- 1Departamento de Medicina Preventiva Animal, Facultad de Ciencias Veterinarias y Pecuarias, Universidad de Chile, Santiago, Chile
- 2Veterinary Population Medicine, College of Veterinary Medicine, University of Minnesota, Saint Paul, MN, United States
- 3Department of Veterinary and Biomedical Sciences, Animal Disease Research and Diagnostic Laboratory, South Dakota State University, Brookings, SD, United States
- 4Department of Primary Industries, Veterinary Research Office, Menangle, NSW, Australia
Rotavirus A (RVA) is a common cause of diarrhea in newborn pigs, leading to significant economic losses. RVA is considered a major public health concern due to genetic evolution, high prevalence, and pathogenicity in humans and animals. The objective of this study was to identify and characterize RVA in swine farms in Chile. A total of 154 samples (86 oral fluids and 68 fecal samples) were collected, from 22 swine farms. 58 (38%) samples belonging to 14 farms were found positive for RVA by real-time RT-PCR. The samples with low Ct values (21) and the two isolates were selected for whole genome sequencing. Nearly complete genomes were assembled from both isolates and partial genomes were assembled from five clinical samples. BLAST analysis confirmed that these sequences are related to human and swine-origin RVA. The genomic constellation was G5/G3-P[7]-I5-R1-C1-M1-A8-N1-T1-E1-H1. Phylogenetic analysis showed that VP4, VP1, VP2, NSP2, NSP3, NSP4, and NSP5 sequences were grouped in monophyletic clusters, suggesting a single introduction. The phylogenies for VP7, VP6, VP3, and NSP1 indicated two different origins of the Chilean sequences. The phylogenetic trees showed that most of the Chilean RVA sequences are closely related to human and swine-origin RVA detected across the world. The results highlight the potential zoonotic nature of RVA circulating in Chilean swine farms. Therefore, it is important to continue RVA whole genome sequencing globally to fully understand its complex epidemiology and early detection and characterization of zoonotic strains.
1. Introduction
Rotavirus A (RVA), a member of the Sedoreoviridae family, genus Rotavirus, is a non-enveloped, icosahedral virus measuring approximately 80 nm in diameter (1). It possesses a triple capsid structure (1, 2). RVA is known to be a causative agent of severe intestinal disease and a leading cause of mortality of 200,000 people per year, especially in children under 5 years of age (3, 4). Furthermore, RVA is commonly associated with diarrhea in farm animals. In swine populations, it is a prevalent pathogen causing gastroenteritis, particularly in newborn pigs (5). The transmission of RVA occurs through the fecal-oral route, resulting in the shortening of villi, sparse and irregular microvilli, and mononuclear cell infiltration of the lamina propria of small intestinal enterocytes (6).
The genome of RVA consists of 11 linear segments of dsRNA that encode 12 structural and non-structural proteins (2). Due to its high prevalence and pathogenicity in both humans and animals, RVA is considered a significant public health concern (4, 7). Traditionally, the genotyping of RVA has been based on the structural proteins VP7 and VP4, denoted as Gx-P[x]. Currently, there are 42G genotypes and 58 P genotypes identified (8).1 However, for the comparison of rotavirus genomes, the classification Gx-P[x]Ix-Rx-Cx-Mx-Ax-Nx-Tx-Ex-Hx is commonly employed, representing the VP7-VP4-VP6-VP1-VP2-VP3-NSP1-NSP2-NSP3-NSP4-NSP5/6 genes, respectively (9, 10). This comprehensive genome comparison enhances our understanding of RVA viral dynamics and aids in comprehending transmission and spread.
Rotavirus A exhibits considerable genetic diversity in swine, with numerous G and P genotypes identified worldwide. Twelve G genotypes (G1 to G6, G8 to G12, and G26) and 16 P genotypes (P[1] to P[8], P[11], P[13], P[19], P[23], P[26], P[27], P[32], and P[34]) of RVA have been associated with pigs (5, 11). However, it is important to note that the distribution and prevalence of specific genotypes can vary across regions and over time. Additionally, genetic variations within the RVA genome, including variations in the NSP4 and VP6 genes, contribute to the overall genetic diversity of the virus. These variations can impact virulence, host range, and the immune response to RVA infection.
Additionally, RVA has the potential for zoonotic transmission and is strong evidence of genetic similarities between human and animal strains, more evident after the full genome sequencing of strains (7, 12, 13). This highlights the importance of ongoing surveillance to assess the extent of transmission and the need for vaccination programs.
Surveillance and molecular characterization of RVA strains in swine populations play a vital role in identifying emerging strains, tracking their spread, and making informed decisions regarding prevention and control measures. However, information on porcine RVA remains limited in Chile, which is also observed for human rotaviruses. Therefore, this study aimed to identify the virus in the swine-intensive population across the country and to characterize porcine RVA through whole genome sequencing (WGS).
2. Materials and methods
2.1. Sample collection
A total of 154 samples, 86 oral fluids and 68 fecal samples were collected from 1 to 80-day-old pigs between 2015 and 2018. These samples were collected from 22 intensive swine farms located in the central-south area of Chile, including the regions Valparaiso, Metropolitan, O’Higgins, Maule, Ñuble, and Araucanía. This geographical region represents approximately 95% of the national pork production.
Fecal samples were primarily collected to determine intestinal pathogens, hence presented diarrhea, while oral fluids were collected for active surveillance of the Influenza A virus from normal pigs. Fecal samples and oral fluids were collected from different populations. Fecal samples were preserved in 10% phosphate-buffered saline (PBS) containing penicillin, streptomycin, and amphotericin B. Each sample, whether fecal or oral fluid, was subjected to centrifugation at 4,000 rpm for 5 min, followed by aliquoting the supernatant into microtubes containing Minimal Essential Medium at 50% dilution (MEM/EBSS, Cytiva, Hyclone™). The MEM was supplemented with 1X TPCK (N-tosyl-L-phenylalanine chloromethyl ketone) Trypsin, 2% Bovine Serum Albumin (BSA-50, Rockland), and 1% antifungal antibiotic solution (Pen-Strep-Amphotericin B solution, 03-033-1B, Biological Industries).
2.2. Rotavirus identification and sequencing
To detect RVA, total RNA was extracted from the samples using Chomczynski-phenol solution (Winkler, BM-1,755, Chile) as per the manufacturer’s instructions. Subsequently, a real-time reverse transcription PCR (RT-qPCR) was conducted to amplify a partial region of the VP6 gene, following the protocol described by Marthaler et al. (14). For samples that tested positive, virus isolation was attempted in MA104 cells. Briefly, MA104 cells were cultivated in MEM supplemented with 2% fetal bovine serum (04-127-1A, Biological Industries), and 2% antifungal and antibiotic solution (03-033-1B, Biological Industries) at 37°C with 5% CO2. Before infecting the MA104 cell monolayers, the cells were washed with 1X TPCK solution in PBS. Then, 250 μL of the sample was inoculated, and 2 h after inoculation at 37°C with 5% CO2, 750 μL of MEM with 2% antifungal, and antibiotic solution was added. The cells were incubated at 37°C with 5% CO2 and examined daily for cytopathic effects (CPE) using optical microscopy. At least two passages were performed and isolation was confirmed by RT-qPCR, as previously described (14).
Samples and isolates with RT-qPCR Ct values below 30 were selected for WGS using the Illumina MiSeq platform at the Molecular Diagnostic Development Laboratory, Veterinary Diagnostic Laboratory of the University of Minnesota (MVDL, UMN), USA. Viral RNA was extracted by Qiagen EZ1 nucleic acid extraction using the Virus Mini Kit v 2.0 (Qiagen, Germantown, MD, USA) followed by library preparation using the Takara Bio Clontech SMARTer Stranded total RNA Seq Pico v2 kit (Takara Bio USA, Inc. Mountain View, CA, USA) and then submitted to the University of Minnesota Genomic Centre for sequencing by Illumina Miseq 150 paired-end cycle. The raw fastq files were analyzed using Chan Zuckerberg ID2 which is an open-source, free, and cloud-based metagenomics platform (15–17). Briefly, we used the Illumina mNGS Pipeline v8.2, which integrates bioinformatic tools including but not limited to the Spliced Transcripts Alignment to a Reference (STAR) algorithm for initial validation, sequence quality was assessed using FastQC software, and Bowtie2 and HISAT2 to remove remaining host reads. Reads were aligned to the NCBI database using Minimap2 and Diamond, and de novo assembling was performed by SPADES. The assembled genomes were for genetic characterization.
To determine the genotypes of the segment sequences, the BV BRC tool for Rotavirus A classification was utilized (18). Phylogenetic analysis was conducted for all segments using reference sequences for each genotype, obtained from the NCBI Virus Variation resource.3 Also, other sequences from Chile were incorporated. The sequences were aligned using MUSCLE (19), and the best nucleotide substitution model was determined using JModeltest2 (20). Finally, maximum likelihood trees were estimated for each segment with 1,000 bootstrap replications in MEGA X (21). The construction of phylogenetic trees for each viral segment was performed using the online tool CIPRES4 (22) employing a combination of the Maximum Likelihood Tree and Neighbor-Joining Tree. Phylogenetic associations were confirmed using 1,000 bootstrap replicates.
3. Results
A total of 154 samples were tested by RT-qPCR, from them 58 (38%) tested positive. In detail, 36 out of 86 oral fluids were positive (41%) and 22 out of 68 fecal samples were positive (32%). Cycle threshold (Ct) values ranged between 14.6 and 33.7, in positive samples fecal samples, the Ct value was significative lower (average 20.3) compared with oral fluids (average 23) (p < 0.05). Fourteen out of 22 farms tested positive for RVA at least once, located in all regions in the study. Twenty-one RT-qPCR positive samples (Ct < 30) and two isolates obtained from feces, confirmed by RT-qPCR were selected for NGS, belonging to 9 different farms. The nearly complete genomes of RVA were assembled from virus isolates. Only partial segments with a sequence length between 238 and 606 nt (Supplementary Table S1) were assembled from clinical samples.
All assembled contigs (partial or complete) were analyzed using the Basic Local Alignment Search Tool (BLAST) at NCBI. The closest hits for most of the contigs were found to be related to either swine or human sequences (Table 1; Supplementary Table S1). Among the sequenced isolates, most of the segments showed similarity to porcine RVA strains collected worldwide. Three segments (VP3, NSP2, and NSP5) showed similarity to RVA strains collected from humans. Additionally, all VP2 segments showed similarity (94.2–94.8%) to equine RVA (Table 1). These sequences were submitted to GenBank under the names RVA/Swine/CHL/ROTA-1/2017 and RVA/Swine/CHL/ROTA-2/2017 (Accession numbers OR192577-OR192610).
The pairwise comparison of RVA/Swine/CHL/ROTA-1/2017 and RVA/Swine/CHL/ROTA-2/2017 segments reported an identity of 80.3 to 100% between them. Duplicate segments were collected from both isolates for some segments. In detail, for VP1 four segments were obtained, with an identity of 97.8 to 99.9%; for VP2, three segments with an identity of 97.6 to 99.9%; for VP3, four segments with an identity of 89.5 to 99.6%; for VP4 has two segments with an identity of 99.8% between them; for NSP1, two segments with an identity 87.4%; for VP6, four segments with an identity of 92.2 to 99.6%; for VP7, four segments with an identity of 80.3 to 100%; for NSP2, four segments with an identity of 87.2 to 99.7%; for NSP3, two identical segments; for NSP4, three segments with an identity of 99.4 to 100%; and NSP5 has two identical segments. The BV-BRC tool classified all internal segments as I5-R1-C1-M1-A8-N1-T1-E1-H1, which are genetically related to viruses collected from humans and swine. Using VP7 and VP4 genotyping the isolate ROTA-1 was classified as G5-P[7] and the isolate ROTA-2 presented G5-P[7] and G3-P[7] evidencing double infection.
Phylogenetic trees were constructed for all segments, and partial sequences were excluded from the analysis. The VP4 phylogeny, specifically genotype P[7], revealed that Chilean RVA strains formed a monophyletic cluster, which also included previously reported strains from 2013 (Figure 1) (23). In addition to closely related Chilean sequences, similar viruses have been identified in swine and bovine populations across different regions globally.
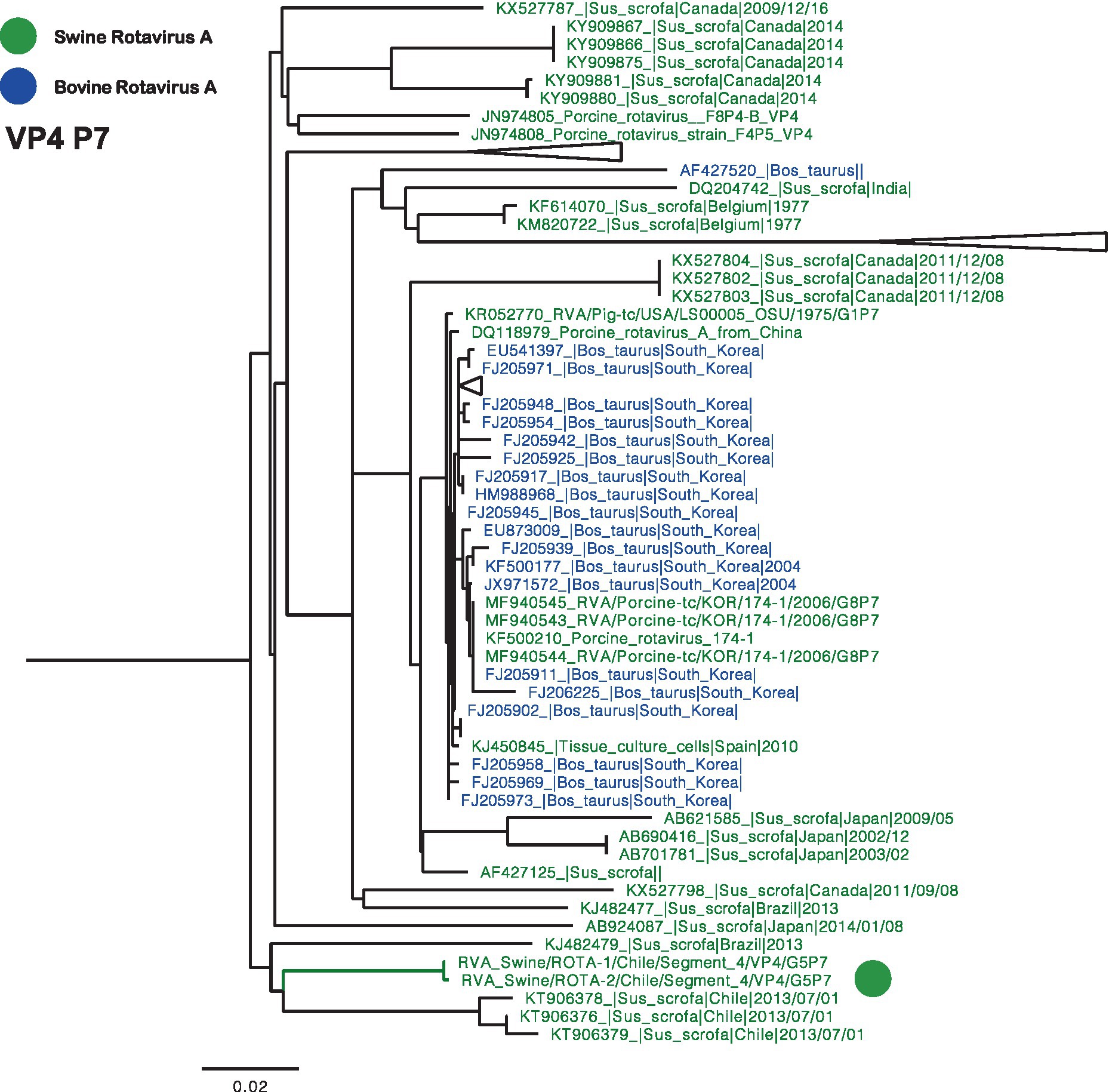
Figure 1. Phylogenetic tree of Rotavirus A segment 4 (VP4), P[7] genotype. The final dataset included 104 sequences. The Chilean sequences are depicted with a green dot. Strains are highlighted in colors: Swine RVA (Green), and Bovine (Blue).
Two separate trees were generated for VP7, representing genotypes G3 and G5 (Figures 2, 3). Notably, the G3 genotype has never been documented in Chile before, making the Chilean strain a unique singleton that shares its origin with strains found in humans, swine, and bovines (Figure 2). On the other hand, the remaining three segments are classified as G5, and the phylogenetic analysis grouped the Chilean sequences into a monophyletic cluster, including the partial sequences reported in 2013 (Figure 3) (23). These viruses have a common origin with strains detected in swine, humans, and bovines from various parts of the world. In the case of related sequences detected in humans, those detected in the Philippines have been associated with zoonotic detections (24).
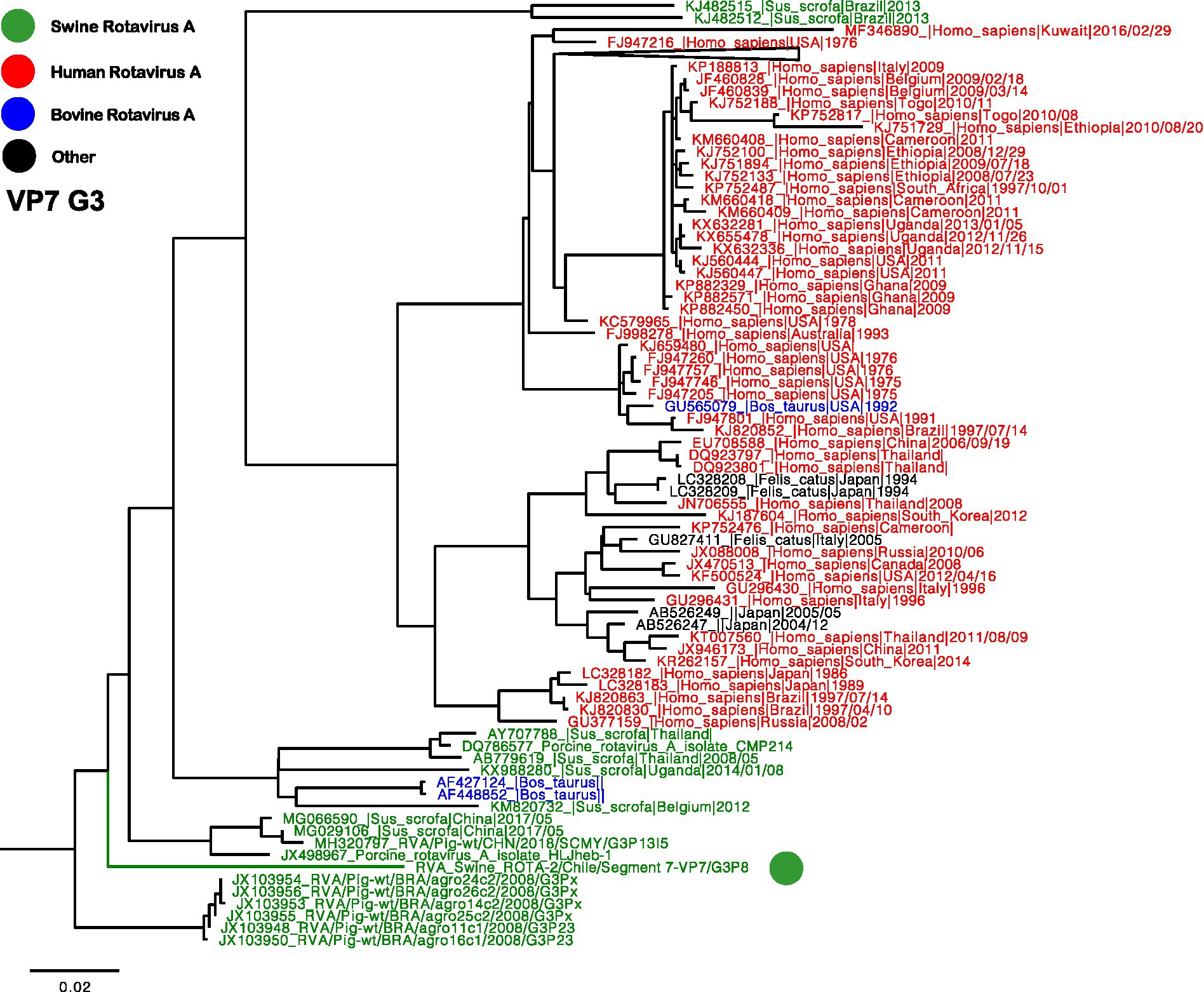
Figure 2. Phylogenetic tree of Rotavirus A segment 9 (VP7), G3 genotype. The final dataset included 371 sequences. The Chilean sequence is depicted with a green dot. Strains are highlighted in colors: Human RVA (Red), Swine RVA (Green), Bovine (Blue), and other origins in black.
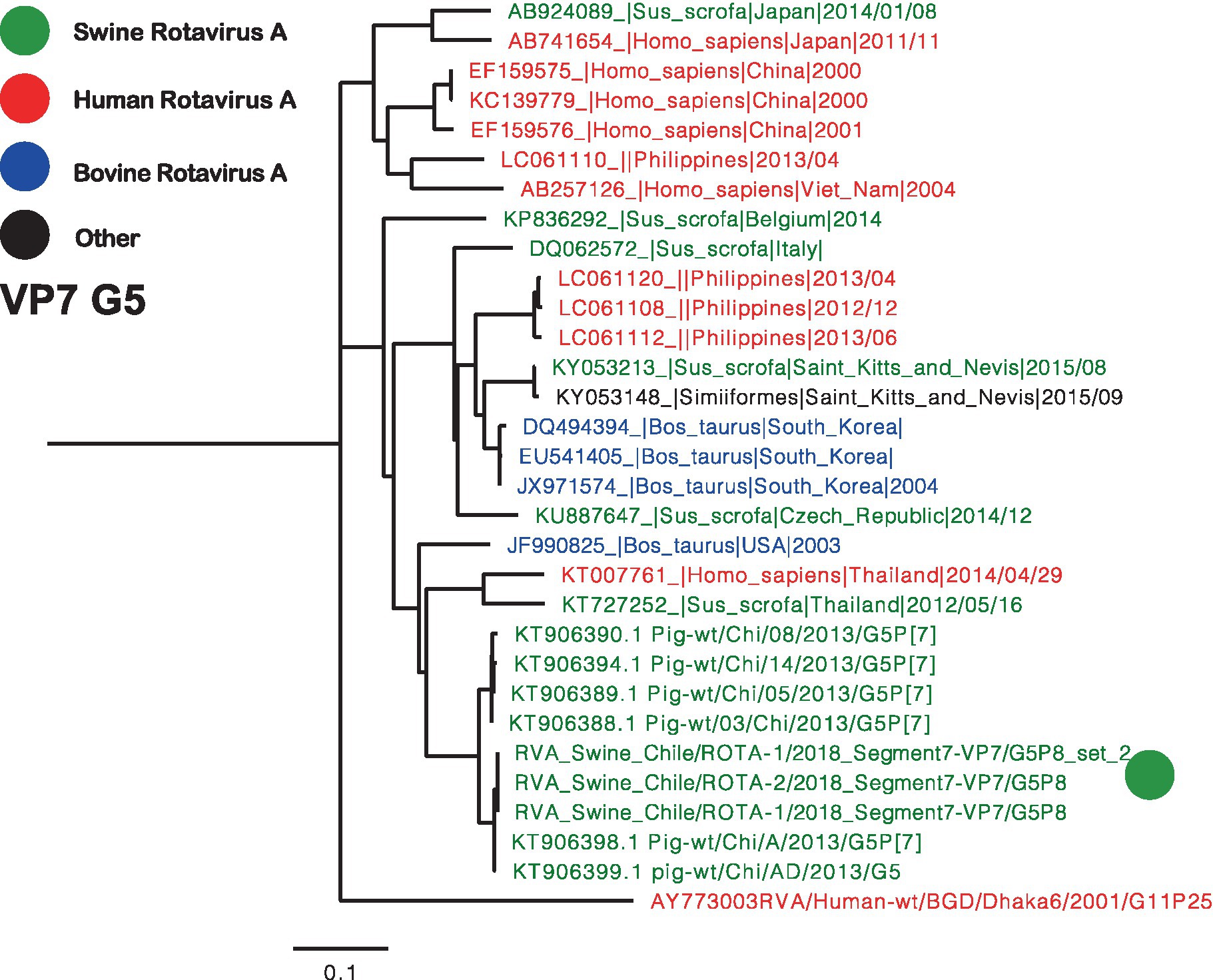
Figure 3. Phylogenetic tree of Rotavirus A segment 9 (VP7), G5 genotype. The final dataset included 31 sequences. The Chilean sequences are depicted with a green dot. Strains are highlighted in colors: Human RVA (Red), Swine RVA (Green), Bovine (Blue), and another origin in black.
The phylogenies for the rest of the genes can be divided into two groups. The phylogenies for VP1, VP2, NSP2, NSP3, NSP4, and NSP5 grouped the Chilean sequences in monophyletic clusters, while the phylogenies for VP6, VP3, NSP1 evidence two different origins of the Chilean sequences (Supplementary Figures S1–S9). In both cases, most of the sequences are more closely related to swine and human RVA sequences reported worldwide. For VP1 phylogeny the closest sequences that have been reported from swine in Belgium have been related to zoonotic events (25). For VP2 the closest sequence belonged to an ancient equine strain RVA/Horse-tc/GBR/H-1/1975/G5P9[7] (JQ309139). The NSP2 phylogeny grouped the Chilean sequences in a monophyletic group and related with human and swine sequences. The NSP3 phylogeny grouped the Chilean sequences with RVA detected in humans from Argentina, Ecuador, and Brazil. For NSP4 the Chilean sequences are more closely with RVA detected in 2006–2007 in swine from Canada (26). The NSP5 sequences are related to swine RVA reported from South Africa. On the other hand, the VP3 phylogeny clustered Chilean sequences into two groups and both groups related to human origin RVA sequences, like NSP1 and VP6 but in this case more closely related to RVA from swine (Supplementary Figures S1–S9).
4. Discussion
Group A rotaviruses are considered important viral agents that cause gastroenteric problems in intensive swine production centers, mainly affecting young pigs (27), and commonly detected in intensive production farms worldwide (28–30). In the present study, RVA was identified in 14 out of 22 intensive farms, confirming the widespread of RVA in Chilean farms, which was first reported in the country in 1983 (31). In South America, RVA has been reported in pigs in most countries such as Argentina, Peru, Brazil, and Colombia (32–35).
Rotavirus A has been identified in both feces and oral fluids, with its presence in feces being attributed to the well-established pathogenicity of the virus (5). The detection of the virus in oral fluids can be primarily attributed to the composition of these fluids in pigs, which may contain remnants of feces (36). However, recently (37) conducted experiments using a murine model, revealing that rotavirus can replicate within salivary glands and spread via saliva (37). This evidence implies the potential for viral excretion through saliva in swine, which should be further investigated.
Rotavirus A exhibits significant genetic diversity, with various genotypes capable of affecting pigs, other animals, and even humans. To date, there are 58 genotypes for P, 42 for G, 39 genotypes for A, 32 genotypes for I and E, 28 genotypes for R, N, T, and H, and 24 genotypes for C and M (RCWG, 2023). This extensive variation necessitates a comprehensive genome characterization to gain a better understanding of the epidemiology and zoonotic potential of the virus. In this study, we conducted a thorough investigation into the viral genome of porcine-origin RVA by employing WGSof isolates and clinical samples. While the full genome sequencing of isolates was successful, we encountered challenges in WGS directly from clinical samples, including fecal and oral fluids. These discrepancies may be attributed to the presence of other agents in direct samples that might have dominated the sequencing run, thus hindering the detection of RVA. We initially viewed the utilization of direct samples for NGS as a promising alternative, given the low Ct values observed in the samples used. However, our findings indicate that this approach is not recommended using the current protocol. One possible explanation for this limitation is the challenge of effectively denaturing double-stranded RNA (dsRNA). Recent reports have highlighted the use of dimethyl sulfoxide (DMSO) as a potential solution to improve dsRNA virus sequencing (38). Another alternative approach could involve preamplification through PCR followed by NGS, as demonstrated by Nyaga et al. (39), but this process is time-consuming. These results are interesting for future studies to improve the NGS attempting RVA sequencing from direct samples.
We were able to obtain the nearly complete genome of RVA from two isolates. The two outer capsid proteins VP7 (G) and VP4 (P) sequenced were more closely related to isolates previously detected in swine. We obtained G5 and G3 genotypes for VP7 and P[7] for VP4, common combinations reported previously (40). The G5 and G3 genotypes are usually detected in pigs, humans, and cattle (41, 42). The G5P[7] genotype combination is the most commonly detected in swine in the Americas (5), thus our findings are consistent with previous reports. Notably, we detected the presence of duplicated segments, including G5 and G3, in the isolates, indicating the potential occurrence of co-infection or mixed infection. This observation is particularly interesting as these samples were collected from individual animals, supporting the possibility of multiple viral strains circulating within the same host (28).
For the remaining segments, we identified the genotypes I5-R1-C1-M1-A8-N1-T1-E1-H1. While genotypes R1-C1-M1-N1-T1-T1-E1-H1 are directly related to human-origin RVA, I5, and A8 are more related to swine-origin RVA (11). Then, we reported constellation G5/G3-P [7] -I5-R1-C1-M1-A8-N1-T1-E1-H1 which is similar to other constellations obtained from swine samples from different countries (25). Using the phylogenetic trees, we established the genetic relationship between Chilean RVA strains and viruses found in swine, humans, and bovine populations across different parts of the world. These results can suggest the potential role of RVA as a zoonotic and/or reverse zoonotic pathogen, capable of reassortment due to its segmented nature (43). It is important to note that the reassortment events of Rotavirus A occur frequently with RVA strains from the same animal species but can also occur with strains originating from animals and humans (44–46). Examples of genetic reassortment of RVA strains from swine to human are the introductions of the swine G9 (11) and the P[6] to human strains (47, 48). Additionally, a case of G5 genotype reassortment between swine and human viruses was reported in children from Brazil, Paraguay, and Argentina (7).
In general, BLAST reported <95% identity of Chilean sequences compared to reference sequences indicating a substantial level of genetic divergence. This high divergence can be attributed to the limited availability of RVA sequences for comparison purposes and phylogeny. The epidemiological significance of our findings may be influenced by the lack of sufficient data. It is important to note that even in Chile, there is a limited information of publicly available human RVA sequences, with only partial VP7 sequences accessible for analysis.
The results obtained from this study suggest that the viruses identified and sequenced may have originated from reassortments of RVA originating from humans, swine, and other animal species. Therefore, improving the NGS for dsRNA viruses such as RVA is mandatory in Chile and other countries. In summary, while the present study provides valuable insights into the genetic diversity and potential origins of RVA, further research is necessary to fully comprehend its complex epidemiology.
Data availability statement
The datasets presented in this study can be found in online repositories. The names of the repository/repositories and accession number(s) can be found at: https://www.ncbi.nlm.nih.gov/ OR192577-OR192610.
Ethics statement
The animal study was approved by all procedures involving animals were approved by the Institutional Committee for Animal Care and Use (CICUA) of the University of Chile under certificate number 02-2016. The study was conducted in accordance with the local legislation and institutional requirements.
Author contributions
VN, SM, and BB-R contributed to the conception and design of the study. CM, VV, BB-R, and VN performed the analysis. CM, CU-E, GR-T, and VN wrote the first draft of the manuscript. All authors contributed to the manuscript revision and approved the submitted version.
Funding
This work was supported by the Animal Virology Laboratory, Faculty of Veterinary and Animal Sciences, Universidad de Chile and Programa Fondecyt 11170877 and 1211517.
Acknowledgments
We are grateful to Barbara Quezada and Naomi Ariyama from Animal Virology Lab, U de Chile, and swine veterinarians for all their support in technical assistance.
Conflict of interest
The authors declare that the research was conducted in the absence of any commercial or financial relationships that could be construed as a potential conflict of interest.
Publisher’s note
All claims expressed in this article are solely those of the authors and do not necessarily represent those of their affiliated organizations, or those of the publisher, the editors and the reviewers. Any product that may be evaluated in this article, or claim that may be made by its manufacturer, is not guaranteed or endorsed by the publisher.
Supplementary material
The Supplementary material for this article can be found online at: https://www.frontiersin.org/articles/10.3389/fvets.2023.1240346/full#supplementary-material
Footnotes
1. ^https://rega.kuleuven.be/cev/-viralmetagenomics/virus-classification/rcwg, Accessed May 30, 2023.
3. ^https://www.ncbi.nlm.nih.gov/genomes/VirusVariation/-Database/nph-select.cgi?taxid=28875
References
1. Matthijnssens, J , Attoui, H , Bányai, K , Brussaard, CPD , Danthi, P , del Vas, M, et al. ICTV Virus Taxonomy Profile: Sedoreoviridae 2022. J Gen Virol. (2022) 103:1–2. doi: 10.1099/jgv.0.001782
2. Crawford, SE , Ramani, S , Tate, JE , Parashar, UD , Svensson, L , Hagbom, M, et al. Rotavirus infection. Nat Rev Dis Primers. (2017) 3:17083. doi: 10.1038/nrdp.2017.83
3. LeClair, CE , and McConnell, KA . Rotavirus, In: StatPearls. Treasure Island, FL: StatPearls Publishing (2023).
4. Parashar, UD , Hummelman, EG , Bresee, JS , Miller, MA , and Glass, RI . Global illness and deaths caused by rotavirus disease in children. Emerg Infect Dis. (2003) 9:565–72. doi: 10.3201/eid0905.020562
5. Vlasova, A , Amimo, J , and Saif, L . Porcine rotaviruses: epidemiology, immune responses and control strategies. Viruses. (2017) 9:48. doi: 10.3390/v9030048
6. Greenberg, HB , and Estes, MK . Rotaviruses: from pathogenesis to vaccination. Gastroenterology. (2009) 136:1939–51. doi: 10.1053/j.gastro.2009.02.076
7. Martella, V , Bányai, K , Matthijnssens, J , Buonavoglia, C , and Ciarlet Zoonotic, M . Zoonotic aspects of rotaviruses. Vet Microbiol. (2010) 140:246. doi: 10.1016/j.vetmic.2009.08.028
8. Park, G-N , Kim, DI , Choe, S , Shin, J , An, B-H , Kim, K-S, et al. Genetic diversity of porcine group a rotavirus strains from pigs in South Korea. Viruses. (2022) 14:2522. doi: 10.3390/v14112522
9. Matthijnssens, J , Ciarlet, M , McDonald, SM , Attoui, H , Bányai, K , Brister, JR, et al. Uniformity of rotavirus strain nomenclature proposed by the rotavirus classification working group (RCWG). Arch Virol. (2011) 156:1397–413. doi: 10.1007/s00705-011-1006-z
10. Matthijnssens, J , Ciarlet, M , Rahman, M , Attoui, H , Bányai, K , Estes, MK, et al. Recommendations for the classification of group a rotaviruses using all 11 genomic RNA segments. Arch Virol. (2008) 153:1621–9. doi: 10.1007/s00705-008-0155-1
11. Monini, M , Zaccaria, G , Ianiro, G , Lavazza, A , Vaccari, G , and Ruggeri, FM . Full-length genomic analysis of porcine rotavirus strains isolated from pigs with diarrhea in northern Italy. Infect Genet Evol. (2014) 25:4–13. doi: 10.1016/j.meegid.2014.03.024
12. Cook, N , Bridger, J , Kendall, K , Gomara, MI , El-Attar, L , and Gray, J . The zoonotic potential of rotavirus. J Infect. (2004) 48:289–302. doi: 10.1016/j.jinf.2004.01.018
13. Müller, H , and Johne, R . Rotaviruses: diversity and zoonotic potential--a brief review. Berl Munch Tierarztl Wochenschr. (2007) 120:108–12.
14. Marthaler, D , Homwong, N , Rossow, K , Culhane, M , Goyal, S , Collins, J, et al. Rapid detection and high occurrence of porcine rotavirus A, B, and C by RT-qPCR in diagnostic samples. J Virol Methods. (2014) 209:30–4. doi: 10.1016/j.jviromet.2014.08.018
15. Kalantar, KL , Carvalho, T , de Bourcy, CFA , Dimitrov, B , Dingle, G , Egger, R, et al. IDseq-An open source cloud-based pipeline and analysis service for metagenomic pathogen detection and monitoring. Gigascience. (2020) 9:giaa111. doi: 10.1093/gigascience/giaa111
16. Ramesh, A , Nakielny, S , Hsu, J , Kyohere, M , Byaruhanga, O , de Bourcy, C, et al. Metagenomic next-generation sequencing of samples from pediatric febrile illness in Tororo, Uganda. PLoS One. (2019) 14:e0218318. doi: 10.1371/journal.pone.0218318
17. Saha, S , Ramesh, A , Kalantar, K , Malaker, R , Hasanuzzaman, M , Khan, LM, et al. Unbiased metagenomic sequencing for pediatric meningitis in Bangladesh reveals neuroinvasive chikungunya virus outbreak and other unrealized pathogens. mBio. (2019) 10:e02877-19. doi: 10.1128/mBio.02877-19
18. Olson, RD , Assaf, R , Brettin, T , Conrad, N , Cucinell, C , Davis, JJ, et al. Introducing the bacterial and viral bioinformatics resource Center (BV-BRC): a resource combining PATRIC, IRD and ViPR. Nucleic Acids Res. (2023) 51:D678–89. doi: 10.1093/nar/gkac1003
19. Edgar, RC . MUSCLE: multiple sequence alignment with high accuracy and high throughput. Nucleic Acids Res. (2004) 32:1792–7. doi: 10.1093/nar/gkh340
20. Darriba, D , Taboada, GL , Doallo, R , and Posada, D . jModelTest 2: more models, new heuristics and parallel computing. Nat Methods. (2012) 9:772–2. doi: 10.1038/nmeth.2109
21. Kumar, S , Stecher, G , Li, M , Knyaz, C , and Tamura, K . MEGA X: molecular evolutionary genetics analysis across computing platforms. (Fabia Ursula Battistuzzi, Ed.). Mol Biol Evol. (2018) 35:1547–9. doi: 10.1093/molbev/msy096
22. Miller, M.A. , Pfeiffer, W. , and Schwartz, T. (2010). Creating the CIPRES science gateway for inference of large phylogenetic trees. pp. 1–8. In: 2010 gateway computing environments workshop (GCE). New Orleans, LA: IEEE.
23. Cañon Jones, H , Cortes, H , Gaggero, A , Levican, J , Castillo-Ruiz, M , Schlotterbeck, T, et al. High genetic diversity of species a rotaviruses detected in swine farms in Chile. J Gen Virol. (2017) 98:539–47. doi: 10.1099/jgv.0.000662
24. Imagawa, T , Saito, M , Yamamoto, D , Saito-Obata, M , Masago, Y , Ablola, AC, et al. Genetic diversity of species a rotaviruses detected in clinical and environmental samples, including porcine-like rotaviruses from hospitalized children in the Philippines. Infect Genet Evol. (2020) 85:104465. doi: 10.1016/j.meegid.2020.104465
25. Theuns, S , Heylen, E , Zeller, M , Roukaerts, IDM , Desmarets, LMB , Van Ranst, M, et al. Complete genome characterization of recent and ancient Belgian pig group a rotaviruses and assessment of their evolutionary relationship with human rotaviruses. J Virol. (2014) 89:1043–57. doi: 10.1128/JVI.02513-14
26. Lamhoujeb, S , Cook, A , Pollari, F , Bidawid, S , Farber, J , and Mattison, K . Rotaviruses from Canadian farm samples. Arch Virol. (2010) 155:1127–37. doi: 10.1007/s00705-010-0700-6
27. Vidal, A , Clilverd, H , Cortey, M , Martín-Valls, GE , Franzo, G , Darwich, L, et al. Full-genome characterization by deep sequencing of rotavirus a isolates from outbreaks of neonatal diarrhoea in pigs in Spain. Vet Microbiol. (2018) 227:12–9. doi: 10.1016/j.vetmic.2018.10.002
28. Chandler-Bostock, R , Hancox, LR , Nawaz, S , Watts, O , Iturriza-Gomara, M , and Mellits, KM . Genetic diversity of porcine group a rotavirus strains in the UK. Vet Microbiol. (2014) 173:27–37. doi: 10.1016/j.vetmic.2014.06.030
29. Hong Anh, P , Carrique-Mas, JJ , Van Cuong, N , Hoa, NT , Lam Anh, N , Duy, DT, et al. The prevalence and genetic diversity of group a rotaviruses on pig farms in the Mekong Delta region of Vietnam. Vet Microbiol. (2014) 170:258–65. doi: 10.1016/j.vetmic.2014.02.030
30. Tonietti, PO , Hora, AS , Silva, FDF , Ruiz, VLA , and Gregori, F . Phylogenetic analyses of the VP4 and VP7 genes of porcine group a rotaviruses in São Paulo state, Brazil: first identification of G5P[23] in piglets. J Clin Microbiol. (2020) 51:2750–3. doi: 10.1128/jcm.01175-13
31. Berríos, EP , Pinochet, VL , Abalos, PP , and Cuevas, PL . Presencia de rotavirus en cerdos lactantes con síndrome diarreico. Avances En Ciencias Veterinarias. (1989) 4:215–228. doi: 10.5354/acv.v4i2.4550
32. Almeida, PR , Lorenzetti, E , Cruz, RS , Watanabe, TT , Zlotowski, P , Alfieri, AA, et al. Diarrhea caused by rotavirus A, B, and C in suckling piglets from southern Brazil: molecular detection and histologic and immunohistochemical characterization. J Vet Diagn Investig. (2018) 30:370–6. doi: 10.1177/1040638718756050
33. Bok, K , Castagnaro, N , Borsa, A , Nates, S , Espul, C , Fay, O, et al. Surveillance for rotavirus in Argentina. J Med Virol. (2001) 65:190–8. doi: 10.1002/jmv.2020
34. Hanssen, H , Hincapié, O , and López, JH . Influenza EN Porcinos DE Antioquia, Colombia. Boletín de la Oficina Sanitaria Panamericana. (1977) 82:35–43.
35. Rojas, MM , Manchego, SA , Rivera, GH , Falcón, PN , Ramírez, VM , and Nieves Sandoval Ch, NSC . Asociación entre rotavirus y la presencia de diarrea en lechones de granjas tecnificadas. Revista de Investigaciones Veterinarias del Perú. (2011) 22:253–60. doi: 10.15381/rivep.v22i3.266
36. Franco-Martínez, L , Ortín-Bustillo, A , Rubio, CP , Escribano, D , López-Arjona, M , García-Manzanilla, E, et al. Effects of pen faeces and feed contamination in biomarkers determination in oral fluid of pigs. Res Vet Sci. (2022) 152:403–9. doi: 10.1016/j.rvsc.2022.09.003
37. Ghosh, S , Kumar, M , Santiana, M , Mishra, A , Zhang, M , Labayo, H, et al. Enteric viruses replicate in salivary glands and infect through saliva. Nature. (2022) 607:345–50. doi: 10.1038/s41586-022-04895-8
38. Wilcox, AH , Delwart, E , and Díaz-Muñoz, SL . Next-generation sequencing of dsRNA is greatly improved by treatment with the inexpensive denaturing reagent DMSO. Microb Genom. (2019) 5:e000315. doi: 10.1099/mgen.0.000315
39. Nyaga, MM , Jere, KC , Esona, MD , Seheri, ML , Stucker, KM , Halpin, RA, et al. Whole genome detection of rotavirus mixed infections in human, porcine and bovine samples co-infected with various rotavirus strains collected from sub-Saharan Africa. Infect Genet Evol. (2015) 31:321–34. doi: 10.1016/j.meegid.2015.02.011
40. Papp, H , László, B , Jakab, F , Ganesh, B , De Grazia, S , Matthijnssens, J, et al. Review of group a rotavirus strains reported in swine and cattle. Vet Microbiol. (2013) 165:190–9. doi: 10.1016/j.vetmic.2013.03.020
41. Degiuseppe, JI , Parra, GI , and Stupka, JA . Genetic diversity of G3 rotavirus strains circulating in Argentina during 1998–2012 assessed by full genome analyses. PLoS One. (2014) 9:e110341. doi: 10.1371/journal.pone.0110341
42. Midgley, SE , Bányai, K , Buesa, J , Halaihel, N , Hjulsager, CK , Jakab, F, et al. Diversity and zoonotic potential of rotaviruses in swine and cattle across Europe. Vet Microbiol. (2012) 156:238–45. doi: 10.1016/j.vetmic.2011.10.027
43. Iturriza-Gómara, M , Isherwood, B , Desselberger, U , and Gray, J . Reassortment in vivo: driving force for diversity of human rotavirus strains isolated in the United Kingdom between 1995 and 1999. J Virol. (2001) 75:3696–705. doi: 10.1128/JVI.75.8.3696-3705.2001
44. Hoshino, Y , Honma, S , Jones, RW , Ross, J , Santos, N , Gentsch, JR, et al. A porcine G9 rotavirus strain shares neutralization and VP7 phylogenetic sequence lineage 3 characteristics with contemporary human G9 rotavirus strains. Virology. (2005) 332:177–88. doi: 10.1016/j.virol.2004.11.006
45. Jing, Z , Zhang, X , Shi, H , Chen, J , Shi, D , Dong, H, et al. A G3P[13] porcine group a rotavirus emerging in China is a reassortant and a natural recombinant in the VP4 gene. Transbound Emerg Dis. (2018) 65:e317–28. doi: 10.1111/tbed.12756
46. Teodoroff, TA , Tsunemitsu, H , Okamoto, K , Katsuda, K , Kohmoto, M , Kawashima, K, et al. Predominance of porcine rotavirus G9 in Japanese piglets with diarrhea: close relationship of their VP7 genes with those of recent human G9 strains. J Clin Microbiol. (2005) 43:1377–84. doi: 10.1128/JCM.43.3.1377-1384.2005
47. Heylen, E , Batoko Likele, B , Zeller, M , Stevens, S , De Coster, S , Conceição-Neto, N, et al. Rotavirus surveillance in Kisangani, the Democratic Republic of the Congo, reveals a high number of unusual genotypes and gene segments of animal origin in non-vaccinated symptomatic children. PLoS One. (2014) 9:e100953. doi: 10.1371/journal.pone.0100953
48. Rotavirus Classification Working Group . RCWG. (2023) Available at: https://rega.kuleuven.be/cev/viralmetagenomics/virus-classification/rcwg (Accessed June 9, 2023).
Keywords: Rotavirus A, pig, NGS, genotyping, zoonoses
Citation: Neira V, Melgarejo C, Urzúa-Encina C, Berrios F, Valdes V, Mor S, Brito-Rodriguez B and Ramirez-Toloza GA (2023) Identification and characterization of porcine Rotavirus A in Chilean swine population. Front. Vet. Sci. 10:1240346. doi: 10.3389/fvets.2023.1240346
Edited by:
Izhar Hyder Qazi, Shaheed Benazir Bhutto University of Veterinary & Animal Sciences, PakistanReviewed by:
Norma Santos, Federal University of Rio de Janeiro, BrazilAisha Khatoon, University of Agriculture, Faisalabad, Pakistan
Reimar Johne, Federal Institute for Risk Assessment (BfR), Germany
Copyright © 2023 Neira, Melgarejo, Urzúa-Encina, Berrios, Valdes, Mor, Brito-Rodriguez and Ramirez-Toloza. This is an open-access article distributed under the terms of the Creative Commons Attribution License (CC BY). The use, distribution or reproduction in other forums is permitted, provided the original author(s) and the copyright owner(s) are credited and that the original publication in this journal is cited, in accordance with accepted academic practice. No use, distribution or reproduction is permitted which does not comply with these terms.
*Correspondence: Victor Neira, dm5laXJhcmFtQGdtYWlsLmNvbQ==