- 1Departamento de Microbiología y Genética, Universidad de Salamanca, Salamanca, Spain
- 2Instituto de Investigación Sanitaria de Navarra and Departamento de Microbiología y Parasitología, Universidad de Navarra, Pamplona, Spain
- 3Departamento de Ciencia Animal, Centro de Investigación y Tecnología Agroalimentaria de Aragón (CITA), Zaragoza, Spain
- 4Instituto Agroalimentario de Aragón-IA2 (CITA-Universidad de Zaragoza), Zaragoza, Spain
- 5Instituto de Investigación Biomédica de Salamanca (IBSAL), Salamanca, Spain
Brucella ovis causes non-zoonotic ovine brucellosis of worldwide distribution and is responsible for important economic losses mainly derived from male genital lesions and reproductive fails. Studies about the virulence mechanisms of this rough species (lacking lipopolysaccharide O-chains) are underrepresented when compared to the main zoonotic Brucella species that are smooth (with O-chains). Zinc intoxication constitutes a defense mechanism of the host against bacterial pathogens, which have developed efflux systems to counterbalance toxicity. In this study, we have characterized three potential B. ovis zinc exporters, including the ZntA ortholog previously studied in B. abortus. Despite an in-frame deletion removing 100 amino acids from B. ovis ZntA, the protein retained strong zinc efflux properties. Only indirect evidence suggested a higher exporter activity for B. abortus ZntA, which, together with differences in ZntR-mediated regulation of zntA expression between B. ovis and B. abortus, could contribute to explaining why the ΔzntR mutant of B. abortus is attenuated while that of B. ovis is virulent. Additionally, B. ovis ZntA was revealed as a powerful cadmium exporter contributing to cobalt, copper, and nickel detoxification, properties not previously described for the B. abortus ortholog. Deletion mutants for BOV_0501 and BOV_A1100, also identified as potential zinc exporters and pseudogenes in B. abortus, behaved as the B. ovis parental strain in all tests performed. However, their overexpression in the ΔzntA mutant allowed the detection of discrete zinc and cobalt efflux activity for BOV_0501 and BOV_A1100, respectively. Nevertheless, considering their low expression levels and the stronger activity of ZntA as a zinc and cobalt exporter, the biological role of BOV_0501 and BOV_A1100 is questionable. Results presented in this study evidence heterogeneity among pathogenic Brucellae regarding zinc export and, considering the virulence of B. ovis ΔzntA, suggest that host-mediated zinc intoxication is not a relevant mechanism to control B. ovis infection.
1 Introduction
Brucella ovis is a gram-negative bacterium of the genus Brucella that causes a serious ovine reproductive disease manifested by male genital lesions, reduced fertility, abortion, and consequent economic losses (1, 2). Most Brucella spp. are smooth bacteria exposing an O-polysaccharide (O-PS) linked to the lipid A-core oligosaccharide. This is the case of B. melitensis, B. abortus, or B. suis, which preferentially infect sheep/goats, cattle, and pigs, respectively, and cause notifiable reproductive and zoonotic diseases. In smooth brucellae, the O-PS is critical for virulence and carries the main epitopes involved in their serodiagnosis (3–5). By contrast, B. ovis is a non-zoonotic rough bacterium with a lipopolysaccharide devoid of O-PS, which also plays an essential role in its virulence and immunogenicity (6).
The unique vaccine available against sheep and goat brucellosis is the live-attenuated B. melitensis Rev. 1 smooth strain, which confers protection against both B. melitensis and B. ovis infection (1, 7). However, since Rev. 1 is infective for humans and induces antibodies against the O-PS, which can interfere with the routine diagnosis required for B. melitensis surveillance, this vaccine is banned in B. melitensis-free areas. Consequently, the incidence of B. ovis infection is increasing in some of these regions (2, 8), which calls for the development of a B. ovis-specific vaccine. Ideally, this vaccine should be safe (non-zoonotic) and not trigger O-PS antibodies. In this context, obtaining attenuated B. ovis strains with potential use as B. ovis homologous vaccine is of interest. Although studies regarding B. ovis are progressively increasing in number, most knowledge about the mechanism and genes involved in Brucella virulence has been obtained from the smooth B. melitensis and B. abortus species. Further research on B. ovis virulence factors and host–pathogen interaction mechanisms is needed to acquire proper knowledge toward the development of vaccine candidates.
An inherent trait of pathogenic smooth and rough Brucellae is that they are facultative intracellular bacteria able to build a replicative niche inside phagocytes that provides a “safe” environment, favoring their escape from the host immune response and the establishment of chronic infections. Accordingly, Brucella mutants showing defects in intracellular survival are usually attenuated in virulence in the natural host and laboratory animals (4). Although the availability of nutrients in the host is a general requirement for the survival and multiplication of bacterial pathogens, it is even more relevant in the restricted intracellular environment. Metal ions are essential micronutrients for various cellular processes involved in bacterial cell viability and virulence, but high concentrations inside the cell lead to serious detrimental effects (9–11). Metals must also be finely balanced in the host, where, in addition, they play an important role in the control of bacterial infections through both metal intoxication mechanisms and a process known as nutritional immunity that restricts metal availability to the pathogen (11). Through evolution, animal hosts have developed several strategies involving metal ions to control the progress of infection, but simultaneously, bacterial pathogens have evolved to minimize the effect of these host defense strategies (10, 11). Since metals cannot be degraded or synthesized by the cell, homeostasis depends on proper coordination of import and export systems (10).
Zinc primarily acts as an enzyme cofactor in bacteria, where 5–6% of proteins are Zn-binding proteins involved in essential metabolic pathways (12). On the contrary, Zn is a highly competitive divalent cation that, when in excess, can displace other metal cofactors from their binding sites in other essential metalloenzymes. To maintain suitable Zn intracellular levels, bacteria have developed three main strategies: (i) Zn-sensing proteins acting as transcriptional regulators, (ii) Zn importer and exporter systems able to mobilize the metal across cell membranes, and (iii) zinc sparing and allocation under extreme Zn scarcity conditions that either increases the levels of Zn-independent proteins with equivalent functions to those performed by Zn-dependent proteins or ensures that Zn is provided, through intracellular chelators acting as Zn reservoirs, to Zn-dependent proteins whose function cannot be replaced (12, 13).
The P1B-type ATPase ZntA and its transcriptional regulator ZntR have been linked to Zn2+ export in smooth B. abortus 2308, and overexpression of zntA was proposed as responsible for the attenuation of the ΔzntR mutant of this strain (14). However, no additional reports about the relevance of Zn2+ efflux systems in other Brucella species have been published until now. This type of study with rough B. ovis could not only contribute to the development of potential vaccine candidates but also provide interesting information about the similarities and differences among pathogenic brucellae that are highly homologous at the DNA level but have important differences regarding pathogenicity and host preference.
In this study, in addition to the ZntA-ZntR system, two other proteins potentially involved in zinc export were identified by analyzing the B. ovis genome. With the aim of determining their relevance for in vitro and in vivo survival of rough B. ovis PA, a panel of 14 genetically modified strains (including deletion mutants, complemented strains, and strains overexpressing selected genes) was constructed and analyzed for gene expression, in vitro survival under excess of zinc and other divalent cations, and for virulence in murine macrophages and mice.
2 Materials and methods
2.1 Cloning vectors, bacterial strains, and culture conditions
PCR-amplified DNA fragments were cloned into pCR®-Blunt vector (Life Technologies, Carlsbad, CA, United States) or into pGEM®-T Easy (Promega, Madison, WI, United States). Plasmid pCVD-KanD was used to construct the recombinant plasmids employed to delete the selected genes in B. ovis PA, according to the procedure summarized below. This plasmid is unable to replicate in Brucella and confers resistance to kanamycin and sensitivity to sucrose (15). Brucella wild-type genes used to complement deletion mutants or to overexpress selected genes in several strains derived from B. ovis PA were cloned into pBBR1MCS4, which confers resistance to carbenicillin and replicates in several copies in the Brucella cytoplasm (16).
Escherichia coli JM109 competent cells were used to replicate plasmids derived from pGEM®-T Easy and pBBR1MCS4. E. coli NZY5α and E. coli CC118 were used for the replication of recombinant plasmids derived from pCR®-Blunt and pCVD-KanD, respectively. Luria-Bertani agar or broth, supplemented with 50 μg/mL of carbenicillin or kanamycin when required, was used as a culture medium for E. coli strains cultured at 37°C.
B. ovis PA was used as a parental strain for the construction of the genetically modified strains obtained in this study (Table 1). B. ovis strains were cultured at 37°C under a 5% CO2 atmosphere and in a culture medium composed of tryptic soy broth or agar (TSB or TSA; Pronadisa-Laboratorios Conda, Torrejón de Ardoz, Spain) supplemented with 0.3% yeast extract (YE; Pronadisa-Laboratorios Conda, Torrejón de Ardoz, Spain) and 5% horse serum (HS; Gibco-Life Technologies, Grand Island, NY, United States). Kanamycin, carbenicillin (50 μg/mL), or 5% sucrose was added to TSA-YE-HS or TSB-YE-HS when required for the selection or maintenance of the genetically modified strains.
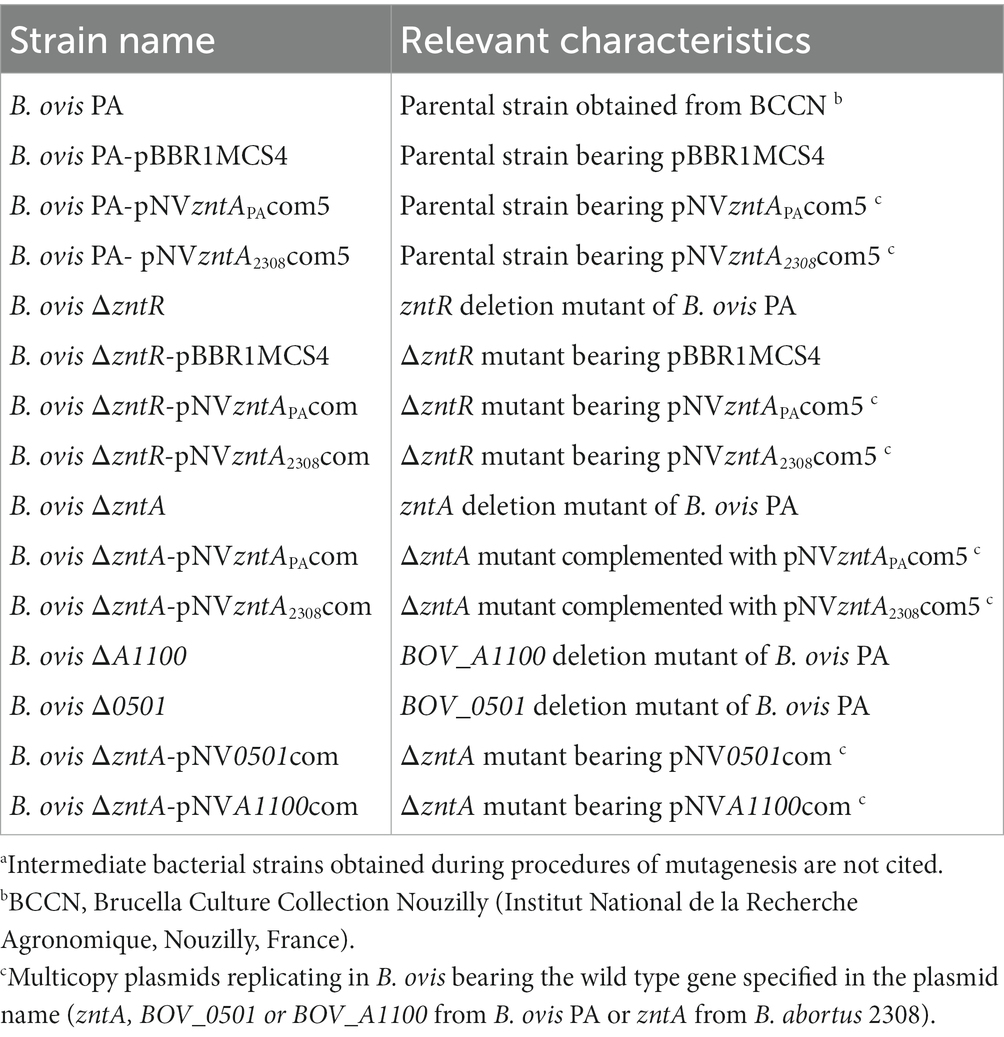
Table 1. Main Brucella ovis bacterial strains used in this studya.
Growth curves in TSB-YE-HS started with bacterial cultures of optical density at 600 nm (OD600) values of 0.05 that were incubated for 3 days at 37°C under a 5% CO2 atmosphere and agitation at 120 rpm. OD600 values were recorded at several time points. The growth curves shown in the figures are representative of three independent experiments with similar results. For evaluation of metal toxicity, 5 mM ZnCl2, 0.2 mM CdCl2, 0.5 mM CoCl2, 1 mM CuCl2, 1 mM NiCl2, 0.8 mM PbCl2, 2 mM MnCl2, 2 mM FeCl2, or 4 μM HgCl2 (Sigma-Aldrich, St. Louis, MO, United States) was added to the culture medium at the beginning of the experiment. The concentrations for each compound were previously selected (data not shown) and correspond to concentrations slightly inhibiting the growth of the parental strain.
2.2 In silico analysis of DNA and protein sequences, primers, and nucleic acid procedures
The B. ovis 63/290 (ATCC 25840) whole genome published sequence (GenBank accession numbers NC_009505 and NC_009504 for chromosomes I and II, respectively) was used to retrieve the nucleotide sequences of the target genes and to design the primers (IDT, Leuven, Belgium) used in this study (Table 2). Genes of B. abortus 2308 were also obtained from the published whole genome (GenBank accession numbers AM040264 and AM040265). The genome database of the Kyoto Encyclopedia of Genes and Genomes (KEGG) was used to identify genes potentially involved in zinc efflux in the genome of B. ovis 63/290.1 Nucleotide sequences of the genes and the amino acid sequences of the corresponding encoded proteins were obtained from the KEGG gene database2 and was also used to identify orthologs and paralogs of the identified genes.
DNA similarity searches were performed with the Basic Local Alignment Search Tool (BLAST)3 and at the Bacterial and Viral Bioinformatics Resource Center (BV-BRC).4 Pairwise sequence alignments of DNA or protein sequences were performed with EMBOSS Needle5 at the European Bioinformatics Institute. Gene Construction Kit (GCK 4.5; Textco Biosoftware, Raleigh, NC, United States) was used for the analysis of nucleotide sequences and schematic drawing of the studied loci. Protein features were retrieved from UniProt Knowledgebase (18),6 transmembrane topology was also analyzed with Phobius7 at the Stockholm Bioinformatics Center, and PSORTb v3.0.2 (Brinkman Laboratory, Simon Fraser University, British Columbia, Canada)8 was also used to predict protein subcellular localization (19).
For high-fidelity PCR reactions to be cloned into pCR®-Blunt, the NZYProof 2x Green Master Mix was used (NZYTech Lda., Lisboa, Portugal), while those to be cloned into pGEM®-T Easy were amplified with the Expand™ Long Template PCR System (Roche, Mannheim, Germany). The Red Taq DNA polymerase master mix (VWR, Leuven, Belgium) was used for verification of the B. ovis recombinant strains obtained in this study.
Relative quantification of RNA transcripts was performed by real-time reverse transcription PCR (RT-qPCR) as previously described (20). In brief, RNA was extracted, with the E.Z.N.A.® Bacterial RNA Kit (Omega Bio-tek Inc., Norcross, GA, United States), from B. ovis strains cultured for 16 h in TSB-YE-HS, supplemented with 1 mM ZnCl2 or 0.05 mM CdCl2 when desired. RNA (1 μg) treated with RNase-free DNaseI (Thermo Fisher Scientific, Vilnius, Lithuania) to remove contaminant DNA was used to synthesize cDNA with the RevertAid H minus first strand cDNA synthesis kit (Thermo Scientific, Vilnius, Lithuania). A reaction lacking retrotranscriptase was used as a control of DNA absence. The cDNAs were submitted to real-time PCR reactions in a StepOnePlus™ apparatus (Applied Biosystems, Foster City, United States) with primer pairs listed in Table 2 and Express SYBR® GreenER™ qPCR Supermix with Premixed ROX (Invitrogen, Life Technologie Corp, Carlsbad, CA, United States). Primer pairs used to evaluate the expression of each gene were as follows: 16SRT-Fw + 16SRT-Rv for 16S, 0501RT-F + 0501RT-R for BOV_0501, zntRRT-F + zntAMUT-F4 for zntR, zntART-F + zntRMUT-F2 for zntA, A1100RT-F + A1100-R for BOV_A1100, and znuART-F + znuART-R for znuA. Relative expression, considering B. ovis PA cultured in TSB-YE-HS and 16S as reference strain and gene (17), was evaluated with the 2−ΔΔCt method using the StepOne™ Software v2.3. Three independent biological samples with three technical replicates were evaluated for each gene, strain, and culture condition. Results are expressed as mean ± SD of the log2 of the relative quantity (log2RQ), and statistical analysis was performed on ΔCt values (21).
2.3 Construction of the Brucella ovis recombinant strains
The procedure followed for the construction of the non-polar in-frame deletion mutants was previously described (22). In brief, the 5′ and 3` ends of each target gene, together with 300–700 bp located upstream or downstream the gene, respectively, were amplified by PCR. The primer pairs used were as follows: 0501MUT-F + 0501OVL-R and 0510OVL-F2 + 0501MUT-R for BOV_0501, zntRMUT-F + zntROVL-R and zntROVL-F + zntRMUT-R for zntR, zntAMUT-F3 + zntAOVL-R and zntAOVL-F3 + zntAMUT-R5 for zntA, and A1100MUT-F + A1100OVL-R and A1100OVL-F + A1100MUT-F for BOV_A1100. The two PCR products obtained for each gene were fused through the overlapping region existing in primers OVL-F and OVL-R, performing an additional PCR reaction with primers MUT-F + MUT-R of each gene (Table 2). The overlapping PCR reactions removed most of the DNA sequence corresponding to each target gene while maintaining the adjacent upstream and downstream regions that allow the recombination events required to produce each in-frame deletion mutant. The resulting fragments were cloned into pCR®-Blunt or pGEM®-T Easy, their nucleotide sequence determined to exclude potential undesired mutations that occurred during PCR amplification, and then subcloned into pCVD-KanD. The resulting plasmids pNV050102, pNVzntR02, pNVzntAst02, and pNVA110002 were electroporated into parental B. ovis PA, and strains with each recombinant plasmid integrated into the chromosome through a first recombination event were selected in TSA-YE-HS-kanamycin plates. These intermediate strains contain one copy of the wild-type gene and one copy of the inactivated gene. Selection of the desired mutant strains was performed by platting the intermediate strains on TSA-YE-HS-sucrose, which favors the detection of bacterial colonies suffering a second recombination event through the regions of homology located upstream or downstream of the target gene. Differentiation between colonies reverting to the wild-type genotype and the desired mutant strains was performed with PCR reactions with a pair of primers located externally to both sides of the region involved in recombination (smaller size in the mutant strain) and with primers MUT-F of each gene and a primer annealing inside each target gene (no amplification with the mutant strain).
To clone each entire gene into pBBR1MCS4, PCR amplification was performed with primers comF+comR (Table 2), and the obtained fragments were first cloned into pCR®-Blunt. After verification of the nucleotide sequence, each gene was excised by digestion with appropriate restriction enzymes and subcloned into pBBR1MCS4 digested with the same enzymes. The resulting plasmids pNV0501com, pNVzntAPAcom5, pNVzntA2308com5, and pNVA1100com were electroporated either into parental B. ovis PA or into selected mutant strains (Table 1), and the recombinant strains selected and maintained in culture medium containing carbenicillin.
2.4 Protein techniques
Protein profiles of whole-cell bacterial lysates were obtained by sodium dodecyl sulfate-polyacrylamide gel electrophoresis (SDS-PAGE), followed by Coomassie blue staining (23). Proteins were separated in 14% acrylamide/bisacrylamide gels using a Protean II xi cell (Bio-Rad, Hercules, CA, United States) and pre-stained protein marker VI (Applichem-Panreac, Barcelona, Spain) as a protein standard.
The proteomic analysis of SDS-PAGE protein bands was performed in the proteomics facility of Centro de Investigación del Cáncer, Salamanca, Spain, following its standardized procedures. In brief, selected protein bands were excised from the gels and trypsin-digested proteins were submitted to reversed-phase LC–MS/MS using a nano-UHPLC system (NanoElute, Bruker Daltonics, Germany) coupled to a hybrid trapped ion mobility-quadrupole time-of-flight mass spectrometer Tims TOF Pro (Bruker Daltonics, Germany) via a modified nano-electrospray ion source (Captive Spray, Bruker Daltonics, Germany). Protein identification was done by searching the MS/MS spectra against the Brucella ovis Uniprot proteome database with the Andromeda algorithm (24) and the MAXQUANT (25). Protein relative abundance was compared using the iBAQ score (26).
2.5 Evaluation of virulence in macrophages and mice
Internalization and intracellular replication of the mutant strains in phagocytic cells were evaluated in J774A.1 murine macrophage as previously described (27). In brief, macrophages fixed on the surface of 96-well sterile plates were infected with each B. ovis strain at a multiplicity of infection of 200 CFU/macrophage. After a period of incubation of 2 h, gentamycin-containing medium (50 μg/mL) was added to the wells to kill extracellular bacteria. Intracellular bacteria were determined in three wells per strain after the lysis of macrophages by plating serial dilutions of the well content on TSA-YE-HS (t0). Intracellular bacteria were also determined in three wells per strain after 24 and 48 h of incubation (t24 and t48) in culture medium containing 20 μg/mL gentamycin. The results were expressed as means ± SD (n = 3) of the log10 CFU/well values at each time point.
Virulence in the mouse model was evaluated as previously described (28). In brief, 6-week-old BALB/c mice (Charles River Laboratories, Chatillon-sur- Chalaronne, France) were intraperitoneally infected with 106 CFU of each bacterial strain. Splenic bacterium accounts were determined in five mice per group at 3 and 7 weeks post-infection (p.i.), which in B. ovis PA correspond to the peak of infection in the acute phase and chronic phase of infection (29). Results were expressed as means ± SD (n = 5) of log10 CFU/spleen values at each time point. The mouse experiments were designed according to the Spanish and European legislation for research with animals (RD 53/2013 and directive 2010/63/EU).
2.6 Statistical analysis
Statistical comparisons were performed on GraphPad Prism Software (GraphPad Software Inc., San Diego, CA, United States) with one-way ANOVA, followed by Tukey’s test or a two-tailed t-test for multiple or single comparisons, respectively. Statistically significant differences (p ≤ 0.05) were established with a 95% confidence interval.
3 Results
3.1 Gene and protein sequences of the ZntA-ZntR zinc efflux system of Brucella ovis PA and other predicted zinc exporters revealed differences between Brucella ovis and Brucella abortus
Using the KEGG gene and genome databases, a search for genes potentially related to zinc export was performed in the genome of B. ovis 63/290 (ATCC 25840) reference strain. The search confirmed that the zntR-zntA locus, involved in Zn2+ export and virulence in B. abortus 2308 (14), was also present in B. ovis, being ZntA defined as a Zn2+/Cd2+-exporting ATPase. In addition, two other genes potentially encoding proteins that might be related to zinc export were identified in the genome of B. ovis 63/290 reference strain: (i) the product of gene BOV_0501, defined as cobalt-zinc-cadmium efflux system protein, and (ii) a ZntA paralog described as a cadmium-translocating P-type ATPase encoded by BOV_A1100.
BOV_0501 is predicted to encode a cytoplasmic membrane protein of 297 amino acids containing six transmembrane (TM) domains, lacking signal peptide, and belonging to the cation diffusion facilitator (CDF) transporter family (Figure 1A). BOV_A1100 is predicted to encode a P-type ATPase of 646 amino acids located at the cytoplasmic membrane, with seven TM domains and lacking signal peptide (Figure 1B). Both proteins exhibit characteristic motifs of CDF and P1b-type ATPase exporters, respectively, which are marked in Figure 1. DNA sequencing of BOV_0501 and BOV_A1100 loci in B. ovis PA, used as parental strain in this study, showed that both genes were identical to those of the B. ovis reference strain (data not shown). On the contrary, BOV_0501 and BOV_A1100 orthologs are annotated as pseudogenes in B. abortus 2308, despite the fact they share 98 and 99.7% of identity with the respective B. ovis genes. The reasons for these annotations are a frameshift mutation close to the 5′-end of the BOV_0501 ortholog (BAB1_0523) due to a 17-bp deletion in the B. abortus 2308 genome and one nucleotide substitution in the BOV_A1100 ortholog (BAB2_1160) resulting in a premature translation stop codon that truncates the B. abortus 2308 protein at amino acid 275 of 646 total amino acids (Supplementary Figure S1). The 17-bp deleted region in the BOV_0501 ortholog of B. abortus 2308 is flanked in B. ovis by direct repeats of 6 bp that could be involved in the deletion process by a slipped mispairing mechanism (49) (Supplementary Figure S1). However, it must be noted that a potential ATG translation start codon, from which a protein of 285 amino acids could be synthesized, and is present at the BAB1_0523 locus (Supplementary Figure S1). Therefore, the possibility of a functional BAB1_0523 protein in B. abortus 2308 cannot be discarded.
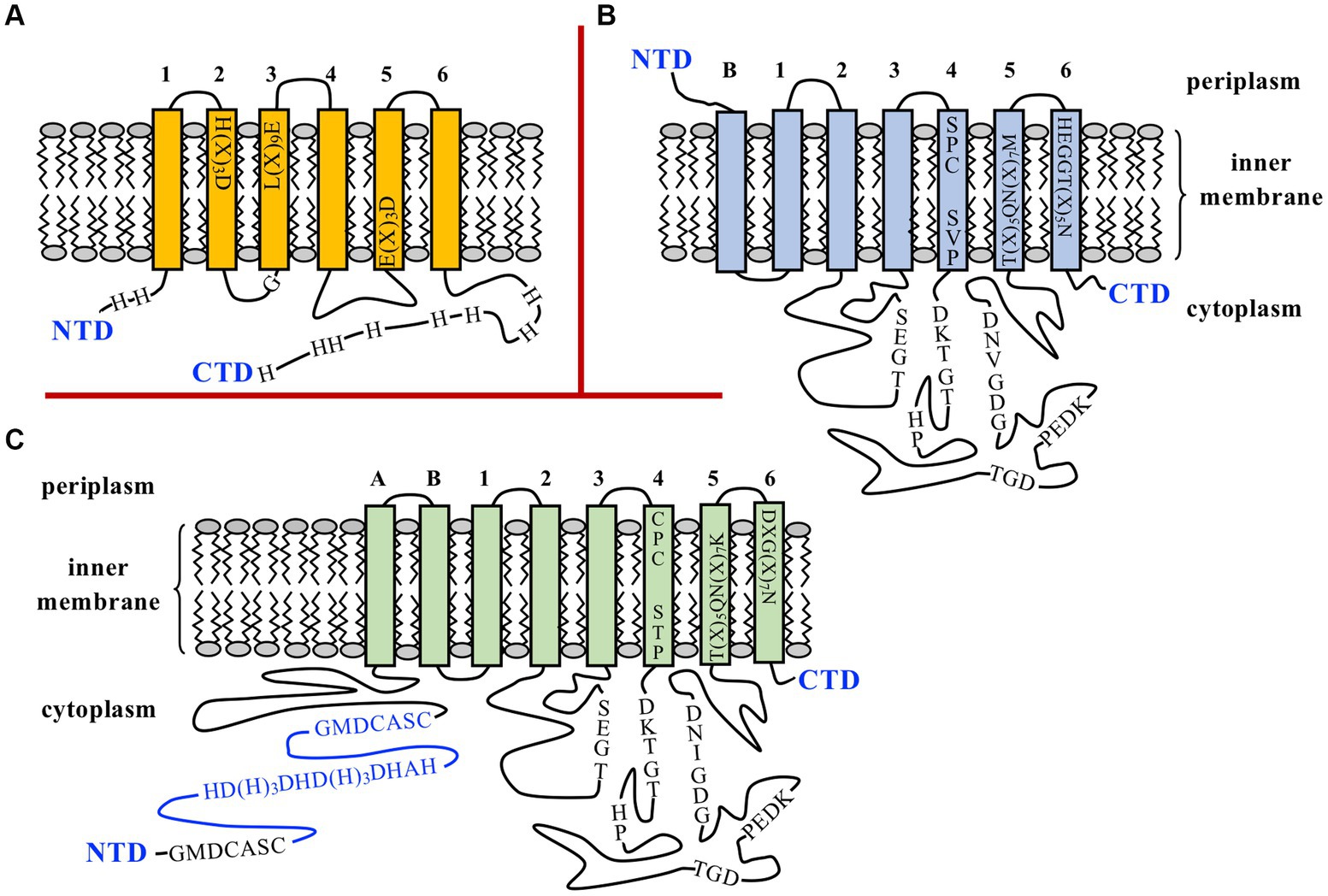
Figure 1. Schematic representation of BOV_0501 (A) and BOV_A1100 (B) inner membrane proteins of Brucella ovis PA and the ZntA Zn2+ exporter of B. abortus 2308 (C). N- and C-terminal domains (NTD and CTD) are shown, and the predicted transmembrane domains (TM) are numbered. In BOV_A1100 and ZntA, the 6 TM domains that are found in all P1B-ATPases are numbered 1 to 6, while the N-terminal TM regions that can be present or not depending on the subgroup of P1B-ATPases are identified as A or B (30). In panel (A) the transmembrane motifs, the Gly residue adjacent to the cytoplasmic side of TM3, and the His residues of the NTD and CTD of BOV_0501, usually found in metal exporters of the cation diffusion facilitator (CDF) family (31–33), are shown. In panels (B,C) the characteristic motifs conserved in the actuator and nucleotide-binding domains of P1B-ATPases are shown in the long cytoplasmic domains connecting TM2 with TM3 and TM4 with TM5 (30, 34–36). Motifs located within TM4-TM6 and that are related to the metal specificity-selectivity of the transporter are also represented (30, 37–39). In panel (C) the two GXXCXXC motifs and the His-rich region, defined as metal binding motifs (40–44), are marked in the cytoplasmic NTD of B. abortus 2308 ZntA, and the region of the NTD that is absent in the B. ovis ZntA is drawn in blue (see also Figure 2).
Regarding the zntR-zntA locus (BOV_1941 and BOV_1942, respectively), zntR is predicted to encode a transcriptional regulator of 140 amino acids belonging to the MerR family and with cytoplasmic localization. Minor differences between B. ovis PA and B. abortus 2308 were detected in zntR (99.8% identity with only one nt substitution that does not modify the encoded protein, data not shown) or in the intergenic zntR-zntA region (2 nt substitutions) that contains inverted repeats of 9 nt that could be involved in ZntR binding (46) (Figure 2B). The ZntA proteins of B. abortus 2308 and B. ovis PA are predicted to be P1B-type ATPases, lacking signal peptide, and located at the cytoplasmic membrane with 8 TM domains (Figure 1C). However, relevant differences between both strains were identified in zntA since they only share 86.9% identity, and an in-frame deletion of 300 bp was evidenced in the B. ovis PA gene (Figure 2). In the zntA gene of B. abortus 2308 (BAB1_2019), two direct repeats of 20 bp flank the fragment absent in B. ovis PA (Figure 2B), which suggests that the B. ovis PA deletion probably occurred through a slipped mispairing mechanism involving both repeats (49). Although it is an in-frame deletion, 100 amino acids are lost in the cytoplasmic N-terminal domain (NTD) of B. ovis PA ZntA, which could have an important effect on the activity of the protein (Figures 1C, 2A,C). One GXXCXXC motif, reported as Zn2+ binding domain in other bacteria (40–43), and a histidine-rich domain also described as a potential target for Zn binding (41–44) are found in the ZntA region of B. abortus 2308 (Figure 2B) that is absent in B. ovis PA. However, an additional GXXCXXC motif and several other motifs located in the last three TM domains that have been reported as involved in the metal translocation pathway of P1B-ATPases (34, 41) are conserved in both B. ovis PA and B. abortus 2308 ZntA (Figures 1C, 2B). Six additional single nt differences (accounting for four amino acid substitutions) were detected in zntA, but they did not result in any translational stop codon.
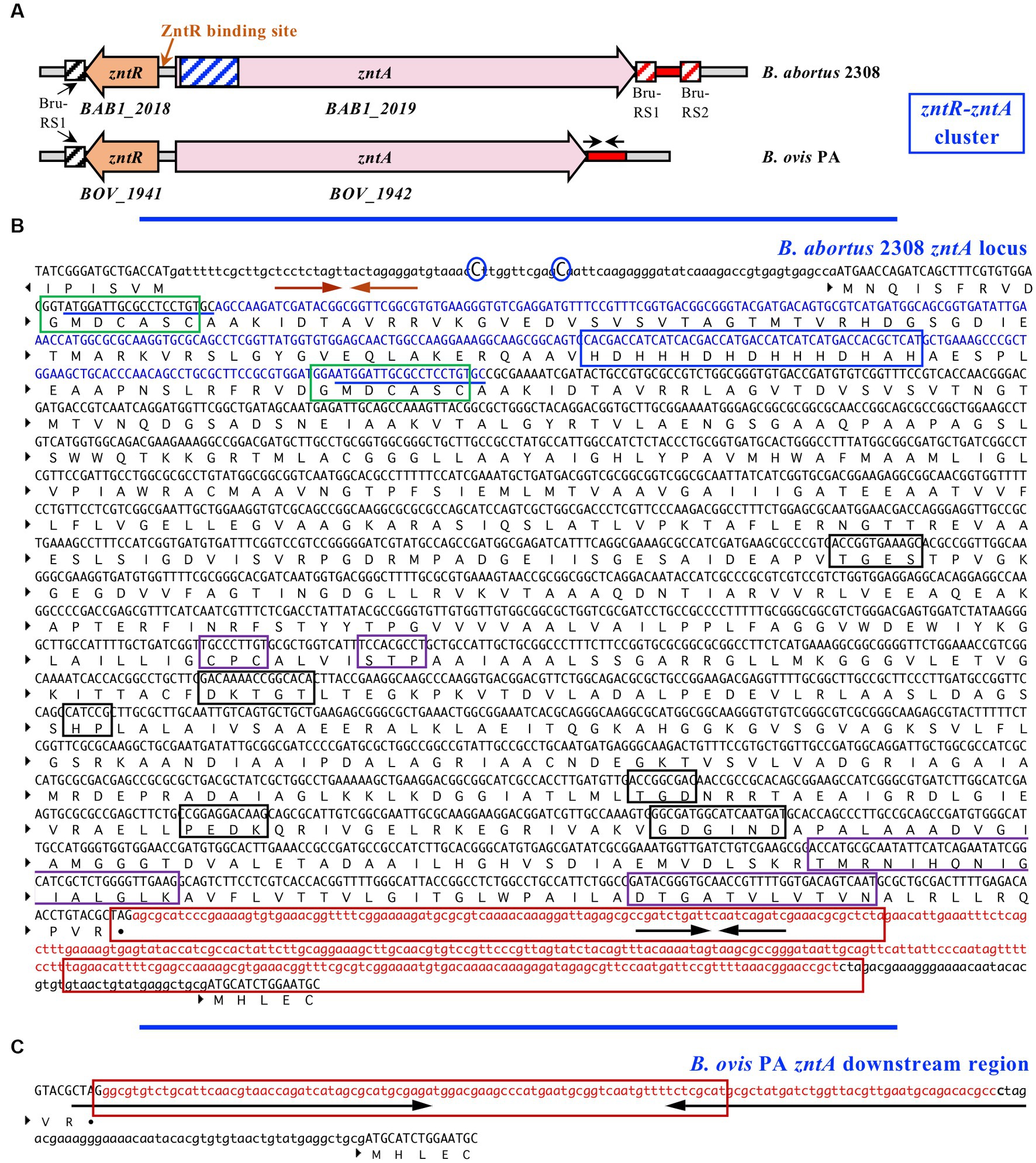
Figure 2. Genetic organization of the ZntR-ZntA zinc export system in B. ovis PA and B. abortus 2308 (A); the nucleotide sequence of B. abortus 2308 zntA and flanking regions (B); and zntA downstream region of B. ovis PA (C). In panel (A) the predicted DNA binding site of the ZntR transcriptional regulator is marked, the 300 nt region of B. abortus 2308 zntA absent in B. ovis PA is represented in blue, the zntA downstream region differing between B. ovis PA and B. abortus 2308 is represented in red, and location of the Bru-RS1 and Bru-RS2 Brucella repetitive elements (45) is represented with boxes. In panel (B) the direct repeats probably involved in the deletion process of the 300 nt absent in zntA of B. ovis PA (blue region) are underlined. The GXXCXXC and His-rich motifs of ZntA, which could be involved in Zn binding (40–44), are framed in green and blue, respectively. Characteristic motifs located within the cytoplasmic domains of P1B-type ATPases (30, 34–36) are framed in black, and those identified in the transmembrane domains of P1B-2 ATPases are framed in purple (30, 37–39). The inverted repeats that could be involved in ZntR binding (46) and the two nt differences (C → T) between B. abortus 2308 and B. ovis PA located in the zntR-zntA intergenic region are marked with orange arrows and blue circles, respectively. Bru-RS1 and Bru-RS2 elements (45) downstream zntA in B. abortus 2308 are framed in red. Black arrows mark the inverted repeats detected downstream zntA in B. abortus 2308 (10 nt) and B. ovis PA (48 nt), which are located inside regions differing in sequence between both strains (red sequences). In panel (C) the 84 nt of the zntA downstream region identical to the 3′-end of IS711 (47, 48) is framed.
Moreover, the intergenic region between zntA and downstream BOV_1943 (BAB1_2020 in B. abortus 2308) completely differed between B. ovis and B. abortus, being constituted in B. ovis PA by long inverted repeats of 48 bp spaced by 31 bp (Figures 2B,C). This region is specific for B. ovis strains since, according to similarity searches performed at BV-BRC, the whole sequence is not found in other Brucella species. Although the mechanism underlying these differences is not evident, 84 bp of this B. ovis PA intergenic sequence (Figure 2C) shows 100% identity with the 3′-end of the insertion sequence IS711, which is more frequently repeated in B. ovis than in B. abortus (47, 48), while the corresponding region in B. abortus 2308 contains one copy of Bru-RS1 and one copy of Bru-RS2 of 103 and 106 bp, respectively, spaced by 155 bp (Figures 2A,B). Bru-RS1 and Bru-RS2 are two related repetitive palindromic elements with similar occurrence among the Brucella species (45). Accordingly, recombination events involving IS711, Bru-RS1, and/or Bru-RS2 could be responsible for these differences. It must be noted that one copy of Bru-RS1 is also present in both B. abortus 2308 and B. ovis preceding the zntR-zntA locus (Figure 2A).
3.2 ZntA is an efficient Zn2+ exporter in Brucella ovis PA and ZntR is required for maximum zntA expression in response to toxic zinc levels
Considering the reported activity of ZntA as a Zn2+ exporter in other bacteria, including B. abortus 2308 (14), and to evaluate whether the remarkable differences of the B. ovis PA ZntA protein described above could abolish the biological activity of the protein, B. ovis PA strains bearing wild-type zntA of B. ovis PA or B. abortus cloned in pBBR1MCS4 were constructed. Surprisingly, when the B. ovis PA strains were incubated in the presence of 5 mM ZnCl2 (a concentration slightly inhibiting growth of the parental strain), overexpression of zntA in B. ovis PA-pNVzntAPAcom5 or B. ovis PA-pNVzntA2308com5, confirmed by RT-qPCR, did not restore the growth pattern obtained in normal medium (Figures 3A,C). Remarkably, when compared to the parental strain and B. ovis PA-pBBR1MCS4, both strains showed in normal medium an overrepresented protein band in SDS-PAGE that, according to LC–MS/MS analysis, does not correspond to the ZntA exporter but to ZnuA (Figure 3B), a periplasmic zinc-binding protein involved in zinc import in B. abortus 2308 (14, 50). These results are in accordance with znuA overexpression detected by RT-qPCR in both strains when compared to B. ovis PA-pBBR1MCS4 (Figure 3C). According to SDS-PAGE results, ZnuA appeared to be more abundant in B. ovis PA-pNVzntA2308com5 than in B. ovis PA-pNVzntAPAcom5 (Figure 3B), which was also in agreement with RT-qPCR results that showed that although both strains exhibited similar levels of zntA overtranscription (log2 RQ mean values of 2.4 and 2.2, respectively), znuA transcripts were more abundant (p ≤ 0.01) in B. ovis PA-pNVzntA2308com5 (log2 RQ mean value of 6.6) than in B. ovis PA-pNVzntAPAcom5 (log2 RQ mean value of 4.4; Figure 3C). On the contrary, both strains exhibited slightly reduced levels of zntR transcripts (p ≤ 0.05) when compared to B. ovis PA-pBBR1MCS4 (Figure 3C).
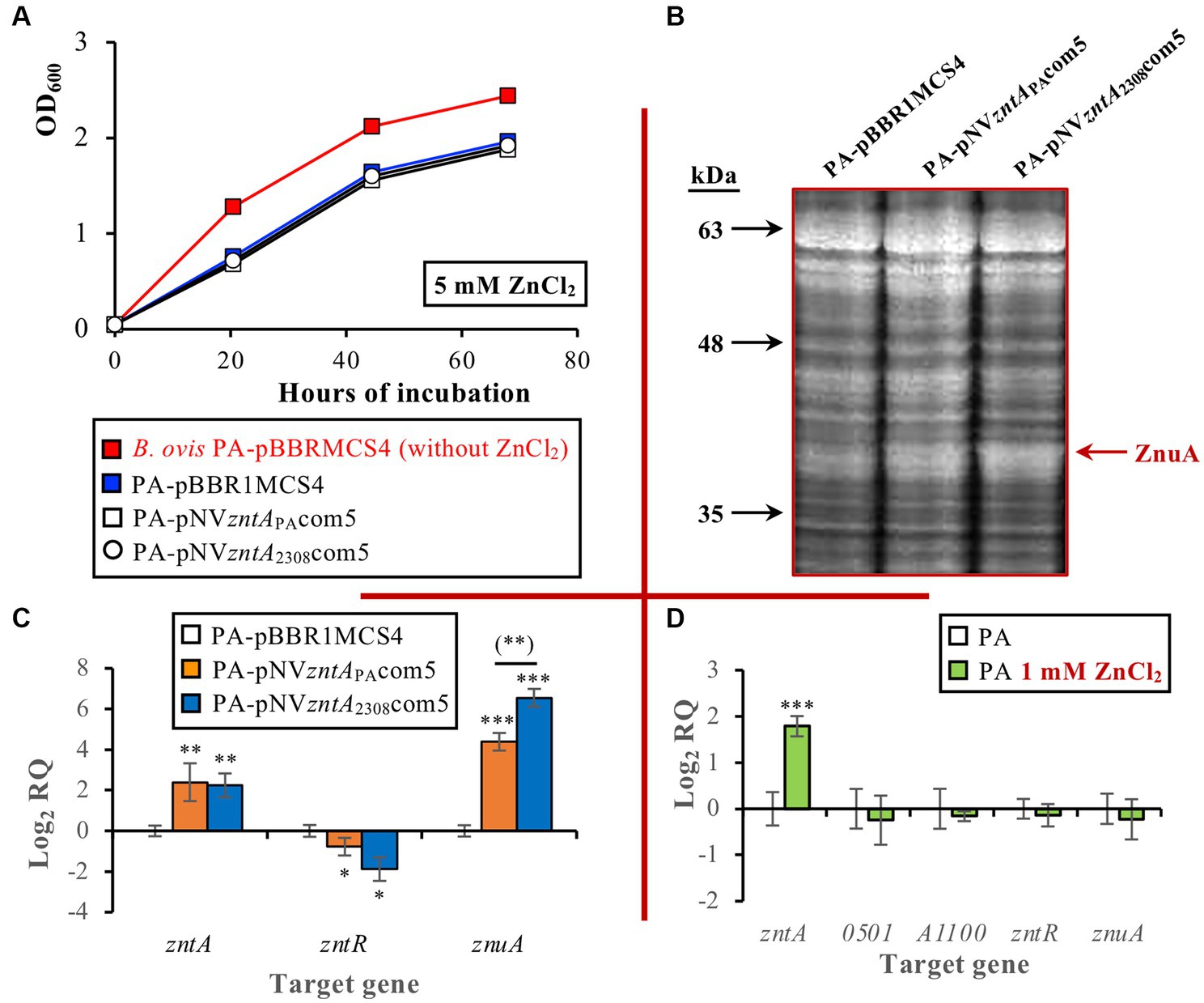
Figure 3. Growth curves of parental B. ovis PA transformed with empty pBBR1MCS4 or with pNVzntAPAcom5 or pNVzntA2308com5 in the presence of 5 mM ZnCl2 (A), proteins of the same strains separated by SDS-PAGE and stained with Coomassie blue (B), and gene expression in the three strains grown in normal medium (C), and of B. ovis PA cultured in the presence or absence of 1 mM ZnCl2 (D). In panel (A) all strains exhibited the same behavior in the normal medium as that shown in red for B. ovis PA-pBBR1MCS4, which was also same as that of parental B. ovis PA (data not shown). In panel (C) statistically significant differences (p ≤ 0.05) when compared to B. ovis PA-pBBR1MCS4 strain are marked with asterisks, and differences between B. ovis PA-pNVzntAPAcom5 and B. ovis PA-pNVzntA2308com5 are marked with asterisks in brackets. In panel (D) statistically significant differences (p ≤ 0.05) when compared to B. ovis PA cultured in the absence of 1 mM ZnCl2 are marked with asterisks. *p ≤ 0.05; **p ≤ 0.01; ***p ≤ 0.001.
An RT-qPCR assay was performed with parental B. ovis PA cultured with or without 1 mM ZnCl2 to determine the effect of exposure to toxic levels of zinc on the expression of genes related to zinc export and znuA. Exposure to 1 mM ZnCl2 increased about 3.5 times the level of transcripts of zntA (log2 RQ mean value of 1.79; p ≤ 0.001) when compared to B. ovis PA cultured in a normal medium, while no statistically significant differences were found in expression of znuA, zntR, BOV_0501, or BOV_A1100 (Figure 3D).
To verify the biological activity of the B. ovis PA ZntA protein, the construction of the zntA deletion mutant was attempted in this strain. The process was challenging, probably due to the long-inverted repeats located downstream of the gene that presumably hamper the required recombination event. The B. ovis PA ΔzntA mutant could only be obtained when the inverted repeats were included as part of the zntA deleted region in the pNVzntAst02 plasmid used to obtain the mutant. The mutant strain was subsequently complemented in trans with either pNVzntAPAcom5 or pNVzntA2308com5. The B. ovis PA ΔzntR mutant was also constructed and subsequently transformed with pBBR1MCS4, pNVzntAPAcom5, and pNVzntA2308com5.
The absence of ZntR or ZntA did not affect the growth of B. ovis PA in a normal medium (data not shown; the ΔzntR and the ΔzntA mutants behaved as parental B. ovis PA in Figure 4A). However, in the presence of 5 mM ZnCl2, the ΔzntR mutant showed a defective growth while the ΔzntA mutant was unable to grow (Figure 4A). Both mutants recovered the parental phenotype after transformation with pNVzntAPAcom5 or pNVzntA2308com5 (Figure 4A), which suggests that growth defects of the ΔzntR mutant under toxic ZnCl2 concentrations are due to underexpression of ZntA. In fact, transcripts for zntA in the ΔzntR mutant were in the order of 30-fold lower (mean log2 RQ value of −4,86) than those detected in the parental strain (p ≤ 0.001), while no statistically significant differences were observed between both strains regarding znuA expression (Figure 4D). The low levels of zntA transcripts in the ΔzntR mutant are in accordance with both its increased sensitivity to ZnCl2 and the recovery of the parental phenotype in strains ΔzntR-pNVzntAPAcom5 and ΔzntR-pNVzntA2308com5 (Figures 4A,D). Therefore, although the low transcription level of zntA in the absence of ZntR allows relevant levels of Zn2+ detoxification in the ΔzntR mutant (Figure 4A), the presence of ZntR is required in B. ovis PA to obtain maximum ZntA levels in response to exposure to toxic concentrations of ZnCl2 (Figures 4A,D). When compared to the parental strain, transcription of BOV_0501 and BOV_A1100 was not increased in the ΔzntR and ΔzntA mutants or in the ΔzntA mutant cultured in the presence of ZnCl2 (data not shown).
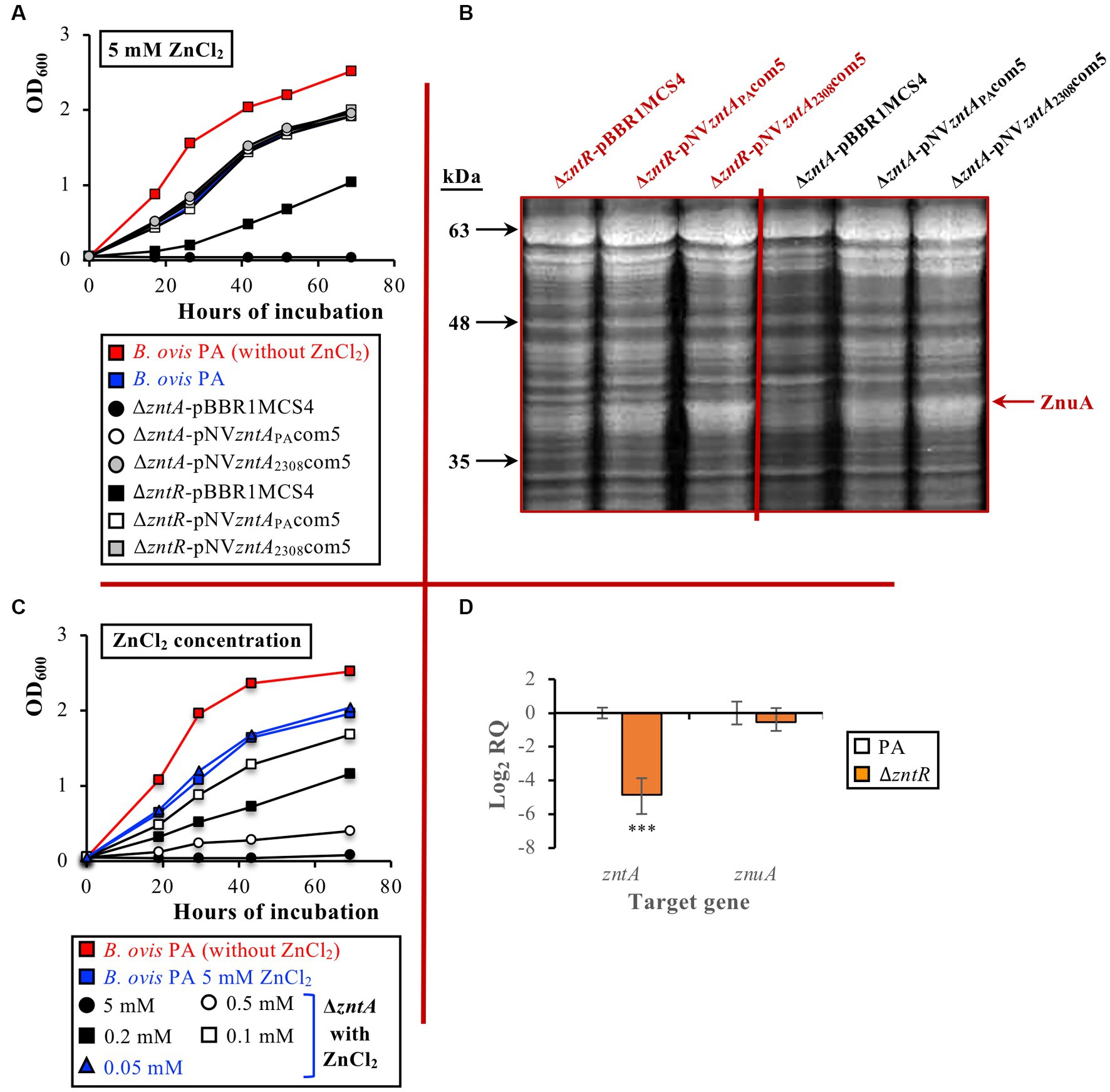
Figure 4. Growth curves of parental B. ovis PA and the ΔzntR and ΔzntA isogenic mutants transformed with empty pBBR1MCS4 or with pNVzntAPAcom5 or pNVzntA2308com5 in the presence of 5 mM ZnCl2 (A), proteins of the same strains separated by SDS-PAGE and stained with Coomassie blue (B), growth curves of the ΔzntA mutant in the presence of several ZnCl2 concentrations (C), and gene expression in the ΔzntR mutant grown in normal medium (D). In panel (A) all strains exhibited the same behavior in the normal medium as that shown in red for B. ovis PA (data not shown). In panel (C) blue curves correspond to ZnCl2 concentrations, giving an equivalent growth pattern in parental B. ovis PA and its isogenic ΔzntA mutant. In panel (D) statistically significant differences (p ≤ 0.05) when compared to B. ovis PA are marked with asterisks. ***p ≤ 0.001.
As described above for B. ovis PA-pNVzntAPAcom5 or B. ovis PA-pNVzntA2308com5 (Figure 4B), complementation in trans of the ΔzntA mutant with pNVzntAPAcom5 or pNVzntA2308com5 led to an increase of the ZnuA periplasmic protein related to zinc import that was perceptible in SDS-PAGE (Figure 4B). Similar results were obtained with the ΔzntR mutant transformed with the same plasmids (Figure 4B).
Since the ΔzntA mutant was unable to grow in the presence of 5 mM ZnCl2, several ZnCl2 concentrations were assayed to determine its degree of sensitivity to this compound in comparison to that of the parental strain. The ΔzntA mutant only recovered a growth pattern equivalent to that obtained with the parental strain incubated with 5 mM ZnCl2 when a ZnCl2 concentration 100 times lower (0.05 mM) was used (Figure 4C). With the ΔzntR mutant, which was more resistant to ZnCl2 toxicity than the ΔzntA mutant (Figure 4A), the parental phenotype was recovered with a ZnCl2 concentration 5 times lower (1 mM; Supplementary Figure S2).
3.3 ZntA is an efficient Cd2+ exporter in Brucella ovis PA also contributing to Co2+, Cu2+, and Ni2+ detoxification
ZntA orthologs in several bacteria have been described as relevant exporters for other toxic divalent cations (mainly Cd2+ but also Pb2+ and Co2+) (40, 46). Accordingly, and since this aspect has not been evaluated in any other pathogenic brucellae, the role of ZntA in resistance to Cd2+, Co2+, Pb2+, Hg2+, Mn2+, Fe2+, Cu2+, and Ni2+ was evaluated in B. ovis PA. Incubation with 0.2 mM CdCl2, a concentration slightly affecting the growth of the parental strain, impaired the growth of the ΔzntR mutant and prevented the multiplication of the ΔzntA mutant (Figure 5A). Overexpression of wild-type ZntA from B. ovis PA or B. abortus 2308 in both mutants restored the parental phenotype (Figure 5A), which reveals the role of the ZntA protein of both B. ovis PA and B. abortus 2308 as a powerful Cd2+ exporter. The high efficiency of ZntA in CdCl2 detoxification was demonstrated when growth under several CdCl2 concentrations was evaluated since parental B. ovis PA tolerated a CdCl2 concentration 2000 times higher than that supported by the isogenic ΔzntA mutant (Figure 5B). The presence of ZntR also contributed to Cd2+ detoxification mediated by ZntA, but tolerance of the ΔzntR mutant to CdCl2 was only twice lower than that of the parental strain (Supplementary Figure S2). Despite this demonstrated role of ZntA as a cadmium exporter, only a discrete increase of zntA transcription, accompanied by increased levels of znuA transcripts, was observed in B. ovis PA incubated with CdCl2 (p ≤ 0.01). The expression of BOV_A1100 and BOV_0501 in the presence of CdCl2 was similar to that observed with the parental strain (Figure 5C).
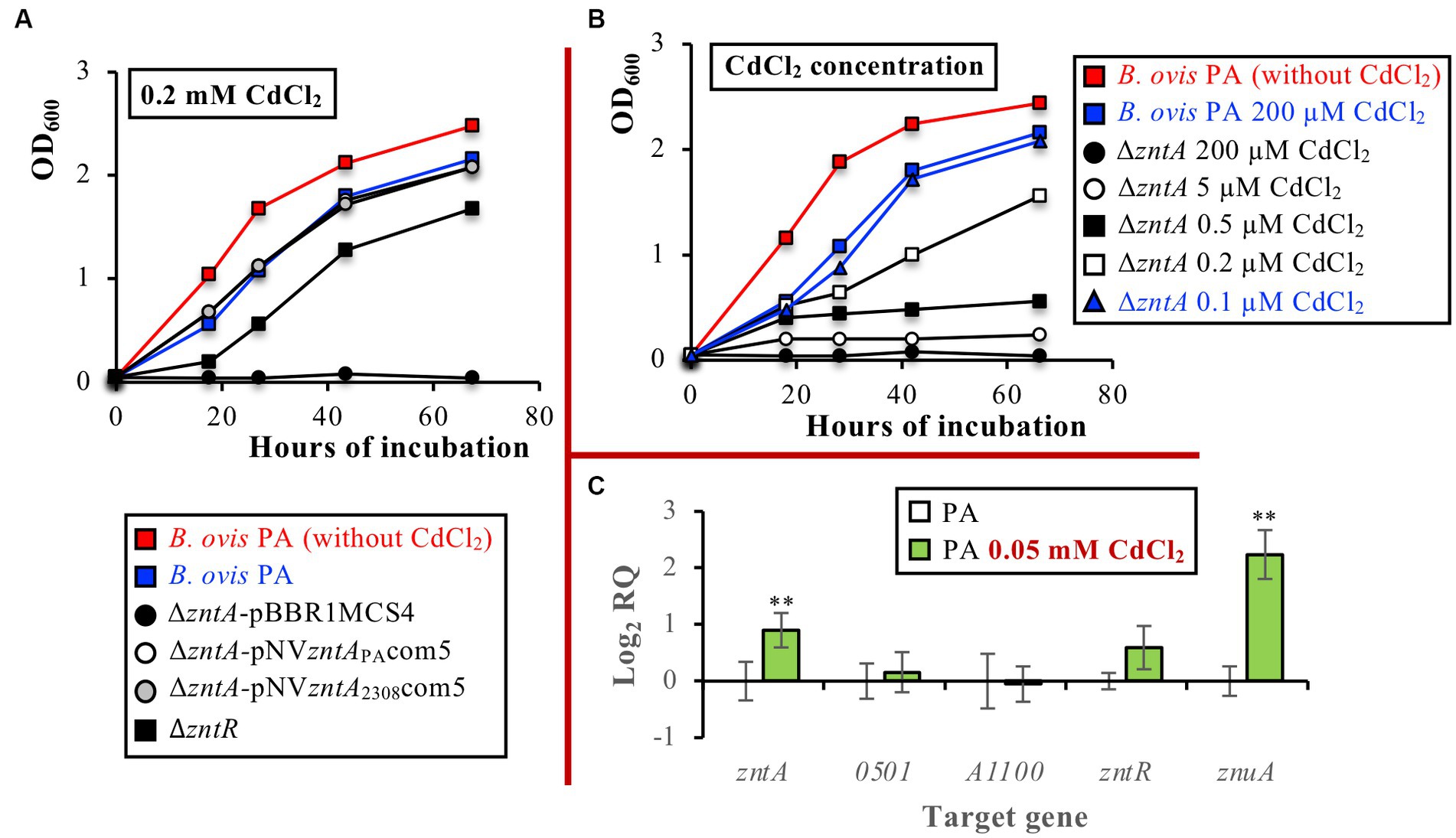
Figure 5. Growth curves of parental B. ovis PA, the ΔzntR mutant, and the ΔzntA mutant transformed with empty pBBR1MCS4 or with pNVzntAPAcom5 or pNVzntA2308com5 in the presence of 0.2 mM CdCl2 (A), growth curves of the ΔzntA mutant in the presence of several CdCl2 concentrations (B), and gene expression in parental B. ovis PA cultured in the presence or absence of 0.05 mM CdCl2 (C). In panel (A) all strains exhibited the same behavior in the normal medium as that shown in red for B. ovis PA (data not shown). In panel (B) blue curves correspond to CdCl2 concentrations, giving an equivalent growth pattern in parental B. ovis PA and its isogenic ΔzntA mutant. In panel (C) statistically significant differences (p ≤ 0.05) when compared to B. ovis PA cultured in the absence of 0.05 mM CdCl2 are marked with asterisks. **p ≤ 0.01.
The B. ovis PA ΔzntA mutant, but not the complemented strains or the ΔzntR mutant, also showed increased sensitivity to 0.5 mM CoCl2, 1 mM CuCl2, and 1 mM NiCl2 (Figure 6), while the absence of ZntA did not modify susceptibility to 0.8 mM PbCl2, 2 mM MnCl2, 2 mM FeCl2, or 4 μM HgCl2 (data not shown). Remarkably, growth with CoCl2 0.5 mM of the ΔzntA-pNVzntAPAcom5 and ΔzntA-pNVzntA2308com5 complemented strains was even better than that obtained with B. ovis PA, while with ZnCl2, CdCl2, CuCl2, and NiCl2, the three strains showed the same growth pattern (Figures 4–6).
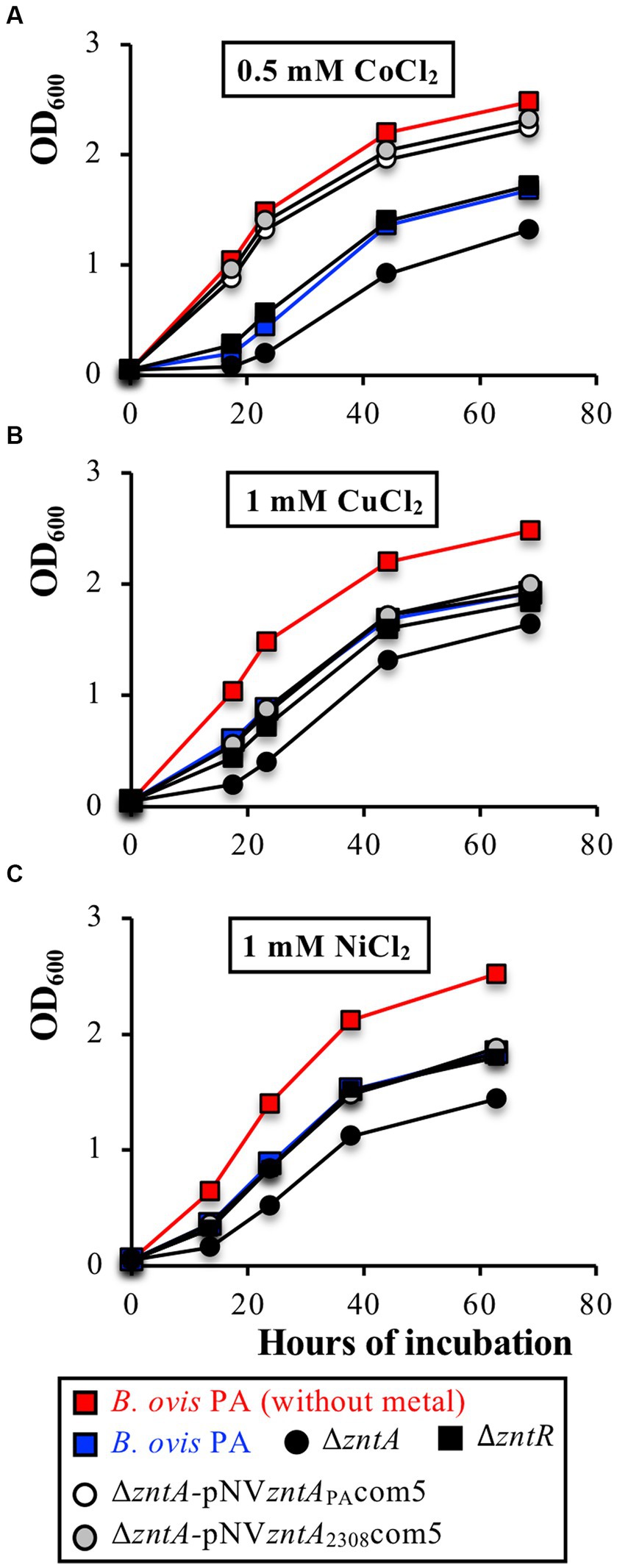
Figure 6. Role of the ZntR-ZntA export system in the survival of B. ovis PA exposed to high concentrations of CoCl2 (A), CuCl2 (B), or NiCl2 (C). The growth curves of ΔzntA-pNVzntAPAcom5 and ΔzntA-pNVzntA2308com5 in each panel are similar. All strains gave the same growth profile in normal medium and are not represented in the figure (see growth of B. ovis PA in red lanes as representative curve). Similarly, B. ovis PA, B. ovis ΔzntR, and B. ovis ΔzntA transformed with non-recombinant pBBR1MCS4 behaved as the respective parental strains and are not shown in the figure. In panel (A) parental B. ovis PA transformed with pNVzntAPAcom5 or pNVzntA2308com5 also exhibited a growth pattern in the presence of 0.5 mM CoCl2 close to that of the parental strain in normal medium (data not shown).
3.4 BOV_0501 and BOV_A1100 have Zn2+ and Co2+ exporter activity, respectively, that is not required for Zn2+ or Co2+ detoxification in Brucella ovis PA when ZntA is present
Deletion mutants for BOV_0501 and BOV_A1100 were also constructed, and their growth was evaluated in the presence of 5 mM ZnCl2, 0.2 mM CdCl2, 0.5 mM CoCl2, 1 mM CuCl2, 1 mM NiCl2, 0.8 mM PbCl2, 2 mM MnCl2, 2 mM FeCl2, or 4 μM HgCl2. Both mutants always behaved as the parental strain and, therefore, no relevance for the detoxification of any of these compounds could be demonstrated for BOV_0501 and BOV_A1100 proteins under the tested conditions (data not shown).
Since both genes are predicted to encode Zn2+ and/or Cd2+ exporters and considering the high efficiency of ZntA as an exporter of both cations in B. ovis PA, recombinant plasmids bearing wild-type BOV_0501 or BOV_A1100 (pNV0501com and pNVA1100com) were introduced into the ΔzntA mutant to evaluate whether overexpression of these genes in strains B. ovis ΔzntA-pNV0501com and B. ovis ΔzntA-pNVA1100com (Table 1) could increase the resistance of the ΔzntA mutant to Zn2+, Cd2+, and/or the other divalent cations analyzed in this study. Strain B. ovis ΔzntA-pNV0501com showed a better growth pattern in the presence of ZnCl2 (Figure 7A), but not in the presence of the other metals (data not shown), than that observed with the ΔzntA mutant. Overexpression of BOV_A1100 in B. ovis ΔzntA-pNVA1100com led to increased resistance of the ΔzntA mutant to Co2+ (Figure 7B) but not to Zn2+ or the other divalent cations tested (data not shown).
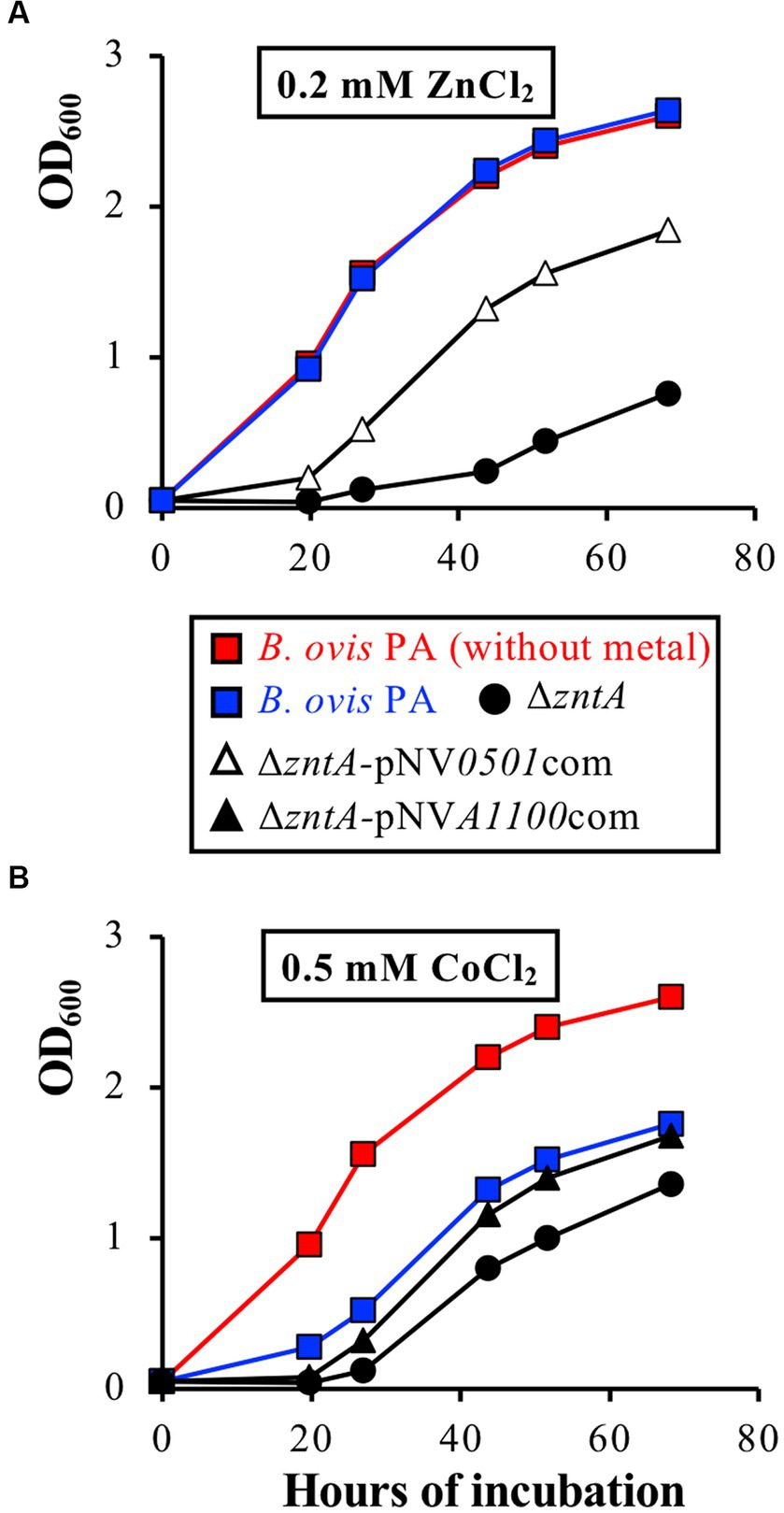
Figure 7. The ability of overexpression of BOV_0501 to restore the growth defects of the B. ovis PA ΔzntA mutant in the presence of ZnCl2 0.2 mM (A) or of overexpression of BOV_A1100 to restore the growth defects of the B. ovis PA ΔzntA mutant in the presence of 0.5 mM CoCl2 (B). All strains gave the same growth profile in normal medium, including strains transformed with empty pBBR1MCS4, and are not represented in the figure (see growth of B. ovis PA in red lanes as representative curve).
Accordingly, although BOV_0501 and BOV_A1100 show Zn2+ and Co2+ efflux activity, respectively, their role as exporters in B. ovis PA does not seem relevant, at least when ZntA is present. It must be noted that transcription of both genes remained low in the parental strain incubated in normal medium or in the presence of 1 mM ZnCl2 or 0.05 mM CdCl2, as also occurred in the ΔzntA and ΔzntR mutants (data not shown).
3.5 ZntR, ZntA, BOV_0501, and BOV_A1100 are not required for the virulence of Brucella ovis PA in macrophages or mice
The internalization and intracellular behavior of the deletion mutants in phagocytic cells was evaluated in J774A.1 murine macrophages. No differences were observed between the deletion mutants and the parental strain regarding internalization or intracellular replication. All strains showed intracellular numbers of about 5 log10 units at t0, a reduction of CFU/well in the order of 1 log10 unit 20 h after internalization, and intracellular replication thereafter to reach intracellular count levels at t44 that were close to those observed at t0 (Figure 8A).
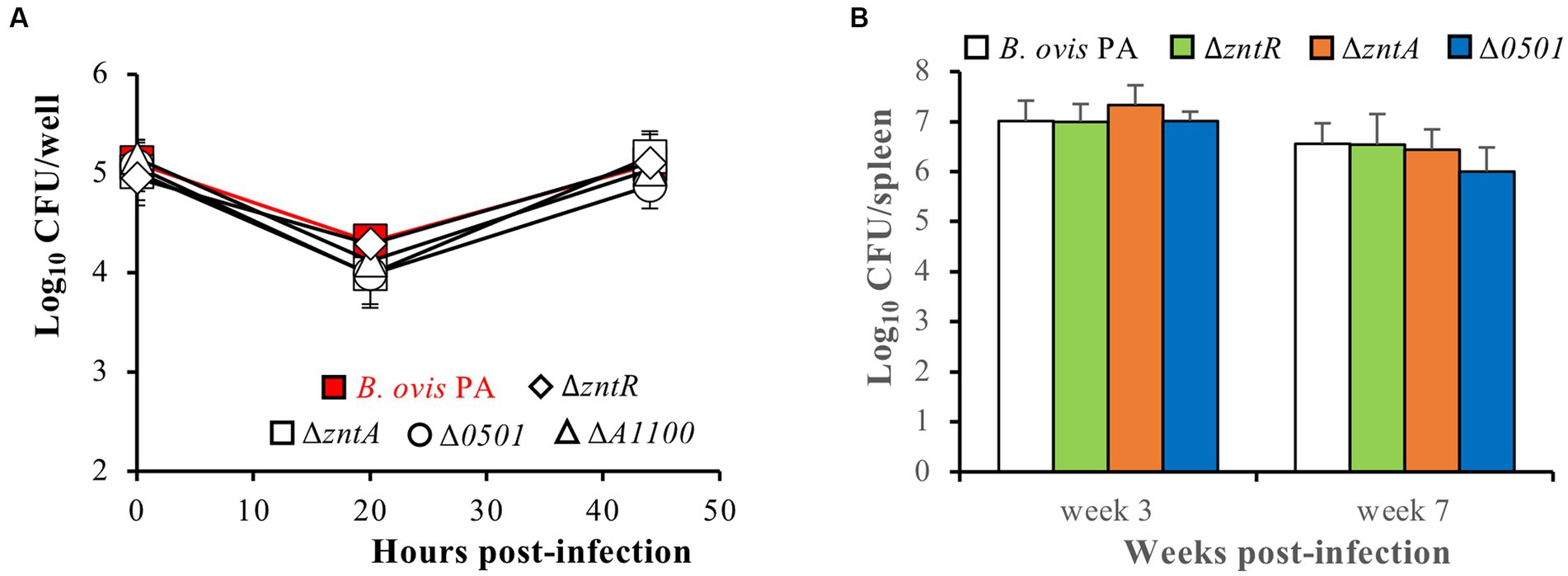
Figure 8. Virulence of the B. ovis PA deletion mutants in J774A.1 murine macrophages (A) and mice intraperitoneally infected with 106 CFU/mice (B). Intracellular bacteria in murine macrophages were enumerated at three time points after infection, and results for each strain and time point are expressed as means ± SD (n = 3) of the log10 CFU/well. Strain colonization in mice was evaluated by CFU accounts in spleen at weeks 3 and 7 post-infection, and results are expressed as means ± SD (n = 5) of the log10 CFUs/spleen. No statistically significant differences were detected in any strain compared to the parental strain.
Taking into account the previous results described for the ZntR-ZntA system in B. abortus 2308 (14) and those obtained here for all B. ovis PA mutants regarding gene expression, metal toxicity, and virulence in macrophages, only the ΔzntR and ΔzntA mutants were considered to merit evaluation in mice. However, since a discrete role in zinc export was detected for BOV_0501 that could be related to the differences observed in the ZntR-ZntA system between B. ovis and B. abortus, the ΔBOV_0501 mutant was also evaluated in mice. Spleen colonization at the acute and chronic phase of infection (weeks 3 and 7 post-infection, respectively) did not show reduced virulence of the mutant strains when compared to the parental strain (Figure 8B), which contrasts with the attenuated phenotype described for the ΔzntR mutant of B. abortus 2308 (14).
4 Discussion
Despite zinc’s essentiality for optimal functioning of the bacterial cell, its intracellular concentration must be finely tuned to avoid toxicity that is mainly derived from the high affinity of zinc by metal binding sites, which leads to mismetallation of metalloproteins that do not require zinc but other metals (9, 11, 13). Zinc intoxication, also detected inside macrophages, is considered part of the innate immune response of the host against microbes (9, 11, 13). Therefore, bacterial pathogens have evolved zinc efflux systems of three main types to fight against host defenses: (i) P-type ATPases, (ii) cation diffusion facilitator family exporters, and (iii) resistance-nodulation-cell division (RND) transporters (11–13).
Out of these three mechanisms of zinc export, only the first one has been evidenced in the genus Brucella, being ZntA, a P-type ATPase exclusively studied in B. abortus 2308 and whose expression is transcriptionally regulated by ZntR in a Zn2+ dependent manner (14). In the present study, the ZntR-ZntA system has also been shown to play a prominent role in circumventing zinc toxicity in B. ovis PA (Figure 4), but when compared to B. abortus 2308, relevant differences regarding ZntA amino acid sequence and activity, regulation of its expression by ZntR, and role in virulence have been demonstrated. According to the absence of 100 amino acids in the B. ovis ZntA protein (Figures 1C, 2), which is one of these main differences, a loss of functionality of the protein would be conceivable. However, results presented in this study demonstrate the increased sensitivity of the B. ovis ΔzntA mutant to toxic ZnCl2 concentrations (Figure 4) and equivalent resistance to this compound mediated by overexpression of B. ovis or B. abortus 2308 zntA (Figures 3, 4), which evidences that the ZntA proteins of both Brucella strains are functional zinc exporters with an apparent equivalent efficiency.
In fact, both Brucella ZntA proteins contain the motifs that are strictly conserved across all P1B-type ATPases (transition and heavy metal transporters) and that are part of the cytoplasmic ATP-binding and actuator domains (Figures 1C, 2B) (30, 34–36). Additionally, their TM4, TM5, and TM6 domains contain the characteristic motifs of the subgroup P1B-2 of P1B-type ATPases, which are Pb2+/Zn2+/Cd2+ efflux pumps (Figure 1C) (30, 37–39). The two Cys of the CPC motif and the Asp residue located in TM4 and TM6, respectively (Figure 1C), are known to be involved in the metal specificity of ZntA-related P1B-type ATPases and required for coordination of Zn2+, Cd2+, and Pb2+ (37, 38). However, a role for ZntA in Pb2+ detoxification could not be demonstrated in B. ovis, which might be related to the amino acid sequence of the cytoplasmic NTD. In E. coli, the NTD of ZntA contains the GXXCXXC and CCCDGAC motifs and can bind Zn2+, Cd2+, and Pb2+, but the ability to bind Pb2+ is lost when the CCCDGAC motif is removed (51). Therefore, the presence of a canonical GXXCXXC metal binding motif (GMDCASC) and the absence of the CCCDGAC motif in the B. ovis ortholog (Figures 1C, 2B) could explain, at least in part, the similar behavior of parental B. ovis PA and its isogenic ΔzntA mutant in the presence of toxic PbCl2 levels, while the growth of the ΔzntA mutant was highly impaired in the presence of toxic ZnCl2 and CdCl2 levels (Figures 4, 5). Considering that the NTD of B. abortus 2308 ZntA contains an additional GMDCASC motif and a His-rich region, also found in some Zn2+/Cd2+ P1B-type ATPases (42–44), a higher Zn2+ export activity could be expected for this ZntA protein. However, this property could only be inferred through indirect evidence provided by the increased levels of znuA transcripts, and of its encoded protein, detected in B. ovis PA-pNVzntA2308com5 when compared to those detected in B. ovis PA-pNVzntAPAcom5 (Figures 3B,C). Since ZnuA is a high-affinity zinc-binding periplasmic protein involved in zinc import (50), these results would be in accordance with a relevant reduction of intracellular zinc levels caused by overexpression of the ZntA zinc exporter, which would require to be counterbalanced by overexpression of zinc importer genes to maintain zinc homeostasis and cell viability.
The remarkable role of B. ovis ZntA in conferring resistance to cadmium (Figure 5) has not been reported before in the genus Brucella. However, it must be noted that this property was not evaluated with the ΔzntA mutant of B. abortus 2308 (14) and that our results confirm the powerful activity as cadmium exporters of both Brucella ZntA proteins (Figure 5A). In fact, the B. ovis PA ΔzntA mutant tolerates concentrations of ZnCl2 and CdCl2 in the order of 100-fold and 2000-fold lower than the parental strain, respectively (Figures 4C, 5B). Accordingly, although the presence of additional exporters contributing to Zn2+ efflux cannot be discarded, a higher efficiency of ZntA as a cadmium exporter could be inferred, considering that levels of zntA transcripts in B. ovis PA cultured in the presence of CdCl2 are not higher than those observed in the presence of ZnCl2 (Figures 3D, 5C). The increment of zntA expression in the presence of CdCl2 was accompanied by higher expression of znuA (Figure 5C), which could be related to an enhanced Zn2+ efflux mediated by ZntA increase. Alternatively, cadmium could compete with zinc for import, as suggested for similar results obtained with Klebsiella pneumoniae (52).
The results obtained in this study confirm that ZntA from both B. ovis PA and B. abortus 2308 also contributes to Co2+, Ni2+, and Cu2+ detoxification (Figure 6), which is in accordance with the reported ability of the three cations to act as substrates for E. coli ZntA, although with a much lower ATPase activity than that observed with Pb2+, Zn2+, and Cd2+ (40). Remarkably and in contrast to the results obtained with the other metals, overexpression of zntA in parental B. ovis PA or the ΔzntA mutant increased their resistance to CoCl2, allowing the recovery of growth patterns equivalent to those observed in normal medium (Figure 6A). Similar results have been reported in Helicobacter pylori, where in trans complementation of the ΔcadA mutant restored Zn2+ and Cd2+ susceptibility of the parental strain but increased the tolerance to Co2+ exposure (53).
Another significant difference observed in the ZntR-ZntA system between B. abortus 2308 and B. ovis refers to the ZntR-mediated regulation of zntA expression and the susceptibility of the ΔzntR mutant to ZnCl2. Thus, the deletion of zntR in B. abortus 2308 did not modify susceptibility to ZnCl2 but led to increased levels of zntA and znuA transcripts (14). ZntR was shown to bind to the zntR-zntA intergenic region in B. abortus 2308 and seemed to limit zntA expression under normal zinc availability conditions while it activated zntA expression in the presence of zinc excess, acting as a canonical MerR-type regulator (14). On the contrary, when compared to the parental strain, the B. ovis PA ΔzntR mutant was about 5-fold more susceptible to ZnCl2 than the parental strain (Supplementary Figure S2) and showed a marked reduction of zntA transcripts and unaltered znuA expression (Figure 4D). These results are consistent with the observation that growth defects of the ΔzntR mutant were abolished when zntA was overexpressed by transformation with pNVzntAPAcom5 or pNVzntA2308com5 (Figure 4A). Accordingly, ZntR does not seem to act in B. ovis PA as a repressor of zntA expression in a normal medium but rather to be an activator required for maximal zntA expression in response to increased Zn2+ levels (Figure 4A). These differences between B. abortus 2308 and B. ovis PA are difficult to explain since both ZntR proteins are identical, but might be dependent on the two nucleotide differences observed between both strains in the zntR-zntA intergenic region (Figure 2B), which could favor zntA transcription in B. ovis PA in normal growth conditions.
Additionally, both zntR and zntA are dispensable for virulence of B. ovis PA in the mouse model (Figure 8), while the ΔzntR mutant of B. abortus 2308 is attenuated (14). The increased level of zntA transcripts, which would reduce zinc availability in the host, was suggested to be the cause of this attenuation. This fact, together with the probable higher efficiency as a zinc exporter of B. abortus 2308 ZntA (Figures 3, 4) and the underexpression of zntA in the B. ovis PA ΔzntR mutant (Figure 4D), could explain, at least in part, the different virulent phenotype of the two ΔzntR mutants. As also observed with B. abortus 2308 (14), the full virulence of the B. ovis PA ΔzntA mutant, despite its high susceptibility to ZnCl2, suggests that host-induced zinc intoxication is not a relevant mechanism for the control of infection, at least in mice. However, the presence of additional Zn2+ exporters that could help to control limited intracellular toxic levels cannot be excluded.
Deletion of BOV_0501 and BOV_A1100, coding for the two other hypothetical zinc exporters identified, did not increase susceptibility to any of the divalent cations analyzed in this study, even though their encoded proteins display the canonical characteristics of known families of metal transporters. Thus, BOV_0501 shows characteristics of membrane proteins of the CDF family that transport metal divalent cations against a proton gradient (31) and that in prokaryotes usually function as dimers and contain approximately 300 amino acids, 6 TM domains, and a His-rich region—frequently located in the cytoplasmic CTD—that is able to bind metal cations and contribute to dimer stability (Figure 1A) (31–33, 54). The two His residues in the short cytoplasmic NTD (Figure 1A) might also be relevant for exporter activity, as reported for some representative members of bacterial CDF proteins (55). Additionally, BOV_0501 contains TM motifs associated with Zn2+ selectivity of the transporter: the L(X)9E motif in TM3 (31) and the H(X)3D and E(X)3D motifs located in TM2 and TM5 (31, 32, 55), respectively (Figure 1A). As observed for ZntA, BOV_A1100 displays the characteristic motifs of the actuator and ATP-binding domains of P1B-type ATPases (Figure 1B). However, motifs of its TM domain correspond to the P1B-4 subgroup that is mainly constituted by Co2+ exporters (30), although some of them also exhibit Zn2+ and Cd2+ efflux activity (56) that have only 6–7 TM regions and lack a cytoplasmic NTD (30, 43, 56). The activity of P1B-4-ATPases is highly dependent on four amino acid residues: a Met in TM1, the Ser and Cys residues of the TM4 SPC motif, and the His residue of the TM6 HEGGT(X)5N motif (43, 56) (Figure 1B). The three latter elements are found in BOV_A1100, but the Met residue is not present in TM1 (Figure 1B), which could explain, at least in part, why the ΔBOV_A1100 mutant of B. ovis PA behaved as the parental strain in the presence of toxic levels of all the divalent cations tested (data not shown). However, two other factors could explain why a role as metal exporter was not detected for BOV_0501 and BOV_A1100: (i) the low level of transcripts of both genes observed in all conditions tested (data not shown) and (ii) the activity of B. ovis PA ZntA as exporter of divalent cations (Figures 4–6) that could compensate the hypothetical defects in metal efflux caused by the absence of BOV_0501 and BOV_A1100. Although overexpression of BOV_0501 and BOV_A1100 in the B. ovis PA ΔzntA mutant suggests that BOV_0501 and BOV_A1100 might contribute to B. ovis Zn2+ and Co2+ homeostasis, respectively (Figure 7), the low level of their transcripts in the conditions tested in this study, together with the absence of noticeable defects caused by their deletion, suggest a minor role for both genes in metal homeostasis.
Although none of the characterized mutants of rough B. ovis showed potential use as a vaccine candidate, the results presented in this study broaden knowledge about metal detoxification in pathogenic Brucellae that has only been studied in smooth species. The differences observed between B. ovis and B. abortus in the ZntR-ZntA system reveal a new source of heterogeneity among pathogenic Brucella species that, despite their high degree of homology at the DNA level, exhibit relevant differences in pathogenicity and host preference (57). The differences described here could also be related to the distinctive traits of B. ovis and B. abortus regarding outer membrane composition and properties (58, 59), which could also affect metal import–export pathways. Studies evaluating zinc import in B. ovis are being conducted in our laboratory to establish a more precise picture of zinc homeostasis in this rough Brucella species.
Data availability statement
The raw data supporting the conclusions of this article will be made available by the authors, without undue reservation.
Ethics statement
The animal study was reviewed and approved by the Bioethics Committee of the University of Salamanca and the competent authority of Junta de Castilla y León, Spain. The study was conducted in accordance with the local legislation and institutional requirements.
Author contributions
BT-C: Conceptualization, Data curation, Investigation, Methodology, Writing – original draft, Writing – review & editing. CT: Data curation, Investigation, Methodology, Writing – review & editing. RC-Á: Investigation, Methodology, Writing – review & editing. PM: Investigation, Methodology, Writing – review & editing. NV: Conceptualization, Data curation, Formal analysis, Funding acquisition, Investigation, Methodology, Project administration, Supervision, Writing – original draft, Writing – review & editing.
Funding
The author(s) declare financial support was received for the research, authorship, and/or publication of this article. This study is part of projects PID2019-107601RB-C33, PID2019-107601RA-C32, and PID2019-107601RB-C31 financed by MCIN/AEI/10.13039/501100011033. Projects were awarded by Ministerio de Ciencia e Innovación, Spain. Financial support to BT-C was provided by project PID2019-107601RB-C33 financed by MCIN/AEI/ 10.13039/501100011033 and by Program Investigo from the Spanish Servicio Público de Empleo Estatal (University of Salamanca call) financed by NextGenerationEU (Recovery Plan, Transformation and Resilience). PM (CITA) work was also supported by the Aragon Government (Grupo de Investigación A21_23R).
Acknowledgments
The authors thank the staff of the DNA sequencing and animal experimentation facilities of the University of Salamanca for their efficient assistance. Collaboration with the proteomics facility of Centro de Investigación del Cáncer of Salamanca is also acknowledged.
Conflict of interest
The authors declare that the research was conducted in the absence of any commercial or financial relationships that could be construed as a potential conflict of interest.
Publisher’s note
All claims expressed in this article are solely those of the authors and do not necessarily represent those of their affiliated organizations, or those of the publisher, the editors and the reviewers. Any product that may be evaluated in this article, or claim that may be made by its manufacturer, is not guaranteed or endorsed by the publisher.
Supplementary material
The Supplementary material for this article can be found online at: https://www.frontiersin.org/articles/10.3389/fvets.2023.1323500/full#supplementary-material
Footnotes
1. ^https://www.kegg.jp/kegg-bin/show_organism?org=T00534
2. ^https://www.kegg.jp/kegg/genes.html
3. ^https://blast.ncbi.nlm.nih.gov
5. ^https://wwwdev.ebi.ac.uk/Tools/jdispatcher/psa/emboss_needle
References
1. OIE. Chapter 3.8.7. Ovine epididymitis (Brucella ovis ). Manual of diagnostic tests and vaccines for terrestrial animals. (2021). Available at: https://www.oie.int/fileadmin/Home/eng/Health_standards/tahm/3.08.7_OVINE_EPID.pdf.
2. Picard-Hagen, N, Berthelot, X, Champion, JL, Eon, L, Lyazrhi, F, Marois, M, et al. Contagious epididymitis due to Brucella ovis: relationship between sexual function, serology and bacterial shedding in semen. BMC Vet Res. (2015) 11:125. doi: 10.1186/s12917-015-0440-7
3. OIE. Chapter 3.1.4. Brucellosis (infection with B. abortus, B. Melitensis and B. suis). Manual of diagnostic tests and vaccines for terrestrial animals. (2021). Available at: https://www.oie.int/fileadmin/Home/eng/Health_standards/tahm/3.01.4_BRUCELLOSIS.pdf.
4. Roop, RM 2nd, Barton, IS, Hopersberger, D, and Martin, DW. Uncovering the hidden credentials of Brucella virulence. Microbiol Mol Biol Rev. (2021) 85:19. doi: 10.1128/MMBR.00021-19
5. Moriyón, I, Blasco, JM, Letesson, JJ, De Massis, F, and Moreno, E. Brucellosis and one health: inherited and future challenges. Microorganisms. (2023) 11:2070. doi: 10.3390/microorganisms11082070
6. Soler-Lloréns, P, Gil-Ramírez, Y, Zabalza-Baranguá, A, Iriarte, M, Conde-Álvarez, R, Zúñiga-Ripa, A, et al. Mutants in the lipopolysaccharide of Brucella ovis are attenuated and protect against B. ovis infection in mice. Vet Res. (2014) 45:72. doi: 10.1186/s13567-014-0072-0
7. Ridler, AL, and West, DM. Control of Brucella ovis infection in sheep. Vet Clin North Am Food Anim Pract. (2011) 27:61–6. doi: 10.1016/j.cvfa.2010.10.013
8. Marín, CM, Mainar, RC, de Miguel, MJ, Andrés, S, Alvarez, JJ, Blasco, JM, et al. Re-emergence of Brucella ovis infection in Aragon (Spain) after the ban of rev 1 vaccination. 72nd Annual Brucellosis Research Conference. (2019). Chicago (IL).
9. Begg, SL. The role of metal ions in the virulence and viability of bacterial pathogens. Biochem Soc Trans. (2019) 47:77–87. doi: 10.1042/BST20180275
10. Chandrangsu, P, Rensing, C, and Helmann, JD. Metal homeostasis and resistance in bacteria. Nat Rev Microbiol. (2017) 15:338–50. doi: 10.1038/nrmicro.2017.15
11. Murdoch, CC, and Skaar, EP. Nutritional immunity: the battle for nutrient metals at the host-pathogen interface. Nat Rev Microbiol. (2022) 20:657–70. doi: 10.1038/s41579-022-00745-6
12. Capdevila, DA, Wang, J, and Giedroc, DP. Bacterial strategies to maintain zinc metallostasis at the host-pathogen Interface. J Biol Chem. (2016) 291:20858–68. doi: 10.1074/jbc.R116.742023
13. Lonergan, ZR, and Skaar, EP. Nutrient zinc at the host-pathogen Interface. Trends Biochem Sci. (2019) 44:1041–56. doi: 10.1016/j.tibs.2019.06.010
14. Sheehan, LM, Budnick, JA, Roop, RM 2nd, and Caswell, CC. Coordinated zinc homeostasis is essential for the wild-type virulence of Brucella abortus. J Bacteriol. (2015) 197:1582–91. doi: 10.1128/JB.02543-14
15. Martín-Martín, AI, Sancho, P, de Miguel, MJ, Fernández-Lago, L, and Vizcaíno, N. Quorum-sensing and BvrR/BvrS regulation, the type IV secretion system, cyclic glucans, and BacA in the virulence of Brucella ovis: similarities to and differences from smooth brucellae. Infect Immun. (2012) 80:1783–93. doi: 10.1128/IAI.06257-11
16. Kovach, ME, Elzer, PH, Hill, DS, Robertson, GT, Farris, MA, Roop, RM 2nd, et al. Four new derivatives of the broad-host-range cloning vector pBBR1MCS, carrying different antibiotic-resistance cassettes. Gene. (1995) 166:175–6. doi: 10.1016/0378-1119(95)00584-1
17. Elhassanny, AE, Anderson, ES, Menscher, EA, and Roop, RM 2nd. The ferrous iron transporter FtrABCD is required for the virulence of Brucella abortus 2308 in mice. Mol Microbiol. (2013) 88:1070–82. doi: 10.1111/mmi.12242
18. UniProt, C. UniProt: the universal protein knowledgebase in 2023. Nucleic Acids Res. (2023) 51:D523–31. doi: 10.1093/nar/gkac1052
19. Yu, NY, Wagner, JR, Laird, MR, Melli, G, Rey, S, Lo, R, et al. PSORTb 3.0: improved protein subcellular localization prediction with refined localization subcategories and predictive capabilities for all prokaryotes. Bioinformatics. (2010) 26:1608–15. doi: 10.1093/bioinformatics/btq249
20. Tartilán-Choya, B, Sidhu-Muñoz, RS, and Vizcaíno, N. The transcriptional regulator MucR, but not its controlled acid-activated chaperone HdeA, is essential for virulence and modulates surface architecture and properties in Brucella ovis PA. Front Vet Sci. (2022) 8:814752. doi: 10.3389/fvets.2021.814752
21. Yuan, JS, Reed, A, Chen, F, and Stewart, CN Jr. Statistical analysis of real-time PCR data. BMC Bioinfo. (2006) 7:85. doi: 10.1186/1471-2105-7-85
22. Sidhu-Muñoz, RS, Tejedor, C, and Vizcaíno, N. The three flagellar loci of Brucella ovis PA are dispensable for virulence in cellular models and mice. Front Vet Sci. (2020) 7:441. doi: 10.3389/fvets.2020.00441
23. Martín-Martín, AI, Caro-Hernández, P, Sancho, P, Tejedor, C, Cloeckaert, A, Fernández-Lago, L, et al. Analysis of the occurrence and distribution of the Omp25/Omp31 family of surface proteins in the six classical Brucella species. Vet Microbiol. (2009) 137:74–82. doi: 10.1016/j.vetmic.2008.12.003
24. Cox, J, Neuhauser, N, Michalski, A, Scheltema, RA, Olsen, JV, and Mann, M. Andromeda: a peptide search engine integrated into the MaxQuant environment. J Proteome Res. (2011) 10:1794–805. doi: 10.1021/pr101065j
25. Cox, J, and Mann, M. MaxQuant enables high peptide identification rates, individualized p.p.b.-range mass accuracies and proteome-wide protein quantification. Nat Biotechnol. (2008) 26:1367–72. doi: 10.1038/nbt.1511
26. Schwanhäusser, B, Busse, D, Li, N, Dittmar, G, Schuchhardt, J, Wolf, J, et al. Global quantification of mammalian gene expression control. Nature. (2011) 473:337–42. doi: 10.1038/nature10098
27. Sidhu-Muñoz, RS, Sancho, P, and Vizcaíno, N. Brucella ovis PA mutants for outer membrane proteins Omp10, Omp19, SP41, and BepC are not altered in their virulence and outer membrane properties. Vet Microbiol. (2016) 186:59–66. doi: 10.1016/j.vetmic.2016.02.010
28. Sancho, P, Tejedor, C, Sidhu-Muñoz, RS, Fernández-Lago, L, and Vizcaíno, N. Evaluation in mice of Brucella ovis attenuated mutants for use as live vaccines against B. ovis infection. Vet Res. (2014) 45:61. doi: 10.1186/1297-9716-45-61
29. Sidhu-Muñoz, RS, Sancho, P, Cloeckaert, A, Zygmunt, MS, de Miguel, MJ, Tejedor, C, et al. Characterization of cell envelope multiple mutants of Brucella ovis and assessment in mice of their vaccine potential. Front Microbiol. (2018) 9:2230. doi: 10.3389/fmicb.2018.02230
30. Smith, AT, Smith, KP, and Rosenzweig, AC. Diversity of the metal-transporting P1B-type ATPases. J Biol Inorg Chem. (2014) 19:947–60. doi: 10.1007/s00775-014-1129-2
31. Kolaj-Robin, O, Russell, D, Hayes, KA, Pembroke, JT, and Soulimane, T. Cation diffusion facilitator family: structure and function. FEBS Lett. (2015) 589:1283–95. doi: 10.1016/j.febslet.2015.04.007
32. Montanini, B, Blaudez, D, Jeandroz, S, Sanders, D, and Chalot, M. Phylogenetic and functional analysis of the cation diffusion facilitator (CDF) family: improved signature and prediction of substrate specificity. BMC Genomics. (2007) 8:107. doi: 10.1186/1471-2164-8-107
33. Barber-Zucker, S, Moran, A, and Zarivach, R. Metal transport mechanism of the cation diffusion facilitator (CDF) protein family - a structural perspective on human CDF (ZnT)-related diseases. RSC Chem Biol. (2021) 2:486–98. doi: 10.1039/D0CB00181C
34. Roberts, CS, Muralidharan, S, Ni, F, and Mitra, B. Structural role of the first four transmembrane helices in ZntA, a P(1B)-type ATPase from Escherichia coli. Biochemistry. (2020) 59:4488–98. doi: 10.1021/acs.biochem.0c00770
35. Klein, JS, and Lewinson, O. Bacterial ATP-driven transporters of transition metals: physiological roles, mechanisms of action, and roles in bacterial virulence. Metallomics. (2011) 3:1098–108. doi: 10.1039/c1mt00073j
36. Liu, J, Dutta, SJ, Stemmler, AJ, and Mitra, B. Metal-binding affinity of the transmembrane site in ZntA: implications for metal selectivity. Biochemistry. (2006) 45:763–72. doi: 10.1021/bi051836n
37. Gallenito, MJ, Irvine, GW, Zhang, L, and Meloni, G. Coordination promiscuity guarantees metal substrate selection in transmembrane primary-active Zn2+ pumps. Chem Commun (Camb). (2019) 55:10844–7. doi: 10.1039/C9CC05936A
38. Dutta, SJ, Liu, J, Hou, Z, and Mitra, B. Conserved aspartic acid 714 in transmembrane segment 8 of the ZntA subgroup of P1B-type ATPases is a metal-binding residue. Biochemistry. (2006) 45:5923–31. doi: 10.1021/bi0523456
39. Zhitnitsky, D, and Lewinson, O. Identification of functionally important conserved trans-membrane residues of bacterial PIB-type ATPases. Mol Microbiol. (2014) 91:777–89. doi: 10.1111/mmi.12495
40. Hou, Z, and Mitra, B. The metal specificity and selectivity of ZntA from Escherichia coli using the acylphosphate intermediate. J Biol Chem. (2003) 278:28455–61. doi: 10.1074/jbc.M301415200
41. Hou, ZJ, Narindrasorasak, S, Bhushan, B, Sarkar, B, and Mitra, B. Functional analysis of chimeric proteins of the Wilson Cu(I)-ATPase (ATP7B) and ZntA, a Pb(II)/Zn(II)/Cd(II)-ATPase from Escherichia coli. J Biol Chem. (2001) 276:40858–63. doi: 10.1074/jbc.M107455200
42. Liu, T, Reyes-Caballero, H, Li, C, Scott, RA, and Giedroc, DP. Multiple metal binding domains enhance the Zn(II) selectivity of the divalent metal ion transporter AztA. Biochemistry. (2007) 46:11057–68. doi: 10.1021/bi7006367
43. Argüello, JM. Identification of ion-selectivity determinants in heavy-metal transport P1B-type ATPases. J Membr Biol. (2003) 195:93–108. doi: 10.1007/s00232-003-2048-2
44. Lee, SW, Glickmann, E, and Cooksey, DA. Chromosomal locus for cadmium resistance in Pseudomonas putida consisting of a cadmium-transporting ATPase and a MerR family response regulator. Appl Environ Microbiol. (2001) 67:1437–44. doi: 10.1128/AEM.67.4.1437-1444.2001
45. Halling, SM, and Bricker, BJ. Characterization and occurrence of two repeated palindromic DNA elements of Brucella spp.: Bru-RS1 and Bru-RS2. Mol Microbiol. (1994) 14:681–9. doi: 10.1111/j.1365-2958.1994.tb01306.x
46. Chaoprasid, P, Nookabkaew, S, Sukchawalit, R, and Mongkolsuk, S. Roles of Agrobacterium tumefaciens C58 ZntA and ZntB and the transcriptional regulator ZntR in controlling Cd2+/Zn2+/Co2+ resistance and the peroxide stress response. Microbiology (Reading). (2015) 161:1730–40. doi: 10.1099/mic.0.000135
47. Tsolis, RM, Seshadri, R, Santos, RL, Sangari, FJ, García Lobo, JM, de Jong, MF, et al. Genome degradation in Brucella ovis corresponds with narrowing of its host range and tissue tropism. PloS One. (2009) 4:e5519. doi: 10.1371/journal.pone.0005519
48. Halling, SM, Tatum, FM, and Bricker, BJ. Sequence and characterization of an insertion sequence, IS711, from Brucella ovis. Gene. (1993) 133:123–7. doi: 10.1016/0378-1119(93)90236-V
49. Peeters, BP, de Boer, JH, Bron, S, and Venema, G. Structural plasmid instability in Bacillus subtilis: effect of direct and inverted repeats. Mol Gen Genet. (1988) 212:450–8. doi: 10.1007/BF00330849
50. Kim, S, Watanabe, K, Shirahata, T, and Watarai, M. Zinc uptake system (znuA locus) of Brucella abortus is essential for intracellular survival and virulence in mice. J Vet Med Sci. (2004) 66:1059–63. doi: 10.1292/jvms.66.1059
51. Liu, J, Stemmler, AJ, Fatima, J, and Mitra, B. Metal-binding characteristics of the amino-terminal domain of ZntA: binding of lead is different compared to cadmium and zinc. Biochemistry. (2005) 44:5159–67. doi: 10.1021/bi0476275
52. Maunders, EA, Ganio, K, Hayes, AJ, Neville, SL, Davies, MR, Strugnell, RA, et al. The role of ZntA in Klebsiella pneumoniae zinc homeostasis. Microbiol Spectr. (2022) 10:e0177321. doi: 10.1128/spectrum.01773-21
53. Herrmann, L, Schwan, D, Garner, R, Mobley, HL, Haas, R, Schafer, KP, et al. Helicobacter pylori cadA encodes an essential Cd(II)-Zn(II)-Co(II) resistance factor influencing urease activity. Mol Microbiol. (1999) 33:524–36. doi: 10.1046/j.1365-2958.1999.01496.x
54. Cotrim, CA, Jarrott, RJ, Martin, JL, and Drew, D. A structural overview of the zinc transporters in the cation diffusion facilitator family. Acta Crystallogr D Struct Biol. (2019) 75:357–67. doi: 10.1107/S2059798319003814
55. Anton, A, Weltrowski, A, Haney, CJ, Franke, S, Grass, G, Rensing, C, et al. Characteristics of zinc transport by two bacterial cation diffusion facilitators from Ralstonia metallidurans CH34 and Escherichia coli. J Bacteriol. (2004) 186:7499–507. doi: 10.1128/JB.186.22.7499-7507.2004
56. Smith, AT, Ross, MO, Hoffman, BM, and Rosenzweig, AC. Metal selectivity of a Cd-, Co-, and Zn-transporting P1B-type ATPase. Biochemistry. (2017) 56:85–95. doi: 10.1021/acs.biochem.6b01022
57. Moreno, E. The one hundred year journey of the genus Brucella (Meyer and Shaw 1920). FEMS Microbiol Rev. (2021):45. doi: 10.1093/femsre/fuaa045
58. Martín-Martín, AI, Sancho, P, Tejedor, C, Fernández-Lago, L, and Vizcaíno, N. Differences in the outer membrane-related properties of the six classical Brucella species. Vet J. (2011) 189:103–5. doi: 10.1016/j.tvjl.2010.05.021
Keywords: Brucella ovis, zinc, cadmium, cobalt, ZntA, ZntR, ZnuA, virulence
Citation: Tartilán-Choya B, Tejedor C, Conde-Álvarez R, Muñoz PM and Vizcaíno N (2024) Characterization of three predicted zinc exporters in Brucella ovis identifies ZntR-ZntA as a powerful zinc and cadmium efflux system not required for virulence and unveils pathogenic Brucellae heterogeneity in zinc homeostasis. Front. Vet. Sci. 10:1323500. doi: 10.3389/fvets.2023.1323500
Edited by:
Min Yue, Zhejiang University, ChinaReviewed by:
Hassan Al-Tameemi, University of Basrah, IraqClayton Caswell, Virginia Tech, United States
Huiling Sun, Beijing Academy of Agriculture and Forestry Sciences, China
Copyright © 2024 Tartilán-Choya, Tejedor, Conde-Álvarez, Muñoz and Vizcaíno. This is an open-access article distributed under the terms of the Creative Commons Attribution License (CC BY). The use, distribution or reproduction in other forums is permitted, provided the original author(s) and the copyright owner(s) are credited and that the original publication in this journal is cited, in accordance with accepted academic practice. No use, distribution or reproduction is permitted which does not comply with these terms.
*Correspondence: Nieves Vizcaíno, vizcaino@usal.es