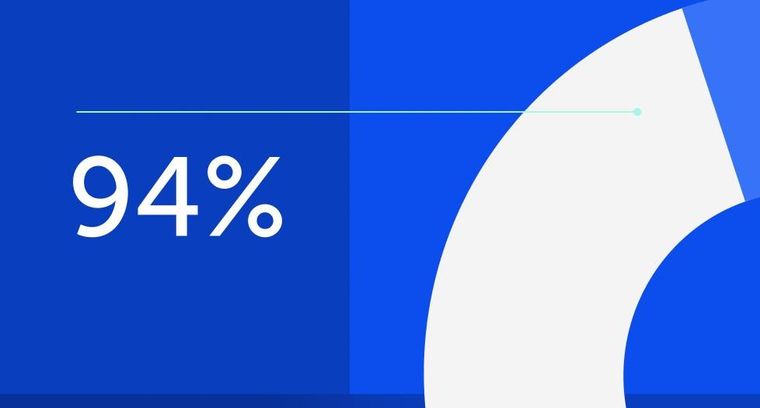
94% of researchers rate our articles as excellent or good
Learn more about the work of our research integrity team to safeguard the quality of each article we publish.
Find out more
ORIGINAL RESEARCH article
Front. Vet. Sci., 16 February 2024
Sec. Animal Nutrition and Metabolism
Volume 11 - 2024 | https://doi.org/10.3389/fvets.2024.1364815
This article is part of the Research TopicAnimal Health and Production: Identifying Challenges and Finding a Way ForwardView all 40 articles
Exogenous supplementation of guanidinoacetic acid can mechanistically regulate the energy distribution in muscle cells. This study aimed to investigate the effects of guanidinoacetic acid supplementation on liver and breast muscle fat deposition, lipid levels, and lipid metabolism-related gene expression in ducks. We randomly divided 480 42 days-old female Jiaji ducks into four groups with six replicates and 20 ducks for each replicate. The control group was fed the basal diet, and the experimental groups were fed the basal diet with 400, 600, and 800 mg/kg (GA400, GA600, and GA800) guanidinoacetic acid, respectively. Compared with the control group, (1) the total cholesterol (p = 0.0262), triglycerides (p = 0.0357), malondialdehyde (p = 0.0452) contents were lower in GA400, GA600 and GA800 in the liver; (2) the total cholesterol (p = 0.0365), triglycerides (p = 0.0459), and malondialdehyde (p = 0.0326) contents in breast muscle were decreased in GA400, GA600 and GA800; (3) the high density lipoprotein (p = 0.0356) and apolipoprotein-A1 (p = 0.0125) contents were increased in GA600 in the liver; (4) the apolipoprotein-A1 contents (p = 0.0489) in breast muscle were higher in GA600 and GA800; (5) the lipoprotein lipase contents (p = 0.0325) in the liver were higher in GA600 and GA800; (6) the malate dehydrogenase contents (p = 0.0269) in breast muscle were lower in GA400, GA600, and GA800; (7) the insulin induced gene 1 (p = 0.0326), fatty acid transport protein 1 (p = 0.0412), and lipoprotein lipase (p = 0.0235) relative expression were higher in GA400, GA600, and GA800 in the liver; (8) the insulin induced gene 1 (p = 0.0269), fatty acid transport protein 1 (p = 0.0234), and lipoprotein lipase (p = 0.0425) relative expression were increased in GA400, GA600, and GA800 in breast muscle. In this study, the optimum dosage of 600 mg/kg guanidinoacetic acid improved the liver and breast muscle fat deposition, lipid levels, and lipid metabolism-related gene expression in ducks.
Lipids are essential for body energy storage and supply and are also important structural components of biofilms (1, 2). The biochemical process of lipid metabolism after absorption is mainly divided into the metabolism of triglycerides, phospholipids, cholesterol, and plasma lipoproteins in animals (2). This process is primarily regulated by hormones, diet nutrition, and biochemical enzyme activity in a complex and precise way, and it is finally transformed into the material components required for various acceptable biochemical reactions (2, 3). Lipid metabolism in animals is a complex biochemical process, which is regulated by various lipases and related genes, such as free fatty acid receptor 4 (FFAR4), fatty acid transport protein 1 (FATP1), insulin induced gene 1 (INSIGI1), lipoprotein lipase (LPL), stearoyl-CoA desaturase (SCD), fatty acid synthase (FAS) and so on (2, 4). Guanidinoacetic acid is a self-existing substance in animals that is a precursor of creatine and plays a vital role in creatine biosynthesis (5, 6). The additional addition of guanidinoacetic acid causes the body to produce a large amount of phosphate group transfer substance, phosphocreatine, which in turn provides energy for the efficient work of tissues such as muscles, brain, and gonads and promotes the continuous distribution of nutrients to the muscles and other tissues, thus changes the animal size (7, 8). Exogenous supplementation of guanidinoacetic acid can mechanistically regulate the energy distribution of muscle cells (9, 10), thus more effectively reducing the activity of related key enzymes through multiple metabolic pathways, inhibiting fatty acid synthesis in the liver, promoting fatty acid β-oxidation, and ultimately reducing the deposition of abdominal fat in broiler chickens by reducing the size or number of abdominal adipocytes (11–13); at the same time, exogenous supplementation of guanidinoacetic acid reduces the consumption of feed-added arginine, so that this part of the amino acid from another pathway, such as through the activation of the Adenosine 5′-monophosphate (AMP)-activated protein kinase signaling pathway, to promote myocyte membrane glucose transporter 4 receptor expression, improve myocyte glucose uptake, which in turn promotes muscle growth and intramuscular fat deposition, and thus improve the quality of meat texture and flavor (14, 15).
Research on guanidinoacetic acid in livestock production has focused on the growth performance and meat quality of pigs, chickens, and ruminants. Sharma et al. found that guanidinoacetic acid improved the abdominal fat yields and breast meat creatine concentration in broilers (16). Majdeddin et al. (17) reported that the guanidinoacetic acid improved the feed conservation and meat quality in broilers. He et al. (18) found that guanidinoacetic acid maximized the growth performance of growing-finishing pigs. Jayaraman et al. (19) reported that guanidinoacetic acid improved average daily gain, feed efficiency, and lean meat yield and reduced back-fat thickness in pigs. Zhang et al. (20) found that the guanidinoacetic acid improved the creatine concentration in Kazakh male lambs. There is minimal research on waterfowl, especially on the fat deposition and distribution in ducks. Therefore, the purpose of this study was to investigate the effects of guanidinoacetic acid on the liver and breast muscle fat deposition, lipid levels, and lipid metabolism-related gene expression in ducks to provide more theoretical bases for their application in the diet of ducks and to contribute to the sustainable and healthy development of waterfowl farming.
All procedures involving care and management were approved by the Institutional Animal Care and Use Committee of the Chinese Academy of Tropical Agricultural Sciences (Approval No.: CATAS-20220819-2).
We randomly divided 480 42 days-old female Jiaji ducks, with 935 ± 5.00 g, into four groups with six replicates and 20 ducks for each replicate. The control group (CG) was fed the lab-formulated basal diet (corn-soybean diets), and the experimental groups were fed the control diet supplemented with 400, 600, and 800 mg/kg guanidinoacetic acid in the form of powder (GA400, GA600, and GA800, respectively). Furthermore, the guanidinoacetic acid, with a concentration ≥99.00%, was bought from Shandong Yatou Biotechnology Co. Ltd., Jinan, China. The composition of the basal diets and nutritional levels are shown in Table 1. The soybean meal (42.00% crude protein, irregular fragment) and peanut meal (47.80% crude protein, irregular fragment) were broken into powder using a grinder (MJW-W Mechanical crusher, Qingdao EPIC Powder Machinery, Qingdao, China), and the mixed with the corn (powder) and other ingredients in a blender (V-200, Wuxi Zhuangcheng Equipment Technology Co., Ltd., Wuxi, China). Finally, put the mixed feed into a double waterproof and moisture-proof feed bag and store it for later use. Feed samples were randomly taken from each treated feed and used to detect the guanidinoacetic acid contents. Before the trial, the duck house was airtightly disinfected using a mixture of formalin and potassium permanganate for 3 days and ventilated. All ducks were reared on a net bed. The light time, humidity, and temperature of the duck house were controlled at 18 h, 70%, and 26°C for 48 days. Use 12-watt LED tubes to adjust the duck house light time requirements. Depending on the growth of ducks, it is decided whether to add extra multiple vitamins and multiple minerals to their drinking water.
One duck with average body weight in each replicate was selected to slaughter after 12 h of fasting to collect the data and samples at the 90 days of age. Determine the abdominal fat percentage of ducks according to NY/T823-2004 standard. Ten grams of the liver and breast muscles in the same part of the ducks were collected and stored at −20°C to detect the biochemistry parameters. About three portions of 5 g liver and breast muscle were collected and stored at −80°C to evaluate the relative expression of genes related to lipid metabolism.
The liver and breast muscles were homogenated with the E6618 TissueMaster™ High-Throughput Tissue Homogenizer, with 1.5/2 mL × 48 Adaptor, Shanghai, China. The cholesterol, triglycerides, phospholipid, malondialdehyde, intramural fat, creatine, high density lipoprotein, low density lipoprotein, apolipoprotein-A1, apolipoprotein-B, lipoprotein lipase, total lipase, and malate dehydrogenase were all determined by the HITACHI Automatic Analyzer 3,500 (HITACHI, Ibaraki-Ken, Japan). The reagent kits used in this trial were purchased from the Nanjing Jiancheng Biotechnology Co., Ltd., Jiangsu, China, and the procedures were carried out strictly following the manufacturer’s instructions. In breast muscle, the fatty acid composition was determined using gas chromatography with TRACE™ 1310E Gas chromatograph (1310E, Shanghai, China). The fatty acids were extracted by a mixture of benzene and petroleum ether (1,1) and rapidly methylated. The content of fatty acids was quantitatively calculated by the normalization method of peak area (21). After extracting the muscle with petroleum ether, the intramuscular fat was obtained by steaming the solvent. The intramuscular fat content was calculated after extraction with petroleum ether using an automatic fat extraction instrument (XD-SXT-210, Shanghai, China) (22).
Following the RNA extraction method, total RNA was isolated from the liver, breast and leg muscles with TRIzol (Sigma, Saint Louis, MO, United States). A 2% agarose gel electrophoresis was performed to assess the quality of the RNA, and total RNA concentration and purity (A260/A280 ratio, 1.80–2.00 is the qualification standard) were ascertained with an ultra-micro spectrophotometer, NanoPhotometer, Implen, German. The primer sequences are shown in Table 2, and primer sequences for duck genes were designed and synthesized by Sangon Biotech, Shanghai, China. All of the process was conducted under RNase-free condition. cDNA was synthesized from RNA using oligo (dT) primers (Invitrogen) in a 40 μL reaction mixture by using a SuperScript III kit (Invitrogen) according to the manufacturer’s instructions. ABI PRISM 7500 SDS thermal cycler (Applied Biosystems, Foster City, CA, United States) was used to determine samples, followed by one cycle at 95°C for 30 s and 40 cycles of 95°C for 5 s and 60°C for 34 s. Based on the 2−∆∆Ct method, relative gene mRNA expressions were normalized by glyceraldehyde-3-phosphate dehydrogenase expression, respectively (23). All of the processes were performed in triplicates under RNase-free conditions. RT-qPCR products were cloned into a pMD18-T vector (TaKaRa) and sequenced by the Sanger method. The sequencing results were compared with the gene sequences in NCBI, and the genes amplified by the primers in Table 2 were verified as the target genes according to the alignment sequence results (23, 24).
Statistical analyses were conducted using SAS 9.4. Data were expressed as mean ± standard error of the mean. Different treatments were statistically compared using one-way ANOVA or Welch ANOVA after the Kolmogorov–Smirnov. The Barteet’s test was used for data that meets the normal distribution, otherwise the Levene’s test was used. Statistical differences among groups were assessed using Duncan’s multiple range test. The Dunnett’ T3 test was used when Welch ANOVA was employed. All experimental data in this study meet the requirement of normal distribution. The test results of all analyses were considered significant at p < 0.05.
In liver, the total cholesterol contents (p = 0.0262), triglycerides contents (p = 0.0357), and malondialdehyde contents (p = 0.0452) were lower in GA400, GA600, and GA800 than in the control group. The phospholipid contents (p = 0.0456) in the liver were increased by 25.00, 41.30, and 23.91%, respectively, in GA400, GA600, and GA800 compared with the control group (Figures 1A–D).
Figure 1. Effects of guanidinoacetic acid on lipid levels in ducks. The data in CG, GA400, GA600 and GA800 were (A) total cholesterol (mmol/L) in the liver 5.49 ± 0.34a, 4.70 ± 0.46b, 3.71 ± 0.24c, 3.68 ± 0.23c, respectively, p = 0.0262; and in breast muscle 4.92 ± 0.20a, 4.51 ± 0.12b, 3.81 ± 0.25c, 3.40 ± 0.12d, respectively, p = 0.0365; (B) triglycerides (mmol/L) in the liver 1.67 ± 0.05a, 1.48 ± 0.04b, 1.39 ± 0.02c, 1.36 ± 0.05c, respectively, p = 0.0357; and in breast muscle 1.93 ± 0.03a, 1.70 ± 0.05b, 1.40 ± 0.04c, 1.39 ± 0.02c, respectively, p = 0.0459; (C) phospholipid (mg/g) in the liver 0.92 ± 0.02c, 1.15 ± 0.03b, 1.30 ± 0.10a, 1.14 ± 0.04b, respectively, P = 0.0456; and in breast 1.09 ± 0.03d, 1.15 ± 0.02c, 1.36 ± 0.11a, 1.20 ± 0.01b, respectively, p = 0.0431; (D) malondialdehyde (mmol/L) in the liver 4.91 ± 0.10a, 4.55 ± 0.18b, 4.13 ± 0.21c, 4.12 ± 0.22c, respectively, p = 0.0452; in breast muscle 4.90 ± 0.32a, 4.30 ± 0.21b, 4.25 ± 0.23b, 3.83 ± 0.09c, respectively, p = 0.0326; (E) intramuscular fat (%) in breast muscle 3.15 ± 0.15b, 3.49 ± 0.06a, 3.49 ± 0.04a, 3.49 ± 0.09a, respectively, p = 0.0235; (F) abdominal fat percentage (%) of ducks 3.77 ± 0.11a, 3.44 ± 0.06b, 3.21 ± 0.04c, 3.23 ± 0.05c, respectively, p = 0.0249; (G) creatine contents in breast muscle 3,920 ± 30.59c, 4,127 ± 26.85b, 4,689 ± 30.24a, 4,691 ± 38.67a, respectively, p = 0.0358.
In breast muscle, the intramuscular fat contents (p = 0.0235) and phospholipid contents (p = 0.0431) were higher in GA400, GA600, and GA800 than in the control group. The total cholesterol (p = 0.0365), triglycerides (p = 0.0459), and malondialdehyde (p = 0.0326) contents in breast muscle were decreased in GA400, GA600, and GA800 compared with the control group (Figures 1A–E).
The abdominal fat percentage (p = 0.0249) of ducks was 8.75, 14.85, and 14.32%, respectively, lower in GA400, GA600, and GA800 than in the control group (Figure 1F).
The creatine contents (p = 0.0358) in breast muscle were 5.28, 19.62, and 19.67%, respectively, higher in GA400, GA600, and GA800 than in the control group (Figure 1G).
In the liver, the high density lipoprotein contents (p = 0.0356) and apolipoprotein-A1 contents (p = 0.0125) were increased by 16.32, 13.92, and 13.92% vs. 33.33, 23.08, and 33.33%, respectively, in GA600 compared with the CG, GA400, and GA800. The low density lipoprotein contents (p = 0.0419) in the liver were lower in GA600 and GA800 than in the control group. The apolipoprotein-B contents (p = 0.0256) were lower in GA400, GA600, and GA800 than in the control group (Table 3).
The high density lipoprotein contents (p = 0.0251) in breast muscle were higher in GA400, GA600, and GA800 than in the control group. The low density lipoprotein contents (p = 0.0357) and apolipoprotein-B contents (p = 0.0457) in breast muscle were decreased in GA400, GA600, and GA800 compared with the control group. The apolipoprotein-A1 contents (p = 0.0489) in breast muscle were 26.67 and 46.67%, respectively, higher in GA600 and GA800 than in the control group (Table 3).
The lipoprotein lipase contents (p = 0.0325) in the liver were 34.05 and 47.03%, respectively, higher in GA600 and GA800 than in the control group. The total lipase contents (p = 0.0256) in the liver were increased in GA400, GA600, and GA800 compared with the control group. The malate dehydrogenase contents (p = 0.0443) in the liver were 30.01, 45.93, and 46.76%, respectively, lower in GA400, GA600, and GA800 than in the control group (Table 4).
In breast muscle, the lipoprotein lipase contents (p = 0.0368) were 2.55, 19.39, and 20.92%, respectively, higher in GA400, GA600, and GA800 than in the control group. The total lipase contents (p = 0.0462) in breast muscle were increased in GA600 and GA800 compared with the control group. The malate dehydrogenase contents (p = 0.0269) in breast muscle were lower in GA400, GA600, and GA800 than in the control group (Table 4).
Dietary guanidinoacetic acid supplementation did not influence the saturated fatty acid (SFA) contents (p = 0.6752), monounsaturated fatty acid (MUFA) contents (p = 0.0910), and polyunsaturated fatty acid (PUFA) contents (p = 0.1257) among groups (Table 5).
In the liver, the relative insulin induced gene 1 expressions (p = 0.0326), fatty acid transport protein 1 expressions (p = 0.0412), and Lipoprotein lipase expressions (p = 0.0235) were 23.00, 31.00, and 30.00% vs. 62.00, 63.00, and 64.00% vs. 23.00, 45.00, and 46.00%, respectively, higher in GA400, GA600, and GA800 than in the control group. The fatty acid synthetase relative expression (p = 0.0235) was decreased by 14.00, 27.00, and 29.00%, respectively, in GA400, GA600, and GA800 compared with the control group (Figure 2).
Figure 2. Effects of guanidinoacetic acid on lipid metabolism-related gene expression in ducks. The data in CG, GA400, GA600, and GA800 were (A) SCD (stearoyl CoA desaturase) in the liver 1.00 ± 0.01, 0.99 ± 0.01; 0.99 ± 0.01, 1.01 ± 0.01, respectively, p = 0.3236; and in breast muscle 1.00 ± 0.01, 1.01 ± 0.01, 1.01 ± 0.01, 0.99 ± 0.01, respectively, p = 0.6328; (B) INSIG1 (insulin induced gene 1) in the liver 1.00 ± 0.01c, 1.23 ± 0.02b, 1.31 ± 0.02a, 1.3 ± 0.03a, respectively, p = 0.0326; and in breast muscle 1 ± 0.01c, 1.34 ± 0.02b, 1.42 ± 0.03a, 1.42 ± 0.02a, respectively, p = 0.0269; (C) FATP1 (fatty acid transport protein 1) in the liver 1 ± 0.01b, 1.62 ± 0.03a, 1.63 ± 0.02a, 1.64 ± 0.03a, respectively, p = 0.0412; and in breast muscle 1.00 ± 0.01b, 1.53 ± 0.02a, 1.52 ± 0.03a, 1.54 ± 0.02a, respectively, p = 0.0234; (D) LPL (lipoprotein lipase) in the liver 1.00 ± 0.01c, 1.23 ± 0.01b, 1.45 ± 0.03a, 1.46 ± 0.03a, respectively, p = 0.0235; and in breast muscle 1 ± 0.01c, 1.29 ± 0.03b, 1.52 ± 0.02a, 1.54 ± 0.04a, respectively, p = 0.0425; (E) FAS (fatty acid synthetase) in the liver 1.00 ± 0.01a, 0.86 ± 0.02b, 0.73 ± 0.02c, 0.71 ± 0.03c, respectively, p = 0.0235; and in breast muscle 1.00 ± 0.01a, 0.76 ± 0.01b, 0.65 ± 0.02c, 0.63 ± 0.03c, respectively, p = 0.0349; (F) FFAR4 (free fatty acid receptor 4) in the liver 1 ± 0.01, 0.99 ± 0.02, 1.01 ± 0.02, 1.01 ± 0.03, respectively, p = 0.5236; and in 1.00 ± 0.01, 0.99 ± 0.01, 1.02 ± 0.03, 1.01 ± 0.02, respectively, p = 0.4571.
In breast muscle, the relative insulin induced gene 1 expressions (p = 0.0269), Fatty acid transport protein 1 expressions (p = 0.0234), and Lipoprotein lipase relative expressions (p = 0.0425) were increased by 34.00, 42.00, and 42.00% vs. 53.00, 52.00, and 54.00% vs. 29.00, 52.00, and 54.00%, respectively, in GA400, GA600, and GA800 compared with the control group. The fatty acid synthetase relative expression (p = 0.0349) was 24.00, 35.00, and 37.00%, respectively, lower in GA400, GA600, and GA800 than in the control group. (Figure 2).
Fat cells of female animals have more mitochondria, which can better metabolize fat and other substances (25). Therefore, female ducks were selected as the research object in this study. Almost all organs and tissues of poultry can synthesize cholesterol, of which 70–80% of cholesterol is synthesized in liver tissue (26–28). Cholesterol is an essential molecule in animals, and its metabolism plays a vital role in animal health (27). Triglyceride is an organic compound produced by esterification of three hydroxyl groups in glycerol and three fatty acid molecules (29). As a non-polar substance, triglyceride is stored in a non-hydrated form and is the energy substance with the largest reserve and production capacity in the animal (30, 31). Phospholipids are lipid compounds containing phospholipid roots consisting of lecithin, inositol phospholipids, and ceruloplasmin (32). Phospholipids play a significant role in activating cells, maintaining primary and primary metabolism and hormone secretion, and enhancing immunity and regeneration (9). In addition, phospholipids promote fat metabolism, lower serum cholesterol, and improve blood circulation (32, 33). Malondialdehyde is the end product of lipid peroxidation, and its production exacerbates cell membrane damage (34, 35). Sharma et al. (16) found that a high dose of guanidinoacetic acid made up for the arginine deficiency in the diet, and increased the contents of intermuscular fat, abdominal fat, and creatine in the breast muscle of broilers. Majdeddin et al. (17) reported that the guanidinoacetic acid improved the muscle creatine concentration in broilers. Majdeddin et al. (9) found that the increased the muscle creatine contents to enhance the energy metabolism in Ross 308 broilers. The cholesterol, malondialdehyde, and triglycerides are products of lipid metabolism (1). In this study, the total cholesterol, malondialdehyde, triglycerides, and abdominal fat percentage were decreased, and the phospholipid, intramuscular fat, and creatine contents were increased in liver and breast muscles in the groups treated with guanidinoacetic acid, especially in GA600, indicating that the guanidinoacetic acid improved the lipid metabolism in ducks, changed the fat deposition and lipid levels in liver and breast muscles.
High density lipoprotein is rich in proteins such as apolipoprotein-A1 and lecithin-cholesterol acyltransferase, and lecithin-cholesterol acyltransferase can be activated by apolipoprotein-A1 in high density lipoprotein (36). In poultry, high density lipoprotein extracts cholesterol from tissue cell membranes and esterifies it in the presence of lecithin-cholesterol acyltransferase (37). The newly formed high density lipoprotein is transformed into a mature one by the repeated action of lecithin cholesterol lipid acyltransferase, which in turn transports cholesterol (esters) from senescent cell membranes in peripheral tissues as well as from plasma to the liver for metabolism (38, 39). High density lipoprotein transports cholesterol from extrahepatic tissues to the liver for metabolism (40). In this study, the high density lipoprotein contents in liver and breast muscles were increased, which coincided with a reduction in cholesterol levels, suggesting that guanidinoacetic acid improved lipid metabolism in ducks.
Low density lipoprotein is formed from the metabolites of very low density lipoproteins, which are rich in cholesterol (esters), and apolipoprotein-B, and thus it is the primary form of endogenous cholesterol synthesized by the liver that is translocated to the tissues (41). The adrenal cortex, testis, ovary, and liver tissues have specific low density lipoprotein receptors on their surfaces, which can specifically recognize and bind apolipoproteins on low density lipoprotein particles (42). Low density lipoprotein in plasma binds to the low density lipoprotein receptor on the cell surface to form the low density lipoprotein-receptor complex, which is then ingested into the intracellular endocytic vesicles through endocytosis and then fused with intracellular lysosomes, where the hydrolytic enzymes within the lysosome degrade the low density lipoprotein, with the proteins hydrolyzed to amino acids and cholesterol esters hydrolyzed to cholesterol and fatty acids (43). Free cholesterol can be incorporated into the plasma membrane of poultry cells or esterified and stored as ester-type cholesterol in cells, otherwise metabolized to generate sterol-type actives, and it can also be used to regulate the level of cholesterol in poultry by feedback inhibition of the synthesis of β-hydroxy-β-methylglutaryl monoacyl-CoA reductase (44, 45). In this study, the low density lipoprotein contents in liver and breast muscles were decreased in GA600 and GA800, which was in line with the increase of high density lipoprotein contents and the decrease of cholesterol contents mentioned above and also suggested that guanidinoacetic acid can change the fat metabolism process by regulating the low density lipoprotein contents.
Most of the apolipoproteins (Apo) involved in lipoprotein formation have a bisexual α-helix structure, with uncharged hydrophobic amino acid residues distributed on the non-polar side of the helix and charged hydrophilic amino acid residues distributed on the polar side of the helix, which is conducive to the binding of apolipoproteins to lipids and stabilizes the structure of lipoproteins (46). Different lipoproteins contain different apolipoproteins, and different apolipoproteins have other functions, but their primary function is binding and transporting lipids (46, 47). Apolipoproteins are also involved in biochemical processes such as the regulation of the key enzyme activities in lipoprotein metabolism and lipoprotein receptor recognition, e.g., the tyrosine on the Apo-A1 molecule is an essential motif for binding to high density lipoproteins (46, 48). The Apo-Bs play a role in the reciprocal transfer and exchange of lipoproteins and proteins during lipoprotein metabolism, e.g., cholesterol transport proteins promote the exchange of cholesteryl esters and triacylglycerols among high density lipoprotein, very low density lipoproteins and low density lipoprotein. In contrast, phospholipid transport proteins encourage the interaction of phospholipids between lipoproteins (46, 49). In addition, Apo-B has an irreplaceable role in the biochemical processes of deposition and transport of triglyceride-rich lipids and lipids (50). In this study, the Apo-A1 levels increased, and Apo-B levels decreased in liver and breast muscles in GA600 and GA800, which also echoes the increase in the high-density lipoprotein contents and the decrease in the contents of low-density lipoprotein and cholesterol mentioned above, which also suggested that guanidine acetic acid can change the process of fat metabolism by regulating the apolipoprotein contents.
Lipoprotein lipase, a key enzyme in the classical regulation of lipid metabolism and one of the rate-limiting enzymes of lipid metabolism has an essential effect on fat deposition and intramuscular fat content in animals (51). Lipoprotein lipase catalyzes the breakdown of triglycerides in triglyceride-rich lipoproteins, celiac particles, and very low-density lipoprotein cores into fatty acids and monoglycerides for oxidative energy supply, storage, and utilization by tissues. It plays a vital role in the glucose and lipid metabolism regulation in animals (52). Animal cells generally have no or only a small number of fat droplets; if a more significant number of fat droplets are present within the cells, they are referred to as fat deposits or steatosis, which are formed to a large extent by the buildup of triglycerides within the fat cells leading to cellular hypertrophy (51, 53). Excessive fatty acid intake, fatty acid oxidation, or impaired lipoprotein synthesis can lead to lipoprotein lipase activity changes, ultimately altering poultry lipid metabolism (54, 55). In this study, the lipoprotein lipase levels in liver and breast muscles were increased in GA600 and GA800, which was in line with the reduction of triglycerides mentioned above and also suggested that guanidinoacetic acid can regulate the fat deposition and distribution by regulating lipoprotein lipase. Malate dehydrogenase is an essential enzyme in the tricarboxylic acid cycle that catalyzes the malate dehydrogenation to produce oxaloacetate and NADH+ H+ (56, 57). This reaction is reversible, but intracellularly produced oxaloacetate is constantly being used to synthesize citric acid, so this reversible reaction tends to proceed more in the direction of oxaloacetate production (58). The fatty acid β-oxidation product acetyl CoA can enter the tricarboxylic acid cycle to generate citric acid for further catabolism, thus indicating that malate dehydrogenase plays a vital role in linking glucose metabolism and fatty acid metabolism in poultry (59, 60). The malate dehydrogenase levels in liver and breast muscles in groups treated with guanidinoacetic acid were decreased in this study, which was in line with the reduction of malondialdehyde, cholesterol, and triglyceride and the increase of phospholipid mentioned above, which also suggested that guanidinoacetic acid can regulate lipid metabolism by regulating malate dehydrogenase.
In poultry, fatty acids or lipoyl CoA activated in the cytosol and the acyl carrier carnitine, also known as L-β-hydroxy-gamma-trimethylaminobutyric acid, enter the mitochondria through the mitochondrial membrane catalyzed by carnitine lipoyl transferase (61, 62). Carnitine lipoyl transferase has two isozymes with different antigenic properties, I and II, flanking the outer and inner mitochondrial membrane, respectively (63). Enzyme I, located in the outer mitochondrial membrane, promotes the conversion of fatty acyl groups to lipoyl carnitine, which is transported into the inner membrane through the lipoyl carnitine carrier on the inner mitochondrial membrane and then converted to lipoyl CoA and released carnitine under the enzyme II action (64). Carnitine lipoyl transferase I is the rate-limiting enzyme of the process, which is inhibited by an increase of malonyl CoA in the process of fat synthesis, thus inhibiting fatty acid oxidation (63, 65). Lipoyl CoA entering the mitochondria of poultry cells undergoes dehydrogenation, addition of water, dehydrogenation, and sulfolysis to form lipoyl CoA and acetyl CoA, which have two fewer carbon atoms than the original (66). For saturated fatty acids with an even number of carbon atoms, such as palmitic acid, octadecanestearic acid, and arachidic acid, the final oxidized product is acetyl CoA, which then enters the tricarboxylic acid cycle for further decomposition (67). Unsaturated fatty acids containing double bonds in the mitochondria will go through multiple rounds of β-oxidation of long-chain unsaturated fatty acids under the enoyl CoA isomerase action, and the cis-double bond that may appear between the β and γ carbon atoms will be isomerized to form a trans-double bond between the α and β positions (68).
Similarly, when β-oxidation proceeds to the appearance of a cis-double bond between the γ and δ carbon atoms, a trans-double bond will be formed by the dehydrogenation reaction catalyzed by lipoyl CoA dehydrogenase between the α and β carbon atoms to produce a dienoic acid, followed by the hydrogenation catalyzed by 2,4-diene-lipoyl CoA reductase, which will reduce this dienoic acid to make the trans monoclinic acid, β,γ-enyl lipoyl CoA, which will then be further converted into a trans-diene acid (69). The latter is further isomerized to α,β-enoyl CoA and continues to be oxidatively metabolized to provide energy for poultry (70). In this study, guanidinoacetic acid supplementation did not influence the saturated fatty acid, monounsaturated fatty acid, and polyunsaturated fatty acid contents among groups, which suggested that guanidinoacetic acid has little effect on fatty acid composition in breast muscles and only has a more significant impact on fat deposition and distribution.
Stearoyl CoA desaturase is a critical enzyme in maintaining fat synthesis and energy homeostasis (71). It introduces a double bond between the 9th and 10th carbon atoms of the saturated fatty acids palmitic acid (C16:0) and stearic acid (C18:0), which are converted to the monounsaturated fatty acids palmitoleic acid (C16:1 n-7) and oleic acid (C18:1 n-9), respectively (72). Free fatty acid receptor 4 is a member of the G-protein-coupled receptor family (GPCRs), which activates various physiological functions such as adipocyte differentiation (73). In this study, the guanidinoacetic acid did not influence the stearoyl CoA desaturase and free fatty acid receptor 4 expressions among groups, which was in line with the reductions in abdominal fat, cholesterol, malondialdehyde, and triglyceride contents, and the insignificant differences in saturated fatty acid, monounsaturated fatty acid, and polyunsaturated fatty acid contents mentioned above. The relative insulin induced gene 1, fatty acid transport protein 1, and lipoprotein lipase expression were increased, the relative fatty acid synthetase expression was decreased in groups treated with guanidinoacetic acid, which echoed the increased lipoprotein lipase, total lipase, phospholipids, high density lipoprotein, apolipoprotein-A1 levels, and reduced low density lipoprotein, apolipoprotein-B, abdominal fat, cholesterol, malondialdehyde, and triglycerides levels mentioned above, and suggested that guanidinoacetic acid can modulate the expression of specific genes to regulate fat deposition and distribution in ducks.
In this study, adding 600 mg/kg dietary guanidinoacetic acid to the diet improved lipid levels, promoted the lipoprotein levels and the lipid metabolism-related gene expressions in ducks.
The raw data supporting the conclusions of this article will be made available by the authors, without undue reservation.
All procedures involving care and management were approved by the Institutional Animal Care and Use Committee of the Chinese Academy of Tropical Agricultural Sciences (Approval No.: CATAS-20220819-2). The study was conducted in accordance with the local legislation and institutional requirements.
HW: Formal analysis, Software, Writing – review & editing. JX: Software, Writing – original draft. WP: Formal analysis, Software, Writing – original draft. FJ: Methodology, Software, Writing – original draft. JQ: Methodology, Software, Writing – original draft. QS: Methodology, Software, Writing – review & editing. GH: Methodology, Project administration, Writing – review & editing.
The author(s) declare financial support was received for the research, authorship, and/or publication of this article. This work was supported by the Hainan Provincial Natural Science Foundation of China (322MS134), Non-profit Research Initiation Funding from Tropical Crops Genetic Resources Institute, CATAS (PZS2023015), and the China Scholarship Council (CSC) National Public Graduate Program for Building Highly Qualified Universities.
QS was employed by the Hainan Xuhuai Technology Co., Ltd.
The remaining authors declare that the research was conducted in the absence of any commercial or financial relationships that could be construed as a potential conflict of interest.
All claims expressed in this article are solely those of the authors and do not necessarily represent those of their affiliated organizations, or those of the publisher, the editors and the reviewers. Any product that may be evaluated in this article, or claim that may be made by its manufacturer, is not guaranteed or endorsed by the publisher.
1. Lim, SA, Su, W, Chapman, NM, and Chi, H. Lipid metabolism in T cell signaling and function. Nat Chem Biol. (2022) 18:470–81. doi: 10.1038/s41589-022-01017-3
2. DeBose-Boyd, RA. Significance and regulation of lipid metabolism. Semin Cell Dev Biol. (2018) 81:97. doi: 10.1016/j.semcdb.2017.12.003
3. Lei, Q, Yang, J, Li, L, Zhao, N, Lu, C, Lu, A, et al. Lipid metabolism and rheumatoid arthritis. Front Immunol. (2023) 14:1190607. doi: 10.3389/fimmu.2023.1190607
4. Bacci, M, Lorito, N, Smiriglia, A, and Morandi, A. Fat and furious: lipid metabolism in antitumoral therapy response and resistance. Trends Cancer. (2021) 7:198–213. doi: 10.1016/j.trecan.2020.10.004
5. Forbes, SC, Cordingley, DM, Cornish, SM, Gualano, B, Roschel, H, Ostojic, SM, et al. Effects of creatine supplementation on brain function and health. Nutrients. (2022) 14:921. doi: 10.3390/nu14050921
6. Ostojic, SM, and Jorga, J. Guanidinoacetic acid in human nutrition: beyond creatine synthesis. Food Sci Nutr. (2023) 11:1606–11. doi: 10.1002/fsn3.3201
7. Semeredi, S, Stajer, V, Ostojic, J, Vranes, M, and Ostojic, SM. Guanidinoacetic acid with creatine compared with creatine alone for tissue creatine content, hyperhomocysteinemia, and exercise performance: a randomized, double-blind superiority trial. Nutrition. (2019) 57:162–6. doi: 10.1016/j.nut.2018.04.009
8. Ostojic, SM. Safety of dietary guanidinoacetic acid: a villain of a good guy? Nutrients. (2021) 14:75. doi: 10.3390/nu14010075
9. Majdeddin, M, Braun, U, Lemme, A, Golian, A, Kermanshahi, H, De Smet, S, et al. Effects of feeding guanidinoacetic acid on oxidative status and creatine metabolism in broilers subjected to chronic cyclic heat stress in the finisher phase. Poult Sci. (2023) 102:102653. doi: 10.1016/j.psj.2023.102653
10. Janes, D Jr, Suehs, B, and Gatlin, DM. Dietary creatine and guanidinoacetic acid supplementation have limited effects on hybrid striped bass. Fish Physiol Biochem. (2023) 49:399–407. doi: 10.1007/s10695-023-01196-3
11. Michiels, J, Maertens, L, Buyse, J, Lemme, A, Rademacher, M, Dierick, NA, et al. Supplementation of guanidinoacetic acid to broiler diets: effects on performance, carcass characteristics, meat quality, and energy metabolism. Poult Sci. (2012) 91:402–12. doi: 10.3382/ps.2011-01585
12. Khalil, S, Saenbungkhor, N, Kesnava, K, Sivapirunthep, P, Sitthigripong, R, Jumanee, S, et al. Effects of guanidinoacetic acid supplementation on productive performance, pectoral myopathies, and meat quality of broiler chickens. Animals. (2021) 11:3180. doi: 10.3390/ani11113180
13. Asiriwardhana, M, and Bertolo, RF. Guanidinoacetic acid supplementation: a narrative review of its metabolism and effects in swine and poultry. Front Anim Sci. (2022) 3:972868. doi: 10.3389/fanim.2022.972868
14. Córdova-Noboa, HA, Oviedo-Rondón, EO, Sarsour, AH, Barnes, J, Ferzola, P, Rademacher-Heilshorn, M, et al. Performance, meat quality, and pectoral myopathies of broilers fed either corn or sorghum based diets supplemented with guanidinoacetic acid. Poult Sci. (2018) 97:2479–93. doi: 10.3382/ps/pey096
15. Majdeddin, M, Golian, A, Kermanshahi, H, De Smet, S, and Michiels, J. Guanidinoacetic acid supplementation in broiler chickens fed on corn-soybean diets affects performance in the finisher period and energy metabolites in breast muscle independent of diet nutrient density. Br Poult Sci. (2018) 59:443–51. doi: 10.1080/00071668.2018.1476678
16. Sharma, NK, Cadogan, DJ, Chrystal, PV, McGilchrist, P, Wilkinson, SJ, Inhuber, V, et al. Guanidinoacetic acid as a partial replacement to arginine with or without betaine in broilers offered moderately low crude protein diets. Poult Sci. (2022) 101:101692. doi: 10.1016/j.psj.2021.101692
17. Majdeddin, M, Braun, U, Lemme, A, Golian, A, Kermanshahi, H, De Smet, S, et al. Guanidinoacetic acid supplementation improves feed conversion in broilers subjected to heat stress associated with muscle creatine loading and arginine sparing. Poult Sci. (2020) 99:4442–53. doi: 10.1016/j.psj.2020.05.023
18. He, DT, Gai, XR, Yang, LB, Li, JT, Lai, WQ, Sun, XL, et al. Effects of guanidinoacetic acid on growth performance, creatine and energy metabolism, and carcass characteristics in growing-finishing pigs. J Anim Sci. (2018) 96:3264–73. doi: 10.1093/jas/sky186
19. Jayaraman, B, La, KV, La, H, Doan, V, Carpena, EM, Rademacher, M, et al. Supplementation of guanidinoacetic acid to pig diets: effects on performance, carcass characteristics, and meat quality. J Anim Sci. (2018) 96:2332–41. doi: 10.1093/jas/sky137
20. Zhang, S, Zang, C, Pan, J, Ma, C, Wang, C, Li, X, et al. Effects of dietary guanidinoacetic acid on growth performance, guanidinoacetic acid absorption and creatine metabolism of lambs. PLoS One. (2022) 17:e0264864. doi: 10.1371/journal.pone.0264864
21. Tajabadi, F, Ghorbani-Nohooji, M, Gholami, A, Ghiasi Yekta, M, Ghasemi, SV, Nabati, F, et al. Gas chromatography/mass spectrometry with chemometric methods for the determination of fatty acid profiles in herbal oils. Biomed Chromatogr. (2023) 37:e5566. doi: 10.1002/bmc.5566
22. Malgwi, IH, Halas, V, Grünvald, P, Schiavon, S, and Jócsák, I. Genes related to fat metabolism in pigs and intramuscular fat content of pork: a focus on nutrigenetics and nutrigenomics. Animals. (2022) 12:150. doi: 10.3390/ani12020150
23. Wu, H, Xu, C, Wang, J, Hu, C, Ji, F, Xie, J, et al. Effects of dietary probiotics and acidifiers on the production performance, colostrum components, serum antioxidant activity and hormone levels, and gene expression in mammary tissue of lactating sows. Animals. (2023) 13:1536. doi: 10.3390/ani13091536
24. Xu, C, Xie, J, Ji, F, Peng, W, Song, Y, Diao, X, et al. Supplementation of dietary semen vaccariae extracts to lactating sow diets: effects on the production performance, milk components, and gene expression related to mammogenesis. Front Vet Sci. (2023) 10:1284552. doi: 10.3389/fvets.2023.1284552
25. Chella Krishnan, K, Vergnes, L, Acín-Pérez, R, Stiles, L, Shum, M, Ma, L, et al. Sex-specific genetic regulation of adipose mitochondria and metabolic syndrome by Ndufv2. Nat Metab. (2021) 3:1552–68. doi: 10.1038/s42255-021-00481-w
26. Luo, J, Yang, H, and Song, BL. Mechanisms and regulation of cholesterol homeostasis. Nat Rev Mol Cell Biol. (2020) 21:225–45. doi: 10.1038/s41580-019-0190-7
27. Schade, DS, Shey, L, and Eaton, RP. Cholesterol review: a metabolically important molecule. Endocr Pract. (2020) 26:1514–23. doi: 10.4158/EP-2020-0347
28. Huo, Y, Ma, F, Li, L, Li, Y, Zhong, G, Liao, J, et al. Effect of copper exposure on the cholesterol metabolism in broiler liver. Biol Trace Elem Res. (2023) 201:5747–55. doi: 10.1007/s12011-023-03609-z
29. Alves-Bezerra, M, and Cohen, DE. Triglyceride metabolism in the liver. Compr Physiol. (2017) 8:1–8. doi: 10.1002/cphy.c170012
30. Galsgaard, KD, Elmelund, E, Johansen, CD, Bomholt, AB, Kizilkaya, HS, Ceutz, F, et al. Glucagon receptor antagonism impairs and glucagon receptor agonism enhances triglycerides metabolism in mice. Mol Metab. (2022) 66:101639. doi: 10.1016/j.molmet.2022.101639
31. Cerk, IK, Wechselberger, L, and Oberer, M. Adipose triglyceride lipase regulation: an overview. Curr Protein Pept Sci. (2018) 19:221–33. doi: 10.2174/1389203718666170918160110
32. Cherian, G, Fraz, A, and Bionaz, M. Evaluating the impact of organic chromium on hepatic phospholipid fatty acid molecular species, transcription of genes associated with lipid metabolism and oxidative status in broiler chickens fed flaxseed. Poult Sci. (2023) 102:102976. doi: 10.1016/j.psj.2023.102976
33. Guido, ME, Garbarino Pico, E, and Caputto, BL. Circadian regulation of phospholipid metabolism in retinal photoreceptors and ganglion cells. J Neurochem. (2001) 76:835–45. doi: 10.1046/j.1471-4159.2001.00081.x
34. Ayala, A, Muñoz, MF, and Argüelles, S. Lipid peroxidation: production, metabolism, and signaling mechanisms of malondialdehyde and 4-hydroxy-2-nonenal. Oxid Med Cell Longev. (2014) 2014:360438. doi: 10.1155/2014/360438
35. Tsikas, D. Assessment of lipid peroxidation by measuring malondialdehyde (MDA) and relatives in biological samples: analytical and biological challenges. Anal Biochem. (2017) 524:13–30. doi: 10.1016/j.ab.2016.10.021
36. Hu, J, Xi, D, Zhao, J, Luo, T, Liu, J, Lu, H, et al. High-density lipoprotein and inflammation and its significance to atherosclerosis. Am J Med Sci. (2016) 352:408–15. doi: 10.1016/j.amjms.2016.06.014
37. Fernandes das Neves, M, Batuca, JR, and Delgado Alves, J. The role of high-density lipoprotein in the regulation of the immune response: implications for atherosclerosis and autoimmunity. Immunology. (2021) 164:231–41. doi: 10.1111/imm.13348
38. Sorci-Thomas, MG, and Thomas, MJ. High density lipoprotein biogenesis, cholesterol efflux, and immune cell function. Arterioscler Thromb Vasc Biol. (2012) 32:2561–5. doi: 10.1161/ATVBAHA.112.300135
39. Graham, A. Modulation of the cellular microrna landscape: contribution to the protective effects of high-density lipoproteins (HDL). Biology. (2023) 12:1232. doi: 10.3390/biology12091232
40. Tosheska Trajkovska, K, and Topuzovska, S. High-density lipoprotein metabolism and reverse cholesterol transport: strategies for raising HDL cholesterol. Anatol J Cardiol. (2017) 18:149–54. doi: 10.14744/AnatolJCardiol.2017.7608
41. Khatana, C, Saini, NK, Chakrabarti, S, Saini, V, Sharma, A, Saini, RV, et al. Mechanistic insights into the oxidized low-density lipoprotein-induced atherosclerosis. Oxid Med Cell Longev. (2020) 2020:5245308. doi: 10.1155/2020/5245308
42. Di, L, and Maiseyeu, A. Low-density lipoprotein nanomedicines: mechanisms of targeting, biology, and theranostic potential. Drug Deliv. (2021) 28:408–21. doi: 10.1080/10717544.2021.1886199
43. Dergunov, AD, Smirnova, EA, Merched, A, Visvikis, S, Siest, G, Yakushkin, VV, et al. Structural peculiarities of the binding of very low density lipoproteins and low density lipoproteins to the LDL receptor in hypertriglyceridemia: role of apolipoprotein E. Biochim Biophys Acta. (2000) 1484:29–40. doi: 10.1016/s1388-1981(99)00197-3
44. Dergunov, AD, Smirnova, EA, Merched, A, Visvikis, S, Siest, G, Yakushkin, VV, et al. Conformation of apolipoprotein E both in free and in lipid-bound form may determine the avidity of triglyceride-rich lipoproteins to the LDL receptor: structural and kinetic study. Biochim Biophys Acta. (2000) 1484:14–28. doi: 10.1016/s1388-1981(99)00196-1
45. Mahmoudian, M, Salatin, S, and Khosroushahi, AY. Natural low- and high-density lipoproteins as mighty bio-nanocarriers for anticancer drug delivery. Cancer Chemother Pharmacol. (2018) 82:371–82. doi: 10.1007/s00280-018-3626-4
46. Su, X, and Peng, D. The exchangeable apolipoproteins in lipid metabolism and obesity. Clin Chim Acta. (2020) 503:128–35. doi: 10.1016/j.cca.2020.01.015
47. Wu, CL, Zhao, SP, and Yu, BL. Intracellular role of exchangeable apolipoproteins in energy homeostasis, obesity and non-alcoholic fatty liver disease. Biol Rev Camb Philos Soc. (2015) 90:367–76. doi: 10.1111/brv.12116
48. Gao, L, Zhang, Y, Wang, X, and Dong, H. Association of apolipoproteins A1 and B with type 2 diabetes and fasting blood glucose: a cross-sectional study. BMC Endocr Disord. (2021) 21:59. doi: 10.1186/s12902-021-00726-5
49. Sniderman, A, Langlois, M, and Cobbaert, C. Update on apolipoprotein B. Curr Opin Lipidol. (2021) 32:226–30. doi: 10.1097/MOL.0000000000000754
50. Vlad, CE, Foia, L, Popescu, R, Ivanov, I, Luca, MC, Delianu, C, et al. Apolipoproteins a and B and PCSK9: nontraditional cardiovascular risk factors in chronic kidney disease and in end-stage renal disease. J Diabetes Res. (2019) 2019:6906278. doi: 10.1155/2019/6906278
51. Wu, SA, Kersten, S, and Qi, L. Lipoprotein lipase and its regulators: an unfolding story. Trends Endocrinol Metab. (2021) 32:48–61. doi: 10.1016/j.tem.2020.11.005
52. Wen, Y, Chen, YQ, and Konrad, RJ. The regulation of triacylglycerol metabolism and lipoprotein lipase activity. Adv Biol. (2022) 6:e2200093. doi: 10.1002/adbi.202200093
53. Basu, D, and Goldberg, IJ. Regulation of lipoprotein lipase-mediated lipolysis of triglycerides. Curr Opin Lipidol. (2020) 31:154–60. doi: 10.1097/MOL.0000000000000676
54. Kobayashi, J, and Mabuchi, H. Lipoprotein lipase and atherosclerosis. Ann Clin Biochem. (2015) 52:632–7. doi: 10.1177/0004563215590451
55. Sylvers-Davie, KL, and Davies, BSJ. Regulation of lipoprotein metabolism by ANGPTL3, ANGPTL4, and ANGPTL8. Am J Physiol Endocrinol Metab. (2021) 321:E493–508. doi: 10.1152/ajpendo.00195.2021
56. Kayamba, F, Faya, M, Pooe, OJ, Kushwaha, B, Kushwaha, ND, Obakachi, VA, et al. Lactate dehydrogenase and malate dehydrogenase: potential antiparasitic targets for drug development studies. Bioorg Med Chem. (2021) 50:116458. doi: 10.1016/j.bmc.2021.116458
57. Provost, JJ. Increasing access for biochemistry research in undergraduate education: the malate dehydrogenase CURE community. J Biol Chem. (2022) 298:102298. doi: 10.1016/j.jbc.2022.102298
58. Eleftheriadis, T, Pissas, G, Golfinopoulos, S, Efthymiadi, M, Liakopoulos, V, and Stefanidis, I. Inhibition of malate dehydrogenase-2 protects renal tubular epithelial cells from anoxia-reoxygenation-induced death or senescence. Biomol Ther. (2022) 12:1415. doi: 10.3390/biom12101415
59. Shimozawa, Y, Matsuhisa, H, Nakamura, T, Himiyama, T, and Nishiya, Y. Reducing substrate inhibition of malate dehydrogenase from Geobacillus stearothermophilus by C-terminal truncation. Protein Eng Des Sel. (2022) 35:gzac008. doi: 10.1093/protein/gzac008
60. Shimozawa, Y, Himiyama, T, Nakamura, T, and Nishiya, Y. Structural analysis and reaction mechanism of malate dehydrogenase from Geobacillus stearothermophilus. J Biochem. (2021) 170:97–105. doi: 10.1093/jb/mvab027
61. de Carvalho, CCCR, and Caramujo, MJ. The various roles of fatty acids. Molecules. (2018) 23:2583. doi: 10.3390/molecules23102583
62. Dyall, SC, Balas, L, Bazan, NG, Brenna, JT, Chiang, N, da Costa, SF, et al. Polyunsaturated fatty acids and fatty acid-derived lipid mediators: recent advances in the understanding of their biosynthesis, structures, and functions. Prog Lipid Res. (2022) 86:101165. doi: 10.1016/j.plipres.2022.101165
63. Ni, M, Solmonson, A, Pan, C, Yang, C, Li, D, Notzon, A, et al. Functional assessment of lipoyltransferase-1 deficiency in cells, mice, and humans. Cell Rep. (2019) 27:1376–1386.e6. doi: 10.1016/j.celrep.2019.04.005
64. Taché, V, Bivina, L, White, S, Gregg, J, Deignan, J, Boyadjievd, SA, et al. Lipoyltransferase 1 gene defect resulting in fatal lactic acidosis in two siblings. Case Rep Obstet Gynecol. (2016) 2016:6520148. doi: 10.1155/2016/6520148
65. Liu, Y, Luo, G, Yan, Y, and Peng, J. A pan-cancer analysis of copper homeostasis-related gene lipoyltransferase 1: its potential biological functions and prognosis values. Front Genet. (2022) 13:1038174. doi: 10.3389/fgene.2022.1038174
66. Rogero, MM, and Calder, PC. Obesity, inflammation, toll-like receptor 4 and fatty acids. Nutrients. (2018) 10:432. doi: 10.3390/nu10040432
67. Bloise, AMNLG, Simões-Alves, AC, Debora Santos, A, Morio, B, and Costa-Silva, JH. Cardiometabolic impacts of saturated fatty acids: are they all comparable? Int J Food Sci Nutr. (2022) 73:1–14. doi: 10.1080/09637486.2021.1940885
68. Gao, X, Su, X, Han, X, Wen, H, Cheng, C, Zhang, S, et al. Unsaturated fatty acids in mental disorders: an umbrella review of meta-analyses. Adv Nutr. (2022) 13:2217–36. doi: 10.1093/advances/nmac084
69. Tahri-Joutey, M, Andreoletti, P, Surapureddi, S, Nasser, B, Cherkaoui-Malki, M, and Latruffe, N. Mechanisms mediating the regulation of peroxisomal fatty acid beta-oxidation by PPARα. Int J Mol Sci. (2021) 22:8969. doi: 10.3390/ijms22168969
70. Hoppenreijs, LJG, Berton-Carabin, CC, Dubbelboer, A, and Hennebelle, M. Evaluation of oxygen partial pressure, temperature and stripping of antioxidants for accelerated shelf-life testing of oil blends using 1H NMR. Food Res Int. (2021) 147:110555. doi: 10.1016/j.foodres.2021.110555
71. AM, ALJ, Syed, DN, and Ntambi, JM. Insights into stearoyl-CoA desaturase-1 regulation of systemic metabolism. Trends Endocrinol Metab. (2017) 28:831–42. doi: 10.1016/j.tem.2017.10.003
72. Ntambi, JM, and Miyazaki, M. Recent insights into stearoyl-CoA desaturase-1. Curr Opin Lipidol. (2003) 14:255–61. doi: 10.1097/00041433-200306000-00005
Keywords: guanidinoacetic acid, fat deposition, lipase activity, fatty acid composition, lipid metabolism genes
Citation: Wu H, Xie J, Peng W, Ji F, Qian J, Shen Q and Hou G (2024) Effects of guanidinoacetic acid supplementation on liver and breast muscle fat deposition, lipid levels, and lipid metabolism-related gene expression in ducks. Front. Vet. Sci. 11:1364815. doi: 10.3389/fvets.2024.1364815
Received: 03 January 2024; Accepted: 02 February 2024;
Published: 16 February 2024.
Edited by:
Izhar Hyder Qazi, Shaheed Benazir Bhutto University of Veterinary & Animal Sciences, PakistanReviewed by:
Xihong Zhou, Chinese Academy of Sciences (CAS), ChinaCopyright © 2024 Wu, Xie, Peng, Ji, Qian, Shen and Hou. This is an open-access article distributed under the terms of the Creative Commons Attribution License (CC BY). The use, distribution or reproduction in other forums is permitted, provided the original author(s) and the copyright owner(s) are credited and that the original publication in this journal is cited, in accordance with accepted academic practice. No use, distribution or reproduction is permitted which does not comply with these terms.
*Correspondence: Guanyu Hou, Z3Vhbnl1aG91QDEyNi5jb20=
†These authors have contributed equally to this work
Disclaimer: All claims expressed in this article are solely those of the authors and do not necessarily represent those of their affiliated organizations, or those of the publisher, the editors and the reviewers. Any product that may be evaluated in this article or claim that may be made by its manufacturer is not guaranteed or endorsed by the publisher.
Research integrity at Frontiers
Learn more about the work of our research integrity team to safeguard the quality of each article we publish.