- 1Department of Clinical Veterinary Medicine, College of Veterinary Medicine, China Agricultural University, Beijing, China
- 2NHC Key Laboratory of Transplant Engineering and Immunology, Frontiers Science Center for Disease-related Molecular Network, West China Hospital, Sichuan University, Chengdu, China
Extracellular vesicles are nanoscale vesicles that transport signals between cells, mediating both physiological and pathological processes. EVs facilitate conserved intercellular communication. By transferring bioactive molecules between cells, EVs coordinate systemic responses, regulating homeostasis, immunity, and disease progression. Given their biological importance and involvement in pathogenesis, EVs show promise as biomarkers for veterinary diagnosis, and candidates for vaccine production, and treatment agents. Additionally, different treatment or engineering methods could be used to boost the capability of extracellular vesicles. Despite the emerging veterinary interest, EV research has been predominantly human-based. Critical knowledge gaps remain regarding isolation protocols, cargo loading mechanisms, in vivo biodistribution, and species-specific functions. Standardized methods for veterinary EV characterization and validation are lacking. Regulatory uncertainties impede veterinary clinical translation. Advances in fundamental EV biology and technology are needed to propel the veterinary field forward. This review introduces EVs from a veterinary perspective by introducing the latest studies, highlighting their potential while analyzing challenges to motivate expanded veterinary investigation and translation.
1 Introduction
Extracellular vesicles (EVs), characterized by lipid bilayer structures, constitute a diverse group of nanovesicles released by various cell types (1). These vesicles play a crucial role in mediating cell-to-cell communication by transferring a series of cargoes, including proteins, RNAs, DNAs, and lipids, thereby influencing normal physiological processes or pathological conditions (2). Notably, EVs act as biomarkers of multiple diseases, such as cardiovascular disease, cancer, and neurodegenerative disease (3), due to their ability to directly reflect the state of their donor cells or tissues. In addition, an increasing number of studies have shown that EVs from different sources have excellent therapeutic potential in various tissue injuries, including cancer, wound healing, and inflammatory disorders (4, 5). Notably, some products related to EVs are already in different stages of clinical trials. For example, ExoFlo™, an EV-based product by Direct Biologic LLC, has decreased the mortality rate associated with respiratory failure or moderate-to-severe acute respiratory distress syndrome (ARDS) due to COVID-19 (6, 7).
Despite the burgeoning interest in EVs within the realms of regenerative medicine and human disease diagnosis, their applicability in veterinary medicine remains relatively understudied. This oversight is attributed, in part, to the unique challenges encountered in veterinary practice, where clinicians operate in the field, relying on clinical signs and diagnostic tools with inherent size limitations. In contrast to human diagnostics, which benefit from readily accessible sampling and analysis settings, veterinary contexts demand rapid responses to prevent economic losses, particularly in the face of swiftly propagating infectious diseases. The imperative for expeditious and accessible diagnostic methods is thus crucial. For the prevention of disease in Veterinary practice, vaccines are one of the important methods. Comparing to traditional vaccine approaches that utilize killed or attenuated agents, EV-based vaccines could guarantee better safety and immunogenicity if utilize properly (8). Furthermore, the prevailing issues of food safety and antibiotic misuse, recognized as key obstacles to the health of both humans and animals, persist, prompting a heightened interest in antibiotic alternatives and targeted medicine within the field of veterinary medicine (9). Therapies based on EVs have shown the potential as strong candidates.
Therefore, the need for noninvasive, easily accessible, and cost-effective disease diagnosis, as well as safe non-hazardous prevention and treatment methods provides EV-based techniques with great opportunities. EVs can be isolated from small amounts of various body fluids, such as blood, urine, and saliva, which can benefit veterinarians when obtaining tissue samples is difficult. The use of native or engineered EVs as treatment agents possesses distinct advantages, including targeted delivery and biocompatibility, which directly address the unique demands of veterinary medicine. Overall, the development of EV technology in veterinary medicine could have great potential and lead to significant advances in the health of different animal species and diseases.
In this review, we focused on discussing the potential use of EVs in veterinary practice by introducing the background of EVs and related research, such as pre-diagnostic techniques, vaccine production, and novel treatments, to provide new insights into animal health. Due to the limitation of scope, only farm animals and pets, but not wildlife and marine species were included in this review.
2 Biological properties of EVs
There is sufficient evidence supporting that EVs play crucial roles in cellular and cross-organ communications because their bioactive cargos can regulate recipient cell signaling. In the following section, the biological properties of EVs are briefly introduced, including the biogenesis, isolation and characterization, cellular crosstalk, and cargos of EVs.
2.1 Biogenesis of EVs
EVs comprise a heterogeneous population of membrane vesicles that originate from different cells and are generated via diverse mechanisms. While the exact mechanisms of EVs are not elucidated, some studies suggest that several pathways are involved in forming different EV subtypes (Figure 1). Several subtypes of EVs have been identified based on their sizes and biogenesis, including exosomes (30–200 nm), microvesicles (200–1000 nm), and apoptotic bodies (1–5 μm) (10, 11). Moreover, according to the different sources of EVs, they can also be divided into eukaryotic-derived EVs (e.g., animal tissue/cells and plants) and prokaryotic-derived EVs (e.g., bacteria).
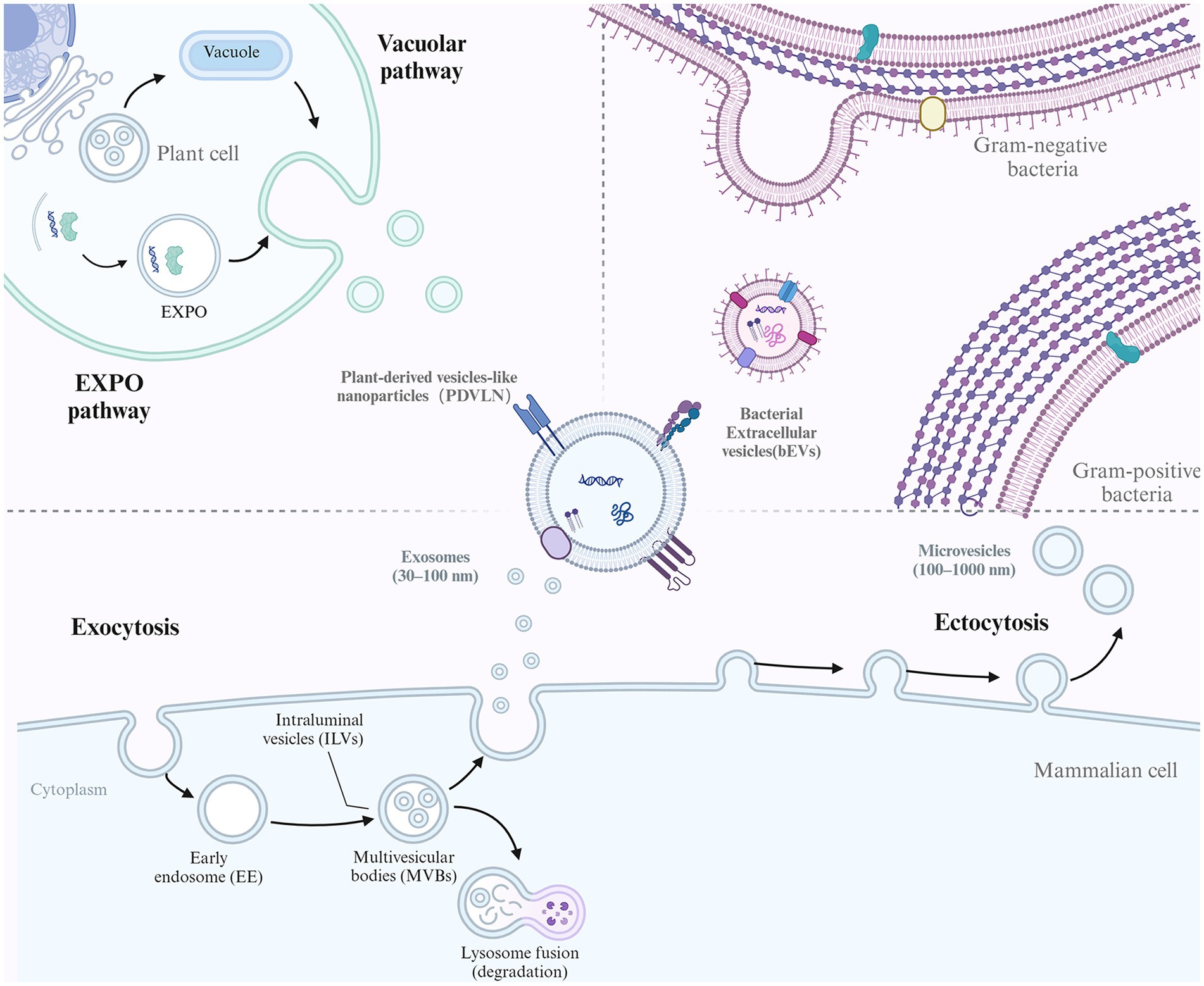
Figure 1. The biogenesis of different EVs. EVs conservatively produced by multiple organisms including mammalian cell, plants cell and Bacteria. For mammalian cell, the biogenesis of exosome and microvesicles (MVs) were illustrated. Exosome biogenesis begins with endocytosis of the plasma membrane to form early endosomes. These endosomes mature into multivesicular bodies (MVBs). The membrane of the MVBs invaginates inward, creating intraluminal vesicles (ILVs). Some MVBs then fuse with the cell membrane, releasing their ILVs as exosomes. In contrast, MVs mainly originate from outward budding of the plasma membrane. For plant cell, EXPO pathway and vacuolar pathway was shown. Bacteria can also secrete EVs as bacterial EVs (bEVs).
Exosomes are formed by the inward budding of endosomal membranes, resulting in multivesicular bodies (MVBs) (12). MVBs further bud to form multiple intraluminal vesicles (ILVs) and finally fuse with lysosomes for degradation or with the plasma membrane for the release of exosomes into the extracellular space. The sorting of specific biomolecules into exosomes is thought to be mediated by the endosomal sorting complex required for transport (ESCRT) machinery, which recognizes and packages specific cargoes into vesicles (13).
Microvesicles, also known as ectosomes or shedding vesicles, are formed by the outward budding of the plasma membrane (14). This process is thought to be mediated by the cytoskeleton and specific membrane-associated proteins, such as flotillin and tetraspanins. The sorting of specific biomolecules into microvesicles is less well understood than that in exosomes, but it is thought to be mediated by various mechanisms, including direct protein–protein interactions and lipid raft-mediated sorting (15). Apoptotic bodies are formed by the fragmentation of the dying cell’s plasma membrane and the condensation of its cytoplasm and organelles into large vesicles during the process of programmed cell death. The formation of apoptotic bodies occurs in several steps, including cell condensation and nucleus pyknosis, contraction of actin-myosin filaments causing blebbing of the plasma membrane, and large apoptotic bodies pinching off from the protrusions as blebbing continues (16). The sorting of specific biomolecules into apoptotic bodies is thought to be mediated by specific proteins and changes in membrane lipid composition (2).
Bacterial EVs (bEVs) are produced by both gram-negative and gram-positive bacteria and are known as outer membrane vesicles (OMVs) and membrane vesicles (MVs), respectively (17). In gram-negative bacteria, OMVs are released as the outer membrane bulges outwardly, disconnecting from the peptidoglycan layer. Overexpressed and misfolded periplasmic proteins can trigger OMV formation, and the VacJ/Yrb ATP-binding cassette transport system is involved in OMV formation (18). Deletion of the VacJ or Yrb gene results in phospholipid accumulation in the outer leaflet, which causes outward bulging of the OM (19). While the asymmetric expansion enlarged, the OMVs were secreted and enriched in phospholipids incorporated into the outer leaflet of the vesicle membrane (20). Gram-positive bacteria have a thick peptidoglycan cell wall and lack OMVs but were found to release MVs in approximately 2009 (21). The exact process is not clear, although it is hypothesized that MVs could be forced through the cell wall due to internal pressure or enzyme activity weakening the wall (22). For example, Staphylococcus aureus-derived MVs contain a peptidoglycan-degrading enzyme that is known to modify gram-positive cell walls (23). Thus, membrane fluidity and cell wall integrity might be the key factors that affect MV release activity.
Plants can also release EVs referred to as plant-derived vesicle-like nanoparticles (PDVLNs) (24). Compared to mammalian and microbial EVs, the biogenesis of PDVLNs is still in its infancy. Several models have been proposed for their secretion. MVB infusion with carrot cells is followed by the release of vesicle-like structures into the wall space (25). Another route of PDVLN release is through the exocyst-positive organelle (EXPO) pathway, a unique plant secretion pathway that was first observed in Arabidopsis (26). In addition, another potential pathway is the vacuolarulolar pathway. As a dynamic organelle of plant cells, vacuoles can release antibacterial and death-inducing proteins outside Arabidopsis cells by fusing with the plasma membrane. While the exact mechanism has not been elucidated, the different mechanisms observed may suggest the heterogeneity of PDVLNs.
2.2 EV components and crosstalk with cells
EVs play an important role in cell-to-cell communication through the transfer of their luminal and membrane cargoes. The membrane cargoes that are exposed on the surface of EVs are strictly associated with the membranous structures and interest researchers because they are more accessible to labeling and analytical approaches, while the luminal cargoes are thought to be the bioactive component that comes to therapeutic effects. As a membrane-originated substance, EVs are rich in lipid components such as cholesterol and sphingomyelin, which contribute to the stability of EVs (27). In EV biogenesis, different selection mechanisms contribute to their diverse protein components. Additionally, large amounts of RNA and DNA are observed in EVs (28). MicroRNA (miRNA), which is seen as a crucial part of immunomodulation, is detected broadly in various kinds of EVs. In addition, long non-coding RNA and mitochondrial DNA cargoes of EVs have also gained researchers’ interest. Overall, components in EVs are, by their nature combinatorial and contextually complex.
With an important role in cell-to-cell communication, EVs can interact with the target cell in different ways, including binding to the cell surface, fusing into the plasma membrane, and being taken up by endocytosis. The structure of EVs is similar to that of cells, with extracellular receptors and ligands positioned on the outside and cytoplasmic proteins and nucleic acids on the inside. EVs can directly interact with cognate receptors on the plasma membrane of the cell, which may serve as the targetability of EVs (29). For delivery of the cargo, EVs must fuse into the plasma membrane through endocytosis. Different factors may affect the process, such as the lipid content, environmental pH, and specific receptor-ligand fusion (30, 31). Cells appear to take up EVs by a variety of endocytic pathways, such as clathrin-dependent endocytosis, caveolin-mediated uptake, and micropinocytosis (31). Overall, the interaction mechanism of EVs and cells depends on the protein, glycoprotein, and lipid content on the surface.
2.3 Isolation and characterization of EVs
EVs can be isolated from various samples (e.g., blood, tissue, urine, milk) through different methods, including ultracentrifugation (UC), density gradient ultracentrifugation, immunoaffinity, ultrafiltration, and size exclusion chromatography (SEC). UC is one of the most mature methods of EV isolation as the gold standard, which separates EVs based on different sizes and densities using a sequence of centrifuge speeds (32). The exact method differs among researchers and samples, but the purpose could be divided into two parts: low-speed centrifugation for separating cells and debris and high-speed centrifugation for collecting EVs. However, with different speeds of centrifugation, it is difficult to identify EVs among other impurities (protein aggregates, virions, subcellular organelles). In addition, high-speed centrifugation may cause damage to EVs, thus reducing their biological activity (32). Density gradient centrifugation is an improved separation strategy based on centrifugation that uses separation media with different densities, such as iodixanol (33). This process results in a higher purity of EV separation; however, it adds a longer centrifugation time (≥16 h), which limits its clinical application (34).
Ultrafiltration is a separation method based on molecular size, removing impurities through the membrane’s different pore sizes or molecular weight cutoffs (MWCO). For the driving force, electric charge, centrifugation, and pressure can be used. One of the greatest advantages of ultrafiltration is that it can study specific subpopulations of EVs by changing the pore size of the filter to obtain different size scales of EVs. The overall procedure is simple compared to other methods, but if the sample has too many impurities, the clogging of the filtering membrane may cause a low recovery rate.
The immunoaffinity capture method selects EVs through antigen–antibody-specific reactions. As mentioned above, certain proteins and receptors are conserved on the membrane of EVs. These proteins can bind to corresponding antibodies to specifically isolate EVs. Antibodies were fixed on different carriers, such as microfluidic chips and magnetic beads, to capture EVs in fluids. It was reported to have higher separation purity. Immunoaffinity methods suit the needs of researchers who require high purity and specific subpopulations of EVs, while the overall yield is lower.
SEC relies on based on the differential elution profiles of particles of different sizes running through a porous polymer, constituting the stationary phase—also known as gel filtration matrix or resin—and carried through the mobile phase of the SEC column. Compared with other isolation methods, SEC is easier to use in a normal lab setting, and without the process of using high speed centrifugation, it preserved better integrity and bioactivity of EVs. However, the process of SEC is relatively time-consuming compared with fast isolation methods like ultrafiltration, thus limiting the yield and usage (35).
Although the abovementioned traditional methods are most widely used in research, many new separation methods are being developed to address with disadvantages, such as large sample consumption, potential damage to EV membrane integrity, and low purity. Among these new technologies, microfluidics offers a promising platform and demonstrates the virtue of high purity and low sample volumes. Its potential has been demonstrated in some research providing novel diagnostic methods (36).
Current obstacles in EV isolation remain unsolved, requiring a standardized procedure for EV production and overcoming the current high-cost and low-yield challenges. To optimize the yield and purity, multiple methods were combined. There is no single optimal separation method, and the exact method is chosen by downstream application and scientific questions.
According to the minimal information for studies of extracellular vesicles, guidelines require each EV preparation to be tested for morphology, particle size, and marker proteins. To visualize the morphology of EV particles, transmission electron microscopy or scanning electron microscopy is commonly used for analyses. For protein markers, at least three positive protein markers included tetraspanins, ESCRT-related proteins, and at least one negative protein marker (37), are required. However, specific biomarkers of different EV subtypes are not yet clear.
3 Extracellular vesicles as biomarkers
Effective monitoring of the health of animals, one of the crucial duties of veterinarians, promotes both welfare and agricultural production efficiency. However, achieving it is difficult due to the complexity of the disease process and limited detection sensitivity. As important mediators of physiological and pathological intracellular communication, EVs carry crucial pathological cargoes from dysfunctional cells and organs and directly reflect the situation of the body. Since EVs can be found in nearly all body fluids (e.g., blood, urine, milk, amniotic fluid), they also provides a noninvasive method of sampling, which suits the need for veterinary diagnoses. Combined with advanced sorting and selection methods, microfluidic chips could provide a promising tool for easy and fast detection (36). Thus, in this section, we will discuss studies identifying EVs as biomarkers for the early detection of diseases that may interest veterinary medicine scientists. Existing studies on biomarker screening for animals are summarized in Table 1.
3.1 Reproductive status
Monitoring reproductive status is one of the significant responsibilities of veterinarians. EVs play an important role in both physiological and pathological processes as biomarkers of fertility, embryo quality, placental quality, and early abortion (37).
Fertility is determined by the complex interplay of health and genetic background. According to previous studies, EVs mediate important fetal-maternal crosstalk during gestation and significantly impact maternal-embryo interactions within the reproductive microenvironment. Multiple studies have demonstrated the bidirectional transfer of EVs across the placental barrier between the fetus and maternal circulation (64). In human health, diverse sources of EVs from various maternal sources, including serum, follicular fluid, and plasma, have been isolated and are believed to have the potential to be indicators of female fertility, while miRNAs are the most representative molecules (37). Similarly, in veterinary medicine, particularly in the dairy industry, where reproduction is economically vital, EV-packaged miRNAs have emerged as promising fertility biomarkers (65). A study on cattle showed that specific blood-borne extracellular vesicles carrying miRNAs could be excellent biomarkers of different pregnancy statuses by comparing the different periods of the pregnancy cycle and different embryonic mortality statuses, but the exact miRNA that could serve the role of the biomarker of embryo death and early pregnancy establishment needs further investigation with an expanded sample size (66). Additionally, exosome miRNA profiling of dairy cows was assessed at different periods of pregnancy, including early pregnancy (~60 days) (39). Specific miRNAs for each pregnancy stage were identified, which may be useful for future biomarker inspection. As proposed by Turner et al., specialized analysis of EV contents may provide key insights to improve reproductive outcomes in dairy cows (40). Beyond maternal fluids, EVs have also been isolated from cargoes, implicating dynamic EV-mediated modulation of the female reproductive tract in anticipation of a possible embryo presence (67). Furthermore, in vitro studies indicate that bovine embryos themselves secrete EVs during blastulation which may enable noninvasive assessment of embryo viability and detection of early embryonic mortality (42). On the genetic side, heifers bred to have different fertility levels develop extreme diversity in fertility breeding values; however, key animal traits (e.g., body weight, milk production) remain similar. A study reported that through proteome profiling of EVs from heifers with divergent genetic merits, a unique protein expression pattern was observed, suggesting that EV-based prognosis tools could be used to improve cattle fertility (43, 44).
In addition to genetic and health factors, various diseases are also determinants of fertility, contributing to poor reproductive efficiency. For example, a study collected exosomes from the plasma of uterine-diseased cows and tested their capacity to decrease PGF2α production in endometrial epithelial cells, suggesting that the cargo of exosomes could provide a useful basis for the early diagnosis of uterine infection (68). Exosome miRNA-218 was detected in endometritis cows’ uterine fluids and was identified as a potential biomarker for the detection of endometritis (45). In another study, circulating exosomes in dairy cows with or without uterine infection were screened for potential protein biomarkers using high-performance liquid chromatography–tandem mass spectrometry, and71 bovine proteins were found to be unique in infected cows (38). Additionally, a study in equines suggested that the size of EVs from uterine lavage and serum could be a promising biomarker for endometritis in mares (41).
In addition to their emerging roles in female fertility, EVs participate extensively in male reproductive physiology. For breeding animals, the quality of semen is one of the crucial factors for male fertility and economic value. Seminal plasma surrounding sperm is an excellent source for detecting reliable noninvasive biomarkers of sperm quality. EVs isolated from the seminal plasma of chickens exhibited distinct characteristics correlating with male fertility status. Specifically, EVs from fertile roosters were smaller in average size, expressed higher levels of HSP90A, and more readily fused with viable sperm compared to EVs from subfertile males. This implicates seminal EVs as potential indicators of avian male fertility (50). Likewise, miRNA profiling of porcine seminal plasma EVs revealed 15 differentially expressed miRNAs between fertile and subfertile boars. Of these miRNAs, miR-205, miR-493-5p, and miR-378b-3p target genes associated with cellular localization and molecular functions that may impair sperm quality when dysregulated (46). Furthermore, miR-21-5p has been proven to prevent sperm capacitation via vinculin inhibition (47), suggesting that the detection of this miRNA is a negative factor in semen quality control. Beyond the assessment of fresh semen, cryotolerance is also crucial, as cryopreservation enables long-term storage and widespread use of superior genetics via artificial insemination. Long-term semen preservation in mammals remains challenging. Current sperm freezing and thawing methods, even the most effective ones, are suboptimal. Imposing these procedures induces structural, biochemical, and functional changes in sperm. This impairs post-thaw sperm functions such as fertilization capacity (48). In swine, three specific miRNAs encapsulated in seminal plasma EVs, including miR-503, miR-103a, and miR-9, were associated with reduced semen cryotolerance. Detection of these miRNAs may predict cryopreservation outcomes and inform the selective use of higher-quality frozen semen (69).
3.2 Metabolic disease
Monitoring for metabolic dysbiosis in livestock herds and controlling the herd-based nutrient balance level is crucial. Most diseases related to metabolism start without clinical signs and are difficult to detect at an early stage. Timely intervention through diet or medication could lead to a better prognosis. The lack of sensitive, noninvasive, and fast-detecting methods is the key challenge for veterinarians, making the development of novel biomarkers important. Metabolic homeostasis emerges from complex, multiorgan crosstalk between adipose tissue, liver, and skeletal muscle. Accumulating evidence supports the role of EVs in metabolic disturbance (49).
Regarding human health, an area of intense research focus involves the emerging metabolic syndromes linked to obesity, nonalcoholic fatty liver disease, diabetes mellitus, and cardiovascular complications. Some studies suggest that the total quantification of EVs in blood may serve as an indicator of metabolic disease progression (70). Additionally, EV cargo such as miRNAs has been explored. One report found that exosomal miR-20b-5b was elevated in patients with type 2 diabetes compared to controls (71). Likewise, another study reported that miR-483-5p encapsulated in serum EVs was associated with obesity and insulin resistance and independently predicted the incidence of type 2 diabetes and cardiovascular events (72). In addition to blood, urinary EVs represent another rich source of biomarkers reflective of metabolic status. For example, Liu et al. identified differential expression of oxidative metabolism-related proteins such as MDH2 in urinary EVs, demonstrating their potential monitoring value (73). Regarding other EV contents, EVs from brown adipose tissue containing 60% proteins were elusive compared to white adipose tissue, including UCP1, Glut1, MIF, and ceruloplasmin, which were suggested to be potential biomarkers of thermogenesis activity (74).
In vitro, detected in both human and swine MSCs with metabolic syndrome, suggesting a shared disorder process (75). Circulating exosomes in transition dairy cows were assessed in one study, and specific proteins, including α-2 macroglobulin, fibrinogen, and oncoprotein-induced transcript 3, were identified in exosomes from the serum of high-risk cows that are prone to metabolic syndrome and liver disease (76). In another report on transitional cows, EVs from cows with high-risk metabolic diseases were identified as endoplasmic and catalase proteins, which were absent in EVs from low-risk animals. They were also tested on Madin-Darby bovine kidney cells and found to have a proinflammatory effect (52). In another study, Quan et al. (77) found that the expression of bovine serum EV miRNAs changes according to different diets. Furthermore, exosomes from high and low transitional disease bulls’ serum were identified, and unique proteins, including serpin A3-7, coiled-coil domain-containing 88A, and inhibin/activin β A chain, were detected only in high-risk cows, which indicates good biomarkers for cow health and fertility (53).
While preliminary, these findings suggest the potential for identifying reproducible EV-based biomarkers that distinguish healthy and metabolically compromised animals.
3.3 Infectious disease
Current diagnosis methods for infectious diseases rely on detecting evidence of both the pathogen and the host response. On the patient side, pathological changes and the immune reaction toward specific pathogens are tested by serological tests and radiological imaging. On the pathogen side, microbiological culture is the gold standard but can be time-consuming. Additionally, pathogens that cannot be cultured easily escape detection (54). Other methods, including microscopy, require trained personnel with proper sampling methods, and molecular diagnostics, including PCR and immunoassays, which are highly sensitive and specific but require expensive equipment and reagents. Given these limitations, EVs play a crucial role in communication between the host and pathogen, making them great fine biomarkers of infectious diseases.
During bacterial infection, both pathogen and host cells release EVs containing indicators of their status. Bacteria-derived EVs contain bacterial antigens and toxins that can be detected as indicators of infection. Meanwhile, host cell EVs carry an immune response against the pathogen. For example, tuberculosis is a contagious, often fatal, and zoonotic disease caused by Mycobacterium tuberculosis (Mtb). The traditional diagnostic methods include sputum culture and molecular diagnostics, such as PCR, for specific genes. These methods are limited due to their high costs and moderate sensitivity (approximately 50%), while microscopic examination is rapid but has low sensitivity (78). Given these shortcomings of existing methods, developing easily detectable biomarkers of active tuberculosis is a global health priority. In vitro and in vivo studies have shown that Mtb-infected cells release EVs with mycobacterial proteins into cell cultures and body fluids (79). Proteomic analyses have identified candidate biomarkers such as the Cfp2 peptide in patient EVs (80, 81). These findings indicate the potential of mycobacterial proteins carried by EVs isolated from infected cells and body fluids as disease biomarkers, although it has emerged obscurely thus far and should be investigated further (79). Interestingly, one of the candidate molecules, Lpqh, a 19 kDa lipoprotein found in the cell wall of Mtb, relays immunological information to other cells, thereby regulating the host immune response in the pathogen’s favor. This lipoprotein is known to be transported via exosomes secreted from M. tuberculosis-infected macrophages. Researchers have reported its application as an efficacious plasma biomarker to distinguish between paratuberculosis and tuberculosis infection in cows. Such EV-based diagnostics could aid tuberculosis clearance in vaccinated dairy herds (82). Another study compared EVs from the milk of healthy and subclinical mastitis cows and discovered that miR-223-3p was upregulated in diseased individuals (55). However, host and bacterial EVs are difficult to differentiate with current processing methods. It is hard to tell whether some authors hypothesize that bacterial molecules identified in EVs during in vivo infection may be the result of a mixed population of host and bacterial EVs.
Unlike bacteria, viruses lack cell membranes and cannot directly release EVs. However, viral infections can still modulate host immunity and EV production. In veterinary medicine, there are several reports on EVs from virus-infected animals. For example, EVs from persistent equine arteritis virus (EAV)-infected stallion semen demonstrated downregulation of miR-128, while the expression of CXCL16, a putative target of miR-128, was significantly enhanced (57). However, this pattern is observed in various inflammatory responses, thus making it less credible as a biomarker. In human medicine, it was reported that examination of plasma EVs from HIV-1 patients showed an increased abundance of exosomes and exosome-associated proteins, such as TSG101, in infected patients, compared to uninfected subjects, and this result was also correlated with the CD4/CD8 ratio. The presence of acetylcholinesterase in EVs was further found to correlate with the length of infection, independent of treatment status, disease progression, and patient age, which suggests the use of EVs as biomarkers for HIV infection and progression (56). Overall, research on EVs as viral disease biomarkers is still preliminary, and more work is needed to determine specificity.
Several parasites have been shown to interact with their hosts through intra- and inter-community communication mechanisms, which were identified to be mediated by EVs through various uptake mechanisms (83). For malaria, for example, EVs were identified in infected individuals and were enriched with typical EV markers as well as parasite proteins (73). Two of these proteins, carbonic anhydrase I and S100A8, were verified to be associated with cerebral malaria in both murine and clinical samples, highlighting the importance of EV protein content as a source of protein markers (84). In veterinary medicine, sporadic studies have been conducted to identify biomarkers for parasite disease. A study used proteomic analysis and miRNA sequencing to identify the difference between diseased dogs with Canine leishmaniosis and healthy dogs, stating that several proteins, such as myo-inositol and carboxylesterase 5 A, might be proteins of interest (58, 85). However, most studies only provide description results instead of testified biomarkers.
3.4 Oncology
Cancer initiation and progression involve communication between emerging malignant cells and other cell types, often mediated by EVs (59). This makes EVs promising minimally invasive biomarkers for early cancer detection, such as liquid biopsies versus invasive tissue sampling. Although each cancer has a unique progression, all require early diagnosis for optimal patient outcomes. EVs circulating in blood or other biofluids represent an alternative to traditional biopsies for profiling tumors. For example, the protein Del-1 in circulating EVs from breast cancer patient plasma shows potential as a biomarker (86). Additionally, urine EVs have been reported in multiple studies as a source of biomarkers for urological cancers, whether to identify cancer grade (87) or the benign and metabolic state of tumors (88), while their utility for the detection of other cancers is less known. Stool EVs containing human and bacterial RNA could also indicate GI microbiome and tract cancers (89).
In veterinary medicine, oncology studies have mainly focused on companion animals. Early studies isolated and characterized EVs from canine, feline, and human mammary tumor cell lines and analyzed their morphology, protein markers, and size distribution (90). In vivo, serum EV levels were significantly higher in canine mast cell tumor patients than in healthy controls (91). Different types of cancer were involved in the research. MiR-15b and miR-343-30 in canine serum-derived EVs could be potential noninvasive biomarkers for canine glioblastoma (62). Additional work profiled miRNA and proteins in EVs from four canine lymphoid tumor lines, finding differential expression of CD82 and certain miRNAs between vincristine-sensitive and resistant cells (92). EVs isolated from canine diffuse B-cell lymphoma patients and dogs also showed increased EV yield compared to healthy animals, with decreases only in dogs with lymphoma (60). Furthermore, analysis of EVs from canine multicentric lymphoma patients receiving CHOP chemotherapy revealed a potential miRNA signature, such as miR-205, correlating with complete/partial response versus no response (93). Additionally, Amelie et al. investigated the correlation between serum EVs and doxorubicin-induced cardiotoxicity, pointing out that miR-502 was upregulated significantly before the third chemotherapeutic dose as a prescient biomarker (61).
4 Extracellular vesicles as vaccines
Developing effective measures to halt and prevent the spread of infectious diseases impacting animal husbandry is critical for achieving high standards of quality and welfare in animal production. Vaccine injection is one of the commonly used measures in veterinary practice. While some traditional vaccine approaches utilize killed or attenuated agents, these approaches may have limitations of safety, immunogenicity, and suitability for disease-free populations at risk. EVs present new opportunities for vaccine development given their bioactivity and safety profile. Specifically, EVs derived from pathogens or host cells have potential applications as either direct vaccine candidates or delivery vehicles. Recent work has highlighted several potential benefits of using exosomes as vaccines. First, exosomes can provide stable conformational conditions to maintain the native structure of antigen proteins. Second, their ability to circulate and reach distal organs improves molecular biodistribution compared to conventional vaccines. Third, the expression of adhesion molecules on exosomes enables more efficient antigen presentation to immune cells. Finally, as a natural mechanism for transporting antigens between cells, exosomes may enhance cross-priming of immune responses (63). Exploring the roles of EVs in next-generation animal vaccines could provide improved protection against pressing infectious diseases in livestock, poultry, aquaculture, and other production contexts. Research is needed to evaluate EV vaccine efficacy, scalability, and feasibility compared to existing options. In this section, we will discuss the potential applications of EVs in vaccine development.
4.1 Viral disease
Extracellular vesicles act as a double-edged sword because of their ability to carry and deliver molecules to target cells during infection. EVs play a crucial role in the pathogenesis of infection but trigger immune responses to confer protection against pathogens (63). Compared to traditional vaccines produced in cell culture or eggs, EV-based vaccines have many advantages. For example, endogenous viruses in avian-derived cell lines interfere with the structure of the introduced exogenous virus, causing an allergic reaction after vaccination, or glycosylation directly affects the immunogenicity of recombinant protein in eggs, whereas EV-based vaccines can provide a more stable conformational condition for the proteins and provide a more efficient presentation to antigen-presenting cells (63).
Influenza viruses significantly impact both human health and animal husbandry, while existing vaccine options have limitations. Recent research by Anticoli et al. explored an exosome-based influenza vaccine approach in mice. They transfected muscle cells to express influenza nucleoprotein fused to a mutated Nef protein. Purified exosomes from these cells were administered to mice, eliciting robust cytotoxic CD8+ T-cell responses against the nucleoprotein. The cytotoxicity induced appeared sufficient to eliminate both peptide-pulsed and nucleoprotein-expressing target cells. These promising results suggest that this exosome vaccine strategy can stimulate viral antigen-specific immunity (94). Compared to conventional influenza vaccines, the exosome platform provides targeted antigen delivery and potentially improved immunogenicity.
In veterinary studies, preliminary trials have demonstrated that EVs can stimulate cellular and antibody responses for several important animal pathogens. The first animal virus tested was lymphocytic choriomeningitis virus (LCMV). In this study, exosomes from dendritic cells infected with LCMV or loaded with an LCMV peptide did not induce significant CD8 T-cell activation or antiviral protection, suggesting that exosomes have a limited contribution to CTL priming during acute LCMV infection (95). However, studies on porcine reproductive and respiratory syndrome virus (PRRSV) showed some positive results. EVs containing microRNAs designed to target the PRRSV receptors sialoadhesin and CD163 were able to suppress receptor expression and reduce PRRSV viral titers when delivered to Sus scrofa cells (96). This demonstrates the potential for exosomes to effectively deliver antiviral microRNA therapeutics and mediate long-lasting protection against different viral strains (96, 97). Additionally, in another study, immunizing pigs with extracellular vesicle-enriched fractions from the serum of PRRSV-recovered animals elicited virus-specific IgG and IFN-γ responses without adverse effects or detectable viral replication, demonstrating the potential of using serum EVs as a safe, immunogenic vaccine strategy against PRRSV. The EV vaccine enabled differentiation between infected and vaccinated animals and showed enhanced immunogenicity when boosted with PRRSV peptides (98). Ongoing studies are exploring EV vaccines for PRRSV, avian influenza, foot and mouth disease, and other major pathogens.
4.2 Infectious non-viral disease
As mentioned above, bacteria can secrete bEVs as OMVs and MVs. The bEVs inherit several pathogen-associated molecular patterns (PAMPs), including antigens, from their donor bacteria and, consequently, can induce an immune response against pathogens. This inherent adjuvant-like property of bEVs makes them well-suited for development as vaccines against bacterial infections (99).
BEVs have the potential to be used to develop vaccines for parent bacteria after genetic detoxification (8). Most studies have focused on OMVs from gram-negative bacteria, and some OMV vaccine candidates have been developed at the preclinical stage, including Vibrio cholerae, Klebsiella pneumoniae, and Bordetella pertussis (100–102). For example, a study used uniformed K. pneumoniae OMVs to vaccinate mice subcutaneously and showed significant protection after infection with a lethal dose of carbapenem-resistant K. pneumoniae. Likewise, for gram-positive bacteria, a study suggested that extracellular vesicles from gene-edited S. aureus could become a vaccine platform and showed benign protection against different strains of S. aureus-established murine sepsis (23). For clinical use, During the outbreak of group B strain-specific meningococcal cases in New Zealand, a national vaccination program was performed using an OMV-based vaccine developed by Chiron Vaccines (Siena, Italy), which was shown to have an estimated 80% vaccine effectiveness for children aged 6 months to <5 years (103).
In addition, bEVs are a good source of bacterial antigens. PAMPs enable interactions with antigen-presenting cells (APCs) and allow bEVs to be phagocytosed by APCs. These characteristics make bEVs an adjuvant to traditional vaccines to boost immunity. One study tested OMVs from the ∆purM Burkholderia pseudomallei strain admixed with heterologous peptides and demonstrated that, compared to the adjuvants alum and CpG DNA, OMV adjuvants induced more antibody and B-cell responses (104). When co-administered with an influenza vaccine, OMVs with attenuated endotoxicity showed an improved antigen-specific T-cell response and efficacy in mice (105).
EVs are also produced by parasites as a conserved communication strategy and are often referred to as exosome-like vesicles. There is accumulating evidence that EVs are released in parasitic diseases, mediating both parasite–parasite intercommunication and parasite–host interactions (106). Based on this, it is plausible that parasite-derived EVs may serve as vaccine candidates against parasitic diseases. For instance, schistosomiasis caused by Schistosoma mansoni results in over 280,000 deaths annually worldwide but lacks a preventive vaccine (107). EVs released by S. mansoni adult worms contain miRNAs and proteins involved in host–parasite interactions (108), including several potential vaccine antigens spanning multiple life cycle stages (109). Investigations are also underway on EV-based vaccines utilizing antigen-presenting cells for parasitic diseases. Toxoplasmosis, a globally prevalent zoonosis caused by Toxoplasma gondii, relies primarily on live, attenuated tachyzoites for veterinary immunization (110). A recent study reported that EVs derived from dendritic cells stimulated with T. gondii lysate elicited protective mucosal and hormonal immune responses against Toxoplasma infection in an animal model (111). Similarly, a study on Eimeria tenella, the parasite of avian coccidiosis, using exosomes from antigen-loaded dendritic cells showed a protective effect on chickens (112), suggesting the potential of EVs as a protective vaccine production.
5 EVs as therapeutic agents
The application of harnessing EVs in the treatment of diseases in multiple fields, including cancer, cardiovascular disorders, and regenerative medicine, is now being recognized (3). However, the studies in veterinary medicine on therapeutic effects are still preliminary, and we summarized existing studies in Table 2. The overall research on EVs in therapy could be divided into research on native and engineered EVs, which will be discussed below (Figure 2).
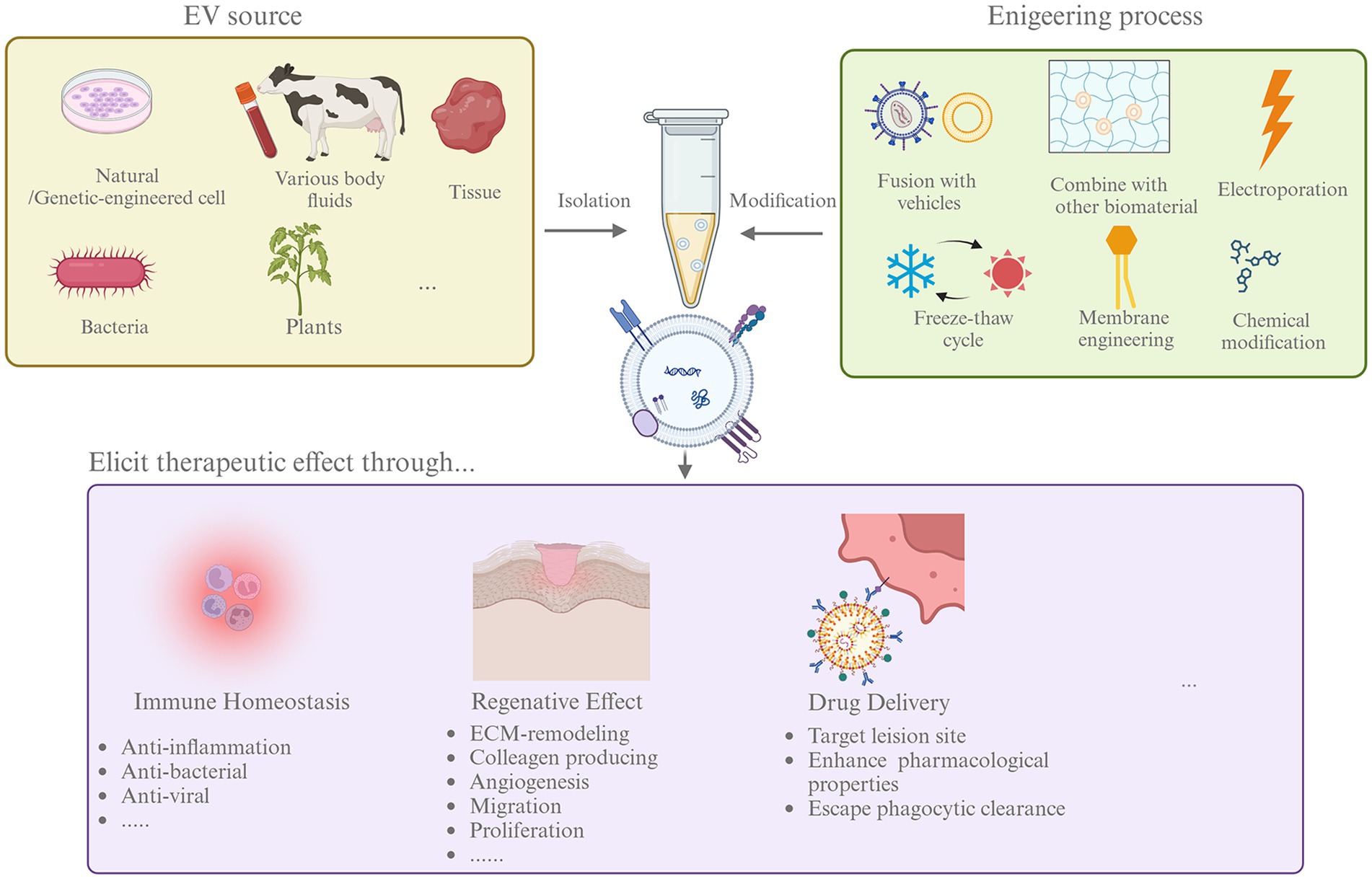
Figure 2. The process of EVs as treatment agents. Therapeutic EVs can be isolated from multiple sources including cultured cells, body fluids and tissue, bacteria, and plants. To enhance the therapeutic effects, EVs can go through different modification by engineering, like fusion with liposome and electroporation. Then, native, or engineered EVs was used in therapies. EVs could affect disease through modifying immune homeostasis, promoting tissue regeneration, and deliver therapeutic agents effectively.
5.1 Native EVs
Native EVs from multiple sources have shown therapeutic effects. Most veterinary medicine research has focused on using native EVs for treatment (119). We will discuss the different sources of native EVs in the following section (Table 2).
5.1.1 Stem cell-derived EVs
Stem cells are attractive to human and veterinary medicine for their propriety of regenerating functional tissue. However, in stem cell therapy, immunogenicity, heterogeneity, and tumorigenicity poses three obstacles, and EVs derived from in vivo culturing stem cell could be one of the solutions. In particular, mesenchymal stem cell-derived exosomes (MSC-exosomes) not only have immunomodulatory and tissue regenerative capacity but also exhibit immune privilege that is appropriate for therapeutic approaches (120). In addition, MSC-exosomes can avoid pulmonary embolisms associated with MSC-based cell therapy. Additionally, MSC-EVs cannot proliferate like cells, which will lead to fewer worries about cancer development. It has been shown that MSC-EVs have a similar therapeutic effect to MSCs themselves. For example, MSC-EVs promote M2 macrophages via PGE2 activation in a manner similar to MSCs (121). In a study using dogs as a cutaneous injury model, MSC-MVs had superior capability in wound healing than their originator cells (113). Another strong therapeutic candidate is adipose tissue-derived mesenchymal stem/stromal cell-derived EVs, which have been tested in a murine model of DSS-induced colitis in a murine model (114) and canine renal ischemia–reperfusion injury (118). An in vivo study tested the anti-inflammatory effect of equine MSC-EVs on chondrocytes, suggesting their therapeutic potential (117). To enhance the therapeutic effect, EVs from preconditioned cells were also investigated. A case report on treating a suspensory ligament injury in a horse using MVs derived from mesenchymal stem cells pretreated with 5-azacytidine and resveratrol. The anti-apoptotic and proliferation-promoting effects of MVs were tested in vivo and in vitro (97).
5.1.2 Blood-derived EVs
Circulating EVs from neonatal individuals have also demonstrated therapeutic functions. In 2019, Yoshida et al. reported that EVs from young mice could delay aging and extend the lifespan in aged mice (122). Thus, the effects of circulating EVs on disease are of research interest. For example, one study found that intramyocardial delivery of plasma exosomes isolated from neonatal mice helped repair adult heart’ structure and function after acute myocardial infarction by promoting angiogenesis (123).
Platelet-rich plasma (PRP) has been used in regenerative medicine for more than 30 years, and numerous encouraging outcomes have been obtained. PRP has shown its capability to repair cardiac disease, skin wounds, musculoskeletal disease, and hair growth (124, 125). However, some limitations still need to be considered. For example, PRP-derived biomolecules may be damaged by lytic enzymes from the extracellular environment once released from platelets. PRP-EVs are expected to be more efficient and safer clinical candidates in therapies, especially in regenerative medicine. Most studies on PRP-EVs are on the hemostasis and angiogenesis capabilities of regenerative medicine, while the effect of PRP-EVs on inflammation is biased (126). In veterinary medicine, a pioneering study reported that commercially purified PRP exosomes (human-sourced) can increase cell proliferation and deposition and reduce cellular apoptosis caused by dexamethasone in vitro in canine tenocytes (116).
5.1.3 Immune cell-derived EVs
Inflammation is the body’s defense mechanism and helps clear infections; when it becomes out of control, it can lead to harmful outcomes, such as cytokine storms causing organ damage. Immune cells such as lymphocytes, macrophages, and mast cells are involved in the immune response. Crosstalk between these cells is crucial in immune homeostasis, and the EVs from those cells are part of this function. Utilizing EVs from immune cells for therapeutic purposes for the disease may be promising.
While current anti-inflammatory drugs target a single pathway, immune cell-derived EVs provide multitarget therapeutics to modulate immune status. Wang et al. reported that p extracellular vesicles derived from peritoneal M2 macrophages (M2-EVs) exhibited superior anti-inflammatory potential by effectively reducing excessive cytokine release, thereby attenuating oxidative stress and multiple organ damage in endotoxin-induced cytokine storms (127).
In cancer treatment, the crosstalk between the tumor microenvironment and immune cells is crucial. For macrophages, M2-polarized pro-tumoral macrophages (TAMs) express the interleukin-4 receptor (IL4R) at higher levels thanM1-polarized, anti-tumoral macrophages. Thus, using M1-polarized macrophage-derived EVs could be a strategy to modify tumor microenvironments. One single study of the proapoptotic effect of extracellular vesicles derived from canine M1-polarized macrophages on canine melanoma and osteosarcoma cell lines suggested that it could be an effective anticancer therapeutic approach (115). In addition, the polarization of macrophages is reversible, and transforming TAMs to a tumor-suppressive M1 phenotype is an important approach for tumor therapy (128). An M1-exosome was transfected with NF-κB p50 siRNA and miR-511-3p to enhance M1 polarization and surface-modified with IL4RPep-1, an IL4R-binding peptide, to target the IL4 receptor of TAMs (named IL4R-Exo (si/mi)). IL-4R-Exos suppress the growth of tumors by reprogramming TAMs into M1-like macrophages and increasing antitumor immunity, indicating a novel cancer immunotherapy (129).
Dendritic cells (DCs), as crucial antigen-presenting cells, play a vital role in disease pathogenesis. DC-derived EVs were found to harbor functional peptide–MHC complexes, T-cell costimulatory molecules, and other molecules essential for antigen presentation, immune cell regulation, and simulation of immune responses (130). For immature DCs, EVs are an attractive candidate for immunosuppressant therapies for autoimmune and inflammatory disorders due to their immune suppressive capabilities. It has been reported in different disease models in mouse collagen-induced arthritis models (131), colitis (132), and IBD (133). For mature DCs, in their nature as antigen-presenting cells, In viral infection, DC-derived EVs carry viral antigens and particles with detrimental effects on the immune system, transmitting pathogen particles and aiding viruses in escaping the immune system. However, evidence for their protective effect is lacking. However, DC-derived EVs may help lead to a protective immune response against bacterial and parasitic infection, and the effect seems to be pathogen-specific. For example, DCs infected with Chlamydia psittaci display enhanced exosome release. The exosomes facilitated the activation of NK cells and upregulated IFN-γ secretion, reducing C. psittaci growth and increasing apoptosis in infected cells, thus clearing further infection (134).
5.1.4 Tissue-derived EVs
EVs from in vitro cultured cells, such as MSCs, showed certain tissue repair potency. However, the efficacy of current EV therapies is unsatisfactory for multiple reasons, such as the importance of large-scale production and the time consumption involved. Moreover, long-term in vitro culturing of cells may affect the phenotypes of the donor cell, thus impairing EV biofunction. Thus, tissue-sourced EVs are a better alternative for treatment (135). Lou et al. reported that neonatal tissue-derived EV therapy is a potent strategy for precision tissue repair in multiple injury models, including skin wounds, acute kidney injuries, and partial nephrectomy (5).
5.1.5 Vesicle-like nanoparticles and bacterial EVs
Vesicle-like nanoparticles derived from edible plants, such as daily vegetables, have demonstrated crosstalk with mammalian cells. Moreover, the oral administration of plant-derived vesicles has been proven safe. Multiple studies have shown that plant-derived extracellular vesicle-like nanoparticles (PDVLNs) can exert therapeutic effects on human diseases. For instance, one study found that PDVLNs derived from grapefruit can be taken up by intestinal macrophages, inducing the expression of the antioxidant gene HO-1 while suppressing the production of proinflammatory cytokines. This ameliorated dextran sulfate sodium-induced colitis in a mouse model (136). While no veterinary research on PDVLNs has been conducted yet, traditional herbal medicines may offer another rich source of bioactive PDVLNs. The clinical benefits of traditional Chinese medicines are often attributed to their multicomponent, multitarget mechanisms of action. Thus, studying PDVLNs may elucidate the therapeutic mechanisms of these traditional remedies (137). For example, one study showed that ginseng-derived nanoparticles can alter macrophage polarization to inhibit melanoma growth (138).
5.2 Engineered EVs
The therapeutic potential of various native EVs has been well established. To further optimize their performance and harness their natural abilities, researchers have worked to equip EVs with new targeting and therapeutic moieties while preserving vesicle integrity. For instance, some studies have functionalized EVs with targeting ligands to enhance their specificity for desired cell types or tissues. Other efforts have focused on loading EVs with therapeutic nucleic acids, proteins, or drugs to augment their treatment capabilities.
5.2.1 Enhancing targeting ability
While EVs demonstrate an inherent ability to preferentially interact with target cells in vitro, this targeting efficiency is often diminished in vivo. For example, Smyth et al. reported that unmodified tumor-derived exosomes exhibit no improvement over liposomes in tumor accumulation or circulation time (139). Moreover, multiple studies note a predominant accumulation of systemically administered EVs in the liver and spleen (140). To enhance EV targeting, researchers have explored modifying vesicles with specific targeting ligands. Strategies to engineer the EV surface fall into two categories: pre-isolation techniques that manipulate the donor cell, including genetic, metabolic, and membrane engineering approaches, and post-isolation techniques that directly modify purified EVs using physical or chemical methods (141).
One of the advantages of EVs is that the editing of parent cells could directly affect EV production and properties. Genetic engineering strategies for surface functionalization of EVs are based on transgene expression of proteins or chimeric proteins containing protein domains that are known to be enriched in EVs. For instance, Mentkowski et al. engineered cardio-sphere-derived cells to express a chimeric protein consisting of Lamp2b fused to a cardiomyocyte-specific peptide (142). The resulting EVs displayed enhanced targeting to cardiomyocytes and improved therapeutic effects in a heart injury model (142). Beyond modifying EV proteins, the glycocalyx is another exploitable target for engineering. Zheng et al. developed a method to co-express glycosylation enzymes and glycoprotein domains in parent cells (143). Specifically, they inserted a glycosylation domain into the extracellular loop of CD63 along with the enzymes FUT7 or FUT9. This approach enabled the display of the glycans sialyl Lewis X or Lewis X on engineered EVs, conferring high specificity for activated endothelial cells or dendritic cells, respectively (143).
Surface engineering of EVs is also possible after isolation, although such post-isolation techniques require additional steps and are more time-consuming than genetic engineering approaches. Post-isolation engineering strategies fall into two main categories: physical interaction methods and chemical modification methods. For physical modification, lipophilic components such as liposomes are often used to fuse with the EV membrane, employing techniques such as freeze–thaw cycling, electroporation, or altering culture pH and salt concentrations. A novel technique-engineered surface of EVs by adsorption. For the chemical approach, EVs were modified through the covalent linkage of molecules to their surface (141). While physical and chemical engineering offer certain advantages, genetic modification of parent cells remains the predominant strategy for EV engineering given its superior controllability and the stability of modifications.
5.2.2 Delivery of therapeutic reagents
Currently, the two mainstream drug delivery systems are liposomes and polymeric nanoparticles (144). Liposomes comprise phospholipid vesicles that can encapsulate both hydrophilic and hydrophobic molecules. Polymeric nanoparticles are synthesized from biocompatible and degradable materials such as natural collagen or synthetic polyacrylates, which facilitating drug transport and extend the drug’s half-life (145). However, the use of liposomes has been reported for rapid clearance via the reticuloendothelial system, and their fast accumulation in the liver and spleen limits the effective concentration of drugs. On the other hand, although polymeric nanoparticles as a delivery platform may have better stability than liposomes, concerns about their safety for long-term use remains (146). Compared to these existing platforms, EVs demonstrate enhanced stability in biological systems and can distribute over short and long distances, even cross biological barriers (147). EVs are unique in protecting and delivering their internal cargo to target cells through ligand-receptor interactions. Previous studies have shown that proteins on EV surfaces facilitate cargo delivery and increase half-lives in circulation by promoting membrane fusion with the target cell and suppressing CD47-mediated phagocytic clearance, enhancing the pharmacological properties of EVs (148). In addition, EVs can load multiple substances, such as proteins, nucleic acids, and synthetic drugs, making them a versatile delivery platform. Thus, developing EVs as a drug delivery platform holds promise.
Cancer therapy represents one prominent application that requires a highly targeted drug delivery platform. Various chemotherapeutic agents can be loaded into EVs, including plant-derived natural compounds such as triptolide (TPL) and synthetic drugs such as paclitaxel. TNF-related apoptosis-inducing ligand (TRAIL) could selectively induce apoptosis of cancer cells, while the application of recombinant TRAIL protein faced the challenges of low bioavailability and resistance of cancer cells. However, EVs from TRAIL-expressing mesenchymal stem cells overcome these limitations, eliciting dose-dependent apoptosis in multiple cancer cell types in vivo, including pronounced apoptosis in TRAIL-resistant cancer cells (149). Another study utilizing TRAIL-expressing engineered EVs to encapsulate TPL demonstrated potent inhibition of tumor growth and reduced toxicity in a melanoma mouse model in vitro (150). MiRNAs also effectively modulate cancer progression; miR-195 is downregulated in drug-resistant melanomas. Tumor-derived EVs loaded with miR-195 reduced tumor volume in vivo and impaired engraftment and growth of xenografts implanted with melanoma cells exposed to MAPK inhibitors (151). Beyond the delivery of drugs and microRNAs to modulate cancer cells, cancer vaccines inducing tumor regression by triggering specific T-cell responses against tumor neoantigens represent another crucial aspect of cancer treatment. OMVs show promise as cancer vaccine candidates due to the enrichment of pathogen-associated molecular patterns, which can activate antigen-presenting cells to elicit effective antigen presentation and robust activation of the adaptive immune activation. Capitalizing on straightforward bacterial genetic editing and plug-and-display systems, OMVs can display diverse tumor antigens to customize cancer vaccines (152).
In addition to systemic administration, EVs can also be combined with biomaterials to achieve efficient localized administration for lesion sites. Hydrogels and scaffolds can be utilized to enhance the retention and therapeutic effects of EVs (153). For example, Self-assembling peptides (SAPs) are a class of biomaterials composed of natural amino acids that can spontaneously form nanoscale hydrogels under ionic saline conditions, exhibiting high biocompatibility (154). In a murine model of renal ischemia–reperfusion injury, mesenchymal stem cell-derived extracellular vesicles loaded into SAP hydrogel demonstrated greater therapeutic potential compared to a single dose of either EVs or SAP alone. This treatment improved renal function by reducing tubular cell apoptosis, pro-inflammatory cytokine expression, and macrophage infiltration (155).
6 Challenge and limitations of the application of EVs
The versatile applications of EVs in veterinary medicine are accompanied by inherent challenges that merit careful consideration. One major concern revolves around the lack of standardized procedures for EV isolation and preservation, given their inherent heterogeneity. This diversity poses difficulties in differentiating between vesicle subtypes and determining their origin, resulting in varying impurities and bioactive capabilities due to disparate isolation methods (33). The absence of a unified standard in isolation procedures introduces a notable level of inconsistency in research outcomes. This variability is particularly pronounced in diagnostic applications reliant on EV-based biomarkers, where challenges arise from potential similarities with circulating exosome miRNAs (51) and variations in protein and lipid content due to isolation methods (156). Preserving EVs, typically stored in PBS at −80°C, introduces challenges, with storage conditions impacting membrane structure efficiency. Achieving consensus on storage conditions and duration is pivotal for maintaining stable EV effects (157).
In addition, the challenge of using EVs for diagnosis tools can be difficult. Although there are business products available like ExoDx Lung by Exosome Diagnostics company reaching decent sensitivity and specificity, the reproducibility of EVs’ test results is still debatable, and it is difficult to compare test results across platforms horizontally, and the traceability chain of results is lacking (51). Especially for veterinary development, without the capability to recruit patients for large clinical trials, the task may be more challenging.
Further, the yield of functional EVs will also be problematic. A large part of EVs comes from cell culture medium, which limits the production. There are studies and methods to boost EVs production from cells, but still a gap in clinical translation (158). To enhance the therapeutic and targeting effect, the engineering process is needed. Various engineering methods were reported and the efficiency remains an obstacle. In veterinary medicine, where treatment doses are contingent upon animal size, the demand for large quantities further accentuates these challenges.
Lastly, cost control emerges as a significant hurdle in the application of EVs. The expense associated with obtaining EVs exceeds that of chemically synthesized medicines, and conserving bioactivity may necessitate additional transportation methods, posing a further economic consideration for treatment applications. Addressing these challenges necessitates concerted efforts in standardization, enhancing reproducibility, scalable production methods, and the formulation of cost-effective strategies to unlock the full potential of EVs in veterinary medicine.
7 Conclusion
EVs represent an emerging field of research in veterinary medicine, though investigations thus far remain largely preliminary. Current veterinary EV studies have principally focused on characterizing EVs across various physiological and pathological states, identifying potential vesicle-based biomarkers, and evaluating the therapeutic promise of EVs. As living standards and prosperity in the pet industry continue rising, demand for highly customized therapies creates a need for antibiotic-free alternatives and regenerative medicine solutions. Veterinary scientists would benefit from collaborating with human clinical medicine, where EVs have already been harnessed for clinical applications. Structured collaborative efforts between human and veterinary researchers will facilitate the translation of EVs into clinical veterinary practice to address emerging needs.
Overall, existing studies provide valuable foundational insights into the extensive potential of EVs as biomarkers and therapeutics in veterinary applications. However, continued investigations are needed to fully characterize EVs, elucidate mechanisms of action, and translate findings into clinical veterinary practice. Interdisciplinary cooperation and adherence to consistent protocols will be essential to properly evaluating, validating, and implementing EV-based veterinary diagnostics and therapies.
Author contributions
YX: Writing – original draft. PL: Writing – review & editing. CX: Writing – review & editing. BH: Writing – review & editing. JL: Writing – review & editing. JG: Writing – review & editing.
Funding
The author(s) declare financial support was received for the research, authorship, and/or publication of this article. This study was financially supported by the National Natural Science Foundation of China (32273082 and U21A20262) and the Beijing-Tianjin-Hebei Collaborative Innovation Community Project (21346601D).
Acknowledgments
We would like to thank Bio-render for providing illustration materials. Figures in this paper were created with BioRender.com.
Conflict of interest
The authors declare that the research was conducted in the absence of any commercial or financial relationships that could be construed as a potential conflict of interest.
Publisher’s note
All claims expressed in this article are solely those of the authors and do not necessarily represent those of their affiliated organizations, or those of the publisher, the editors and the reviewers. Any product that may be evaluated in this article, or claim that may be made by its manufacturer, is not guaranteed or endorsed by the publisher.
Abbreviations
EVs, Extracellular vesicles; ARDS, Acute respiratory distress syndrome; MVBs, Multivesicular bodies; ILVs, Intraluminal vesicles; ESCRT, Endosomal sorting complex required for transport; bEVs, Bacterial EVs; OMVs, Outer membrane vesicles; MVs, membrane vesicles; PDVLNs, plant-derived vesicle-like nanoparticles; EXPO, exocyst-positive organelle; UC, ultracentrifugation; SEC, size exclusion chromatography; MWCO, molecular weight cutoffs; miRNAs, micro RNAs; LCMV, lymphocytic choriomeningitis virus; PRRSV, porcine reproductive and respiratory syndrome virus; PAMPs, pathogen-associated molecular patterns; APCs, antigen-presenting cells; MSC, Mesenchymal stem cell; PRP, platelet-rich plasma; DCs, Dendritic cell; RES, reticuloendothelial system; TPL, triptolide; TRAIL, TNF-related apoptosis-inducing ligand; SAP, Self-assembling peptides.
References
1. Lou, P, Liu, S, Xu, X, Pan, C, Lu, Y, and Liu, J. Extracellular vesicle-based therapeutics for the regeneration of chronic wounds: current knowledge and future perspectives. Acta Biomater. (2021) 119:42–56. doi: 10.1016/j.actbio.2020.11.001
2. Van Niel, G, D’Angelo, G, and Raposo, G. Shedding light on the cell biology of extracellular vesicles. Nat Rev Mol Cell Biol. (2018) 19:213–28. doi: 10.1038/nrm.2017.125
3. Cheng, L, and Hill, AF. Therapeutically harnessing extracellular vesicles. Nat Rev Drug Discov. (2022) 21:379–99. doi: 10.1038/s41573-022-00410-w
4. Song, J, Song, B, Yuan, L, and Yang, G. Multiplexed strategies toward clinical translation of extracellular vesicles. Theranostics. (2022) 12:6740–61. doi: 10.7150/thno.75899
5. Lou, P, Liu, S, Wang, Y, Lv, K, Zhou, X, Li, L, et al. Neonatal tissue-derived extracellular vesicle therapy (NEXT): a potent strategy for precision regenerative medicine. Adv Mater. (2023) 35:e2300602. doi: 10.1002/adma.202300602
6. Direct Biologics, LLC. Bone marrow mesenchymal stem cell derived extracellular vesicles for hospitalized patients with moderate-to-severe ARDS: A phase III clinical trial. Report No.: NCT05354141. (2023). Available at: https://clinicaltrials.gov/study/NCT05354141 (Accessed Aug 24, 2023).
7. Cully, M. Exosome-based candidates move into the clinic. Nat Rev Drug Discov. (2021) 20:6–7. doi: 10.1038/d41573-020-00220-y
8. Gnopo, YMD, Watkins, HC, Stevenson, TC, DeLisa, MP, and Putnam, D. Designer outer membrane vesicles as immunomodulatory systems – reprogramming bacteria for vaccine delivery. Adv Drug Deliv Rev. (2017) 114:132–42. doi: 10.1016/j.addr.2017.05.003
9. Huemer, M, Mairpady Shambat, S, Brugger, SD, and Zinkernagel, AS. Antibiotic resistance and persistence-implications for human health and treatment perspectives. EMBO Rep. (2020) 21:e51034. doi: 10.15252/embr.202051034
10. Crescitelli, R, Lässer, C, and Lötvall, J. Isolation and characterization of extracellular vesicle subpopulations from tissues. Nat Protoc. (2021) 16:1548–80. doi: 10.1038/s41596-020-00466-1
11. Wang, Y, Zhao, M, Liu, S, Guo, J, Lu, Y, Cheng, J, et al. Macrophage-derived extracellular vesicles: diverse mediators of pathology and therapeutics in multiple diseases. Cell Death Dis. (2020) 11:924. doi: 10.1038/s41419-020-03127-z
12. Han, QF, Li, WJ, Hu, KS, Gao, J, Zhai, WL, Yang, JH, et al. Exosome biogenesis: machinery, regulation, and therapeutic implications in cancer. Mol Cancer. (2022) 21:207. doi: 10.1186/s12943-022-01671-0
13. Juan, T, and Fürthauer, M. Biogenesis and function of ESCRT-dependent extracellular vesicles. Semin Cell Dev Biol. (2018) 74:66–77. doi: 10.1016/j.semcdb.2017.08.022
14. Ashoub, MH, Salavatipour, MS, Kasgari, FH, Valandani, HM, and Khalilabadi, RM. Extracellular microvesicles: biologic properties, biogenesis, and applications in leukemia. Mol Cell Biochem. (2023) 2023:734. doi: 10.1007/s11010-023-04734-y
15. Clancy, JW, Schmidtmann, M, and D’Souza-Schorey, C. The ins and outs of microvesicles. FASEB Bioadv. (2021) 3:399–406. doi: 10.1096/fba.2020-00127
16. Bertheloot, D, Latz, E, and Franklin, BS. Necroptosis, pyroptosis and apoptosis: an intricate game of cell death. Cell Mol Immunol. (2021) 18:1106–21. doi: 10.1038/s41423-020-00630-3
17. Xie, J, Li, Q, Haesebrouck, F, Van Hoecke, L, and Vandenbroucke, RE. The tremendous biomedical potential of bacterial extracellular vesicles. Trends Biotechnol. (2022) 40:1173–94. doi: 10.1016/j.tibtech.2022.03.005
18. Sartorio, MG, Pardue, EJ, Feldman, MF, and Haurat, MF. Bacterial outer membrane vesicles: from discovery to applications. Annu Rev Microbiol. (2021) 75:609–30. doi: 10.1146/annurev-micro-052821-031444
19. Roier, S, Zingl, FG, Cakar, F, Durakovic, S, Kohl, P, Eichmann, TO, et al. A novel mechanism for the biogenesis of outer membrane vesicles in gram-negative bacteria. Nat Commun. (2016) 7:10515. doi: 10.1038/ncomms10515
20. Kulp, A, and Kuehn, MJ. Biological functions and biogenesis of secreted bacterial outer membrane vesicles. Annu Rev Microbiol. (2010) 64:163–84. doi: 10.1146/annurev.micro.091208.073413
21. Lee, EY, Choi, DY, Kim, DK, Kim, JW, Park, JO, Kim, S, et al. Gram-positive bacteria produce membrane vesicles: proteomics-based characterization of Staphylococcus aureus-derived membrane vesicles. Proteomics. (2009) 9:5425–36. doi: 10.1002/pmic.200900338
22. Toyofuku, M, Schild, S, Kaparakis-Liaskos, M, and Eberl, L. Composition and functions of bacterial membrane vesicles. Nat Rev Microbiol. (2023) 21:415–30. doi: 10.1038/s41579-023-00875-5
23. Wang, X, Thompson, CD, Weidenmaier, C, and Lee, JC. Release of Staphylococcus aureus extracellular vesicles and their application as a vaccine platform. Nat Commun. (2018) 9:1379. doi: 10.1038/s41467-018-03847-z
24. Feng, J, Xiu, Q, Huang, Y, Troyer, Z, Li, B, and Zheng, L. Plant-derived vesicle-like nanoparticles as promising biotherapeutic tools: present and future. Adv Mater. (2023) 35:e2207826. doi: 10.1002/adma.202207826
25. Halperin, W, and Jensen, WA. Ultrastructural changes during growth and embryogenesis in carrot cell cultures. J Ultrastruct Res. (1967) 18:428–43. doi: 10.1016/S0022-5320(67)80128-X
26. Hatsugai, N, Iwasaki, S, Tamura, K, Kondo, M, Fuji, K, Ogasawara, K, et al. A novel membrane fusion-mediated plant immunity against bacterial pathogens. Genes Dev. (2009) 23:2496–506. doi: 10.1101/gad.1825209
27. Maas, SLN, Breakefield, XO, and Weaver, AM. Extracellular vesicles: unique intercellular delivery vehicles. Trends Cell Biol. (2017) 27:172–88. doi: 10.1016/j.tcb.2016.11.003
28. O’Brien, K, Breyne, K, Ughetto, S, Laurent, LC, and Breakefield, XO. RNA delivery by extracellular vesicles in mammalian cells and its applications. Nat Rev Mol Cell Biol. (2020) 21:585–606. doi: 10.1038/s41580-020-0251-y
29. Hoshino, A, Costa-Silva, B, Shen, TL, Rodrigues, G, Hashimoto, A, Tesic Mark, M, et al. Tumour exosome integrins determine organotropic metastasis. Nature. (2015) 527:329–35. doi: 10.1038/nature15756
30. Parolini, I, Federici, C, Raggi, C, Lugini, L, Palleschi, S, De Milito, A, et al. Microenvironmental pH is a key factor for exosome traffic in tumor cells. J Biol Chem. (2009) 284:34211–22. doi: 10.1074/jbc.M109.041152
31. Mulcahy, LA, Pink, RC, and Carter, DRF. Routes and mechanisms of extracellular vesicle uptake. J Extracell Vesicles. (2014) 3:3. doi: 10.3402/jev.v3.24641
32. Visan, KS, Lobb, RJ, Ham, S, Lima, LG, Palma, C, Edna, CPZ, et al. Comparative analysis of tangential flow filtration and ultracentrifugation, both combined with subsequent size exclusion chromatography, for the isolation of small extracellular vesicles. J Extracell Vesicles. (2022) 11:e12266. doi: 10.1002/jev2.12266
33. Veerman, RE, Teeuwen, L, Czarnewski, P, Güclüler Akpinar, G, Sandberg, A, Cao, X, et al. Molecular evaluation of five different isolation methods for extracellular vesicles reveals different clinical applicability and subcellular origin. J Extracell Vesicles. (2021) 10:e12128. doi: 10.1002/jev2.12128
34. Cvjetkovic, A, Lötvall, J, and Lässer, C. The influence of rotor type and centrifugation time on the yield and purity of extracellular vesicles. J Extracell Vesicles. (2014) 3:3. doi: 10.3402/jev.v3.23111
35. Monguió-Tortajada, M, Gálvez-Montón, C, Bayes-Genis, A, Roura, S, and Borràs, FE. Extracellular vesicle isolation methods: rising impact of size-exclusion chromatography. Cell Mol Life Sci. (2019) 76:2369–82. doi: 10.1007/s00018-019-03071-y
36. Contreras-Naranjo, JC, Wu, HJ, and Ugaz, VM. Microfluidics for exosome isolation and analysis: enabling liquid biopsy for personalized medicine. Lab Chip. (2017) 17:3558–77. doi: 10.1039/C7LC00592J
37. Capra, E, and Lange-Consiglio, A. The biological function of extracellular vesicles during fertilization, early embryo—maternal crosstalk and their involvement in reproduction: review and overview. Biomol Ther. (2020) 10:1510. doi: 10.3390/biom10111510
38. Almughlliq, FB, Koh, YQ, Peiris, HN, Vaswani, K, McDougall, S, Graham, EM, et al. Proteomic content of circulating exosomes in dairy cows with or without uterine infection. Theriogenology. (2018) 114:173–9. doi: 10.1016/j.theriogenology.2018.03.024
39. Zhao, G, Guo, S, Jiang, K, Zhang, T, Wu, H, Qiu, C, et al. MiRNA profiling of plasma-derived exosomes from dairy cows during gestation. Theriogenology. (2019) 130:89–98. doi: 10.1016/j.theriogenology.2019.03.001
40. Turner, N, Abeysinghe, P, Sadowski, P, and Mitchell, MD. Exosomal cargo may hold the key to improving reproductive outcomes in dairy cows. Int J Mol Sci. (2021) 22:2024. doi: 10.3390/ijms22042024
41. Ibrahim, S, Hedia, M, Taqi, MO, Derbala, MK, Mahmoud, KGM, Ahmed, Y, et al. Extracellular vesicles in low volume uterine lavage and serum: novel and promising biomarker for endometritis in Arabian mares. BMC Vet Res. (2022) 18:42. doi: 10.1186/s12917-022-03137-3
42. Mellisho, EA, Briones, MA, Velásquez, AE, Cabezas, J, Castro, FO, and Rodríguez-Álvarez, L. Extracellular vesicles secreted during blastulation show viability of bovine embryos. Reproduction. (2019) 158:477–92. doi: 10.1530/REP-19-0233
43. Koh, YQ, Peiris, HN, Vaswani, K, Almughlliq, FB, Meier, S, Burke, CR, et al. Proteome profiling of exosomes derived from plasma of heifers with divergent genetic merit for fertility. J Dairy Sci. (2018) 101:6462–73. doi: 10.3168/jds.2017-14190
44. Abeysinghe, P, Turner, N, Mosaad, E, Logan, J, and Mitchell, MD. Dynamics of inflammatory cytokine expression in bovine endometrial cells exposed to cow blood plasma small extracellular vesicles (sEV) may reflect high fertility. Sci Rep. (2023) 13:5425. doi: 10.1038/s41598-023-32045-1
45. Wang, X, Yao, X, Xie, T, Chang, Z, Guo, Y, and Ni, H. Exosome-derived uterine miR-218 isolated from cows with endometritis regulates the release of cytokines and chemokines. Microb Biotechnol. (2020) 13:1103–17. doi: 10.1111/1751-7915.13565
46. Dlamini, NH, Nguyen, T, Gad, A, Tesfaye, D, Liao, SF, Willard, ST, et al. Characterization of extracellular vesicle-coupled miRNA profiles in seminal plasma of boars with divergent semen quality status. Int J Mol Sci. (2023) 24:3194. doi: 10.3390/ijms24043194
47. Xie, Y, Xu, Z, Wu, C, Zhou, C, Zhang, X, Gu, T, et al. Extracellular vesicle-encapsulated miR-21-5p in seminal plasma prevents sperm capacitation via vinculin inhibition. Theriogenology. (2022) 193:103–13. doi: 10.1016/j.theriogenology.2022.09.014
48. Roca, J, Rodriguez-Martinez, H, Padilla, L, Lucas, X, and Barranco, I. Extracellular vesicles in seminal fluid and effects on male reproduction. An overview in farm animals and pets. Anim Reprod Sci. (2022) 246:106853. doi: 10.1016/j.anireprosci.2021.106853
49. Isaac, R, Reis, FCG, Ying, W, and Olefsky, JM. Exosomes as mediators of intercellular crosstalk in metabolism. Cell Metab. (2021) 33:1744–62. doi: 10.1016/j.cmet.2021.08.006
50. Cordeiro, L, Lin, HLH, Carvalho, AV, Grasseau, I, Uzbekov, R, and Blesbois, E. First insights on seminal extracellular vesicles in chickens of contrasted fertility. Reproduction. (2021) 161:489–98. doi: 10.1530/REP-20-0462
51. Song, S, Zhu, L, Wang, C, and Yang, Y. In vitro diagnostic technologies for the detection of extracellular vesicles: current status and future directions. VIEW. (2023) 4:20220011. doi: 10.1002/VIW.20220011
52. Crookenden, MA, Walker, CG, Peiris, H, Koh, Y, Almughlliq, F, Vaswani, K, et al. Effect of circulating exosomes from transition cows on Madin-Darby bovine kidney cell function. J Dairy Sci. (2017) 100:5687–700. doi: 10.3168/jds.2016-12152
53. Almughlliq, FB, Koh, YQ, Peiris, HN, Vaswani, K, Holland, O, Meier, S, et al. Circulating exosomes may identify biomarkers for cows at risk for metabolic dysfunction. Sci Rep. (2019) 9:13879. doi: 10.1038/s41598-019-50244-7
54. Ipinmoroti, AO, and Matthews, QL. Extracellular vesicles: roles in human viral infections, immune-diagnostic, and therapeutic applications. Pathogens. (2020) 9:1056. doi: 10.3390/pathogens9121056
55. Saenz-de-Juano, MD, Silvestrelli, G, Bauersachs, S, and Ulbrich, SE. Determining extracellular vesicles properties and miRNA cargo variability in bovine milk from healthy cows and cows undergoing subclinical mastitis. BMC Genomics. (2022) 23:189. doi: 10.1186/s12864-022-08377-z
56. Dias, MVS, Costa, CS, and daSilva, LLP. The ambiguous roles of extracellular vesicles in HIV replication and pathogenesis. Front Microbiol. (2018) 9:2411. doi: 10.3389/fmicb.2018.02411
57. Carossino, M, Dini, P, Kalbfleisch, TS, Loynachan, AT, Canisso, IF, Shuck, KM, et al. Downregulation of MicroRNA eca-mir-128 in seminal exosomes and enhanced expression of CXCL16 in the stallion reproductive tract are associated with long-term persistence of equine arteritis virus. J Virol. (2018) 92:18. doi: 10.1128/JVI.00015-18
58. Da Cruz, AB, Carneiro, FM, Maia, MM, Pereira I de, S, Taniwaki, NN, Namiyama, GM, et al. Dogs with canine visceral leishmaniasis have a boost of extracellular vesicles and miR-21-5p up-expression. Parasite Immunol. (2023) 45:e13004. doi: 10.1111/pim.13004
59. Kalluri, R, and McAndrews, KM. The role of extracellular vesicles in cancer. Cell. (2023) 186:1610–26. doi: 10.1016/j.cell.2023.03.010
60. Kulka, M, Brennan, K, and Mc, GM. Investigation of canine extracellular vesicles in diffuse large B-cell lymphomas. PLoS One. (2022) 17:e0274261. doi: 10.1371/journal.pone.0274261
61. Beaumier, A, Robinson, SR, Robinson, N, Lopez, KE, Meola, DM, Barber, LG, et al. Extracellular vesicular microRNAs as potential biomarker for early detection of doxorubicin-induced cardiotoxicity. J Vet Intern Med. (2020) 34:1260–71. doi: 10.1111/jvim.15762
62. Narita, M, Nishida, H, Asahina, R, Nakata, K, Yano, H, Dickinson, PJ, et al. Expression of microRNAs in plasma and in extracellular vesicles derived from plasma for dogs with glioma and dogs with other brain diseases. Am J Vet Res. (2020) 81:355–60. doi: 10.2460/ajvr.81.4.355
63. Schorey, JS, Cheng, Y, Singh, PP, and Smith, VL. Exosomes and other extracellular vesicles in host-pathogen interactions. EMBO Rep. (2015) 16:24–43. doi: 10.15252/embr.201439363
64. Ortega, MA, Fraile-Martínez, O, García-Montero, C, Paradela, A, Asunción Sánchez-Gil, M, Rodriguez-Martin, S, et al. Unfolding the role of placental-derived extracellular vesicles in pregnancy: from homeostasis to pathophysiology. Front Cell Dev Biol. (2022) 10:1060850. doi: 10.3389/fcell.2022.1060850
65. Abeysinghe, P, Turner, N, Morean Garcia, I, Mosaad, E, Peiris, HN, and Mitchell, MD. The role of Exosomal epigenetic modifiers in cell communication and fertility of dairy cows. Int J Mol Sci. (2020) 21:9106. doi: 10.3390/ijms21239106
66. Pohler, KG, Green, JA, Moley, LA, Gunewardena, S, Hung, WT, Payton, RR, et al. Circulating microRNA as candidates for early embryonic viability in cattle. Mol Reprod Dev. (2017) 84:731–43. doi: 10.1002/mrd.22856
67. Hamdi, M, Cañon-Beltrán, K, Mazzarella, R, Cajas, YN, Leal, CLV, Gutierrez-Adan, A, et al. Characterization and profiling analysis of bovine oviduct and uterine extracellular vesicles and their miRNA cargo through the estrous cycle. FASEB J. (2021) 35:e22000. doi: 10.1096/fj.202101023R
68. Almughlliq, FB, Koh, YQ, Peiris, HN, Vaswani, K, McDougall, S, Graham, EM, et al. Effect of exosomes from plasma of dairy cows with or without an infected uterus on prostaglandin production by endometrial cell lines. J Dairy Sci. (2017) 100:9143–52. doi: 10.3168/jds.2017-13261
69. Pedrosa, AC, Torres, MA, Alkmin, DV, Pinzon, JEP, Massami Kitamura Martins, SM, da Silveira, JC, et al. Spermatozoa and seminal plasma small extracellular vesicles miRNAs as biomarkers of boar semen cryotolerance. Theriogenology. (2021) 174:60–72. doi: 10.1016/j.theriogenology.2021.07.022
70. Martínez, MC, and Andriantsitohaina, R. Extracellular vesicles in metabolic syndrome. Circ Res. (2017) 120:1674–86. doi: 10.1161/CIRCRESAHA.117.309419
71. Katayama, M, Wiklander, OPB, Fritz, T, Caidahl, K, El-Andaloussi, S, Zierath, JR, et al. Circulating Exosomal miR-20b-5p is elevated in type 2 diabetes and could impair insulin action in human skeletal muscle. Diabetes. (2019) 68:515–26. doi: 10.2337/db18-0470
72. Gallo, W, Esguerra, JLS, Eliasson, L, and Melander, O. miR-483-5p associates with obesity and insulin resistance and independently associates with new onset diabetes mellitus and cardiovascular disease. PLoS One. (2018) 13:e0206974. doi: 10.1371/journal.pone.0206974
73. Liu, T, Wang, Y, Zhao, M, Jiang, J, Li, T, and Zhang, M. Differential expression of aerobic oxidative metabolism-related proteins in diabetic urinary exosomes. Front Endocrinol (Lausanne). (2022) 13:992827. doi: 10.3389/fendo.2022.992827
74. Camino, T, Lago-Baameiro, N, Sueiro, A, Bravo, SB, Couto, I, Santos, FF, et al. Brown adipose tissue sheds extracellular vesicles that carry potential biomarkers of metabolic and thermogenesis activity which are affected by high fat diet intervention. Int J Mol Sci. (2022) 23:10826. doi: 10.3390/ijms231810826
75. Li, Y, Meng, Y, Zhu, X, Van Wijnen, A, Eirin, A, and Lerman, LO. Metabolic syndrome is associated with altered mRNA and miRNA content in human circulating extracellular vesicles. Front Endocrinol (Lausanne). (2021) 12:687586. doi: 10.3389/fendo.2021.687586
76. Crookenden, MA, Walker, CG, Peiris, H, Koh, Y, Heiser, A, Loor, JJ, et al. Short communication: proteins from circulating exosomes represent metabolic state in transition dairy cows. J Dairy Sci. (2016) 99:7661–8. doi: 10.3168/jds.2015-10786
77. Quan, S, Nan, X, Wang, K, Jiang, L, Yao, J, and Xiong, B. Different diets change the expression of bovine serum extracellular vesicle-miRNAs. Animals. (2019) 9:1137. doi: 10.3390/ani9121137
78. Steingart, KR, Henry, M, Ng, V, Hopewell, PC, Ramsay, A, Cunningham, J, et al. Fluorescence versus conventional sputum smear microscopy for tuberculosis: a systematic review. Lancet Infect Dis. (2006) 6:570–81. doi: 10.1016/S1473-3099(06)70578-3
79. Wang, J, Wang, Y, Tang, L, and Garcia, RC. Extracellular vesicles in mycobacterial infections: their potential as molecule transfer vectors. Front Immunol. (2019) 10:1929. doi: 10.3389/fimmu.2019.01929
80. Giri, PK, Kruh, NA, Dobos, KM, and Schorey, JS. Proteomic analysis identifies highly antigenic proteins in exosomes from M. tuberculosis-infected and culture filtrate protein-treated macrophages. Proteomics. (2010) 10:3190–202. doi: 10.1002/pmic.200900840
81. Kruh-Garcia, NA, Wolfe, LM, Chaisson, LH, Worodria, WO, Nahid, P, Schorey, JS, et al. Detection of Mycobacterium tuberculosis peptides in the exosomes of patients with active and latent M. tuberculosis infection using MRM-MS. PLoS One. (2014) 9:e103811. doi: 10.1371/journal.pone.0103811
82. Palacios, A, Sampedro, L, Sevilla, IA, Molina, E, Gil, D, Azkargorta, M, et al. Mycobacterium tuberculosis extracellular vesicle-associated lipoprotein LpqH as a potential biomarker to distinguish paratuberculosis infection or vaccination from tuberculosis infection. BMC Vet Res. (2019) 15:188. doi: 10.1186/s12917-019-1941-6
83. Carrera-Bravo, C, Koh, EY, and Tan, KSW. The roles of parasite-derived extracellular vesicles in disease and host-parasite communication. Parasitol Int. (2021) 83:102373. doi: 10.1016/j.parint.2021.102373
84. Tiberti, N, Latham, SL, Bush, S, Cohen, A, Opoka, RO, John, CC, et al. Exploring experimental cerebral malaria pathogenesis through the characterisation of host-derived plasma microparticle protein content. Sci Rep. (2016) 6:37871. doi: 10.1038/srep37871
85. Esteves, S, Lima, C, Costa, I, Osorio, H, Fernandez-Becerra, C, Santarem, N, et al. Characterization and proteomic analysis of plasma EVs recovered from healthy and diseased dogs with canine leishmaniosis. Int J Mol Sci. (2023) 24:5490. doi: 10.3390/ijms24065490
86. Moon, PG, Lee, JE, Cho, YE, Lee, SJ, Jung, JH, Chae, YS, et al. Identification of developmental endothelial Locus-1 on circulating extracellular vesicles as a novel biomarker for early breast Cancer detection. Clin Cancer Res. (2016) 22:1757–66. doi: 10.1158/1078-0432.CCR-15-0654
87. McKiernan, J, Donovan, MJ, O’Neill, V, Bentink, S, Noerholm, M, Belzer, S, et al. A novel urine exosome gene expression assay to predict high-grade prostate Cancer at initial biopsy. JAMA Oncol. (2016) 2:882–9. doi: 10.1001/jamaoncol.2016.0097
88. Clos-Garcia, M, Loizaga-Iriarte, A, Zuñiga-Garcia, P, Sánchez-Mosquera, P, Rosa Cortazar, A, González, E, et al. Metabolic alterations in urine extracellular vesicles are associated to prostate cancer pathogenesis and progression. J Extracell Vesicles. (2018) 7:1470442. doi: 10.1080/20013078.2018.1470442
89. Northrop-Albrecht, EJ, Taylor, WR, Huang, BQ, Kisiel, JB, and Lucien, F. Assessment of extracellular vesicle isolation methods from human stool supernatant. J Extracell Vesicles. (2022) 11:e12208. doi: 10.1002/jev2.12208
90. Sammarco, A, Finesso, G, Cavicchioli, L, Ferro, S, Caicci, F, Zanetti, R, et al. Preliminary investigation of extracellular vesicles in mammary cancer of dogs and cats: identification and characterization. Vet Comp Oncol. (2018) 16:489–96. doi: 10.1111/vco.12405
91. Simundic, M, Svara, T, Stukelj, R, Krek, JL, Gombac, M, Kralj-Iglic, V, et al. Concentration of extracellular vesicles isolated from blood relative to the clinical pathological status of dogs with mast cell tumours. Vet Comp Oncol. (2019) 17:456–64. doi: 10.1111/vco.12489
92. Asada, H, Tomiyasu, H, Uchikai, T, Ishihara, G, Goto-Koshino, Y, Ohno, K, et al. Comprehensive analysis of miRNA and protein profiles within exosomes derived from canine lymphoid tumour cell lines. PLoS One. (2019) 14:e0208567. doi: 10.1371/journal.pone.0208567
93. Garnica, TK, Lesbon, JCC, Ávila, ACFCM, Rochetti, AL, Matiz, ORS, Ribeiro, RCS, et al. Liquid biopsy based on small extracellular vesicles predicts chemotherapy response of canine multicentric lymphomas. Sci Rep. (2020) 10:366. doi: 10.1038/s41598-020-77366-7
94. Anticoli, S, Manfredi, F, Chiozzini, C, Arenaccio, C, Olivetta, E, Ferrantelli, F, et al. An exosome-based vaccine platform imparts cytotoxic T lymphocyte immunity against viral antigens. Biotechnol J. (2018) 13:e1700443. doi: 10.1002/biot.201700443
95. Coppieters, K, Barral, AM, Juedes, A, Wolfe, T, Rodrigo, E, Théry, C, et al. No significant CTL cross-priming by dendritic cell-derived exosomes during murine lymphocytic choriomeningitis virus infection. J Immunol. (2009) 182:2213–20. doi: 10.4049/jimmunol.0802578
96. Montaner-Tarbes, S, Del Portillo, HA, Montoya, M, and Fraile, L. Key gaps in the knowledge of the porcine respiratory reproductive syndrome virus (PRRSV). Front Vet Sci. (2019) 6:38. doi: 10.3389/fvets.2019.00038
97. Kornicka-Garbowska, K, Pędziwiatr, R, Woźniak, P, Kucharczyk, K, and Marycz, K. Microvesicles isolated from 5-azacytidine-and-resveratrol-treated mesenchymal stem cells for the treatment of suspensory ligament injury in horse-a case report. Stem Cell Res Ther. (2019) 10:394. doi: 10.1186/s13287-019-1469-5
98. Montaner-Tarbes, S, Novell, E, Tarancón, V, Borrás, FE, Montoya, M, Fraile, L, et al. Targeted-pig trial on safety and immunogenicity of serum-derived extracellular vesicles enriched fractions obtained from porcine respiratory and reproductive virus infections. Sci Rep. (2018) 8:17487. doi: 10.1038/s41598-018-36141-5
99. Micoli, F, and MacLennan, CA. Outer membrane vesicle vaccines. Semin Immunol. (2020) 50:101433. doi: 10.1016/j.smim.2020.101433
100. Wu, G, Ji, H, Guo, X, Li, Y, Ren, T, Dong, H, et al. Nanoparticle reinforced bacterial outer-membrane vesicles effectively prevent fatal infection of carbapenem-resistant Klebsiella pneumoniae. Nanomedicine. (2020) 24:102148. doi: 10.1016/j.nano.2019.102148
101. Schild, S, Nelson, EJ, and Camilli, A. Immunization with Vibrio cholerae outer membrane vesicles induces protective immunity in mice. Infect Immun. (2008) 76:4554–63. doi: 10.1128/IAI.00532-08
102. Fransen, F, Stenger, RM, Poelen, MCM, van Dijken, HH, Kuipers, B, Boog, CJP, et al. Differential effect of TLR2 and TLR4 on the immune response after immunization with a vaccine against Neisseria meningitidis or Bordetella pertussis. PLoS One. (2010) 5:e15692. doi: 10.1371/journal.pone.0015692
103. Galloway, Y, Stehr-Green, P, McNicholas, A, and O’Hallahan, J. Use of an observational cohort study to estimate the effectiveness of the New Zealand group B meningococcal vaccine in children aged under 5 years. Int J Epidemiol. (2009) 38:413–8. doi: 10.1093/ije/dyn228
104. Prior, JT, Davitt, C, Kurtz, J, Gellings, P, McLachlan, JB, and Morici, LA. Bacterial-derived outer membrane vesicles are potent adjuvants that drive humoral and cellular immune responses. Pharmaceutics. (2021) 13:131. doi: 10.3390/pharmaceutics13020131
105. Lee, TY, Kim, CU, Lee, P, Seo, SH, Bae, EH, Kim, YS, et al. Outer membrane vesicle increases the efficacy of an influenza vaccine in a diet-induced obese mouse model. Immunol Lett. (2020) 219:27–33. doi: 10.1016/j.imlet.2019.12.009
106. Twu, O, and Johnson, PJ. Parasite extracellular vesicles: mediators of intercellular communication. PLoS Pathog. (2014) 10:e1004289. doi: 10.1371/journal.ppat.1004289
107. LoVerde, PT. Schistosomiasis. Adv Exp Med Biol. (2019) 1154:45–70. doi: 10.1007/978-3-030-18616-6_3
108. Samoil, V, Dagenais, M, Ganapathy, V, Aldridge, J, Glebov, A, Jardim, A, et al. Vesicle-based secretion in schistosomes: analysis of protein and microRNA (miRNA) content of exosome-like vesicles derived from Schistosoma mansoni. Sci Rep. (2018) 8:3286. doi: 10.1038/s41598-018-21587-4
109. Sotillo, J, Pearson, M, Potriquet, J, Becker, L, Pickering, D, Mulvenna, J, et al. Extracellular vesicles secreted by Schistosoma mansoni contain protein vaccine candidates. Int J Parasitol. (2016) 46:1–5. doi: 10.1016/j.ijpara.2015.09.002
110. Assolini, JP, Concato, VM, Gonçalves, MD, Carloto, ACM, Conchon-Costa, I, Pavanelli, WR, et al. Nanomedicine advances in toxoplasmosis: diagnostic, treatment, and vaccine applications. Parasitol Res. (2017) 116:1603–15. doi: 10.1007/s00436-017-5458-2
111. Jung, BK, Kim, ED, Song, H, Chai, JY, and Seo, KY. Immunogenicity of exosomes from dendritic cells stimulated with toxoplasma gondii lysates in Ocularly immunized mice. Korean J Parasitol. (2020) 58:185–9. doi: 10.3347/kjp.2020.58.2.185
112. Del Cacho, E, Gallego, M, Lee, SH, Lillehoj, HS, Quilez, J, Lillehoj, EP, et al. Induction of protective immunity against Eimeria tenella infection using antigen-loaded dendritic cells (DC) and DC-derived exosomes. Vaccine. (2011) 29:3818–25. doi: 10.1016/j.vaccine.2011.03.022
113. El-Tookhy, OS, Shamaa, AA, Shehab, GG, Abdallah, AN, and Azzam, OM. Histological evaluation of experimentally induced critical size defect skin wounds using Exosomal solution of mesenchymal stem cells derived microvesicles. Int J Stem Cells. (2017) 10:144–53. doi: 10.15283/ijsc17043
114. An, JH, Li, Q, Ryu, MO, Nam, AR, Bhang, DH, Jung, YC, et al. TSG-6 in extracellular vesicles from canine mesenchymal stem/stromal is a major factor in relieving DSS-induced colitis. PLoS One. (2020) 15:e0220756. doi: 10.1371/journal.pone.0220756
115. Lee, KM, An, JH, Yang, SJ, Park, SM, Lee, JH, Chae, HK, et al. Influence of canine macrophage-derived extracellular vesicles on apoptosis in canine melanoma and osteosarcoma cell lines. Anticancer Res. (2021) 41:719–30. doi: 10.21873/anticanres.14823
116. Qi, J, Liu, Q, Reisdorf, RL, Boroumand, S, Behfar, A, Moran, SL, et al. Characterization of a purified exosome product and its effects on canine flexor tenocyte biology. J Orthop Res. (2020) 38:1845–55. doi: 10.1002/jor.24587
117. Arevalo-Turrubiarte, M, Baratta, M, Ponti, G, Chiaradia, E, and Martignani, E. Extracellular vesicles from equine mesenchymal stem cells decrease inflammation markers in chondrocytes in vitro. Equine Vet J. (2022) 54:1133–43. doi: 10.1111/evj.13537
118. Liu, H, Huang, L, Chen, F, Zhong, Z, Ma, X, Zhou, Z, et al. Adipose-derived mesenchymal stem cells secrete extracellular vesicles: a potential cell-free therapy for canine renal ischaemia-reperfusion injury. Vet Med Sci. (2023) 9:1134–42. doi: 10.1002/vms3.1105
119. Moccia, V, Sammarco, A, Cavicchioli, L, Castagnaro, M, Bongiovanni, L, and Zappulli, V. Extracellular vesicles in veterinary medicine. Animals. (2022) 12:2716. doi: 10.3390/ani12192716
120. Phinney, DG, and Pittenger, MF. Concise review: MSC-derived exosomes for cell-free therapy. Stem Cells. (2017) 35:851–8. doi: 10.1002/stem.2575
121. Hyvärinen, K, Holopainen, M, Skirdenko, V, Ruhanen, H, Lehenkari, P, Korhonen, M, et al. Mesenchymal stromal cells and their extracellular vesicles enhance the anti-inflammatory phenotype of regulatory macrophages by downregulating the production of interleukin (IL)-23 and IL-22. Front Immunol. (2018) 9:771. doi: 10.3389/fimmu.2018.00771
122. Yoshida, M, Satoh, A, Lin, JB, Mills, KF, Sasaki, Y, Rensing, N, et al. Extracellular vesicle-contained eNAMPT delays aging and extends lifespan in mice. Cell Metab. (2019) 30:329–342.e5. doi: 10.1016/j.cmet.2019.05.015
123. Li, X, Lian, Y, Wu, Y, Ye, Z, Feng, J, Zhao, Y, et al. Neonatal plasma exosomes contribute to endothelial cell-mediated angiogenesis and cardiac repair after acute myocardial infarction. Int J Mol Sci. (2023) 24:3196. doi: 10.3390/ijms24043196
124. Muchedzi, TA, and Roberts, SB. A systematic review of the effects of platelet rich plasma on outcomes for patients with knee osteoarthritis and following total knee arthroplasty. Surgeon. (2018) 16:250–8. doi: 10.1016/j.surge.2017.08.004
125. Gentile, P, Calabrese, C, De Angelis, B, Dionisi, L, Pizzicannella, J, Kothari, A, et al. Impact of the different preparation methods to obtain autologous non-activated platelet-rich plasma (A-PRP) and activated platelet-rich plasma (AA-PRP) in plastic surgery: wound healing and hair regrowth evaluation. Int J Mol Sci. (2020) 21:431. doi: 10.3390/ijms21020431
126. Wu, J, Piao, Y, Liu, Q, and Yang, X. Platelet-rich plasma-derived extracellular vesicles: a superior alternative in regenerative medicine? Cell Prolif. (2021) 54:e13123. doi: 10.1111/cpr.13123
127. Wang, Y, Liu, S, Li, L, Li, L, Zhou, X, Wan, M, et al. Peritoneal M2 macrophage-derived extracellular vesicles as natural multitarget nanotherapeutics to attenuate cytokine storms after severe infections. J Control Release. (2022) 349:118–32. doi: 10.1016/j.jconrel.2022.06.063
128. Zhang, J, Zhou, X, and Hao, H. Macrophage phenotype-switching in cancer. Eur J Pharmacol. (2022) 931:175229. doi: 10.1016/j.ejphar.2022.175229
129. Gunassekaran, GR, Poongkavithai Vadevoo, SM, Baek, MC, and Lee, B. M1 macrophage exosomes engineered to foster M1 polarization and target the IL-4 receptor inhibit tumor growth by reprogramming tumor-associated macrophages into M1-like macrophages. Biomaterials. (2021) 278:121137. doi: 10.1016/j.biomaterials.2021.121137
130. Hodge, AL, Baxter, AA, and Poon, IKH. Gift bags from the sentinel cells of the immune system: the diverse role of dendritic cell-derived extracellular vesicles. J Leukoc Biol. (2022) 111:903–20. doi: 10.1002/JLB.3RU1220-801R
131. Kim, SH, Lechman, ER, Bianco, N, Menon, R, Keravala, A, Nash, J, et al. Exosomes derived from IL-10-treated dendritic cells can suppress inflammation and collagen-induced arthritis. J Immunol. (2005) 174:6440–8. doi: 10.4049/jimmunol.174.10.6440
132. Yang, X, Meng, S, Jiang, H, Chen, T, and Wu, W. Exosomes derived from interleukin-10-treated dendritic cells can inhibit trinitrobenzene sulfonic acid-induced rat colitis. Scand J Gastroenterol. (2010) 45:1168–77. doi: 10.3109/00365521.2010.490596
133. Cai, Z, Zhang, W, Yang, F, Yu, L, Yu, Z, Pan, J, et al. Immunosuppressive exosomes from TGF-β1 gene-modified dendritic cells attenuate Th17-mediated inflammatory autoimmune disease by inducing regulatory T cells. Cell Res. (2012) 22:607–10. doi: 10.1038/cr.2011.196
134. Radomski, N, Karger, A, Franzke, K, Liebler-Tenorio, E, Jahnke, R, Matthiesen, S, et al. Chlamydia psittaci-infected dendritic cells communicate with NK cells via exosomes to activate antibacterial immunity. Infect Immun. (2019) 88:e00541–19. doi: 10.1128/IAI.00541-19
135. Yin, B, Ni, J, Witherel, CE, Yang, M, Burdick, JA, Wen, C, et al. Harnessing tissue-derived extracellular vesicles for osteoarthritis Theranostics. Theranostics. (2022) 12:207–31. doi: 10.7150/thno.62708
136. Wang, B, Zhuang, X, Deng, ZB, Jiang, H, Mu, J, Wang, Q, et al. Targeted drug delivery to intestinal macrophages by bioactive nanovesicles released from grapefruit. Mol Ther. (2014) 22:522–34. doi: 10.1038/mt.2013.190
137. Zhang, X, Du, Q, Liu, C, Yang, Y, Wang, J, Duan, S, et al. Rhodioloside ameliorates depressive behavior via up-regulation of monoaminergic system activity and anti-inflammatory effect in olfactory bulbectomized rats. Int Immunopharmacol. (2016) 36:300–4. doi: 10.1016/j.intimp.2016.05.008
138. Cao, M, Yan, H, Han, X, Weng, L, Wei, Q, Sun, X, et al. Ginseng-derived nanoparticles alter macrophage polarization to inhibit melanoma growth. J Immunother Cancer. (2019) 7:326. doi: 10.1186/s40425-019-0817-4
139. Smyth, T, Kullberg, M, Malik, N, Smith-Jones, P, Graner, MW, and Anchordoquy, TJ. Biodistribution and delivery efficiency of unmodified tumor-derived exosomes. J Control Release. (2015) 199:145–55. doi: 10.1016/j.jconrel.2014.12.013
140. Wiklander, OPB, Nordin, JZ, O’Loughlin, A, Gustafsson, Y, Corso, G, Mäger, I, et al. Extracellular vesicle in vivo biodistribution is determined by cell source, route of administration and targeting. J Extracell Vesicles. (2015) 4:26316. doi: 10.3402/jev.v4.26316
141. Richter, M, Vader, P, and Fuhrmann, G. Approaches to surface engineering of extracellular vesicles. Adv Drug Deliv Rev. (2021) 173:416–26. doi: 10.1016/j.addr.2021.03.020
142. Mentkowski, KI, and Lang, JK. Exosomes engineered to express a cardiomyocyte binding peptide demonstrate improved cardiac retention in vivo. Sci Rep. (2019) 9:10041. doi: 10.1038/s41598-019-46407-1
143. Zheng, W, He, R, Liang, X, Roudi, S, Bost, J, Coly, PM, et al. Cell-specific targeting of extracellular vesicles though engineering the glycocalyx. J Extracell Vesicles. (2022) 11:e12290. doi: 10.1002/jev2.12290
144. De Jong, WH, and Borm, PJA. Drug delivery and nanoparticles:applications and hazards. Int J Nanomedicine. (2008) 3:133–49. doi: 10.2147/IJN.S596
145. Tao, SC, Li, XR, Wei, WJ, Wei, ZY, Zhang, CR, Wang, F, et al. Polymeric coating on β-TCP scaffolds provides immobilization of small extracellular vesicles with surface-functionalization and ZEB1-loading for bone defect repair in diabetes mellitus. Biomaterials. (2022) 283:121465. doi: 10.1016/j.biomaterials.2022.121465
146. Li, C, Zhang, J, Zu, YJ, Nie, SF, Cao, J, Wang, Q, et al. Biocompatible and biodegradable nanoparticles for enhancement of anti-cancer activities of phytochemicals. Chin J Nat Med. (2015) 13:641–52. doi: 10.1016/S1875-5364(15)30061-3
147. Bastos, N, Ruivo, CF, da Silva, S, and Melo, SA. Exosomes in cancer: use them or target them? Semin Cell Dev Biol. (2018) 78:13–21. doi: 10.1016/j.semcdb.2017.08.009
148. Kamerkar, S, LeBleu, VS, Sugimoto, H, Yang, S, Ruivo, CF, Melo, SA, et al. Exosomes facilitate therapeutic targeting of oncogenic KRAS in pancreatic cancer. Nature. (2017) 546:498–503. doi: 10.1038/nature22341
149. Yuan, Z, Kolluri, KK, Gowers, KHC, and Janes, SM. TRAIL delivery by MSC-derived extracellular vesicles is an effective anticancer therapy. J Extracell Vesicles. (2017) 6:1265291. doi: 10.1080/20013078.2017.1265291
150. Jiang, L, Gu, Y, Du, Y, Tang, X, Wu, X, and Liu, J. Engineering exosomes endowed with targeted delivery of Triptolide for malignant melanoma therapy. ACS Appl Mater Interfaces. (2021) 13:42411–28. doi: 10.1021/acsami.1c10325
151. Santos, NL, Bustos, SO, Reis, PP, Chammas, R, and Andrade, LNS. Extracellular vesicle-packaged miR-195-5p sensitizes melanoma to targeted therapy with kinase inhibitors. Cell. (2023) 12:1317. doi: 10.3390/cells12091317
152. Zhao, X, Zhao, R, and Nie, G. Nanocarriers based on bacterial membrane materials for cancer vaccine delivery. Nat Protoc. (2022) 17:2240–74. doi: 10.1038/s41596-022-00713-7
153. Wang, C, Wang, M, Xu, T, Zhang, X, Lin, C, Gao, W, et al. Engineering bioactive self-healing antibacterial exosomes hydrogel for promoting chronic diabetic wound healing and complete skin regeneration. Theranostics. (2019) 9:65–76. doi: 10.7150/thno.29766
154. Lou, P, Liu, S, Wang, Y, Pan, C, Xu, X, Zhao, M, et al. Injectable self-assembling peptide nanofiber hydrogel as a bioactive 3D platform to promote chronic wound tissue regeneration. Acta Biomater. (2021) 135:100–12. doi: 10.1016/j.actbio.2021.08.008
155. Zhou, Y, Liu, S, Zhao, M, Wang, C, Li, L, Yuan, Y, et al. Injectable extracellular vesicle-released self-assembling peptide nanofiber hydrogel as an enhanced cell-free therapy for tissue regeneration. J Control Release. (2019) 316:93–104. doi: 10.1016/j.jconrel.2019.11.003
156. Brennan, K, Martin, K, FitzGerald, SP, O’Sullivan, J, Wu, Y, Blanco, A, et al. A comparison of methods for the isolation and separation of extracellular vesicles from protein and lipid particles in human serum. Sci Rep. (2020) 10:1039. doi: 10.1038/s41598-020-57497-7
157. Görgens, A, Corso, G, Hagey, DW, Jawad Wiklander, R, Gustafsson, MO, Felldin, U, et al. Identification of storage conditions stabilizing extracellular vesicles preparations. J Extracell Vesicles. (2022) 11:e12238. doi: 10.1002/jev2.12238
Keywords: extracellular vesicles, exosome, biomarker, vaccine, therapy, veterinary medicine
Citation: Xiong Y, Lou P, Xu C, Han B, Liu J and Gao J (2024) Emerging role of extracellular vesicles in veterinary practice: novel opportunities and potential challenges. Front. Vet. Sci. 11:1335107. doi: 10.3389/fvets.2024.1335107
Edited by:
Zhixin Lei, Wuhan University of Technology, ChinaReviewed by:
Haseeb Khaliq, Cholistan University of Veterinary and Animal Sciences, PakistanBin He, Wuhan Academy of Agricultural Sciences, China
Copyright © 2024 Xiong, Lou, Xu, Han, Liu and Gao. This is an open-access article distributed under the terms of the Creative Commons Attribution License (CC BY). The use, distribution or reproduction in other forums is permitted, provided the original author(s) and the copyright owner(s) are credited and that the original publication in this journal is cited, in accordance with accepted academic practice. No use, distribution or reproduction is permitted which does not comply with these terms.
*Correspondence: Jian Gao, Z2FvamlhbjIwMTZAY2F1LmVkdS5jbg==