- 1Department of Animal Science, University of Tennessee, Knoxville, TN, United States
- 2East Tennessee AgResearch and Education Center-Little River Animal and Environmental Unit, University of Tennessee, Walland, TN, United States
Bovine mastitis is one of the most common diseases of dairy cattle. Even though different infectious microorganisms and mechanical injury can cause mastitis, bacteria are the most common cause of mastitis in dairy cows. Staphylococci, streptococci, and coliforms are the most frequently diagnosed etiological agents of mastitis in dairy cows. Staphylococci that cause mastitis are broadly divided into Staphylococcus aureus and non-aureus staphylococci (NAS). NAS is mainly comprised of coagulase-negative Staphylococcus species (CNS) and some coagulase-positive and coagulase-variable staphylococci. Current staphylococcal mastitis control measures are ineffective, and dependence on antimicrobial drugs is not sustainable because of the low cure rate with antimicrobial treatment and the development of resistance. Non-antimicrobial effective and sustainable control tools are critically needed. This review describes the current status of S. aureus and NAS mastitis in dairy cows and flags areas of knowledge gaps.
1 Introduction
Mastitis is an inflammation of mammary glands usually caused by bacteria. It can also be caused by fungi or occasionally by mechanical injury, resulting in increased milk somatic cell count (SCC) and/or abnormal changes in milk and gland tissue (1). Mastitis incurs huge economic losses to dairy farming worldwide; in the United States (U.S.) dairy industry alone, economic losses are more than $2 billion annually (2–4). Clinical mastitis costs $444 for each case during 30 days in milk (DIM) post-calving (2). Staphylococcus aureus and non-aureus staphylococci (NAS) cause mastitis in dairy cows. S. aureus is a major contagious mammary pathogen on the U.S. dairy farms and throughout the globe (5). NAS comprises more than 50 different species of coagulase-negative staphylococci (6–9) and some coagulase-positive and coagulase-variable staphylococci (8, 10–16). Approximately 95% of coagulase-positive Staphylococcus isolates from bovine mastitis are S. aureus (17), and about 15% of NAS have been linked to bovine mastitis (18, 19). S. chromogenes is a predominant NAS (19–21) consistently isolated from subclinical mastitis cases (22, 23), cows’ udder, and teat skin (24, 25).
Management-based mastitis control measures have been developed and implemented with mild success in reducing contagious bacteria such as S. aureus and S. agalactiae (26–28) but limited success due to the application disparities across mastitis management (29). Dependence on antimicrobial drugs to control S. aureus and NAS is not sustainable due to limited success (30, 31) and the emergence of bacteria resistant to the commonly used antimicrobial drugs (32, 33).
Currently, one commercial bacterin vaccine is claimed to have some effects against S. aureus mastitis in dairy cows in the US. However, studies evaluating the efficacy of this commercial vaccine found no significant difference between vaccinated and unvaccinated control cows (34–36). Another polyvalent commercial bacterin vaccine containing inactivated high biofilm-forming S. aureus strain SP 140 and E. coli J5 strain is available in Europe and a few other countries for the control of mastitis caused by S. aureus, NAS, E. coli, and other coliforms in dairy cows. Some efficacy studies on this vaccine concluded that vaccination with the polyvalent bacterin reduced mastitis incidence, severity, and duration (37–39), whereas others concluded that vaccination with the polyvalent bacterin did not induce a significant reduction in staphylococcal intramammary infection (IMI) between vaccinated and unvaccinated groups (40–43). However, Freick et al. (42) found a significantly lower SCC in cows vaccinated with an autogenous vaccine compared to the unvaccinated group. Based on published vaccine efficacy studies in the United States, currently available vaccines cannot be recommended as part of the routine measures for controlling mastitis due to S. aureus and NAS in dairy cattle. Therefore, effective and sustainable non-antimicrobial bovine S. aureus and NAS mastitis control tools are urgently needed.
2 Bovine staphylococcal mastitis
Staphylococcus belongs to the family of Staphylococcaceae (44–46). Based on the 16S rRNA gene sequence similarity and analysis of overall genome-related indices such as DNA–DNA hybridization, average nucleotide identity, and average amino acid identity analyses, some Staphylococcus subspecies were reclassified as novel species. Five Staphylococcus species (S. sciuri, S. fleurettii, S. lentus, S. stepanovicii, and S. vitulinus) were reassigned to the new Mammaliicoccus genus (47). Since our focus is on the genus Staphylococcus, we did not include the Mammaliicoccus genus in this review. Staphylococci are opportunistic commensal or opportunistic environmental bacteria that inhabit the nostrils, mucus membranes, and skin of mammals and birds (15, 48). More than 60 valid species exist in the Staphylococcus genus (44, 48, 49). In dairy cattle, mastitis is usually caused by Staphylococcus aureus (5) and NAS, which comprises coagulase-negative Staphylococcus species (CNS) (6, 19, 21) and some coagulase-positive and coagulase variable staphylococci (8, 50, 51).
Staphylococci are non-motile facultative anaerobic [except S. saccharolyticus and S. aureus subsp. anaerobius, which are anaerobic (48)] cocci that grow in an aggregating grape-like cluster due to perpendicular division planes. They are biochemically positive or negative or variable for coagulase, negative for oxidase, and positive for gram staining and catalase (44, 48, 49). Staphylococci can survive in the environment over an extended period (52, 53). They are usually catalase-positive, but some catalase-negative rare strains have also been reported (54, 55). All Staphylococcus species are lysed by lysostaphin except a few rare species (55, 56). Staphylococci have a low G/C content of approximately 27–41% in the chromosomal DNA, and most strains grow at 10% NaCl (48). Some species of staphylococci produce coagulase (Coa) and/or von Willebrand factor binding protein (vWbp), both of which can bind to prothrombin and convert it to a complex that can convert fibrinogen in the blood to fibrin (57–59). Coagulase-positive S. aureus is considered a major pathogenic species (15, 48, 55), whereas NAS are considered minor pathogens (15, 48, 55). Though a majority of coagulase-positive Staphylococcus species from bovine mastitis are S. aureus (17), non-aureus coagulase-positive or variable staphylococci occasionally cause mastitis and other diseases in animals, including dairy cows. Staphylococcus intermedius, S. pseudintermedius, and S. coagulans are coagulase-positive Staphylococcus species that cause different diseases in dogs and cats and occasionally rare or sporadic cases of bovine mastitis (10–12). S. aureus subs. Anaerobius (newly reclassified as S. aureus) is coagulase-positive and causes chronic purulent subcutaneous inflammation near superficial lymph nodes in sheep and goats (16, 60). Some coagulase variable species (S. hyicus and S. agnetis) cause mastitis in dairy cows (8). Staphylococcus hyicus causes different diseases in pigs (13–15). Some studies reported the presence of atypical strains of S. chromogenes that cause clotting of plasma (61).
There are also coagulase-negative variants of S. aureus (62, 63). Some coagulase-negative Staphylococcus species (S. chromogenes, S. simulans, S. xylosus, S. haemolyticus, and S. epidermidis) (8, 18, 19) are increasingly reported as the cause of subclinical mastitis and some clinical mastitis in dairy cows (8, 19, 64). The NAS comprises diverse species that vary in pathogenicity, epidemiological distribution, and genomic composition. Describing each species individually and studying its virulence, pathogenicity, distribution, effect on milk somatic cell count (SCC), and milk production losses is more helpful for controlling mastitis caused by these groups of bacteria.
2.1 Staphylococcus aureus mastitis
Staphylococcus aureus is a major contagious mastitis pathogen in the US dairy farms and throughout the globe (5, 65, 66). There are different S. aureus strains (67–69) that also vary in their ability to spread in herds (70, 71), cause mastitis (72–74), incur losses in milk yield (75), possess virulence traits (76–78), and invade mammary epithelial cells (79, 80), but a single strain is reported to predominate in a herd (72). Some dominant clones are reported to cause mastitis worldwide (71, 81–83). Campos et al. reported that genotypes CC97, CC1, CC5, CC8, and CC398 are the most predominant lineages isolated from dairy herds worldwide (71). Of these, CC97 and CC151 seem more pathogenic than others based on molecular and genomic comparative analysis (84). A study on S. aureus isolates from clinical and subclinical cases of mastitis in Finland found five clonal complexes, including CC97, CC133, CC151, CC479, and CC522 (85). The authors evaluated the presence of a total of 296 virulence factors and found 219 were present in all isolates (85). The authors concluded that there was no association between the presence of virulence factors and clinical outcomes of infection, but the presence of virulence factors varied with clonal complexes (85).
Staphylococcus aureus usually causes subclinical mastitis (SCM) and chronic mastitis with high SCC (30, 86). There are considerable variations in the mastitis caused by S. aureus, ranging from the peracute form with the development of gangrene in the udder, which usually occurs during early lactation, to more common subclinical chronic forms resulting in increased SCC and decreased milk production (87, 88). In general, S. aureus mastitis decreased in farms that fully applied mastitis control programs. In dairy farms with low bulk tank milk SCC, the cow-level prevalence of S. aureus IMI is 1–10%. However, in farms with high bulk tank milk SCC, the cow-level prevalence of S. aureus IMI may increase to 50–75% with individual udder quarter IMI prevalence of 10–25% (89, 90). The prevalence of S. aureus IMI in heifers is 5–15% at parturition (89, 91). Staphylococcus aureus mastitis treatment with antibiotics is not effective, and the cure rate is very low (30).
2.2 Mastitis due to non-aureus staphylococci
2.2.1 NAS as minor pathogens/commensals in the mammary glands
NAS is a group of over 50 different species of coagulase-negative staphylococci, along with some coagulase-positive and variable staphylococci. Despite the presence of different species, only about 15–20 species are associated with bovine IMI, and the most frequent isolates include S. chromogenes, S. simulans, S. xylosus, S. haemolyticus, and S. epidermidis (8, 18, 19). NAS are increasingly reported as the most frequent isolates from lactating dairy cows (6, 20, 25, 92). Some NAS are frequently reported as etiology of subclinical mastitis in dairy ruminants (6–9, 93, 94), while others occasionally cause mastitis in dairy cows as well as other diseases in animals (10–15). Some studies reported S. chromogenes, S. simulans, S. epidermidis, and S. xylosus as major isolates from teat skin and teat tips, whereas other studies identified S. chromogenes, S. haemolyticus, and S. xylosus as major isolates from milk samples (95–98). S. chromogenes usually colonize the skin of teat and udder in heifers during calving (24, 99, 100), bovine milk of primiparous cows during first lactation (101, 102), and milk of cows with mastitis, especially primiparous cows (25, 101–103). S. simulans is usually isolated from the milk of cows with mastitis (101, 104–106). S. agnetis is a coagulase variable (107) species originally isolated from cows with mastitis and very similar to S. hyicus (105). Based on molecular data, S. simulans is usually isolated from milk with mastitis, but S. chromogenes can be associated with subclinical mastitis and skin microbiota (24, 100). S. epidermidis colonizes the teat apices of dairy cows and healthy human skin (108, 109). NAS inhabit different ecological niches, including bedding materials and different parts of the animal body, including udder and teat skin, nostrils, and teat canal (110). The epidemiological distribution of these groups of bacteria, their spread mechanisms, and reservoirs vary and are affected by environmental, managemental, and host factors (19, 22, 111, 112). The natural habitat of each species needs to be determined to differentiate environmental and host-adapted species (64, 113) to design appropriate control measures for these groups of bacteria.
2.2.2 Genetic diversity and virulence factors of NAS
NAS are genetically different in their ability to cause mastitis in dairy cows (114, 115). They have species-specific virulence factors and pathogenicity that affect the productivity of dairy animals. NAS also form a biofilm that enables them to colonize milking utensils and milkers’ hands, which helps their spread and transmission (116, 117). They also vary in their susceptibility to antimicrobial drugs (19, 112).
2.2.3 Host immune responses against NAS IMI
Macrophages are the first responders of the innate immunity in the mammary glands, with the subsequent recruitment of neutrophils from systemic circulation into the mammary glands (118). Staphylococcus species vary in their ability to induce inflammatory reactions in the mammary glands and increase SCC, with the highest counts usually caused by S. aureus. However, NAS, such as S. chromogenes, S. hyicus, S. agnetis, S. simulans, and S. xylosus are also reported to cause increased SCC similar to S. aureus (87, 119). Staphylococcal IMI, especially S. aureus, usually increases SCC initially, which leads to subclinical mastitis. If S. aureus resists clearance by host defense, the infection becomes chronic, and SCC decreases to a modest level (120). NAS occasionally causes clinical mastitis with SCC, usually ranging in the low to moderate increase, but may cause significantly increased SCC (22).
In experimental challenge infection, S. simulans caused more inflammatory reactions than S. epidermidis (121). Similarly, in field studies, S. simulans caused more clinical mastitis than other NAS (101, 106). Another study found that S. chromogenes originally isolated from milk with mastitis induced more inflammatory reactions than S. chromogenes originated from teat apex (122). However, it is unclear if this difference is because strain differences in virulence or teat skin colonizing strains are non-pathogenic microbiota. In contrast, strains from intramammary areas are pathogenic microbiota. In another study, S. epidermidis and S. haemolyticus were shown to cause high SCC (123). In some studies, a slight increase above 100,000 cells/mL was reported for quarters infected with NAS (109, 124), whereas in another study, SCC varied from as low as 70,000 cells/mL to as high as 123,000 cells/mL of milk depending on the species of NAS involved (20). Some NAS species, such as S. agnetis, S. hyicus, and S. simulans, cause clinical mastitis more frequently than others (101, 104, 105), whereas some others, such as S. epidermidis cause mild inflammatory responses compared to S. simulans (121). However, S. epidermidis was also reported to cause high SCC in subclinical cases of mastitis (123).
Another study found that S. agnetis was more phagocytosed by murine macrophages than S. simulans or S. chromogenes but more resistant to killing by phagocytic cells similar to S. simulans and S. aureus, whereas S. chromogenes was more efficiently killed than S. simulans and S. agnetis (125). Despite observed differences in opsonophagocytic killing of S. simulans and S. chromogenes by phagocytic cells, both can exist in the mammary glands throughout lactation with increased SCC (103, 126). In another study, S. haemolyticus was better phagocytosed by blood neutrophils than S. aureus and S. chromogenes, and both S. aureus and NAS did not prevent intracellular reactive oxygen species (ROS) production in blood and milk neutrophils (127). The authors showed that S. chromogenes induced less ROS in milk neutrophils than S. aureus but induced ROS comparable to S. aureus from blood neutrophils and more ROS from blood neutrophils than S. haemolyticus. Transcripts and protein level evaluations of expression of proinflammatory chemokines and cytokines in the udder of cows with chronic mastitis due to coagulase-positive and coagulase-negative Staphylococci showed no difference between the Staphylococci (128). In another study, S. aureus was known to cause persistent intramammary infection-induced proliferation of CD4+ and CD8+ lymphocytes, whereas S. aureus (originated from nostrils) and S. chromogenes strains (known to cause persistent IMI) had no effect on T and B cell proliferation (129). The authors showed that both S. aureus and S. chromogenes originating from persistent IMI significantly increased IL-17A and IFN-γ production from peripheral blood mononuclear cells. Peripheral blood mononuclear cells (PBMC) from multiparous cows produced significantly higher IL-17A and IFN-γ; multiparous cows tend to have a higher B-lymphocyte and a lower T-lymphocytes proliferative response than primiparous and nulliparous cows.
Staphylococci can resist opsonophagocytic killing by forming capsules and other extracellular polysaccharides (130–132). There are differences among NAS species in their susceptibility to opsonophagocytic killing by macrophages (125). The pathogenic mechanisms responsible for the differences between NAS and S. aureus strains are still unknown and need further investigation. These differences could be due to yet unknown novel virulence factors. Therefore, further investigation is required.
2.2.4 Role of NAS on udder health, milk quality, and SCC
The prevalence of NAS in quarter milk samples in the US and European dairy cattle farms ranges from 27 to 55% (21, 133). Similarly, the prevalence of NAS in bulk tank milk of herds ranges from 43% to 60 or 90% (7, 19, 134). In different countries, NAS species are increasingly reported as an etiology of subclinical mastitis in cows, goats, and sheep (135). Differences in cattle housing, grouping, and age affect NAS prevalence and bacterial count (64). Variations in study methodologies and methods of species identification affect the prevalence assessment of mastitis due to these groups of bacteria (113, 136).
Some studies consider NAS as minor pathogens that cause only a slight increase in SCC and mild clinical mastitis (CM) with no effect (137–139) or little effect on milk production (96, 101, 124, 140–146) In contrast, others report a higher milk production in infected animals than in noninfected animals (142, 147). Some investigators reported no differences among NAS species in individual quarter milk SCC (9, 148), while others reported differences between species (119, 149). A recent study reported that IMI with S. chromogenes early in lactation led to a significantly increased quarter SCC (124). Some NAS species, such as S. chromogenes, S. simulans, and S. xylosus, induced increased SCC comparable to S. aureus (119, 149). Similar to differences observed for the effect on SCC, species-specific differences in persistence were also reported (19, 103, 119, 123). NAS can cause increased SCC (142) and play a role in clinical mastitis development in well-managed herds (142).
The persistence of NAS IMI depends on the specificity of the species involved. Persistent IMI by S. chromogenes and other NAS species induce increased SCC compared to transiently infected quarters (145). However, the authors concluded that both transient and persistent IMI were not significantly associated with quarter milk yield during early lactation (145). Yet, milk yield from quarters recovered from S. chromogenes IMI was significantly lower than uninfected quarters (145), which might indicate some sequential effect in milk production.
NAS species induce only mild inflammatory response with mild to moderate increase in SCC in the infected quarter, reducing milk quality and price, and low bulk tank milk SCC may discourage producers from intervening in IMI, allowing these pathogens to cause continuous loss of productivity (124, 143, 146). In dairy cows with subclinical infection with these groups of pathogens at peak lactation can result in approximately 1.8 kg/d reduction in milk production (94, 146). Because of a modest increase in milk SCC, the IMI due to NAS may not account for increased SCC in dairy farms that already have high SCC due to major mastitis pathogens. Data from farms also showed that NAS species are more prevalent in farms with low bulk tank milk SCC (8, 142), which may indicate that current mastitis control measures that reduce the incidence of some contagious bacteria such as S. aureus and S. agalactiae may not be effective on NAS. The occurrence of mastitis due to these groups of bacteria varies with farms, and economic losses due to subclinical mastitis of these bacteria are difficult to estimate due to the absence of easy and producer-friendly accurate diagnostic tools at the farm level (146, 147, 150). Similar to differences observed for the effect on milk SCC, species-specific differences in persistence have also been reported (19, 103, 119, 123). All these observations clearly indicate that further detailed investigations at the individual species level are required to determine the role of each species in bovine mastitis. Therefore, it is important to study each species of NAS individually and determine their virulence factors, pathogenicity to the host, and disease pathogenesis mechanisms to determine their role in causing mastitis, milk quality, and economic losses.
Some NAS species produce different antimicrobial agents, including bacteriocin, subtilosin A, lysostaphin, and Lugdunin, potentially protecting the colonization of udder or their microenvironmental niches by other bacteria (151–154). Under in vitro conditions, NAS species inhibit biofilm formation by bacterial mastitis pathogens (155), and metabolites from NAS species prevent the expression of S. aureus agr-related genes known to regulate the expression of virulence genes (156). Similarly, under in vivo conditions, the udder, pre-colonized by some strains of NAS, was shown to resist colonization by major bacterial mastitis pathogens (157–160). However, even though pre-colonization of the udder by some members of NAS species seems protective against colonization by major mastitis pathogens, some NAS species themselves were isolated and identified as the etiology of mastitis and shown to be responsible for milk production losses (94, 142, 146). It has also been shown that priming the murine mammary glands with S. chromogenes induced innate responses that reduced the growth of S. uberis (161). However, the authors did not clearly demonstrate if priming with S. chromogenes itself induced mastitis rather than enhancing protective innate immunity. Another study showed that intramammary challenge with S. chromogenes during a dry period resulted in colonization of challenged quarters by S. chromogenes, which induced high SCC, IFN-γ, and IgG2 production in challenged quarters but lower IL-6 and IL-10 in both challenged and colonized and non-colonized quarters (162). To conclude these findings as protective, it is important to determine how long the colonized quarters were shedding S. chromogenes without causing mastitis and if intramammary infusion of other bacterial mastitis pathogens into these S. chromogenes colonized quarters can prevent IMI or mastitis. Detailed controlled experimental challenge studies under in vivo conditions in dairy cows are critically needed to determine the roles of colonization of udder quarters by specific NAS species on mastitis status, milk quality, and milk production losses.
2.2.5 Therapeutic measures and antimicrobial resistance of NAS
Staphylococci are known to become resistant to several antibiotics, including methicillin resistance, which is important for public health (163, 164). Methicillin-resistant Staphylococcus aureus (MRSA) infection can only be treated with limited antibiotics and needs long-term treatment (163, 165–167). MRSA infection is zoonotic (168), and continuous antimicrobial susceptibility surveillance is very crucial to control the transmission of this strain from animal production to humans and vice versa (169). They may transfer resistance traits to S. aureus or other bacteria, resulting in the emergence of multidrug-resistant strains (94, 135). The prevalence of infection by these groups of bacteria is on the rise mainly due to the spread of drug resistance among these groups (135). The most frequently seen resistance among staphylococci is resistance due to the production of β-lactamases, with more common production among subclinical non-aureus staphylococci isolates than clinical isolates (170). They exhibit resistance to multiple classes of antimicrobial drugs (32, 171, 172). The response to the treatment of S. aureus mastitis during lactation is poor (30, 173–175), with a 25–75% quarter cure rate for treatment at dry-off and 3–63% for short-term treatment during lactation (174, 175).
A recent antimicrobial susceptibility study involving S. aureus and NAS from bovine mastitic milk samples in Finland showed the presence of the blaZ gene and penicillin resistance of 9.3% in S. aureus and 28.9% in all NAS (176). The proportion of penicillin-resistant isolates was highest in S. epidermidis and lowest in S. simulans. The S. epidermidis is the predominant species carrying the mecA gene. Some phenotypically penicillin-susceptible staphylococci have the blaZ gene, but isolates negative for blaZ or mec rarely manifest resistance, indicating that genotypic AMR testing (176) may be good for the choice of antimicrobial drug for treatment. Another study from Switzerland to determine intramammary microbiome and resistome from the milk of healthy dairy cows reported a high prevalence of resistance to clindamycin and oxacillin (65 and 30%, respectively) in S. xylosus but not associated with chromosomal or plasmid-borne ARGs (177). The authors found that most resistance was justified by the presence of mobile genetic elements such as tetK-positive plasmids.
2.3 Universal staphylococcal virulence regulators
Staphylococci are opportunistic commensal bacteria (23, 178) that can cause different diseases such as superficial skin infections, endocarditis, osteomyelitis, necrotizing fasciitis in humans (179) and mastitis, necrotizing endometritis, pyometra, exudative epidermitis, cystitis, and otitis in animals (30, 180). However, it is important to emphasize that the virulence factors of human-adapted and bovine-adapted strains may differ. Nevertheless, understanding similarities and differences between bovine-adapted strains and human-adapted strains at cellular and molecular (genomic, transcriptomic, proteomic, and metabolomic) levels is critically important to control infection caused by Staphylococcus. To inhabit or colonize different hostile microenvironmental niches, such as in the host body, S. aureus regulates the expression of its different virulence genes (181). The function of these different virulence factors can be attachment to host cells, immune evasion, nutrient breakdown, and acquisition (182, 183). The virulence factors of S. aureus and NAS are encoded from the chromosome and mobile genetic elements [e.g., phages or prophages, plasmids, pathogenicity islands (SaPIs), and staphylococcal cassette chromosome mec (SCCmec)] (182, 184). These different pathogenicity factors are controlled by universal virulence regulators (regulons), such as the two-component regulatory systems (TCS) that comprise 16 different TCS (185–187), and the DNA binding cytoplasmic proteins, such as the staphylococcal accessory regulator A (SarA) (188). Its homologs SarR, SarS, SarT, and other protein families (189–191) are essential for the pathogenesis of S. aureus infections. The TCS, such as the accessory gene regulator AC (AgrAC) (187), the S. aureus exoprotein expression locus RS (SaeRS) (192, 193), the staphylococcal respiratory regulator AB (SrrAB) (194–196), and the autolysis-regulated locus RS (ArlRS) (197–199) regulate the expression of many virulence factors at different growth phases of the staphylococci (Figure 1). Out of the 16 TCS, the WalKR (WalK-histidine kinase and WalR-response regulator) controls cell wall metabolism and is essential for the viability of S. aureus; the other 15 are not active in multiple strains (200–202). S. aureus survives in the hostile host body or environmental niches by coordinated expression of its cytoplasmic regulators (185). These include the SarA family of regulators, repressor of toxin (Rot), multiple gene regular A (MgrA) (203), alternative sigma factors (SigB and SigH), and various TCS such as AgrCA, SaeRS, SrrAB, and ArlRS.
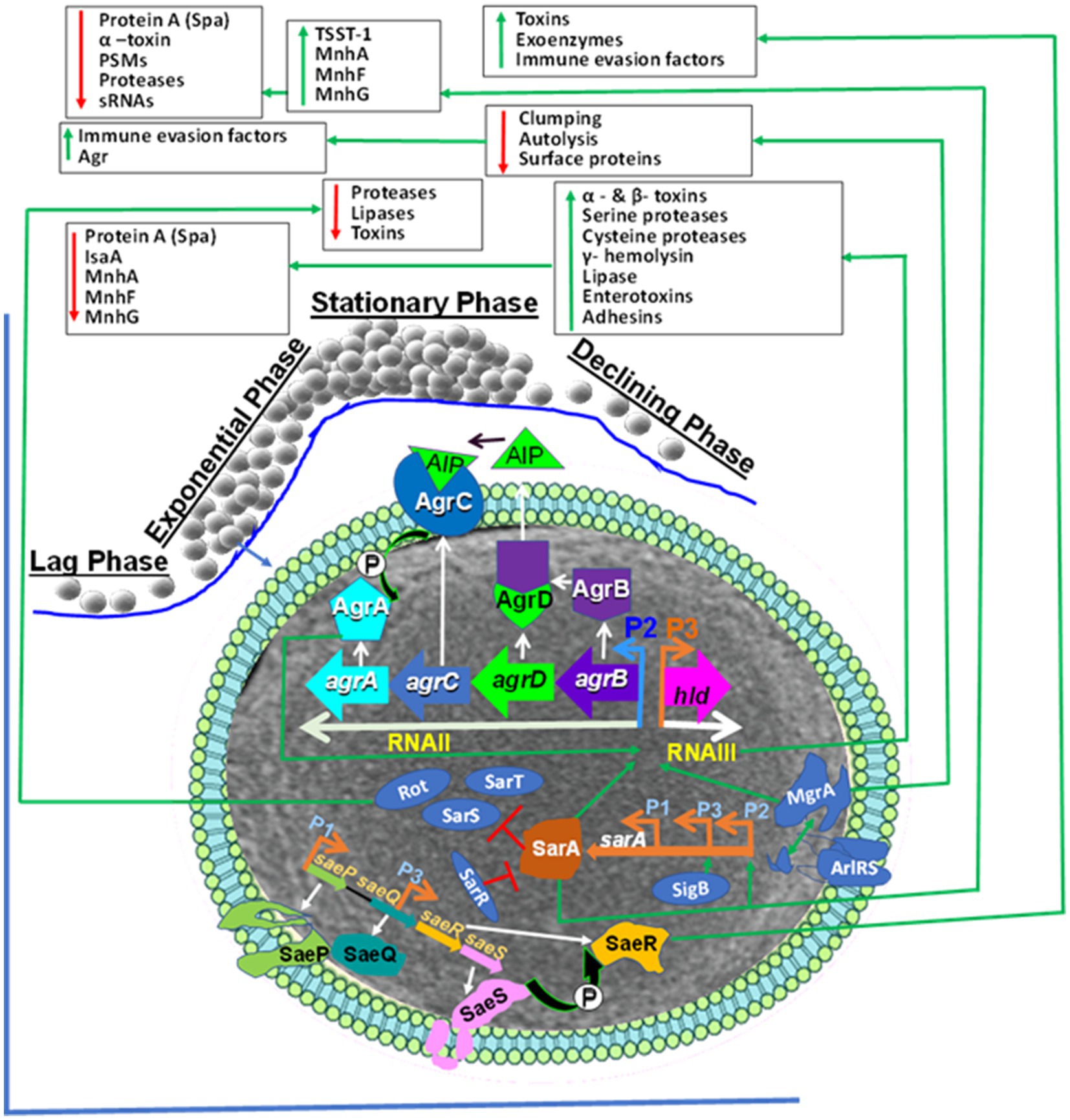
Figure 1. Staphylococcus aureus Universal Virulence Regulators. AgrA: accessory gene regulator A; AIP: Autoinducing peptide (AIP); SarA: staphylococcal accessory regulator A; SaeRS: S. aureus exoprotein expression locus RS; AgrAC: accessory gene regulator AC AgrAC; Rot: repressor of toxin; SigB: the alternative sigma factor; ArlRS: the autolysis regulated locus RS., Spa: Staphylococcal protein A, psms: phenol-soluble modulins, sRNAs: Small RNA regulators, TSST-1: toxic shock syndrome toxin-1. The S. aureus global regulators consist of the agr, ArlRS, SaeRS, and the SarA homologs (SarA, Rot and MgrA). The agr system induction causes expression of toxins and enzymes.The AIP is encoded from AgrD. The AgrD is processed to AgrB by SpsB peptidase. The extracellular AIP is detected by histidine kinase AgrC. This induced phosphorylation that transfers phosphate to AgrA that induces activation and binding to the P2 and P3 promoters inducing expression of RNAII and RNAIII, respectively. The RNAII comprises agrBDCA operon that encodes AgrB, AgrD, AgrC and AgrA. RNAIII is the major effector of the agr system through inducing target genes. Activated AgrA binds to promoters of PSMs genes and induces their expression. The SaeRS induce expression of exo-proteins. The SaeS phosphorylates its associated response regulator SaeR. This cause activation of SaeR which binds to the promoter region and induce expression of different virulence factors. The sae gene consists of saeP, saeQ, saeR and saeS that are under the control of the P1 promoter. SarA: sarA is induced from P1, P2 and P3 promoters and trigger expression of exo-proteins but represses spa. The alternative sigma factor σB (SigB) induces sarA through binding to the P3 promoter and prevents agr activity. The SarR binding to all three promoters prevents expression. SarA is an inducer of the agr system, and it represses the three SarA-like proteins SarH1, SarT and Rot. Rot regulates toxins and extracellular proteases and agr activation prevents Rot translation. MgrA: Induces expression of efflux pumps and capsule but represses surface proteins. The ArlRS induced by unknown factor and then activate MgrA but represses agr and autolysis. It down-regulates surface proteins, enabling ClfA/ClfB to interact with fibrinogen.
SarA and SaeRS act together to decrease protease production and help in biofilm formation in S. aureus (204). The sarA mutation decreases biofilm but increases sensitivity to antibiotics and the expression of alpha toxin, which is a pathogenicity factor. saeRS induces the transcription of fnbA and other S. aureus surface proteins. The saeRS mutation decreases surface proteins and biofilm formation (204) but increases efficacy with antimicrobial treatment (205). The mutation of sarA increases extracellular proteases, which decrease the ability to bind to fibronectin, therefore limiting the accumulation of surface-associated proteins. Several of these regulatory mechanisms have not been well studied in bovine-adapted strains of staphylococci. It is very important to understand the regulatory mechanisms of both human-adapted and bovine-adapted strains, their similarities and differences, and how these regulatory mechanisms change if human-adapted strains infect bovine or vice versa.
2.3.1 Staphylococcal virulence factors
A study on the presence of a total of 296 virulence factors in S. aureus from bovine mastitis found 219 were present in all isolates (85). The authors concluded that there was no association between the presence of virulence factors and clinical outcomes of infection, but the presence of virulence factors varied with clonal complexes.
The presence of virulence genes and antimicrobial resistance genes varies among S. aureus isolates from bovine mastitis. The major factor causing disease is not the presence or absence of a specific virulence factor or resistance gene in a given isolate. Instead, it is their opportunistic pathogenic ability to acquire any virulence gene or resistance gene under certain environmental pressure. However, the ability to acquire mobile genetic elements that may disseminate within or across different lineages is much more important (206). It has been shown that the SOS responses from antimicrobial drug pressure promote horizontal gene transfer of pathogenicity islands (207, 208).
S. aureus has different virulence factors (VFs) that are responsible for mastitis pathogenesis, such as adhesion and internalization into host cells, tissue damage, evasion of host immunity, and getting nutrients from the host (209, 210). However, detailed pathogenic mechanisms and effects of several VFs in mastitis pathogenesis are still poorly defined. The disease severity is influenced by the expression of virulence genes (211) of the pathogen, the immunological defense of the host, and environmental stress factors (212). However, understanding detailed mechanisms of pathogenesis and associated symptoms needs further investigation (213).
A comparative analysis of S. aureus and NAS virulence factors from clinical and subclinical bovine mastitis did not show any association between the presence of any virulence factors and the clinical outcome of mastitis (214). Similarly, a comparative genomic analysis of S. aureus from subclinical and clinical bovine mastitis did not find any association between the presence of virulence genes and the clinical outcome of mastitis (215). However, the authors found that S. aureus from clinical and subclinical mastitis were separated based on sequence variation of membrane-bound lipoprotein (215). However, another genomic study on S. aureus from clinical and subclinical mastitis reported an association of multiple genes with the clinical outcome of mastitis (216), but these genes were clustered in the same clonal complex (CC). Some authors suggest that a combination of certain virulence genes appears to cause mastitis than any single virulence gene (213). One study reported some level of differences in the virulence genes of S. aureus isolates from subclinical and gangrenous mastitis in sheep (217).
A study on the presence of known virulence genes and their regulation in S. aureus isolates from bovine mastitis found that all isolates were in Agr I and II classes, but sarT and sarU were lacking in some isolates. On the other hand, sarB and sarD were absent from all isolates. Most of the regulatory genes were present in all bovine isolates. The rot gene coding for the transcriptional regulator was present in all bovine isolates (85). The authors reported that toxins were variably present in S. aureus from bovine mastitis (85). Another study reported the presence of all hemolysin genes in S. aureus from bovine mastitis (85), and all were negative for the chemotaxis inhibitory protein of S. aureus (CHIPS) but were positive for the staphylococcal complement inhibitor gene (scn) (85). It has been shown that the presence of genes coding for cell-wall-anchored proteins such as sasC, sasD, sasF, sash, sasG, and sasK varies among bovine isolates but sasB and bap gene was absent from all isolates (85, 218).
Intracellular invasion and infection were possibly mediated by the cysteine proteases SspB and SspC, which were evident in all the isolates (219). Proteins associated with bovine immune invasions, such as Sbi, Cap, and AdsA, were identified in the isolates. All the isolates demonstrated crucial virulence characteristics, including hemolysis induction and biofilm formation (220). Some isolates were positive for agr and sarA systems associated with quorum sensing (221). All isolates were positive for intercellular adhesion, such as icaA, icaB, icaC, icaD, and icaR (220). Some isolates were positive for the spa gene (222). All isolates were positive for Ssp serine protease, which is responsible for in vivo multiplication and intracellular survival (223). The majority of the isolates were positive for the second immunoglobulin-binding protein (Sbi), which is responsible for immune evasion (224). All the isolates were positive for serotype eight capsular polysaccharide (Cap) and adenosine synthase A (AdsA), which are responsible for bovine immune evasion. All isolates were also positive for cysteine proteases (staphopain B [SspB] and staphopain C [SspC]), which enable biofilm production and intracellular colonization of S. aureus (219, 225).
Staphylococcus aureus and NAS virulence factors can be divided into two groups: (1) non-secretory or cell wall-associated structural parts and (2) secretory parts.
2.3.2 Non-secretory virulence factors
These are surface proteins associated with the peptidoglycan cell wall that help to colonize host tissues (226) during staphylococcal pathogenesis. Additionally, non-secretory surface proteins are involved in evading host immune responses, invading host cells and tissues, and forming physical barriers such as biofilms.
Staphylococcal protein A (SpA) is present in the cell walls of S. aureus and NAS. It binds Fcγ domains of the IgG and prevents the immunoglobulin-mediated removal of S. aureus from the body (227). It also binds the Fab of IgM cross-linking B-cell receptors, which leads to the programmed death of B lymphocytes (228). Consequently, immunoglobulins cannot effectively clear S. aureus infection due to the effects of protein A (229).
2.3.2.1 Biofilm formation
A biofilm is an extracellular matrix composed of exopolysaccharides, surface proteins, and nucleic acids (230, 231) that protect bacteria against host immunity and antimicrobial drugs (232–234). Biofilms bind to the host tissue surfaces by polysaccharide intercellular adhesin (235). Proteases promote the detachment of attached bacteria and increase entry into intracellular areas or invasion (236). The biofilm formation by S. aureus may enhance their colonization of the mammary gland and protection from host phagocytic cells (237, 238), resulting in chronic mastitis (239–242). However, the role of biofilm formation in mastitis pathogenesis remains unresolved and needs detailed in vivo study.
A previous study on 90 NAS found that barring a few (3.3%), the majority (96.7%) of them had some ability to form a biofilm (243). Other studies also found that 90% of NAS were positive for biofilm, and at least 11 species were identified in each study (244–247).
Staphylococci form biofilm through different mechanisms (235) that vary with species and the microenvironmental niche (237). Some of the mechanisms include the production of polysaccharide intercellular adhesin (PIA), surface proteins including biofilm-associated protein (Bap) (230, 248), slime, teichoic acids, and extracellular DNA (eDNA) (249–251).
The intercellular adhesin (ica) operon encodes different proteins (IcaA, IcaB, IcaC, IcaD, and IcaR) (235, 252, 253). Each of these proteins has a different function; for example, IcaR controls the ica operon, the induction of icaA and icaD at the same time promotes slime formation, and icaC encodes receptor protein (249, 250, 254). The presence or absence of these different ica genes in this operon also varies with strains. A previous study found that approximately 24.1 and 21.4% of NAS isolates were positive for the icaA and icaD genes, respectively (255), whereas all S. aureus isolates (100%) were positive for the icaD gene (255). The majority (73.2%) of NAS were positive for icaA and icaD genes (256). However, the majority (81.7%) of the icaA and icaD positive NAS were negative for the bap gene (256). Contrary to S. aureus, despite being negative for icaA and icaD genes, NAS species form a biofilm, indicating that these genes are not always essential for phenotypic mechanisms (256).
Slime is an exopolysaccharide layer or extracapsular layer of some biofilm that increases adhesion to host cells and protects bacteria from opsonophagocytic killing and the effect of antibiotics but is not found on all biofilms (257, 258). The formation of biofilm/slime depends on the strain. A study on staphylococci reported that 80% of S. aureus produced slime and formed strong biofilms (255), whereas approximately 87 and 84.2% of NAS with and without slime formation, respectively, produced strong biofilms (255).
Biofilm-associated protein is a high-molecular-weight surface protein responsible for cellular aggregation and biofilm formation in staphylococci (259, 260). Staphylococcus aureus from cases of bovine mastitis may carry ica and bap genes, be positive for the ica gene but negative for the bap gene, or be negative for both (261). A previous study (261) showed that bap-positive S. aureus was more able to cause IMI and less susceptible to antibiotics if it produced biofilm in vitro, which may show the enhancing ability of Bap and associated chronic S. aureus IMI.
An evaluation of the link between the presence of ica locus genes, slime formation, and the presence of Bap protein with biofilm formation did not show a consistent association of biofilm formation with any of these factors. A study on S. aureus from cases of bovine mastitis showed that all isolates tested carry icaA and icaD genes (262, 263), most of which were slime producers (262). The presence of bap, icaA, and icaD was linked with biofilm synthesis. However, most S. aureus isolates negative for these genes were biofilm formers (264). Similarly, all slime-positive ones could not form biofilm in vitro (262). Therefore, the presence of ica genes is linked with biofilm; however, ica genes are not mandatory for biofilm production since some ica-negative S. aureus can produce biofilm using different mechanisms (265, 266).
2.3.2.2 Role of biofilms in the pathogenesis of bovine mastitis
The role of biofilms in bovine mastitis is still unclear. Most studies on the role of biofilm in bovine mastitis were focused on the characterization of the biofilm-forming capability of different bacterial mastitis pathogens in vitro using different methods (microtiter plates with crystal violet staining for bacterial biomass quantification, Congo red Agar test, and standard tube method for biofilm formation assay) (116). The majority of S. aureus isolates from cases of mastitis form biofilm in vitro, but that may not be the case under in vivo conditions. The physiological characteristics of biofilm formation in vitro are different from in vivo, as also seen with P. aeruginosa during human infections (267). The role of biofilm in human infections is well known since the finding of bacterial aggregates in the lungs of cystic fibrosis patients (268) in 1977 and the first report of a medical biofilm causing recurrent infection in 1982 (269). Despite these findings in human medicine, most studies focus on in vitro characterization in veterinary medicine. In human medicine, biofilm is responsible for several diseases ranging from wound infections to lung infections, osteomyelitis, urinary tract infections, dental plaque, and endocarditis (270).
In vivo, there are interactions among bacteria, host immune response, and antimicrobial drugs administered for treatment, which is not the case under in vitro conditions. Therefore, more in vivo studies on dairy cows are required to determine the role of biofilm in the pathogenesis of S. aureus and NAS mastitis. Only two studies have reported biofilm formation inside the mammary glands of dairy cows with mastitis (271, 272). One reported the clustering of S. aureus bacteria in the alveolar lumen and lactiferous ducts of mammary glands of experimentally challenged cows using microscopy (271). The second study reported the presence of polysaccharide intercellular adhesions (PIA) in the swabs obtained from different parts of the mammary glands of slaughtered dairy cows with S. aureus mastitis using fluorescence microscopy (272). One study found that S. aureus biofilm had less invasive ability in mammary epithelial cells compared to planktonic S. aureus cultures, and the biofilm culture triggered less cellular response than the planktonic cultures. Both planktonic and biofilm forms of culture triggered the induction of IL-6 by mammary alveolar cells, which could be an anti-inflammatory response (273). This is in line with the role of biofilm in human disease, where biofilms do not induce any specific immune responses (274) when the cell density is low to avoid detection by immunity but increase expression of the virulence factors (275) when cell density is high. However, in vitro studies showed no difference in host cell invasion between biofilm former and non-biofilm former (276, 277). The most important question is how biofilm resists host immunological responses (278). More detailed in vivo studies in dairy cows are needed to determine the role of biofilm in the pathogenesis of bovine mastitis. Currently, the most preferred diagnostic method to detect bacterial biofilms in tissue is peptide nucleic acid fluorescence in situ hybridization (PNA-FISH), which uses probes that hybridize to bacterial ribosomal RNA that can be detected by confocal laser scanning microscopy (CLSM). This is a sensitive method preferred in the research on biofilm in humans (279–283). This method can be used on mammary glands in dairy cows.
Detailed knowledge of the genotypic and phenotypic requirements of S. aureus and NAS to produce biofilm, especially in vivo, may improve our understanding of the pathogenesis of staphylococcal IMI and may allow us to develop methods to disintegrate or decrease biofilm formation or increase its removal.
2.3.2.3 Coagulase, von Willebrand factor binding protein, and staphylokinase
These staphylococcal proteins serve as cofactors to activate host zymogens (284). Coagulase (Coa) and von Willebrand factor binding protein (vWbp) interact with prothrombin, causing activation of zymogen (inactive form) that converts fibrinogen, a plasma protein produced by the liver, to fibrin. Fibrin catalyzes blood clot formation, inhibiting bacterial killing by phagocytic cells (284–286). Staphylokinase (Sak) is encoded from lysogenic phage and interacts with plasmin in serum, leading to the conversion of plasminogen to plasmin, resulting in the lysis of fibrin clots (287).
2.3.3 Staphylococcal secretory (secreted) virulence factors
Exotoxins are secreted toxins that represent approximately 10% of the total secretory product of S. aureus (288). The majority of S. aureus isolates from cases of bovine mastitis produce exotoxins such as hemolysins, nucleases, proteases, lipases, hyaluronidase, and collagenase (289). Staphylococcal exotoxins can be divided into cytotoxins and superantigens. Cytotoxins damage host cell membranes, causing target cells lysis and inflammation. Superantigens induce increased cytokine production and trigger B and T cell proliferation.
2.3.3.1 Cytotoxins or cell membrane-damaging toxins
Staphylococcal α-toxin (hemolysin-α or Hla) is a 33 kDa pore-forming toxin encoded by the hla gene from chromosome through agr system and causes membrane damage and cell lysis (290, 291). It causes the lysis of different cells (e.g., erythrocytes, platelets, endothelial cells, epithelial cells, and certain leukocytes) (292, 293). It binds to A Disintegrin and metalloproteinase domain-containing protein 10 (ADAM-10) receptors on cells that determine its species and cell type specificity (294). In mice, it causes cleavage of E-Cadherin, which is the junction protein, and the loss of the epithelial barrier (295). β-toxin (hemolysin-β or Hlb) is non-pore forming but causes hydrolysis of the sphingomyelin component of the cell membrane (leukocytes and red blood cells) (296). γ- toxin (hemolysin-γ or Hlg) is a bi-component (S [slow, HlgA or HlgC and F fast, HlgB]) pore-forming toxin encoded from core genome where F binds to phosphatidylcholine of cells, and S binds to cell membranes causing lysis (macrophages, neutrophils) and monocytes (297, 298). δ-toxin (hemolysin-δ or Hld) causes lysis of neutrophils, monocytes, and degranulation of mast cells (299). All (α, β, and γ) toxins require specific receptors, but δ-toxin does not require a specific receptor to cause cell lysis and is believed to belong to phenol soluble modulins (300).
Phenol soluble modulins (PSM) are amphipathic (both lipophilic and hydrophilic) peptides encoded from psmα and psmβ operons on the chromosome and induced by the agr system (298). The PSM causes cell death, biofilm production, and modulation of immunity (284). α- and β- hemolysins and PSM induce breaks in the cell membranes of the immune cells and trigger inflammatory reactions (301). A previous study showed that approximately 69% of hemolytic S. aureus isolates are positive for β-toxin, which may indicate its effect on virulence and pathogenicity (233). The α- and β- hemolysins enhance invasion and exacerbate the spread and transmission of infection (233). The ability to invade cells and stay in the intracellular area enhances chronic recurrent infection (235).
Leukocidins are 32–35 kDa toxins encoded on the core genome or phage (298) that cause damage to leukocytes such as macrophages, neutrophils, monocytes, and dendritic cells (227, 236, 302). LukMF is encoded by the temperate phage ΦSa1 and is present in most S. aureus isolates of bovine, ovine, and caprine mastitis cases (303, 304). It binds to the C-C chemokine receptors, also known as beta-chemokine receptors (CCR1, CCR2, and CCR5) on neutrophils and macrophages, leading to cell lysis (293, 305).
2.3.3.2 Staphylococcal superantigens
Staphylococcal superantigens bind to T cell receptor (TCR) Vβ domains on T cells with major histocompatibility complex (MHC) class II protein on antigen-presenting cells (APC) that result in activation and proliferation of T cells without antigen processing and presentation (299). T-cell superantigens are exotoxins produced by S. aureus that range between 19–30 kDa and are resistant to heat, proteolysis, and desiccation (306). There are also superantigen-like proteins, previously called staphylococcal enterotoxin-like proteins (307). However, because of their lack of emetic but strong mitogenic properties, they were renamed staphylococcal superantigens (308). They are mainly involved in immune evasion (309). They are broadly divided into staphylococcal enterotoxins (SEs), Staphylococcal enterotoxin-like superantigens (SE-ls), and toxic shock syndrome toxin-1 (TSST-1).
Enterotoxins are water-soluble, stable extracellular proteins that are resistant to heat and enzymatic degradation (310–312). Enterotoxins include SEA, SEB, SECn, SED, SEE, and SEG (313) that bind to receptors on the host cell surface and trigger a series of signaling and responses inside the cell, causing emesis (308). They are superantigens that bind to MHC-II outside antigen binding site and to T-cell receptors on CD4+ cells and trigger potent polyclonal activation of T cells and increased release of inflammation mediating cytokines that lead to shock and death (236).
The presence of enterotoxin genes and the protein production capability of NAS species are still being studied, and there is a lack of understanding of their enterotoxigenic effects (314). NAS from bovine IMI tends to have variable SE genes that are continuously being lost with proceeding generations compared to S. aureus isolates containing SE genes (315, 316).
Staphylococcal food poisoning is intoxication due to the consumption of food that contains preformed enterotoxins from staphylococci that multiply in food that is inappropriately stored or handled (317–319). The first staphylococcal food poisoning was reported in 1884 in Michigan (US) by Vaughan and Sternberg due to the ingestion of contaminated cheese (320). Staphylococcal enterotoxins are produced over different temperatures, pH, salt concentrations, and water content (321). S. aureus can be killed by heating the food, but the SE remains active and can cause food poisoning (310). Staphylococcus aureus grows well in milk and milk products, which is a main source of human infection (322).
Two major factors for S. aureus multiplication and growth are improper milk storage temperature and unhygienic handling of foodstuff (322, 323). Higher starch and protein in food, pH, water activity, and warm temperature increase enterotoxin production (322). S. aureus can survive in a pH of 4.5–7.0, a low water activity of 0.86, and a salt concentration of up to 20%, which would normally kill bacteria (324, 325). Lower pH decreases S. aureus attachment to solid surfaces, subsequently decreasing the ability to colonize and cause infection (326).
The sea gene is present in temperate bacteriophages, and when bacteriophages infect bacteria, it becomes integrated into the bacterial chromosome as a prophage and remains as part of the genome (327). Under stressful conditions of improper food preservation, the prophage gets activated, multiplies the phage genome, and produces new bacteriophages (328). To avoid the multiplication of S. aureus, milk must be refrigerated at all times, from production to consumption (310, 329). Milk should be pasteurized to kill pathogenic bacteria in milk, but pasteurization does not detoxify already produced enterotoxins (330, 331).
Milk from cows with subclinical mastitis due to NAS, if consumed, can affect human health in different ways (113, 150). Therefore, the consumption of raw milk must be discouraged, and pasteurization of milk is recommended for safety and improved shelf life (146). Even though proper pasteurization is expected to kill pathogenic bacteria, the mobile genetic elements (e.g., plasmids) mediated resistance genes in bacteria may not be destroyed by pasteurization and could transform the carrier bacteria to become viable but nonculturable (VBNC) form (332, 333). Toxins produced by NAS due to inappropriate cooling during manufacturing and post-processing contamination are resistant to extreme heating or cold and can cause foodborne intoxication (146, 150).
The roles of different virulence factors of S. aureus and NAS in the pathogenesis of mastitis in dairy cows require detailed study since most of S. aureus and NAS isolates from cases of bovine mastitis are known to carry these virulence genes, but their expression and production of proteins and their phenotypic effects or exact roles in mastitis are not well defined.
2.4 Intracellular survival of Staphylococcus aureus
S. aureus can internalize into and multiply in different types of phagocytic and non-phagocytic cells (206). Viable S. aureus has been demonstrated in macrophages from milk samples of cows with mastitis (206). S. aureus can persist in the intracellular area of immune cells of different species (334, 335). However, the detailed molecular mechanisms of how S. aureus survives in the intracellular area are not fully defined. One of the mechanisms believed to be responsible for the intracellular survival of S. aureus is the induction of the formation of autophagy, which leads to the formation of autophagosomes that cannot bind to lysosomes to form autolysosomes that destroy S. aureus (334). Autophagy is a host defense mechanism or a eukaryotic cell’s homeostatic mechanism for survival during cellular stress and for destruction and clearance of intracellular pathogens (336, 337). It has been shown that infection of bovine phagocytic cells by S. aureus induces the formation of autophagy, and the autophagosomes increase the number of viable intracellular S. aureus (334). Other studies have also shown that S. aureus could utilize autophagy to survive in cells (338, 339). Similarly, autophagy was induced in bovine mammary epithelial cells challenged by S. aureus, but the autophagic flux was obstructed, leading to an increased number of intracellular S. aureus (340). Inhibition of the formation of autophagosomes in bovine mammary epithelial cells improved the clearance of intracellular S. aureus, whereas enhancing the formation of autophagosomes with the inhibition of the degradation of the autolysosomes increased the number of S. aureus inside bovine mammary epithelial cells (340). Several pathogens have developed mechanisms to avoid or even utilize the autophagic process to persist and multiply in host cells (341). Some studies show that S. aureus internalized into intracellular areas and remains in a membrane-bound vacuole, being converted to small colony variants (SCVs) with atypical small morphology and dormant biochemical properties, enabling it to survive in intracellular areas protected from host defenses and effects of antimicrobial drugs (342, 343) in dairy cows with a history of chronic intramammary S. aureus infection (78, 344). Cytotoxic S. aureus strains internalized into epithelial cells and could exit from the phagosome into the cytosol, where they multiplied and employed staphylococcal cysteine proteases and induced host cell death (219). The authors also reported the presence of serotype eight capsular polysaccharides (Cap), adenosine synthase A (AdsA), cysteine proteases (staphopain B SspB, and staphopain C, SspC), which are responsible for biofilm production and intracellular survival (219, 225) in all isolates. S. aureus can switch its phenotypes between wild types and small colony variants and survive inside cells, causing persistent intramammary colonization leading to recurrent bovine mastitis.
3 Host defense against staphylococcal mastitis
3.1 Natural defense
3.1.1 Physical barriers
The teat canal opening is closed by the smooth muscle sphincter or Rosette of Furstenberg (345, 346) and keratin plug, a wax-like product of stratified squamous epithelial cells in the teat canal (346). Keratin contains bacteriostatic fatty acids (347) and fibrous structural proteins (348–350). Fibrous proteins are produced by stratified squamous epithelial cells in the teat canal that bind to bacteria and induce changes in the cell wall that make them prone to osmotic pressure and death (346). Fibrous proteins inhibit Streptococcus agalactiae and Staphylococcus aureus (351) and are functionally similar to bovine neutrophils (352). Keratin plug breakage (353) or interference with keratin formation due to damage by a faulty milking machine (354) increases bacterial invasion and colonization (355). After milking, the teat canal remains open for about 2 h, and during this time, bacteria can enter the intramammary area (356–358).
Despite the presence of these physical barriers (sphincter muscle and keratin plug) and bacteriostatic fatty acids and scleroproteins, S. aureus can gain access to intramammary areas and cause IMI during dry and lactation periods, as confirmed by previous studies (160, 359) or remain alive for several days after being infused a few millimeters inside the teat canal (360–362). The contaminant microorganisms from milking liners or milkers’ hands can be propelled from the open teat area into the teat cistern by fluctuating milking machine pressure, which is believed to be the major way for the spread of contagious mastitis pathogens to the proximal part of the mammary glands (363).
3.1.2 Mammary gland microbiome and long non-coding RNA (lncRNA) and microRNA (miRNA) in milk
Mastitis has long been associated with a variety of bacterial pathogens. However, approximately 10–40% of clinical mastitis cases yield “no significant growth” following routine bacteriologic culture. Current advances in sequencing technology allow the comparison of culture-negative quarters with clinical mastitis to that of clinically normal quarters (364). Recent sequencing studies have revealed that milk, once considered sterile, is actually home to a complex microbial community with great diversity (365). Normal milk hosts a diverse community of non-culturable bacteria. Several bacterial species were differentially abundant in the clinical mastitis samples compared to the control quarters. Some culture-negative clinical cases have demonstrated almost 100% abundance of some species (e.g., Mycoplasma sp.). Further investigation is needed to determine the roles of mammary gland microflora in SCC and the physiologic basis for these associations, as well as to evaluate the microbial dynamics during and following IMI. Given the increasing recognition of the complex and important role of microbiota in host health, an analysis of the microbiota under health and disease conditions would provide important information on the role of microbiota in udder health.
Long non-coding RNA (lncRNA) is a novel endogenous non-coding RNA molecule with a length of more than 200 nucleotides (nt) (366) that is involved in transcriptional and epigenetic regulation of human and animal genes (367, 368). lncRNAs are emerging as critical regulators of gene expression in the immune system (369). lncRNAs are expressed in a highly lineage-specific manner and control the differentiation and function of innate and adaptive immune cell types (369). In the body’s immune response, lncRNAs regulate the occurrence and development of various inflammatory diseases, including bovine mastitis. Wang et al. (370) identified differentially expressed lncRNAs in the mammary epithelial cells induced by E. coli and S. aureus using high-throughput sequencing. Currently, only four lncRNAs —lncRNA H19 (371–373), lncRNA TUB (370), lncRNA XIST (374), and LRRC75A-AS1 (375)—have been studied with respect to their role in bovine mastitis.
3.2 Immunity
Mammary gland infection by bacteria or fungi induces immune responses (376, 377). Two types of immunity are induced by infection: innate and adaptive (378). Both are very important for the immune-mediated control of invading pathogens in mammary glands.
3.2.1 Innate immunity
The skin, teat sphincter, and teat canal membranes serve as the first line of defense. Once the physical barriers are compromised, innate immunity gets involved. The teat canal tissue expresses toll-like receptors (TLRs) and secretes cytokines and antimicrobial peptides (379, 380). Innate immunity is divided into cellular (Leukocytes: neutrophils, macrophages, lymphocytes, and mammary epithelial cells) and humoral (Lactoferrin, transferrin, lysozyme, lactoperoxidase, and myeloperoxidase, complement systems, cytokines, chemokines, host defense peptides) components (346, 381).
3.2.1.1 Cellular
Neutrophils are the most abundant (80%) leukocytes during IMI, and they are recruited by innate immunity (382). Neutrophils are recruited to the site of infection following chemical signals (chemoattractants), which include C5a, C3a, and IL-8 from the infection site (383, 384). The production of chemoattractants can be triggered by staphylococcal lipoteichoic acid (LTA) that attracts neutrophils and monocytes to the infection sites (385). Bone marrow produces neutrophils, which enter blood circulation and circulate through blood under normal circumstances. When there is IMI, their production is increased, and they are recruited from blood circulation into the infection site following chemoattractants. At high concentrations of chemoattractants, neutrophils slow down their movements through blood by binding with their cell surface receptor to the ligand on endothelial surfaces and move out of the blood into the infection site by squeezing themselves (diapedesis) between endothelial cells (386).
Some S. aureus strains can avoid getting killed by neutrophils (387, 388) and stay inside phagocytic cells. In that case, the natural killer cells (NK) or cytotoxic T cells kill infected phagocytic cells, releasing S. aureus for another possibility of killing by phagocytic cells (389). If S. aureus is not controlled by innate immunity, adaptive immunity takes over the battle through antibodies specifically produced against S. aureus that bind to bacteria and clear them by opsonophagocytic killing of phagocytic cells. Previous studies (390–392) have demonstrated that IL-8 is the most important chemoattractant for neutrophils-based quick response. A quick and effective cellular response is required to control S. aureus IMI from developing into mastitis.
3.2.1.2 Humoral
Lactoferrin deprives the infected area of iron, leading to oxidative stress, preventing bacterial multiplication and growth, and assisting the survival of host cells (393).
The complement system is a series of proteolytic processes involving 30 plasma and cell surface proteins that lead to the production of proinflammatory mediators, opsonins, and membrane attack complexes (394). There are three complement pathways that clear invading pathogens. These include (1) classical, (2) lectin, and (3) alternative systems (395). The C3a and C5a are anaphylatoxins that induce histamine, vasodilation, and inflammation to eliminate or remove pathogens (395). The membrane attack complex (MAC) breaks holes, or pores, into the invading bacteria’s cell membranes, causing irreparable damage (396).
Antimicrobial peptides (AMPs) are small peptides of 10 to 60 amino acids that are commonly present in animals (mammals, amphibians, insects, aquatic), plants, and microorganisms with a broad spectrum of antimicrobial activity on bacteria, fungi, parasites, and viruses (397, 398). Almost all AMPS are cationic, but some are anionic (350, 398).
Antimicrobial peptides are also produced by different tissue cells, such as PMNs, macrophages, and mucosal epithelial cells. Antimicrobial peptides that are present in cattle are defensins, cathelicidins, and anionic peptides (399). Domestic animals have many cationic AMPS and a few anionic AMPS (400). Other mammalian AMPS are histatins (401) and dermcidin (402). Antimicrobial peptides kill microbes by different mechanisms, including the induction of ion channel formation (e.g., defensins) (403) and flocculation of intracellular contents (e.g., anionic peptides) (404), thereby affecting transport and energy metabolism (e.g., bactenecins) (405, 406).
β-defensins are AMPS mainly produced by polymorphonuclear cells (407–409). Lipopolysaccharide (LPS) and lipoteichoic acid (LTA) induce the production of β-defensins by mammary epithelial cells (410).
Type 3 immunity – Mastitis is usually caused by bacterial infections such as streptococci, staphylococci, and coliform bacteria, which is characterized by massive recruitment of neutrophils into mammary glands. Consequently, cell-mediated immunity, especially type 3 immunity, is the most likely intramammary defense mechanism. However, this mechanism is not well investigated. Efforts toward improving intramammary immunity against bacterial mastitis pathogens through better vaccine design that enhances type 3 immunity can be beneficial in controlling and understanding effective intramammary immunity.
Recent studies have shown that both innate and adaptive cell-mediated type 3 effector immunity have the capability to function as effectors on epithelial and mucosal surfaces (411, 412). Type 3 immunity is characterized by the recruitment of neutrophils, production of antimicrobial defenses by epithelial cells, involvement of type 3 innate lymphoid cells (ILC3s), expression of cytokines (IL-17A, IL-17F, IL-22), and transcription factors (retinoic acid-related orphan receptors γt and α -Rorγt and Rorα) (412, 413). Cells that are responsible for type 3 immunity include ILC3s, γδ T cells, CD4+ helper T cells (Th17), and CD8+ cytotoxic T cells (Tc17) (414). IL-17A-producing CD4+ cells were isolated from ruminants, and the Th17 cells were purified and cultured in vitro (258, 415, 416). The CD4 and CD8 lymphocytes with characteristic features of memory lymphocytes were detected in the milk from healthy and infected udder quarters (392, 417). The RORγt-expressing and IL-17A-producing CD4+ T cells were detected in mouse mammary glands, but CD8+ T cells expressing RORγt were not yet detected (418, 419). The innate immune system receptors [e.g., Toll-like receptors (TLR); TLR1, TLR2, TLR3, TLR4, and dectin-1] expressing T 17 cells and γδ T cells that can respond to mammary associated molecular patterns (MAMPs) were detected (420, 421). They could also secrete IL17A and IL-22 without interacting with the T-cell receptor (TCR) in the presence of IL-1β and IL-23. Bovine WC1+ γδ T cells, CD4+ (T17), and CD8+ T cells produce IL-17A (415, 416, 422, 423). In the peripheral tissues, a majority of the bovine γδ T cells are WC1- and functionally different from the WC1+ cells (420). Specific γδ T cells were shown to be recruited into milk during infection (391, 424). The ILC3 reside in the parenchymal tissues and mucosal-epithelial surfaces, where they function as effectors of cell-mediated innate immunity to protect against infection by pathogens and regulate inflammation and homeostasis (425). Bovine ILCs have not been detected yet, but human and mice ILCs have been shown to exist, and human ILCs can respond to pathogen-associated molecular patterns (PAMPs), whereas mice ILCs cannot. The ILC3 are stimulated by IL-23 and IL-1α or IL-1β and produce effectors such as IL17A, IL-17F and IL-22 (425).
3.2.2 Adaptive immunity
Adaptive (acquired) immunity is a more advanced immune system that exists in higher vertebrates (426, 427). It consists of humoral (immunoglobulin-mediated) and cellular (cell-mediated) immunity. The innate immunity creates the basis for the induction of adaptive immunity during phagocytosis, processing, and presenting of antigens of infecting staphylococci to the immune system (428). Due to this process, adaptive immunity takes approximately a week to respond to an infecting pathogen. Adaptive immunity involves antigen processing and presentation by antigen-presenting cells (APCs). An antigen can be processed and presented to the naïve T cells circulating in the body by binding to major histocompatibility molecule I (MHC-I) or (MHC-II). All nucleated body cells can process and present antigens generated in the intracellular area coupled to MHC-I molecule, but only professional antigen-presenting cells can process and present extracellular antigens coupled to MHC-II molecules. There are three types of professional antigen-presenting cells. These are macrophages, B-lymphocytes, and dendritic cells. The mature naïve T cells released from the thymus and circulating in the blood frequently exit from blood circulation into regional lymph nodes at high endothelial venules where they bind to foreign antigen attached to MHC-II by its T cell receptor (TCR) and become activated T helper cells (e.g., Th1 or Th2 or Th17). The helper T cells activate B-cells to become antibody-producing plasma cells or activate other T-cells to become cytotoxic effector cells depending on the type and location of antigen in the body (429).
To prevent the body from future attack by the same etiological agent, the adaptive immune system produces memory T cells (430) and B cells. For antigens generated in the intracellular area, the helper T cells activate CD8+ T cells to become effector cytotoxic T cells that kill infected cells. For extracellular pathogens, the T helper cells activate B-cells to become antibody-producing plasma cells. The antibody binds to the pathogen and leads to its removal by opsonophagocytic mechanism (431) or block bacterial binding to host tissue surface receptors (382).
Adaptive immunity produces antibodies or activated cytotoxic T cells that remove pathogens and memory cells (T and B cells) that keep the information about a specific pathogen for quicker response in case of future attack by the same pathogen.
4 Host-pathogen-environment interactions as risk factors for staphylococcal mastitis
There are many host, pathogen, and environmental risk factors for mastitis. The host risk factors include age/parity, lactation stage, somatic cell count, heredity, anatomical structure of the udder and teat, local defense mechanisms or immune competence, colonization with less pathogenic pathogens, and the presence of other diseases (432). Parity is one factor; a cow on its third lactation or greater is prone to developing clinical mastitis (433). An increase in the number of lactations increases the chance of exposure to mastitis pathogens and deterioration of previous infections (433). Cows are more likely to develop clinical mastitis (CM) during the first 30 days postpartum, with >50% of cases of mastitis occurring during this period than the remaining days of lactation (434). However, 80% of the CM cases occurring after 30 DIM were due to new IMI (434).
Pathogen risk factors include the type of pathogen (staphylococci), volume, genotype of the strain (74, 435–438), ability to form biofilm (439–441), formation of small colony variant (78, 343), frequency of exposure, methicillin-resistant S. aureus (MRSA) (442), attachment and internalization ability (79, 271), and resistance to antimicrobials (443, 444). The type of bacterial species affects infection duration, severity, treatment outcomes, and milk yield. More than 50% of recurring CM cases are due to the same pathogen that caused mastitis in the same animal previously (445).
The environmental and/or managemental risk factors include faulty milking machines, udder injury, hygiene, climate, nutrition, the season of the year, housing, and biosecurity measures (446). The prevalence of mastitis can be affected by post-milking teat dipping, clean and dry bedding, cleaning teat orifice with antiseptic solution before giving intramammary infusion, milking cows with CM last, good maintenance for the milking machine, preventing udder trauma, and climate. Warm and humid climates support the multiplication and growth of bacteria and the risk of IMI and mastitis (446).
Staphylococcus species vary in their ability to induce inflammatory reactions in the mammary glands, and SCC with the highest counts is usually caused by S. aureus. However, other NAS species such as S. chromogenes, S. hyicus, S. agnetis, S. simulans, and S. xylosus have also been reported to cause increased SCC similar to S. aureus (87, 119). S. simulans, S. agnetis, and S. hyicus cause robust inflammatory responses (101, 104, 105, 107). S. simulans is more resistant to phagocytic killing, whereas S. chromogenes can be easily phagocytosed and killed. S. simulans is usually isolated from the milk of cows with mastitis (101, 104–106). In field studies, S. simulans caused more clinical mastitis than others (101, 106), and experimentally-induced mastitis by S. simulans caused a stronger inflammatory response than S. epidermidis (121). Similarly, another study found that S. chromogenes originally isolated from milk with mastitis induced more inflammatory reactions than S. chromogenes from the teat apex (122). In another study, S. epidermidis and S. haemolyticus caused high SCC (123). In some studies, a slight increase above 100,000 cells/mL was reported for quarters infected with NAS (109, 124), whereas in another study, SCC varied from as low as 70,000 cells/mL to as high as 123,000 depending on the species of NAS involved (20). Some NAS species (S. agnetis, S. hyicus, S. simulans) caused clinical mastitis more frequently than others (101, 104, 105), whereas some others (e.g., S. epidermidis) caused mild inflammatory responses than S. simulans (121). Based on molecular data, S. simulans was usually isolated from milk with mastitis, but S. chromogenes can be associated with subclinical mastitis as well as skin microbiota (24, 100). Despite observed differences in the opsonophagocytic killing between S. simulans and S. chromogenes, both can usually exist in the mammary glands throughout lactation and be responsible for increased SCC (103, 126). Under controlled experimental infection (121), the majority of S. simulans induced chronic infection. S. agnetis was more phagocytosed by murine macrophages than S. simulans (125) but more resistant to killing, similar to S. simulans and S. aureus (125). S. aureus usually caused subclinical mastitis that often became chronic with a moderate increase in milk SCC. NAS occasionally caused clinical mastitis with SCC, usually ranging in the low to moderate increase, but could cause significantly increased high SCC (22).
The pathogenesis mechanisms responsible for the differences between NAS and S. aureus are still unknown and need further investigation. In some studies, S. simulans was different from other NAS in opsonophagocytic killing (125). However, other studies that used neutrophils instead of macrophages, which were recruited to the mammary gland after macrophages initiated an inflammatory response, reported significant differences in opsonophagocytic killing among S. aureus strains (447). All observed differences were not correlated with the type of mastitis (clinical or subclinical) (125). There was a difference in the opsonophagocytic killing of some NAS by murine macrophages (125). Staphylococci can resist opsonophagocytic killing by the formation of capsules and other extracellular polysaccharides (130–132). There are differences among NAS isolates in their susceptibility to opsonophagocytic killing by macrophages (125). These differences could be due to yet unknown novel virulence factors. Therefore, further investigation is required.
5 Pathogenesis of staphylococcal mastitis and clinical manifestation
S. aureus and NAS enter the intramammary area either by progressive colonization from the teat apex or propelled into the intramammary area during milking machine vacuum fluctuations (80). Staphylococcus aureus binds to the α-5β1 integrin on the mammary epithelial cell surface through fibronectin-binding proteins (FnBPs) (448). The presence of FnBP is vital for adherence, but its expression may vary with S. aureus strains (448). This initial adherence leads to actin polymerization, cytoskeleton formation, and entry of bacterium into the host cell (448).
Staphylococcal mastitis affects physical and chemical properties and microbial status in milk due to pathological changes in the udder tissue (449). These changes in milk and gland tissue are characterized by visible abnormal local inflammatory signs in milk and gland tissue or systemically in the animal body (15). Staphylococcus aureus mastitis can manifest as peracute, acute, or chronic clinical forms or subclinical forms. Subclinical S. aureus mastitis is the most common udder infection in dairy cows (213), but S. aureus is also one of the most common causes of clinical mastitis in dairy cows (450, 451). Clinical S. aureus mastitis varies from mild changes in milk to peracute gangrenous mastitis with severe systemic manifestations and death of infected cows (213). Severe cases occur occasionally in dairy cows (452–454). Severe peracute gangrenous mastitis has been reported in other species, including sheep (217), goats (455), rabbits (456), and humans (457). Clinical S. aureus mastitis is characterized by swollen, red, hot, and painful udder with total loss or reduced milk yield (359). Subclinical S. aureus mastitis does not show clinically visible abnormal inflammatory changes in the milk and/or gland tissues but reduces milk yield and quality. The occurrence of SCM is 15–40 times higher than CM (458). S. aureus mastitis is usually subclinical and chronic, with low cure rates even with antibiotic treatment (89).
Acute and peracute S. aureus mastitis is manifested by sudden onset with the swollen udder, fever, and purulent inflammation. The sudden onset during the first few days after parturition may develop into gangrene and is highly fatal. Local clinical mastitis may develop into systemic acute or peracute mastitis manifested by increased temperature, pulse, and respiratory rates, anorexia, toxemia, muscle weakness, ruminal stasis, and dehydration (459). Chronic S. aureus mastitis is manifested by high SCC, gradual inflammatory process, necrosis, fibrosis, atrophy of the udder, decrease in milk production, occasional clots in milk, and watery milk. Chronically infected cows must be culled before the infection spreads through the whole herd (215).
6 Diagnosis of staphylococcal mastitis
6.1 Clinical signs
Clinical mastitis causes damage to the blood-milk barrier in the gland epithelial lining and breaks tight junctions, causing the leakage of blood, cells, and other extracellular fluid components (460) into milk and udder tissue, resulting in visible abnormal changes in milk and mammary gland tissue as clinical signs (460). Leukocytes, especially neutrophils, are recruited to the gland to fight off infection. The fight results in dead bacteria, mammary gland cells, and tissue forming purulent inflammatory fluid or pus that are usually seen when foremilk is stripped out prior to milking. The influx of fluid and white blood cells results in a swollen gland, and the increased flow of blood to the infected area causes redness/hyperemia and increased heat on the gland tissue surface. The gland tissue becomes painful to touch due to increased pressure on local nerve fibers, and the death of milk-producing cells leads to decreased or loss of milk yield, which altogether constitute cardinal signs of inflammation or mastitis (460). Most studies consider NAS species as minor pathogens that cause only a slight increase in SCC and mild clinical mastitis (CM) (96, 142–145). However, differences among species are not well defined and understood.
6.2 Bacteriological culture
Bacteriological culture is a good method for diagnosing S. aureus IMI. However, because of the cyclical shedding of S. aureus through milk, more than two consecutive milk samples are required to increase the sensitivity of the culture result (461, 462). Individual quarter milk culture has higher sensitivity (463) than composite milk culture, but the sensitivity of bacterial culture is affected by the type of sample (individual or composite), volume, and time interval of repeated samplings. Individual quarter milk sampling at one-day intervals with 0.1 mL volume culturing separately is expected to have sensitivities of 90 to 95%, whereas individual quarter milk sampling at three or four-day intervals with 0.1 mL volume culturing separately is expected to have sensitivities of 94 to 99%. Daily individual quarter milk culturing separately provides a sensitivity of 97% and a specificity of 97 to 100%.
S. aureus in milk samples from clinical mastitis ranges between 104 and 105 CFU /mL, but only one colony needs to be positive (464). However, S. aureus and NAS can be isolated from udder quarter milk samples of dairy cows without an increase in SCC (465, 466). Subclinical and clinical mastitis cases due to NAS had 103–104 and 105–106 CFU/mL of bacterial counts, respectively (18). A milk sample containing at least 10 NAS or 1,000 CFU/mL of milk with SCC > 100,000 cells/mL is considered subclinical mastitis. Composite milk culture increases the number of false-negative results than individual quarter milk culture; however, culturing 500 μL than 10 μL increases sensitivity. Reports from different studies indicated that freezing milk samples had no effect on S. aureus count or increased count because of cell death and release of intracellular bacteria (467–469). Staphylococci are differentiated from other gram-positive cocci, especially streptococci, by positive coagulase and catalase tests. S. aureus may cause double hemolysis on blood agar characterized by an outer zone of incomplete hemolysis due to β-hemolysin with an inner zone of complete hemolysis due to α-hemolysin (470, 471), but the production of hemolysins varies with strains (471). A tube coagulase test is an important test, and S. aureus is coagulase-positive with 100% specificity within 24 h. Coagulase-positive Staphylococcus species can be differentiated by matrix-assisted laser desorption ionization time-of-flight mass spectrometry (MALDI-TOF MS) or inoculating a colony from blood agar plate with hemolysis after 24 h into S. aureus CHROMagar plates; mauve to rose colonies (470) is a positive diagnosis for S. aureus. NAS isolation and individual pure colonies can be obtained following National Mastitis Council Guidelines (472), and each pure colony is identified at the species level by MALDI-TOF MS (96, 473).
6.3 MALDI-TOF MS
The MS principle involves ionizing chemical compounds to generate charged molecules and measure their mass-to-charge ratio. Such molecular “signatures” can be used for rapid bacterial identification from isolated colonies. It can differentiate S. aureus from the other coagulase-positive Staphylococcus species (474–476). In a previous study testing 152 staphylococcal species, 99.3% were identified correctly (477). Another study found that the MALDI-TOF MS achieved 100% specificity and sensitivity when characterizing coagulase-positive and negative strains of staphylococcal species isolates (478). The limitation of MALDI-TOF MS is the lack of non-clinical isolates in the comprehensive database for comparison, for example, cases of NAS mastitis (474).
6.4 Somatic cell count
A healthy individual quarter has SCC < 100,000 cells/mL, and an individual quarter infected with minor pathogens has SCC > 100,000 cells/mL, whereas an individual quarter infected with major pathogens has SCC > 350,000 cells /mL. If composite milk (from 4 quarters of a cow) SCC < 200,000 cells/mL, milk production loss is not expected or minimal, but a few quarters may have an infection (479, 480). The bulk tank SCC threshold of ≤200,000 cells/mL of milk is used to determine high-quality milk that qualifies for premium milk sale price. The International Dairy Federation considers an SCC > 200,000 cells/mL as a case of subclinical mastitis regardless of any determination of the presence of microorganisms. In addition to monitoring SCC at bulk tank milk level to determine high milk quality that can be sold at premium prices, individual cow SCC is used to identify and treat or segregate specific subclinically infected animals to continue to ensure high milk quality and low transmission of pathogens during milking.
Different indirect testing methods can detect the presence of an inflammatory response in milk samples. These include the Sodium Lauryl Sulphate Test (SLST), California Mastitis Test (CMT) (481), White Side Test (WST), electric conductivity (EC), pH Multistix strips, detection of enzymes, Tanuchek kits, DeLaval cell Counter (DCC), flow cytometry, and Surf Field Mastitis Test (SFMT).
7 Control of staphylococcal mastitis
7.1 Management
The National Institute for Research into Dairying developed a five-point mastitis control measure in England (482), and later, these measures were adopted by the National Mastitis Council (NMC) as a five-point mastitis management program or Five-Point Plan for the Control of Contagious Mastitis (483–485). The Five-Point Plan comprises (1) post-milking teat dipping in antiseptic solutions, (2) antibiotic dry cow therapy at the end of each lactation, (3) treatment of clinical cases, (4) culling of cows with chronic mastitis, and (5) proper maintenance of the milking machine to maintain stable teat end vacuum pressure. After implementing this plan, infection rates decreased by up to 50%, and cow-to-cow transmission also decreased gradually. The NMC improved the five-point plan to a ten-point plan by adding five additional measures such as (6) setting goals for udder health, (7) keeping cows in a clean, dry, suitable environment, (8) good record keeping, (9) regular monitoring of udder health status, and (10) periodic review of the mastitis control program. Current S. aureus control measures include maintaining healthy teat condition, pre-milking teat dipping in antiseptic solution and drying, using disposable gloves during milking, keeping the milking machine in good condition, post-milking teat dipping in antiseptic solution, dry cow therapy, cull chronic cases, and milking infected cows last. Teat dipping in antiseptic solution pre- and post-milking decreased new IMI by 50 to 65% compared to control cows without dipping teats (486).
7.2 Use of antimicrobial drugs
7.2.1 Therapeutic
Prudent antimicrobial drug use and antibiotic stewardship in dairy farms are strongly recommended to reduce the development of antimicrobial resistance (AMR). The chance of cure by antibiotic treatment depends on treatment plans for cows and pathogen-related risk factors (487, 488); however, these factors are not considered during the treatment of S. aureus mastitis (30). The cure rates for subclinical S. aureus mastitis range from 4 to 92% (489, 490). The chance of cure of an infected quarter decreased when SCC increased (> 250,000 cells/mL) (491), cow aged, another quarter of the cow had IMI, hindquarter infected, and high prevalence of S. aureus IMI before drying off (30).
Management-based mastitis control measures have been developed and implemented with mild success in reducing contagious bacteria such as S. aureus and S. agalactiae (26–28) but limited success due to application disparities across mastitis management (29).
The cure rate of S. aureus mastitis with intramammary treatment during lactation or at dry-off is poor (30, 173–175) and rarely exceeds 50%. S. aureus IMI usually exists throughout the lactation period due to limited anti-microbial drug access to the S. aureus in the purulent inflammatory fluids or formation of micro-abscess and fibrosis (492–494), S. aureus formation of L-forms (495, 496) or small colony variant (78), β-lactamase production (488, 497), survival of S. aureus in the intracellular area of phagocytic cells (76, 498) and internalization into mammary epithelial cells (79, 271, 499). The selection of antibiotics for treatment based on in vitro susceptibility testing may not be effective under in vivo conditions. However, for S. aureus mastitis cases of less than 2 weeks’ time, an in vitro susceptibility test can be used as a predictor of cure, but not for chronic cases of mastitis (490). The importance of antibiotic susceptibility testing for the treatment of clinical mastitis is arguable (500), yet the majority agree that it is better to do susceptibility testing (30) than treat without testing.
Antibiotics approved for use in dairy cattle for treatment and the prevention of mastitis and other dairy cattle diseases (bovine respiratory diseases, feet infection, metritis, diarrhea, or scours) include cephalosporins, fluoroquinolones, aminoglycosides, penicillin, sulfonamides, macrolides, amphenicols, tetracyclines, and lincosamides (501, 502). In the US, there are seven approved intramammary (IMM) antimicrobial drugs (502, 503). These include lincosamides (pirlimycin) and beta-lactams which include cephapirin (first-generation cephalosporin, 1GC), ceftiofur (third-generation cephalosporins, 3GC), aminopenicillins (amoxicillin and hetacillin), penicillin G, and penicillinase-resistant penicillin (cloxacillin) (376, 502).
Clavulanic acid with amoxicillin or cloxacillin with ampicillin overcomes β-lactamase resistance, making them antimicrobial drugs of choice for intramammary formulation. The 1GC 3GC and erythromycin are effective against β-lactamases-producing staphylococci.
Antibiotic treatment of subclinical S. aureus mastitis during lactation is not economical because of the low cure rate, milk disposal during treatment, and lack of increased milk yield after treatment (30). Intramammary infusion of long-acting antibiotics at drying off (dry cow therapy) is more effective, with a 40–70% successful clearance rate (504). The cure rate of a lactating cow with antibiotic treatment depends on the length of infection, number of udder quarters infected, type of quarter (hind or front) infected, strain of S. aureus, immunity of the cow, type of antibiotic used for treatment, and length of treatment with better cure rate and longer treatment (489). Current recommendations are to use both IMM and parenteral antibiotics or treat only with IMM for 4–8 days. Penicillin G is the antibiotic of choice for penicillin-sensitive S. aureus strains. The IMM of pirlimycin is effective when administered for 8 days (505). Extended treatment with pirlimycin decreased transmission and clinical mastitis in the herd (505).
A previous study on subclinical S. aureus mastitis treatment with IMM antibiotics showed no difference between treated and untreated controls with bacterial cure rates of 65, 47, and 43% for erythromycin and penicillin, cloxacillin, and amoxicillin and cephapirin, respectively, (89). Treatment of clinical S. aureus mastitis with extended cefquinome IMM improved clinical cures from 60 to 84% but did not change bacterial cures (491). The treatment of subclinical S. aureus mastitis with simultaneous IMM of amoxicillin and intramuscular injection of procaine penicillin G achieved a cure rate of 50% (506).
Staphylococci are known to become resistant to several antibiotics, including methicillin resistance, which is important for public health (163, 164). Methicillin-resistant Staphylococcus aureus (MRSA) infection can only be treated with limited antibiotics and needs long-term treatments (163, 165–167). MRSA infection is zoonotic (168), and continuous antimicrobial susceptibility surveillance is crucial to control the transmission of this strain from animal production to humans and vice versa (169). They may transfer resistance traits to S. aureus or other bacteria, resulting in the emergence of multidrug-resistant strains (94, 135). The prevalence of infection by these groups of bacteria is on the rise mainly due to the spread of resistance to antimicrobial drugs among these groups (135). The most frequently seen resistance among staphylococci is resistance due to the production of β-lactamases, with more common production among subclinical non-aureus staphylococci isolates than clinical isolates (170). They exhibit resistance to multiple classes of antimicrobial drugs (32, 171, 172).
7.2.2 Prophylactic
In general, under an ideal dairy farming situation, cows are in lactation for about 300 days, and the dry period is about 60 days. Dairy cows are prone to IMI during the first 2 weeks of the dry period and during the transition period (507–509). The risk of IMI is high during the first 2 weeks of the dry period because of increased colonization of teat skin by bacteria due to the absence of pre- and post-milking teat dip in antiseptic solutions known to reduce bacterial colonization and IMI. During the transition period, dairy cows experience various metabolic, immunological, and physiological changes, increasing the risk of periparturient diseases (510). The high risk of IMI during the transition period is associated with parturition-inducing immunosuppressive hormones (e.g., cortisol), negative energy balance, and parturition-related stress (508).
In general, IMI during the dry period is expected to be low due to the involution and closure of the teat opening by the keratin plug in the teat canal. However, teat canal closure after drying off varies from animal to animal (511). Some bacteria may enter into the intramammary area by crossing the keratin plug or when the keratin plug is broken by intramammary infusion. Dry cow therapy (DCT) has been used as the major preventive tool for new IMI, as well as to cure IMI or subclinical mastitis established during the previous lactation (511, 512). Additional benefits of DCT include no milk disposal and treatment with antibiotics during the dry period to achieve high bacteriologic cure rates. There are two kinds of DCT. These are blanket and selective DCT. Blanket dry cow therapy (BDCT) is an IMM of long-acting antibiotic into all quarters of lactating cows on farms at drying off. The BDCT is the most common form of usage in over 90% of dairy farms in the US (513). According to the US Department of Agriculture (USDA) survey results, 85% of conventional dairy farms use BDCT (514), which is estimated to account for one-third of the total antibiotics used on conventional farms in the US (515). According to the 2013 USDA National Animal Health Monitoring System (NAHMS) survey, antibiotics used for the treatment of mastitis accounted for 85.4% of antibiotics used on US dairy farms (USDA, 2016). BDCT is of growing concern because this practice exposes healthy animals to antimicrobials, allowing for antimicrobial selection pressure on commensal and opportunistic bacteria to develop AMR.
Selective dry cow therapy (SDCT) selectively treats only quarters of an infection during drying-off. Despite decreasing antibiotic usage, SDCT is only applied in 10% of US dairy operations (501), and the risk of missing IMI exists when compared to BDCT (114, 516, 517). The concern is the increased risk of IMI could influence herd health and profitability (518, 519). SDCT needs to be evaluated in great detail before fully implementing it across dairy operations. However, with growing concern about the use of antibiotics in food animals, BDCT is being extensively reviewed and has motivated research into alternative disease control measures (520). Finding alternatives, such as effective vaccines, for preventive antibiotics use at dry-off is key in controlling mastitis and easing concerns of AMR.
Alternatives to antibiotics, such as internal teat sealants, are shown to reduce IMI during the dry period (521) and reduce new IMI after calving when used with or without antibiotics (522). Another alternative is boosting the nutritional supplement of dairy cows with diet or supplementation of feed with nutrients that boost the immune system. Well-known dietary ingredients in the dairy industry, vitamin E and selenium (Se), when fed daily, promote immune competency and reduce the duration of clinical mastitis (523, 524).
7.2.3 Antimicrobial resistance
Specific antibiotic usage data are not available from dairy farms in the US, and it is not possible to know the exact amount of antibiotics used. Information on doses, frequency, duration, and diseases treated are also not known. However, the US Food and Drug Administration (FDA) report showed that more than 16,155 kg of medically important antimicrobial drugs intended for intramammary therapy were sold in 2019 (525). A previous review showed no widespread resistance among mastitis pathogens (444). However, some studies have shown that the treatment of mastitis with antibiotics is associated with AMR and changes in the diversity of mastitis pathogens (526, 527). Similarly, other studies (528) have established a positive association between antimicrobial drug use (pirlimycin, ampicillin, erythromycin, and tetracycline) and increased resistance among gram-positive mastitis pathogens. Another previous study (529) showed higher resistance among bacterial mastitis pathogens from conventional dairy farms (ampicillin, erythromycin, penicillin, and tetracycline) than organic dairy farms, indicating antibiotics usage increases antimicrobial resistance. Yet another study (33) on S. aureus isolates from cases of mastitis in East Tennessee showed that about 34.3% were resistant to at least one of the 10 tested antimicrobial drugs. The authors also indicated an increasing trend in AMR in S. aureus for some antimicrobials (e.g., tetracycline).
S. aureus resistance to penicillin is well known. Penicillin-resistant S. aureus decreased in the US between 1994 and 2001 (530, 531), but resistance levels differ considerably across countries (532, 533) and within a country. The prevalence of penicillin-resistant S. aureus isolates from bovine mastitis in the US ranged from 30 to 70% (66, 531, 532). S. aureus resistance to macrolides ranged from 14 to 17% based on phenotypic testing (534).
A study on 121 NAS isolates from cases of bovine mastitis found that methicillin resistance was commonly observed among some (S. epidermidis and S. haemolyticus) isolates (535). Multiple NAS isolates were positive for mecA or mecC gene located on the staphylococcal cassette chromosome mec (SCCmec) (243). The mecA and its variant mecC encode for methicillin resistance. However, the use of mecA solely as a methicillin resistance marker provided false positives due to the ancestor of mecA naturally occurring in NAS (536).
A study on S. aureus from Canada identified the major facilitator superfamily (MFS) of transporters such as tet (37), NorA, and NorB efflux genes in all isolates from bovine mastitis (220). AMR genes, such as the mepA gene, code for multidrug export protein MepA and its repressor mepR. Additionally, the norA gene coding for quinolone resistance protein NorA and its regulators arlS (signal transduction histidine-protein kinase ArlS) and arlR (response regulator ArlR) were identified. Other genes detected in some isolates were tet (37) (tetracycline efflux MFS transporter), LmrS (major facilitator superfamily multidrug efflux pump), and mgrA (HTH-type transcriptional regulator MgrA, also known as NorR), which is a positive regulator for norA expression and repressor for norB and tet38. Finally, the murA (antibiotic-resistant murA transferase), glpT (antibiotic-resistant GlpT), and fosB (fosfomycin thiol transferase) were detected in some isolates (85).
7.3 Vaccines
Different vaccines were evaluated for the control of S. aureus mastitis in dairy cows (537). Vaccination with simultaneous antibiotic administration (36, 86, 538) and vaccination with autogenous vaccines (539) were shown to have some protective effects.
There is only one bacterin vaccine for S. aureus mastitis in the US, but recent efficacy studies concluded that it cannot be recommended to control S. aureus mastitis in the US because of its limited efficacy (34–36). Another bacterin vaccine is available in Europe for the control of mastitis caused by S. aureus, non-aureus staphylococci, and E. coli. Some efficacy studies with this bacterin vaccine concluded that it reduced the incidence, severity, and duration of mastitis (37–39), whereas others concluded that it did not confer a reduction in S. aureus mastitis (40–43).
In the US, based on reported efficacy results, there is no recommended vaccine for the control of S. aureus mastitis. Major obstacles to developing an effective S. aureus mastitis vaccine are the bacterial ability to survive in the intracellular area of phagocytic and non-phagocytic cells, strain variation, and variation of virulence factors and mechanisms with strain (540) that lead to different clinical symptoms in infected host (229). Further, the physiology of the mammary gland is such that the effector immunity is diluted with a large volume of milk that is removed two to three times daily (34, 541). An optimized vaccination regimen is critically required to achieve protection by an effective vaccine (542).
8 Priority research gaps that need to be addressed
Based on current literature, the following research gaps are evident and need to be addressed:
1. Staphylococcus aureus is a zoonotic bacteria that mainly causes human endovascular infections and bovine mastitis. Yet differences and similarities between human-adapted and bovine-adapted strains at cellular and molecular levels are not well defined, and further investigation and evaluation are needed to develop improved, knowledge-based control tools.
2. Non-aureus staphylococci comprise more than 50 species of diverse groups, including coagulase-negative, some coagulase-positive, and coagulase-variable staphylococci that vary in virulence, pathogenicity, and epidemiological distribution. Each species requires a focused, detailed study to understand its role in milk somatic cell count, the development of IMI and mastitis, and its contribution to normal milk microbiota and intramammary homeostasis.
3. Staphylococci are one of the major host-adapted opportunistic bacteria that live with a host for several decades and have several virulence factors. They are considered one of the intramammary microbiota in bovines, as determined by metagenomic sequencing at one time, but can cause IMI and mastitis at another time. However, there is a need for further investigation, especially from the perspective of innate and adaptive immunity, to understand the interactions between staphylococci and bovine hosts that allow them to remain opportunistic.
4. Current advances in sequencing technology allow the comparison of culture-negative quarters with clinical mastitis to that of clinically normal quarters. Sequencing studies reveal that normal milk hosts a diverse community of non-culturable bacteria. Several bacterial species were differentially abundant in the clinical mastitis samples compared to the control quarters. Some culture-negative clinical cases demonstrated almost 100% abundance of some species (e.g., Mycoplasma sp.). Further investigation is needed to determine the roles of mammary gland microflora in SCC and the physiologic basis for these associations.
5. S. aureus can internalize into and multiply in different types of phagocytic and non-phagocytic cells. In humans, S. aureus and NAS are also known to form biofilm in vivo, which is known to be responsible for infection resistance to the host’s immune response and antimicrobial drug treatment. However, the detailed molecular mechanisms of how S. aureus survives in the intracellular area of phagocytic and non-phagocytic cells and the role of biofilm in the pathogenesis of bovine mastitis need further investigation.
9 Conclusion
1. Mastitis is the most common disease of dairy cows and incurs huge economic losses in dairy farming worldwide. Bovine mastitis is an inflammation of the udder of dairy cows, usually caused by bacteria, which results in increased milk SCC and loss or reduced milk production. The most common bacterial etiology of mastitis are staphylococci, streptococci, and coliforms. Staphylococci are a major bacteria that cause mastitis and huge economic losses to dairy farms. According to recent reports, there are more than 60 valid species in the Staphylococcus genes, and each species varies in many aspects, including genetic makeup, pathogenicity, and ability to cause disease; even strains within species differ in their pathogenicity, virulence, and host adaptation. Because of these variations, each species of Staphylococcus should be considered different in its ability to cause mastitis, and appropriate control measures need to be designed based on the knowledge of each species. Current control measures for mastitis due to S. aureus and NAS are not fully effective. An improved understanding of virulence factors of dairy cows adapted strains of S. aureus and NAS, their pathogenesis, and host immunological responses is required to develop effective and sustainable non-antibiotic control tools such as vaccines, prophylactic therapy, and other innovative tools.
Author contributions
OK: Conceptualization, Writing – original draft, Writing – review & editing. JV: Writing – review & editing.
Funding
The author(s) declare that no financial support was received for the research, authorship, and/or publication of this article.
Acknowledgments
The authors thank UT Dairy Heath Research Group for their constructive comments and suggestions.
Conflict of interest
The authors declare that the research was conducted in the absence of any commercial or financial relationships that could be construed as a potential conflict of interest.
Publisher’s note
All claims expressed in this article are solely those of the authors and do not necessarily represent those of their affiliated organizations, or those of the publisher, the editors and the reviewers. Any product that may be evaluated in this article, or claim that may be made by its manufacturer, is not guaranteed or endorsed by the publisher.
References
1. Olde Riekerink, RGM, Barkema, HW, Kelton, DF, and Scholl, DT. Incidence rate of clinical mastitis on Canadian dairy farms. J Dairy Sci. (2008) 91:1366–77. doi: 10.3168/jds.2007-0757
2. Rollin, E, Dhuyvetter, KC, and Overton, MW. The cost of clinical mastitis in the first 30 days of lactation: an economic modeling tool. Prev Vet Med. (2015) 122:257–64. doi: 10.1016/j.prevetmed.2015.11.006
3. Liang, D, Arnold, LM, Stowe, CJ, Harmon, RJ, and Bewley, JM. Estimating US dairy clinical disease costs with a stochastic simulation model. J Dairy Sci. (2017) 100:1472–86. doi: 10.3168/jds.2016-11565
4. Bramley, AJ, and National Mastitis, C. NMC. (1996). Current concepts of bovine mastitis, 4th ed. Madison, WI: National Mastitis Council.
5. USDA APHIS Veterinary Services (VS), the Center for Epidemiology and Animal Health (CEAH). Prevalence of Contagious Mastitis Pathogens on US dairy operations, 2007, Info sheet, Fort Collins, CO (2008). Available at: https://www.aphis.usda.gov/animal_health/nahms/dairy/downloads/dairy07/Dairy07_is_ContMastitis_1.pdf (Accessed April 26, 2024).
6. Condas, LA, De Buck, J, Nobrega, DB, Carson, DA, Naushad, S, De Vliegher, S, et al. Prevalence of non-aureus staphylococci species causing intramammary infections in Canadian dairy herds. J Dairy Sci. (2017) 100:5592–612. doi: 10.3168/jds.2016-12478
7. De Visscher, A, Piepers, S, Haesebrouck, F, Supre, K, and De Vliegher, S. Coagulase-negative Staphylococcus species in bulk milk: prevalence, distribution, and associated subgroup- and species-specific risk factors. J Dairy Sci. (2017) 100:629–42. doi: 10.3168/jds.2016-11476
8. De Buck, J, Ha, V, Naushad, S, Nobrega, DB, Luby, C, Middleton, JR, et al. Non-aureus staphylococci and bovine udder health: current understanding and knowledge gaps. Front Vet Sci. (2021) 8:360. doi: 10.3389/fvets.2021.658031
9. Becker, K, Heilmann, C, and Peters, G. Coagulase-negative staphylococci. Clin Microbiol Rev. (2014) 27:870–926. doi: 10.1128/CMR.00109-13
10. Devriese, LA, Vancanneyt, M, Baele, M, Vaneechoutte, M, De Graef, E, Snauwaert, C, et al. Staphylococcus pseudintermedius sp. nov., a coagulase-positive species from animals. Int J Syst Evol Microbiol. (2005) 55:1569–73. doi: 10.1099/ijs.0.63413-0
11. Bannoehr, J, Ben Zakour, NL, Waller, AS, Guardabassi, L, Thoday, KL, Van Den Broek, AHM, et al. Population genetic structure of the Staphylococcus intermedius group: insights into agr diversification and the emergence of methicillin-resistant strains. J Bacteriol. (2007) 189:8685–92. doi: 10.1128/JB.01150-07
12. Sasaki, T, Kikuchi, K, Tanaka, Y, Takahashi, N, Kamata, S, and Hiramatsu, K. Reclassification of phenotypically identified Staphylococcus intermedius strains. J Clin Microbiol. (2007) 45:2770–8. doi: 10.1128/JCM.00360-07
13. Roberson, JR, Fox, LK, Hancock, DD, Gay, JM, and Besser, TE. Prevalence of coagulase-positive staphylococci, other than Staphylococcus aureus, in bovine mastitis. Am J Vet Res. (1996) 57:54–8. doi: 10.2460/ajvr.1996.57.01.54
14. Götz, F, Bannerman, T, and Schleifer, K-H. The genera Staphylococcus and Macrococcus. Springer US (2006). 5–75.
16. De La Fuente, R, Suarez, G, and Schleifer, KH. Staphylococcus aureus subsp. anaerobius subsp. nov., the causal agent of abscess disease of sheep. Int J Syst Bacteriol. (1985) 35:99–102. doi: 10.1099/00207713-35-1-99
17. Fox, LK, and Hancock, DD. Effect of segregation on prevention of intramammary infections by Staphylococcus aureus. J Dairy Sci. (1989) 72:540–4. doi: 10.3168/jds.S0022-0302(89)79138-4
18. Wald, R, Hess, C, Urbantke, V, Wittek, T, and Baumgartner, M. Characterization of Staphylococcus species isolated from bovine quarter Milk samples. Animals. (2019) 9:1–25. doi: 10.3390/ani9050200
19. Jenkins, SN, Okello, E, Rossitto, PV, Lehenbauer, TW, Champagne, J, Penedo, MCT, et al. Molecular epidemiology of coagulase-negative Staphylococcus species isolated at different lactation stages from dairy cattle in the United States. PeerJ. (2019) 7:1–25. doi: 10.7717/peerj.6749
20. Condas, LAZ, De Buck, J, Nobrega, DB, Carson, DA, Roy, JP, Keefe, GP, et al. Distribution of non-aureus staphylococci species in udder quarters with low and high somatic cell count, and clinical mastitis. J Dairy Sci. (2017) 100:5613–27. doi: 10.3168/jds.2016-12479
21. Gillespie, BE, Headrick, SI, Boonyayatra, S, and Oliver, SP. Prevalence and persistence of coagulase-negative Staphylococcus species in three dairy research herds. Vet Microbiol. (2009) 134:65–72. doi: 10.1016/j.vetmic.2008.09.007
22. Taponen, S, and Pyorala, S. Coagulase-negative staphylococci as cause of bovine mastitis- not so different from Staphylococcus aureus? Vet Microbiol. (2009) 134:29–36. doi: 10.1016/j.vetmic.2008.09.011
23. Vanderhaeghen, W, Piepers, S, Leroy, F, Van Coillie, E, Haesebrouck, F, and De Vliegher, S. Identification, typing, ecology and epidemiology of coagulase negative staphylococci associated with ruminants. Vet J. (2015) 203:44–51. doi: 10.1016/j.tvjl.2014.11.001
24. Taponen, S, Björkroth, J, and Pyörälä, S. Coagulase-negative staphylococci isolated from bovine extramammary sites and intramammary infections in a single dairy herd. J Dairy Res. (2008) 75:422–9. doi: 10.1017/S0022029908003312
25. Thorberg, BM, Danielsson-Tham, ML, Emanuelson, U, and Persson, WK. Bovine subclinical mastitis caused by different types of coagulase-negative staphylococci. J Dairy Sci. (2009) 92:4962–70. doi: 10.3168/jds.2009-2184
26. Rowe, S, Tranter, W, and Laven, R. Longitudinal study of herd udder hygiene and its association with clinical mastitis in pasture-based dairy cows. J Dairy Sci. (2021) 104:6051–60. doi: 10.3168/jds.2020-19254
27. Bekuma, A, and Galmessa, U. Review on hygienic milk products practice and occurrence of mastitis in cow’s milk. Agricul Res Technol. (2018) 18:1–11. doi: 10.19080/ARTOAJ.2018.18.556053
28. Bhakat, C, Mohammad, A, Mandal, D, Mandal, A, Rai, S, Chatterjee, A, et al. Readily usable strategies to control mastitis for production augmentation in dairy cattle: A review. Veterinary World. (2020) 13:2364–70. doi: 10.14202/vetworld.2020.2364-2370
29. Stevens, M, Piepers, S, and De Vliegher, S. The effect of mastitis management input and implementation of mastitis management on udder health, milk quality, and antimicrobial consumption in dairy herds. J Dairy Sci. (2019) 102:2401–15. doi: 10.3168/jds.2018-15237
30. Barkema, HW, Schukken, YH, and Zadoks, RN. Invited review: the role of cow, pathogen, and treatment regimen in the therapeutic success of bovine Staphylococcus aureus mastitis. J Dairy Sci. (2006) 89:1877–95. doi: 10.3168/jds.S0022-0302(06)72256-1
31. McDougall, S, Parker, KI, Heuer, C, and Compton, CW. A review of prevention and control of heifer mastitis via non-antibiotic strategies. Vet Microbiol. (2009) 134:177–85. doi: 10.1016/j.vetmic.2008.09.026
32. Sawant, AA, Gillespie, BE, and Oliver, SP. Antimicrobial susceptibility of coagulase-negative Staphylococcus species isolated from bovine milk. Vet Microbiol. (2009) 134:73–81. doi: 10.1016/j.vetmic.2008.09.006
33. Abdi, RD, Gillespie, BE, Vaughn, J, Merrill, C, Headrick, SI, Ensermu, DB, et al. Antimicrobial resistance of Staphylococcus aureus isolates from dairy cows and genetic diversity of resistant isolates. Foodborne Pathog Dis. (2018) 15:449–58. doi: 10.1089/fpd.2017.2362
34. Middleton, JR, Ma, J, Rinehart, CL, Taylor, VN, Luby, CD, and Steevens, BJ. Efficacy of different Lysigin formulations in the prevention of Staphylococcus aureus intramammary infection in dairy heifers. J Dairy Res. (2006) 73:10–9. doi: 10.1017/S0022029905001354
35. Luby, CD, Middleton, JR, Ma, J, Rinehart, CL, Bucklin, S, Kohler, C, et al. Characterization of the antibody isotype response in serum and milk of heifers vaccinated with a Staphylococcus aureus bacterin (Lysigin). J Dairy Res. (2007) 74:239–46. doi: 10.1017/S0022029907002476
36. Luby, CD, and Middleton, JR. Efficacy of vaccination and antibiotic therapy against Staphylococcus aureus mastitis in dairy cattle. Vet Rec. (2005) 157:89–90. doi: 10.1136/vr.157.3.89
37. Bradley, AJ, Breen, JE, Payne, B, White, V, and Green, MJ. An investigation of the efficacy of a polyvalent mastitis vaccine using different vaccination regimens under field conditions in the United Kingdom. J Dairy Sci. (2015) 98:1706–20. doi: 10.3168/jds.2014-8332
38. Piepers, S, Prenafeta, A, Verbeke, J, De Visscher, A, March, R, and De Vliegher, S. Immune response after an experimental intramammary challenge with killed Staphylococcus aureus in cows and heifers vaccinated and not vaccinated with Startvac, a polyvalent mastitis vaccine. J Dairy Sci. (2017) 100:769–82. doi: 10.3168/jds.2016-11269
39. Schukken, YH, Bronzo, V, Locatelli, C, Pollera, C, Rota, N, Casula, A, et al. Efficacy of vaccination on Staphylococcus aureus and coagulase-negative staphylococci intramammary infection dynamics in 2 dairy herds. J Dairy Sci. (2014) 97:5250–64. doi: 10.3168/jds.2014-8008
40. Tashakkori, N, Khoramian, B, Farhoodi Moghadam, M, Heidarpour, M, Mashayekhi, K, and Farzaneh, N. Evaluating the effectiveness of two bovine mastitis vaccines and their influences on oxidant and antioxidant capacities of milk. Trop Anim Health Prod. (2020) 52:1493–501. doi: 10.1007/s11250-019-02156-x
41. Landin, H, Mork, MJ, Larsson, M, and Waller, KP. Vaccination against Staphylococcus aureus mastitis in two Swedish dairy herds. Acta Vet Scand. (2015) 57:81. doi: 10.1186/s13028-015-0171-6
42. Freick, M, Frank, Y, Steinert, K, Hamedy, A, Passarge, O, and Sobiraj, A. Mastitis vaccination using a commercial polyvalent vaccine or a herd-specific Staphylococcus aureus vaccine. Results of a controlled field trial on a dairy farm. Tierarztl Prax Ausg G Grosstiere Nutztiere. (2016) 44:219–29. doi: 10.15653/TPG-150912
43. Prenafeta, A, March, R, Foix, A, Casals, I, and Costa, L. Study of the humoral immunological response after vaccination with a Staphylococcus aureus biofilm-embedded bacterin in dairy cows: possible role of the exopolysaccharide specific antibody production in the protection from Staphylococcus aureus induced mastitis. Vet Immunol Immunopathol. (2010) 134:208–17. doi: 10.1016/j.vetimm.2009.09.020
44. Garrity, GM, Bell, JA, and Lilburn, TG. Taxonomic outline of the prokaryotes. New York: Bergey’s manual of systematic bacteriology (2004).
45. Schleifer, K-H, and Bell, JA. “Staphylococcaceae” fam. nov. Bergey's Manual of Systematics of Archaea and Bacteria. (2015). doi: 10.1002/9781118960608.fbm00118:1-1
46. Ludwig, W, Schleifer, KH, and Whitman, WB. Bacillus class nov. editors P VosDe, GM Garrity, D Jones, NR Krieg, W Ludwig, and FA Rainey. (2nd Edn.). New York, NY: Springer. (2009).
47. Madhaiyan, M, Wirth, JS, and Saravanan, VS. Phylogenomic analyses of the Staphylococcaceae family suggest the reclassification of five species within the genus Staphylococcus as heterotypic synonyms, the promotion of five subspecies to novel species, the taxonomic reassignment of five staphylococci. Int J Syst Evol Microbiol. (2020) 70:5926–36. doi: 10.1099/ijsem.0.004498
49. Cole, K, Foster, D, Russell, JE, Golubchik, T, Llewelyn, M, Wilson, DJ, et al. Draft genome sequences of 64 type strains of 50 species and 25 subspecies of the genus Staphylococcus Rosenbach 1884. Microbiol Res Announc. (2019) 8:1–3. doi: 10.1128/MRA.00062-19
50. Shwani, A, Adkins, PRF, Ekesi, NS, Alrubaye, A, Calcutt, MJ, Middleton, JR, et al. Whole-genome comparisons of Staphylococcus agnetis isolates from cattle and chickens. Appl Environ Microbiol. (2020) 86:1–3. doi: 10.1128/AEM.00484-20
51. Naseem, MN, Turni, C, Gilbert, R, Raza, A, Allavena, R, McGowan, M, et al. Role of Staphylococcus agnetis and Staphylococcus hyicus in the pathogenesis of Buffalo Fly skin lesions in cattle. Microbiol Spectrum. (2022) 10:e00873–22. doi: 10.1128/spectrum.00873-22
52. Neely, AN, and Maley, MP. Survival of enterococci and staphylococci on hospital fabrics and plastic. J Clin Microbiol. (2000) 38:724–6. doi: 10.1128/JCM.38.2.724-726.2000
53. Wagenvoort, JHT, Sluijsmans, W, and Penders, RJR. Better environmental survival of outbreak vs. sporadic MRSA isolates. J Hosp Infect. (2000) 45:231–4. doi: 10.1053/jhin.2000.0757
54. Över, U, Tüç, Y, and Söyletir, G. Catalase-negative Staphylococcus aureus: a rare isolate of human infection. Clin Microbiol Infect. (2000) 6:681–2. doi: 10.1046/j.1469-0691.2000.00153.x
55. Becker, K, and von Eiff, C. Staphylococcus, Micrococcus, and other catalase-positive cocci In: J Versalovic, KC Carroll, G Funke, JH Jorgensen, ML Landry, and DW Warnock, editors. 10th ed. Washington, DC: ASM Press (2011)
56. Savini, V, Catavitello, C, Bianco, A, Balbinot, A, and D'Antonio, D. Epidemiology, pathogenicity and emerging resistances in Staphylococcus pasteuri: from mammals and lampreys, to man. Recent Pat Antiinfect Drug Discov. (2009) 4:123–9. doi: 10.2174/157489109788490352
57. Bjerketorp, J, Jacobsson, K, and Frykberg, L. The von Willebrand factor-binding protein (vWbp) of Staphylococcus aureus is a coagulase. FEMS Microbiol Lett. (2004) 234:309–14. doi: 10.1111/j.1574-6968.2004.tb09549.x
58. Loeb, L . The influence of certain Bacteria on the coagulation of the blood. J Med Res. (1903) 10:407–19.
59. Viana, D, Blanco, J, Tormo-Más, MÁ, Selva, L, Guinane, CM, Baselga, R, et al. Adaptation of Staphylococcus aureus to ruminant and equine hosts involves SaPI-carried variants of von Willebrand factor-binding protein. Mol Microbiol. (2010) 77:1583–94. doi: 10.1111/j.1365-2958.2010.07312.x
60. Elbir, H, Robert, C, Nguyen, TT, Gimenez, G, El Sanousi, SM, Flock, JI, et al. Staphylococcus aureus subsp. anaerobius strain ST1464 genome sequence. Stand Genomic Sci. (2013) 9:1–11. doi: 10.4056/sigs.3748294
61. Dos Santos, DC, Lange, CC, Avellar-Costa, P, Dos Santos, KRN, Brito, MAVP, and Giambiagi-Demarval, M. Staphylococcus chromogenes, a coagulase-negative Staphylococcus species that can clot plasma. J Clin Microbiol. (2016) 54:1372–5. doi: 10.1128/JCM.03139-15
62. Akineden, Ö, Hassan, AA, Schneider, E, and Usleber, E. A coagulase-negative variant of Staphylococcus aureus from bovine mastitis milk. J Dairy Res. (2011) 78:38–42. doi: 10.1017/S0022029910000774
63. Fox, LK, Besser, TE, and Jackson, SM. Evaluation of a coagulase-negative variant of Staphylococcus aureus as a cause of intramammary infections in a herd of dairy cattle. J Am Vet Med Assoc. (1996) 209:1143–6. doi: 10.2460/javma.1996.209.06.1143
64. Pyorala, S, and Taponen, S. Coagulase-negative staphylococci-emerging mastitis pathogens. Vet Microbiol. (2009) 134:3–8. doi: 10.1016/j.vetmic.2008.09.015
65. Vidlund, J, Gelalcha, BD, Swanson, S, Fahrenholz, IC, Deason, C, Downes, C, et al. Pathogenesis. Diagnosis: Control, and Prevention of Bovine Staphylococcal Mastitis. IntechOpen (2022).
66. Abdi, RD, Gillespie, BE, Ivey, S, Pighetti, GM, Almeida, RA, and Kerro, DO. Antimicrobial resistance of major bacterial pathogens from dairy cows with high somatic cell count and clinical mastitis. Animals. (2021) 11:1–14.
67. Aarestrup, FM, Dangler, CA, and Sordillo, LM. Prevalence of coagulase gene polymorphism in Staphylococcus aureus isolates causing bovine mastitis. Can J Vet Res. (1995) 59:124–8.
68. Zadoks, RN, van Leeuwen, WB, Kreft, D, Fox, LK, Barkema, HW, Schukken, YH, et al. Comparison of Staphylococcus aureus isolates from bovine and human skin, milking equipment, and bovine milk by phage typing, pulsed-field gel electrophoresis, and binary typing. J Clin Microbiol. (2002) 40:3894–902. doi: 10.1128/JCM.40.11.3894-3902.2002
69. Smith, EM, Green, LE, Medley, GF, Bird, HE, Fox, LK, Schukken, YH, et al. Multilocus sequence typing of intercontinental bovine Staphylococcus aureus isolates. J Clin Microbiol. (2005) 43:4737–43. doi: 10.1128/JCM.43.9.4737-4743.2005
70. Smith, TH, Fox, LK, and Middleton, JR. Outbreak of mastitis caused by one strain of Staphylococcus aureus in a closed dairy herd. J Am Vet Med Assoc. (1998) 212:553–6. doi: 10.2460/javma.1998.212.04.553
71. Campos, B, Pickering, AC, Rocha, LS, Aguilar, AP, Fabres-Klein, MH, De Oliveira Mendes, TA, et al. Diversity and pathogenesis of Staphylococcus aureus from bovine mastitis: current understanding and future perspectives. BMC Vet Res. (2022) 18:115. doi: 10.1186/s12917-022-03197-5
72. Zadoks, R, van Leeuwen, W, Barkema, H, Sampimon, O, Verbrugh, H, Schukken, YH, et al. Application of pulsed-field gel electrophoresis and binary typing as tools in veterinary clinical microbiology and molecular epidemiologic analysis of bovine and human Staphylococcus aureus isolates. J Clin Microbiol. (2000) 38:1931–9. doi: 10.1128/JCM.38.5.1931-1939.2000
73. Haveri, M, Taponen, S, Vuopio-Varkila, J, Salmenlinna, S, and Pyorala, S. Bacterial genotype affects the manifestation and persistence of bovine Staphylococcus aureus intramammary infection. J Clin Microbiol. (2005) 43:959–61. doi: 10.1128/JCM.43.2.959-961.2005
74. Vaughn, JM, Abdi, RD, Gillespie, BE, and Kerro, DO. Genetic diversity and virulence characteristics of Staphylococcus aureus isolates from cases of bovine mastitis. Microb Pathog. (2020) 144:104171. doi: 10.1016/j.micpath.2020.104171
75. Middleton, JR, and Fox, LK. Influence of Staphylococcus aureus strain on mammary quarter milk production. Vet Rec. (2002) 150:411–3. doi: 10.1136/vr.150.13.411
76. Mullarky, IK, Su, C, Frieze, N, Park, YH, and Sordillo, LM. Staphylococcus aureus agr genotypes with enterotoxin production capabilities can resist neutrophil bactericidal activity. Infect Immun. (2001) 69:45–51. doi: 10.1128/IAI.69.1.45-51.2001
77. Fox, LK, Zadoks, RN, and Gaskins, CT. Biofilm production by Staphylococcus aureus associated with intramammary infection. Vet Microbiol. (2005) 107:295–9. doi: 10.1016/j.vetmic.2005.02.005
78. Atalla, H, Gyles, C, Jacob, CL, Moisan, H, Malouin, F, and Mallard, B. Characterization of a Staphylococcus aureus small Colony variant (SCV) associated with persistent bovine mastitis. Foodborne Pathog Dis. (2008) 5:785–99. doi: 10.1089/fpd.2008.0110
79. Hensen, SM, Pavicic, MJ, Lohuis, JA, and Poutrel, B. Use of bovine primary mammary epithelial cells for the comparison of adherence and invasion ability of Staphylococcus aureus strains. J Dairy Sci. (2000) 83:418–29. doi: 10.3168/jds.S0022-0302(00)74898-3
80. Côté-Gravel, J, and Malouin, F. Symposium review: features of Staphylococcus aureus mastitis pathogenesis that guide vaccine development strategies. J Dairy Sci. (2019) 102:4727–40. doi: 10.3168/jds.2018-15272
81. Fitzgerald, JR . Livestock-associated Staphylococcus aureus: origin, evolution and public health threat. Trends Microbiol. (2012) 20:192–8. doi: 10.1016/j.tim.2012.01.006
82. Richardson, EJ, Bacigalupe, R, Harrison, EM, Weinert, LA, Lycett, S, Vrieling, M, et al. Gene exchange drives the ecological success of a multi-host bacterial pathogen. Nat Ecol Evol. (2018) 2:1468–78. doi: 10.1038/s41559-018-0617-0
83. Byrne, WJ, Ball, HJ, Brice, N, McCormack, R, Baker, SE, Ayling, RD, et al. Application of an indirect ELISA to milk samples to identify cows with Mycoplasma bovis mastitis. Vet Rec. (2000) 146:368–9. doi: 10.1136/vr.146.13.368
84. Naushad, S, Nobrega, DB, Naqvi, SA, Barkema, HW, and Buck, JD. Genomic analysis of bovine Staphylococcus aureus isolates from Milk to elucidate diversity and determine the distributions of antimicrobial and virulence genes and their association with mastitis. mSystems. (2020) 5:e00063–20.
85. Åvall-Jääskeläinen, S, Koort, J, Simojoki, H, and Taponen, S. Genomic analysis of Staphylococcus aureus isolates associated with Peracute non-gangrenous or gangrenous mastitis and comparison with other mastitis-associated Staphylococcus aureus isolates. Front Microbiol. (2021) 12:688819. doi: 10.3389/fmicb.2021.688819
86. Sears, PM, and McCarthy, KK. Management and treatment of staphylococcal mastitis. Vet Clin North Am Food Anim Pract. (2003) 19:171–85. doi: 10.1016/S0749-0720(02)00079-8
87. Taponen, S, Myllys, V, and Pyörälä, S. Somatic cell count in bovine quarter milk samples culture positive for various Staphylococcus species. Acta Vet Scand. (2022) 64:32. doi: 10.1186/s13028-022-00649-8
88. Woudstra, S, Wente, N, Zhang, Y, Leimbach, S, Gussmann, MK, Kirkeby, C, et al. Strain diversity and infection durations of Staphylococcus spp. and Streptococcus spp. causing intramammary infections in dairy cows. J Dairy Sci. (2023) 106:4214–31. doi: 10.3168/jds.2022-22942
89. Mammary Gland. In Constable PD, Hinchcliff KW, Done SH, Grünberg W (editors), Veterinary Medicine (11th Edition) W.B. Saunders (2017) 1904–2001.
90. Dufour, S, Dohoo, I, Barkema, H, DesCôteaux, L, Devries, T, Reyher, K, et al. Manageable risk factors associated with the lactational incidence, elimination, and prevalence of Staphylococcus aureus intramammary infections in dairy cows. J Dairy Sci. (2012) 95:1283–300. doi: 10.3168/jds.2011-4711
91. Owens, W, Nickerson, S, Boddie, R, Tomita, G, and Ray, C. Prevalence of mastitis in dairy heifers and effectiveness of antibiotic therapy. J Dairy Sci. (2001) 84:814–7. doi: 10.3168/jds.S0022-0302(01)74538-9
92. Tenhagen, BA, Köster, G, Wallmann, J, and Heuwieser, W. Prevalence of mastitis pathogens and their resistance against antimicrobial agents in dairy cows in Brandenburg, Germany. J Dairy Sci. (2006) 89:2542–51. doi: 10.3168/jds.S0022-0302(06)72330-X
93. Dalanezi, FM, Joaquim, SF, Guimarães, FF, Guerra, ST, Lopes, BC, Schmidt, EMS, et al. Influence of pathogens causing clinical mastitis on reproductive variables of dairy cows. J Dairy Sci. (2020) 103:3648–55. doi: 10.3168/jds.2019-16841
94. Ruiz-Romero, RA, and Vargas-Bello-Pérez, E. Non-aureus staphylococci and mammaliicocci as a cause of mastitis in domestic ruminants: current knowledge, advances, biomedical applications, and future perspectives – a systematic review. Vet Res Commun. (2023) 47:1067–84. doi: 10.1007/s11259-023-10090-5
95. Koop, G, De Visscher, A, Collar, CA, Bacon, DAC, Maga, EA, Murray, JD, et al. Short communication: identification of coagulase-negative Staphylococcus species from goat milk with the API staph identification test and with transfer RNA-intergenic spacer PCR combined with capillary electrophoresis. J Dairy Sci. (2012) 95:7200–5. doi: 10.3168/jds.2012-5747
96. Rosa, NM, Penati, M, Fusar-Poli, S, Addis, MF, and Tola, S. Species identification by MALDI-TOF MS and gap PCR–RFLP of non-aureus Staphylococcus, Mammaliicoccus, and Streptococcus spp. associated with sheep and goat mastitis. Vet Res. (2022) 53:84. doi: 10.1186/s13567-022-01102-4
97. Ruiz-Romero, A, Martínez-Gómez, D, Cervantes-Olivares, RA, Díaz-Aparicio, E, and Ducoing-Watty, AE. Evaluation of pro- and anti-inflammatory interleukins in the mammary gland of goats experimentally infected with Staphylococcus chromogenes. Pol J Vet Sci. (2020) 23:511–9. doi: 10.24425/pjvs.2020.134700
98. Traversari, J, van den Borne, BHP, Dolder, C, Thomann, A, Perreten, V, and Bodmer, M. Non-aureus staphylococci species in the Teat Canal and Milk in four commercial Swiss dairy herds. Front Vet Sci. (2019) 6:00186. doi: 10.3389/fvets.2019.00186
99. De Vliegher, S, Laevens, H, Devriese, LA, Opsomer, G, Leroy, JL, Barkema, HW, et al. Prepartum teat apex colonization with Staphylococcus chromogenes in dairy heifers is associated with low somatic cell count in early lactation. Vet Microbiol. (2003) 92:245–52. doi: 10.1016/S0378-1135(02)00363-2
100. White, DG, Harmon, RJ, Matos, JE, and Langlois, BE. Isolation and identification of coagulase-negative Staphylococcus species from bovine body sites and streak canals of nulliparous heifers. J Dairy Sci. (1989) 72:1886–92. doi: 10.3168/jds.S0022-0302(89)79307-3
101. Taponen, S, Simojoki, H, Haveri, M, Larsen, HD, and Pyörälä, S. Clinical characteristics and persistence of bovine mastitis caused by different species of coagulase-negative staphylococci identified with API or AFLP. Vet Microbiol. (2006) 115:199–207. doi: 10.1016/j.vetmic.2006.02.001
102. Rajala-Schultz, PJ, Smith, KL, Hogan, JS, and Love, BC. Antimicrobial susceptibility of mastitis pathogens from first lactation and older cows. Vet Microbiol. (2004) 102:33–42. doi: 10.1016/j.vetmic.2004.04.010
103. Mørk, T, Jørgensen, HJ, Sunde, M, Kvitle, B, Sviland, S, Waage, S, et al. Persistence of staphylococcal species and genotypes in the bovine udder. Vet Microbiol. (2012) 159:171–80. doi: 10.1016/j.vetmic.2012.03.034
104. Waage, S, Mork, T, Roros, A, Aasland, D, Hunshamar, A, and Odegaard, SA. Bacteria associated with clinical mastitis in dairy heifers. J Dairy Sci. (1999) 82:712–9. doi: 10.3168/jds.S0022-0302(99)75288-4
105. Myllys, V . Staphylococci in heifer mastitis before and after parturition. J Dairy Res. (1995) 62:51–60. doi: 10.1017/S0022029900033665
106. Jarp, J . Classification of coagulase-negative staphylococci isolated from bovine clinical and subclinical mastitis. Vet Microbiol. (1991) 27:151–8. doi: 10.1016/0378-1135(91)90006-2
107. Taponen, S, Supré, K, Piessens, V, Van Coillie, E, De Vliegher, S, and Koort, JMK. Staphylococcus agnetis sp. nov., a coagulase-variable species from bovine subclinical and mild clinical mastitis. Int J Syst Evol Microbiol. (2012) 62:61–5. doi: 10.1099/ijs.0.028365-0
108. Brown, MM, and Horswill, AR. Staphylococcus epidermidis—skin friend or foe? PLoS Pathog. (2020) 16:e1009026. doi: 10.1371/journal.ppat.1009026
109. Wuytack, A, De Visscher, A, Piepers, S, Boyen, F, Haesebrouck, F, and De Vliegher, S. Distribution of non-aureus staphylococci from quarter milk, teat apices, and rectal feces of dairy cows, and their virulence potential. J Dairy Sci. (2020) 103:10658–75. doi: 10.3168/jds.2020-18265
110. Adkins, PRF, Placheta, LM, Borchers, MR, Bewley, JM, and Middleton, JR. Distribution of staphylococcal and mammaliicoccal species from compost-bedded pack or sand-bedded freestall dairy farms. J Dairy Sci. (2022) 105:6261–70. doi: 10.3168/jds.2021-21500
111. Naqvi, SA, De Buck, J, Dufour, S, and Barkema, HW. Udder health in Canadian dairy heifers during early lactation. J Dairy Sci. (2018) 101:3233–47. doi: 10.3168/jds.2017-13579
112. Adkins, PRF, Dufour, S, Spain, JN, Calcutt, MJ, Reilly, TJ, Stewart, GC, et al. Cross-sectional study to identify staphylococcal species isolated from teat and inguinal skin of different-aged dairy heifers. J Dairy Sci. (2018) 101:3213–25. doi: 10.3168/jds.2017-13974
113. El-Jakee, JK, Aref, NE, Gomaa, A, El-Hariri, MD, Galal, HM, Omar, SA, et al. Emerging of coagulase negative staphylococci as a cause of mastitis in dairy animals: an environmental hazard. Int J Vet Sci Med. (2013) 1:74–8. doi: 10.1016/j.ijvsm.2013.05.006
114. Cameron, M, McKenna, SL, Macdonald, KA, Dohoo, IR, Roy, JP, and Keefe, GP. Evaluation of selective dry cow treatment following on-farm culture: risk of postcalving intramammary infection and clinical mastitis in the subsequent lactation. J Dairy Sci. (2014) 97:270–84. doi: 10.3168/jds.2013-7060
115. Vakkamaki, J, Taponen, S, Heikkila, AM, and Pyorala, S. Bacteriological etiology and treatment of mastitis in Finnish dairy herds. Acta Vet Scand. (2017) 59:33. doi: 10.1186/s13028-017-0301-4
116. Pedersen, RR, Krömker, V, Bjarnsholt, T, Dahl-Pedersen, K, Buhl, R, and Jørgensen, E. Biofilm research in bovine mastitis. Front Vet Sci. (2021) 8:8. doi: 10.3389/fvets.2021.656810
117. Silva, V, Correia, E, Pereira, JE, González-Machado, C, Capita, R, Alonso-Calleja, C, et al. Exploring the biofilm formation capacity in S. Pseudintermedius and coagulase-negative staphylococci species. Pathogens. (2022) 11:1–16. doi: 10.3390/pathogens11060689
118. Mosser, DM, and Edwards, JP. Exploring the full spectrum of macrophage activation. Nat Rev Immunol. (2008) 8:958–69. doi: 10.1038/nri2448
119. Supré, K, Haesebrouck, F, Zadoks, RN, Vaneechoutte, M, Piepers, S, and De Vliegher, S. Some coagulase-negative Staphylococcus species affect udder health more than others. J Dairy Sci. (2011) 94:2329–40. doi: 10.3168/jds.2010-3741
120. Bramley, A, Cullor, J, Erskine, RJ, Fox, L, Harmon, R, Hogan, J, et al. Current concepts of bovine mastitis. Verona: National Mastitis Council Publications (2003).
121. Simojoki, H, Salomaki, T, Taponen, S, Iivanainen, A, and Pyorala, S. Innate immune response in experimentally induced bovine intramammary infection with Staphylococcus simulans and S. epidermidis. Vet Res. (2011) 42:49. doi: 10.1186/1297-9716-42-49
122. Piccart, K, Verbeke, J, De Visscher, A, Piepers, S, Haesebrouck, F, and De Vliegher, S. Local host response following an intramammary challenge with Staphylococcus fleurettii and different strains of Staphylococcus chromogenes in dairy heifers. Vet Res. (2016) 47:56. doi: 10.1186/s13567-016-0338-9
123. Nyman, AK, Fasth, C, and Waller, KP. Intramammary infections with different non-aureus staphylococci in dairy cows. J Dairy Sci. (2018) 101:1403–18. doi: 10.3168/jds.2017-13467
124. Valckenier, D, Piepers, S, De Visscher, A, and De Vliegher, S. The effect of intramammary infection in early lactation with non-aureus staphylococci in general and Staphylococcus chromogenes specifically on quarter milk somatic cell count and quarter milk yield. J Dairy Sci. (2020) 103:768–82. doi: 10.3168/jds.2019-16818
125. Åvall-Jääskeläinen, S, Koort, J, Simojoki, H, and Taponen, S. Bovine-associated CNS species resist phagocytosis differently. BMC Vet Res. (2013) 9:227. doi: 10.1186/1746-6148-9-227
126. Taponen, S, Koort, J, Bjorkroth, J, Saloniemi, H, and Pyorala, S. Bovine intramammary infections caused by coagulase-negative staphylococci may persist throughout lactation according to amplified fragment length polymorphism-based analysis. J Dairy Sci. (2007) 90:3301–7. doi: 10.3168/jds.2006-860
127. Souza, RM, Souza, FN, Batista, CF, Piepers, S, De Visscher, A, Santos, KR, et al. Distinct behavior of bovine-associated staphylococci species in their ability to resist phagocytosis and trigger respiratory burst activity by blood and milk polymorphonuclear leukocytes in dairy cows. J Dairy Sci. (2022) 105:1625–37. doi: 10.3168/jds.2021-20953
128. Kawecka-Grochocka, E, Zalewska, M, Rzewuska, M, Kościuczuk, E, Ząbek, T, Sakowski, T, et al. Expression of cytokines in dairy cattle mammary gland parenchyma during chronic staphylococcal infection. Vet Res. (2021) 52:132. doi: 10.1186/s13567-021-01003-y
129. Souza, FN, Santos, KR, Ferronatto, JA, Ramos Sanchez, EM, Toledo-Silva, B, Heinemann, MB, et al. Bovine-associated staphylococci and mammaliicocci trigger T-lymphocyte proliferative response and cytokine production differently. J Dairy Sci. (2023) 106:2772–83. doi: 10.3168/jds.2022-22529
130. Ohshima, Y, Schumacher-Perdreau, F, Peters, G, Quie, PG, and Pulverer, G. Antiphagocytic effect of the capsule of Staphylococcus simulans. Infect Immun. (1990) 58:1350–4. doi: 10.1128/iai.58.5.1350-1354.1990
131. Nanra, JS, Buitrago, SM, Crawford, S, Ng, J, Fink, PS, Hawkins, J, et al. Capsular polysaccharides are an important immune evasion mechanism for Staphylococcus aureus. Hum Vaccin Immunother. (2013) 9:480–7. doi: 10.4161/hv.23223
132. Spiliopoulou, AI, Krevvata, MI, Kolonitsiou, F, Harris, LG, Wilkinson, TS, Davies, AP, et al. An extracellular Staphylococcus epidermidis polysaccharide: relation to polysaccharide intercellular Adhesin and its implication in phagocytosis. BMC Microbiol. (2012) 12:76. doi: 10.1186/1471-2180-12-76
133. De Visscher, A, Piepers, S, Haesebrouck, F, and De Vliegher, S. Intramammary infection with coagulase-negative staphylococci at parturition: species-specific prevalence, risk factors, and effect on udder health. J Dairy Sci. (2016) 99:6457–69. doi: 10.3168/jds.2015-10458
134. Yun, MJ, Yoon, S, and Lee, YJ. Monitoring and characteristics of major mastitis pathogens from bulk tank Milk in Korea. Animals (Basel). (2020) 10:1–11. doi: 10.3390/ani10091562
135. Persson Waller, K, Aspán, A, Nyman, A, Persson, Y, and Grönlund, AU. CNS species and antimicrobial resistance in clinical and subclinical bovine mastitis. Vet Microbiol. (2011) 152:112–6. doi: 10.1016/j.vetmic.2011.04.006
136. Vanderhaeghen, W, Piepers, S, Leroy, F, Van Coillie, E, Haesebrouck, F, and De Vliegher, S. Invited review: effect, persistence, and virulence of coagulase-negative Staphylococcus species associated with ruminant udder health. J Dairy Sci. (2014) 97:5275–93. doi: 10.3168/jds.2013-7775
137. Compton, CWR, Heuer, C, Parker, K, and McDougall, S. Epidemiology of mastitis in pasture-grazed Peripartum dairy heifers and its effects on productivity. J Dairy Sci. (2007) 90:4157–70. doi: 10.3168/jds.2006-880
138. Pearson, LJ, Williamson, JH, Turner, SA, Lacy-Hulbert, SJ, and Hillerton, JE. Peripartum infection with Streptococcus uberis but not coagulase-negative staphylococci reduced milk production in primiparous cows. J Dairy Sci. (2013) 96:158–64. doi: 10.3168/jds.2012-5508
139. Paradis, ME, Bouchard, E, Scholl, DT, Miglior, F, and Roy, JP. Effect of nonclinical Staphylococcus aureus or coagulase-negative staphylococci intramammary infection during the first month of lactation on somatic cell count and milk yield in heifers. J Dairy Sci. (2010) 93:2989–97. doi: 10.3168/jds.2009-2886
140. Gröhn, YT, Wilson, DJ, González, RN, Hertl, JA, Schulte, H, Bennett, G, et al. Effect of pathogen-specific clinical mastitis on milk yield in dairy cows. J Dairy Sci. (2004) 87:3358–74. doi: 10.3168/jds.S0022-0302(04)73472-4
141. Timms, LL, and Schultz, LH. Dynamics and significance of coagulase-negative Staphylococcal Intramammary Infections1. J Dairy Sci. (1987) 70:2648–57. doi: 10.3168/jds.S0022-0302(87)80335-1
142. Schukken, YH, Gonzalez, RN, Tikofsky, LL, Schulte, HF, Santisteban, CG, Welcome, FL, et al. CNS mastitis: nothing to worry about? Vet Microbiol. (2009) 134:9–14. doi: 10.1016/j.vetmic.2008.09.014
143. Tomazi, T, Gonçalves, JL, Barreiro, JR, Arcari, MA, and Dos Santos, MV. Bovine subclinical intramammary infection caused by coagulase-negative staphylococci increases somatic cell count but has no effect on milk yield or composition. J Dairy Sci. (2015) 98:3071–8. doi: 10.3168/jds.2014-8466
144. Valckenier, D, Piepers, S, De Visscher, A, Bruckmaier, RM, and De Vliegher, S. Effect of intramammary infection with non-aureus staphylococci in early lactation in dairy heifers on quarter somatic cell count and quarter milk yield during the first 4 months of lactation. J Dairy Sci. (2019) 102:6442–53. doi: 10.3168/jds.2018-15913
145. Valckenier, D, Piepers, S, Schukken, YH, De Visscher, A, Boyen, F, Haesebrouck, F, et al. Longitudinal study on the effects of intramammary infection with non-aureus staphylococci on udder health and milk production in dairy heifers. J Dairy Sci. (2021) 104:899–914. doi: 10.3168/jds.2020-18685
146. Heikkilä, AM, Liski, E, Pyörälä, S, and Taponen, S. Pathogen-specific production losses in bovine mastitis. J Dairy Sci. (2018) 101:9493–504. doi: 10.3168/jds.2018-14824
147. Piepers, S, Schukken, YH, Passchyn, P, and De Vliegher, S. The effect of intramammary infection with coagulase-negative staphylococci in early lactating heifers on milk yield throughout first lactation revisited. J Dairy Sci. (2013) 96:5095–105. doi: 10.3168/jds.2013-6644
148. Hogan, JS, White, DG, and Pankey, JW. Effects of teat dipping on intramammary infections by staphylococci other than Staphylococcus aureus. J Dairy Sci. (1987) 70:873–9. doi: 10.3168/jds.S0022-0302(87)80086-3
149. Fry, PR, Middleton, JR, Dufour, S, Perry, J, Scholl, D, and Dohoo, I. Association of coagulase-negative staphylococcal species, mammary quarter milk somatic cell count, and persistence of intramammary infection in dairy cattle. J Dairy Sci. (2014) 97:4876–85. doi: 10.3168/jds.2013-7657
150. Hussein, OH, Abdel Hameed, KG, and El-Malt, LM. Prevalence and public health hazards of subclinical mastitis in dairy cows. SVU-Int J Vet Sci. (2022) 5:52–64. doi: 10.21608/svu.2022.131652.1189
151. Bastos, MDCDF, Coutinho, BG, and Coelho, MLV. Lysostaphin: A Staphylococcal Bacteriolysin with potential clinical applications. Pharmaceuticals. (2010) 3:1139–61. doi: 10.3390/ph3041139
152. Chang, S-C, Kao, C-Y, Lin, L-C, Hidrosollo, JH, and Lu, J-J. Lugdunin production and activity in Staphylococcus lugdunensis isolates are associated with its genotypes. Microbiol Spectrum. (2023) 11:e01298–23. doi: 10.1128/spectrum.01298-23
153. Carson, DA, Barkema, HW, Naushad, S, and De Buck, J. Bacteriocins of non-aureus staphylococci isolated from bovine Milk. Appl Environ Microbiol. (2017) 83:1–21. doi: 10.1128/AEM.01015-17
154. dos Santos, NJ, Fagundes, PC, de Paiva Brito, MA, dos Santos, KR, and do Carmo de Freire Bastos, M. Production of bacteriocins by coagulase-negative staphylococci involved in bovine mastitis. Vet Microbiol. (2005) 106:61–71. doi: 10.1016/j.vetmic.2004.10.014
155. Isaac, P, Bohl, LP, Breser, ML, Orellano, MS, Conesa, A, Ferrero, MA, et al. Commensal coagulase-negative Staphylococcus from the udder of healthy cows inhibits biofilm formation of mastitis-related pathogens. Vet Microbiol. (2017) 207:259–66. doi: 10.1016/j.vetmic.2017.05.025
156. Toledo-Silva, B, de Souza, FN, Mertens, K, Piepers, S, Haesebrouck, F, and De Vliegher, S. Bovine-associated non-aureus staphylococci suppress Staphylococcus aureus biofilm dispersal in vitro yet not through agr regulation. Vet Res. (2021) 52:1–11. doi: 10.1186/s13567-021-00985-z
157. Stevens, M, Piepers, S, Supre, K, and De Vliegher, S. Antimicrobial consumption on dairy herds and its association with antimicrobial inhibition zone diameters of non-aureus staphylococci and Staphylococcus aureus isolated from subclinical mastitis. J Dairy Sci. (2018) 101:3311–22. doi: 10.3168/jds.2017-13365
158. De Vliegher, S, Opsomer, G, Vanrolleghem, A, Devriese, L, Sampimon, O, Sol, J, et al. In vitro growth inhibition of major mastitis pathogens by Staphylococcus chromogenes originating from teat apices of dairy heifers. Vet Microbiol. (2004) 101:215–21. doi: 10.1016/j.vetmic.2004.03.020
159. Reyher, KK, Dohoo, IR, Scholl, DT, and Keefe, GP. Evaluation of minor pathogen intramammary infection, susceptibility parameters, and somatic cell counts on the development of new intramammary infections with major mastitis pathogens. J Dairy Sci. (2012) 95:3766–80. doi: 10.3168/jds.2011-5148
160. Kerro Dego, O, Pacha, PA, Gillespie, BE, and Pighetti, GM. Experimental Staphylococcus aureus mastitis infection model by teat dipping in bacterial culture suspension in dairy cows. Animals. (2020) 10:1–12. doi: 10.3390/ani10050751
161. Vander Elst, N, Bellemans, J, Steenbrugge, J, Geeroms, C, Breyne, K, Piepers, S, et al. Priming of the murine mammary gland with Staphylococcus chromogenes IM reduces bacterial growth of Streptococcus uberis: a proof-of-concept study. Vet Res. (2023) 54:28. doi: 10.1186/s13567-023-01156-y
162. Beuckelaere, L, De Visscher, A, Souza, FN, Meyer, E, Haesebrouck, F, Piepers, S, et al. Colonization and local host response following intramammary Staphylococcus chromogenes challenge in dry cows. Vet Res. (2021) 52:137. doi: 10.1186/s13567-021-01007-8
163. Crespi, E, Pereyra, AM, Puigdevall, T, Rumi, MV, Testorelli, MF, Caggiano, N, et al. Antimicrobial resistance studies in staphylococci and streptococci isolated from cows with mastitis in Argentina. J Vet Sci. (2022) 23:e12. doi: 10.4142/jvs.21062
164. Fergestad, ME, De Visscher, A, L'Abee-Lund, T, Tchamba, CN, Mainil, JG, Thiry, D, et al. Antimicrobial resistance and virulence characteristics in 3 collections of staphylococci from bovine milk samples. J Dairy Sci. (2021) 104:10250–67. doi: 10.3168/jds.2020-19988
165. Adkins, PRF, Dufour, S, Spain, JN, Calcutt, MJ, Reilly, TJ, Stewart, GC, et al. Molecular characterization of non-aureus Staphylococcus spp. from heifer intramammary infections and body sites. J Dairy Sci. (2018) 101:5388–403. doi: 10.3168/jds.2017-13910
166. Mahato, S, Mistry, HU, Chakraborty, S, Sharma, P, Saravanan, R, and Bhandari, V. Identification of variable traits among the methicillin resistant and sensitive coagulase negative staphylococci in Milk samples from Mastitic cows in India. Front Microbiol. (2017) 8:1–7. doi: 10.3389/fmicb.2017.01446
167. Virdis, S, Scarano, C, Cossu, F, Spanu, V, Spanu, C, and De Santis, EPL. Antibiotic resistance in Staphylococcus aureus and coagulase negative staphylococci isolated from goats with subclinical mastitis. Vet Med Int. (2010) 2010:1–6. doi: 10.4061/2010/517060
168. Kizerwetter-Świda, M, Chrobak-Chmiel, D, and Rzewuska, M. Current challenges of veterinary microbiological diagnostics concerning the susceptibility of staphylococci to antibiotics. Postępy Mikrobiol. (2018) 57:270–7. doi: 10.21307/PM-2018.57.3.270
169. Persson, Y, Nyman, AK, and Gronlund-Andersson, U. Etiology and antimicrobial susceptibility of udder pathogens from cases of subclinical mastitis in dairy cows in Sweden. Acta Vet Scand. (2011) 53:36. doi: 10.1186/1751-0147-53-36
170. De Los, SR, González-Revello, Á, Majul, L, Umpiérrez, A, Aldrovandi, A, Gil, A, et al. Subclinical bovine mastitis associated with Staphylococcus spp. in eleven Uruguayan dairy farms. J Infect Develop Countries. (2022) 16:630–7. doi: 10.3855/jidc.12960
171. Gurler, H, Findik, A, and Sezener, MG. Determination of antibiotic resistance profiles and biofilm production of Staphylococcus spp. isolated from Anatolian water buffalo milk with subclinical mastitis. Pol J Vet Sci. (2023) 25:51–9. doi: 10.24425/pjvs.2022.140840
172. Ibrahim, ES, Dorgham, SM, Mansour, AS, Abdalhamed, AM, and Khalaf, DD. Genotypic characterization of mecA gene and antibiogram profile of coagulase-negative staphylococci in subclinical mastitic cows. Vet World. (2022) 15:2186–91. doi: 10.14202/vetworld.2022.2186-2191
173. Oliver, SP, Gillespie, BE, Headrick, SJ, Moorehead, H, Lunn, P, Dowlen, HH, et al. Efficacy of extended Ceftiofur Intramammary therapy for treatment of subclinical mastitis in lactating dairy cows. J Dairy Sci. (2004) 87:2393–400. doi: 10.3168/jds.S0022-0302(04)73361-5
174. Roy, JP, DesCôteaux, L, DuTremblay, D, Beaudry, F, and Elsener, J. Efficacy of a 5-day extended therapy program during lactation with cephapirin sodium in dairy cows chronically infected with Staphylococcus aureus. Can Vet J. (2009) 50:1257–62.
175. Shpigel, NY, Kass, PH, and Saran, A. A comparative randomized field trial on intramammary and intramuscular dry cow antibiotic treatment of subclinical Staphylococcus aureus mastitis in dairy cows. J Vet Med A Physiol Pathol Clin Med. (2006) 53:418–22. doi: 10.1111/j.1439-0442.2006.00848.x
176. Taponen, S, Tölli, H-T, and Rajala-Schultz, PJ. Antimicrobial susceptibility of staphylococci from bovine milk samples in routine microbiological mastitis analysis in Finland. Front Vet Sci. (2023):10.
177. Romanò, A, Ivanovic, I, Segessemann, T, Vazquez Rojo, L, Widmer, J, Egger, L, et al. Elucidation of the bovine Intramammary Bacteriome and Resistome from healthy cows of Swiss dairy farms in the Canton Tessin. Front Microbiol. (2023) 14:1–25. doi: 10.3389/fmicb.2023.1183018
178. Brown, SP, Cornforth, DM, and Mideo, N. Evolution of virulence in opportunistic pathogens: generalism, plasticity, and control. Trends Microbiol. (2012) 20:336–42. doi: 10.1016/j.tim.2012.04.005
179. Lowy, FD . Staphylococcus aureus infections. N Engl J Med. (1998) 339:520–32. doi: 10.1056/NEJM199808203390806
181. Novick, RP . Autoinduction and signal transduction in the regulation of staphylococcal virulence. Mol Microbiol. (2003) 48:1429–49. doi: 10.1046/j.1365-2958.2003.03526.x
182. Jenul, C, and Horswill, AR. Regulation of Staphylococcus aureus virulence. Microbiol Spectrum. (2019) 6:1–34. doi: 10.1128/microbiolspec.GPP3-0031-2018
183. Scali, F, Camussone, C, Calvinho, LF, Cipolla, M, and Zecconi, A. Which are important targets in development of S. aureus mastitis vaccine? Res Vet Sci. (2015) 100:88–99. doi: 10.1016/j.rvsc.2015.03.019
184. Saber, H, Jasni, AS, Tengku Jamaluddin, TZM, and Ibrahim, R. A review of Staphylococcal cassette chromosome mec (SCCmec) types in coagulase-negative staphylococci (CoNS) species. Malaysian J Med Sci. (2017) 24:7–18. doi: 10.21315/mjms2017.24.5.2
185. Somerville, GA, and Proctor, RA. At the crossroads of bacterial metabolism and virulence factor synthesis in staphylococci. Microbiol Mol Biol Rev. (2009) 73:233–48. doi: 10.1128/MMBR.00005-09
186. Villanueva, M, García, B, Valle, J, Rapún, B, De Los, R, Mozos, I, et al. Sensory deprivation in Staphylococcus aureus. Nat Commun. (2018) 9:1–12.doi: 10.1038/s41467-018-02949-y
187. Recsei, P, Kreiswirth, B, O'Reilly, M, Schlievert, P, Gruss, A, and Novick, RP. Regulation of exoprotein gene expression in Staphylococcus aureus by agr. Mol Gen Genet MGG. (1986) 202:58–61. doi: 10.1007/BF00330517
188. Bayer, MG, Heinrichs, JH, and Cheung, AL. The molecular architecture of the Sar locus in Staphylococcus aureus. J Bacteriol. (1996) 178:4563–70. doi: 10.1128/jb.178.15.4563-4570.1996
189. Manna, AC, Bayer, MG, and Cheung, AL. Transcriptional analysis of different promoters in the Sar locus in Staphylococcus aureus. J Bacteriol. (1998) 180:3828–36. doi: 10.1128/JB.180.15.3828-3836.1998
190. Schmidt, KA, Manna, AC, Gill, S, and Cheung, AL. SarT, a repressor of alpha-hemolysin in Staphylococcus aureus. Infect Immun. (2001) 69:4749–58. doi: 10.1128/IAI.69.8.4749-4758.2001
191. Manna, AC, and Cheung, AL. sarU, a sarA homolog, is repressed by SarT and regulates virulence genes in Staphylococcus aureus. Infect Immun. (2003) 71:343–53. doi: 10.1128/IAI.71.1.343-353.2003
192. Liu, Q, Yeo, WS, and Bae, T. The SaeRS two-component system of Staphylococcus aureus. Genes. (2016) 7:81. doi: 10.3390/genes7100081
193. Giraudo, AT, Raspanti, CG, Calzolari, A, and Nagel, R. Characterization of a Tn551-mutant of Staphylococcus aureus defective in the production of several exoproteins. Can J Microbiol. (1994) 40:677–81. doi: 10.1139/m94-107
194. Yarwood, JM, McCormick, JK, and Schlievert, PM. Identification of a novel two-component regulatory system that acts in global regulation of virulence factors of Staphylococcus aureus. J Bacteriol. (2001) 183:1113–23. doi: 10.1128/JB.183.4.1113-1123.2001
195. Throup, JP, Zappacosta, F, Lunsford, RD, Annan, RS, Carr, SA, Lonsdale, JT, et al. The srhSR gene pair from Staphylococcus aureus: genomic and proteomic approaches to the identification and characterization of gene function. Biochemistry. (2001) 40:10392–401. doi: 10.1021/bi0102959
196. Mashruwala, AA, and Boyd, JM. The Staphylococcus aureus SrrAB regulatory system modulates hydrogen peroxide resistance factors, which imparts protection to Aconitase during aerobic growth. PLoS One. (2017) 12:e0170283. doi: 10.1371/journal.pone.0170283
197. Fournier, B, Klier, A, and Rapoport, G. The two-component system ArlS-ArlR is a regulator of virulence gene expression in Staphylococcus aureus. Mol Microbiol. (2001) 41:247–61. doi: 10.1046/j.1365-2958.2001.02515.x
198. Fournier, B, and Hooper, DC. A new two-component regulatory system involved in adhesion, autolysis, and extracellular proteolytic activity of Staphylococcus aureus. J Bacteriol. (2000) 182:3955–64. doi: 10.1128/JB.182.14.3955-3964.2000
199. Crosby, HA, Tiwari, N, Kwiecinski, JM, Xu, Z, Dykstra, A, Jenul, C, et al. The Staphylococcus aureus ArlRS two-component system regulates virulence factor expression through MgrA. Mol Microbiol. (2020) 113:103–22. doi: 10.1111/mmi.14404
200. White, MJ, Boyd, JM, Horswill, AR, and Nauseef, WM. Phosphatidylinositol-specific phospholipase C contributes to survival of Staphylococcus aureus USA300 in human blood and neutrophils. Infect Immun. (2014) 82:1559–71. doi: 10.1128/IAI.01168-13
201. Matsuo, M, Kato, F, Oogai, Y, Kawai, T, Sugai, M, and Komatsuzawa, H. Distinct two-component systems in methicillin-resistant Staphylococcus aureus can change the susceptibility to antimicrobial agents. J Antimicrob Chemother. (2010) 65:1536–7. doi: 10.1093/jac/dkq141
202. Dubrac, S, Boneca, IG, Poupel, O, and Msadek, T. New insights into the WalK/WalR (YycG/YycF) essential signal transduction pathway reveal a major role in controlling Cell Wall metabolism and biofilm formation in Staphylococcus aureus. J Bacteriol. (2007) 189:8257–69. doi: 10.1128/JB.00645-07
203. Luong, TT, Newell, SW, and Lee, CY. Mgr, a novel global regulator in Staphylococcus aureus. J Bacteriol. (2003) 185:3703–10. doi: 10.1128/JB.185.13.3703-3710.2003
204. Mrak, LN, Zielinska, AK, Beenken, KE, Mrak, IN, Atwood, DN, Griffin, LM, et al. saeRS and sarA act synergistically to repress protease production and promote biofilm formation in Staphylococcus aureus. PLoS One. (2012) 7:e38453. doi: 10.1371/journal.pone.0038453
205. Rom, JS, Atwood, DN, Beenken, KE, Meeker, DG, Loughran, AJ, Spencer, HJ, et al. Impact of Staphylococcus aureus regulatory mutations that modulate biofilm formation in the USA300 strain LAC on virulence in a murine bacteremia model. Virulence. (2017) 8:1776–90. doi: 10.1080/21505594.2017.1373926
206. Bravo-Santano, N, Ellis, JK, Mateos, LM, Calle, Y, Keun, HC, Behrends, V, et al. Intracellular Staphylococcus aureus modulates host central carbon metabolism to activate autophagy. mSphere. (2018) 3: 1–16. doi: 10.1128/mSphere.00374-18
207. Úbeda, C, Maiques, E, Knecht, E, Lasa, Í, Novick, RP, and Penadés, JR. Antibiotic-induced SOS response promotes horizontal dissemination of pathogenicity island-encoded virulence factors in staphylococci. Mol Microbiol. (2005) 56:836–44. doi: 10.1111/j.1365-2958.2005.04584.x
208. Beaber, JW, Hochhut, B, and Waldor, MK. SOS response promotes horizontal dissemination of antibiotic resistance genes. Nature. (2004) 427:72–4. doi: 10.1038/nature02241
209. Sutra, L, and Poutrel, B. Virulence factors involved in the pathogenesis of bovine intramammary infections due to Staphylococcus aureus. J Med Microbiol. (1994) 40:79–89. doi: 10.1099/00222615-40-2-79
210. Foster, TJ, Geoghegan, JA, Ganesh, VK, and Hook, M. Adhesion, invasion and evasion: the many functions of the surface proteins of Staphylococcus aureus. Nat Rev Microbiol. (2014) 12:49–62. doi: 10.1038/nrmicro3161
211. Wolf, C, Kusch, H, Monecke, S, Albrecht, D, Holtfreter, S, von Eiff, C, et al. Genomic and proteomic characterization of Staphylococcus aureus mastitis isolates of bovine origin. Proteomics. (2011) 11:2491–502. doi: 10.1002/pmic.201000698
212. Aitken, SL, Corl, CM, and Sordillo, LM. Immunopathology of mastitis: insights into disease recognition and resolution. J Mammary Gland Biol Neoplasia. (2011) 16:291–304. doi: 10.1007/s10911-011-9230-4
213. Rainard, P, Foucras, G, Fitzgerald, JR, Watts, J, Koop, G, and Middleton, J. Knowledge gaps and research priorities in Staphylococcus aureus mastitis control. Transbound Emerg Dis. (2018) 65:149–65. doi: 10.1111/tbed.12698
214. Avall-Jaaskelainen, S, Taponen, S, Kant, R, Paulin, L, Blom, J, Palva, A, et al. Comparative genome analysis of 24 bovine-associated Staphylococcus isolates with special focus on the putative virulence genes. PeerJ. (2018) 6:e4560. doi: 10.7717/peerj.4560
215. Rocha, LS, Silva, DM, Silva, MP, Vidigal, PMP, Silva, JCF, Guerra, ST, et al. Comparative genomics of Staphylococcus aureus associated with subclinical and clinical bovine mastitis. PLoS One. (2019) 14:e0220804-e. doi: 10.1371/journal.pone.0220804
216. Hoekstra, J, Zomer, AL, Rutten, VPMG, Benedictus, L, Stegeman, A, Spaninks, MP, et al. Genomic analysis of European bovine Staphylococcus aureus from clinical versus subclinical mastitis. Sci Rep-UK. (2020) 10:1–11. doi: 10.1038/s41598-020-75179-2
217. Vautor, E, Cockfield, J, Le Marechal, C, Le Loir, Y, Chevalier, M, Robinson, DA, et al. Difference in virulence between Staphylococcus aureus isolates causing gangrenous mastitis versus subclinical mastitis in a dairy sheep flock. Vet Res. (2009) 56:56. doi: 10.1051/vetres/2009039
218. Schneewind, O, and Missiakas, D. Sortases, surface proteins, and their roles in Staphylococcus aureus disease and vaccine development. Microbiol Spectrum. (2019) 7:173–88. doi: 10.1128/microbiolspec.PSIB-0004-2018
219. Stelzner, K, Boyny, A, Hertlein, T, Sroka, A, Moldovan, A, Paprotka, K, et al. Intracellular Staphylococcus aureus employs the cysteine protease staphopain A to induce host cell death in epithelial cells. PLoS Pathog. (2021) 17:e1009874. doi: 10.1371/journal.ppat.1009874
220. Majumder, S, Sackey, T, Viau, C, Park, S, Xia, J, Ronholm, J, et al. Genomic and phenotypic profiling of Staphylococcus aureus isolates from bovine mastitis for antibiotic resistance and intestinal infectivity. BMC Microbiol. (2023) 23:43. doi: 10.1186/s12866-023-02785-1
221. Le, KY, and Otto, M. Quorum-sensing regulation in staphylococci—an overview. Front Microbiol. (2015) 6:1–8. doi: 10.3389/fmicb.2015.01174
222. Hong, X, Qin, J, Li, T, Dai, Y, Wang, Y, Liu, Q, et al. Staphylococcal protein A promotes colonization and immune evasion of the epidemic healthcare-associated MRSA ST239. Front Microbiol. (2016) 7:1–14. doi: 10.3389/fmicb.2016.00951
223. Rice, K, Peralta, R, Bast, D, De Azavedo, J, and McGavin, MJ. Description of Staphylococcus serine protease (ssp) operon in Staphylococcus aureus and nonpolar inactivation of sspA -encoded serine protease. Infect Immun. (2001) 69:159–69. doi: 10.1128/IAI.69.1.159-169.2001
224. Smith, EJ, Visai, L, Kerrigan, SW, Speziale, P, and Foster, TJ. The Sbi protein is a multifunctional immune evasion factor of Staphylococcus aureus. Infect Immun. (2011) 79:3801–9. doi: 10.1128/IAI.05075-11
225. Dubin, G . Extracellular proteases of Staphylococcus spp. Biol Chem. (2002) 383:1075–86. doi: 10.1515/BC.2002.116
226. Pi, Y, Chen, W, and Ji, Q. Structural basis of Staphylococcus aureus surface protein SdrC. Biochemistry. (2020) 59:1465–9. doi: 10.1021/acs.biochem.0c00124
227. Todar, K. Staphylococcus aureus and Staphylococcal disease. Todar's online textbook of bacteriology. (2020) 1–6. Available at: https://textbookofbacteriology.net/staph.html
228. Becker, S, Frankel, MB, Schneewind, O, and Missiakas, D. Release of protein A from the cell wall of Staphylococcus aureus. Proc Natl Acad Sci. (2014) 111:1574–9. doi: 10.1073/pnas.1317181111
229. Daum, RS, and Spellberg, B. Progress toward a Staphylococcus aureus vaccine. Clin Infect Dis. (2012) 54:560–7. doi: 10.1093/cid/cir828
230. Schiffer, CJ, Abele, M, Ehrmann, MA, and Vogel, RF. Bap-independent biofilm formation in Staphylococcus xylosus. Microorganisms. (2021) 9:2610. doi: 10.3390/microorganisms9122610
231. Flemming, H-C, and Wingender, J. The biofilm matrix. Nat Rev Microbiol. (2010) 8:623–33. doi: 10.1038/nrmicro2415
232. Stewart, PS, and Costerton, JW. Antibiotic resistance of bacteria in biofilms. Lancet. (2001) 358:135–8. doi: 10.1016/S0140-6736(01)05321-1
233. Coelho, SM, Pereira, IA, Soares, LC, Pribul, BR, and Souza, MM. Short communication: profile of virulence factors of Staphylococcus aureus isolated from subclinical bovine mastitis in the state of Rio de Janeiro, Brazil. J Dairy Sci. (2011) 94:3305–10. doi: 10.3168/jds.2010-3229
234. Matilla-Cuenca, L, Toledo-Arana, A, and Valle, J. Anti-biofilm molecules targeting functional amyloids. Antibiotics. (2021) 10:1–18. doi: 10.3390/antibiotics10070795
235. Pérez, VKC, da Costa, GM, Guimarães, AS, Heinemann, MB, Lage, AP, and Dorneles, EMS. Relationship between virulence factors and antimicrobial resistance in Staphylococcus aureus from bovine mastitis. J Global Antimicrobial Res. (2020) 22:792–802. doi: 10.1016/j.jgar.2020.06.010
236. Magro, G, Biffani, S, Minozzi, G, Ehricht, R, Monecke, S, Luini, M, et al. Virulence genes of S. aureus from dairy cow mastitis and contagiousness risk. Toxins. (2017) 9:1–12. doi: 10.3390/toxins9060195
237. Raza, A, Muhammad, G, Sharif, S, and Atta, A. Biofilm producing Staphylococcus aureus and bovine mastitis: a review. Molecular microbiology research. (2013) 33:1–8. doi: 10.5376/mmr.2013.03.0001
238. Arciola, CR, Campoccia, D, Ravaioli, S, and Montanaro, L. Polysaccharide intercellular adhesin in biofilm: structural and regulatory aspects. Front Cell Infect Microbiol. (2015) 5:7. doi: 10.3389/fcimb.2015.00007
239. Calvinho, LF, and Dallard, BE. Staphylococcus aureus chronic intramammary infections in dairy cows: Pathogen-specific characteristics. CABI Rev. (2023):13. doi: 10.1079/cabireviews.2023.0007
240. Ster, C, Lebeau, V, Leclerc, J, Fugère, A, Veh, KA, Roy, J-P, et al. In vitro antibiotic susceptibility and biofilm production of Staphylococcus aureus isolates recovered from bovine intramammary infections that persisted or not following extended therapies with cephapirin, pirlimycin or ceftiofur. Vet Res. (2017) 48:56. doi: 10.1186/s13567-017-0463-0
241. Melchior, M, Van Osch, M, Graat, R, Van Duijkeren, E, Mevius, D, Nielen, M, et al. Biofilm formation and genotyping of Staphylococcus aureus bovine mastitis isolates: evidence for lack of penicillin-resistance in Agr-type II strains. Vet Microbiol. (2009) 137:83–9. doi: 10.1016/j.vetmic.2008.12.004
242. Fabres-Klein, MH, Caizer Santos, MJ, Contelli Klein, R, Nunes De Souza, G, and De Oliveira Barros Ribon, A. An association between milk and slime increases biofilm production by bovine Staphylococcus aureus. BMC Vet Res. (2015) 11:1–8. doi: 10.1186/s12917-015-0319-7
243. Srednik, ME, Tremblay, YDN, Labrie, J, Archambault, M, Jacques, M, Fernandez Cirelli, A, et al. Biofilm formation and antimicrobial resistance genes of coagulase-negative staphylococci isolated from cows with mastitis in Argentina. FEMS Microbiol Lett. (2017) 364:1–8. doi: 10.1093/femsle/fnx0001
244. Piessens, V, De Vliegher, S, Verbist, B, Braem, G, Van Nuffel, A, De Vuyst, L, et al. Characterization of coagulase-negative Staphylococcus species from cows' milk and environment based on bap, icaA, and mecA genes and phenotypic susceptibility to antimicrobials and teat dips. J Dairy Sci. (2012) 95:7027–38. doi: 10.3168/jds.2012-5400
245. Osman, K, Abd El-Razik, K, Marie, H, and Arafa, A. Relevance of biofilm formation and virulence of different species of coagulase-negative staphylococci to public health. Eur J Clin Microbiol Infect Dis. (2015) 34:2009–16. doi: 10.1007/s10096-015-2445-3
246. Simojoki, H, Hyvonen, P, Plumed Ferrer, C, Taponen, S, and Pyorala, S. Is the biofilm formation and slime producing ability of coagulase-negative staphylococci associated with the persistence and severity of intramammary infection? Vet Microbiol. (2012) 158:344–52. doi: 10.1016/j.vetmic.2012.02.031
247. Tremblay, YD, Lamarche, D, Chever, P, Haine, D, Messier, S, and Jacques, M. Characterization of the ability of coagulase-negative staphylococci isolated from the milk of Canadian farms to form biofilms. J Dairy Sci. (2013) 96:234–46. doi: 10.3168/jds.2012-5795
248. Valle, J, Fang, X, and Lasa, I. Revisiting bap multidomain protein: more than sticking Bacteria together. Front Microbiol. (2020) 11:613581. doi: 10.3389/fmicb.2020.613581
249. Gotz, F . Staphylococcus and biofilms. Mol Microbiol. (2002) 43:1367–78. doi: 10.1046/j.1365-2958.2002.02827.x
251. Dhanawade, NB, Kalorey, DR, Srinivasan, R, Barbuddhe, SB, and Kurkure, NV. Detection of intercellular adhesion genes and biofilm production in Staphylococcus aureus isolated from bovine subclinical mastitis. Vet Res Commun. (2010) 34:81–9. doi: 10.1007/s11259-009-9326-0
252. Vasileiou, NGC, Chatzopoulos, DC, Gougoulis, DA, Sarrou, S, Katsafadou, AI, Spyrou, V, et al. Slime-producing staphylococci as causal agents of subclinical mastitis in sheep. Vet Microbiol. (2018) 224:93–9. doi: 10.1016/j.vetmic.2018.08.022
253. Cantekin, Z, Ergun, Y, Solmaz, H, and Tek, E. Detection of slime genes and antiseptic/antibiotic resistance genes in Staphylococcal isolates from Damascus goats with subclinical mastitis. Revue Méd Vét. (2019) 170:7–9.
254. Bissong, MEA, and Ateba, CN. Genotypic and phenotypic evaluation of biofilm production and antimicrobial resistance in Staphylococcus aureus isolated from milk, north West Province, South Africa. Antibiotics. (2020) 9:156. doi: 10.3390/antibiotics9040156
255. Gajewska, J, and Chajęcka-Wierzchowska, W. Biofilm formation ability and presence of adhesion genes among coagulase-negative and coagulase-positive staphylococci isolates from raw cow’s milk. Pathogens. (2020) 9:654. doi: 10.3390/pathogens9080654
256. Felipe, V, Morgante, CA, Somale, PS, Varroni, F, Zingaretti, ML, Bachetti, RA, et al. Evaluation of the biofilm forming ability and its associated genes in Staphylococcus species isolates from bovine mastitis in Argentinean dairy farms. Microb Pathog. (2017) 104:278–86. doi: 10.1016/j.micpath.2017.01.047
257. Milanov, D, Lazić, S, Vidić, B, Petrović, J, Bugarski, D, and Šeguljev, Z. Slime production and biofilm forming ability by Staphylococcus aureus bovine mastitis isolates. Acta Vet Brno. (2010) 60:217–26. doi: 10.2298/AVB1003217M
258. Cunha, P, Le Vern, Y, Gitton, C, Germon, P, Foucras, G, and Rainard, P. Expansion, isolation and first characterization of bovine Th17 lymphocytes. Sci Rep-UK. (2019) 9:1–14. doi: 10.1038/s41598-019-52562-2
259. Cucarella, C, Solano, C, Valle, J, Amorena, B, Lasa, INI, and PenadéS, JR. Bap, a Staphylococcus aureus surface protein involved in biofilm formation. J Bacteriol. (2001) 183:2888–96. doi: 10.1128/JB.183.9.2888-2896.2001
260. Taglialegna, A, Navarro, S, Ventura, S, Garnett, JA, Matthews, S, Penades, JR, et al. Staphylococcal bap proteins build amyloid scaffold biofilm matrices in response to environmental signals. PLoS Pathog. (2016) 12:e1005711. doi: 10.1371/journal.ppat.1005711
261. Cucarella, C, Tormo, MA, Ubeda, C, Trotonda, MP, Monzón, M, Peris, C, et al. Role of biofilm-associated protein bap in the pathogenesis of bovine Staphylococcus aureus. Infect Immun. (2004) 72:2177–85. doi: 10.1128/IAI.72.4.2177-2185.2004
262. Vasudevan, P, Nair, MKM, Annamalai, T, and Venkitanarayanan, KS. Phenotypic and genotypic characterization of bovine mastitis isolates of Staphylococcus aureus for biofilm formation. Vet Microbiol. (2003) 92:179–85. doi: 10.1016/S0378-1135(02)00360-7
263. Szweda, P, Schielmann, M, Milewski, S, Frankowska, A, and Jakubczak, A. Biofilm production and presence of Ica and bap genes in Staphylococcus aureus strains isolated from cows with mastitis in the eastern Poland. Pol. J Microbiol. (2012) 61:65–9. doi: 10.33073/pjm-2012-009
264. Notcovich, S, Denicolo, G, Flint, S, Williamson, N, Gedye, K, Grinberg, A, et al. Biofilm-forming potential of Staphylococcus aureus isolated from bovine mastitis in New Zealand. Vet Sci. (2018) 5:8. doi: 10.3390/vetsci5010008
265. O’Gara, JP . Ica and beyond: biofilm mechanisms and regulation in Staphylococcus epidermidis and Staphylococcus aureus. FEMS Microbiol Letters. (2007) 270:179–88. doi: 10.1111/j.1574-6968.2007.00688.x
266. Otto, M . Staphylococcal infections: mechanisms of biofilm maturation and detachment as critical determinants of pathogenicity. Annu Rev Med. (2013) 64:175–88. doi: 10.1146/annurev-med-042711-140023
267. Cornforth, DM, Dees, JL, Ibberson, CB, Huse, HK, Mathiesen, IH, Kirketerp-Møller, K, et al. Pseudomonas aeruginosa transcriptome during human infection. Proc Natl Acad Sci. (2018) 115:E5125–34. doi: 10.1073/pnas.1717525115
268. Hoiby, N, Flensborg, EW, Beck, B, Friis, B, Jacobsen, SV, and Jacobsen, L. Pseudomonas aeruginosa infection in cystic fibrosis. Diagnostic and prognostic significance of Pseudomonas aeruginosa precipitins determined by means of crossed immunoelectrophoresis. Scand. J Respir Dis. (1977) 58:65–79.
269. Marrie, TJ, Nelligan, J, and Costerton, JW. A scanning and transmission electron microscopic study of an infected endocardial pacemaker lead. Circulation. (1982) 66:1339–41. doi: 10.1161/01.CIR.66.6.1339
270. Lebeaux, D, and Ghigo, JM. Management of biofilm-associated infections: what can we expect from recent research on biofilm lifestyles? Med Sci. (2012) 28:727–39. doi: 10.1051/medsci/2012288015
271. Hensen, SM, Pavičić, MJAMP, Lohuis, JACM, de Hoog, JAM, and Poutrel, B. Location of Staphylococcus aureus within the experimentally infected bovine udder and the expression of capsular polysaccharide type 5 in situ. J Dairy Sci. (2000) 83:1966–75. doi: 10.3168/jds.S0022-0302(00)75073-9
272. Schönborn, S, and Krömker, V. Detection of the biofilm component polysaccharide intercellular adhesin in Staphylococcus aureus infected cow udders. Vet Microbiol. (2016) 196:126–8. doi: 10.1016/j.vetmic.2016.10.023
273. Bohl, LP, Isaac, P, Breser, ML, Orellano, MS, Correa, SG, Tolosa de Talamoni, NG, et al. Interaction between bovine mammary epithelial cells and planktonic or biofilm Staphylococcus aureus: the bacterial lifestyle determines its internalization ability and the pathogen recognition. Microb Pathog. (2021) 152:104604. doi: 10.1016/j.micpath.2020.104604
274. Jensen, P, Givskov, M, Bjarnsholt, T, and Moser, C. The immune system vs. Pseudomonas aeruginosa biofilms. FEMS Immunol Med Microbiol. (2010) 59:292–305. doi: 10.1111/j.1574-695X.2010.00706.x
275. Bjarnsholt, T, and Givskov, M. The role of quorum sensing in the pathogenicity of the cunning aggressor Pseudomonas aeruginosa. Anal Bioanal Chem. (2007) 387:409–14. doi: 10.1007/s00216-006-0774-x
276. Zaatout, N, Ayachi, A, and Kecha, M. Interaction of primary mammary bovine epithelial cells with biofilm-forming staphylococci associated with subclinical bovine mastitis. Iran J Vet Res. (2019) 20:27–32.
277. Oliveira, M, Bexiga, R, Nunes, SF, and Vilela, CL. Invasive potential of biofilm-forming staphylococci bovine subclinical mastitis isolates. J Vet Sci. (2011) 12:95–7. doi: 10.4142/jvs.2011.12.1.95
278. Mulcahy, LR, Isabella, VM, and Lewis, K. Pseudomonas aeruginosa biofilms in disease. Microb Ecol. (2014) 68:1–12. doi: 10.1007/s00248-013-0297-x
279. Høiby, N, Bjarnsholt, T, Moser, C, Jensen, P, Kolpen, M, Qvist, T, et al. Diagnosis of biofilm infections in cystic fibrosis patients. APMIS. (2017) 125:339–43. doi: 10.1111/apm.12689
280. Kirketerp-Møller, K, Jensen, P, Fazli, M, Madsen, KG, Pedersen, J, Moser, C, et al. Distribution, organization, and ecology of bacteria in chronic wounds. J Clin Microbiol. (2008) 46:2717–22. doi: 10.1128/JCM.00501-08
281. Fazli, M, Bjarnsholt, T, Høiby, N, Givskov, M, and Tolker-Nielsen, T. PNA-based fluorescence in situ hybridization for identification of bacteria in clinical samples. Methods Mol Biol. (2014) 1211:261–71. doi: 10.1007/978-1-4939-1459-3_21
282. Rudkjøbing, VB, Thomsen, TR, Xu, Y, Melton-Kreft, R, Ahmed, A, Eickhardt, S, et al. Comparing culture and molecular methods for the identification of microorganisms involved in necrotizing soft tissue infections. BMC Infect Dis. (2016) 16:652. doi: 10.1186/s12879-016-1976-2
283. Malic, S, Hill, KE, Hayes, A, Percival, SL, Thomas, DW, and Williams, DW. Detection and identification of specific bacteria in wound biofilms using peptide nucleic acid fluorescent in situ hybridization (PNA FISH). Microbiology. (2009) 155:2603–11. doi: 10.1099/mic.0.028712-0
284. Tam, K, and Torres, VJ. Staphylococcus aureus secreted toxins and extracellular enzymes. Microbiol Spectrum. (2019) 7:1–59. doi: 10.1128/microbiolspec.GPP3-0039-2018
285. McAdow, M, Missiakas, DM, and Schneewind, O. Staphylococcus aureus secretes coagulase and von Willebrand factor binding protein to modify the coagulation cascade and establish host infections. J Innate Immun. (2012) 4:141–8. doi: 10.1159/000333447
286. Crosby, HA, Kwiecinski, J, and Horswill, AR. Staphylococcus aureus aggregation and coagulation mechanisms, and their function in host–pathogen interactions. Adv Appl Microbiol. (2016) 96:1–41. doi: 10.1016/bs.aambs.2016.07.018
287. Parry, MAA, Fernandez-Catalan, C, Bergner, A, Huber, R, Hopfner, K-P, Schlott, B, et al. The ternary microplasmin–staphylokinase–microplasmin complex is a proteinase–cofactor–substrate complex in action. Nat Struct Biol. (1998) 5:917–23. doi: 10.1038/2359
288. Kusch, H, and Engelmann, S. Secrets of the secretome in Staphylococcus aureus. Int J Med Microbiol. (2014) 304:133–41. doi: 10.1016/j.ijmm.2013.11.005
289. Dinges, MM, Orwin, PM, and Schlievert, PM. Exotoxins of Staphylococcus aureus Clinical Microbiology Reviews. (2000) 13:16–34. doi: 10.1128/CMR.13.1.16-34.2000
290. Gouaux, JE, Braha, O, Hobaugh, MR, Song, L, Cheley, S, Shustak, C, et al. Subunit stoichiometry of staphylococcal alpha-hemolysin in crystals and on membranes: a heptameric transmembrane pore. Proc Natl Acad Sci. (1994) 91:12828–31. doi: 10.1073/pnas.91.26.12828
291. Xiong, YQ, Willard, J, Yeaman, MR, Cheung, AL, and Bayer, AS. Regulation of Staphylococcus aureus alpha-toxin gene (hla) expression by agr, sarA, and sae in vitro and in experimental infective endocarditis. J Infect Dis. (2006) 194:1267–75. doi: 10.1086/508210
292. Cooper, LZ, Madoff, MA, and Weinstein, L. Heat stability and species range of purified staphylococcal alpha-toxin. J Bacteriol. (1966) 91:1686–92. doi: 10.1128/jb.91.5.1686-1692.1966
293. Nygaard, TK, Pallister, KB, Dumont, AL, Dewald, M, Watkins, RL, Pallister, EQ, et al. Alpha-toxin induces programmed cell death of human T cells, B cells, and monocytes during USA300 infection. PLoS One. (2012) 7:e36532. doi: 10.1371/journal.pone.0036532
294. Wilke, GA, and Wardenburg, JB. Role of a disintegrin and metalloprotease 10 in Staphylococcus aureus α-hemolysin–mediated cellular injury. Proc Natl Acad Sci. (2010) 107:13473–8. doi: 10.1073/pnas.1001815107
295. Inoshima, I, Inoshima, N, Wilke, GA, Powers, ME, Frank, KM, Wang, Y, et al. A Staphylococcus aureus pore-forming toxin subverts the activity of ADAM10 to cause lethal infection in mice. Nat Med. (2011) 17:1310–4. doi: 10.1038/nm.2451
296. Kong, C, Neoh, HM, and Nathan, S. Targeting Staphylococcus aureus toxins: A potential form of anti-virulence therapy. Toxins. (2016) 8:1–21. doi: 10.3390/toxins8030072
297. Meyer, F, Girardot, R, Piémont, Y, Prévost, G, and Colin, DA. Analysis of the specificity of Panton-valentine leucocidin and gamma-hemolysin F component binding. Infect Immun. (2009) 77:266–73. doi: 10.1128/IAI.00402-08
298. Grumann, D, Nübel, U, and Bröker, BM. Staphylococcus aureus toxins – their functions and genetics. Infect Genet Evol. (2014) 21:583–92. doi: 10.1016/j.meegid.2013.03.013
299. Kim, M-K . Staphylococcus aureus toxins: from their pathogenic roles to anti-virulence therapy using natural products. Biotechnol Bioprocess Eng. (2019) 24:424–35. doi: 10.1007/s12257-019-0059-9
300. Yoshida, A . Staphylococcal delta-hemolysin. I. Purification and chemical properties. Biochim Biophys Acta. (1963) 71:544–53. doi: 10.1016/0006-3002(63)91126-0
301. Oliveira, D, Borges, A, and Simoes, M. Staphylococcus aureus toxins and their molecular activity in infectious diseases. Toxins. (2018) 10:1–19. doi: 10.3390/toxins10060252
302. Thomsen, IP, Dumont, AL, James, DB, Yoong, P, Saville, BR, Soper, N, et al. Children with invasive Staphylococcus aureus disease exhibit a potently neutralizing antibody response to the cytotoxin LukAB. Infect Immun. (2014) 82:1234–42. doi: 10.1128/IAI.01558-13
303. Rainard, P, Corrales, JC, Barrio, MB, Cochard, T, and Poutrel, B. Leucotoxic activities of Staphylococcus aureus strains isolated from cows, ewes, and goats with mastitis: importance of LukM/LukF'-PV leukotoxin. Clin Diagn Lab Immunol. (2003) 10:272–7. doi: 10.1128/CDLI.10.2.272-277.2003
304. Yamada, T, Tochimaru, N, Nakasuji, S, Hata, E, Kobayashi, H, Eguchi, M, et al. Leukotoxin family genes in Staphylococcus aureus isolated from domestic animals and prevalence of lukM-lukF-PV genes by bacteriophages in bovine isolates. Vet Microbiol. (2005) 110:97–103. doi: 10.1016/j.vetmic.2005.07.006
305. Vrieling, M, Koymans, KJ, Heesterbeek, DA, Aerts, PC, Rutten, VP, de Haas, CJ, et al. Bovine Staphylococcus aureus secretes the Leukocidin LukMF' to kill migrating neutrophils through CCR1. MBio. (2015) 6:e00335. doi: 10.1128/mBio.00335-15
306. Spaulding, AR, Salgado-Pabón, W, Kohler, PL, Horswill, AR, Leung, DYM, and Schlievert, PM. Staphylococcal and streptococcal Superantigen exotoxins. Clin Microbiol Rev. (2013) 26:422–47. doi: 10.1128/CMR.00104-12
307. Fitzgerald, JR, Reid, SD, Ruotsalainen, E, Tripp, TJ, Liu, M, Cole, R, et al. Genome diversification in Staphylococcus aureus: molecular evolution of a highly variable chromosomal region encoding the Staphylococcal exotoxin-like family of proteins. Infect Immun. (2003) 71:2827–38. doi: 10.1128/IAI.71.5.2827-2838.2003
308. Lina, G, Gregory, S, Hiramatsu, K, Jouvin-Marche, E, and Mariuzza, R. Standard nomenclature for the Superantigens expressed by Staphylococcus. J Infect Dis. (2004) 189:2334–6. doi: 10.1086/420852
309. Langley, R, Patel, D, Jackson, N, Clow, F, and Fraser, JD. Staphylococcal superantigen super-domains in immune evasion. Crit Rev Immunol. (2010) 30:149–65. doi: 10.1615/CritRevImmunol.v30.i2.40
310. Le Loir, Y, Baron, F, and Gautier, M. Staphylococcus aureus and food poisoning. Genetics Mol Res. (2003) 1:63–76.
311. Denny, CB, Tan, PL, and Bohrer, CW. Heat inactivation of Staphylococcal enterotoxin A. J Food Sci. (1966) 31:762–7. doi: 10.1111/j.1365-2621.1966.tb01938.x
312. Genigeorgis, CA . Present state of knowledge on staphylococcal intoxication. Int J Food Microbiol. (1989) 9:327–60. doi: 10.1016/0168-1605(89)90100-1
313. Ortega, E, Abriouel, H, Lucas, R, and Gálvez, A. Multiple roles of Staphylococcus aureus enterotoxins: pathogenicity, Superantigenic activity, and correlation to antibiotic resistance. Toxins. (2010) 2:2117–31. doi: 10.3390/toxins2082117
314. Podkowik, M, Park, JY, Seo, KS, Bystron, J, and Bania, J. Enterotoxigenic potential of coagulase-negative staphylococci. Int J Food Microbiol. (2013) 163:34–40. doi: 10.1016/j.ijfoodmicro.2013.02.005
315. Park, JY, Fox, LK, Seo, KS, McGuire, MA, Park, YH, Rurangirwa, FR, et al. Detection of classical and newly described staphylococcal superantigen genes in coagulase-negative staphylococci isolated from bovine intramammary infections. Vet Microbiol. (2011) 147:149–54. doi: 10.1016/j.vetmic.2010.06.021
316. Ubeda, C, Barry, P, Penades, JR, and Novick, RP. A pathogenicity island replicon in Staphylococcus aureus replicates as an unstable plasmid. Proc Natl Acad Sci USA. (2007) 104:14182–8. doi: 10.1073/pnas.0705994104
317. Asao, T, Kumeda, Y, Kawai, T, Shibata, T, Oda, H, Haruki, K, et al. An extensive outbreak of staphylococcal food poisoning due to low-fat milk in Japan: estimation of enterotoxin A in the incriminated milk and powdered skim milk. Epidemiol Infect. (2003) 130:33–40. doi: 10.1017/S0950268802007951
318. Schlievert, PM, and Case, LC. Molecular analysis of staphylococcal superantigens. Methods Mol Biol. (2007) 391:113–26. doi: 10.1007/978-1-59745-468-1_9
319. Thomas, DY, Jarraud, S, Lemercier, B, Cozon, G, Echasserieau, K, Etienne, J, et al. Staphylococcal enterotoxin-like toxins U2 and V, two new staphylococcal superantigens arising from recombination within the enterotoxin gene cluster. Infect Immun. (2006) 74:4724–34. doi: 10.1128/IAI.00132-06
320. Hennekinne, JA, De Buyser, ML, and Dragacci, S. Staphylococcus aureus and its food poisoning toxins: characterization and outbreak investigation. FEMS Microbiol Rev. (2012) 36:815–36. doi: 10.1111/j.1574-6976.2011.00311.x
321. Adams, MR, and Moss, MO. Bacterial agents of foodborne illness—Staphylococcus aureus. Food Microbiology. Cambridge: Royal Society of Chemistry (2008).
322. Abril, AG, Villa, TG, Barros-Velázquez, J, Cañas, B, Sánchez-Pérez, A, Calo-Mata, P, et al. Staphylococcus aureus exotoxins and their detection in the dairy industry and mastitis. Toxins. (2020) 12:537. doi: 10.3390/toxins12090537
323. Khairullah, AR, Sudjarwo, SA, Effendi, MH, Harijani, N, Tyasningsih, W, Rahmahani, J, et al. A review of methicillin-resistant Staphylococcus aureus (MRSA) on Milk and Milk products: public health importance. Sys Rev Pharm. (2020) 11
324. Valero, A, Perez-Rodriguez, F, Carrasco, E, Fuentes-Alventosa, JM, Garcia-Gimeno, RM, and Zurera, G. Modelling the growth boundaries of Staphylococcus aureus: effect of temperature, pH and water activity. Int J Food Microbiol. (2009) 133:186–94. doi: 10.1016/j.ijfoodmicro.2009.05.023
325. Medvedova, A, Havlikova, A, Lehotova, V, and Valik, L. Staphylococcus aureus 2064 growth as affected by temperature and reduced water activity. Ital J Food Saf. (2019) 8:8287. doi: 10.4081/ijfs.2019.8287
326. Cunha, RC, Rosa, MDH, Silva, C, Santos, FDS, and Leite, FPL. Staphylococcal slime layers and biofilm from different origins. Ciência Rural. (2019):49.
327. Betley, MJ, and Mekalanos, JJ. Staphylococcal enterotoxin A is encoded by phage. Science. (1985) 229:185–7. doi: 10.1126/science.3160112
328. Wallin-Carlquist, N, Cao, R, Márta, D, Da Silva, ASA, Schelin, J, and Rådström, P. Acetic acid increases the phage-encoded enterotoxin A expression in Staphylococcus aureus. BMC Microbiol. (2010) 10:147. doi: 10.1186/1471-2180-10-147
329. Schelin, J, Wallin-Carlquist, N, Thorup Cohn, M, Lindqvist, R, and Barker, GC. The formation of Staphylococcus aureus enterotoxin in food environments and advances in risk assessment. Virulence. (2011) 2:580–92. doi: 10.4161/viru.2.6.18122
330. Necidova, L, Bogdanovicova, K, Harustiakova, D, and Bartova, K. Short communication: pasteurization as a means of inactivating staphylococcal enterotoxins A, B, and C in milk. J Dairy Sci. (2016) 99:8638–43. doi: 10.3168/jds.2016-11252
331. Wang, W, Lin, X, Jiang, T, Peng, Z, Xu, J, Yi, L, et al. Prevalence and characterization of Staphylococcus aureus cultured from raw Milk taken from dairy cows with mastitis in Beijing, China. Front Microbiol. (2018) 9:1123. doi: 10.3389/fmicb.2018.01123
332. Taher, EM, Hemmatzadeh, F, Aly, SA, Elesswy, HA, and Petrovski, KR. Survival of staphylococci and transmissibility of their antimicrobial resistance genes in milk after heat treatments. LWT. (2020) 129:109584. doi: 10.1016/j.lwt.2020.109584
333. Taher, EM, Hemmatzadeh, F, Aly, SA, Elesswy, HA, and Petrovski, KR. Molecular characterization of antimicrobial resistance genes on farms and in commercial milk with emphasis on the effect of currently practiced heat treatments on viable but nonculturable formation. J Dairy Sci. (2020) 103:9936–45. doi: 10.3168/jds.2020-18631
334. Hébert, A, Sayasith, K, Sénéchal, S, Dubreuil, P, and Lagacé, J. Demonstration of intracellular Staphylococcus aureus in bovine mastitis alveolar cells and macrophages isolated from naturally infected cow milk. FEMS Microbiol Lett. (2000) 193:57–62. doi: 10.1016/S0378-1097(00)00455-9
335. Elliott, GR, Peterson, PK, Verbrugh, HA, Freiberg, MR, Hoidal, JR, and Quie, PG. Influence of subinhibitory concentrations of penicillin, cephalothin, and clindamycin on Staphylococcus aureus growth in human phagocytic cells. Antimicrob Agents Chemother. (1982) 22:781–4. doi: 10.1128/AAC.22.5.781
336. Cai, J, Li, J, Zhou, Y, Wang, J, Li, J, Cui, L, et al. Staphylococcus aureus facilitates its survival in bovine macrophages by blocking autophagic flux. J Cell Mol Med. (2020) 24:3460–8. doi: 10.1111/jcmm.15027
337. Wileman, T . Autophagy as a defence against intracellular pathogens. Essays Biochem. (2013) 55:153–63. doi: 10.1042/bse0550153
338. Neumann, Y, Bruns, SA, Rohde, M, Prajsnar, TK, Foster, SJ, and Schmitz, I. Intracellular Staphylococcus aureus eludes selective autophagy by activating a host cell kinase. Autophagy. (2016) 12:2069–84. doi: 10.1080/15548627.2016.1226732
339. Escoll, P, and Rolando, M, Buchrieser C. Modulation of Host Autophagy during Bacterial Infection: Sabotaging Host Munitions for Pathogen Nutrition. Front Immunol. (2016);7, 7, doi: 10.3389/fimmu.2016.00081
340. Steele, S, Brunton, J, and Kawula, T. The role of autophagy in intracellular pathogen nutrient acquisition. Front Cell Infect Microbiol. (2015):5.
341. Wang, H, Zeng, X, Mo, Y, He, B, Lin, H, and Lin, J. Enterobactin-specific antibodies induced by a novel Enterobactin conjugate vaccine. Appl Environ Microbiol. (2019) 85:1–15. doi: 10.1128/AEM.00358-19
342. Casanova, JE . Bacterial autophagy: offense and defense at the host–pathogen Interface. Cell Mol Gastroenterol Hepatol. (2017) 4:237–43. doi: 10.1016/j.jcmgh.2017.05.002
343. von Eiff, C . Staphylococcus aureus small colony variants: a challenge to microbiologists and clinicians. Int J Antimicrob Agents. (2008) 31:507–10. doi: 10.1016/j.ijantimicag.2007.10.026
344. Atalla, H, Gyles, C, and Mallard, B. Staphylococcus aureus small colony variants (SCVs) and their role in disease. Anim Health Res Rev. (2011) 12:33–45. doi: 10.1017/S1466252311000065
345. Sompolinsky, D, Cohen, M, and Ziv, G. Epidemiological and biochemical studies on thiamine-less dwarf-Colony variants of Staphylococcus aureus as etiological agents of bovine mastitis. Infect Immun. (1974) 9:217–28. doi: 10.1128/iai.9.2.217-228.1974
346. Ezzat Alnakip, M, Quintela-Baluja, M, Böhme, K, Fernández-No, I, Caamaño-Antelo, S, Calo-Mata, P, et al. The immunology of mammary gland of dairy ruminants between healthy and inflammatory conditions. J Vet Med. (2014) 2014:1–31. doi: 10.1155/2014/659801
347. Paulrud, CO . Basic concepts of the bovine Teat Canal. Vet Res Commun. (2005) 29:215–45. doi: 10.1023/B:VERC.0000047496.47571.41
348. Zigo, F, Vasil, M, Ondrasovicova, S, Vyrostkova, J, Bujok, J, and Pecka-Kielb, E. Maintaining optimal mammary gland health and prevention of mastitis. Front Vet Sci. (2021) 8:607311. doi: 10.3389/fvets.2021.607311
349. Wang, B, McKittrick, O, and Meyers, MA. Keratin: structure, mechanical properties, occurrence in biological organisms, and efforts at bioinspiration. Prog Mater Sci. (2016) 76:229–318. doi: 10.1016/j.pmatsci.2015.06.001
350. Bragulla, HH, and Homberger, DG. Structure and functions of keratin proteins in simple, stratified, keratinized and cornified epithelia. J Anat. (2009) 214:516–59. doi: 10.1111/j.1469-7580.2009.01066.x
351. Smolenski, GA, Cursons, RT, Hine, BC, and Wheeler, TT. Keratin and S100 calcium-binding proteins are major constituents of the bovine teat canal lining. Vet Res. (2015) 46:113. doi: 10.1186/s13567-015-0227-7
352. Hibbitt, KG, and Benians, M. Some effects in vivo of the Teat Canal and effects in vitro of cationic proteins on staphylococci. J Gen Microbiol. (1971) 68:123–8. doi: 10.1099/00221287-68-1-123
353. Gennaro, R, Dewald, B, Horisberger, U, Gubler, HU, and Baggiolini, M. A novel type of cytoplasmic granule in bovine neutrophils. J Cell Biol. (1983) 96:1651–61. doi: 10.1083/jcb.96.6.1651
354. Nickerson, SC . Resistance mechanisms of the bovine udder: new implications for mastitis control at the teat end. J Am Vet Med Assoc. (1987) 191:1484–8.
355. Capuco, AV, Mein, GA, Nickerson, SC, Jack, LJ, Wood, DL, Bright, SA, et al. Influence of pulsationless milking on teat canal keratin and mastitis. J Dairy Sci. (1994) 77:64–74. doi: 10.3168/jds.S0022-0302(94)76929-0
356. Bramley, AJ, and Dodd, FH. Reviews of the progress of dairy science: mastitis control – progress and prospects. J Dairy Res. (1984) 51:481–512. doi: 10.1017/S0022029900023797
357. Trevisi, E, and Minuti, A. Assessment of the innate immune response in the periparturient cow. Res Vet Sci. (2018) 116:47–54. doi: 10.1016/j.rvsc.2017.12.001
358. Capuco, AV, Bright, SA, Pankey, JW, Wood, DL, Miller, RH, and Bitman, J. Increased susceptibility to intramammary infection following removal of teat canal keratin. J Dairy Sci. (1992) 75:2126–30. doi: 10.3168/jds.S0022-0302(92)77972-7
360. Merrill, C, Ensermu, DB, Abdi, RD, Gillespie, BE, Vaughn, J, Headrick, SI, et al. Immunological responses and evaluation of the protection in dairy cows vaccinated with staphylococcal surface proteins. Vet Immunol Immunopathol. (2019) 214:109890. doi: 10.1016/j.vetimm.2019.109890
361. Craven, N, and Williams, MR. Defences of the bovine mammary gland against infection and prospects for their enhancement. Vet Immunol Immunopathol. (1985) 10:71–127. doi: 10.1016/0165-2427(85)90039-X
362. Newbould, FHS, and Neave, FK. The effect of inoculating the bovine teat duct with small numbers of Staphylococcus aureus. J Dairy Res. (1965) 32:171–9. doi: 10.1017/S0022029900018501
363. Griffin, TK, Williams, RL, Grindal, RJ, Neave, FK, and Westgarth, DR. Use of deflector shields to reduce intramammary infection by preventing impacts on the teat ends of cows during machine milking. J Dairy Res. (1983) 50:397–404. doi: 10.1017/S0022029900032623
364. Kuehn, JS, Gorden, PJ, Munro, D, Rong, R, Dong, Q, Plummer, PJ, et al. Bacterial community profiling of Milk samples as a means to understand culture-negative bovine clinical mastitis. PLoS One. (2013) 8:e61959. doi: 10.1371/journal.pone.0061959
365. Oikonomou, G, Addis, MF, Chassard, C, Nader-Macias, MEF, Grant, I, Delbès, C, et al. Milk microbiota: what are we exactly talking about? Front Microbiol. (2020) 11:1–15. doi: 10.3389/fmicb.2020.00060
366. Ren, Z, Yu, Y, Chen, C, Yang, D, Ding, T, Zhu, L, et al. The triangle relationship between long noncoding RNA, RIG-I-like receptor signaling pathway, and glycolysis. Front Microbiol. (2021) 12:1–16. doi: 10.3389/fmicb.2021.807737
367. Dykes, IM, and Emanueli, C. Transcriptional and post-transcriptional gene regulation by long non-coding RNA. Genomics Proteomics Bioinformatics. (2017) 15:177–86. doi: 10.1016/j.gpb.2016.12.005
368. Yao, ZT, Yang, YM, Sun, MM, He, Y, Liao, L, Chen, KS, et al. New insights into the interplay between long non-coding RNAs and RNA-binding proteins in cancer. Cancer Commun. (2022) 42:117–40. doi: 10.1002/cac2.12254
369. Chen, YG, Satpathy, AT, and Chang, HY. Gene regulation in the immune system by long noncoding RNAs. Nat Immunol. (2017) 18:962–72. doi: 10.1038/ni.3771
370. Wang, H, Wang, X, Li, X, Wang, Q, Qing, S, Zhang, Y, et al. A novel long non-coding RNA regulates the immune response inMAC-T cells and contributes to bovine mastitis. FEBS J. (2019) 286:1780–95. doi: 10.1111/febs.14783
371. Yang, W, Li, X, Qi, S, Li, X, Zhou, K, Qing, S, et al. lncRNA H19 is involved in TGF-β1-induced epithelial to mesenchymal transition in bovine epithelial cells through PI3K/AKT signaling pathway. PeerJ. (2017) 5:e3950-e. doi: 10.7717/peerj.3950
372. Baulida, J . Epithelial-to-mesenchymal transition transcription factors in cancer-associated fibroblasts. Mol Oncol. (2017) 11:847–59. doi: 10.1002/1878-0261.12080
373. Zeisberg, M, Hanai, J-I, Sugimoto, H, Mammoto, T, Charytan, D, Strutz, F, et al. BMP-7 counteracts TGF-β1–induced epithelial-to-mesenchymal transition and reverses chronic renal injury. Nat Med. (2003) 9:964–8. doi: 10.1038/nm888
374. Ma, M, Pei, Y, Wang, X, Feng, J, Zhang, Y, and Gao, M-Q. LncRNA XIST mediates bovine mammary epithelial cell inflammatory response via NF-κB/NLRP3 inflammasome pathway. Cell Prolif. (2019) 52:e12525. doi: 10.1111/cpr.12525
375. Wang, X, Wang, H, Zhang, R, Li, D, and Gao, M-Q. LRRC75A antisense lncRNA1 knockout attenuates inflammatory responses of bovine mammary epithelial cells. Int J Biol Sci. (2020) 16:251–63. doi: 10.7150/ijbs.38214
376. Ruegg, PL . What is success? A narrative review of research evaluating outcomes of antibiotics used for treatment of clinical mastitis. Front Vet Sci. (2021) 8:639641. doi: 10.3389/fvets.2021.639641
377. Van Saun, RJ . (2016). The role of nutrition in mastitis prevention is reviewed relative to its impact on immune response of dairy cows. Available at: https://extension.psu.edu/nutrition-immunity-and-mastitis (Accessed April 26, 2024).
378. Thompson-Crispi, KA, and Mallard, BA. Type 1 and type 2 immune response profiles of commercial dairy cows in 4 regions across Canada. Can J Vet Res. (2012) 76:120–8.
379. Rinaldi, M, Li, RW, Bannerman, DD, Daniels, KM, Evock-Clover, C, Silva, MVB, et al. A sentinel function for teat tissues in dairy cows: dominant innate immune response elements define early response to E. coli mastitis. Funct Integr Genomics. (2010) 10:21–38. doi: 10.1007/s10142-009-0133-z
380. Whelehan, CJ, Meade, KG, Eckersall, PD, Young, FJ, and O’Farrelly, C. Experimental Staphylococcus aureus infection of the mammary gland induces region-specific changes in innate immune gene expression. Vet Immunol Immunopathol. (2011) 140:181–9. doi: 10.1016/j.vetimm.2010.11.013
381. Rich, RR, Fleisher, TA, Shearer, WT, Schroeder, HW Jr, Frew, AJ, and Weyand, CM. Clinical immunology e-book: Principles and practice Elsevier Health Sciences (2012). Available at: https://books.google.com/books?id=jaH_qwUqKDgC
382. Thomer, L, Schneewind, O, and Missiakas, D. Pathogenesis of Staphylococcus aureus bloodstream infections. Ann Rev Pathol. (2016) 11:343–64. doi: 10.1146/annurev-pathol-012615-044351
383. Oviedo-Boyso, J, Valdez-Alarcón, JJ, Cajero-Juárez, M, Ochoa-Zarzosa, A, López-Meza, JE, Bravo-Patiño, A, et al. Innate immune response of bovine mammary gland to pathogenic bacteria responsible for mastitis. J Infect. (2007) 54:399–409. doi: 10.1016/j.jinf.2006.06.010
384. Rambault, M, Gilbert, FB, Roussel, P, Tessier, A, David, V, Germon, P, et al. Neutrophils expressing major histocompatibility complex class II molecules circulate in blood and milk during mastitis and show high microbicidal activity. J Dairy Sci. (2023) 106:4245–56. doi: 10.3168/jds.2022-22728
385. Fournier, BND, and Philpott, DJ. Recognition of Staphylococcus aureus by the innate immune system. Clin Microbiol Rev. (2005) 18:521–40. doi: 10.1128/CMR.18.3.521-540.2005
386. Sen, HN . Elements of the immune system and concepts of intraocular inflammatory disease pathogenesis. Uveitis. Whitcup and Nussenblatt’s Uveitis (5th Edition) Whitcup SM, Sen HN. editors (London: Elsevier). (2010) 1–28. doi: 10.1016/B978-0-323-48014-7.00001-4
387. Murphy, MP, Niedziela, DA, Leonard, FC, and Keane, OM. The in vitro host cell immune response to bovine-adapted Staphylococcus aureus varies according to bacterial lineage. Sci Rep. (2019) 9:6134. doi: 10.1038/s41598-019-42424-2
388. Shearer, HL, Loi, VV, Weiland, P, Bange, G, Altegoer, F, Hampton, MB, et al. MerA functions as a hypothiocyanous acid reductase and defense mechanism in Staphylococcus aureus. Mol Microbiol. (2023) 119:456–70. doi: 10.1111/mmi.15035
389. Bröker, B, Mrochen, D, and Péton, V. The T cell response to Staphylococcus aureus. Pathogens. (2016) 5:31. doi: 10.3390/pathogens5010031
390. Riollet, C, Rainard, P, and Poutrel, B. Cells and Cytokines in Inflammatory Secretions of Bovine Mammary Gland. In Mol JA, Clegg RA (ed), Biol Mammary Gland. Boston, MA: Springer US (2002):247–58. doi: 10.1007/0-306-46832-8_30
391. Riollet, C, Rainard, P, and Poutrel, B. Cell subpopulations and cytokine expression in cow milk in response to chronic Staphylococcus aureus infection. J Dairy Sci. (2001) 84:1077–84. doi: 10.3168/jds.S0022-0302(01)74568-7
392. Riollet, C, Rainard, P, and Poutrel, B. Cells and cytokines in inflammatory secretions of bovine mammary gland. Adv Exp Med Biol. (2000) 480:247–58.
393. Actor, JK, Hwang, SA, and Kruzel, ML. Lactoferrin as a natural immune modulator. Curr Pharm Des. (2009) 15:1956–73. doi: 10.2174/138161209788453202
394. Dunkelberger, JR, and Song, W-C. Complement and its role in innate and adaptive immune responses. Cell Res. (2010) 20:34–50. doi: 10.1038/cr.2009.139
396. Janeway, CA, Travers, P, Walport, M, and Shlomchik, MJ. The components of the immune system In: Immunobiology: The immune system in health and disease. 5th ed: New York: Garland Science. (2001).
397. Malkoski, M, Dashper, SG, O'Brien-Simpson, NM, Talbo, GH, Macris, M, Cross, KJ, et al. Kappacin, a novel antibacterial peptide from bovine Milk. Antimicrob Agents Chemother. (2001) 45:2309–15. doi: 10.1128/AAC.45.8.2309-2315.2001
398. Huan, Y, Kong, Q, Mou, H, and Yi, H. Antimicrobial peptides: classification, design, application and research Progress in multiple fields. Front Microbiol. (2020) 11:1–21. doi: 10.3389/fmicb.2020.582779
399. Brogden, KA, Ackermann, M, and Huttner, KM. Small, anionic, and charge-neutralizing propeptide fragments of zymogens are antimicrobial. Antimicrob Agents Chemother. (1997) 41:1615–7. doi: 10.1128/AAC.41.7.1615
400. Brogden, KA, Ackermann, M, McCray, PB, and Tack, BF. Antimicrobial peptides in animals and their role in host defences. Int J Antimicrob Agents. (2003) 22:465–78. doi: 10.1016/S0924-8579(03)00180-8
401. Tsai, H, and Bobek, LA. Human salivary Histatins: promising anti-fungal therapeutic agents. Critical Rev Oral Biol Med. (1998) 9:480–97. doi: 10.1177/10454411980090040601
402. Schittek, B, Hipfel, R, Sauer, B, Bauer, J, Kalbacher, H, Stevanovic, S, et al. Dermcidin: a novel human antibiotic peptide secreted by sweat glands. Nat Immunol. (2001) 2:1133–7. doi: 10.1038/ni732
403. Kagan, BL, Selsted, ME, Ganz, T, and Lehrer, RI. Antimicrobial defensin peptides form voltage-dependent ion-permeable channels in planar lipid bilayer membranes. Proc Natl Acad Sci. (1990) 87:210–4. doi: 10.1073/pnas.87.1.210
404. Brogden, KA, De Lucca, AJ, Bland, J, and Elliott, S. Isolation of an ovine pulmonary surfactant-associated anionic peptide bactericidal for Pasteurella haemolytica. P Natl Acad Sci USA. (1996) 93:412–6. doi: 10.1073/pnas.93.1.412
405. Skerlavaj, B, Romeo, D, and Gennaro, R. Rapid membrane permeabilization and inhibition of vital functions of gram-negative bacteria by bactenecins. Infect Immun. (1990) 58:3724–30. doi: 10.1128/iai.58.11.3724-3730.1990
406. Kopeikin, PM, Zharkova, MS, Kolobov, AA, Smirnova, MP, Sukhareva, MS, Umnyakova, ES, et al. Caprine Bactenecins as promising tools for developing new antimicrobial and antitumor drugs. Front Cell Infect Microbiol. (2020) 10:10. doi: 10.3389/fcimb.2020.552905
407. Goldammer, T, Zerbe, H, Molenaar, A, Schuberth, HJ, Brunner, RM, Kata, SR, et al. Mastitis increases mammary mRNA abundance of beta-defensin 5, toll-like-receptor 2 (TLR2), and TLR4 but not TLR9 in cattle. Clin Diagn Lab Immunol. (2004) 11:174–85.
408. Schroder, JM . Epithelial antimicrobial peptides: innate local host response elements. Cell Mol Life Sci. (1999) 56:32–46. doi: 10.1007/s000180050004
409. Zasloff, M . Antimicrobial peptides in health and disease. N Engl J Med. (2002) 347:1199–200. doi: 10.1056/NEJMe020106
410. Strandberg, Y, Gray, C, Vuocolo, T, Donaldson, L, Broadway, M, and Tellam, R. Lipopolysaccharide and lipoteichoic acid induce different innate immune responses in bovine mammary epithelial cells. Cytokine. (2005) 31:72–86. doi: 10.1016/j.cyto.2005.02.010
411. Eberl, G, Colonna, M, Di Santo, JP, and McKenzie, ANJ. Innate lymphoid cells: A new paradigm in immunology. Science. (2015) 348:1–8. doi: 10.1126/science.aaa6566
412. Annunziato, F, Romagnani, C, and Romagnani, S. The 3 major types of innate and adaptive cell-mediated effector immunity. J Allergy Clin Immunol. (2015) 135:626–35. doi: 10.1016/j.jaci.2014.11.001
413. Gaffen, SL . An overview of IL-17 function and signaling. Cytokine. (2008) 43:402–7. doi: 10.1016/j.cyto.2008.07.017
414. Rainard, P, Cunha, P, Martins, RP, Gilbert, FB, Germon, P, and Foucras, G. Type 3 immunity: a perspective for the defense of the mammary gland against infections. Vet Res. (2020) 51:129. doi: 10.1186/s13567-020-00852-3
415. Wattegedera, SR, Corripio-Miyar, Y, Pang, Y, Frew, D, McNeilly, TN, Palarea-Albaladejo, J, et al. Enhancing the toolbox to study IL-17A in cattle and sheep. Vet Res. (2017) 48:20. doi: 10.1186/s13567-017-0426-5
416. Elnaggar, MM, Abdellrazeq, GS, Dassanayake, RP, Fry, LM, Hulubei, V, and Davis, WC. Characterization of αβ and γδ T cell subsets expressing IL-17A in ruminants and swine. Dev Comp Immunol. (2018) 85:115–24. doi: 10.1016/j.dci.2018.04.003
417. Taylor, BC, Keefe, RG, Dellinger, JD, Nakamura, Y, Cullor, JS, and Stott, JL. T cell populations and cytokine expression in milk derived from normal and bacteria-infected bovine mammary glands. Cell Immunol. (1997) 182:68–76. doi: 10.1006/cimm.1997.1215
418. Porcherie, A, Gilbert, FB, Germon, P, Cunha, P, Trotereau, A, Rossignol, C, et al. IL-17A is an important effector of the immune response of the mammary gland to Escherichia coli infection. J Immunol. (2016) 196:803–12. doi: 10.4049/jimmunol.1500705
419. Betts, CB, Pennock, ND, Caruso, BP, Ruffell, B, Borges, VF, and Schedin, P. Mucosal immunity in the female murine mammary gland. J Immunol. (2018) 201:734–46. doi: 10.4049/jimmunol.1800023
420. Baldwin, CL, and Telfer, JC. The bovine model for elucidating the role of γδ T cells in controlling infectious diseases of importance to cattle and humans. Mol Immunol. (2015) 66:35–47. doi: 10.1016/j.molimm.2014.10.024
421. Bonneville, M, O'Brien, RL, and Born, WK. γδ T cell effector functions: a blend of innate programming and acquired plasticity. Nat Rev Immunol. (2010) 10:467–78. doi: 10.1038/nri2781
422. Peckham, RK, Brill, R, Foster, DS, Bowen, AL, Leigh, JA, Coffey, TJ, et al. Two distinct populations of bovine IL-17+ T-cells can be induced and WC1+IL-17+γδ T-cells are effective killers of protozoan parasites. Sci Rep-UK. (2015) 4:1–5. doi: 10.1038/srep05431
423. Steinbach, S, Vordermeier, HM, and Jones, GJ. CD4+ and γδ T cells are the main producers of IL-22 and IL-17A in lymphocytes from Mycobacterium bovis-infected cattle. Sci Rep-UK. (2016) 6:29990. doi: 10.1038/srep29990
424. Soltys, J, and Quinn, MT. Selective recruitment of T-cell subsets to the udder during staphylococcal and streptococcal mastitis: analysis of lymphocyte subsets and adhesion molecule expression. Infect Immun. (1999) 67:6293–302. doi: 10.1128/IAI.67.12.6293-6302.1999
425. Klose, CSN, and Artis, D. Innate lymphoid cells as regulators of immunity, inflammation and tissue homeostasis. Nat Immunol. (2016) 17:765–74. doi: 10.1038/ni.3489
426. Flajnik, MF, and Kasahara, M. Origin and evolution of the adaptive immune system: genetic events and selective pressures. Nat Rev Genet. (2010) 11:47–59. doi: 10.1038/nrg2703
427. Travis, J . On the origin of the immune system. Science. (2009) 324:580–2. doi: 10.1126/science.324_580
428. Wilson, GJ, Tuffs, SW, Wee, BA, Seo, KS, Park, N, Connelley, T, et al. Bovine Staphylococcus aureus Superantigens stimulate the entire T cell repertoire of cattle. Infect Immun. (2018) 86:1–16. doi: 10.1128/IAI.00505-18
429. Shishido, SN, Varahan, S, Yuan, K, Li, X, and Fleming, SD. Humoral innate immune response and disease. Clin Immunol. (2012) 144:142–58. doi: 10.1016/j.clim.2012.06.002
430. Krzymińska, S, Szczuka, E, and Kaznowski, A. Staphylococcus haemolyticus strains target mitochondria and induce caspase-dependent apoptosis of macrophages. Antonie Van Leeuwenhoek. (2012) 102:611–20. doi: 10.1007/s10482-012-9756-5
432. Sordillo, LM, and Streicher, KL. Mammary gland immunity and mastitis susceptibility. J Mammary Gland Biol Neoplasia. (2002) 7:135–46. doi: 10.1023/A:1020347818725
433. Elghafghuf, A, Dufour, S, Reyher, K, Dohoo, I, and Stryhn, H. Survival analysis of clinical mastitis data using a nested frailty Cox model fit as a mixed-effects Poisson model. Prev Vet Med. (2014) 117:456–68. doi: 10.1016/j.prevetmed.2014.09.013
434. Green, MJ, Green, LE, Medley, GF, Schukken, YH, and Bradley, AJ. Influence of dry period bacterial intramammary infection on clinical mastitis in dairy cows. J Dairy Sci. (2002) 85:2589–99. doi: 10.3168/jds.S0022-0302(02)74343-9
435. Anderson, KL, Lyman, RL, Bodeis-Jones, SM, and White, DG. Genetic diversity and antimicrobial susceptibility profiles among mastitis-causing Staphylococcus aureus isolated from bovine milk samples. Am J Vet Res. (2006) 67:1185–91. doi: 10.2460/ajvr.67.7.1185
436. Graber, H, Naskova, J, Studer, E, Kaufmann, T, Kirchhofer, M, Brechbühl, M, et al. Mastitis-related subtypes of bovine Staphylococcus aureus are characterized by different clinical properties. J Dairy Sci. (2009) 92:1442–51. doi: 10.3168/jds.2008-1430
437. Capurro, A, Aspan, A, Artursson, K, and Waller, KP. Genotypic variation among Staphylococcus aureus isolates from cases of clinical mastitis in Swedish dairy cows. Vet J. (2010) 185:188–92. doi: 10.1016/j.tvjl.2009.05.007
438. Lundberg, Å, Aspán, A, Nyman, A, Unnerstad, HE, and Waller, KP. Associations between bacterial genotype and outcome of bovine clinical Staphylococcus aureus mastitis. Acta Vet Scand. (2014) 56:1–8. doi: 10.1186/1751-0147-56-2
439. Oliveira, M, Nunes, SF, Carneiro, C, Bexiga, R, Bernardo, F, and Vilela, CL. Time course of biofilm formation by Staphylococcus aureus and Staphylococcus epidermidis mastitis isolates. Vet Microbiol. (2007) 124:187–91. doi: 10.1016/j.vetmic.2007.04.016
440. Oliveira, M, Bexiga, R, Nunes, SF, Carneiro, C, Cavaco, LM, Bernardo, F, et al. Biofilm-forming ability profiling of Staphylococcus aureus and Staphylococcus epidermidis mastitis isolates. Vet Microbiol. (2006) 118:133–40. doi: 10.1016/j.vetmic.2006.07.008
441. Melchior, MB, Fink-Gremmels, J, and Gaastra, W. Comparative assessment of the antimicrobial susceptibility of Staphylococcus aureus isolates from bovine mastitis in biofilm versus planktonic culture. J Vet Med B Infect Dis Vet Public Health. (2006) 53:326–32. doi: 10.1111/j.1439-0450.2006.00962.x
442. Middleton, JR, Fales, WH, Luby, CD, Oaks, JL, Sanchez, S, Kinyon, JM, et al. Surveillance of Staphylococcus aureus in veterinary teaching hospitals. J Clin Microbiol. (2005) 43:2916–9. doi: 10.1128/JCM.43.6.2916-2919.2005
443. Bradley, AJ . Bovine mastitis: an evolving disease. Vet J. (2002) 164:116–28. doi: 10.1053/tvjl.2002.0724
444. Oliver, SP, Murinda, SE, and Jayarao, BM. Impact of antibiotic use in adult dairy cows on antimicrobial resistance of veterinary and human pathogens: a comprehensive review. Foodborne Pathog Dis. (2011) 8:337–55. doi: 10.1089/fpd.2010.0730
445. Bradley, AJ, and Green, MJ. Aetiology of clinical mastitis in six Somerset dairy herds. Vet Rec. (2001) 148:683–6. doi: 10.1136/vr.148.22.683
446. Hogan, J. S., and Smith, K. L. A practical look at environmental mastitis. The Compendium on continuing education for the practicing veterinarian (USA) (1987) 9:F342.
447. Aarestrup, FM, Scott, NL, and Sordillo, LM. Ability of Staphylococcus aureus coagulase genotypes to resist neutrophil bactericidal activity and phagocytosis. Infect Immun. (1994) 62:5679–82. doi: 10.1128/iai.62.12.5679-5682.1994
448. Josse, J, Laurent, F, and Diot, A. Staphylococcal adhesion and host cell invasion: fibronectin-binding and other mechanisms. Front Microbiol. (2017) 8:2433. doi: 10.3389/fmicb.2017.02433
449. Hussein, HA, Abd El-Razik, KAE-H, Gomaa, AM, Elbayoumy, MK, Abdelrahman, KA, and Hosein, HI. Milk amyloid A as a biomarker for diagnosis of subclinical mastitis in cattle. Vet World. (2018) 11:34–41. doi: 10.14202/vetworld.2018.34-41
450. Koivula, M, Pitkälä, A, Pyörälä, S, and Mäntysaari, EA. Distribution of bacteria and seasonal and regional effects in a new database for mastitis pathogens in Finland. Acta Agricul Scandinavica Sect A. (2007) 57:89–96. doi: 10.1080/09064700701488941
451. Wellnitz, O, and Bruckmaier, RM. The innate immune response of the bovine mammary gland to bacterial infection. Vet J. (2012) 192:148–52. doi: 10.1016/j.tvjl.2011.09.013
452. Matsunaga, T, Kamata, S-I, Kakiichi, N, and Uchida, K. Characteristics of Staphylococcus aureus isolated from peracute, acute and chronic bovine mastitis. J Vet Med Sci. (1993) 55:297–300. doi: 10.1292/jvms.55.297
453. Shibahara, T, and Nakamura, K. Pathology of acute necrotizing mastitis caused by Staphylococcus aureus in a dairy cow. JARQ. (1999) 33:139–42. Available at: https://www.jircas.go.jp/sites/default/files/publication/jarq/33-2-139-142_0.pdf
454. Shafi, TA, Gupta, DK, and Bansal, BK. Diagnosis and treatment of gangrenous mastitis in crossbred cows. Intas Polivet. (2015) 16. Available at: https://go.gale.com/ps/i.do?id=GALE%7CA450799293&sid=googleScholar&v=2.1&it=r&linkaccess=abs&issn=09721738&p=AONE&sw=w&userGroupName=tel_k_journeycol&aty=ip
455. Rainard, P, Gitton, C, Chaumeil, T, Fassier, T, Huau, C, Riou, M, et al. Host factors determine the evolution of infection with Staphylococcus aureus to gangrenous mastitis in goats. Vet Res. (2018) 49:72. doi: 10.1186/s13567-018-0564-4
456. Corpa, JM, Hermans, K, and Haesebrouck, F. Main pathologies associated with Staphylococcus aureus infections in rabbits: a review. World Rabbit Sci. (2010) 17:115–25. doi: 10.4995/wrs.2009.651
457. Agrawal, S, Jayant, K, and Agarwal, R. Breast gangrene: a rare source of severe sepsis. Case Reports. (2014). doi: 10.1136/bcr-2013-203467
458. Ashraf, A, and Imran, M. Diagnosis of bovine mastitis: from laboratory to farm. Trop Anim Health Prod. (2018) 50:1193–202. doi: 10.1007/s11250-018-1629-0
459. Martins, SAM, Martins, VC, Cardoso, FA, Germano, J, Rodrigues, M, Duarte, C, et al. Biosensors for on-farm diagnosis of mastitis. Front Bioeng Biotechnol. (2019) 7:186. doi: 10.3389/fbioe.2019.00186
460. Kandeel, SA, Megahed, AA, Ebeid, MH, and Constable, PD. Ability of milk pH to predict subclinical mastitis and intramammary infection in quarters from lactating dairy cattle. J Dairy Sci. (2019) 102:1417–27. doi: 10.3168/jds.2018-14993
461. Sears, PM, Smith, BS, English, PB, Herer, PS, and Gonzalez, RN. Shedding pattern of Staphylococcus aureus from bovine Intramammary infections. J Dairy Sci. (1990) 73:2785–9. doi: 10.3168/jds.S0022-0302(90)78964-3
462. Newbould, FH . Antibiotic treatment of experimental Staphylococcus aureus infections of the bovine mammary gland. Can J Comp Med. (1974) 38:411–6.
463. Mahmmod, YS, Toft, N, Katholm, J, Grønbæk, C, and Klaas, IC. Bayesian estimation of test characteristics of real-time PCR, bacteriological culture and California mastitis test for diagnosis of intramammary infections with Staphylococcus aureus in dairy cattle at routine milk recordings. Prev Vet Med. (2013) 112:309–17. doi: 10.1016/j.prevetmed.2013.07.021
464. Juronen, D, Kuusk, A, Kivirand, K, Rinken, A, and Rinken, T. Immunosensing system for rapid multiplex detection of mastitis-causing pathogens in milk. Talanta. (2018) 178:949–54. doi: 10.1016/j.talanta.2017.10.043
465. Rall, VL, Miranda, ES, Castilho, IG, Camargo, CH, Langoni, H, Guimaraes, FF, et al. Diversity of Staphylococcus species and prevalence of enterotoxin genes isolated from milk of healthy cows and cows with subclinical mastitis. J Dairy Sci. (2014) 97:829–37. doi: 10.3168/jds.2013-7226
466. Petersson-Wolfe, CS, Mullarky, IK, and Jones, GM. Staphylococcus aureus mastitis: Cause, detection, and control. (2010).
467. Schukken, YH, Smit, JAH, Grommers, FJ, Vandegeer, D, and Brand, A. Effect of freezing on bacteriologic culturing of mastitis Milk samples. J Dairy Sci. (1989) 72:1900–6. doi: 10.3168/jds.S0022-0302(89)79309-7
468. Murdough, PA, Deitz, KE, and Pankey, JW. Effects of freezing on the viability of nine pathogens from quarters with subclinical mastitis. J Dairy Sci. (1996) 79:334–6. doi: 10.3168/jds.S0022-0302(96)76368-3
469. Pehlivanoglu, F, Yardimci, H, and Turutoglu, H. Freezing and thawing milk samples before culture to improvediagnosis of bovine staphylococcal mastitis. Veterinarski Arhiv. (2015) 85:59–65.
470. Graber, HU, Pfister, S, Burgener, P, Boss, R, Meylan, M, and Hummerjohann, J. Bovine Staphylococcus aureus: diagnostic properties of specific media. Res Vet Sci. (2013) 95:38–44. doi: 10.1016/j.rvsc.2013.02.023
471. Moraveji, Z, Tabatabaei, M, Shirzad Aski, H, and Khoshbakht, R. Characterization of hemolysins of Staphylococcus strains isolated from human and bovine, southern Iran. Iran J Vet Res. (2014) 15:326–30.
472. Adkins, PRF, Middleton, JR, Fox, LK, Pighetti, GM, Petersson-Wolfe, C, and National, MC. Laboratory handbook on bovine mastitis. Third edition ed. New Prague, Minnesota: National Mastitis Council, Inc. New Prague, Minnesota (2017).
473. Monistero, V, Barberio, A, Cremonesi, P, Castiglioni, B, Morandi, S, Lassen, DCK, et al. Genotyping and antimicrobial susceptibility profiling of Streptococcus uberis isolated from a clinical bovine mastitis outbreak in a dairy farm. Antibiotics. (2021) 10:644. doi: 10.3390/antibiotics10060644
474. Rodrigues, NMB, Bronzato, GF, Santiago, GS, Botelho, LAB, Moreira, BM, Coelho, ID, et al. The matrix-assisted laser desorption ionization–time of flight mass spectrometry (MALDI-TOF MS) identification versus biochemical tests: a study with enterobacteria from a dairy cattle environment. Braz J Microbiol. (2017) 48:132–8. doi: 10.1016/j.bjm.2016.07.025
475. Tomazi, T, Gonçalves, JL, Barreiro, JR, Braga, PADC, Silva LF, PE, Eberlin, MN, et al. Identification of coagulase-negative staphylococci from bovine Intramammary infection by matrix-assisted laser desorption ionization–time of flight mass spectrometry. J Clin Microbiol. (2014) 52:1658–63. doi: 10.1128/JCM.03032-13
476. Freney, J, Kloos, WE, Hajek, V, Webster, JA, Bes, M, Brun, Y, et al. Recommended minimal standards for description of new staphylococcal species. Int J Syst Evol Microbiol. (1999) 49:489–502. doi: 10.1099/00207713-49-2-489
477. Dubois, D, Leyssene, D, Chacornac, JP, Kostrzewa, M, Schmit, PO, Talon, R, et al. Identification of a variety of Staphylococcus species by matrix-assisted laser desorption ionization-time of flight mass spectrometry. J Clin Microbiol. (2010) 48:941–5. doi: 10.1128/JCM.00413-09
478. da Motta, CC, Rojas, ACCM, Dubenczuk, FC, Botelho, LAB, Moreira, BM, de Oliveira Coelho, SM, et al. Verification of molecular characterization of coagulase positive Staphylococcus from bovine mastitis with matrix-assisted laser desorption ionization, time-offlight mass spectrometry (MALDI-TOF MS) mass spectrometry. Afr J Microbiol Res. (2014) 8:3861–6. doi: 10.5897/AJMR2014.7071
479. Busanello, M, Rossi, RS, Cassoli, LD, Pantoja, JCF, and Machado, PF. Estimation of prevalence and incidence of subclinical mastitis in a large population of Brazilian dairy herds. J Dairy Sci. (2017) 100:6545–53. doi: 10.3168/jds.2016-12042
480. Middleton, JR, Saeman, A, Fox, LK, Lombard, J, Hogan, JS, and Smith, KL. The National Mastitis Council: a global organization for mastitis control and milk quality, 50 years and beyond. J Mammary Gland Biol Neoplasia. (2014) 19:241–51. doi: 10.1007/s10911-014-9328-6
481. Schalm, OW, and Noorlander, DO. Experiments and observations leading to development of the California mastitis test. J Am Vet Med Assoc. (1957) 130:199–204.
482. Neave, FK, Dodd, FH, Kingwill, RG, and Westgarth, DR. Control of mastitis in the dairy herd by hygiene and management. J Dairy Sci. (1969) 52:696–707. doi: 10.3168/jds.S0022-0302(69)86632-4
483. Ruegg, PL . A 100-year review: mastitis detection, management, and prevention. J Dairy Sci. (2017) 100:10381–97. doi: 10.3168/jds.2017-13023
484. Hillerton, JE, Bramley, AJ, Staker, RT, and McKinnon, CH. Patterns of intramammary infection and clinical mastitis over a 5 year period in a closely monitored herd applying mastitis control measures. J Dairy Res. (1995) 62:39–50. doi: 10.1017/S0022029900033653
485. Blowey, RW In: P Edmondson , editor. Mastitis control in dairy herds. 2nd ed. Cambridge, MA: CABI (2010)
486. Azizoglu, RO, Lyman, R, and Anderson, KL. Bovine Staphylococcus aureus: dose response to iodine and chlorhexidine and effect of iodine challenge on antibiotic susceptibility. J Dairy Sci. (2013) 96:993–9. doi: 10.3168/jds.2012-5857
487. Deluyker, HA, Van Oye, SN, and Boucher, JF. Factors affecting cure and somatic cell count after pirlimycin treatment of subclinical mastitis in lactating cows. J Dairy Sci. (2005) 88:604–14. doi: 10.3168/jds.S0022-0302(05)72724-7
488. Sol, J, Sampimon, OC, Barkema, HW, and Schukken, YH. Factors associated with cure after therapy of clinical mastitis caused by Staphylococcus aureus. J Dairy Sci. (2000) 83:278–84. doi: 10.3168/jds.S0022-0302(00)74875-2
489. Owens, WE, Watts, JL, Boddie, RL, and Nickerson, SC. Antibiotic treatment of mastitis: comparison of intramammary and intramammary plus intramuscular therapies. J Dairy Sci. (1988) 71:3143–7. doi: 10.3168/jds.S0022-0302(88)79915-4
490. Owens, WE, Ray, CH, Watts, JL, and Yancey, RJ. Comparison of success of antibiotic therapy during lactation and results of antimicrobial susceptibility tests for bovine mastitis. J Dairy Sci. (1997) 80:313–7. doi: 10.3168/jds.S0022-0302(97)75940-X
491. Swinkels, J, Cox, P, Schukken, Y, and Lam, T. Efficacy of extended cefquinome treatment of clinical Staphylococcus aureus mastitis. J Dairy Sci. (2013) 96:4983–92. doi: 10.3168/jds.2012-6197
492. Ziv, G, and Storper, M. Intramuscular treatment of subclinical staphylococcal mastitis in lactating cows with penicillin G, methicillin and their esters. J Vet Pharmacol Ther. (1985) 8:276–83. doi: 10.1111/j.1365-2885.1985.tb00957.x
493. Sordillo, LM, Nickerson, SC, and Akers, RM. Pathology of Staphylococcus aureus mastitis during lactogenesis: relationships with bovine mammary structure and function. J Dairy Sci. (1989) 72:228–40. doi: 10.3168/jds.S0022-0302(89)79101-3
494. Erskine, RJ, Wagner, S, and DeGraves, FJ. Mastitis therapy and pharmacology. Vet Clin North Am Food Anim Pract. (2003) 19:109–38, vi. doi: 10.1016/S0749-0720(02)00067-1
495. Owens, WE, and Nickerson, SC. Morphologic study of Staphylococcus aureus L-form, reverting, and intermediate colonies in situ. J Clin Microbiol. (1989) 27:1382–6. doi: 10.1128/jcm.27.6.1382-1386.1989
496. Brouillette, E, Martinez, A, Boyll, BJ, Allen, NE, and Malouin, F. Persistence of a Staphylococcus aureus small-colony variant under antibiotic pressure in vivo. FEMS Immunol Med Microbiol. (2004) 41:35–41. doi: 10.1016/j.femsim.2003.12.007
497. Taponen, S, Dredge, K, Henriksson, B, Pyyhtia, AM, Suojala, L, Junni, R, et al. Efficacy of intramammary treatment with procaine penicillin G vs. procaine penicillin G plus neomycin in bovine clinical mastitis caused by penicillin‐susceptible, gram‐positive bacteria – a double blind field study. J Vet Pharmacol Ther. (2003) 26:193–8. doi: 10.1046/j.1365-2885.2003.00473.x
498. Yancey, RJ, Sanchez, MS, and Ford, CW. Activity of antibiotics against Staphylococcus aureus within polymorphonuclear neutrophils. Eur J Clin Microbiol Infect Dis. (1991) 10:107–13. doi: 10.1007/BF01964421
499. Kerro Dego, O, van Dijk, JE, and Nederbragt, H. Factors involved in the early pathogenesis of bovine Staphylococcus aureus mastitis with emphasis on bacterial adhesion and invasion. Review Vet Q. (2002) 24:181–98. doi: 10.1080/01652176.2002.9695135
500. Constable, PD, and Morin, DE. Treatment of clinical mastitis. Using antimicrobial susceptibility profiles for treatment decisions. Vet Clin North Am Food Anim Pract. (2003) 19:139–55. doi: 10.1016/S0749-0720(02)00068-3
501. USDA APHIS , veterinary services, National Animal Health Monitoring System. Milk Quality, Milking Procedures, and Mastitis on U.S. Daries. 2014, report 2, Fort Collins, CO Available at https://www.aphis.usda.gov/animal_health/nahms/dairy/downloads/dairy14/Dairy14_dr_Mastitis.pdf, accessed on 4/26/2024 (online) (2016).
502. Benti, DG, Getahun, EA, and Oudessa Kerro, D. Antimicrobial usage for the Management of Mastitis in the USA: impacts on Antimicrobial Resistance and Potential alternative Approaches. In: Mastitis in Dairy Cattle, Sheep and Goats. Oudessa Kerro D. editor (2021) 6. doi: 10.5772/intechopen.101533
503. APHIS, USDA . Milk Quality, Milking Procedures, and Mastitis on U.S. Dairies, 2014. Veterinary Services, National Animal Health Monitoring System, Fort Collins, CO. Available at https://www.aphis.usda.gov/animal_health/nahms/dairy/downloads/dairy14/Dairy14_dr_Mastitis.pdf (Accessed April 26, 2024).
504. Shephard, R, Burman, S, and Marcun, P. A comparative field trial of cephalonium and cloxacillin for dry cow therapy for mastitis in Australian dairy cows. Aust Vet J. (2004) 82:624–9. doi: 10.1111/j.1751-0813.2004.tb12610.x
505. Barlow, JW, Zadoks, RN, and Schukken, YH. Effect of lactation therapy on Staphylococcus aureus transmission dynamics in two commercial dairy herds. BMC Vet Res. (2013) 9:28–12. doi: 10.1186/1746-6148-9-28
506. Petzer, I-M, Etter, EMC, Donkin, EF, Webb, EC, and Karzis, J. Epidemiological and partial budget analysis for treatment of subclinical Staphylococcus aureus intramammary infections considering microbiological and cytological scenarios. Prev Vet Med. (2017) 148:66–77. doi: 10.1016/j.prevetmed.2017.10.005
507. Drackley, JK . ADSA foundation scholar award. Biology of dairy cows during the transition period: the final frontier? J Dairy Sci. (1999) 82:2259–73. doi: 10.3168/jds.S0022-0302(99)75474-3
508. Esposito, G, Irons, PC, Webb, EC, and Chapwanya, A. Interactions between negative energy balance, metabolic diseases, uterine health and immune response in transition dairy cows. Anim Reprod Sci. (2014) 144:60–71. doi: 10.1016/j.anireprosci.2013.11.007
509. Pinedo, PJ, Fleming, C, and Risco, CA. Events occurring during the previous lactation, the dry period, and peripartum as risk factors for early lactation mastitis in cows receiving 2 different intramammary dry cow therapies. J Dairy Sci. (2012) 95:7015–26. doi: 10.3168/jds.2012-5398
510. Redfern, EA, Sinclair, LA, and Robinson, PA. Dairy cow health and management in the transition period: the need to understand the human dimension. Res Vet Sci. (2021) 137:94–101. doi: 10.1016/j.rvsc.2021.04.029
511. Williamson, JH, Woolford, MW, and Day, AM. The prophylactic effect of a dry-cow antibiotic against Streptococcus uberis. N Z Vet J. (1995) 43:228–34. doi: 10.1080/00480169.1995.35898
512. USDA APHIS . Veterinary Services Center for Epidemiology and Animal Health. 2008. Antibiotic Use on U.S. Dairy Operations, 2002 and 2007, Info Sheet, Fort Collins, CO. Available at https://www.aphis.usda.gov/animal_health/nahms/dairy/downloads/dairy07/Dairy07_is_AntibioticUse_1.pdf (Accessed on April 26, 2024).
513. Bonsaglia, EC, Gomes, MS, Canisso, IF, Zhou, Z, Lima, SF, Rall, VL, et al. Milk microbiome and bacterial load following dry cow therapy without antibiotics in dairy cows with healthy mammary gland. Sci Rep-Uk. (2017) 7:1–10. doi: 10.1038/s41598-017-08790-5
514. FDA . (2014). Summary Report On Antimicrobials Sold or Distributed for Use in Food-Producing Animals, Available at https://www.fda.gov/media/94906/download (Accessed April 26, 2024).
515. Armstrong, J. Selective dry cow therapy. University of Minnesota Extension 2020, Available at https://extension.umn.edu/dairy-milking-cows/selective-dry-cow-therapy. (Accessed April 26, 2024).
516. Browning, JW, Mein, GA, Brightling, P, Nicholls, TJ, and Barton, M. Strategies for mastitis control: dry cow therapy and culling. Aust Vet J. (1994) 71:179–81. doi: 10.1111/j.1751-0813.1994.tb03383.x
517. Rowe, SM, Godden, SM, Nydam, DV, Gorden, PJ, Lago, A, Vasquez, AK, et al. Randomized controlled non-inferiority trial investigating the effect of 2 selective dry-cow therapy protocols on antibiotic use at dry-off and dry period intramammary infection dynamics. J Dairy Sci. (2020) 103:6473–92. doi: 10.3168/jds.2019-17728
518. Berry, EA, and Hillerton, JE. The effect of selective dry cow treatment on new intramammary infections. J Dairy Sci. (2002) 85:112–21. doi: 10.3168/jds.S0022-0302(02)74059-9
519. Scherpenzeel, CGM, Den Uijl, IEM, Van Schaik, G, Riekerink, RGMO, Hogeveen, H, and Lam, TJGM. Effect of different scenarios for selective dry-cow therapy on udder health, antimicrobial usage, and economics. J Dairy Sci. (2016) 99:3753–64. doi: 10.3168/jds.2015-9963
520. Ekong, PS, Abdelfattah, EM, Okello, E, Williams, DR, Lehenbauer, TW, Karle, BM, et al. 2018 survey of factors associated with antimicrobial drug use and stewardship practices in adult cows on conventional California dairies: immediate post-senate bill 27 impact. PeerJ. (2021) 9:e11596. doi: 10.7717/peerj.11596
521. McParland, S, Dillon, PG, Flynn, J, Ryan, N, Arkins, S, and Kennedy, A. Effect of using internal teat sealant with or without antibiotic therapy at dry-off on subsequent somatic cell count and milk production. J Dairy Sci. (2019) 102:4464–75. doi: 10.3168/jds.2018-15195
522. Rabiee, AR, and Lean, IJ. The effect of internal teat sealant products (Teatseal and Orbeseal) on intramammary infection, clinical mastitis, and somatic cell counts in lactating dairy cows: A meta-analysis. J Dairy Sci. (2013) 96:6915–31. doi: 10.3168/jds.2013-6544
523. Spears, JW, and Weiss, WP. Role of antioxidants and trace elements in health and immunity of transition dairy cows. Vet J. (2008) 176:70–6. doi: 10.1016/j.tvjl.2007.12.015
524. Weiss, WP, Hogan, JS, Todhunter, DA, and Smith, KL. Effect of vitamin E supplementation in diets with a low concentration of selenium on mammary gland health of dairy cows. J Dairy Sci. (1997) 80:1728–37. doi: 10.3168/jds.S0022-0302(97)76105-8
525. FDA . (2018). Summary report on antimicrobials sold or distributed for use in food-producing animals 2018. Available at: https://www.fda.gov/media/133411/ (Accessed April 26, 2024).
526. Lago, A, Godden, SM, Bey, R, Ruegg, PL, and Leslie, K. The selective treatment of clinical mastitis based on on-farm culture results: II. Effects on lactation performance, including clinical mastitis recurrence, somatic cell count, milk production, and cow survival. J Dairy Sci. (2011) 94:4457–67. doi: 10.3168/jds.2010-4047
527. Myllys, V, Honkanen-Buzalski, T, Huovinen, P, Sandholm, M, and Nurmi, E. Association af changes in the bacterial ecology of bovine mastitis with changes in the use of milking machines and antibacterial drugs. Acta Vet Scand. (1994) 35:363–9. doi: 10.1186/BF03548309
528. Pol, M, and Ruegg, PL. Relationship between antimicrobial drug usage and antimicrobial susceptibility of gram-positive mastitis pathogens. J Dairy Sci. (2007) 90:262–73. doi: 10.3168/jds.S0022-0302(07)72627-9
529. Tikofsky, LL, Barlow, JW, Santisteban, C, and Schukken, YH. A comparison of antimicrobial susceptibility patterns for Staphylococcus aureus in organic and conventional dairy herds. Microb Drug Resist. (2003) 9:39–45. doi: 10.1089/107662903322541883
530. Erskine, RJ, Walker, RD, Bolin, CA, Bartlett, PC, and White, DG. Trends in antibacterial susceptibility of mastitis pathogens during a seven-year period. J Dairy Sci. (2002) 85:1111–8. doi: 10.3168/jds.S0022-0302(02)74172-6
531. Makovec, JA, and Ruegg, PL. Antimicrobial resistance of bacteria isolated from dairy cow milk samples submitted for bacterial culture: 8,905 samples (1994-2001). J Am Vet Med Assoc. (2003) 222:1582–9. doi: 10.2460/javma.2003.222.1582
532. De Oliveira, A, Watts, J, Salmon, S, and Aarestrup, FM. Antimicrobial susceptibility of Staphylococcus aureus isolated from bovine mastitis in Europe and the United States. J Dairy Sci. (2000) 83:855–62. doi: 10.3168/jds.S0022-0302(00)74949-6
533. Costa, EO, Benites, NR, Guerra, JL, and Melville, PA. Antimicrobial susceptibility of Staphylococcus spp. isolated from mammary parenchymas of slaughtered dairy cows. J Vet Med B Infect Dis Vet Public Health. (2000) 47:99–103. doi: 10.1046/j.1439-0450.2000.00319.x
534. Erskine, RJ, Cullor, J, and Schaellibaum, M. (2004). Bovine mastitis pathogens and trends in resistance to antibacterial drugs. National Mastitis Council Research Committee Report proceedings of National Mastitis Council 400–414, Available at: http://wwwnmconlineorg/docs/ResPaperpdf (Accessed April 26, 2024).
535. Fessler, AT, Billerbeck, C, Kadlec, K, and Schwarz, S. Identification and characterization of methicillin-resistant coagulase-negative staphylococci from bovine mastitis. J Antimicrob Chemother. (2010) 65:1576–82. doi: 10.1093/jac/dkq172
536. Couto, I, de Lencastre, H, Severina, E, Kloos, W, Webster, JA, Hubner, RJ, et al. Ubiquitous presence of a mecA homologue in natural isolates of Staphylococcus sciuri. Microb Drug Resist. (1996) 2:377–91. doi: 10.1089/mdr.1996.2.377
537. Pereira, UP, Oliveira, DG, Mesquita, LR, Costa, GM, and Pereira, LJ. Efficacy of Staphylococcus aureus vaccines for bovine mastitis: a systematic review. Vet Microbiol. (2011) 148:117–24. doi: 10.1016/j.vetmic.2010.10.003
538. Smith, GW, Lyman, RL, and Anderson, KL. Efficacy of vaccination and antimicrobial treatment to eliminate chronic intramammary Staphylococcus aureus infections in dairy cattle. J Am Vet Med Assoc. (2006) 228:422–5. doi: 10.2460/javma.228.3.422
539. Hwang, CY, Pak, SI, and Han, HR. Effects of autogenous toxoid-bacterin in lactating cows with Staphylococcus aureus subclinical mastitis. J Vet Med Sci. (2000) 62:875–80. doi: 10.1292/jvms.62.875
540. Lin, L, Ibrahim, AS, Xu, X, Farber, JM, Avanesian, V, Baquir, B, et al. Th1-Th17 cells mediate protective adaptive immunity against Staphylococcus aureus and Candida albicans infection in mice. PLoS Pathog. (2009) 5:e1000703. doi: 10.1371/journal.ppat.1000703
541. Middleton, JR, Luby, CD, and Adams, DS. Efficacy of vaccination against staphylococcal mastitis: a review and new data. Vet Microbiol. (2009) 134:192–8. doi: 10.1016/j.vetmic.2008.09.053
Keywords: bovine staphylococcal mastitis, Staphylococcus aureus , non-aureus staphylococci, dairy cow, control, host immune responses, staphylococcal virulence factors, antimicrobial resistance of staphylococci
Citation: Kerro Dego O and Vidlund J (2024) Staphylococcal mastitis in dairy cows. Front. Vet. Sci. 11:1356259. doi: 10.3389/fvets.2024.1356259
Edited by:
Patrizia Nebbia, University of Turin, ItalyReviewed by:
Tom Grunert, University of Veterinary Medicine Vienna, AustriaPiera Anna Martino, University of Milan, Italy
Copyright © 2024 Kerro Dego and Vidlund. This is an open-access article distributed under the terms of the Creative Commons Attribution License (CC BY). The use, distribution or reproduction in other forums is permitted, provided the original author(s) and the copyright owner(s) are credited and that the original publication in this journal is cited, in accordance with accepted academic practice. No use, distribution or reproduction is permitted which does not comply with these terms.
*Correspondence: Oudessa Kerro Dego, QWwtaGF5YW5pQGhvdG1haWwuY29t