- 1Commonwealth Scientific and Industrial Research Organisation (CSIRO), Agriculture and Food, Werribee, VIC, Australia
- 2School of Chemical Engineering, The University of New South Wales, Sydney, NSW, Australia
The ruminant digestive system is uniquely designed to make efficient use of high-fibre feed, including forages. Between 40 to 100% of the ruminant diet consists of forages which are high in fibre and up to 70% of this may remain undigested in the ruminant gut, with substantial impact on feed utilisation rate and productivity and the economic and environmental sustainability of livestock production systems. In ruminants, feed costs can make up to 70% of the overall cost of producing an animal product. Increasing feed utilisation efficiency, i.e., more production with less feed lowers feeding costs and improves livestock economic viability. Strategies for improving nutrient utilisation in animal feed has been investigated over the years. Incorporation of fibre digesting enzymes in the feed to facilitate the digestion of the residual fibre in hind gut is one of the proposed strategies. However, delivering such enzymes to the hind gut in active state is challenging due to the unfavourable biochemical environment (pH, microbial proteases) of ruminant’s gastrointestinal tract. This review discusses the potential application of microencapsulation for protected and targeted delivery of enzymes into the hind gut of ruminants.
1 Introduction
Ruminants include cattle, sheep, goats, deer, and other cud-chewing cloven-hoofed mammals that are poly-gastric herbivorous with a unique gastrointestinal tract that allows them to digest plant materials and makes them an important part of the food chains all over the world (1, 2). Unlike monogastric animals, the ruminant’s digestive system has one stomach with four chambers. These chambers are the rumen, the reticulum, the omasum, and the abomasum. The energy requirements of ruminant animals are met predominantly through the production of volatile fatty acids (VFAs) by the microorganisms that live in the ruminant’s stomach, mostly in the rumen, in a symbiotic relationship with the ruminant animal (3). This symbiotic relationship is beneficial to ruminants as well as the microorganisms. On the one hand, the microorganisms benefit by having a warm, wet, nutrient-rich environment to reside in. In other words, the host animal provides an anaerobic fermentation chamber for the microbes to break down the ingested feed into microbial proteins and VFAs to supply high-quality nutrients for the ruminant animal. On the other hand, a major benefit that a ruminant animal gets from the symbiotic relationship is the ability of microorganisms to digest fibre in forage-based diets (4, 5).
The enteric fermentation that takes place in the rumen is adversely correlated to environmental sustainability since it results in greenhouse gas (GHG) emissions. Several studies have been conducted to reduce ruminant methane emissions since GHG profile of the cattle dairy sector is dominated by methane emissions that is produced during the feed energy utilisation process (6). Improving ruminant productivity and energy efficiency by increasing feed digestibility, for example, is the one of the most cost-effective and promising techniques for reducing ruminant methane gas emissions (6, 7).
The digestibility of plant cell-wall material such as cellulose, hemicellulose, pectin, and lignin, as the primary source of feed for ruminants, has a significant economic implication in both developed and developing countries. However, the rumen’s ability to digest fibre is not optimum, as evidenced by the fermentability of fibre recovered from faeces (5). Ruminant animals may not digest up to 70% of their diet that contains fibrous components (8). Therefore, researchers have attempted to enhance feed utilisation rate in the rumen by manipulation of ruminal fermentation and improving diet management (7).
Ruminants lack the enzymes required to digest fibrous materials. As such, they rely on microbes within their digestive system for producing digestive enzymes (9). Therefore, most of the approaches for enhancing feed utilisation in ruminants are based on manipulation of rumen fermentation genetically or via incorporation of fibrolytic microorganisms or enzymes in ruminant feed (5). The addition of highly fibrolytic ruminal microorganisms or their enzyme extract have the potential to increase fibre digestibility and feed utilisation in ruminants (10, 11). Several in vitro and animal studies have been conducted on the use of exogenous enzyme formulations to improve feed utilisation and productivity in ruminants (12, 13). Nevertheless, so far, the outcomes in terms of productivity improvement are inconsistent (12, 13). This has been attributed to the use of feed enzyme formulations that are not specifically formulated for the target application, the mode of delivery of the enzymes and its effect on their stability and activity (alone or mixed with the feed, the proportion of the feed in the mix), variation in the stability of exogenous enzyme formulations in the rumen environment, and limited understanding of the rumen microbiota and their enzymes that would have enabled better design of exogenous enzyme supplements for synergistic activity with the microbial enzymes in the rumen (13).
The stability and activity of enzymes is highly dependent on environmental factors such as pH, ionic strength, temperature, and the presence of proteases and other (bio)chemicals that enhance or inhibit their activity and/or cause denaturation. Studies indicate that exogenous enzymes that bind to the feed seem to be more effective possibly due to more resistance to degradation by microbial proteases in the rumen (13, 14). The rumen gastrointestinal (GI) tract is a highly complex and harsh environment comprising of the rumen with its microbiota and their enzymes as well as the very low pH environment of the abomasum, which can negatively affect the activity of exogenous enzymes. In this respect, technologies that protect enzymes against environmental stress are of great value. Microencapsulation is one such technology that enables protection of feed enzymes against the harsh environment in the rumen and abomasum and targeted release in the GI tract for effective enhancement of feed utilisation rate.
Microencapsulation protects bioactive compounds against biotic and abiotic stresses in their environment by enclosing them in a carrier matrix (15, 16). The technology not only helps to maintain biological activity of susceptible bioactive compounds during ingestion but also enable their release at a specific location (15). Desai and Jin Park (17) described microencapsulation as a technology that was invented about 65 years ago to encapsulate liquids, gases, or solids in sealed capsules and control the release of their content under certain conditions. Several methods have been developed for producing microcapsules. Chemical methods, physicochemical methods, and physical methods are the three broad categories of microcapsule formation technologies. The method used is determined by the size of microcapsules, both the core and the wall’s chemical/physical properties, the bioactive core’s applications, economics, core sensitivity, and mechanisms of release (18, 19). Microencapsulation technology is widely used in the pharmaceutical and the food industries (15). It is also used in the ruminant feed industry to a lesser extent, to protect active compounds from rumen degradation, allowing for increased bioavailability of these compounds in the lower gastrointestinal tract of ruminants (20). A number of studies investigated microencapsulation for the delivery of actives such as essential oils (21), polyunsaturated fatty acids (22) and vitamins (23, 24) to ruminants resulting in better outcomes compared to the same amount of non-encapsulated ingredients. Microencapsulated feed supplement such as essential oils, butyrate, and bacteria are already available in the market. However, limited information is available in the public domain on microencapsulation of enzymes for post-rumen delivery in ruminants. This review briefly discusses feed utilisation efficiency in ruminants and the use of exogenous fibrolytic enzymes for improving feed utilisation and the challenges to delivery of enzymes to the ruminant GI system post rumen followed by a discussion on the potential of microencapsulation technology to overcome these challenges.
2 Feed utilisation in ruminants
Soluble carbohydrates as well as soluble cell wall carbohydrates such as sugars, starches, soluble fibres, pectins and β-glucans are rapidly digested in the rumen with very minimal amounts escaping to post-ruminal digestion. The majority of these soluble carbohydrates are degraded in both the small intestine and hindgut if they are not degraded in the rumen (25). However, insoluble cell wall carbohydrates, for example, neutral detergent fibre (NDF) are slowly degraded in the rumen. As a result, the retention time of the fibre in the rumen determines the extent of rumen digestion. For instance, grinding fibre into small particles may help it move through the rumen more quickly, however ruminal digestion of that fibre particle may be reduced as a result of the reduced surface area available for rumen microbes to attach and digest these fibrous materials (26). Huhtanen et al. (25) pointed out that there is no digestion of fibre in the small intestine since both soluble and insoluble fibre digestion is dependent on microbial fermentation. However, fibre that is not degraded by the rumen may be digested in the hindgut (27). However, much fewer efforts have been dedicated to understanding the digestion rate of fibre residues in the hindgut.
Factors limiting fibre digestion in the rumen involve microbial enzymes such as β 1–4 cellulase enzyme complex, allowing plant cell wall polysaccharides to be hydrolysed in the rumen. However, ruminants may not digest up to 70% of their diet, particularly the fibrous components, and only 10–35 percent of energy intake is received as net energy by the ruminant animal (8). Four main factors have been identified as limiting factors for fibre digestion in the rumen: (i) animal factors that boost the nutrient’s availability through salivation, digesta kinetics, and mastication (i.e., ruminant fibre intake and mastication alter the pace of passage through the digestive tract, with higher intakes resulting in decreased total fibre digestion), (ii) microbiological factors that affect adhesion to the hydrolytic enzyme complexes of microbes in the rumen, (iii) the population of the most common fibre-digesting microbes, (iv) the biodegradability and the chemical composition of plant material’s insoluble component (28). Rumen pH appears to be a major factor in fibre digestion. A moderate drop in pH to around 6.0 causes a slight reduction in fibre digestion, but the number of fibrolytic microorganisms is unaffected. Further reduction of pH to 5.5 or 5.0 results in slowed growth rate and fewer fibrolytic bacteria, and fibre digestion may be halted. The drop in pH is caused by inadequate fibre with a high level of starch in the diet or fibre that has been chopped finely, which adversely affects chewing periods and consequently saliva production (29). Other factors such the level of lignification of the feed, and cellulolytic enzyme inhibition may affect fibre digestion (30). Kung (29) noted that lignin acts as an intercellular cement, giving plants stiffness, but reducing fermentability. According to Allen and Mertens (31), the indigestible fraction of fibre is the most significant independent constraint on fibre digestion. This fraction makes up 1/3 to 1/2 of the total fibre fraction. The digestibility of various plant components in the rumen is illustrated in Figure 1.
3 Approaches for improving feed utilisation in ruminants
Improving fibre digestion in ruminants has been a focus of research in rumen microbiology for several decades. Ruminants rely on the microbial population within their rumen to produce the necessary enzymes for fibre digestion, as they lack the required endogenous enzymes themselves (9). The supplementation of highly fibrolytic ruminal microorganisms or their enzyme extracts has been found to increase fibre degradability in ruminants (10). However, the effects on growth traits and feed efficiency ratio were not constant, and the impact may vary depending on breeds and dosages used. Further research may be required to assess the optimal dosage and specific benefits of exogenous fibrolytic enzymes supplementation in ruminants’ nutrition.
Other factors such as diet composition, feed processing methods, environmental stress and rumen microbial populations can also influence fibre digestion and feed utilisation in ruminants. For instance, diets containing high levels of readily fermentable carbohydrates may impede the efficacy of enzymes in fibre digestion (32). Therefore, a comprehensive understanding of the interactions between enzymes, microorganisms, and the rumen environment is necessary to optimise the use of enzymes for enhancing feed utilisation.
3.1 Enzyme supplementation for enhancing feed utilisation in livestock
The digestive efficiency of ruminants is not optimal, and a significant portion of feed remains undigested due to indigestible anti-nutritional factors or the absence of specific enzymes (33). Feed enzyme additives primarily target the fibrous fraction of forages, with limited exploration of amylases for starch utilisation (33–35). Forages typically contain 30–70% neutral detergent fibre (NDF) on a dry matter (DM) basis. Despite optimal feeding conditions, the digestibility of NDF in the digestive tract of ruminants tends to be below 65%, and ruminal NDF digestibility is often less than 50%. As feed constitutes a substantial cost in animal production, supplementing it with specific enzymes enhances the nutritional value of ingredients, improving digestion efficiency and overall animal performance (33). The supplementation of animal feeds with exogenous enzymes has been successfully practiced since the late 1980s (36). Today, the overall value of the feed enzyme market has expanded approximately fourfold compared to its early 2000s valuation. Table 1 provides a comprehensive review on enzyme additives for ruminants endeavouring to articulate a well-founded rationale for their efficacious integration into both beef and dairy diets.
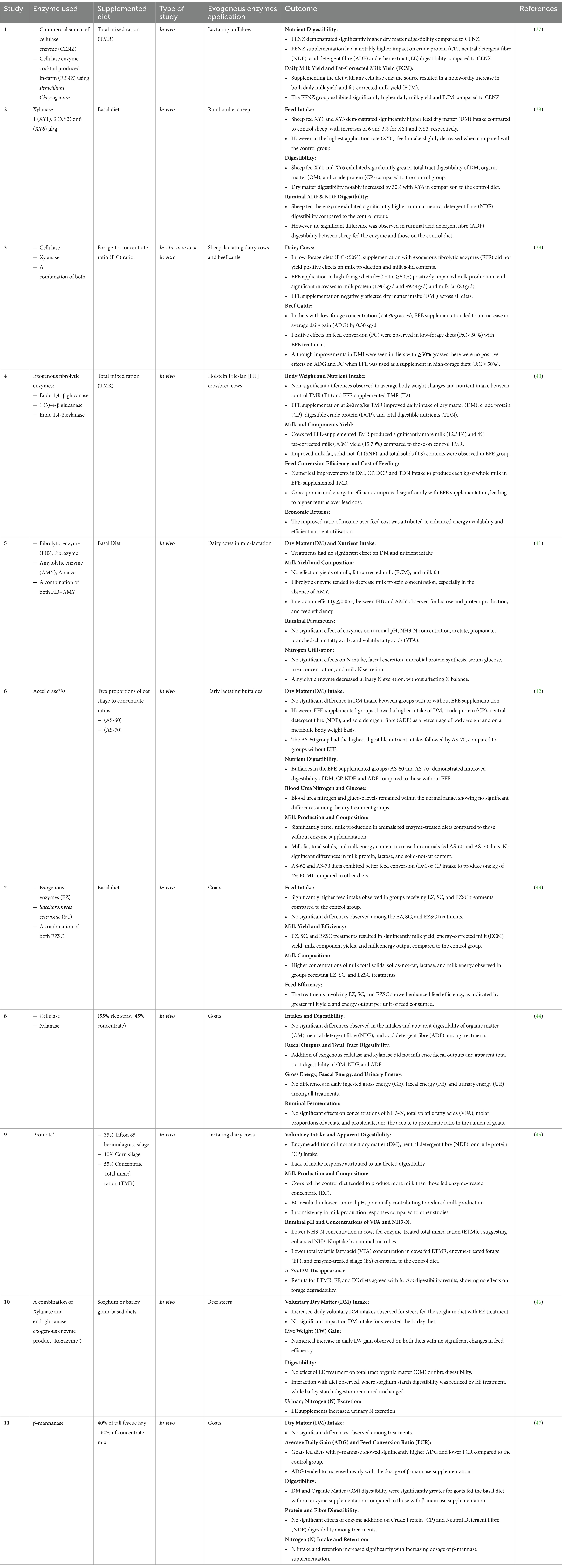
Table 1. Summary of relevant studies on the use of exogenous enzymes for enhancing feed utilisation in ruminants.
The reported outcomes from various studies demonstrate the potential of enzyme supplementation on rumen function, nutrient digestibility, milk production, and growth performance. However, it should be noted that the outcomes of using exogenous fibrolytic enzymes (EFE) is so far inconsistent, and their activity and efficiency varies depending on the type and quality of the diet and animal species. For example, the studies by Lee et al. (47), Zilio et al. (41) and Miller et al. (46) collectively contribute diverse insights into the complex effects of exogenous enzyme supplementation in ruminants. Lee et al. (47) and Zilio et al. (41) share a common observation, revealing no significant impact on dry matter (DM) intake with enzyme supplementation. However, Miller et al. (46) diverges by noting an increased intake, specifically in the sorghum-fed group, underlining the influence of both enzyme type and dietary composition. Furthermore, while Zilio et al. (41) and Miller et al. (46) converge in reporting no significant impact on overall digestibility with enzyme treatment, Lee et al. (47) presents a unique finding of reduced digestibility with β-mannase supplementation, emphasising the need to consider specific enzymes and substrates in understanding the broader implications. Lastly, while Zilio et al. (41) and Miller et al. (46) do not find significant effects on milk yield and composition, Lee et al. (47) leaves this aspect unexplored, highlighting the potential for a more comprehensive understanding by integrating studies that consider both animal performance and milk production outcomes. One of the reasons for the inconsistent results is the susceptibility of enzymes to proteolytic degradation by rumen microorganisms (39, 48). Enzymes can be degraded by proteases present in the rumen, limiting their effectiveness for enhancing fibre digestion. The divergence in outcomes has been ascribed to various factors, including the enzyme formulation, enzymatic activity, dosage level, mechanism of action, supply method, stability of the enzymes within the rumen, the overall composition of the diet and experimental conditions (41). This variability in enzyme activity and degradation highlights the complexity of the rumen ecosystem and the challenges associated with utilising exogenous enzymes as a reliable approach for improving fibre digestion.
Further research is needed to explore strategies that can enhance the stability and effectiveness of exogenous enzymes in the rumen. This may involve the development of enzyme formulations that are more resistant to proteolytic degradation or utilising enzyme delivery systems that can protect enzymes until they reach the site of action in the gastrointestinal tract of ruminants. Nonetheless, the use of enzymes represents a promising approach to maximise feed utilisation and improve the efficiency of ruminant production systems. These advancements will contribute to the development of more effective and reliable approaches for enhancing fibre digestion and optimising feed utilisation in ruminant production systems.
3.2 Challenges for delivery of enzymes to ruminant gut
As discussed earlier, the application of exogenous fibrolytic enzymes has a significant potential for improving feed utilisation efficiency in ruminants. However, delivering enzymes in active form to the rumen and beyond in the GI tract of ruminants is quite challenging especially due to the susceptibility of enzymes to environmental factors such as pH as well as proteolytic degradation. The temperature and pH conditions in the GI tract of ruminants is summarised in Figure 2. The rumen houses a highly complex and diverse microbiota and is considered as a “fermentation vat” since it contains microbial communities that feed on forages and produce the enzymes required to degrade the components of plant cell walls such as hemicellulose, cellulose, pectin, and lignin as well as proteases (3, 26). Thus, exogenous enzymes may not maintain their activity and contribute to fibre digestion in the rumen as they may be degraded by proteases. The abomasum with its very low pH and pepsin presents another challenging environment to exogenous enzymes so do trypsin in the small intestine and the microbiota and their enzymes in the hind gut.
One way of overcoming this challenge is the use of microencapsulation, i.e., enclosing the enzyme in a carrier matrix that protect it against environmental stress and enable its targeted delivery at a specific location within the GI tract. Microencapsulation helps to protect the encapsulated core material (enzyme or other active ingredients) against ruminal degradation by lowering its reactivity or transference to the outside environment, and/or from the unfavourable environmental conditions such as pH, and microbial communities, resulting in increased bioavailability of the active ingredient in the lower GI tract of ruminants (20, 49). Microencapsulation is effective in ensuring rumen by-pass of active ingredients and their bioavailability (50). For instance, a study by Yoshimaru et al. (51) showed that spray dried microcapsules of proteases have successfully passed through rumen with little degradation and their content was released in the abomasum to increase protein absorption in the intestines. The success of the microencapsulation process depends on the appropriate selection of the wall materials and encapsulation method, that protect the active core and allow it to be released in active form at the desired location (52–54). This innovative approach not only protects the encapsulated core material, such as enzymes, from environmental stress but also ensures their targeted delivery, ultimately leading to increased bioavailability in the lower gastrointestinal tract of ruminants.
4 Microencapsulation techniques for enhancing stability and controlled release of enzymes
In various industrial sectors where enzymes play a pivotal role, the issue of enzyme stability emerges as a crucial obstacle in optimising their efficacy. Despite the potential benefits of exogenous enzyme supplementation in improving fibre digestibility and nutrient absorption, the practical application of these enzymes is frequently impeded by challenges pertaining to their stability (39, 41, 48). Roy Choudhury (55) stated that enzymes are most effective when the pH and temperature are maintained within a limited range. Based on that, the shape of the active site of an enzyme can be altered by heating or changing the pH of the enzyme’s surroundings, rendering it inactive. Factors such as extreme pH and temperature variations, exposure to proteolytic enzymes, and interactions with dietary components can lead to enzyme denaturation, degradation, or loss of activity before reaching their intended targets (56–59). Thus, there is a critical need for research and development efforts aimed at enhancing enzyme stability across various environments. Addressing these stability issues is paramount to unlocking the full potential of enzyme supplementation. Thus, microencapsulating these enzymes is a viable option for improving their application in commercial products and industrial processes (60).
Microencapsulation is a versatile technique that has been widely utilised for the encapsulation of various compounds, including water-soluble substances like enzymes. This process involves the coating or surrounding of individual particles, referred to as core or active materials, with a protective wall material, resulting in the formation of microcapsules or microspheres (61, 62). Microcapsules consist of an inner core containing the enzyme and a polymer membrane that encapsulates it, while microspheres are composed of a polymer matrix with the enzyme uniformly dispersed or dissolved within it as shown in Figure 3 (64, 65). Microencapsulation has emerged as a promising technique for the protected delivery of enzymes to the gastrointestinal (GI) tracts of both humans and animals, including poultry (62, 66). This innovative approach involves encapsulating enzymes within protective coatings, allowing for controlled release and targeted delivery within the GI environment (67).
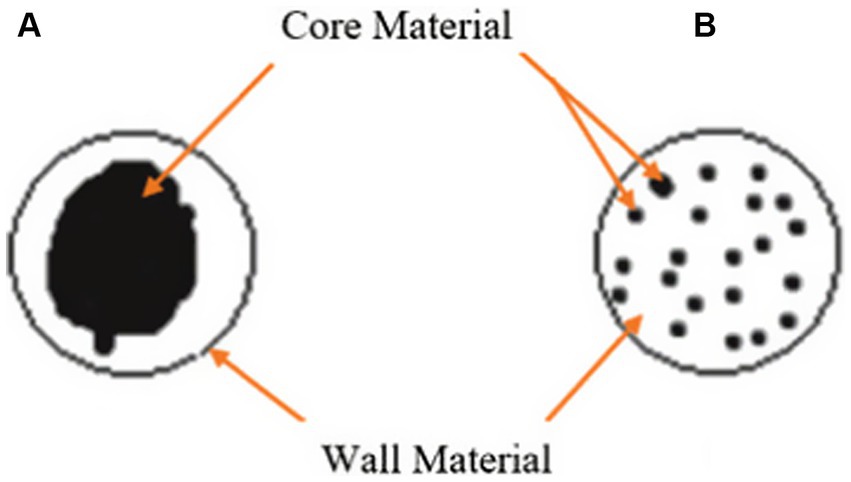
Figure 3. Schematic diagrams of two representative types of microencapsulation preparations, (A) Microcapsule, (B) Microsphere (63).
The primary objective of microencapsulation is to protect the encapsulated enzyme from harsh environmental conditions, such as pH, temperature, and oxidative stress, thereby enhancing its stability and prolonging its shelf life (54). The encapsulation process creates a barrier between the enzyme and its surroundings, preventing degradation and maintaining its activity during storage or when exposed to unfavourable conditions. Table 2 shows a summary of studies on microencapsulation techniques for enzyme protection against environmental stress.
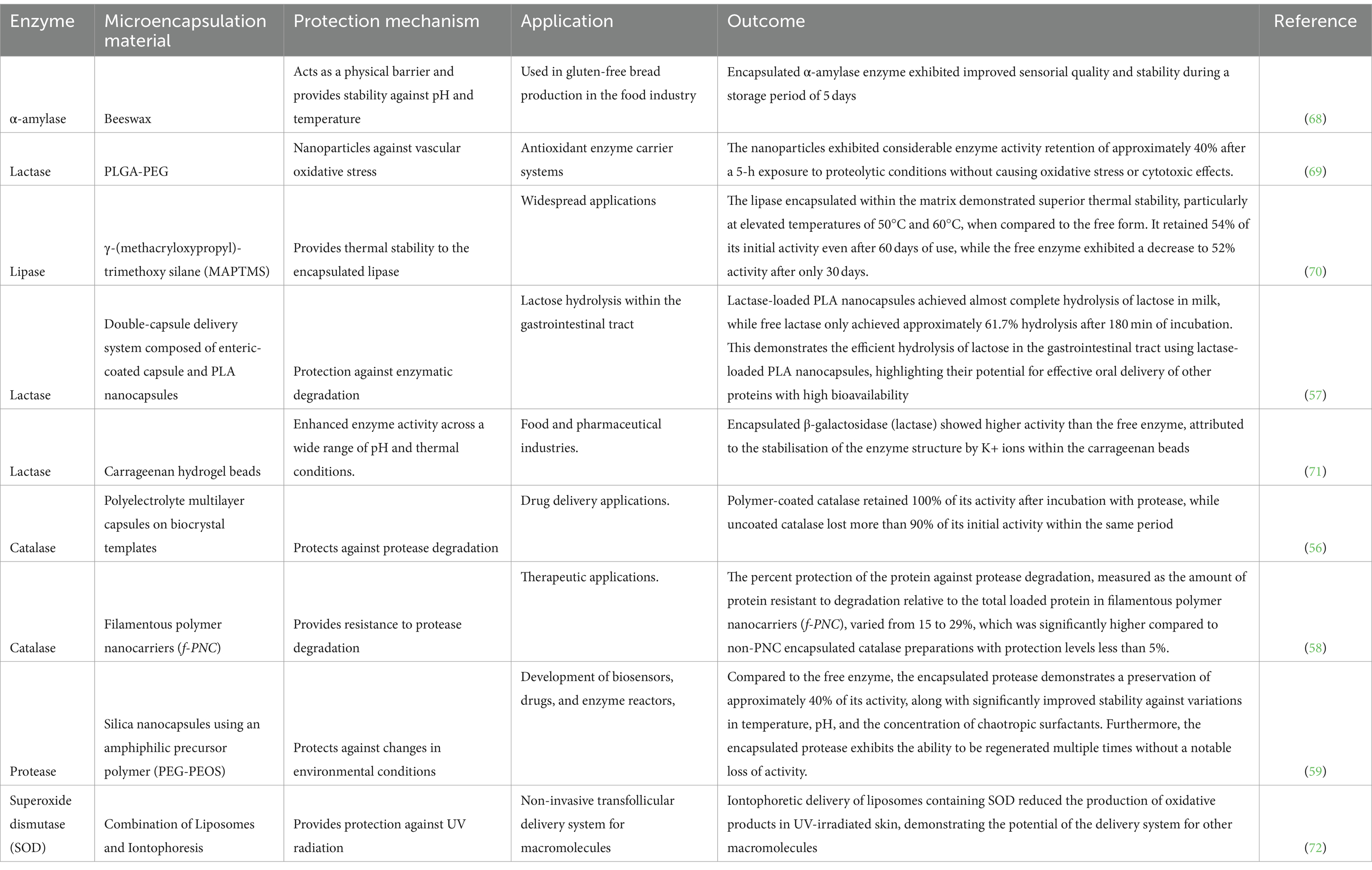
Table 2. Summary of relevant studies on microencapsulation techniques for enzyme protection against environmental stress.
One of the significant advantages of microencapsulation is its ability to improve the stability of enzymes. Studies have shown a significant increase in the thermal stability of encapsulated enzymes compared to their free counterparts. For example, microencapsulation of phytase by alginate beads was reported to significant increase the stability of the enzyme from 20 to 79% (73). In Another study conducted by Zhao et al. (74), lipase encapsulation resulted in the preservation of 87.5% of its activity following exposure to a thermal treatment at 70°C for 2 h, whereas the unencapsulated enzyme experienced a substantial loss of 99.5% activity under identical conditions. Similarly, Yang et al. (70) noted that lipase encapsulated within a matrix retained higher activity at elevated temperatures (50°C and 60°C) for an extended period compared to the free form. This enhanced thermal stability is attributed to the protective effect of the encapsulating material, which acts as a shield against heat-induced denaturation and degradation. Furthermore, microencapsulation provides a buffer against pH variations, maintaining enzyme activity over a broader pH range. Zhang et al. (59) found that encapsulated protease retained approximately 40% of its activity under varying pH conditions, indicating improved stability compared to the free enzyme. This stability is crucial for applications in diverse pH environments, such as industrial processes or gastrointestinal delivery. Additionally, Free enzymes are susceptible to proteolytic degradation, limiting their functionality in biological systems. However, encapsulation offers protection against proteases, as observed by Sari et al. (69) and Simone et al. (58). These studies reported significant enzyme activity retention even after exposure to proteolytic conditions, highlighting the potential of encapsulation in enhancing enzyme stability in biological fluids or environments rich in proteases.
In addition to improving stability, microencapsulation offers several other benefits for enzymes. It allows controlled release of the encapsulated enzyme, enabling targeted delivery to specific sites or controlled release over a prolonged period (75, 76). This controlled release is particularly advantageous in applications where a sustained enzyme activity is required, such as in drug delivery systems or in biotechnology industries. The key parameters influencing release rates are interactions between the wall material and the core, core’s volatility, the ratio of the core to the wall material, and the wall material’s viscosity grade (77). The primary mechanisms involved in core release are shown in Table 3. More than one mechanism is used in practice (17).
Furthermore, microencapsulation provides a means to enhance the loading capacity of enzymes. The encapsulating material can accommodate a high concentration of enzymes, maximising their content within the microcapsules or microspheres. This high enzyme loading capacity ensures that a significant amount of active enzyme is available for the desired application. For example, the successful encapsulation of Flavourzyme® into a cross-linked chitosan matrix enabled improved loading capacity of Flavourzyme® in chitosan-TPP microparticles and could be a promising option for utilising commercial proteases in food processing (78). Thomas et al. (79) and Anjani (80) highlighted the efficacy of a microcapsule system in facilitating the efficient loading of enzymes while ensuring extended shelf life and sustained activity. Moreover, Weng et al. (73) successfully optimised the overall efficiency of phytase encapsulation by employing a three-fluid nozzle spray drying technique, resulting in a substantial enzyme loading capacity of 48 wt%. These findings collectively highlight the potential of different encapsulation strategies in enhancing enzyme loading and preserving their activity for diverse applications in various fields.
Overall, microencapsulation has emerged as a promising approach for the effective delivery and protection of water-soluble compounds, including enzymes. The technique offers improved stability, controlled release, and enhanced loading capacity, making it a valuable tool in various fields such as pharmaceuticals, biotechnology, food, and cosmetics. Further research and development in microencapsulation methods and materials hold great potential for advancing the application of enzymes in diverse industries.
4.1 Selection of encapsulating methods and wall materials
Microencapsulation techniques are classified into three groups: (i) physicochemical methods such as thermal gelation, coacervation (phase separation), and emulsification, (ii) chemical methods including polymerisation and interfacial polycondensation, (iii) physical (mechanical) methods like solvent evaporation, spray drying, fluidized bed coating, centrifugation, and extrusion (81, 82). Blaine (52) listed five techniques that are often employed in the production of micro and nanoparticles, including fluidized bed coating, spray freezing, coacervation, extrusion, and spray drying. The most appropriate encapsulation method for a given application is determined by the core type, the microcapsule’s application, the particle size required, chemical and physical features of the wall and core materials, the required release mechanism, cost, and production scale (52, 54). There are several studies in the literature on the microencapsulation of various active compounds for food, feed, cosmetic and pharmaceutical applications. This literature review is focused on microencapsulation techniques that are relevant to water soluble actives such as enzymes.
The Encapsulating materials should be chosen carefully due to their impact on the capsule’s stability and efficiency. The choice of the encapsulating materials is considered as one of the most important and first steps in the development of encapsulation techniques. The materials are selected based on the active component, the core material, and the desired characteristics of the final product (53). The wall material should have the following characteristics to be successfully delivered to the desired location: (i) not reacting with the core, (ii) ability to keep the core sealed and stable inside the capsule, and (iii) ability to give optimum protection to the core against unfavourable circumstances (18, 19, 76, 83). To protect the core materials from ruminal degradation, wall materials should have the following specific properties: (i) be insoluble in an animal’s rumen and abomasum with a pH greater than 6.0 and less than 2.0 respectively, (ii) be able to withstand microbial attack, (iii) have mechanical qualities that allow them to endure breakage (20). However, as reported by Desai and Jin Park (17) most wall materials lack all of the desired qualities. Thus, a frequent approach involves combining two or more wall materials. Other essential properties to consider are cost-effectiveness and suitability and safety for feed application.
5 Microencapsulation application in the ruminant feed industry
In animal nutrition, microencapsulation can be utilised for a variety of applications. It can be used to deliver substances to a specific location of the gastrointestinal (GI) tract (i.e., targeting a particular site of the intestinal tract as a dietary component of the feed), to mask the flavour of products with undesirable flavour, to ensure product stability during long periods of storage, or to increase the bioavailability of a product to improve animal performance (84). Several studies have contributed to refining methods for preserving nutrient integrity during ruminal passage. Yoshimaru et al. (51) demonstrated the successful transit of spray-dried microcapsules of protease through the rumen with minimal degradation, releasing their content in the abomasum. Similarly, studies by Yoshimaru et al. (85) highlighted the potential of spray-dried L-lysine with zein to resist ruminal damage and pass through the rumen with minimal degradation. Recent investigations, such as (86), have explored microencapsulation with ethyl cellulose and carbomer to protect L-carnitine in the rumen, showcasing improved antioxidant capacity compared to unprotected supplementation. Likewise, encapsulation of soybean meal with zein or wheat gluten demonstrated enhanced ruminal protection compared to unprotected soybean meal (52).
The field has seen diverse approaches, including studies comparing microencapsulation methods and wall materials for nutrient protection. Chauhan and Gautam (87) assessed pan coating and fluidized bed coating methods, determining that choline chloride granules coated with cellulose-based polymer and hydrogenated vegetable oils using fluidized bed coating exhibited high rumen bypass potency. Conversely, Pretorius (88) found that evaluated wall materials like zein and kafirin displayed consistent release curves during ruminal transit, with protein coatings failing to provide complete protection. Chiang et al. (89) and Cao et al. (90) delved into the stability of protected amino acids and the controlled release of L-carnitine, emphasising the importance of coating composition and levels. Other authors have also highlighted the coating levels influenced the efficacy of active ingredients release at the desired location (86, 91–93). Notably, Ibrahim and Hassen (67) employed the solid-in-oil-in-water (S/O/W) technique to encapsulate Mimosa tannin, achieving smaller diameters, lower density, and high encapsulation efficiency. The study demonstrated controlled release dynamics, with a fraction released in the stimulated rumen, and specific percentages in the abomasum and small intestine.
Furthermore, Carrillo-Díaz et al. (94) stated that understanding the efficacy of encapsulated materials necessitates a consideration of various factors, including enzyme type and dose, forage composition, and the forage-to-concentrate ratio. In vitro results may not perfectly align with in vivo effects, highlighting the intricate interplay of exogenous fibrolytic enzymes (EFEs), ruminal fluid, and animal-specific conditions. In conclusion, numerous studies underscore the potential of microencapsulation in delivering various compounds to distinct regions of the ruminant GI tract. Despite the complexities involved, these endeavours collectively contribute to advancing our understanding of microencapsulation’s feasibility and efficacy in optimising nutrient delivery for improved animal nutrition and well-being.
6 Conclusion and future perspectives
Low feed utilisation efficiency is still a major problem in livestock production with forage as the main feedstock. Various approaches such as breeding and genetic approaches for breeds with higher feed utilisation efficiency and supplementing the feed with exogenous microorganisms and fibre-digesting enzymes have been evaluated for improving feed utilisation efficiency with different degree of success. The use of exogenous enzymes in particular has significant potential for enhancing fibre digestibility in rumen and post-rumen. However, better enzyme formulations to synergistically work with rumen microbial enzymes and delivery systems that protect exogenous enzymes during delivery to the rumen and beyond in the GI tract of ruminants are required in order to fully benefit from enzyme supplementation. In this regard, the use of microencapsulation for protected and targeted delivery of enzymes has a huge potential. Improving feed utilisation rate in ruminants, will result in increasing production of milk and meat for human consumption and improving the efficiency of livestock production in a more cost-effective manner as well as reducing environmental footprint. Although many studies and reviews on the delivery of encapsulated active compounds in ruminants have been undertaken, it is still unknown if the delivery of encapsulated enzymes in active form to the rumen and the hindgut of ruminants would be successful. Therefore, further studies are needed to develop microencapsulation approaches that enable the delivery of enzymes at specific targets in the GI tract of ruminants. Additionally, studies evaluating the stability of encapsulated enzymes under simulated gastrointestinal conditions, such as variations in pH, temperature, and enzymatic activity are needed. Assessing the bioavailability and efficacy of encapsulated enzymes in vivo is essential to determine their impact on fibre digestion and feed utilisation efficiency in ruminants. Investigating the long-term effects of microencapsulated enzyme supplementation on animal health, productivity, and performance is also critical for ensuring safety and sustainability. These studies will help refine microencapsulation approaches for enzyme delivery in ruminants, ultimately enhancing fibre digestion, feed utilisation efficiency, and livestock productivity in a sustainable manner.
Author contributions
NA: Visualization, Conceptualization, Investigation, Writing – original draft, Writing – review & editing. FT: Funding acquisition, Resources, Supervision, Writing – review & editing. NT: Conceptualization, Funding acquisition, Resources, Supervision, Writing – review & editing.
Funding
The author(s) declare that financial support was received for the research, authorship, and/or publication of this article. The authors declare that this study received funding from ProAgni Pty Ltd. The funder was not involved in the study design, collection, analysis, interpretation of data, the writing of this article, or the decision to submit it for publication.
Acknowledgments
The authors gratefully acknowledge the support of Commonwealth Scientific and Industrial Research Organization (CSIRO), the University of New South Wales (UNSW), Sydney, Australia in the framework of the excellence initiative and funding.
Conflict of interest
The authors declare that the research was conducted in the absence of any commercial or financial relationships that could be construed as a potential conflict of interest.
The author(s) declared that they were an editorial board member of Frontiers, at the time of submission. This had no impact on the peer review process and the final decision.
Publisher’s note
All claims expressed in this article are solely those of the authors and do not necessarily represent those of their affiliated organizations, or those of the publisher, the editors and the reviewers. Any product that may be evaluated in this article, or claim that may be made by its manufacturer, is not guaranteed or endorsed by the publisher.
References
1. Delano, ML, Mischler, SA, and Underwood, WJ. Biology and diseases of ruminants: sheep, goats, and cattle. Laboratory Animal Med. (2002) 519:611. doi: 10.1016/B978-012263951-7/50017-X
2. Xie, F, Jin, W, Si, H, Yuan, Y, Tao, Y, Liu, J, et al. An integrated gene catalog and over 10,000 metagenome-assembled genomes from the gastrointestinal microbiome of ruminants. Microbiome. (2021) 9:137. doi: 10.1186/s40168-021-01078-x
3. Parish, JA, Rivera, JD, and Boland, HT. Understanding the ruminant animal digestive system ruminant. Mississippi State University. (2017):1–7. doi: 10.1088/1755-1315/663
4. Castillo-González, AR, Burrola-Barraza, ME, Viveros, J, and Chavez-Martinez, A. Rumen microorganisms and fermentation. Archivos de medicina veterinaria. (2013) 46:349–61. doi: 10.4067/S0301-732X2014000300003
5. Krause, DO, Denman, SE, Mackie, RI, Morrison, M, Rae, AL, Attwood, GT, et al. Opportunities to improve fiber degradation in the rumen: microbiology, ecology, and genomics. FEMS Microbiol Rev. (2003) 27:663–93. doi: 10.1016/S0168-6445(03)00072-X
6. Gerber, P, Vellinga, T, Opio, C, and Steinfeld, H. Productivity gains and greenhouse gas emissions intensity in dairy systems. Livest Sci. (2011) 139:100–8. doi: 10.1016/j.livsci.2011.03.012
7. Shibata, M, and Terada, F. Factors affecting methane production and mitigation in ruminants. Anim Sci J. (2010) 81:2–10. doi: 10.1111/j.1740-0929.2009.00687.x
8. Varga, GA, and Kolver, ES. Microbial and animal limitations to Fiber digestion and utilization. J Nutr. (1997) 127:819S–23S. doi: 10.1093/jn/127.5.819S
9. Keomanivong, FE . The impact of digestive enzymes in the ruminant animal. Fargo, North Dakota: North Dakota State University (2016).
10. Lee, SS, Ha, JK, and Cheng, KJ. Influence of an anaerobic fungal culture administration on in vivo ruminal fermentation and nutrient digestion. Anim Feed Sci Technol. (2000) 88:201–17. doi: 10.1016/S0377-8401(00)00216-9
11. Rode, LM, Mcallister, TA, Beauchemin, KA, Morgavi, DP, Nsereko, VL, Yang, WZ, et al. Enzymes as direct-feed additives for ruminants In: R Renaville and A Burny, editors. Biotechnology in animal husbandry. Dordrecht: Springer Netherlands (2002)
12. Beauchemin, KA, Colombatto, D, and Morgavi, DP. A rationale for the development of feed enzyme products for ruminants. Can J Anim Sci. (2004) 84:23–36. doi: 10.4141/A02-103
13. Meale, SJ, Beauchemin, KA, Hristov, AN, Chaves, AV, and Mcallister, TA. Board-invited review: opportunities and challenges in using exogenous enzymes to improve ruminant production. J Anim Sci. (2014) 92:427–42. doi: 10.2527/jas.2013-6869
14. Beauchemin, KA, Colombatto, D, Morgavi, DP, Yang, WZ, and Rode, LM. Mode of action of exogenous cell wall degrading enzymes for ruminants. Can J Anim Sci. (2004) 84:13–22. doi: 10.4141/A02-102
15. Dias, MI, Ferreira, ICFR, and Barreiro, MF. Microencapsulation of bioactives for food applications. Food Funct. (2015) 6:1035–52. doi: 10.1039/C4FO01175A
16. Gürbüz, E, Keresteci, B, Günneç, C, and Baysal, G. Encapsulation applications and production techniques in the food industry. J Nutr Health Sci. (2020) 7:106.
17. Desai, KGH, and Jin Park, H. Recent developments in microencapsulation of food ingredients. Dry Technol. (2005) 23:1361–94. doi: 10.1081/DRT-200063478
18. Gharsallaoui, A, Roudaut, G, Chambin, O, Voilley, A, and Saurel, R. Applications of spray-drying in microencapsulation of food ingredients: an overview. Food Res Int. (2007) 40:1107–21. doi: 10.1016/j.foodres.2007.07.004
19. Shahidi, F, and Han, XQ. Encapsulation of food ingredients. Crit Rev Food Sci Nutr. (1993) 33:501–47. doi: 10.1080/10408399309527645
20. Sahraei Belverdy, M., Assadi-Alamouti, A., and Azizi, M. (2019). Microencapsulation in the ruminant feed industry. Animals. (2020).
21. Mottin, C, Ornaghi, MG, Carvalho, VM, Guerrero, A, Vital, ACP, Ramos, TR, et al. Carcass characteristics and meat evaluation of cattle finished in temperate pasture and supplemented with natural additive containing clove, cashew oil, castor oils, and a microencapsulated blend of eugenol, thymol, and vanillin. J Sci Food Agric. (2022) 102:1271–80. doi: 10.1002/jsfa.11465
22. Nguyen, QV, Le, HV, Nguyen, DV, Nish, P, Otto, JR, Malau-Aduli, BS, et al. Supplementing dairy ewes grazing low quality pastures with plant-derived and rumen-protected oils containing Eicosapentaenoic acid and docosahexaenoic acid pellets increases body condition score and Milk, fat, and protein yields. Animals. (2018) 8:16. doi: 10.3390/ani8120241
23. Bonomi, A . Dairy cattle ration integration with rumen-protected pantothenic acid. Effects on milk production and reproductive efficiency. Rivista di Scienza dell'Alimentazione. (2000) 29:321–38.
24. Bonomi, A, Quarantelli, A, Sabbioni, A, and Superchi, P. Protected B vitamins for dairy cattle ration integration: effects on productivity and reproductive efficiency (experimental contribution). Rivista di Scienza dell'Alimentazione. (1993) 22:335–49.
25. Huhtanen, P., Ahvenjärvi, S., Weisbjerg, M. R., and Nørgaard, P. Digestion and passage of fibre in ruminants. Leiden, The Netherlands: Wageningen Academic (2006).
26. Moran, J . Tropical dairy farming: Feeding Management for Small Holder Dairy Farmers in the humid tropics. Australia: Department of primary industries, Csiro, Landlinks Press (2005).
27. Godoy-Vitorino, F, Goldfarb, KC, Karaoz, U, Leal, S, Garcia-Amado, MA, Hugenholtz, P, et al. Comparative analyses of foregut and hindgut bacterial communities in hoatzins and cows. ISME J. (2012) 6:531–41. doi: 10.1038/ismej.2011.131
28. Cheng, K-J, Forsberg, C, Minato, H, and Costerton, J. Microbial ecology and physiology of feed degradation within the rumen. Physiological aspects of digestion and metabolism in ruminants. US. Elsevier: Academic Press (1991).
29. Kung, L . The role of Fiber in ruminant ration formulation. Australia: Department of Animal & Food Sciences, University of Delaware (2014).
30. Hoover, WH . Chemical factors involved in ruminal Fiber Digestion1. J Dairy Sci. (1986) 69:2755–66. doi: 10.3168/jds.S0022-0302(86)80724-X
31. Allen, MS, and Mertens, DR. Evaluating constraints on Fiber digestion by rumen microbes. J Nutr. (1988) 118:261–70. doi: 10.1093/jn/118.2.261
32. Cardozo, PW, Calsamiglia, S, Ferret, A, and Kamel, C. Screening for the effects of natural plant extracts at different pH on in vitro rumen microbial fermentation of a high-concentrate diet for beef cattle1. J Anim Sci. (2005) 83:2572–9. doi: 10.2527/2005.83112572x
33. Evans, C., Irving, H., Vehmanperä, J., Juntunen, K., Patience, J., Li, Q., et al. (2022). Enzymes in farm animal nutrition. cabi.
34. Hristov, AN, Basel, CE, Melgar, A, Foley, AE, Ropp, JK, Hunt, CW, et al. Effect of exogenous polysaccharide-degrading enzyme preparations on ruminal fermentation and digestibility of nutrients in dairy cows. Anim Feed Sci Technol. (2008) 145:182–93. doi: 10.1016/j.anifeedsci.2007.05.051
35. Klingerman, CM, Hu, W, Mcdonell, EE, Derbedrosian, MC, and Kung, L. An evaluation of exogenous enzymes with amylolytic activity for dairy cows. J Dairy Sci. (2009) 92:1050–9. doi: 10.3168/jds.2008-1339
36. Brufau, J, Francesch, M, and Pérez-Vendrell, AM. The use of enzymes to improve cereal diets for animal feeding. J Sci Food Agric. (2006) 86:1705–13. doi: 10.1002/jsfa.2557
37. Azzaz, HH, Abd El Tawab, AM, Khattab, MSA, Szumacher-Strabel, M, Cieślak, A, Murad, HA, et al. Effect of Cellulase enzyme produced from Penicilliumchrysogenum on the Milk production, composition, amino acid, and fatty acid profiles of Egyptian buffaloes fed a high-forage diet. Animals. (2021) 11:3066. doi: 10.3390/ani11113066
38. Alonso, MU, Camacho, LM, Cipriano, M, Kholif, AM, Lopez, S, Mariezcurrena, MD, et al. Effects of xylanase supplementation on feed intake, digestibility and ruminal fermentation in Rambouillet sheep. J Agric Sci. (2016) 154:1110–7. doi: 10.1017/S0021859616000216
39. Tirado-González, DN, Tirado-Estrada, G, Miranda-Romero, LA, Ramírez-Valverde, R, Medina-Cuéllar, SE, and Salem, AZM. Effects of addition of exogenous Fibrolytic enzymes on digestibility and Milk and meat production – A systematic review. Ann Anim Sci. (2021) 21:1159–92. doi: 10.2478/aoas-2021-0001
40. Lunagariya, P, Gupta, R, Parnerkar, S, Mehta, BM, and Hadiya, KK. Effect of exogenous fibrolytic enzymes in total mixed ration on milk yield, composition, feed efficiency in Holstein Friesian crossbred cows. Indian J Anim Sci. (2019) 89:876–80.
41. Zilio, EMC, Del Valle, TA, Ghizzi, LG, Takiya, CS, Dias, MSS, Nunes, AT, et al. Effects of exogenous fibrolytic and amylolytic enzymes on ruminal fermentation and performance of mid-lactation dairy cows. J Dairy Sci. (2019) 102:4179–89. doi: 10.3168/jds.2018-14949
42. Arif, M, Al-Sagheer, AA, Salem, AZM, El-Hack, MEA, Swelum, AA, Saeed, M, et al. Influence of exogenous fibrolytic enzymes on milk production efficiency and nutrient utilization in early lactating buffaloes fed diets with two proportions of oat silage to concentrate ratios. Livest Sci. (2019) 219:29–34. doi: 10.1016/j.livsci.2018.11.007
43. Kholif, AE, Abdo, MM, Anele, UY, El-Sayed, MM, and Morsy, TA. Saccharomyces cerevisiae does not work synergistically with exogenous enzymes to enhance feed utilization, ruminal fermentation and lactational performance of Nubian goats. Livest Sci. (2017) 206:17–23. doi: 10.1016/j.livsci.2017.10.002
44. Lu, Q, Jiao, J, Tang, S, He, Z, Zhou, C, Han, X, et al. Effects of dietary cellulase and xylanase addition on digestion, rumen fermentation and methane emission in growing goats. Arch Anim Nutr. (2015) 69:251–66. doi: 10.1080/1745039X.2015.1039760
45. Dean, D, Staples, C, Kim, SC, and Adesogan, A. Effect of method of adding a Fibrolytic enzyme to dairy cow diets on feed intake digestibility, Milk production, ruminal fermentation, and blood metabolites. Anim Nutr Feed Technol. (2013) 13:337–53.
46. Miller, DR, Elliott, R, and Norton, BW. Effects of an exogenous enzyme, Roxazyme® G2, on intake, digestion and utilisation of sorghum and barley grain-based diets by beef steers. Anim Feed Sci Technol. (2008) 145:159–81. doi: 10.1016/j.anifeedsci.2007.05.045
47. Lee, J-J, Seo, J, Jung, JK, Lee, J, Lee, J-H, and Seo, S. Effects of β-mannanase supplementation on growth performance, nutrient digestibility, and nitrogen utilization of Korean native goat (Capra hircus coreanae). Livest Sci. (2014) 169:83–7. doi: 10.1016/j.livsci.2014.08.018
48. Mendoza, GD, Loera-Corral, O, Plata-Pérez, FX, Hernández-García, PA, and Ramírez-Mella, M. Considerations on the use of exogenous fibrolytic enzymes to improve forage utilization. Sci World J. (2014) 2014:247437. doi: 10.1155/2014/247437
49. Bartosz, T, and Irene, T. Polyphenols encapsulation – application of innovation technologies to improve stability of natural products. Physical Sci Rev. (2016) 1:11. doi: 10.1515/psr-2015-0005
50. Amin, N, Tagliapietra, F, Arango, S, Guzzo, N, and Bailoni, L. Free and microencapsulated essential oils incubated in vitro: ruminal stability and fermentation parameters. Animals. (2021) 11:180. doi: 10.3390/ani11010180
51. Yoshimaru, T, Shibata, M, Fukugomori, T, and Matsumoto, K. Preparation and characteristics of rumen-bypass microcapsules for improvement of productivity in ruminants. J Agric Food Chem. (1999) 47:554–7. doi: 10.1021/jf980708l
52. Blaine, KL . Development of protective films for enhancing ruminal bypass of micronutrients. Manhattan, Kansas: Kansas State University (2011).
53. Ozturk, E, and Temiz, U. Encapsulation methods and use in animal nutrition. Selcuk J Agricul Food Sci. (2018) 32:624–31. doi: 10.15316/SJAFS.2018.145
54. Suave, J, Dallagnol, E, Pezzin, A, Silva, D, Meier, M, and Soldi, V. Microencapsulação: Inovação em diferentes áreas. Revista Saúde e Ambiente/Health Environ J. (2006) 7:12–20.
55. Roy Choudhury, AK . Introduction to enzymes In: R Nayak , editor. Sustainable Technologies for Fashion and Textiles. UK: Woodhead Publishing (2020).
56. Caruso, F, Trau, D, Möhwald, H, and Renneberg, R. Enzyme encapsulation in layer-by-layer engineered polymer multilayer capsules. Langmuir. (2000) 16:1485–8. doi: 10.1021/la991161n
57. He, H, Zhang, X, and Sheng, Y. Enteric-coated capsule containing β-galactosidase-loaded polylactic acid nanocapsules: enzyme stability and milk lactose hydrolysis under simulated gastrointestinal conditions. J Dairy Res. (2014) 81:479–84. doi: 10.1017/S0022029914000491
58. Simone, EA, Dziubla, TD, Discher, DE, and Muzykantov, VR. Filamentous polymer Nanocarriers of tunable stiffness that encapsulate the therapeutic enzyme catalase. Biomacromolecules. (2009) 10:1324–30. doi: 10.1021/bm900189x
59. Zhang, C, Yan, K, Hu, C, Zhao, Y, Chen, Z, Zhu, X, et al. Encapsulation of enzymes in silica nanocapsules formed by an amphiphilic precursor polymer in water. J Mater Chem B. (2015) 3:1261–7. doi: 10.1039/C4TB01701C
60. Andrea, T, Marcela, F, Lucía, C, Esther, F, Elena, M, and Simona, M. Microencapsulation of lipase and Savinase enzymes by spray drying using Arabic gum as wall material. J Encapsulation Adsorption Sci. (2016) 6:161–73. doi: 10.4236/jeas.2016.64012
61. Ré, IM . Microencapsulation by spray drying. Dry Technol. (1998) 16:1195–236. doi: 10.1080/07373939808917460
62. Miles, DM . Stabilisation and encapsulation studies on xylanase for animal feed improvement. UK: University of Leeds (2004).
63. Bhutkar, S, and Shanmuganathan, K. Polymer based microcapsules for encapsulation In: J Parameswaranpillai, V Salim, H Pulikkalparambil, S Mavinkere Rangappa, and IH Suchart Siengchin, editors. Micro- and Nano-containers for smart applications. Singapore: Springer Singapore (2022)
64. Lengyel, M, Kállai-Szabó, N, Antal, V, Laki, AJ, and Antal, I. Microparticles, microspheres, and microcapsules for advanced drug delivery. Sci Pharm. (2019) 87:20. doi: 10.3390/scipharm87030020
65. Ma, G, and Yue, H. Advances in uniform polymer microspheres and microcapsules: preparation and biomedical applications. Chin J Chem. (2020) 38:911–23. doi: 10.1002/cjoc.202000135
66. Sbehat, M, Mauriello, G, and Altamimi, M. Microencapsulation of probiotics for food functionalization. Literature Rev Microorgan. (2022) 10:1948. doi: 10.3390/microorganisms10101948
67. Ibrahim, SL, and Hassen, A. Characterization, density and in vitro controlled release properties of Mimosa (Acacia mearnsii) tannin encapsulated in palm and sunflower oils. Animals. (2021) 11:2919. doi: 10.3390/ani11102919
68. Haghighat-Kharazi, S, Milani, JM, Kasaai, MR, and Khajeh, K. Microencapsulation of α-amylase in beeswax and its application in gluten-free bread as an anti-staling agent. Lwt. (2018) 92:73–9. doi: 10.1016/j.lwt.2018.01.049
69. Sari, E, Tunc-Sarisozen, Y, Mutlu, H, Shahbazi, R, Ucar, G, and Ulubayram, K. Icam-1 targeted catalase encapsulated Plga-b-peg nanoparticles against vascular oxidative stress. J Microencapsul. (2015) 32:687–98. doi: 10.3109/02652048.2015.1073384
70. Yang, G, Wu, J, Xu, G, and Yang, L. Improvement of catalytic properties of lipase from Arthrobacter sp. by encapsulation in hydrophobic sol–gel materials. Bioresour Technol. (2009) 100:4311–6. doi: 10.1016/j.biortech.2009.03.069
71. Zhang, Z, Zhang, R, Chen, L, and Mcclements, DJ. Encapsulation of lactase (β-galactosidase) into κ-carrageenan-based hydrogel beads: impact of environmental conditions on enzyme activity. Food Chem. (2016) 200:69–75. doi: 10.1016/j.foodchem.2016.01.014
72. Kigasawa, K, Miyashita, M, Kajimoto, K, Kanamura, K, Harashima, H, and Kogure, K. Efficient intradermal delivery of superoxide dismutase using a combination of liposomes and iontophoresis for protection against Uv-induced skin damage. Biol Pharm Bull. (2012) 35:781–5. doi: 10.1248/bpb.35.781
73. Weng, Y, Ranaweera, S, Zou, D, Cameron, AP, Chen, X, Song, H, et al. Improved enzyme thermal stability, loading and bioavailability using alginate encapsulation. Food Hydrocoll. (2023) 137:108385. doi: 10.1016/j.foodhyd.2022.108385
74. Zhao, ZY, Liu, J, Hahn, M, Qiao, S, Middelberg, APJ, and He, L. Encapsulation of lipase in mesoporous silica yolk–shell spheres with enhanced enzyme stability. RSC Adv. (2013) 3:22008. doi: 10.1039/c3ra43382j
75. Gouin, S . Microencapsulation: industrial appraisal of existing technologies and trends. Trends Food Sci Technol. (2004) 15:330–47. doi: 10.1016/j.tifs.2003.10.005
76. Silva, PTD, Fries, LLM, Menezes, CRD, Holkem, AT, Schwan, CL, Wigmann, ÉF, et al. Microencapsulation: concepts, mechanisms, methods and some applications in food technology. Ciência Rural. (2014) 44:1304–11. doi: 10.1590/0103-8478cr20130971
77. Roberts, DD, and Taylor, AJ. Flavor release: A rationale for its study. US: American Chemical Society (2000).
78. Busto, MD, González-Temiño, Y, Albillos, SM, Ramos-Gómez, S, Pilar-Izquierdo, MC, Palacios, D, et al. Microencapsulation of a commercial food-grade protease by spray drying in cross-linked chitosan particles. Food Secur. (2022) 11:2077. doi: 10.3390/foods1142077
79. Thomas, GM, Shouyuan, J, Chantal, K, Chavinya, DR, Gagan, KJ, Jelena, R-K, et al. Gas modulating microcapsules for spatiotemporal control of hypoxia. bioRxiv. (2022):506302. doi: 10.1101/2022.09.02.506302
80. Anjani, K. Microencapsulation of flavour-enhancing enzymes for acceleration of cheddar cheese ripening. Sydney: Western Sydney University (2007).
81. Caballero, B., Trugo, L., and Finglas, P. Encyclopedia of food sciences and nutrition. Elsevier Science BV (2003) 1–10.
82. Sahoo, CK, Sahoo, NK, Rao, SRM, Sudhakar, M, and Satyanarayana, K. Drug delivery systems In: ML Bruschi , editor. Strategies to modify the drug release from pharmaceutical systems. UK: Woodhead Publishing (2015).
83. Nazzaro, F, Orlando, P, Fratianni, F, and Coppola, R. Microencapsulation in food science and biotechnology. Curr Opin Biotechnol. (2012) 23:182–6. doi: 10.1016/j.copbio.2011.10.001
84. Pereira, A., Greene, L., and Samuels, W. A. (2008). Microencapsulation to the Maxx: A new delivery system for food and bioactive ingredients in animals.
85. Yoshimaru, T, Takahashi, H, and Matsumoto, K. Microencapsulation of L-lysine for improving the balance of amino acids in ruminants. J Faculty Agricul Kyushu University. (2000) 44:359–65. doi: 10.5109/24338
86. Zheng, L, Han, R, Jiang, W, Chen, L, Yu, W, Zhong, W-G, et al. Evaluation of unprotected and rumen-protected L-carnitine in vitro and in vivo. Can J Anim Sci. (2021) 102:232–242. doi: 10.1139/cjas-2021-0006
87. Chauhan, S, and Gautam, B. In-vitro study on dissolution profiling of choline chloride encapsulated by different microencapsulation technologies and active forms. Indian J Animal Nutrition. (2020) 37:44–7. doi: 10.5958/2231-6744.2020.00008.0
88. Pretorius, C . Kafirin and zein as coatings for the controlled release of amino acid supplements. South Africa: University of Pretoria (2011).
89. Chiang, Y-W, Wang, T-H, and Lee, W-C. Chitosan coating for the protection of amino acids that were entrapped within hydrogenated fat. Food Hydrocoll. (2009) 23:1057–61. doi: 10.1016/j.foodhyd.2008.04.007
90. Cao, Q-R, Lee, E-S, Choi, Y-J, Cho, C-S, and Lee, B-J. Rumen bypass and biodistribution of l-carnitine from dual-layered coated pellets in cows, in vitro and in vivo. Int J Pharm. (2008) 359:87–93. doi: 10.1016/j.ijpharm.2008.03.017
91. Kuang, SS, Oliveira, JC, and Crean, AM. Microencapsulation as a tool for incorporating bioactive ingredients into food. Crit Rev Food Sci Nutr. (2010) 50:951–68. doi: 10.1080/10408390903044222
92. Pandey, S, Swamy, SMV, Gupta, A, Koli, A, Patel, S, Maulvi, F, et al. Multiple response optimisation of processing and formulation parameters of pH sensitive sustained release pellets of capecitabine for targeting colon. J Microencapsul. (2018) 35:259–71. doi: 10.1080/02652048.2018.1465138
93. Sprockel, OL, and Price, JC. Development of an emulsion-solvent evaporation technique for microencapsulation of Drus-resin complexes. Drug Dev Ind Pharm. (1990) 16:361–76. doi: 10.3109/03639049009114891
94. Carrillo-Díaz, M. I., Miranda-Romero, L. A., Chávez-Aguilar, G., Zepeda-Batista, J. L., González-Reyes, M., García-Casillas, A. C., et al. (2022). Improvement of ruminal neutral detergent fiber degradability by obtaining and using exogenous fibrolytic enzymes from white-rot fungi. Animals 12, 843.
Keywords: ruminants digestive system, undigested fibre, feed utilisation, enzyme delivery, microencapsulation
Citation: Almassri N, Trujillo FJ and Terefe NS (2024) Microencapsulation technology for delivery of enzymes in ruminant feed. Front. Vet. Sci. 11:1352375. doi: 10.3389/fvets.2024.1352375
Edited by:
Jean-Christophe Jacquier, University College Dublin, IrelandReviewed by:
Shad Mahfuz, Sylhet Agricultural University, BangladeshSercan Karav, Çanakkale Onsekiz Mart University, Türkiye
Copyright © 2024 Almassri, Trujillo and Terefe. This is an open-access article distributed under the terms of the Creative Commons Attribution License (CC BY). The use, distribution or reproduction in other forums is permitted, provided the original author(s) and the copyright owner(s) are credited and that the original publication in this journal is cited, in accordance with accepted academic practice. No use, distribution or reproduction is permitted which does not comply with these terms.
*Correspondence: Netsanet Shiferaw Terefe, bmV0c2FuZXQuc2hpZmVyYXd0ZXJlZmVAY3Npcm8uYXU=