs, BS, Cavallini, D, Fustini, M, and Formigoni, A. Limiting total mixed ration availability alters eating and rumination patterns of lactating dairy cows. JDS Commun. (2021) 2:186–90. doi: 10.3168/jdsc.2020-0074
4. Gorniak, T, Meyer, U, Südekum, K-H, and Dänicke, S. Impact of mild heat stress on dry matter intake, milk yield and milk composition in mid-lactation Holstein dairy cows in a temperate climate. Arch Anim Nutr. (2014) 68:358–69. doi: 10.1080/1745039X.2014.950451
5. Bernabucci, U, Basiricò, L, Morera, P, Dipasquale, D, Vitali, A, Piccioli Cappelli, F, et al. Effect of summer season on milk protein fractions in Holstein cows. J Dairy Sci. (2015) 98:1815–27. doi: 10.3168/jds.2014-8788
6. Gao, ST, Guo, J, Quan, SY, Nan, XM, Fernandez, MVS, Baumgard, LH, et al. The effects of heat stress on protein metabolism in lactating Holstein cows. J Dairy Sci. (2017) 100:5040–9. doi: 10.3168/jds.2016-11913
7. West, JW. Effects of heat-stress on production in dairy cattle. J Dairy Sci. (2003) 86:2131–44. doi: 10.3168/jds.S0022-0302(03)73803-X
8. Cowley, FC, Barber, DG, Houlihan, AV, and Poppi, DP. Immediate and residual effects of heat stress and restricted intake on milk protein and casein composition and energy metabolism. J Dairy Sci. (2015) 98:2356–68. doi: 10.3168/jds.2014-8442
9. Rhoads, ML, Rhoads, RP, Van Baale, MJ, Collier, RJ, Sanders, SR, Weber, WJ, et al. Effects of heat stress and plane of nutrition on lactating Holstein cows: I. Production, metabolism, and aspects of circulating somatotropin. J Dairy Sci. (2009) 92:1986–97. doi: 10.3168/jds.2008-1641
10. Ma, L, Yang, Y, Zhao, X, Wang, F, Gao, S, and Bu, D. Heat stress induces proteomic changes in the liver and mammary tissue of dairy cows independent of feed intake: an iTRAQ study. PLoS One. (2019) 14:e0209182. doi: 10.1371/journal.pone.0209182
11. Shyy, TT, Asch, BB, and Asch, HL. Concurrent collapse of keratin filaments, aggregation of organelles, and inhibition of protein synthesis during the heat shock response in mammary epithelial cells. J Cell Biol. (1989) 108:997–1008. doi: 10.1083/jcb.108.3.997
12. Collier, RJ, Collier, JL, Rhoads, RP, and Baumgard, LH. Invited review: genes involved in the bovine heat stress response. J Dairy Sci. (2008) 91:445–54. doi: 10.3168/jds.2007-0540
13. Hu, H, Wang, JQ, Gao, HN, Li, SL, Zhang, YD, and Zhen, N. Heat-induced apoptosis and gene expression in bovine mammary epithelial cells. Anim Prod Sci. (2016) 56:918–26. doi: 10.1071/AN14420
14. Li, C, Wang, Y, Li, L, Han, Z, Mao, S, and Wang, G. Betaine protects against heat exposure–induced oxidative stress and apoptosis in bovine mammary epithelial cells via regulation of ROS production. Cell Stress Chaperones. (2019) 24:453–60. doi: 10.1007/s12192-019-00982-4
15. Bai, H, Li, T, Yu, Y, Zhou, N, Kou, H, Guo, Y, et al. Cytoprotective effects of taurine on heat-induced bovine mammary epithelial cells in vitro. Cells. (2021) 10:258. doi: 10.3390/cells10020258
16. Yang, M, Kuang, M, Wang, G, Ali, I, Tang, Y, Yang, C, et al. Choline attenuates heat stress-induced oxidative injury and apoptosis in bovine mammary epithelial cells by modulating PERK/Nrf-2 signaling pathway. Mol Immunol. (2021) 135:388–97. doi: 10.1016/j.molimm.2021.05.002
17. Salama, AAK, Duque, M, Wang, L, Shahzad, K, Olivera, M, and Loor, JJ. Enhanced supply of methionine or arginine alters mechanistic target of rapamycin signaling proteins, messenger RNA, and micro RNA abundance in heat-stressed bovine mammary epithelial cells in vitro. J Dairy Sci. (2019) 102:2469–80. doi: 10.3168/jds.2018-15219
18. Osorio, JS, Ji, P, Drackley, JK, Luchini, D, and Loor, JJ. Supplemental Smartamine M or MetaSmart during the transition period benefits postpartal cow performance and blood neutrophil function. J Dairy Sci. (2013) 96:6248–63. doi: 10.3168/jds.2012-5790
19. Wang, C, Liu, HY, Wang, YM, Yang, ZQ, Liu, JX, Wu, YM, et al. Effects of dietary supplementation of methionine and lysine on milk production and nitrogen utilization in dairy cows. J Dairy Sci. (2010) 93:3661–70. doi: 10.3168/jds.2009-2750
20. Dong, X, Zhou, Z, Wang, L, Saremi, B, Helmbrecht, A, Wang, Z, et al. Increasing the availability of threonine, isoleucine, valine, and leucine relative to lysine while maintaining an ideal ratio of lysine: methionine alters mammary cellular metabolites, mammalian target of rapamycin signaling, and gene transcription. J Dairy Sci. (2018) 101:5502–14. doi: 10.3168/jds.2017-13707
21. Yue, S, Qian, J, Du, J, Liu, X, Xu, H, Liu, H, et al. Heat stress negatively influence mammary blood flow, mammary uptake of amino acids and milk amino acids profile of lactating Holstein dairy cows. Pak Vet J. (2023) 43:73–8. doi: 10.29261/pakvetj/2023.002
22. Mu, T, Kong, G, Han, Z, and Li, H. Cytoprotection of methionine on hyperthermia-induced damage in bovine mammary epithelial cells. Cell Biol Int. (2014) 38:971–6. doi: 10.1002/cbin.10271
23. Han, Z-Y, Mu, T, and Yang, Z. Methionine protects against hyperthermia-induced cell injury in cultured bovine mammary epithelial cells. Cell Stress Chaperones. (2015) 20:109–20. doi: 10.1007/s12192-014-0530-7
24. Fu, L, Zhang, L, Liu, L, Yang, H, Zhou, P, Song, F, et al. Effect of heat stress on bovine mammary cellular metabolites and gene transcription related to amino acid metabolism, amino acid transportation and mammalian target of rapamycin (mTOR) signaling. Animals. (2021) 11:3153. doi: 10.3390/ani11113153
25. NRC. Nutrient requirements of dairy cattle. 7th ed. Washington, DC: National Academies Press (2001).
26. Haque, MN, Rulquin, H, Andrade, A, Faverdin, P, Peyraud, JL, and Lemosquet, S. Milk protein synthesis in response to the provision of an “ideal” amino acid profile at 2 levels of metabolizable protein supply in dairy cows. J Dairy Sci. (2012) 95:5876–87. doi: 10.3168/jds.2011-5230
27. Collier, RJ, Stiening, CM, Pollard, BC, VanBaale, MJ, Baumgard, LH, Gentry, PC, et al. Use of gene expression microarrays for evaluating environmental stress tolerance at the cellular level in cattle1. J Anim Sci. (2006) 84:E1–E13. doi: 10.2527/2006.8413_supplE1x
28. Li, J, Wang, X, Sun, A, Li, H, Luo, Y, He, F, et al. Comparative transcriptomic analysis of spermatozoa from Xiangxi and Simmental bulls under heat stress: implications for fertility prediction. Pak Vet J. (2023) 43:184–8. doi: 10.29261/pakvetj/2022.083
29. Livak, KJ, and Schmittgen, TD. Analysis of relative gene expression data using real-time quantitative PCR and the 2(-Delta Delta C(T)) method. Methods. (2001) 25:402–8. doi: 10.1006/meth.2001.1262
30. Langmead, B, and Salzberg, SL. Fast gapped-read alignment with bowtie 2. Nat Methods. (2012) 9:357–9. doi: 10.1038/nmeth.1923
31. Kim, D, Langmead, B, and Salzberg, SL. HISAT: a fast spliced aligner with low memory requirements. Nat Methods. (2015) 12:357–60. doi: 10.1038/nmeth.3317
32. Pertea, M, Pertea, GM, Antonescu, CM, Chang, T-C, Mendell, JT, and Salzberg, SL. String tie enables improved reconstruction of a transcriptome from RNA-seq reads. Nat Biotechnol. (2015) 33:290–5. doi: 10.1038/nbt.3122
33. Pertea, M, Kim, D, Pertea, GM, Leek, JT, and Salzberg, SL. Transcript-level expression analysis of RNA-seq experiments with HISAT, StringTie and Ballgown. Nat Protoc. (2016) 11:1650–67. doi: 10.1038/nprot.2016.095
34. Love, MI, Huber, W, and Anders, S. Moderated estimation of fold change and dispersion for RNA-seq data with DESeq2. Genome Biol. (2014) 15:550. doi: 10.1186/s13059-014-0550-8
35. Wang, S, Cao, Z, Wu, Q, Idrees, A, Almutairi, MH, and Dong, H. A comparative analysis and verification of differentially expressed miRNAs could provide new insights for the treatment of endometritis in yaks. Pak Vet J. (2023) 43:486–92. doi: 10.29261/pakvetj/2023.061
36. Feder, ME, and Hofmann, GE. Heat-shock proteins, molecular chaperones, and the stress response: evolutionary and ecological physiology. Annu Rev Physiol. (1999) 61:243–82. doi: 10.1146/annurev.physiol.61.1.243
37. Li, G, Yu, X, Portela Fontoura, AB, Javaid, A, de la Maza-Escolà, VS, Salandy, NS, et al. Transcriptomic regulations of heat stress response in the liver of lactating dairy cows. BMC Genomics. (2023) 24:410. doi: 10.1186/s12864-023-09484-1
38. Srikanth, K, Kwon, A, Lee, E, and Chung, H. Characterization of genes and pathways that respond to heat stress in Holstein calves through transcriptome analysis. Cell Stress Chaperones. (2017) 22:29–42. doi: 10.1007/s12192-016-0739-8
39. Kapila, N, Sharma, A, Kishore, A, Sodhi, M, Tripathi, PK, Mohanty, AK, et al. Impact of heat stress on cellular and transcriptional adaptation of mammary epithelial cells in riverine Buffalo (Bubalus Bubalis). PLoS One. (2016) 11:e0157237. doi: 10.1371/journal.pone.0157237
40. Gaughan, JB, Bonner, SL, Loxton, I, and Mader, TL. Effects of chronic heat stress on plasma concentration of secreted heat shock protein 70 in growing feedlot cattle1. J Anim Sci. (2013) 91:120–9. doi: 10.2527/jas.2012-5294
41. Saibil, H. Chaperone machines for protein folding, unfolding and disaggregation. Nat Rev Mol Cell Biol. (2013) 14:630–42. doi: 10.1038/nrm3658
42. Sottile, ML, and Nadin, SB. Heat shock proteins and DNA repair mechanisms: an updated overview. Cell Stress Chaperones. (2018) 23:303–15. doi: 10.1007/s12192-017-0843-4
43. Hassan, F, Nawaz, A, Rehman, MS, Ali, MA, Dilshad, SMR, and Yang, C. Prospects of HSP70 as a genetic marker for thermo-tolerance and immuno-modulation in animals under climate change scenario. Anim Nutr. (2019) 5:340–50. doi: 10.1016/j.aninu.2019.06.005
44. Zhou, J, Yue, S, Xue, B, Wang, Z, Wang, L, Peng, Q, et al. Enhanced supply of methionine regulates protein synthesis in bovine mammary epithelial cells under hyperthermia condition. J Anim Sci Technol. (2021) 63:1126–41. doi: 10.5187/jast.2021.e93
45. Marzullo, L, Turco, MC, and De Marco, M. The multiple activities of BAG3 protein: mechanisms. Biochim Biophys Acta Gen Subj. (2020) 1864:129628. doi: 10.1016/j.bbagen.2020.129628
46. Chao, DT, and Korsmeyer, SJ. BCL-2 FAMILY: regulators of cell death. Annu Rev Immunol. (1998) 16:395–419. doi: 10.1146/annurev.immunol.16.1.395
47. Jacobs, AT, and Marnett, LJ. HSF1-mediated BAG3 expression attenuates apoptosis in 4-Hydroxynonenal-treated Colon Cancer cells via stabilization of anti-apoptotic Bcl-2 proteins. J Biol Chem. (2009) 284:9176–83. doi: 10.1074/jbc.M808656200
48. Rebez, EB, Sejian, V, Silpa, MV, and Dunshea, FR. Heat stress and histopathological changes of vital organs: a novel approach to assess climate resilience in farm animals. Sustain For. (2023) 15:1242. doi: 10.3390/su15021242
49. Yue, S, Wang, Z, Wang, L, Peng, Q, and Xue, B. Transcriptome functional analysis of mammary gland of cows in heat stress and Thermoneutral condition. Animals. (2020) 10:1015. doi: 10.3390/ani10061015
50. Nan, X, Bu, D, Li, X, Wang, J, Wei, H, Hu, H, et al. Ratio of lysine to methionine alters expression of genes involved in milk protein transcription and translation and mTOR phosphorylation in bovine mammary cells. Physiol Genomics. (2014) 46:268–75. doi: 10.1152/physiolgenomics.00119.2013
51. Wang, F, van Baal, J, Ma, L, Loor, JJ, Wu, ZL, Dijkstra, J, et al. Short communication: relationship between lysine/methionine ratios and glucose levels and their effects on casein synthesis via activation of the mechanistic target of rapamycin signaling pathway in bovine mammary epithelial cells. J Dairy Sci. (2019) 102:8127–33. doi: 10.3168/jds.2018-15916
52. Qi, H, Meng, C, Jin, X, Li, X, Li, P, and Gao, X. Methionine promotes Milk protein and fat synthesis and cell proliferation via the SNAT2-PI3K signaling pathway in bovine mammary epithelial cells. J Agric Food Chem. (2018) 66:11027–33. doi: 10.1021/acs.jafc.8b04241
53. Liu, H, Zhao, K, and Liu, J. Effects of glucose availability on expression of the key genes involved in synthesis of milk fat, lactose and glucose metabolism in bovine mammary epithelial cells. PLoS One. (2013) 8:e66092. doi: 10.1371/journal.pone.0066092
54. Hu, L, Chen, Y, Cortes, IM, Coleman, DN, Dai, H, Liang, Y, et al. Supply of methionine and arginine alters phosphorylation of mechanistic target of rapamycin (mTOR), circadian clock proteins, and α-s1-casein abundance in bovine mammary epithelial cells. Food Funct. (2020) 11:883–94. doi: 10.1039/C9FO02379H
55. Appuhamy, JADRN, Knoebel, NA, Nayananjalie, WAD, Escobar, J, and Hanigan, MD. Isoleucine and leucine independently regulate mTOR signaling and protein synthesis in MAC-T cells and bovine mammary tissue slices. J Nutr. (2012) 142:484–91. doi: 10.3945/jn.111.152595
56. Wang, M, Xu, B, Wang, H, Bu, D, Wang, J, and Loor, J-J. Effects of arginine concentration on the in vitro expression of casein and mTOR pathway related genes in mammary epithelial cells from dairy cattle. PLoS One. (2014) 9:e95985. doi: 10.1371/journal.pone.0095985
57. Che, L, Xu, M, Gao, K, Wang, L, Yang, X, Wen, X, et al. Valine supplementation during late pregnancy in gilts increases colostral protein synthesis through stimulating mTOR signaling pathway in mammary cells. Amino Acids. (2019) 51:1547–59. doi: 10.1007/s00726-019-02790-7
58. Conejos, JRV, Ghassemi Nejad, J, Kim, J-E, Moon, J-O, Lee, J-S, and Lee, H-G. Supplementing with L-tryptophan increases medium protein and alters expression of genes and proteins involved in Milk protein synthesis and energy metabolism in bovine mammary cells. IJMS. (2021) 22:2751. doi: 10.3390/ijms22052751
59. Lin, X, Li, S, Zou, Y, Zhao, F-Q, Liu, J, and Liu, H. Lysine stimulates protein synthesis by promoting the expression of ATB0, + and activating the mTOR pathway in bovine mammary epithelial cells. J Nutr. (2018) 148:1426–33. doi: 10.1093/jn/nxy140
Keywords: heat stress, nutrition requirement, amino acid, milk secretion ability, RNA sequencing
Citation: Fu L, You Y, Zeng Y, Ran Q, Zhou Y, Long R, Yang H, Chen J, Loor JJ, Wang G, Zhang L and Dong X (2024) Varying the ratio of Lys: Met through enhancing methionine supplementation improved milk secretion ability through regulating the mRNA expression in bovine mammary epithelial cells under heat stress. Front. Vet. Sci. 11:1393372. doi: 10.3389/fvets.2024.1393372
Edited by:
Izhar Hyder Qazi, Shaheed Benazir Bhutto University of Veterinary &Animal Sciences, PakistanReviewed by:
Damiano Cavallini, University of Bologna, ItalyIlias Giannenas, Aristotle University of Thessaloniki, Greece
Copyright © 2024 Fu, You, Zeng, Ran, Zhou, Long, Yang, Chen, Loor, Wang, Zhang and Dong. This is an open-access article distributed under the terms of the Creative Commons Attribution License (CC BY). The use, distribution or reproduction in other forums is permitted, provided the original author(s) and the copyright owner(s) are credited and that the original publication in this journal is cited, in accordance with accepted academic practice. No use, distribution or reproduction is permitted which does not comply with these terms.
*Correspondence: Li Zhang, emhhbmdsaTAzMDk0QDE2My5jb20=; Xianwen Dong, ZHh3Y3F4a3lAMTYzLmNvbQ==
†These authors have contributed equally to this work