- 1Faculty of Veterinary Medicine, Department of Internal Medicine, Selcuk University, Konya, Türkiye
- 2Faculty of Veterinary Medicine, Department of Internal Medicine, Hatay Mustafa Kemal University, Hatay, Türkiye
Hypoglycemia is a condition associated with neonatal diarrhea in calves, leading to increased mortality and neurological clinical signs. The aim of the present study was to determine the development of brain damage in hypoglycemic calves with neonatal diarrhea and the diagnostic and prognostic significance of these biomarkers. Ten healthy and 50 hypoglycemic calves with diarrhea were included in the study. Clinical examination, blood gases and complete blood count were performed at admission. Blood serum calcium-binding protein B (S100B), neuron-specific enolase (NSE), glial fibrillary acidic protein (GFAP), ubiquitin carboxyl-terminal hydrolysis isoenzyme-1 (UCHL-1), activitin A (ACT), adrenomodullin (AM) concentrations, and creatine kinase-BB (CK-BB) enzyme activity were measured using commercial bovine-specific ELISA kits to assess brain damage. Of the hypoglycemic calves enrolled in the study, 13 (26%) survived and 37 (74%) died. In addition, 32 (64%) of the calves had severe acidosis and 24 (48%) had sepsis. S100B, GFAP, UCHL-1, CK-BB (p < 0.001) and NSE (p < 0.05) concentrations were significantly higher in hypoglycemic calves compared to healthy calves, while ACT concentrations were lower. Blood glucose concentration was negatively correlated with serum S100B, GFAP, UCHL-1, and CK-BB enzyme activity and positively correlated with ACT in hypoglycemic calves (p < 0.01). Brain injury biomarkers were not predictive of mortality (p > 0.05). Morever, severe hypoglycemia, severe acidosis and sepsis variables were not found to have sufficient capacity to predict mortality when considered alone or together (p > 0.05). In conclusion, brain damage may develop as a consequence of hypoglycemia in calves. S100B, NSE, GFAP, UCHL-1, ACT, and CK-BB concentrations can be used to diagnose brain damage in hypoglycemic calves. However, the variables of severe hypoglycemia, severe acidosis, and sepsis together with the biomarkers of brain injury have a limited value in predicting the prognosis of neonatal calves with diarrhea.
1. Introduction
Neonatal diarrhea remains an important herd health problem in suckling calves, which can lead to mortality and economic losses (1). Hypoglycemia, azotemia, hyponatremia, hyperkalemia, septicemia, hyperlactatemia, and strong ion (metabolic) acidosis are the most common laboratory complications in calves with diarrhea (2, 3). Among these complications, hypoglycemia has been reported to occur in a very small percentage of cases of acute diarrhea and is associated with increased mortality (4).
Under physiological conditions, glucose is the primary energy substrate for the brain in both animals and humans. Decreased plasma glucose levels for various reasons impair brain glucose metabolism, resulting in functional brain damage (5). In addition, hypoglycemia can cause dysfunction of blood–brain barrier (BBB) permeability (6) and structural and functional disturbances in the peripheral nervous system (5). Therefore, it has been reported that the risk of permanent brain damage in hypoglycemic neonates is high (7, 8).
Recently, brain injury biomarker concentrations have been used in the diagnosis and prognosis of various central nervous system (CNS) disorders (9–12). Among these biomarkers, calcium-binding protein B (S100B) and neuron-specific enolase (NSE) have been reported to have increased concentrations in hypoglycemic newborns in association with the development of brain injury (8). Concentration of glial fibrillary acidic protein (GFAP), an intermediate cytoskeletal filament protein specific for astrocytes, have been found to increase as a result of glial damage during abnormal glucose homeostasis (11, 13). Ubiquitin C-terminal hydrolase-1 (UCHL-1) is a reliable biomarker that is widely expressed in neurons and neuroendocrine cells (14). Clinical studies in calves (12) have reported that UCHL-1 is a useful biomarker for the detection of hypoxic–ischemic encephalopathy. Activin A (ACT) protein has an important biological effect on neuronal cell differentiation (15). It has been found that ACT concentrations increase after neuronal damage related to oxygen–glucose deprivation, and exogenous administration of ACT has a neuroprotective effect by preventing apoptosis in neurons (10). Adrenomodullin (AM), a hypotensive vasodilator peptide, is synthesized in the organism as preproadrenomodulin. Studies in the rat model showed that oxygen and glucose deprivation increased AM in several brain regions compared to control animals (9). Creatine kinase-BB (CK-BB) is an isoenzyme that is found in astrocytes (16), and it has been reported that the activity of the CK-BB enzyme is significantly increased in infants with neurological disorders (17).
Although our knowledge of the brain damage caused by hypoglycemia in human medicine is now well advanced, the studies in veterinary medicine are still limited. The present study was designed with the hypothesis that hypoglycemia may lead to brain damage in neonatal calves. The aim of this study was to evaluate brain damage in hypoglycemic calves using brain-specific biomarkers and to determine their diagnostic and prognostic significance.
2. Materials and methods
The study was conducted between January 2022 and March 2023 at the Department of Internal Medicine, Faculty of Veterinary Medicine, Selcuk University, Konya, Türkiye. The study protocol was approved by the Institutional Ethics Committee of the Faculty of Veterinary Medicine, Selcuk University (No. 2022/07).
2.1. Study groups
Ten healthy calves (8 Holstein and 2 Simmental), > 280 days gestation, 2–14 days old, were enrolled in the study as a control group. Calves were considered healthy based on clinical examination and laboratory findings (18). Calves were born naturally at the faculty farm. Calves with dystocia, prematurity, congenital abnormalities, hypoglycemia, acidemia, and suspected infection were excluded from the study.
Fifty calves with diarrhea (26 Holstein, 12 Simmental, 7 Brown Swiss, and 5 Charolais), > 280 days gestation, 2–14 days old were enrolled in the study as the hypoglycemic group. All calves were hospitalized for 3 days and received standard care and a feeding protocol after admission to the neonatal intensive care unit (19). Criteria for hypoglycemia were defined as a blood glucose concentration < 79.2 mg/dL. Mild hypoglycemia and severe hypoglycemia were also defined as blood glucose concentrations between 36 and 79 mg/dL and < 36 mg/dL, respectively (20). In the first step, the results of the study (blood gas analysis, CBC and brain related biomarkers) were compared between healthy (n = 10) and hypoglycemic (n = 50) calves. The hypoglycemic calves were then divided into mild hypoglycemic (n = 8) and severe hypoglycemic groups (n = 42) and the concentrations of brain related biomarkers were compared. Next, brain-related biomarker concentrations were compared between surviving (n = 13) and non-surviving (n = 37) hypoglycemic calves. Finally, calves with sepsis, severe acidosis (pH < 7.20) (21), and severe hypoglycemia were adjusted to find a model for mortality. Sepsis was described as the existence of systemic inflammatory response syndrome (SIRS) and a suspected or proven infection. Definitions for SIRS were based on the presence of the two or more of the following abnormalities: leukocyte count (leukocytosis or leukopenia, or band neutrophils >10%), abnormal rectal temperature, tachycardia, and tachypnea (22).
2.2. Clinical examination
All clinical examinations followed a standardized protocol and were performed by the same investigators (MI and AE) on admission and during hospitalization. Degree of enophthalmos (none, mild to moderate, severe), mental status (alert, depressed, comatose), suckling reflex (strong, weak, absent), and posture (standing, sternal, lateral recumbency) were assessed. Heart rate (beats/min), rectal temperature (°C), respiratory rate (breaths/min), mucous membranes (hyperemic or cyanotic), and capillary refill time were also recorded (4, 19).
2.3. Collection of blood samples
Blood samples were collected from the calves at the time of admission. Blood samples for blood gas analysis, complete blood count (CBC), and biomarkers of brain injury were collected from the jugular vein. For blood gas measurements, plastic syringes containing sodium heparin were used. Tubes containing K3EDTA were used to analyze the CBC. Blood gas and CBC measurements were performed within 5 to 10 min after the sample was collected. Non-anticoagulant tubes were used for serum collection. Blood samples collected for biomarker analysis were kept at room temperature for 15 min and then centrifuged at 20 × g for 10 min. Sera were collected and stored at −80°C.
2.3.1. Blood gas analysis
Venous blood pH, partial carbon dioxide pressure (pCO2), partial oxygen pressure (pO2), oxygen saturation (SO2), potassium (K), sodium (Na), calcium (Ca), chlorine (Cl), glucose (Glu), lactate (Lac), base deficit (BE), and bicarbonate (HCO3) were measured using an automated blood gas analyzer (ABL 90 Flex, Radiometer, Brea, CA, United States).
2.3.2. Complete blood count (CBC) analysis
Total leukocytes (WBC), lymphocytes (Lym), monocytes (Mon), granulocytes (Gra), erythrocytes (RBC), hematocrit (HCT), hemoglobin (Hb), and platelets (PLT) were measured using an automated cell counter (MS4e, Melet Schlosing Laboratories, Osny, France).
2.3.3. Evaluation of brain-related biomarkers
Serum S100B, UCHL-1, (Bioassay Technology Laboratory, Shanghai, China), NSE, GFAP, ACT, AM (MyBioSource, San Diego, CA, United States), and CK-BB (ELK Biotechnology Co., Ltd., Wuhan, China) concentrations were measured using commercial bovine-specific ELISA test kits according to the manufacturer’s instructions. Bovine S100B commercial ELISA kit (Bioassay Technology Laboratory, Shanghai, China, Lot: 202110012), bovine NSE commercial ELISA kit (MyBioSource®, San Diego, CA, United States, Lot: 36379821), bovine GFAP commercial sandwich ELISA kit (MyBioSource®, San Diego, CA, United States, Lot: 34358721), bovine UCHL-1 commercial ELISA kit (Bioassay Technology Laboratory, Shanghai, China, Lot: 202110012), bovine ACT commercial ELISA kit (MyBioSource®, San Diego, CA, United States, Lot: 20211022C), bovine AM commercial ELISA kit (MyBioSource®, San Diego, CA, United States, Lot: 38400921), and bovine CK-BB commercial ELISA kit (ELK Biotechnology, Wuhan, China, Lot: 20330054610) were used for biomarker ELISA analyses. The intra-assay coefficient of variation (CV), inter-assay CV, and minimum detectable concentrations (MDC) for biomarkers were ≤ 8%, ≤ 10%, and 0. 26 ng/mL for S100B, ≤ 8%, ≤ 12% and > 0.06 ng/mL for NSE, ≤ 8%, ≤ 12% and > 0.06 ng/mL for GFAP, ≤ 8%, ≤ 10%, and 35.7 ng/mL for UCHL-1, < 10%, < 10% and 1.0 pg/mL for ACT, ≤8%, ≤12% and 5 pg/mL for AM, and < 8%, < 10% and 0.59 ng/mL for CK-BB, respectively.
2.4. Statistical analysis
2.4.1. Power analysis
The 95% confidence interval (CI) and effect size (margin of error) for the hypoglycemic calves with diarrhea were included in the calculation. Previous study in neonatal calves with asphyxia has demonstrated brain damage in 50% calves (12). Based on this assumption, 50 neonatal calves were considered necessary to identify brain damage associated with hypoglycemia with 80% power and 5% alpha error level using a 2-tailed test.
2.4.2. Analysis of variances
The SPSS 25 statistical program (IBM Corp®, 2017, Armonk, NY, United States) was used to evaluate the data. The Kolmogorov–Smirnov test was used to determine normality of variables and homogeneity of variances. Parametric data were expressed as mean ± SD and evaluated by Student’s t-test. Non-parametric data were expressed as median (minimum/maximum) and evaluated using the Mann–Whitney U test. The Spearman correlation test was used to determine the correlation between variables. Binary logistic regression was used to evaluate the association of severe hypoglycemia, severe acidemia, and sepsis with mortality. The goodness of fit of the model was assessed using Pearson chi-squared. Receiver operating characteristic (ROC) analysis was performed to determine the prognostic cut-off, sensitivity, and specificity of the variables in non-surviving and surviving hypoglycemic calves. In addition, the same test was used to evaluate the ability of severe hypoglycemia, severe acidosis, and sepsis to predict mortality. Statistical significance was considered as p < 0.05.
3. Results
3.1. Clinical findings
Fifty hypoglycemic calves with diarrhea and 10 healthy calves were included in the study. The mean body weight of the calves was 42.37 ± 2.79 in the hypoglycemic group and 44.54 ± 2.35 in the healthy group. The most prominent clinical signs in hypoglycemic calves were hypothermia, lethargy, lateral recumbency, loss of suckling reflex, severe depression, and coma. CNS-related symptoms such as convulsions, opisthotonos, and nystagmus were seen in 6 (12%) hypoglycemic calves. Of the hypoglycemic calves, 37 (74%) did not survive and 13 (26%) survived. It was determined that 42 (84%) of the hypoglycemic calves had severe hypoglycemia. In addition, 32 (64%) and 24 (48%) calves had severe acidosis and sepsis, respectively.
3.2. Blood gas and CBC analysis
At the time of admission, pH, pO2, SO2, glucose, BE, and HCO3 levels of hypoglycemic calves were significantly lower and pCO2, lactate, and K levels were higher than healthy calves (p < 0.05). Total leukocytes, Lym, Mon, RBC, Hb, and PLT levels of hypoglycemic calves were significantly higher than healthy calves (p < 0.05) (Table 1).
3.3. Brain-related biomarkers analysis
Biomarker concentrations of healthy and hypoglycemic calves are shown in Table 2. S100B, GFAP, UCHL-1, CK-BB (p < 0.001) and NSE (p < 0.05) concentrations of hypoglycemic calves were significantly higher than the control group. ACT concentrations were significantly lower in hypoglycemic calves compared to healthy calves. There was no significant change in AM concentrations between hypoglycemic and healthy calves (p > 0.05) (Table 2). Additionally, no significant difference in brain-related biomarker concentrations was observed between mildly and severely hypoglycemic calves (Table 3).
3.4. Correlation analysis
There was a negative correlation between blood glucose concentration and serum S100B, GFAP, UCHL-1 concentration and CK-BB enzyme activity, and positive correlation with ACT (p < 0.01) (Figure 1). There was no association between glucose concentration and serum AM and NSE.
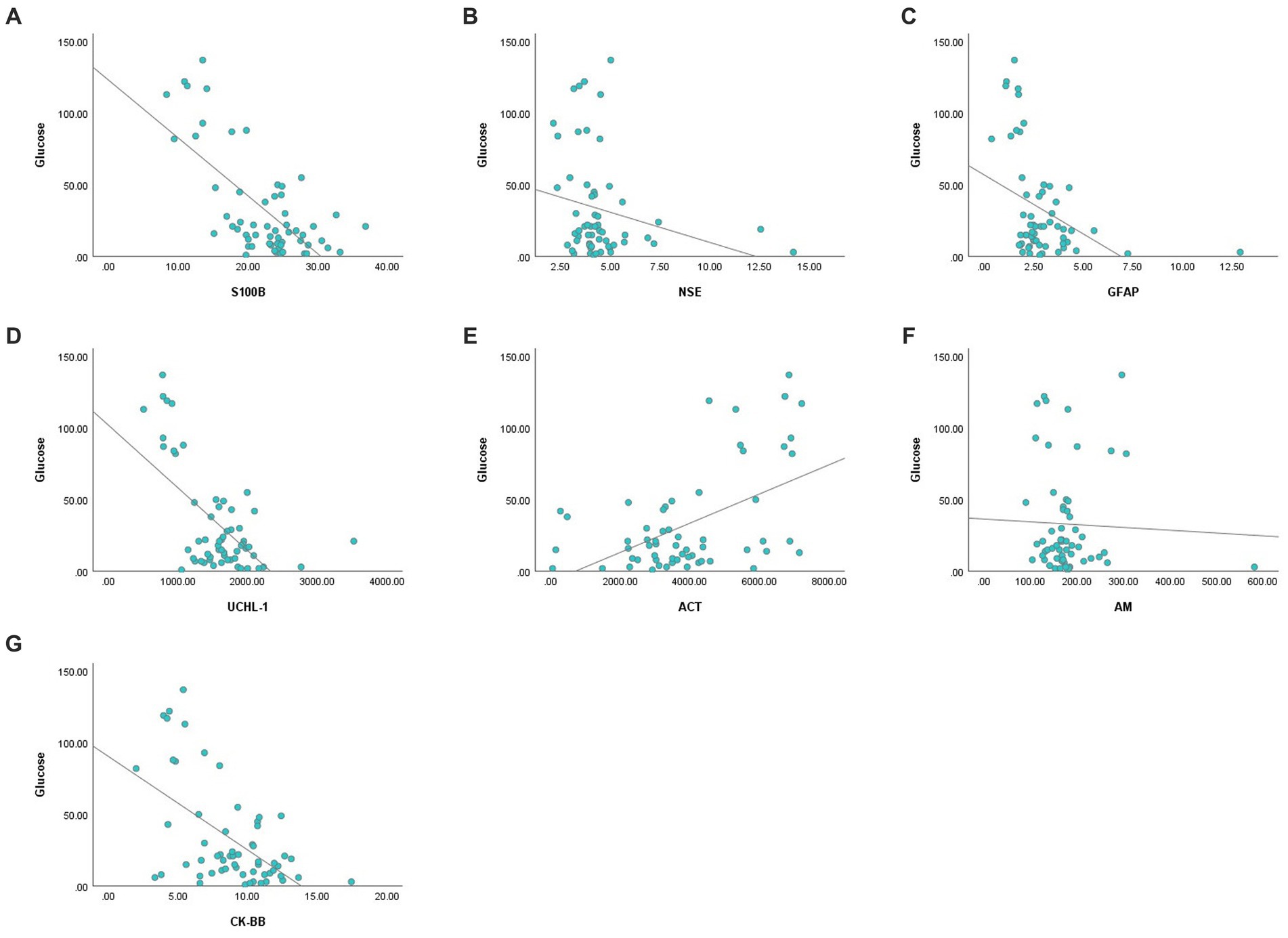
Figure 1. Correlation analysis graphs between blood glucose and serum concentrations of S100B (A), NSE (B), GFAP (C), UCHL-1 (D), ACT (E), AM (F), and CK-BB (G).
3.5. Prognostic indicators analysis
3.5.1. Brain-related biomarker
None of S100B, NSE, GFAP, UCHL-1, ACT, AM, and CK-BB were found to be significant (p > 0.05) in predicting mortality in calves with hypoglycemia (Table 4; Figure 2).
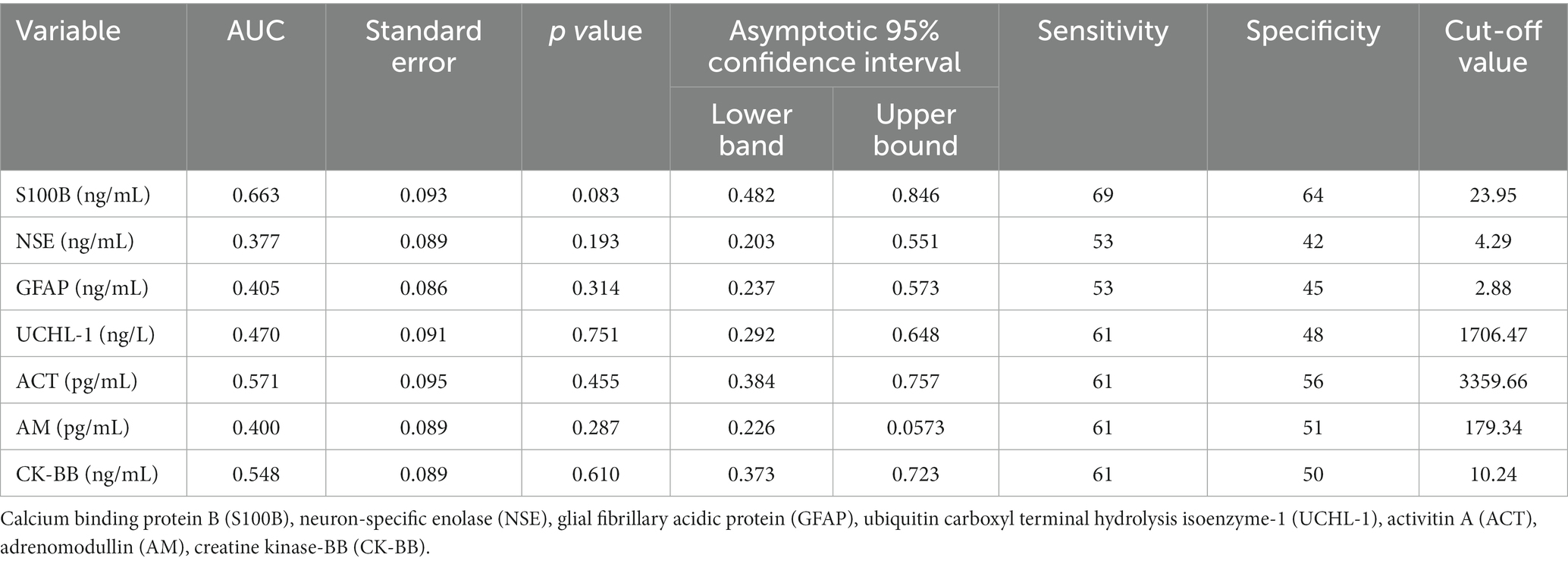
Table 4. The area under the curve (AUC), standard error, confidence interval (95%), optimal cut-off values, and corresponding sensitivity and specificity for predicting mortality in non-surviving calves with hypoglycemia.
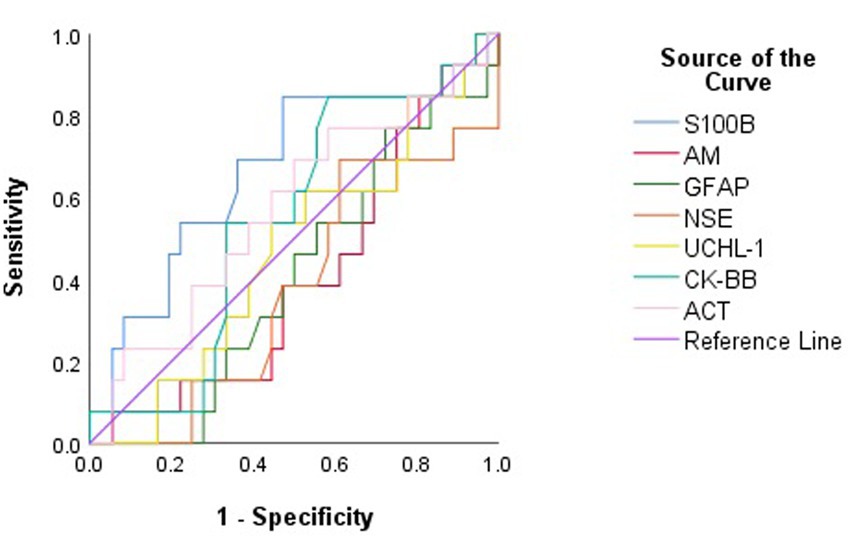
Figure 2. Receiver operating characteristic (ROC) curve analysis to discriminate between surviving and non-surviving hypoglycemic calves based on serum concentrations of brain biomarkers.
3.5.2. Logistic regression analysis and capacity of the model
Logistic regression analysis showed that the presence of sepsis, severe acidosis (pH < 7.20), and severe hypoglycemia (glucose <36 mg/dL) were not significantly associated with mortality when each variable was included in the analysis separately (Table 5). Furthermore, when sepsis, severe acidosis, and severe hypoglycemia were considered together, this model was found to be inadequate in predicting mortality (R chi-squared = 2.789, p = 0.425).
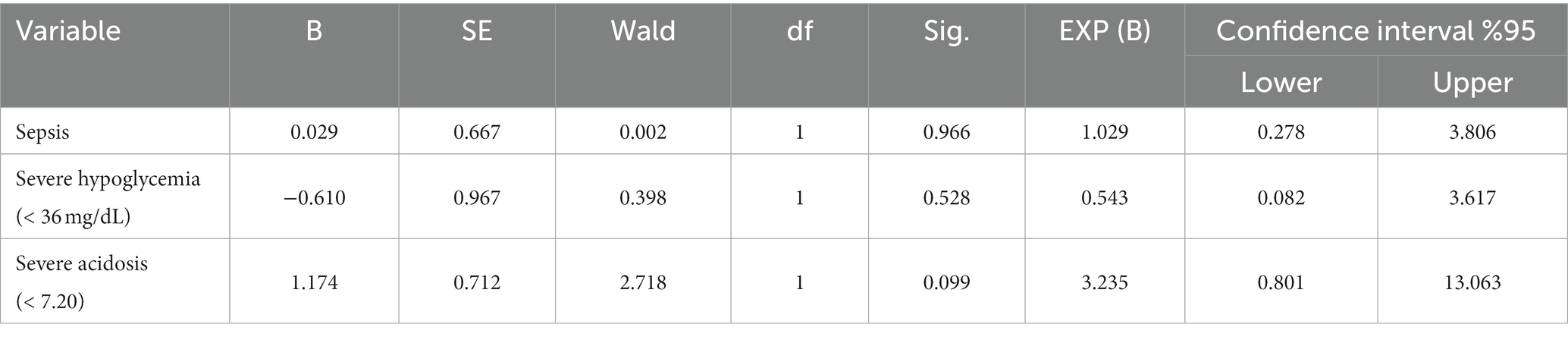
Table 5. Logistic regression for mortality comparing sepsis, severe hypoglycemia, and severe acidosis in hypoglycemic calves.
The ROC curve for sepsis to predict mortality showed an area under the curve (AUC) (95% CI) of 0.512 (0.328–0.697), with a sensitivity of 48% and a specificity of 54%. For severe hypoglycemia, the AUC (95% CI) was 0.496 (0.312–0.680), with 83% sensitivity and 16% specificity. For severe acidosis, the AUC (95% CI) was 0.621 (0.438–0.803), with a sensitivity of 70% and a specificity of 54% (Figure 3).
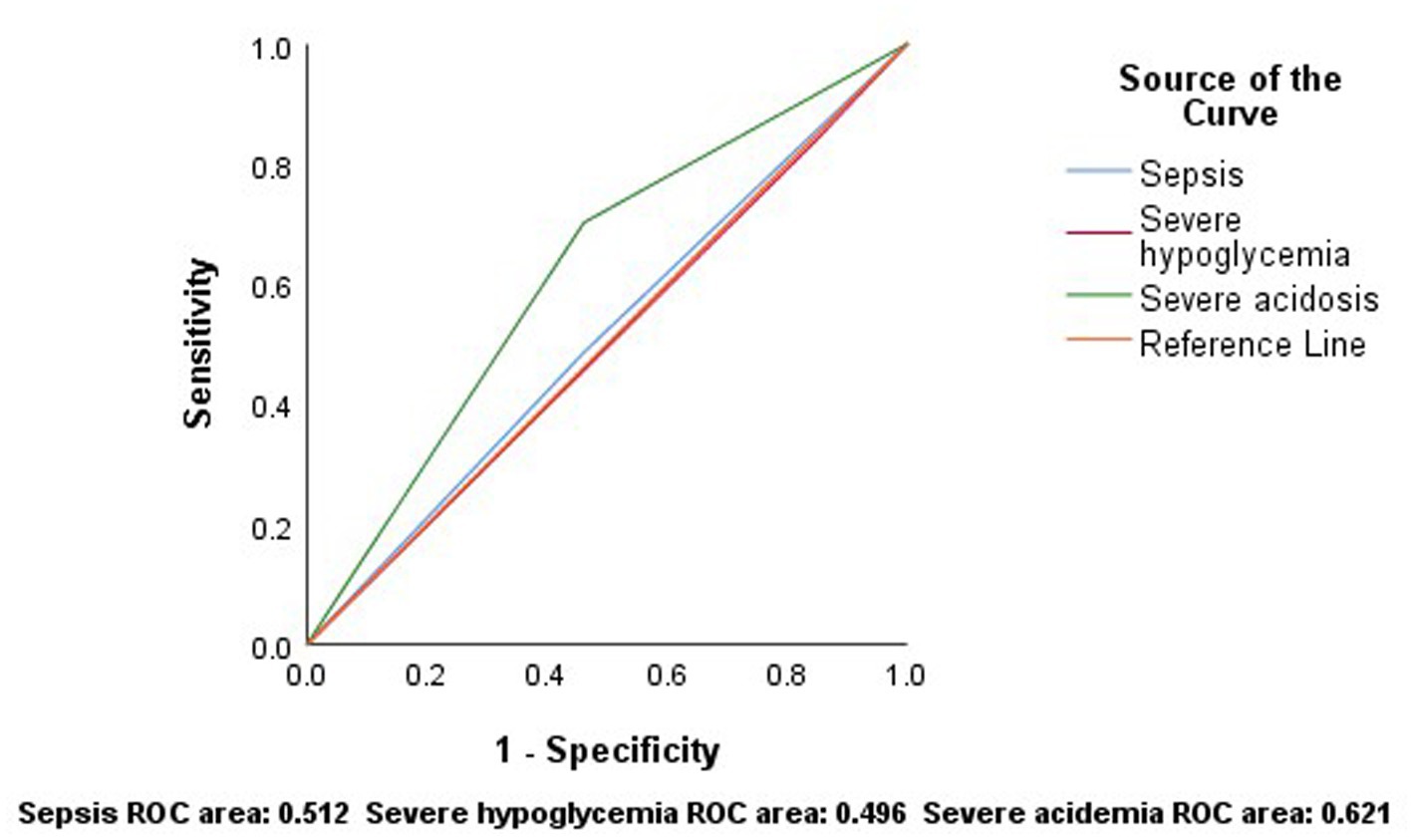
Figure 3. According to the results of ROC analysis, sepsis, severe hypoglycemia and severe acidosis have low sensitivity and specificity in predicting mortality.
4. Discussion
In this study, S100B, NSE, GFAP, UCHL-1, ACT, AM concentrations, and CK-BB enzyme activity were measured in blood serum samples from hypoglycemic calves with neonatal diarrhea. Our results showed that significant changes in S100B, NSE, GFAP, UCHL-1, ACT concentrations, and CK-BB enzyme activity occurred in calves with diarrhea related to hypoglycemia, and hypoglycemia was associated with high mortality. However, the biomarkers of brain injury were not useful in the prediction of mortality in calves with hypoglycemia. In addition, severe hypoglycemia, severe acidosis and sepsis variables were found to be insufficient to predict mortality alone or together.
Hypoglycemia in calves is a condition resulting from neonatal diarrhea, endotoxemia and asphyxia and is associated with mortality (4, 19, 23). The poor prognosis of severely hypoglycemic calves is explained by concurrent health problems including diffuse peritonitis, septicemia and acidosis (4). Trefz et al. (4) found that the survival rate was 74.0% for normoglycemic calves and 20.6% for calves with severe hypoglycemia. In the study, the survival rates of calves with plasma glucose concentrations <1 mmol/L and 1–1.9 mmol/L were 9.6 and 26.4%, respectively. In another study, the mortality rate of calves with severe hypoglycemia was reported to be 79.4% (19). In the present study, 26% of 50 hypoglycemic calves survived and 74% died. In addition, severe acidosis was observed in 64% and sepsis in 48% of hypoglycemic calves. The high mortality rate of hypoglycemic calves in our study may be related to malnutrition, sepsis and metabolic acidosis (4).
Hypoglycemia in humans and newborn calves is usually asymptomatic. Similar to previous studies (4, 24, 25), only 12% of enrolled calves had CNS-related clinical signs. The lower incidence of clinical signs related to the CNS in hypoglycemic calves may be explained by the fact that newborn dogs and calves are more tolerant to the deleterious effects of hypoglycemia due to their ability to use L-lactate as a fuel for the brain (26, 27).
Considering the potential adverse effects of hypoglycemia on the brain, this study investigated brain damage biomarkers in hypoglycemic calves with neonatal diarrhea. Because there is limited research on these biomarkers in the veterinary setting, our findings are discussed with the human literature.
Concurrent increases in the concentrations of S100B (released from astrocytes and oligodendrocytes) and NSE (released from neurons) have been interpreted as indicators of brain damage (28, 29). However, data on the relationship between these biomarkers and brain damage due to glucose dysregulation is limited. While serum S100B concentrations do not change in diabetic patients with metabolically impaired BBB (30), elevated S100B and NSE concentrations after severe hypoglycemia have been evaluated as indicators of permanent neurological damage (31). Higher concentrations of S100B and NSE have been reported in hypoglycemic children admitted to the pediatric intensive care unit (8). In addition, in vitro studies have shown that long-term glucose deprivation increases S100B and NSE release independently of hypoxia (32–34). In this study, hypoglycemic calves had significantly higher S100B and NSE concentrations than healthy calves (p < 00.5). Simultaneous elevation of S100B and NSE concentrations in hypoglycemic calves has been associated with the development of brain damage (8, 31–34). However, as S100B and NSE are not entirely brain specific, it should not be overlooked that they may also originate from peripheral tissues (35).
Astrocytes play a critical role in maintaining neuronal homeostasis in the brain by providing alternative fuel to neurons under hypoglycemic conditions (36, 37). Glial fibrillary acidic protein (GFAP), an intermediate cytoskeletal filament protein specific for astrocytes, is a key indicator of astrocyte activation. Expression of this protein outside the CNS is quite low, and the main causes of high serum concentrations are astrocyte activation after brain injury and regional necrosis (13). It has been reported that hypoglycemia-associated brain damage developed and GFAP release increased in rats experimentally induced with hypoglycemia (38). Similarly, an increase in the number of GFAP-positive astrocytes was found in rats with transient hypoglycemic coma (11). In contrast, GFAP expression was found to decrease in rat astrocytes with hyperglycemia (36, 37), and GFAP expression increased after correction of hyperglycemia (37). The authors suggest that astrocytes play a neuroprotective role during abnormal glucose homeostasis (36, 37). In the present study, GFAP concentrations were significantly higher in hypoglycemic calves than in healthy calves (p < 0.05). High GFAP concentrations have been associated with hypoglycemia-induced glial damage, astrocyte activation (11, 13) and neuroprotective role (36, 37).
Ubiquitin C-terminal hydrolase-1 (UCHL-1) is a highly abundant protein in neurons and neuroendocrine cells, constituting up to 5–10% of total neuronal proteins (14). Elevated concentrations in blood and cerebrospinal fluid (CSF) are associated with neuronal damage and increased permeability of the BBB (39). In a study of asphyxiated calves, elevated concentrations were associated with hypoxic–ischemic encephalopathy (12). It has also been reported to play a neuroprotective role in the repair process of damaged axons and neurons (40). In the present study, UCHL-1 concentrations in hypoglycemic calves were significantly higher than in healthy calves (p < 0.05). The high UHCL-1 concentrations in hypoglycemic calves may be due to neuroprotective properties rather than neuronal damage. In addition, it should be considered that neuroendocrine cells may be responsible for high UHCL-1 concentrations in hypoglycemic calves (14).
Activin A (ACT) has been shown as a neuronal protector in many CNS disorders (15, 41). In vitro and clinical studies have reported that high glucose concentrations increase ACT release (42–44). In contrast, ACT expression has been found to decrease after oxygen–glucose deprivation (10). Investigators (10, 42–44) have suggested a protective role for ACTs against the deleterious effects of inflammation, oxidative stress, and glucose dysregulation. In calves with perinatal asphyxia, low ACT concentrations were associated with species difference, oxidative stress, and overuse due to its role in repair (12). In the present study, serum ACT concentrations were found to be lower in hypoglycemic calves compared to healthy calves (p < 0.05). Low ACT concentrations in hypoglycemic calves may be associated with oxidative stress and neuroprotective properties (10, 12, 42, 43).
In hypoxic ischemia and hypoglycemia, increased AM expression has been observed in central cortical neurons, endothelial and perivascular glial cells (9). Similarly, a significant increase in plasma AM concentrations has been reported in hyperglycemic infants (45). However, no significant change in circulating AM concentrations was observed in insulin-induced hypoglycemia (46). This has been attributed to factors such as AM being unstable, having a short half-life of 20 min, and binding to some proteins in the circulation (47, 48). On the other hand, it has been suggested that AM concentrations are not affected by plasma glucose concentrations (49, 50). In the present study, there was no statistically significant difference in AM concentrations and no correlation with glucose concentrations (p > 0.05). These results suggest that AM does not play a role in the regulatory hormonal response to hypoglycemia (46, 49).
CK isoenzyme BB (CK-BB) is found at high levels in the brain and its activity is increased in peripheral blood in brain injury (17, 51). CK-BB is expressed by astrocytic glial cells (16). Experimentally, CK-BB enzyme activity was found to increase during insulin-induced hypoglycemia and showed a positive correlation with insulin dose (17). The increase in CK-BB enzyme activity in hypoglycemic cases has been related to the role of CK in brain energy demand and ATP production (brain energy hemostasis) (16, 52, 53). In the present study, the serum CK-BB enzyme activity of hypoglycemic calves was higher than that of healthy calves (p < 0.05). The elevated CK-BB enzyme activity in hypoglycemic calves is thought to be a response to brain energy and ATP demands (16, 52, 53) due to increased glycolysis, oxidative phosphorylation and sympathetic activation as a result of hypoglycemia.
Acute hypoglycemia alters blood levels of brain-derived proteins due to brain damage and BBB dysfunction caused by endothelial dysfunction and increased oxidative stress (6, 13). In the present study, blood glucose concentrations correlated negatively with S100B, GFAP, UCHL-1 concentrations and CK-BB enzyme activity and positively with ACT concentration. The simultaneous increase or decrease in serum concentrations of brain damage biomarkers and the significant correlation of these biomarkers with blood glucose concentrations suggest that brain damage develops in hypoglycemic calves (S100B, NSE, GFAP) and neuroprotective mechanisms (GFAP, UCHL-1, ACT, CK-BB) are activated to prevent damage (13).
Histopathologically, severe degeneration of glial cells (astrocytes, oligodendrocytes) occurs as a result of hypoglycemia, whereas ischemic neuronal damage is rare (7). Assessing the cell types from which brain-derived proteins originate in the present study, it can be assumed that glial cell damage (S100B, GFAP, CK-BB) rather than neuronal damage (NSE, UCHL-1) occurs in hypoglycemic calves (7). However, histopathology studies are required to prove this in hypoglycemic calves.
In the present study, although there was a significant difference in brain injury biomarker concentrations between healthy and hypoglycemic calves, these biomarker concentrations were not good indicators for predicting mortality. Therefore, to determine the importance of other clinicopathologic variables on mortality, a logistic regression model was constructed. In the present study, the variables of severe hypoglycemia, severe acidosis, and sepsis were not significant in predicting prognosis when evaluated alone or together. Furthermore, when assessing model capacity, severe hypoglycemia, severe acidosis, and sepsis had low sensitivity and specificity in predicting mortality in hypoglycemic calves. These results are consistent with the view that laboratory parameters have limited value in predicting mortality, but the presence of specific clinical abnormalities provides valuable prognostic information (19). Based on our clinical experience, the survival of all calves within the first 12 h indicates that the duration of hypoglycemia could have a direct effect on mortality.
However, the study has some limitations, including (i) the absence of histopathologic evaluation of hypoglycemic calves for brain damage, (ii) the lack of measurement of CSF concentrations of the biomarkers used, and (iii) no inclusion of a group of non-hypoglycemic calves with diarrhea. All these aspects deserve to be addressed in further studies.
The results from the present study conclude that hypoglycemia-associated brain damage developed in hypoglycemic calves with diarrhea. This damage occurred in glial cell populations rather than neurons and caused changes in serum concentrations of brain biomarkers. In addition, hypoglycemia increased mortality, but biomarkers of brain injury were not useful in predicting mortality due to low sensitivity and specificity, and severe hypoglycemia, severe acidosis, and sepsis variables alone or together were not effective in predicting mortality.
Data availability statement
The raw data supporting the conclusions of this article will be made available by the authors, without undue reservation.
Ethics statement
The animal study was reviewed and approved by Ethics Committee of the Faculty of Veterinary Medicine, Selcuk University. The studies were conducted in accordance with the local legislation and institutional requirements. Written informed consent was obtained from the owners for the participation of their animals in this study.
Author contributions
MI and AN: writing—original draft preparation, conceptualization, and methodology. MI and AE: investigation. SSI and MKD: data curation. MO: writing—review and editing. All authors contributed to the article and approved the submitted version.
Funding
This research was supported by the Selcuk University Scientific Research Project Office with project number 22401100.
Conflict of interest
The authors declare that the research was conducted in the absence of any commercial or financial relationships that could be construed as a potential conflict of interest.
Publisher’s note
All claims expressed in this article are solely those of the authors and do not necessarily represent those of their affiliated organizations, or those of the publisher, the editors and the reviewers. Any product that may be evaluated in this article, or claim that may be made by its manufacturer, is not guaranteed or endorsed by the publisher.
References
1. Boranbayeva, T, Karahan, AG, Tulemissova, Z, Myktybayeva, R, and Ozkaya, S. Properties of a new probiotic candidate and Lactobacterin-Tk2 against diarrhea in calves. Probiot Antimicro. (2020) 12:918–28. doi: 10.1007/s12602-020-09649-4
2. Trefz, FM, Constable, PD, Sauter-Louis, C, Lorch, A, Knubben-Schweizer, G, and Lorenz, I. Hyperkalemia in neonatal diarrheic calves depends on the degree of dehydration and the cause of the metabolic acidosis but does not require the presence of Acidemia. J Dairy Sci. (2013) 96:7234–44. doi: 10.3168/jds.2013-6945
3. Trefz, FM, Constable, PD, and Lorenz, I. Quantitative physicochemical analysis of Acid-Base balance and clinical utility of anion gap and strong ion gap in 806 neonatal calves with diarrhea. J Vet Intern Med. (2015) 29:678–87. doi: 10.1111/jvim.12556
4. Trefz, FM, Feist, M, and Lorenz, I. Hypoglycaemia in hospitalised neonatal calves: prevalence, associated conditions and impact on prognosis. Vet J. (2016) 217:103–8. doi: 10.1016/j.tvjl.2016.10.001
5. Mohseni, S. Neurologic damage in hypoglycemia. Handb Clin Neurol. (2014) 126:513–32. doi: 10.1016/B978-0-444-53480-4.00036-9
6. Sajja, RK, Green, KN, and Cucullo, L. Altered Nrf2 signaling mediates hypoglycemia-induced blood-brain barrier endothelial dysfunction in vitro. PLoS One. (2015) 10:e0122358. doi: 10.1371/journal.pone.0122358
7. Vannucci, RC, and Vannucci, SJ. Hypoglycemic brain injury. Semin Neonatol. (2001) 6:147–55. doi: 10.1053/siny.2001.0044
8. Vanhorebeek, I, Gielen, M, Boussemaere, M, Wouters, PJ, Grandas, FG, Mesotten, D, et al. Glucose dysregulation and neurological injury biomarkers in critically ill children. J Clin Endocrinol Metab. (2010) 95:4669–79. doi: 10.1210/jc.2010-0805
9. Serrano, J, Alonso, D, Fernandez, AP, Encinas, JM, Lopez, JC, Castro-Blanco, S, et al. Adrenomedullin in the central nervous system. Microsc Res Tech. (2002) 57:76–90. doi: 10.1002/jemt.10053
10. Xu, G, He, J, Guo, H, Mei, C, Wang, J, Li, Z, et al. Activin a prevents neuron-like Pc12 cell apoptosis after oxygen-glucose deprivation. Neural Regen Res. (2013) 8:1016–24. doi: 10.3969/j.issn.1673-5374.2013.11.007
11. Tomita, N, Nakamura, T, Sunden, Y, and Morita, T. Histopathological and Immunohistochemical analysis of the cerebral white Matter after transient hypoglycemia in rat. J Vet Med Sci. (2020) 82:68–76. doi: 10.1292/jvms.19-0502
12. Ok, M, Naseri, A, Ates, MB, Ider, M, Uney, K, Sevinc, M, et al. The usefulness of serum brain damage biomarkers in detection and evaluation of hypoxic ischemic encephalopathy in calves with perinatal asphyxia. Animals. (2022) 12:3223. doi: 10.3390/ani12223223
13. Kawata, K, Liu, CY, Merkel, SF, Ramirez, SH, Tierney, RT, and Langford, D. Blood biomarkers for brain injury: what are we measuring? Neurosci Biobehav R. (2016) 68:460–73. doi: 10.1016/j.neubiorev.2016.05.009
14. Day, INM, and Thompson, RJ. Uchl1 (Pgp 9.5): Neuronal Biomarker and Ubiquitin System Protein. Prog Neurobiol. (2010) 90:327–62. doi: 10.1016/j.pneurobio.2009.10.020
15. Florio, P, Gazzolo, D, Luisi, S, and Petraglia, F. Activin a in brain injury. Adv Clin Chem. (2007) 43:117–30. doi: 10.1016/S0065-2423(06)43004-3
16. Tachikawa, M, Fukaya, M, Terasaki, T, Ohtsuki, S, and Watanabe, M. Distinct cellular expressions of Creatine synthetic enzyme Gamt and Creatine kinases Uck-mi and Ck-B suggest a novel neuron-glial relationship for brain energy homeostasis. Eur J Neurosci. (2004) 20:144–60. doi: 10.1111/j.1460-9568.2004.03478.x
17. Mitani, S, Okumura, K, Matsui, H, Toki, Y, Hashimoto, H, Ito, T, et al. Insulin alters cardiac muscle Creatine kinase activity. Heart Vessel. (2000) 15:23–9. doi: 10.1007/s003800070044
18. Aiello, SE, Moses, MA, and Allen, DG. The Merck veterinary manual: Merck & Company. NJ, USA: Incorporated White Station (2016).
19. Trefz, FM, Lorenz, I, Lorch, A, and Constable, PD. Clinical signs, profound Acidemia, hypoglycemia, and hypernatremia are predictive of mortality in 1,400 critically ill neonatal calves with diarrhea. PLoS One. (2017) 12:e0182938. doi: 10.1371/journal.pone.0182938
20. Smith, G, Gunn, A, McGuirk, S, and Izoo, M. Manifestations and Management of Disease in neonatal ruminants. Large animal internal medicine (5th Edn.). Elseiver, New York, (2015): 303–306.
21. Trefz, FM, Lorenz, I, and Constable, PD. Effects of profound Acidemia on the dynamic glucose and insulin response and plasma potassium and phosphorus concentrations during an intravenous glucose tolerance test in neonatal calves. J Dairy Sci. (2017) 100:9163–76. doi: 10.3168/jds.2017-12690
22. Naseri, A, Sen, I, Turgut, K, Guzelbektes, H, and Constable, PD. Echocardiographic assessment of left ventricular systolic function in neonatal calves with naturally occurring Sepsis or septic shock due to diarrhea. Res Vet Sci. (2019) 126:103–12. doi: 10.1016/j.rvsc.2019.08.009
23. Ballou, MA, Cobb, CJ, Hulbert, LE, and Carroll, JA. Effects of intravenous Escherichia Coli dose on the pathophysiological response of colostrum-fed Jersey calves. Vet Immunol Immunopathol. (2011) 141:76–83. doi: 10.1016/j.vetimm.2011.02.008
24. Tennant, B, Harrold, D, and Reina-Guerra, M. Hypoglycemia in neonatal calves associated with acute diarrhea. Cornell Vet. (1968) 58:136–46.
25. Klein, KA, Clark, C, and Allen, AL. Hypoglycemia in sick and moribund farmed elk calves. Can Vet J. (2002) 43:778–81.
26. Gardiner, RM. The effects of Hypoglycaemia on cerebral blood flow and metabolism in the new-born calf. J Physiol. (1980) 298:37–51. doi: 10.1113/jphysiol.1980.sp013065
27. Hernandez, MJ, Vannucci, RC, Salcedo, A, and Brennan, RW. Cerebral blood flow and metabolism during hypoglycemia in newborn dogs. J Neurochem. (1980) 35:622–8. doi: 10.1111/j.1471-4159.1980.tb03701.x
28. Kapural, M, Krizanac-Bengez, L, Barnett, G, Perl, J, Masaryk, T, Apollo, D, et al. Serum S-100 Beta as a possible marker of blood-brain barrier disruption. Brain Res. (2002) 940:102–4. doi: 10.1016/S0006-8993(02)02586-6
29. Marchi, N, Cavaglia, M, Fazio, V, Bhudia, S, Hallene, K, and Janigro, D. Peripheral markers of blood-brain barrier damage. Clin Chim Acta. (2004) 342:1–12. doi: 10.1016/j.cccn.2003.12.008
30. Hovsepyan, MR, Haas, MJ, Boyajyan, AS, Guevorkyan, AA, Mamikonyan, AA, Myers, SE, et al. Astrocytic and neuronal biochemical markers in the sera of subjects with diabetes mellitus. Neurosci Lett. (2004) 369:224–7. doi: 10.1016/j.neulet.2004.07.071
31. Strachan, MW, Abraha, HD, Sherwood, RA, Lammie, GA, Deary, IJ, Ewing, FM, et al. Evaluation of serum markers of neuronal damage following severe hypoglycaemia in adults with insulin-treated diabetes mellitus. Diabetes Metab Res Rev. (1999) 15:5–12. doi: 10.1002/(sici)1520-7560(199901/02)15:1<5::aid-dmrr2>3.0.co;2-s
32. Fontella, FU, Cimarosti, H, Crema, LM, Thomazi, AP, Leite, MC, Salbego, C, et al. Acute and repeated restraint stress influences cellular damage in rat hippocampal slices exposed to oxygen and glucose deprivation. Brain Res Bull. (2005) 65:443–50. doi: 10.1016/j.brainresbull.2005.02.026
33. Gerlach, R, Demel, G, Konig, HG, Gross, U, Prehn, JH, Raabe, A, et al. Active secretion of S100b from astrocytes during metabolic stress. Neuroscience. (2006) 141:1697–701. doi: 10.1016/j.neuroscience.2006.05.008
34. Gursoy, M, and Buyukuysal, RL. Mechanism of S100b release from rat cortical slices determined under basal and stimulated conditions. Neurochem Res. (2010) 35:429–36. doi: 10.1007/s11064-009-0075-9
35. Leviton, A, and Dammann, O. Brain damage markers in children. Neurobiol Clinical Aspects Acta Paediatr. (2002) 91:9–13. doi: 10.1080/080352502753457851
36. Afsari, ZH, Renno, WM, and Abd-El-Basset, E. Alteration of glial fibrillary acidic proteins immunoreactivity in astrocytes of the spinal cord diabetic rats. Anat Rec. (2008) 291:390–9. doi: 10.1002/ar.20678
37. Hashish, H. Alteration of glial fibrillary acidic protein immunoreactivity in astrocytes of the cerebellum of diabetic rats and potential effect of insulin and ginger. Anat Physiol. (2015) 5:167. doi: 10.4172/2161-0940.1000167
38. Sahin, K, Tuzcu, M, Orhan, C, Ali, S, Sahin, N, Gencoglu, H, et al. Chromium modulates expressions of neuronal plasticity markers and glial fibrillary acidic proteins in hypoglycemia-induced brain injury. Life Sci. (2013) 93:1039–48. doi: 10.1016/j.lfs.2013.10.009
39. Mondello, S, Palmio, J, Streeter, J, Hayes, RL, Peltola, J, and Jeromin, A. Ubiquitin Carboxy-terminal hydrolase L1 (Uch-L1) is increased in cerebrospinal fluid and plasma of patients after epileptic seizure. BMC Neurol. (2012):12. doi: 10.1186/1471-2377-12-85
40. Matuszczak, E, Tylicka, M, Komarowska, MD, Debek, W, and Hermanowicz, A. Ubiquitin Carboxy-terminal hydrolase L1- physiology and pathology. Cell Biochem Funct. (2020) 38:533–40. doi: 10.1002/cbf.3527
41. Mukerji, SS, Rainey, RN, Rhodes, JL, and Hall, AK. Delayed Activin a administration attenuates tissue death after transient focal cerebral ischemia and is associated with decreased stress-responsive kinase activation. J Neurochem. (2009) 111:1138–48. doi: 10.1111/j.1471-4159.2009.06406.x
42. Andersen, GO, Ueland, T, Knudsen, EC, Scholz, H, Yndestad, A, Sahraoui, A, et al. Activin a levels are associated with abnormal glucose regulation in patients with myocardial infarction: potential counteracting effects of Activin a on inflammation. Diabetes. (2011) 60:1544–51. doi: 10.2337/db10-1493
43. Brown, ML, Ungerleider, N, Bonomi, L, Andrzejewski, D, Burnside, A, and Schneyer, A. Effects of Activin a on survival, function and gene expression of pancreatic islets from non-diabetic and diabetic human donors. Islets. (2014) 6:e1017226. doi: 10.1080/19382014.2015.1017226
44. Wu, H, Mezghenna, K, Marmol, P, Guo, T, Moliner, A, Yang, SN, et al. Differential regulation of mouse pancreatic islet insulin secretion and Smad proteins by Activin ligands. Diabetologia. (2014) 57:148–56. doi: 10.1007/s00125-013-3079-6
45. El-Habashy, SA, Matter, RM, El-Hadidi, ES, and Afifi, HR. Plasma Adrenomedullin level in Egyptian children and adolescents with type 1 diabetes mellitus: relationship to microvascular complications. Diabetol Metab Syndr. (2010) 2:12. doi: 10.1186/1758-5996-2-12
46. Ehlenz, K, Koch, B, Preuss, P, Simon, B, Koop, I, and Lang, RE. High levels of circulating Adrenomedullin in severe illness: correlation with C-reactive protein and evidence against the adrenal medulla as site of origin. Exp Clin Endocr Diab. (1997) 105:156–62. doi: 10.1055/s-0029-1211745
47. Hirayama, N, Kitamura, K, Imamura, T, Kato, J, Koiwaya, Y, and Eto, T. Secretion and clearance of the mature form of Adrenomedullin in humans. Life Sci. (1999) 64:2505–9. doi: 10.1016/s0024-3205(99)00208-8
48. Morgenthaler, NG, Struck, J, Alonso, C, and Bergmann, A. Measurement of Midregional Proadrenomedullin in plasma with an Immunoluminometric assay. Clin Chem. (2005) 51:1823–9. doi: 10.1373/clinchem.2005.051110
49. Garcia-Unzueta, MT, Montalban, C, Pesquera, C, Berrazueta, JR, and Amado, JA. Plasma Adrenomedullin levels in type 1 diabetes-relationship with clinical parameters. Diabetes Care. (1998) 21:999–1003. doi: 10.2337/diacare.21.6.999
50. Nakamura, T, Honda, K, Ishikawa, S, Kitamura, K, Eto, T, and Saito, T. Plasma Adrenomedullin levels in patients with non-insulin dependent diabetes mellitus: close relationships with diabetic complications. Endocr J. (1998) 45:241–6. doi: 10.1507/endocrj.45.241
51. Lyons, SA, and Kettenmann, H. Oligodendrocytes and microglia are selectively vulnerable to combined hypoxia and hypoglycemia injury in vitro. J Cereb Blood Flow Metab. (1998) 18:521–30. doi: 10.1097/00004647-199805000-00007
52. Jiang, ZL, Harada, T, Yokokawa, M, Kohzuki, M, and Sato, T. Muscle damage induced by experimental hypoglycemia. Metabolism. (1998) 47:1472–6. doi: 10.1016/s0026-0495(98)90072-5
Keywords: hypoglycemia, neonatal calf, brain injury, biomarkers, prognosis
Citation: Ider M, Naseri A, Ok M, Erturk A, Durgut MK and Iyigun SS (2023) Surveilling brain damage using brain biomarkers in hypoglycemic neonatal calves with diarrhea. Front. Vet. Sci. 10:1240846. doi: 10.3389/fvets.2023.1240846
Edited by:
Tabaran Alexandru Flaviu, University of Agricultural Sciences and Veterinary Medicine of Cluj-Napoca, RomaniaReviewed by:
Derek Foster, North Carolina State University, United StatesAhmet Kursat Azkur, Kırıkkale University, Türkiye
Copyright © 2023 Ider, Naseri, Ok, Erturk, Durgut and Iyigun. This is an open-access article distributed under the terms of the Creative Commons Attribution License (CC BY). The use, distribution or reproduction in other forums is permitted, provided the original author(s) and the copyright owner(s) are credited and that the original publication in this journal is cited, in accordance with accepted academic practice. No use, distribution or reproduction is permitted which does not comply with these terms.
*Correspondence: Merve Ider, bS5pZGVyQHNlbGN1ay5lZHUudHI=