- 1Lethbridge Research and Development Centre, Agriculture and Agri-Food Canada, Lethbridge, AB, Canada
- 2Western College of Veterinary Medicine, University of Saskatchewan, Saskatoon, SK, Canada
An increase in chronic, non-responsive bovine respiratory disease (BRD) infections in North American feedlot cattle is observed each fall, a time when cattle are administered multiple antimicrobial treatments for BRD. A number of factors are responsible for BRD antimicrobial treatment failure, with formation of biofilms possibly being one. It is widely accepted that biofilms play a role in chronic infections in humans and it has been hypothesized that they are the default lifestyle of most bacteria. However, research on bacterial biofilms associated with livestock is scarce and significant knowledge gaps exist in our understanding of their role in AMR of the bacterial BRD complex. The four main bacterial species of the BRD complex, Mannheimia haemolytica, Pasteurella multocida, Histophilus somni, and Mycoplasma bovis are able to form biofilms in vitro and there is evidence that at least H. somni retains this ability in vivo. However, there is a need to elucidate whether their biofilm-forming ability contributes to pathogenicity and antimicrobial treatment failure of BRD. Overall, a better understanding of the possible role of BRD bacterial biofilms in clinical disease and AMR could assist in the prevention and management of respiratory infections in feedlot cattle. We review and discuss the current knowledge of BRD bacteria biofilm biology, study methodologies, and their possible relationship to AMR.
1 Introduction
Bacterial biofilms are highly organized communities that can adhere to biotic or abiotic surfaces or thrive as non-surface-attached aggregates (1). They are embedded in a self-produced extracellular matrix (ECM) formed of polysaccharides, nucleic acids, and proteins also known as extracellular polymeric substances (EPS; Figure 1). Compared to free-living (planktonic) bacteria, biofilm communities are known to be more resistant to host immune responses, chemical disinfectants, and other environmental stressors such as antimicrobials (2). It was suggested that planktonic single cells may be a transitory state and that biofilms are the default lifestyle of bacteria (3), which can be 1,000 times more resistant to antimicrobials (4). It is well-established that human chronic infections caused by bacteria are often mediated by biofilms, as it is estimated they are involved in ⁓ 80% of human infections like those caused by Pseudomonas aeruginosa, Staphylococcus epidermidis, Staphylococcus aureus, or Escherichia coli (5, 6). Research on biofilms associated with livestock species is scarce. In Veterinary Medicine, biofilms can play an important role in otitis externa and endometritis in dogs (by Staphylococcus intermedius and P. aeruginosa), mastitis in dairy cattle (mainly caused by Staphylococcus aureus), and pneumonia in swine (Mycoplasma hyopneumoniae) (2). Aside from causing chronic infections, biofilms promote the survival of bacteria within the host until conditions are such that clinical disease ensues (7).
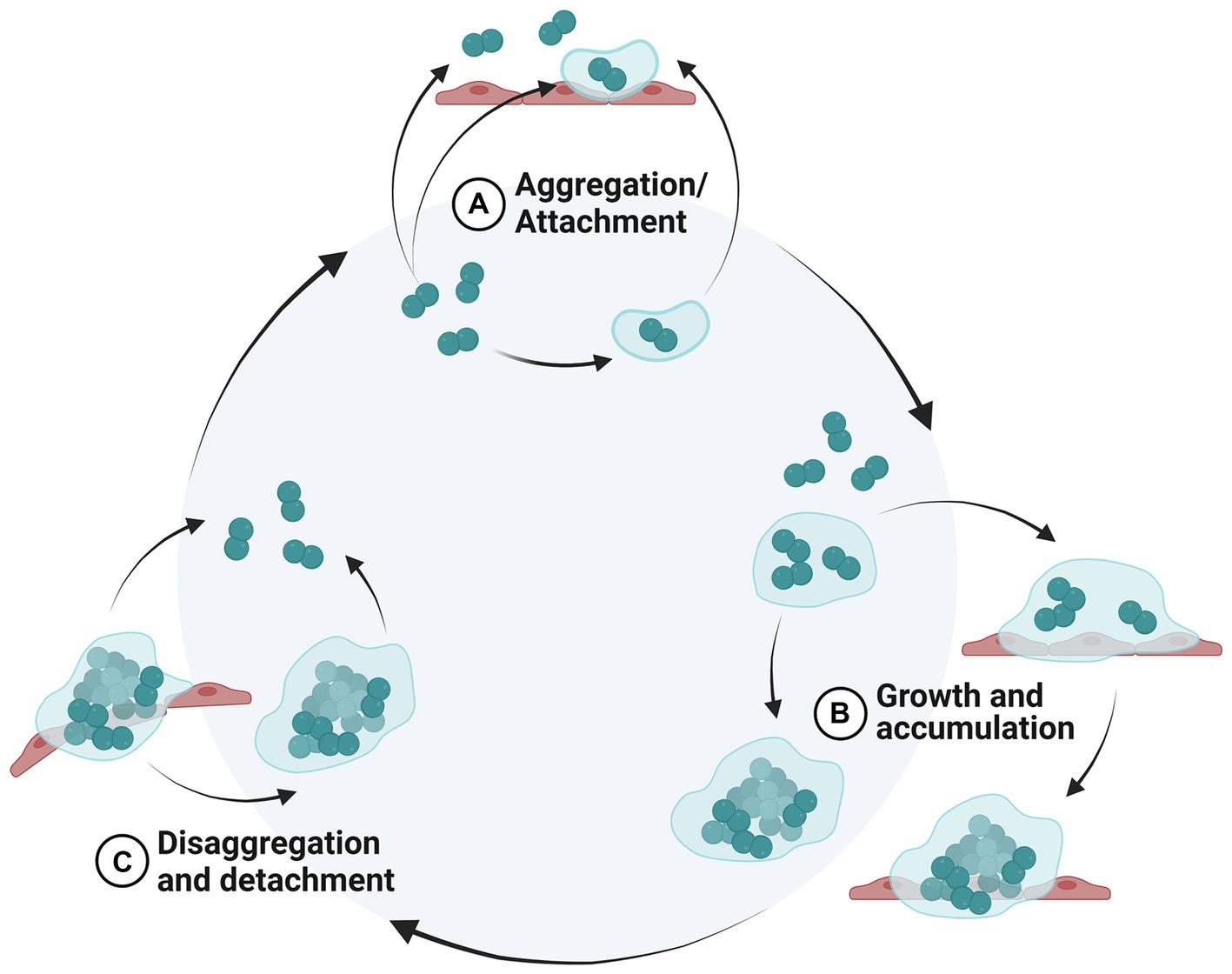
Figure 1. Biofilm life cycle. New proposed model of a biofilm life cycle modified from (1). Inside the light blue circle represents non-surface-attached biofilm aggregates, whereas outside the circle represents the surfaced-attached biofilm. (A) Bacterial cells aggregate or attach to a biotic or abiotic surface, (B) bacterial cell colonies increase in size by cell division (growth) and capturing surrounding cells (accumulation), (C) bacterial cells are sloughed from the biofilm as single cells or aggregates. Created in BioRender.
As the name implies, bovine respiratory disease (BRD) refers to any respiratory disease of cattle, which frequently occurs in young beef and dairy calves. However, BRD is most commonly associated with pneumonia in beef cattle after feedlot arrival, giving rise to it being described as “shipping fever” as clinical symptoms are seen in calves following shipping from ranches and auctions to feedlots (8). Shipping fever is a multifactorial disease with a constellation of risk factors such as inclement weather, management practices, and microbial pathogens contributing to pathogenesis. Aside from viruses (bovine herpes virus 1, bovine virus diarrhea virus type 1 or type 2, bovine respiratory syncytial virus, and parainfluenza virus 3), the bacteria most commonly associated with BRD include the Pasteurellaceae spp. (Mannheimia haemolytica, Pasteurella multocida, Histophilus somni) and Mycoplasma bovis (8). Two additional bacterial species, Bibersteinia trehalosi and Trueperella pyogenes, are less frequently associated with BRD in North America (9). BRD accounts for 70–80% of feedlot morbidities and 40–50% of total feedlot mortalities, costing the North American feedlot cattle industry over 3 billion $ per year (10). Despite the advent of new antimicrobials and vaccines, and the existence of pre-conditioning programs, the burden BRD poses on the North American feedlot industry has remained largely unchanged over the last 45 years (11). The epidemiology of BRD is well-known, and hence metaphylaxis is frequently administered to calves upon feedlot arrival with the aim of mitigating the incidence and severity of BRD. Pasteurellaceae infections usually respond favorably if antimicrobials are administered during the early stages of BRD (12, 13). But during BRD peak season (the fall in Canada and the US), there is an increase in chronic respiratory infections, which strains feedlot resources (i.e., chronic pen capacity, personnel availability and morale) and presents an animal welfare concern and an economic burden (13). One of the challenges of BRD is its polymicrobial nature since different BRD bacteria and/ or viruses can coexist within the same calf (14, 15). Chronically infected cattle frequently have a history of receiving multiple antimicrobials, a practice that can select for antimicrobial-resistant (AMR) bacteria, which in turn could lead to a reduced therapeutic efficacy (16). Aside from AMR, there are additional factors that may contribute to BRD antimicrobial treatment failure such as viral infection, advanced state of the disease, insufficient antimicrobial concentration reached at the site of infection, antimicrobial handling/ storage under sub-optimal conditions, and possibly biofilms (Figure 2) (4, 13). To date, only H. somni biofilms have been confirmed to occur in vivo in cattle with clinical disease (17), whereas further research is required to determine if M. haemolytica and P. multocida form biofilms within the respiratory tract (18, 19). Mycoplasma bovis causes severe chronic damage to the lungs of calves and is responsible for chronic pneumonia and polyarthritis syndrome (CPPS) in feedlot cattle (20). M. bovis is capable of forming prolific in vitro biofilms (21) that readily form on inert surfaces and dead tissue (22). Therefore, biofilm formation, combined with antimicrobial resistance, could be a major player in the chronic nature of M. bovis infections. Regarding B. trehalosi and T. pyogenes biofilms, to date, no peer-reviewed literature is available related to the feedlot cattle respiratory tract.
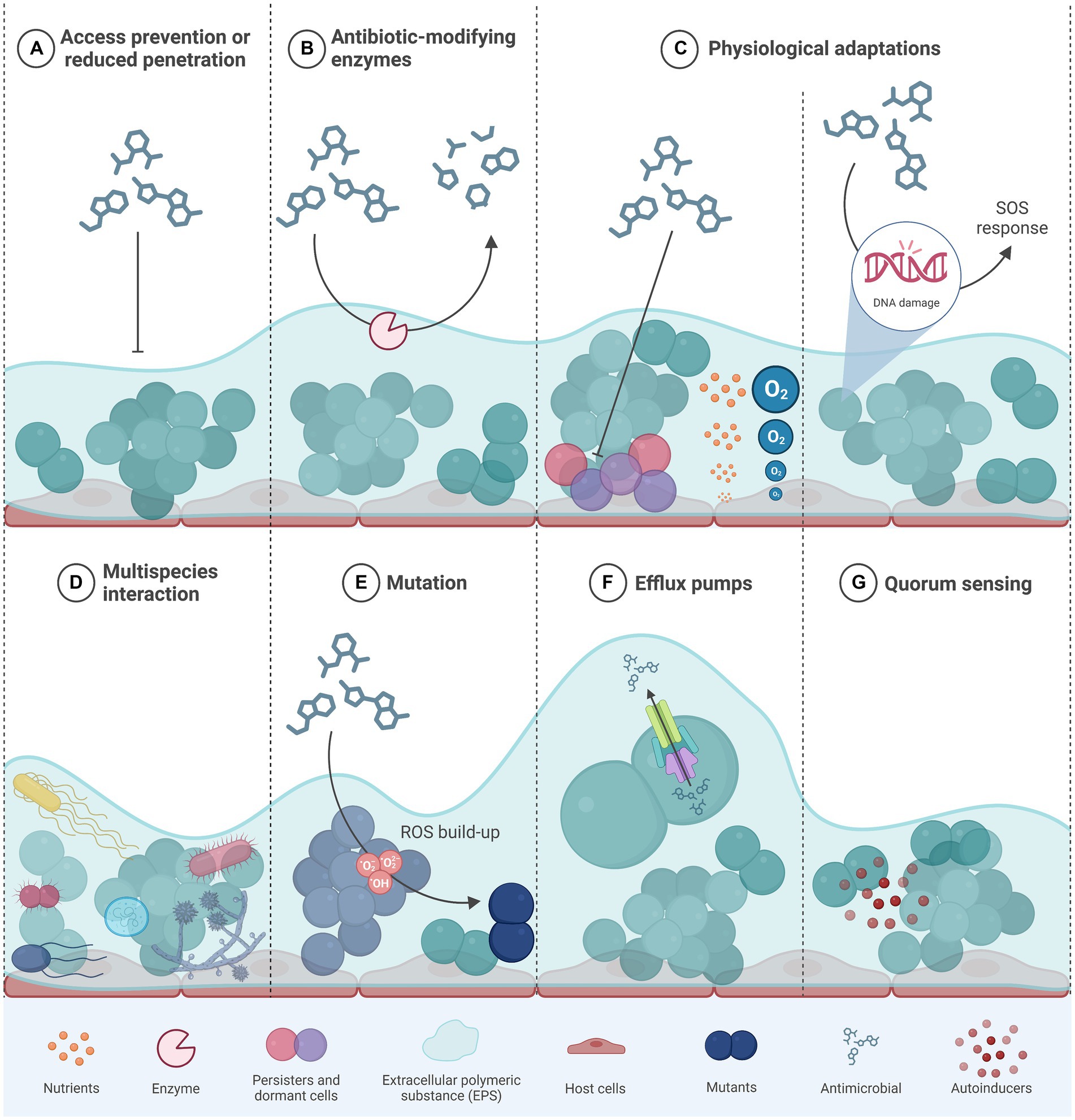
Figure 2. Different mechanisms that confer antibiotic resistance and/ or tolerance to bacteria within a biofilm. Modified from (4). (A) the components of the extracellular matrix form a physical barrier to diffusion of antimicrobials; (B) the presence of antibiotic-modifying enzymes secreted by some bacteria within the biofilm provides protection to both antimicrobial resistant and susceptible cells; (C) biofilms generate concentration gradients of nutrients or oxygen that promote the emergence of dormant cells and/or persister cells within the deeper biofilm layers; (D) through quorum sensing, bacterial cells from different species communicate so as to trigger physiological responses that decrease susceptibility to antimicrobials; (E) antimicrobials that target DNA trigger the SOS response upon DNA damage that ultimately confers AMR through other mechanisms such as mutation; (F) some efflux pumps are capable of transporting antimicrobials outside of the bacterial cell, thus preventing their deleterious effects; (G) quorum sensing bacterial cell–cell communication systems are involved in the synthesis and structure of biofilms and in cellular responses that can confer AMR; AMR, antimicrobial resistance; eDNA, extracellular DNA; ROS, reactive oxygen species. Created in BioRender.
To what extent biofilms may play a role in BRD is largely unknown (2, 17–19). A better understanding of the possible role of BRD bacterial biofilms in clinical disease could assist in the prevention and management of respiratory infections in feedlot cattle. Considering that the North American cattle industry is moving toward precision metaphylactic use of antimicrobials (AMU) in calves1, an understanding of the role biofilms in AMR and BRD infections is imperative.
2 Search strategy
In July 2023, a search building strategy was followed through the medical subject headings (MeSH) at the PubMed website (accessed on July 3rd, 2023),1 to systematically identify peer-reviewed articles about BRD bacteria biofilms. The following searches were carried out: (“Biofilms”[Mesh]) AND “Bovine Respiratory Disease Complex”[Majr]; (“Biofilms”[Mesh]) AND “Pasteurella multocida”[Mesh]; (“Biofilms”[Mesh]) AND “Mannheimia haemolytica”[Mesh]; (“Biofilms”[Mesh]) AND “Haemophilus somnus”[Majr] (Histophilus somni); (“Biofilms”[Mesh]) AND “Mycoplasma bovis”[Majr]; (“Biofilms”[Mesh]) AND “Trueperella pyogenes”[Mesh]; (“Biofilms”[Mesh]) AND “Arcanobacterium pyogenes”[Mesh] (“Biofilms”[Mesh]) AND “Actinomyces pyogenes”[Mesh] (“Biofilms”[Mesh]) AND “Corynebacterium pyogenes”[Mesh] (“Biofilms”[Mesh]) AND “Bibersteinia trehalosi”[Mesh].
Additionally, the following keyword terms were searched in (non-MESH) PubMed and Scopus (accessed on July 3rd 2023;2 only articles published in English were considered): Bovine respiratory disease biofilm; Pasteurella multocida biofilm; Mannheimia haemolytica biofilm; Histophilus somni biofilm; Mycoplasma bovis biofilm; Trueperella pyogenes biofilm; Arcanobacterium pyogenes biofilm; Actinomyces pyogenes biofilm; Corynebacterium pyogenes biofilm, and Bibersteinia trehalosi biofilm. Any peer-reviewed manuscripts cited in the literature identified by the aforementioned searches that addressed the biology of BRD bacterial biofilms were also included.
As a systematic review, all the articles identified by the described methodology were included. Those articles addressing BRD-related bacterial biofilms in non-bovine hosts were included as well, e.g., P. multocida associated with fowl cholera or respiratory infections in swine. In total, 46 BRD biofilm studies were included.
3 Biofilm biology in BRD bacteria
3.1 Mannheimia haemolytica
Morck et al. observed glycocalyx-encased microcolonies of Gram-negative bacteria with morphology compatible with M. haemolytica (Pasteurella haemolytica at the time) in lung tissue from calves with fibrinous pneumonia (16). M. haemolytica was isolated from the diseased lung tissue, but the bacterial species contained in microcolonies were not confirmed. Subsequently, Olson et al. (23) documented higher AMR in M. haemolytica biofilms compared to planktonic cells, an observation supported by a later study (24). The first scanning electron microscopy images of M. haemolytica biofilms were published in 2011 (25) and in 2015, Boukahil and Czuprynski demonstrated that M. haemolytica formed protein-rich biofilms on plastic surfaces (24). Among the proteins identified, OmpA, OmpP2 (an amyloid-like protein) and OmpH (a porin protein) were particularly prevalent (26, 27).
Boukahil et al. showed that M. haemolytica formed biofilms on fixed primary bovine epithelial cells and that mucin inhibited biofilm formation (28). The formation of biofilms in vitro was also greater for M. haemolytica isolates from the deep lung of pneumonic cattle as compared to those obtained from the nasopharynx of healthy cattle (29). However, M. haemolytica isolates used in this study were not serotyped to confirm if they were either A2 or A1, which are more likely to be associated with healthy versus diseased calves, respectively. The same study demonstrated that stress-induced molecules in cattle (norepinephrine, epinephrine, and substance P, a small peptide belonging to neurokinins) promoted biofilm dispersion. It is widely accepted that stress caused by the transportation of calves to feedlots can promote the proliferation of M. haemolytica serotype A1 in the upper respiratory tract, increasing the risk of it flowing to the lower respiratory tract where it can cause disease (29). Therefore, it was hypothesized that these 3 stress-related elements could promote the disruption of M. haemolytica biofilms and the movement of this pathogen into the lower respiratory tract.
3.2 Histophilus somni
Histophilus somni causes fibrino-suppurative bronchopneumonia and septicemia as well as polyarthritis, myocarditis, pericarditis, pleuritis, and laryngitis in feedlot cattle (17). It is capable of forming biofilms both in vitro and in vivo (17, 30–33). It is speculated that commensal and pathogenic H. somni strains form biofilms that differ in architecture and shape. Previous studies reported that a commensal strain formed a thin, heterogeneous, and filamentous biofilm whereas a pathogenic strain formed thick, homogenous, mound-shaped microcolonies encased in an amorphous extracellular matrix with prominent water channels (30). The EPS of H. somni biofilms is a branched mannose polymer with occasional terminal galactose residues whose synthesis is enhanced in the presence of increased concentrations of salt or with anaerobiosis (34). In vivo H. somni biofilms were detected in the cardiopulmonary tissue of cattle after experimental challenge, supporting their role in pathogenesis (17). Others have described H. somni biofilms on bovine myocardial and brain microvascular endothelium, suggesting they play a role in the pathogenesis of H. somni mediated heart and brain infections (32).
Different molecular processes that have an impact on H. somni biofilm formation have been described in the literature. Universal stress proteins (Usp) are ubiquitously related to stress responses and adaptation in bacteria. The UspE protein is thought to be a global regulator in H. somni and is associated with biofilm formation, EPS production, and lipooligosaccharide (LOS) truncation (35). A H. somni mutant strain 2,336::TnuspE failed to produce biofilms in vitro and was EPS deficient when grown on glass coverslips in well plates (35). Comparative transcriptomics of mutant and wildtype strains grown under planktonic and biofilm states revealed that 181 genes were differentially expressed between the two cell forms. Quorum sensing (QS) plays an important regulatory role in biofilm formation in many bacteria. The luxS gene encodes an enzyme involved in the biosynthesis of AI-2, a QS small molecule thought to be a universal signaling component in bacteria. However, luxS H. somni mutants showed no impaired biofilm formation compared to a wildtype H. somni strain (35), suggesting that luxS may not play a role the formation of biofilms by H. somni. Small RNAs (sRNAs) primarily have post-transcriptional gene regulatory functions in bacteria; sRNAs HS9 and HS97 were shown to interact with the transcription factor sigma 54, which may influence biofilm formation and other functions in H. somni (23).
3.3 Pasteurella multocida
There are 5 serogroups of P. multocida based on their capsular type or capsular polysaccharide (CPS): A, B, D, E and F. Like M. haemolytica, P. multocida biofilm formation has been demonstrated in vitro but only indirectly in vivo in cattle (19, 36–40). The exopolysaccharide (ExPS; biofilm matrix) of P. multocida type A mainly consists of glycogen and proteins, but the protein fraction in P. multocida biofilms maybe less prominent than in other bacterial species (7, 40). The amount of capsule synthesis in P. multocida appears to be inversely related to its biofilm formation capabilities, a trait that does not seem to be limited to any specific serogroup and can be reversed through mutagenesis or in vitro passage (7). Capsular inhibition of biofilm formation is presumably a consequence of the blockage of proteins essential for adhesion to surfaces (7). Petruzzi et al. proposed a P. multocida transmission model for fowl cholera in which biofilms result in chronic infections, whereas planktonic P. multocida was associated with acute, symptomatic infections (7, 19). However, it is unknown if this model is applicable to P. multocida infections in cattle.
Several studies have identified specific genes in P. multocida that are inovled in cell adhesion and biofilm formation. A complete cluster of seven tad genes (tadABCDEFG; tad locus) related to nonspecific adherence in Actinobacillus actinomycetemcomitans were found to be highly conserved in a P. multocida isolate (41). In P. multocida strain HB03 (GenBank accession no. CP00332), the tad locus contained the 14 intact genes that this locus typically harbors, i.e., flp1-flp2-tadV-rcpCAB-tadZABCDEFG (42). However, the specific involvement of tad genes in biofilm formation in P. multocida requires further evaluation as they are not conserved across all strains (38, 39, 42–45). The csrA gene is involved in glycogen synthesis and as a result, it plays a role in biofilm formation (7). Other P. multocida genes with possible direct or indirect biofilm regulation roles include xylB, sgbU, hexD, aspA, comE, qseC, and pmorf0222 (7, 43, 46–48). Of special interest is the qseC gene and its role in QS modulating the transcription of thousands of genes. This highlights that biofilm formation in P. multocida may be a complex biological process, that is likely influenced by major stress-responding molecular mechanisms (47).
3.4 Mycoplasma bovis
Mycoplasma bovis is considered one of the most pathogenic Mycoplasma species of cattle, causing a broad spectrum of diseases that includes arthritis, genital disorders, mastitis, pneumonia, keratoconjunctivitis, and otitis media (49). Due to genome erosion, mycoplasmas have lost a substantial number of genes including some essential for cell survival and those related to cell wall synthesis (degenerative evolution). Therefore, they have a limited ability to synthesize macromolecules needed for cell growth (50). As a consequence, most mycoplasmas have adopted a parasitic, facultative intracellular symbiotic life style where they depend on their host to provide fatty acids, nucleic acid precursors, lipid precursors, amino acids, and vitamins (51, 52). However, some studies have reported that M. bovis can survive in environmental samples for up to 8 months or after surface washing and disinfection (53–56), which suggest that it may form biofilms in these environments.
Eighty-one different isolates from 11 different mycoplasma species of veterinary interest were tested for their ability to form biofilms (21). These included M. bovis, M. mycoides subsp. mycoides, and Mycoplasma spp. bovine group 7. Differences in biofilm formation were observed, both across and within species. Some M. bovis isolates formed prolific biofilms, while others were less abundant. These findings support the hypothesis that M. bovis did not lose its ability to form biofilms as a result of degenerative evolution, which may speak to the importance of its role in bacterial survival. A correlation between the expression of specific variable surface proteins (Vsp; related to adherence) and biofilm capabilities have been described in M. bovis (21). Moreover, M. bovis biofilms showed more resistance to heat and desiccation, but not to antimicrobials like oxytetracycline, danofloxacin, and enrofloxacin (21). However, heat and desiccation were tested using 48-h old biofilms whereas resistance to antimicrobials was assessed using 24-h old biofilms, raising the possibility that biofilm maturity may have influenced responses.
Chen et al. used the whole-cell protein fraction in an immunoproteomics study to characterize the host response to M. bovis infections and identify new vaccine and diagnostic targets. Convalescent sera samples were obtained from calves experimentally infected with M. bovis. Six immunoreactive proteins specific to biofilms, but not to planktonic cells were detected, with most being of cytoplasmatic origin (57). The detection of biofilm antigens in convalescent sera provided indirect evidence of the involvement of biofilms in M. bovis infections.
3.5 Trueperella pyogenes and Bibersteinia trehalosi
In North America, the identification of these two bacterial species in BRD studies is limited (9), presumably due to their infrequent involvement in clinical BRD. The search strategies defined in the M&M section identified no publications related to B. trehalosi biofilms. Regarding T. pyogenes, several biofilm peer-reviewed studies were identified; however, none of them included T. pyogenes isolates originating from the respiratory tract of feedlot cattle.
Trueperella pyogenes chronic infections have been associated with biofilms in mastitic and metritic dairy cows and are thought to contribute to unresponsiveness of these conditions to antimicrobial therapy (58, 59). Even though T. pyogenes isolated from infections in dairy cows and forest musk deer has been shown to form biofilms in vitro (58, 60–62), in vivo biofilm formation has yet to be formally demonstrated. Only one study reported the in vivo formation of T. pyogenes biofilms in mice (60). However, biofilms were detected on hydro-gel contact lenses (i.e., surgically introduced in the murine reproductive system before T. pyogenes challenge) rather than on the uterine tissue. It has been reported that P. aeruginosa and E. coli may inhibit T. pyogenes in vitro biofilm production through the production of QS signal molecules, which may explain the progression toward dominance of these bacterial species in the abscesses of musk deer (63). Studies related to the molecular regulation of T. pyogenes biofilms found that the expression of the virulence factors hemolytic exotoxin pyolisin (PLO) and TatD DNases are linked to biofilm formation (58, 64). The ploS/ploR genes from the two-component regulatory system, and the luxS gene may also play a role in the formation of biofilms by T. pyogenes (65).
4 Polymicrobial BRD biofilms
Not many studies have addressed the polymicrobial nature of BRD infections in biofilm experiments. Mannheimia haemolytica and P. multocida inhibited biofilm formation by contact-dependent inhibition, a type of inhibition that is mediated by cell adhesion of one species to another (66). In contrast, in vivo H. somni biofilm studies revealed the presence of Pasteurella multocida in the respiratory tract of calves, suggesting the existence of polymicrobial BRD bacteria biofilms (17). Later, a possible synergistic relationship between H. somni and P. multocida in biofilm formation was described (40). In this study, it was observed that when both species were grown together in vitro, the average biomass and biofilm thickness, and the total carbohydrate and protein content of the biofilm were greater than in single-species biofilms. It was suggested that H. somni cells interact with P. multocida cells to enhance aggregation and the formation of polymicrobial biofilms. Furthermore, gene expression in P. multocida was altered in a manner that increased its persistence and ability to contribute to the formation of multi-species biofilms with H. somni. When two 18-month old calves were challenged with H. somni and sera was collected, Petruzzi et al. detected a more robust (bovine) host immune response (ELISA) to biofilms compared to planktonic cells of H. somni or P. multocida. These experiments highlighted the possible role of polymicrobial biofilms in BRD infections (39). The detection of P. multocida while studying H. somni biofilms was a serendipitous but feasible finding, as the three Pasteurellaceae species have common nutritional requirements (17, 40, 66). The lack of detection of M. bovis in these multi-species biofilms could be a reflection of its unique nutritional requirements. Different culture conditions may be required to determine if the 4 main BRD bacterial species can all reside within multi-species biofilms.
Studies on the responses of polymicrobial BRD biofilms to antimicrobials are lacking. Polymicrobial biofilms can show higher recalcitrance to antimicrobials through a number of mechanisms including enzymatic inactivation, changes in the expression of antimicrobial resistance genes (ARG), exchange of ARGs among members within the biofilm, inhibition of electron transport or altered membrane fluidity (67). In the case of the Pasteurellaceae, horizontal gene transfer is of particular interest due to the presence of integrative and conjugative elements (ICE) and other mobile genetic elements that readily promote the exchange of ARGs among members possibly within a biofilm community. Pasteurellaceae ICE can contain up to 12 different ARGs that can be transferred from one bacterial cell to another in a single event (68). A polymicrobial BRD biofilm could represent a scenario where Pasteurellaceae species coexist as a community in close proximity, thereby dramatically increasing the opportunity for exchange of ARGs.
5 How biofilms affect BRD antimicrobial treatment
The Calgary Biofilm Device (CBD) (69) was used to evaluate the susceptibility of H. somni, M. haemolytica, P. multocida, and T. pyogenes (Arcanobacterium pyogenes at the time) biofilms to several antimicrobials in vitro (36). The isolates tested originated from field cases of cattle with BRD. Interestingly, free-living and sessile P. multocida and H. somni cells showed similar sensitivities, whereas M. haemolytica and T. pyogenes biofilms were less sensitive than planktonic cells to 4/7 and 7/7 antimicrobials, respectively. Boukahil and Czuprynski also reported that M. haemolytica (a single isolate from a pneumonic bovine lung) biofilms exhibited increased resistance to 5 different antimicrobials (chlortetracycline, erythromycin, gentamycin, tulathromycin, and florfenicol) as compared to planktonic cells (28). The authors also noted that it required higher concentrations of antimicrobials to eradicate biofilms (MBEC) formed on epithelial cells as compared to polystyrene. It is possible that differences in methodology may account for the variability in antimicrobial sensitivity, as the medium used to grow bacteria can interfere with the architectural formation and density of biofilms (4, 29).
Another study investigated the possible relationship between the tilmicosin (TIL) MIC of M. haemolytica and P. multocida with BRD outcome after TIL treatment (70). The bacterial isolates were obtained from the upper respiratory tract and only those samples that provided either M. haemolytica or P. multocida were included. Among calves that had M. haemolytica or P. multocida with a susceptible TIL-MIC, only 61.0 and 63.6% responded to TIL treatment, respectively. Whether biofilms played a role in TIL treatment failure was not investigated.
When a bacterial biofilm is involved in a clinical infection, antimicrobial treatment typically kills planktonic cells, but fails to kill all cells that reside within the biofilm matrix. This may generate a transient relief of symptoms followed by the recurrence of clinical symptoms as new bacterial cells detach from the biofilm and reinitiate infection (22). Most standard antimicrobial susceptibility testing of bacteria is carried out using planktonic cells (71). Some have proposed that pneumonic pasteurellosis responds favorably to antimicrobials when treated at the onset of the disease, whereas pneumonia caused by H. somni or M. bovis does not (72). Others have reported an overall BRD antimicrobial treatment success rate of 70% (73, 74). Although biofilms may contribute to treatment failure, other factors such as interactions between the antimicrobials administrated, the method of administration, host, and the environment all likely play a role in the success or failure of antimicrobial therapy (73).
Non-attached aggregate biofilms (also known as microcolonies) have been observed in human open wounds, the lungs of cystic fibrosis patients, otitis media infections, and in soft tissue fillers. These aggregates have also been observed in vitro in liquid media used to grow P. aeruginosa (75). Interestingly, biofilm aggregates can exhibit similar phenotypic characteristics to that of surface-attached biofilms such as higher AMR (75). Susceptibility testing standards recommend to incubate planktonic cultures of M. haemolytica, P. multocida, H. somni, and T. pyogenes in microdilution for 18–24 h (71, 76). For M. bovis and B. trehalosi, there is no current antimicrobial susceptibility test (AST) standards (76), and different incubation times ranging from 48 to 96 h have been reported in the literature for M. bovis. Whether BRD bacteria form biofilm aggregates in broth during the aforementioned incubation times for AST is unknown.
6 Methodologies employed to study bacteria biofilms
Different growth media can influence biofilm architecture and its response to antimicrobials (4). For instance, Boukahil et al. reported higher MBECs for biofilms grown in the presence of primary bovine bronchial epithelial cells (BBEC) compared to those grown in 24-well polystyrene plates (28). Incubation time can impact maturity and growth of the biofilm, with differences occurring across bacterial species. Currently, there are numerous protocols that have been employed to assess the ability of BRD bacteria to form biofilms (Supplementary Table 1). This makes it challenging to compare results across studies. However, some common methodology features have been employed (Supplementary Table 1). With M. haemolytica, incubation times of at least 36 h are needed to form robust biofilms (24). With a few exceptions, at least 48 h of incubation are needed to form P. multocida biofilms. Likewise, H. somni biofilms require at least 48 h to form and shaking during incubation should be avoided to minimize aeration (30, 40). Regarding T. pyogenes, there seems to be good agreement about the media to use to grow biofilms (BHI supplemented with fetal bovine serum) for at least 24 h. Most of the in vitro biofilm studies with BRD bacteria conducted to date have investigated the formation of biofilms on abiotic surfaces like polystyrene plates, glass coverslips or tubes as opposed to eukaryotic cells (Figure 3).
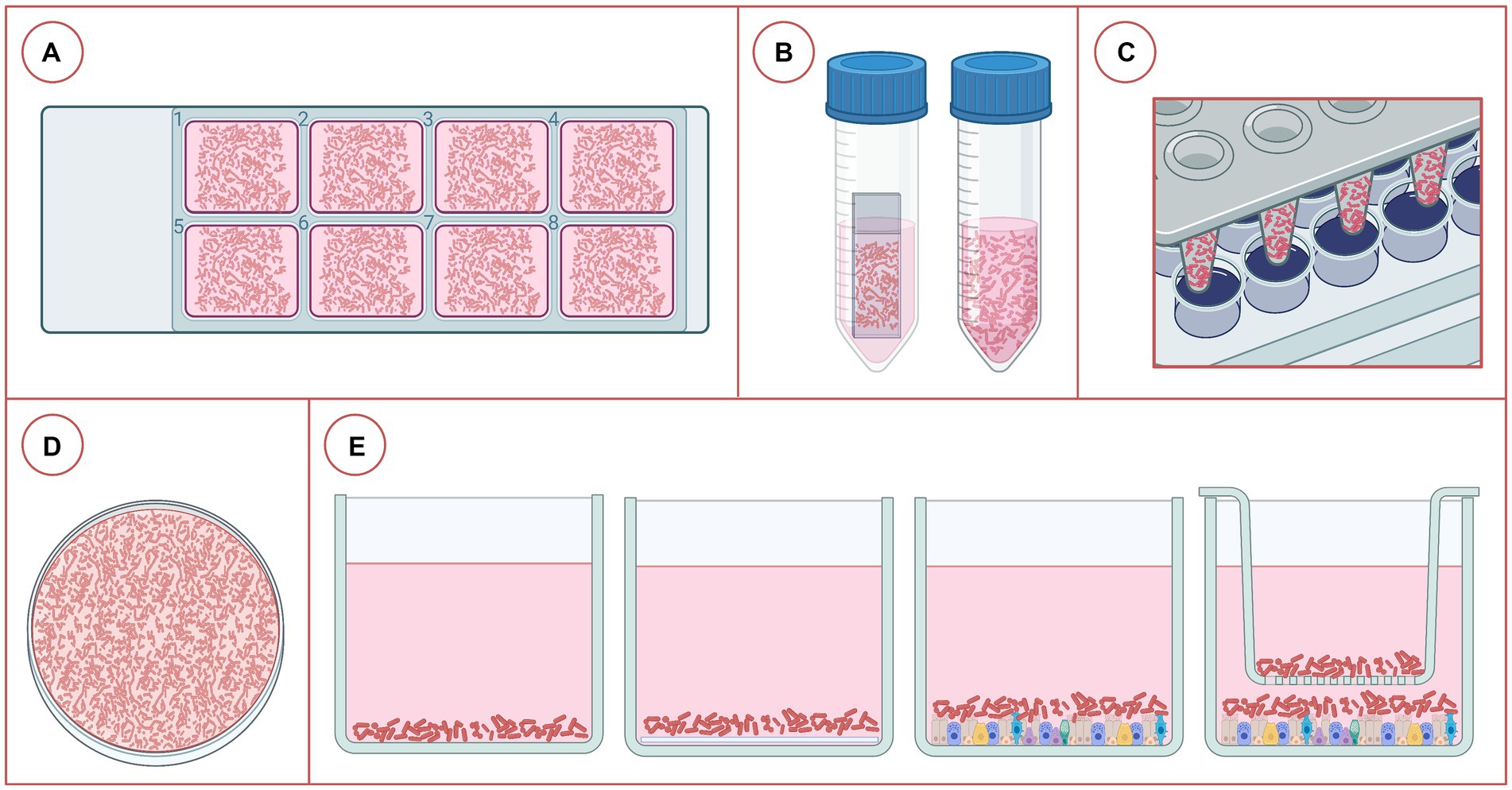
Figure 3. Different platforms used for the study of bacterial biofilms associated with bovine respiratory disease. (A) Eight-chamber cover glass slides, (B) coverslip in a tube or just the tube with growth medium, (C) Calgary Biofilm Device, (D) petri dish containing liquid medium, (E) plates with 6, 24, 48, or 96 wells, from left to right: just liquid medium, liquid medium + coverslip, medium + fixed eukaryotic cells (2D culture), and medium + fixed eukaryotic cells + transwell (2D culture). Created in BioRender.
Other less common approaches to studying BRD bacteria biofilms were found in the literature. The Calgary Biofilm Device (CBD) consists of pegs attached to a lid that fits inside a 96-well microplate containing growth medium that can be inoculated with bacteria, enabling high throughput antimicrobial susceptibility testing (AST; Supplementary Table 1; Figure 3). In another study, BBECs were fixed in tissue culture wells to prevent their detachment as result of the accumulation of bacterial end products after 12 h of incubation (28). This method had minimal effect on epithelial cells or tertiary protein structure, making it suitable for biofilm studies. The authors also fixed BBECs in joined wells (66) to investigate the impact that competitive inhibition may have on the formation of biofilms by M. haemolytica and P. multocida (66). Lastly, polymicrobial biofilms have been used to study M. haemolytica – P. multocida and H. somni – P. multocida interactions (40, 66), but surprisingly few of these studies have been undertaken even though BRD is clearly a polymicrobial disease.
Aside from the medium and incubation time used to grow biofilms, the presence of mammalian cells to mimic the natural host environment also influences biofilm characteristics. With regard to BRD bacteria, most biofilm studies have grown bacteria in a variety of broth media, and only in a couple of instances have they been formed using a submerged tissue system (STC) (28, 66). However, STCs have limitations in the study of host-pathogen interactions because they lack the diversity of cell types present in host tissues (77).
Air-liquid interface (ALI) systems are an alternative to STCs, but presently they have only be used to elucidate host-pathogen interactions for M. haemolytica, not to specifically investigate the impact of biofilms on AMR (66, 77). ALIs allow for cell differentiation and the formation of tight junctions, which are more representative of host tissues, but still have some limitations. For example, they do not fully represent the eukaryotic tissue architecture and the differentiation of eukaryotic cells requires prolonged incubation periods (14–42 d) (77). Ideally, the assembly of 3D cell culture systems or airway organoids, combined with multi-bacterial species approaches could provide new insight into the possible impact of BRD bacteria biofilms on antimicrobial susceptibility. The development of 3D bovine airway organoid models would provide a more accurate representation of the architecture, composition, and environment of host tissues (77).
In vivo detection, direct or indirectly, of BRD bacterial biofilms has been reported in the literature (17, 18, 32, 40). Additionally, there is direct evidence supporting the existence of in vivo P. multocida biofilms in pulmonary tissue from chickens suffering from induced-chronic avian cholera (19). Different techniques have been employed with different tissues to verify biofilm formation including; histopathology, crystal violet (CV) staining, fluorescein isothiocyanate (FITC)-conjugated Griffonia simplicifolia lectin (GS-II), fluorescent in situ hybridization (FISH), and transmission (TEM) and scanning electron microscopy (SEM: Supplementary Table 1).
7 Treatments targeting BRD bacterial biofilms
As new technologies are developed and improved, so are novel approaches to fight biofilm recalcitrant infections. An example is the use of CRISPR-Cas to prevent the formation of E. coli biofilms in urinary catheters (78). In this work, genes related to bacterial cell adhesion, QS, and biofilm formation were targeted by CRISPR-Cas9, resulting in a reduction in biofilm formation. Likewise, the use of regulatory micro RNAs (miRNA) has the potential to enhance the ability of antimicrobials to control biofilms. Human airway epithelial cells (AEC) secret miRNA in extracellular vesicles (EV) that have been shown to decrease P. aeruginosa biofilm formation and reduce resistance to beta-lactam antibiotics (79). A comprehensive review of the current strategies that are being explored to combat biofilms is beyond the scope of this article, but Uruen et al. provides an excellent overview. Within the context of AMR, studies addressing anti-biofilm forming strategies for the bacteria involved in BRD are limited, with only three peer-reviewed articles being identified (80–82).
Aside from causing suppurative bronchopneumonia in cattle, P. multocida is the causative agent of atrophic rhinitis in swine. Both mycophenolate mofetil and indocyanine green inhibited biofilm formation by P. multocida serotypes A and D in swine, but were ineffective against preformed biofilms (80). In this scenario, these molecules could possibly be used to prevent the formation of biofilms (80). However, the authors did not elaborate on the possibility of these compounds adversely effecting animal health or their likelihood of meeting the requirement for regulatory approval. Another study evaluated the biofilm-killing properties of several essential oils on bacteria related to porcine respiratory infections (81). However, P. multocida was not included in the experiment based on the authors assumption it was not capable of forming strong biofilms. However, as outlined above, P. multocida has been shown to form biofilms in vitro. Considering that thyme and winter savory oils disrupted stablished Streptococcus suis and Actinobacillus pleuropneumoniae biofilms, such additives may have value in P. multocida biofilms, but are likely to be more relevant altering intestinal as opposed to respiratory microbiomes. A third study described the biofilm inhibitory properties of two different ethanol extracts which were shown to be effective against P. multocida with minimal negative effects on host tissues (82).
Regarding T. pyogenes, the Phage vB_EcoM-UFV13 (UFV13 in short) was effective in reducing T. pyogenes (isolated from dairy cattle) cell adhesion and decreasing biofilm formation (58). Nanomaterials are emerging as potential antimicrobial agents. In a different study, silver nanoparticles (AgNP) targeting multidrug resistant T. pyogenes (dairy cattle clinical endometritis) decreased bacterial cell viability and biofilm formation by increasing oxidative stress (83). More recently, luteolin, a natural flavonoid found in plants, inhibited T. pyogenes biofilm-formation in vitro and was able to disperse pre-formed biofilms by decreasing TatD DNases binding to extracellular DNA (84). Additionally, luteolin significantly reduced clinical symptoms in a rat endometritis model caused by T. pyogenes (60). However, the in vivo presence of T. pyogenes biofilms was demonstrated on contact lenses pre-introduced in the rat uterus rather than on the uterus tissue itself.
8 Conclusion and future directions
The field of BRD bacteria biofilms has significant knowledge gaps. Further research would be of interest for the livestock industry considering their potential to increase AMR in BRD bacteria (9) and to enhance the exchange of ARGs (14, 15). The in vivo study of BRD bacterial biofilms in clinically healthy and ill calves would help define their role in pathogenesis (whether during chronic infection, opportunistic state in healthy calves, or both) and possibly shed insight into improved approaches for antimicrobial therapy.
Bacterial aggregate biofilms are either free-floating or embedded in the host tissues and present the traditional (surfaced-attached) biofilm mode of growth (1). Considering that bacteria involved in chronic infections in humans tend to aggregate but not necessarily attach to a surface (75), the possible presence of biofilm aggregates of BRD bacteria in host tissues should be further explored. Particularly when one considers that these could promote the mass movement of BRD bacteria into the lower lung. Filtering and cloning is a common technique used in the isolation and purification of mycoplasmas from biological samples as it is assumed that their cells naturally aggregate and exist as clumps of mixed strains (85, 86). The small cellular diameter and lack of a cell wall of mycoplasmas enables individual, pleomorphic cells to pass through a 0.22 μm filter, whereas cell aggregates are trapped on the filter membrane. Moreover, the presence of mycoplasma cell clumps was demonstrated in vitro as a larger bacterial cell count was recovered after liquid growth/ aggregates were subjected to freeze/ thaw cycles or sonication (87). Therefore, the evaluation of whether mycoplasma cell clumps represent non-adherent biofilm aggregates should also be further explored.
Lastly, the existence of BRD bacteria biofilm aggregates in in vitro susceptibility testing systems, like broth microdilution should be investigated to determine if they have an impact on ASTs. Higher MBEC were observed when M. haemolytica biofilms were grown on epithelial cells as compared to polystyrene (28). This highlights the importance of developing standard AST protocols for BRD bacteria biofilms in order to further assess their possible role in antimicrobial failure and enable comparison of studies across laboratories. For this, a consensus on the definition of a robust and mature biofilm for each BRD bacterial species should be reached to avoid testing antimicrobials on biofilms at different stages of development (75). Aside from viruses, different BRD bacterial species can coexist within healthy or diseased, cattle (14, 15). Following a polymicrobial approach in the study of biofilms (40, 88) would shed light into possible synergisms within the BRD bacterial complex. Coupling 3D tissue systems with multi-omics approaches (i.e., genomics, transcriptomics, metabolomics) would generate insight into the bacterial genes / virulence factors associated with biofilms and the challenge they pose to antimicrobial therapy.
Author contributions
TM: Project administration, Resources, Supervision, Writing – review & editing. SA-L: Conceptualization, Writing – original draft. RZ: Conceptualization, Writing – review & editing. MJ: Funding acquisition, Resources, Writing – review & editing.
Funding
The author(s) declare that financial support was received for the research, authorship, and/or publication of this article. This research was funded by the Saskatchewan Agricultural Development Fund (Grants #20060116, #20130170, #20160253) and the Beef Cattle Research Council Grants (Grant ANH.30.17) and (ANH.16.21C).
Conflict of interest
The authors declare that the research was conducted in the absence of any commercial or financial relationships that could be construed as a potential conflict of interest.
Publisher’s note
All claims expressed in this article are solely those of the authors and do not necessarily represent those of their affiliated organizations, or those of the publisher, the editors and the reviewers. Any product that may be evaluated in this article, or claim that may be made by its manufacturer, is not guaranteed or endorsed by the publisher.
Supplementary material
The Supplementary material for this article can be found online at: https://www.frontiersin.org/articles/10.3389/fvets.2024.1353551/full#supplementary-material
Footnotes
References
1. Sauer, K, Stoodley, P, Goeres, DM, Hall-Stoodley, L, Burmolle, M, Stewart, PS, et al. The biofilm life cycle: expanding the conceptual model of biofilm formation. Nat Rev Microbiol. (2022) 20:608–20. doi: 10.1038/s41579-022-00767-0
2. Nesse, LL, Osland, AM, and Vestby, LK. The role of biofilms in the pathogenesis of animal bacterial infections. Microorganisms. (2023) 11:1–22. doi: 10.3390/microorganisms11030608
3. Kragh, KN, Hutchison, JB, Melaugh, G, Rodesney, C, Roberts, AE, Irie, Y, et al. Role of multicellular aggregates in biofilm formation. MBio. (2016) 7:e00237. doi: 10.1128/mBio.00237-16
4. Uruen, C, Chopo-Escuin, G, Tommassen, J, Mainar-Jaime, RC, and Arenas, J. Biofilms as promoters of bacterial antibiotic resistance and tolerance. Antibiotics (Basel). (2020) 10:1–36. doi: 10.3390/antibiotics10010003
5. Olivares, E, Badel-Berchoux, S, Provot, C, Prevost, G, Bernardi, T, and Jehl, F. Clinical impact of antibiotics for the treatment of Pseudomonas aeruginosa biofilm infections. Front Microbiol. (2019) 10:2894. doi: 10.3389/fmicb.2019.02894
6. Romling, U, and Balsalobre, C. Biofilm infections, their resilience to therapy and innovative treatment strategies. J Intern Med. (2012) 272:541–61. doi: 10.1111/joim.12004
7. Petruzzi, B, Briggs, RE, Tatum, FM, Swords, WE, De Castro, C, Molinaro, A, et al. Capsular polysaccharide interferes with biofilm formation by Pasteurella multocida serogroup a. MBio. (2017) 8:1–17. doi: 10.1128/mBio.01843-17
8. Griffin, D, Chengappa, MM, Kuszak, J, and McVey, DS. Bacterial pathogens of the bovine respiratory disease complex. Vet Clin North Am Food Anim Pract. (2010) 26:381–94. doi: 10.1016/j.cvfa.2010.04.004
9. Andres-Lasheras, S, Jelinski, M, Zaheer, R, and McAllister, TA. Bovine respiratory disease: conventional to culture-independent approaches to studying antimicrobial resistance in North America. Antibiotics (Basel). (2022) 11:1–26. doi: 10.3390/antibiotics11040487
10. Hilton, WM . BRD in 2014: where have we been, where are we now, and where do we want to go? Anim Health Res Rev. (2014) 15:120–2. doi: 10.1017/S1466252314000115
11. Smith, RA, Step, DL, and Woolums, AR. Bovine respiratory disease: looking back and looking forward, what do we see? Vet Clin North Am Food Anim Pract. (2020) 36:239–51. doi: 10.1016/j.cvfa.2020.03.009
12. Radostits, OM, Gay, CC, Hinchcliff, KW, and Constable, PD. Veterinary medicine In: A textbook of the diseases of cattle, horses, sheep, pigs and goats. Eds. J Rodenhuis and R Demetriou-Swanwick. 10th Edition Toronto, Canada: Elsevier (2010).
13. Booker, CW, and Lubbers, BV. Bovine respiratory disease treatment failure: impact and potential causes. Vet Clin North Am Food Anim Pract. (2020) 36:487–96. doi: 10.1016/j.cvfa.2020.03.007
14. Andres-Lasheras, S, Ha, R, Zaheer, R, Lee, C, Booker, CW, Dorin, C, et al. Prevalence and risk factors associated with antimicrobial resistance in bacteria related to bovine respiratory disease-a broad cross-sectional study of beef cattle at entry into Canadian feedlots. Front Vet Sci. (2021) 8:692646. doi: 10.3389/fvets.2021.692646
15. Klima, CL, Zaheer, R, Cook, SR, Booker, CW, Hendrick, S, Alexander, TW, et al. Pathogens of bovine respiratory disease in north American feedlots conferring multidrug resistance via integrative conjugative elements. J Clin Microbiol. (2014) 52:438–48. doi: 10.1128/JCM.02485-13
16. Thanner, S, Drissner, D, and Walsh, F. Antimicrobial Resistance in Agriculture. MBio. (2016) 7:e02227–15. doi: 10.1128/mBio.02227-15
17. Sandal, I, Shao, JQ, Annadata, S, Apicella, MA, Boye, M, Jensen, TK, et al. Histophilus somni biofilm formation in cardiopulmonary tissue of the bovine host following respiratory challenge. Microbes Infect. (2009) 11:254–63. doi: 10.1016/j.micinf.2008.11.011
18. Morck, DW, Olson, ME, Acres, SD, Daoust, PY, and Costerton, JW. Presence of bacterial glycocalyx and fimbriae on Pasteurella haemolytica in feedlot cattle with pneumonic pasteurellosis. Can J Vet Res. (1989) 53:167–71.
19. Petruzzi, B, Dalloul, RA, LeRoith, T, Evans, NP, Pierson, FW, and Inzana, TJ. Biofilm formation and avian immune response following experimental acute and chronic avian cholera due to Pasteurella multocida. Vet Microbiol. (2018) 222:114–23. doi: 10.1016/j.vetmic.2018.07.005
20. Hermeyer, K, Jacobsen, B, Spergser, J, Rosengarten, R, and Hewicker-Trautwein, M. Detection of Mycoplasma bovis by in-situ hybridization and expression of inducible nitric oxide synthase, nitrotyrosine and manganese superoxide dismutase in the lungs of experimentally-infected calves. J Comp Pathol. (2011) 145:240–50. doi: 10.1016/j.jcpa.2010.12.005
21. McAuliffe, L, Ellis, RJ, Miles, K, Ayling, RD, and Nicholas, RAJ. Biofilm formation by mycoplasma species and its role in environmental persistence and survival. Microbiology (Reading). (2006) 152:913–22. doi: 10.1099/mic.0.28604-0
22. Costerton, JW, Stewart, PS, and Greenberg, EP. Bacterial biofilms: a common cause of persistent infections. Science. (1999) 284:1318–22. doi: 10.1126/science.284.5418.1318
23. Subhadra, B, Cao, D, Jensen, R, Caswell, C, and Inzana, TJ. Identification and initial characterization of Hfq-associated sRNAs in Histophilus somni strain 2336. PLoS One. (2023) 18:e0286158. doi: 10.1371/journal.pone.0286158
24. Boukahil, I, and Czuprynski, CJ. Characterization of Mannheimia haemolytica biofilm formation in vitro. Vet Microbiol. (2015) 175:114–22. doi: 10.1016/j.vetmic.2014.11.012
25. Haig, S-H . Adherence of Mannheimia haemolytica to ovine bronchial epithelial cells. Biosci Horiz. (2011) 4:50–60. doi: 10.1093/biohorizons/hzr007
26. Montes Garcia, JF, Vaca, S, Delgado, NL, Uribe-Garcia, A, Vazquez, C, Sanchez Alonso, P, et al. Mannheimia haemolytica OmpP2-like is an amyloid-like protein, forms filaments, takes part in cell adhesion and is part of biofilms. Antonie Van Leeuwenhoek. (2018) 111:2311–21. doi: 10.1007/s10482-018-1122-9
27. Figueroa-Valenzuela, C, Montes-Garcia, JF, Vazquez-Cruz, C, Zenteno, E, Pereyra, MA, and Negrete-Abascal, E. Mannheimia haemolytica OmpH binds fibrinogen and fibronectin and participates in biofilm formation. Microb Pathog. (2022) 172:105788. doi: 10.1016/j.micpath.2022.105788
28. Boukahil, I, and Czuprynski, CJ. Mannheimia haemolytica biofilm formation on bovine respiratory epithelial cells. Vet Microbiol. (2016) 197:129–36. doi: 10.1016/j.vetmic.2016.11.012
29. Pillai, DK, Cha, E, and Mosier, D. Role of the stress-associated chemicals norepinephrine, epinephrine and substance P in dispersal of Mannheimia haemolytica from biofilms. Vet Microbiol. (2018) 215:11–7. doi: 10.1016/j.vetmic.2017.11.025
30. Sandal, I, Hong, W, Swords, WE, and Inzana, TJ. Characterization and comparison of biofilm development by pathogenic and commensal isolates of Histophilus somni. J Bacteriol. (2007) 189:8179–85. doi: 10.1128/JB.00479-07
31. O'Toole, D, Sondgeroth, KS, and Somni, H In: K Aktories , editor. Biology, molecular basis of pathogenesis, and host immunity. Blacksburg, VA: Springer (2016)
32. O'Toole, D, Hunter, R, Allen, T, Zekarias, B, Lehmann, J, Kim, KS, et al. Effect of Histophilus somni on heart and brain microvascular endothelial cells. Vet Pathol. (2017) 54:629–39. doi: 10.1177/0300985817691581
33. Elswaifi, SF, Scarratt, WK, and Inzana, TJ. The role of lipooligosaccharide phosphorylcholine in colonization and pathogenesis of Histophilus somni in cattle. Vet Res. (2012) 43:49. doi: 10.1186/1297-9716-43-49
34. Sandal, I, Inzana, TJ, Molinaro, A, De Castro, C, Shao, JQ, Apicella, MA, et al. Identification, structure, and characterization of an exopolysaccharide produced by Histophilus somni during biofilm formation. BMC Microbiol. (2011) 11:186. doi: 10.1186/1471-2180-11-186
35. Pan, Y, Subhadra, B, Sandal, I, Dickerman, A, and Inzana, TJ. The role of uspE in virulence and biofilm formation by Histophilus somni. Vet Microbiol. (2021) 263:109267. doi: 10.1016/j.vetmic.2021.109267
36. Olson, ME, Ceri, H, Morck, DW, Buret, AG, and Read, RR. Biofilm bacteria: formation and comparative susceptibility to antibiotics. Can J Vet Res. (2002) 66:86–92.
37. Saha, O, Islam, MR, Rahman, MS, Hoque, MN, Hossain, MA, and Sultana, M. First report from Bangladesh on genetic diversity of multidrug-resistant Pasteurella multocida type B:2 in fowl cholera. Vet World. (2021) 14:2527–42. doi: 10.14202/vetworld.2021.2527-2542
38. Prajapati, A, Chanda, MM, Dhayalan, A, Yogisharadhya, R, Chaudhary, JK, Mohanty, NN, et al. Variability in in vitro biofilm production and antimicrobial sensitivity pattern among Pasteurella multocida strains. Biofouling. (2020) 36:938–50. doi: 10.1080/08927014.2020.1833192
39. Nguyen, PV, Le, CT, Nguyen, XH, Nguyen, TM, and Nguyen, KCT. First study on capsular serotypes and virulence factors of Pasteurella multocida isolates from phan rang sheep in Vietnam. Vet World. (2023) 16:281–90. doi: 10.14202/vetworld.2023.281-290
40. Petruzzi, B, Dickerman, A, Lahmers, K, Scarratt, WK, and Inzana, TJ. Polymicrobial biofilm interaction between Histophilus somni and Pasteurella multocida. Front Microbiol. (2020) 11:1561. doi: 10.3389/fmicb.2020.01561
41. Kachlany, SC, Planet, PJ, Bhattacharjee, MK, Kollia, E, DeSalle, R, Fine, DH, et al. Nonspecific adherence by Actinobacillus actinomycetemcomitans requires genes widespread in bacteria and archaea. J Bacteriol. (2000) 182:6169–76. doi: 10.1128/JB.182.21.6169-6176.2000
42. Peng, Z, Wang, X, Zhou, R, Chen, H, Wilson, BA, and Wu, B. Pasteurella multocida: genotypes and genomics. Microbiol Mol Biol Rev. (2019) 83:1–37. doi: 10.1128/MMBR.00014-19
43. Zhan, L, Zhang, J, Zhao, B, Li, X, Zhang, X, Hu, R, et al. Genomic and transcriptomic analysis of bovine Pasteurella multocida serogroup a strain reveals insights into virulence attenuation. Front Vet Sci. (2021) 8:765495. doi: 10.3389/fvets.2021.765495
44. Prajapati, A, Yogisharadhya, R, Mohanty, NN, Mendem, SK, Nizamuddin, A, Chanda, MM, et al. Comparative genome analysis of Pasteurella multocida serogroup B:2 strains causing haemorrhagic septicaemia (HS) in bovines. Gene. (2022) 826:146452. doi: 10.1016/j.gene.2022.146452
45. de Emery, BD, Furian, TQ, Pilatti, RM, Chitolina, GZ, Borges, KA, Salle, CTP, et al. Evaluation of the biofilm formation capacity of Pasteurella multocida strains isolated from cases of fowl cholera and swine lungs and its relationship with pathogenicity. Pesqui Vet Bras. (2017) 37:1041–8. doi: 10.1590/s0100-736x2017001000001
46. Wang, Z, Li, L, Liu, P, Wang, C, Lu, Q, Liu, L, et al. Role of aspartate ammonia-lyase in Pasteurella multocida. BMC Microbiol. (2020) 20:369. doi: 10.1186/s12866-020-02049-2
47. Yang, Y, Hu, P, Gao, L, Yuan, X, Hardwidge, PR, Li, T, et al. Deleting qseC downregulates virulence and promotes cross-protection in Pasteurella multocida. Vet Res. (2021) 52:140. doi: 10.1186/s13567-021-01009-6
48. Xu, T, Zheng, Y, Liu, B, Kou, M, Jiang, Q, Liu, J, et al. Pmorf0222, a virulence factor in Pasteurella multocida, activates nuclear factor kappa B and mitogen-activated protein kinase via toll-like receptor 1/2. Infect Immun. (2023) 91:e0019322. doi: 10.1128/iai.00193-22
49. Calcutt, MJ, Lysnyansky, I, Sachse, K, Fox, LK, Nicholas, RAJ, and Ayling, RD. Gap analysis of Mycoplasma bovis disease, diagnosis and control: an aid to identify future development requirements. Transbound Emerg Dis. (2018) 65:91–109. doi: 10.1111/tbed.12860
50. Sirand-Pugnet, P, Citti, C, Barre, A, and Blanchard, A. Evolution of mollicutes: down a bumpy road with twists and turns. Res Microbiol. (2007) 158:754–66. doi: 10.1016/j.resmic.2007.09.007
51. Citti, C, Dordet-Frisoni, E, Nouvel, LX, Kuo, CH, and Baranowski, E. Horizontal gene transfers in mycoplasmas (Mollicutes). Curr Issues Mol Biol. (2018) 29:3–22. doi: 10.21775/cimb.029.003
52. Nicholas, R, Ayling, RD, and McAuliffe, L. Mycoplasma diseases of ruminants. Wallingford, Oxfordshire: CABI (2008).
53. Gonzalez, RN, and Wilson, DJ. Mycoplasmal mastitis in dairy herds. Vet Clin North Am Food Anim Pract. (2003) 19:199–221. doi: 10.1016/S0749-0720(02)00076-2
54. Justice-Allen, A, Trujillo, J, Corbett, R, Harding, R, Goodell, G, and Wilson, D. Survival and replication of Mycoplasma species in recycled bedding sand and association with mastitis on dairy farms in Utah. J Dairy Sci. (2010) 93:192–202. doi: 10.3168/jds.2009-2474
55. Soehnlen, MK, Aydin, A, Murthy, KS, Lengerich, EJ, Hattel, AL, Houser, BA, et al. Epidemiology of Mycoplasma bovis in Pennsylvania veal calves. J Dairy Sci. (2012) 95:247–54. doi: 10.3168/jds.2011-4309
56. Piccinini, R, Gosney, F, Snel, GGM, Luini, MV, and Nicholas, RAJ. Environmental survival of Mycoplasma bovis on a white veal farm. Vet Rec Case Rep. (2015) 3:1–3. doi: 10.1136/vetreccr-2015-000207
57. Chen, S, Hao, H, Zhao, P, Ji, W, Li, M, Liu, Y, et al. Differential immunoreactivity to bovine convalescent serum between Mycoplasma bovis biofilms and planktonic cells revealed by comparative immunoproteomic analysis. Front Microbiol. (2018) 9:379. doi: 10.3389/fmicb.2018.00379
58. da Silva, DV, Dias, RS, Kropinski, AM, da Silva, XA, Ferro, CG, Vidigal, PMP, et al. A T4virus prevents biofilm formation by Trueperella pyogenes. Vet Microbiol. (2018) 218:45–51. doi: 10.1016/j.vetmic.2018.03.025
59. Jost, BH, and Billington, SJ. Arcanobacterium pyogenes: molecular pathogenesis of an animal opportunist. Antonie Van Leeuwenhoek. (2005) 88:87–102. doi: 10.1007/s10482-005-2316-5
60. Zhang, L, Cai, Y, Li, L, Chen, C, Zhao, H, Zhang, Z, et al. Effects of luteolin on biofilm of Trueperella pyogenes and its therapeutic effect on rat endometritis. Int J Mol Sci. (2022) 23:1–14. doi: 10.3390/ijms232214451
61. Zhao, K, Tian, Y, Yue, B, Wang, H, and Zhang, X. Virulence determinants and biofilm production among Trueperella pyogenes recovered from abscesses of captive forest musk deer. Arch Microbiol. (2013) 195:203–9. doi: 10.1007/s00203-013-0869-7
62. Ozturk, D, Turutoglu, H, Pehlivanoglu, F, and Guler, L. Virulence genes, biofilm production and antibiotic susceptibility in Trueperella pyogenes isolated from cattle. Israel J Vet Med. (2016) 71:36–42.
63. Zhao, K, Li, W, Huang, T, Song, X, Zhang, X, and Yue, B. Comparative transcriptome analysis of Trueperella pyogenes reveals a novel antimicrobial strategy. Arch Microbiol. (2017) 199:649–55. doi: 10.1007/s00203-017-1338-5
64. Zhang, Z, Liang, Y, Yu, L, Chen, M, Guo, Y, Kang, Z, et al. TatD DNases contribute to biofilm formation and virulence in Trueperella pyogenes. Front Microbiol. (2021) 12:758465. doi: 10.3389/fmicb.2021.758465
65. Rzewuska, M, Kwiecien, E, Chrobak-Chmiel, D, Kizerwetter-Swida, M, Stefanska, I, and Gierynska, M. Pathogenicity and virulence of Trueperella pyogenes: a review. Int J Mol Sci. (2019) 20:1–33. doi: 10.3390/ijms20112737
66. Boukahil, I, and Czuprynski, CJ. Mutual antagonism between Mannheimia haemolytica and Pasteurella multocida when forming a biofilm on bovine bronchial epithelial cells in vitro. Vet Microbiol. (2018) 216:218–22. doi: 10.1016/j.vetmic.2018.02.015
67. Orazi, G, and O'Toole, GA. "it takes a village": mechanisms underlying antimicrobial recalcitrance of polymicrobial biofilms. J Bacteriol. (2019) 202:1–18. doi: 10.1128/JB.00530-19
68. Ha, R, Zaheer, R, Sargeant, C, Klima, C, and TA, MA. Intra- and inter-species horizontal transfer potential of integrative and conjugative elements carrying antimicrobial resistance genes. Sherbrooke, QC, Canada: Canadian Society of Microbiologists (2019).
69. Ceri, H, Olson, ME, Stremick, C, Read, RR, Morck, D, and Buret, A. The Calgary biofilm device: new technology for rapid determination of antibiotic susceptibilities of bacterial biofilms. J Clin Microbiol. (1999) 37:1771–6. doi: 10.1128/JCM.37.6.1771-1776.1999
70. McClary, DG, Loneragan, GH, Shryock, TR, Carter, BL, Guthrie, CA, Corbin, MJ, et al. Relationship of in vitro minimum inhibitory concentrations of tilmicosin against Mannheimia haemolytica and Pasteurella multocida and in vivo tilmicosin treatment outcome among calves with signs of bovine respiratory disease. J Am Vet Med Assoc. (2011) 239:129–35. doi: 10.2460/javma.239.1.129
71. CLSI. Performance standards for antimicrobial disk and dilution susceptibility tests for bacteria isolated from animals. CLSI standard VET01. Eds. KL Leirer, AEM Jenkins, and L Martin. 5th Wayne, PA: Clinical and Laboratory Standards Institute (2018)
72. Constable, PD, Hinchcliff, KW, Done, SH, and Grünberg, W. Veterinary medicine. A textbook of the diseases of cattle, horses, sheep, pigs and goats. Eds. P. D. Constable, K. W. Hinchcliff, S. H. Done, and W. Grünberg. 11th Edition St. Louis, Missouri: Elsevier (2017).
73. Lubbers, BV . Pharmacological considerations of antibiotic failures in bovine respiratory disease cases. Anim Health Res Rev. (2020) 21:177–8. doi: 10.1017/S1466252320000122
74. Avra, TD, Abell, KM, Shane, DD, Theurer, ME, Larson, RL, and White, BJ. A retrospective analysis of risk factors associated with bovine respiratory disease treatment failure in feedlot cattle. J Anim Sci. (2017) 95:1521–7. doi: 10.2527/jas2016.1254
75. Alhede, M, Kragh, KN, Qvortrup, K, Allesen-Holm, M, van Gennip, M, Christensen, LD, et al. Phenotypes of non-attached Pseudomonas aeruginosa aggregates resemble surface attached biofilm. PLoS One. (2011) 6:e27943. doi: 10.1371/journal.pone.0027943
76. CLSI. Methods for antimicrobial susceptibility testing of infrequently isolated or fastidious bacteria isolated from animals. CLSI standard VET06. Eds. JP Christopher, L Maritn, and MA Russell. 1st ed. Wayne, PA: Clinical and Laboratory Standards Institute (2016)
77. Gandhi, NN, Inzana, TJ, and Rajagopalan, P. Bovine airway models: approaches for investigating bovine respiratory disease. ACS Infect Dis. (2023) 9:1168–79. doi: 10.1021/acsinfecdis.2c00618
78. Alshammari, M, Ahmad, A, AlKhulaifi, M, Al Farraj, D, Alsudir, S, Alarawi, M, et al. Reduction of biofilm formation of Escherichia coli by targeting quorum sensing and adhesion genes using the CRISPR/Cas9-HDR approach, and its clinical application on urinary catheter. J Infect Public Health. (2023) 16:1174–83. doi: 10.1016/j.jiph.2023.05.026
79. Koeppen, K, Nymon, A, Barnaby, R, Bashor, L, Li, Z, Hampton, TH, et al. Let-7b-5p in vesicles secreted by human airway cells reduces biofilm formation and increases antibiotic sensitivity of P. aeruginosa. Proc Natl Acad Sci USA. (2021) 118:1–10. doi: 10.1073/pnas.2105370118
80. Kubera, A, Thamchaipenet, A, and Shoham, M. Biofilm inhibitors targeting the outer membrane protein a of Pasteurella multocida in swine. Biofouling. (2017) 33:14–23. doi: 10.1080/08927014.2016.1259415
81. LeBel, G, Vaillancourt, K, Bercier, P, and Grenier, D. Antibacterial activity against porcine respiratory bacterial pathogens and in vitro biocompatibility of essential oils. Arch Microbiol. (2019) 201:833–40. doi: 10.1007/s00203-019-01655-7
82. Qasim, N, Shahid, M, Yousaf, F, Riaz, M, Anjum, F, Faryad, MA, et al. Therapeutic potential of selected varieties of Phoenix dactylifera L. against microbial biofilm and free radical damage to DNA. Dose-Response. (2020) 18:155932582096260. doi: 10.1177/1559325820962609
83. Gurunathan, S, Choi, YJ, and Kim, JH. Antibacterial efficacy of silver nanoparticles on endometritis caused by Prevotella melaninogenica and Arcanobacterum pyogenes in dairy cattle. Int J Mol Sci. (2018) 19:1–20. doi: 10.3390/ijms19041210
84. Zhang, Z, Guo, Y, Guo, Y, Zhang, L, Niu, S, Tian, C, et al. Molecular basis for luteolin as a natural TatD DNase inhibitor in Trueperella pyogenes. Int J Mol Sci. (2022) 23:1–13. doi: 10.3390/ijms23158374
86. Sagne, E, Citti, C, and Dordet-Frisoni, E. Bacterial conjugation protocol for ruminant mycoplasmas. Bio Protoc. (2021) 11:e3893. doi: 10.21769/BioProtoc.3893
87. Dabrazhynetskaya, A, Furtak, V, Volokhov, D, Beck, B, and Chizhikov, V. Preparation of reference stocks suitable for evaluation of alternative NAT-based mycoplasma detection methods. J Appl Microbiol. (2014) 116:100–8. doi: 10.1111/jam.12352
Keywords: biofilms, antimicrobial resistance, bovine respiratory disease, Mannheimia haemolytica , Pasteurella multocida , Histophilus somni , Mycoplasma bovis
Citation: Andrés-Lasheras S, Zaheer R, Jelinski M and McAllister TA (2024) Role of biofilms in antimicrobial resistance of the bacterial bovine respiratory disease complex. Front. Vet. Sci. 11:1353551. doi: 10.3389/fvets.2024.1353551
Edited by:
Getahun E. Agga, Agricultural Research Service (USDA), United StatesReviewed by:
Tara G. McDaneld, Agricultural Research Service (USDA), United StatesJon Schoonmaker, Purdue University, United States
Copyright © 2024 Andrés-Lasheras, Zaheer, Jelinski and McAllister. This is an open-access article distributed under the terms of the Creative Commons Attribution License (CC BY). The use, distribution or reproduction in other forums is permitted, provided the original author(s) and the copyright owner(s) are credited and that the original publication in this journal is cited, in accordance with accepted academic practice. No use, distribution or reproduction is permitted which does not comply with these terms.
*Correspondence: Tim A. McAllister, dGltLm1jYWxsaXN0ZXJAQUdSLkdDLkNB