- 1Sports Science Division, Equine Research Institute, Japan Racing Association, Shimotsuke, Japan
- 2Department of Biological Sciences, Graduate School of Sciences and Technology for Innovation, Yamaguchi University, Yamaguchi, Japan
- 3Department of Veterinary Pathophysiology and Animal Health, Graduate School of Agricultural and Life Sciences, The University of Tokyo, Bunkyo, Japan
- 4Racehorse Hospital, Miho Training Center, Inashiki, Japan
Heat acclimatization or acclimation training in horses is practiced to reduce physiological strain and improve exercise performance in the heat, which can involve metabolic improvement in skeletal muscle. However, there is limited information concerning the acute signaling responses of equine skeletal muscle after exercise in a hot environment. The purpose of this study was to investigate the hypothesis that exercise in hot conditions induces greater changes in heat shock proteins and mitochondrial-related signaling in equine skeletal muscle compared with exercise in cool conditions. Fifteen trained Thoroughbred horses [4.6 ± 0.4 (mean ± SE) years old; 503 ± 14 kg] were assigned to perform a treadmill exercise test in cool conditions [COOL; Wet Bulb Globe Temperature (WBGT), 12.5°C; n = 8] or hot conditions (HOT; WBGT, 29.5°C; n = 7) consisting of walking at 1.7 m/s for 1 min, trotting at 4 m/s for 5 min, and cantering at 7 m/s for 2 min and at 90% of VO2max for 2 min, followed by walking at 1.7 m/s for 20 min. Heart rate during exercise and plasma lactate concentration immediately after exercise were measured. Biopsy samples were obtained from the middle gluteal muscle before and at 4 h after exercise, and relative quantitative analysis of mRNA expression using real-time RT-PCR was performed. Data were analyzed with using mixed models. There were no significant differences between the two groups in peak heart rate (COOL, 213 ± 3 bpm; HOT, 214 ± 4 bpm; p = 0.782) and plasma lactate concentration (COOL, 13.1 ± 1.4 mmoL/L; HOT, 17.5 ± 1.7 mmoL/L; p = 0.060), while HSP-70 (COOL, 1.9-fold, p = 0.207; HOT, 2.4-fold, p = 0.045), PGC-1α (COOL, 3.8-fold, p = 0.424; HOT, 8.4-fold, p = 0.010), HIF-1α (COOL, 1.6-fold, p = 0.315; HOT, 2.2-fold, p = 0.018) and PDK4 (COOL, 7.6-fold, p = 0.412; HOT, 14.1-fold, p = 0.047) mRNA increased significantly only in HOT at 4 h after exercise. These data indicate that acute exercise in a hot environment facilitates protective response to heat stress (HSP-70), mitochondrial biogenesis (PGC-1α and HIF-1α) and fatty acid oxidation (PDK4).
1. Introduction
Heat acclimatization or acclimation training has generally been practiced in humans to reduce physiological strain, decrease aerobic metabolic rate, and improve exercise performance in the heat (1–5). Studies in humans and rodents have shown that these adaptations involve changes at the cellular level, including improved cytoprotection against heat stress, stimulated mitochondrial biogenesis, enhanced fatty acid oxidation, and angiogenesis promotion (6–9).
Heat shock proteins (HSPs) are classified as molecular chaperones that play a central role in the cellular stress response to a hot environment, and increased expression of HSPs has a protective function against cell injury associated with adverse stresses (10, 11). In addition, Henstridege et al. demonstrated that genetic overexpression of HSP-70 upregulates oxidative metabolism in rodent skeletal muscle and that HSP-70 may play an important role in the regulation of mitochondrial biogenesis (12). HSP-90, a member of the HSPs, also has specific characteristics and stimulates the activation of signaling transduction pathways distinct from those stimulated by HSP-70. Katschinski et al. demonstrated that the chaperone activity of HSP-90 is involved in hypoxic-inducible factor-1α (HIF-1α) stabilization in vitro (13). HIF-1α regulates vascular endothelial growth factor (VEGF), which is implicated as important modulator of angiogenesis and facilitates migration. Both HSP-70 and HSP-90 augment proportionally increased cellular heat stress (14), in particular, HSP-70 gene expression increases synergistically when combined with exercise and hot conditions in rats (15). In addition, Morton et al. indicated that there is a sex specific HSP adaptation caused by estrogen in human skeletal muscle (16), and Gillum et al. have demonstrated that male increased HSP-72 more than female in peripheral blood mononuclear cells in response to running at 65% VO2peak for 60 min in the heat (ambient temperature, 42°C; relative humidity, 20%) (17). In contrast, Mee et al. have demonstrated that there is no sex difference in leucocyte HSP72 mRNA after cycling at 65% VO2peak for 90 min under a hot environment (ambient temperature, 40°C; relative humidity, 40%) in humans (18). However, there is limited information concerning the sex differences on HSPs in Thoroughbred horses.
Several studies have demonstrated that chronic heat stress can upregulate mitochondrial-related factors in vitro and in vivo, including humans and rodents. Repeated exposure to high temperature (40°C) can induce mitochondrial biogenesis and mitochondrial oxidative efficiency in C2C12 myotubes (19, 20). In addition, Maunder et al. demonstrated that endurance training under environmental heat stress (ambient temperature, 33°C; relative humidity, 80%) improves endurance performance and induces mitochondrial adaptation in humans (6). The increased energy demands associated with physiological strain activate the expression of the muscle peroxisome proliferator-activated receptor γ coactivator-1α (PGC-1α), a master regulator of mitochondrial biogenesis, gene expression, which can induce pyruvate dehydrogenase kinase 4 (PDK4) gene expression via estrogen-related receptor α (21). PDK4 facilitates the inactivation of pyruvate dehydrogenase complex, promoting an increase in mitochondrial fatty acid oxidation and a concurrent decrease in glucose oxidation (22). In terms of acute exercise, Tamura et al. demonstrated that post-exercise in the chamber where ambient temperature regulated 40°C upregulates PGC-1α expression in mouse skeletal muscle (7). In contrast, Heesch et al. demonstrated that acute exercise in a hot environment (ambient temperature, 33°C; relative humidity, 60%) blunts mitochondrial biogenesis related gene expression both immediately and at 3 h postexercise in humans (23). Therefore, the effect of acute exercise in a hot environment on mitochondrial adaptations is still controversial and needs further investigation.
Thoroughbreds have a high capacity for energy production and produce heat that elevates core temperature by approximately 1°C per minute during strenuous exercise (24). Furthermore, when these horses exercise in hot and humid environments (ambient temperature, 32–24°C; relative humidity, 80–85%), including racing or competition during daytime in summer, where heat dissipation is challenging, their core and muscle temperatures can rise to over 42°C during high-intensity exercise (25). Previously, we reported that exercise in an environment with an ambient temperature that exceeds body temperature causes a significant elevation of core body temperature and leads to decreased running economy (26). In addition, several other studies have demonstrated that exercise in a hot environment increases physiological strain and impairs exercise performance (25, 27). As a countermeasure to reduce these deleterious effects of heat stress, it is recommended that horses train in hot conditions similar to human athletes (28), and several studies have demonstrated that repeated exposure to heat stress can improve aerobic exercise performance and reduces metabolic rate in horses (29, 30). Therefore, we hypothesized that heat acclimatization or acclimation training would induce not only physiological adaptations but also cellular adaptations in skeletal muscle in horses. Several studies reported that the mRNA expression of heat shock proteins and mitochondria-associated proteins is increased after acute exercise in temperate conditions (31–33) and that there are no sex differences in the enzyme activities related to oxidative capacity in equine skeletal muscle (34). However, there is limited information concerning the differences in the acute signaling responses of equine skeletal muscle after exercise in a hot environment compared to exercise in a cool environment. Understanding these skeletal muscle responses can help construct a training strategy for races and competitions in a hot environment.
The purpose of this study was to investigate the hypothesis that exercise in hot conditions induces greater changes in heat shock proteins and mitochondrial-related signaling in equine skeletal muscle compared to exercise in cool conditions.
2. Materials and methods
2.1. Animals
Fifteen Thoroughbred horses [one male, seven geldings and seven females; age, 4.6 ± 0.4 (mean ± SE) years; body weight, 503 ± 14 kg; maximal oxygen consumption (VO2max), 174 ± 7 (ml/min kg)] were studied. Their characteristics are presented in Table 1. This study was conducted in Shimotsuke city (Japan) from February to March (average ambient temperature: 5–10°C) when the horses were not acclimatized to hot conditions. Horses had moderate-intensity training (1.7 m/s for 2 min, 4.0 m/s for 5 min, 7.0 m/s for 2 min, and 10.0 m/s for 2 min) on a treadmill (SÄTO AB, Knivsta, Sweden) at a 6% incline in cool conditions [ambient temperature, 20°C; relative humidity, 40%; Wet Bulb Globe Temperature (WBGT), 15°C; Temperature Humidity Index (THI), 62.8] 2 days/week for 4 weeks and walked for 1 h/day in a walker for 5 days/week and were kept in a 400 m2 paddock for 6 h/day prior to the experiment. After training, the horses performed preliminary incremental exercise tests in cool conditions to determine their VO2max. Following a warm up at 4 m/s for 3 min, the horses began exercising up a 6% incline for 2 min each at 1.7, 4, 6, 8, 10, 12 and 13 m/s until the houses could not maintain their position at the front of the treadmill with human encouragement.
2.2. Oxygen consumption
The procedure for measuring oxygen consumption has been described previously (35–37). Horses wore a 25 cm diameter open-flow mask on the treadmill, with a rheostat-controlled blower drawing air. Air flowed through 25 cm diameter tubing and across a pneumotachograph (LF-150B, Vise Medical, Chiba, Japan) connected to a differential pressure transducer (TF-5, Vise Medical, Chiba, Japan); this ensured that the bias flows during measurements were identical to those used during calibrations. Oxygen and CO2 concentrations were measured by an O2 and CO2 analyzer (MG-360, Vise Medical, Chiba, Japan), and calibrations were performed to calculate rates of O2 consumption and CO2 production with electronic mass flow meters (CR-300, Kofloc, Kyoto, Japan) using the N2-dilution/CO2-addition mass-balance technique (38). Gas analyzer and mass flow meter outputs were recorded on personal computer and analyzed with commercial hardware and software (DI-720 and Windaq Pro+, DATAQ, Akron, OH). VO2 was calculated for the final 30 s of each step at the incremental exercise test.
2.3. Experimental design
Horses were assigned to two groups, horses exercising in cool conditions (COOL, n = 8) or horses exercising in hot conditions (HOT, n = 7), to match their run time and VO2max from the preliminary incremental exercise test. Each horse performed a treadmill exercise test consisting of walking at 1.7 m/s for 1 min, trotting at 4 m/s for 5 min, cantering at 7 m/s for 2 min, and eliciting 90% VO2max (10.4 ± 0.2 m/s) for 2 min, followed by walking at 1.7 m/s for 20 min in either cool conditions (COOL group, ambient temperature, 18.1 ± 0.5°C; relative humidity, 28.0 ± 2.5%; WBGT, 12.5 ± 0.5°C; THI, 61.2 ± 0.4) or hot conditions (HOT group, ambient temperature, 38.2 ± 0.4°C; relative humidity, 31.7 ± 0.4%; WBGT, 29.5 ± 0.3°C; THI, 77.3 ± 0.3). The room temperature was controlled using air conditioners (RAS-AP140DG4, Hitachi, Tokyo, Japan) and oil heaters (HPS360, Orion, Nagano, Japan). Ambient temperature, relative humidity, and WBGT were measured using a portable monitoring device (WBGT-213B, Kyoto Electronics Manufacturing, Kyoto, Japan).
2.4. Heart rate and plasma lactate concentration
A heart rate monitor (S810, Polar, Kempele, Finland) was attached around the thorax and mean heart rate was calculated for the final 30 s of each step of the exercise test. Venous blood was collected from the jugular vein via an 18-gauge needle into a heparin tube immediately after exercise. Blood samples were centrifuged (AX-511, Tomy industrial, Tokyo, Japan) at 1740 ×g for 10 min to measure plasma lactate concentration with a lactate analyzer (Biosen S-Line, EKF-diagnostic GmbH, Barleben, Germany).
2.5. Muscle biopsy
Before exercise (pre) and 4 h after exercise, muscle samples (~50 mg wet weight) were obtained from the same area (the two sampling points were approximately 2 cm apart) at the mid-section of the gluteus medius muscle and from the same depth (5 cm below the skin surface) by needle biopsy under local anesthesia (Lidocaine, Fujisawa pharmaceutical, Osaka, Japan). All muscle samples were immediately frozen by liquid nitrogen and stored at −80°C until analyzed.
2.6. RNA isolation and real time RT-PCR
The procedure for RT-PCR has been described previously (39). Total RNA was extracted from each muscle sample with a TRIZOL regimen (Molecular Probes, Breda, Netherlands). The purity and quantity of total RNA were determined by measuring the absorbance of aliquots at 260 and 280 nm. Total RNA was then treated for 30 min at 37°C with TURBO DNase (Ambion, Austin, TX, United States) to remove genomic DNA from samples. DNase-treated RNA (0.5 μg) was used to synthesize first-stand cDNA with an Exscript™ RT reagent Kit (Takara, Tokyo, Japan). Thereafter, the cDNA products were analyzed by real-time PCR using the SYBR Green PCR Master Mix protocol in a StepOne™ Real Time PCR System (Applied Biosystems Japan, Tokyo, Japan).
The amplification program included an initial denaturation step at 95°C for 10 min, 40 cycles of denaturation at 95°C for 30 s, and annealing/extension at 58°C for 1 min. The amount of glyceraldehyde-3-phosphate dehydrogenase (GAPDH) mRNA was estimated as an internal control (40). Each mRNA was normalized to GAPDH by subtracting the cycle threshold (Ct) value of GAPDH from the Ct value of the gene target [ΔCt (target)]. The relative expression of the target gene was calculated as the relative quantification value for the pre value. Following the relative expression, dissociation curve analysis detected no nonspecific amplification in cDNA samples.
The sequences of the specific primers used in this study are presented in Table 2. Each PCR primer was designed by Primer Express® software (Applied Biosystems Japan, Tokyo, Japan), and oligonucleotides were purchased from FASMAC (FASMAC, Kanagawa, Japan).
2.7. Statistical procedures
All data are presented as mean ± standard error (SE). Differences in heart rate and plasma lactate concentration between COOL and HOT during exercise were analyzed using Student’s t-test. We analyzed mRNA expressions using mixed model with time, group and sex as fixed effects and individual horse as a random effect. When a significant effect or interaction was observed, Tukey’s tests were used as post hoc tests. For all analyses, significance was set as p ≤ 0.05. Statistical software (JMP 16.2.0, SAS Institute Inc., Cary, NC) was used for all data analyses.
3. Results
3.1. Heart rate and plasma lactate concentration
There were no differences between groups in peak heart rate during exercise (COOL, 213 ± 3 bpm; HOT, 214 ± 4 bpm; p = 0.782; Figure 1A). Plasma lactate concentration immediately after exercise in HOT tended to be higher than that in COOL, but the difference was not significant (COOL, 13.1 ± 1.4 mmoL/L; HOT, 17.5 ± 1.7 mmoL/L; p = 0.060; Figure 1B).
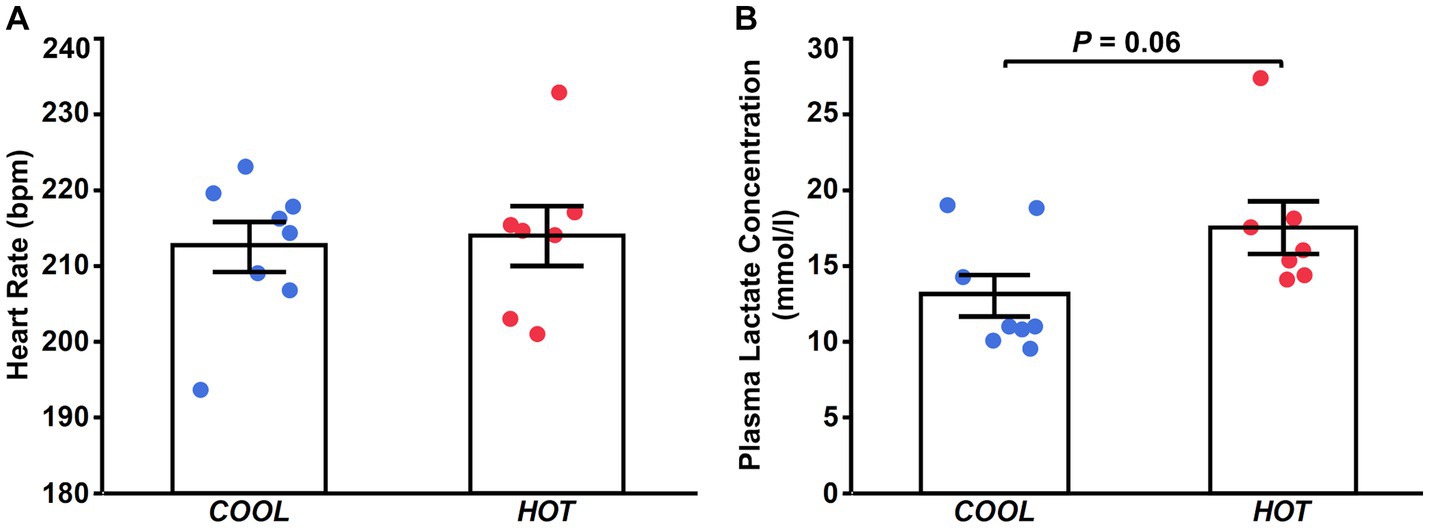
Figure 1. Heart rate (A) at the end of exercise and plasma lactate concentration immediately after exercise (B) in COOL (blue) and HOT (red).
3.2. Gene expression
HSP-70 mRNA expression demonstrated a significant time effect (2.1-fold, p = 0.003), but not a group effect (p = 0.433) or interaction (p = 0.462, Figure 2A). At 4 h after exercise, HSP-70 mRNA increased significantly only in HOT compared to pre (2.4-fold, p = 0.045), but not in COOL (1.9-fold, p = 0.207). In contrast, HSP-90 mRNA did not change in either group at 4 h (COOL, 1.3-fold, p = 0.189; HOT, 1.4-fold, p = 0.080, Figure 2B), although a time effect was observed (1.4-fold, p = 0.005) similar to HSP-70. PGC-1α mRNA demonstrated a significant time effect (6.0-fold, p = 0.002), but not a group effect (p = 0.080) or interaction (p = 0.110, Figure 3A). PGC-1α mRNA levels increased in HOT (8.4-fold, p = 0.010) at 4 h compared to pre, but not in COOL (3.8-fold, p = 0.424). PDK4 mRNA expression demonstrated a significant time effect (10.6-fold, p = 0.006), but not a group effect (p = 0.270) or interaction (p = 0.300, Figure 3B). In addition, an increase in PDK4 mRNA was observed only in HOT at 4 h compared to pre (14.1-fold, p = 0.047), but not in COOL (7.6-fold, p = 0.412). SDHa mRNA expression did not reveal a significant time effect (1.1-fold, p = 0.235), group effect (p = 0.559), or interaction (p = 0.503, Figure 3C). HIF-1α mRNA expression demonstrated a significant time effect (1.9-fold, p = 0.002), but not a group effect (p = 0.274) or interaction (p = 0.212, Figure 4A). A significant increase in HIF-1α mRNA levels was observed only in HOT at 4 h compared to pre (2.2-fold, p = 0.018), but not in COOL (1.6-fold, p = 0.315). VEGF, ANGPT1 and CD31 mRNA expressions demonstrated significant time effects (2.4-fold, p = 0.006, 1.5-fold, p = 0.019, 1.7-fold, p = 0.020, respectively; Figures 4B–D), but did not change at 4 h compared to pre in either group (VEGF: COOL, 2.3-fold, p = 0.293; HOT, 2.5-fold, p = 0.136; ANGPT1: COOL, 1.7-fold, p = 0.221; HOT, 1.3-fold, p = 0.901; CD31: COOL, 1.7-fold, p = 0.122; HOT, 1.7-fold, p = 0.153). PFK mRNA did not show a significant time effect (1.1-fold, p = 0.227), group effect (p = 0.434), or interaction (p = 0.185, Figure 5A). MCT1 and MCT4 mRNA expression demonstrated a significant time effect (1.8-fold, p = 0.022; 1.3-fold, p = 0.023, respectively; Figures 5B,C) but did not change in both groups at 4 h compared to pre (MCT1: COOL, 1.9-fold, p = 0.067; HOT, 1.6-fold, p = 0.146; MCT4: COOL, 1.3-fold, p = 0.442; HOT, 1.3-fold, p = 0.767). We did not observe any significant effects on sex in any valuables (HSP-70, p = 0.268; HSP-90, p = 0.184; PGC-1α, p = 0.164; PDK4, p = 0.446; SDHa, p = 0.803; HIF-1α, p = 0.461; VEGF, p = 0.424; ANGPT1, p = 0.245; CD31, p = 0.414; PFK, p = 0.358; MCT1, p = 0.374; MCT4, p = 0.720).
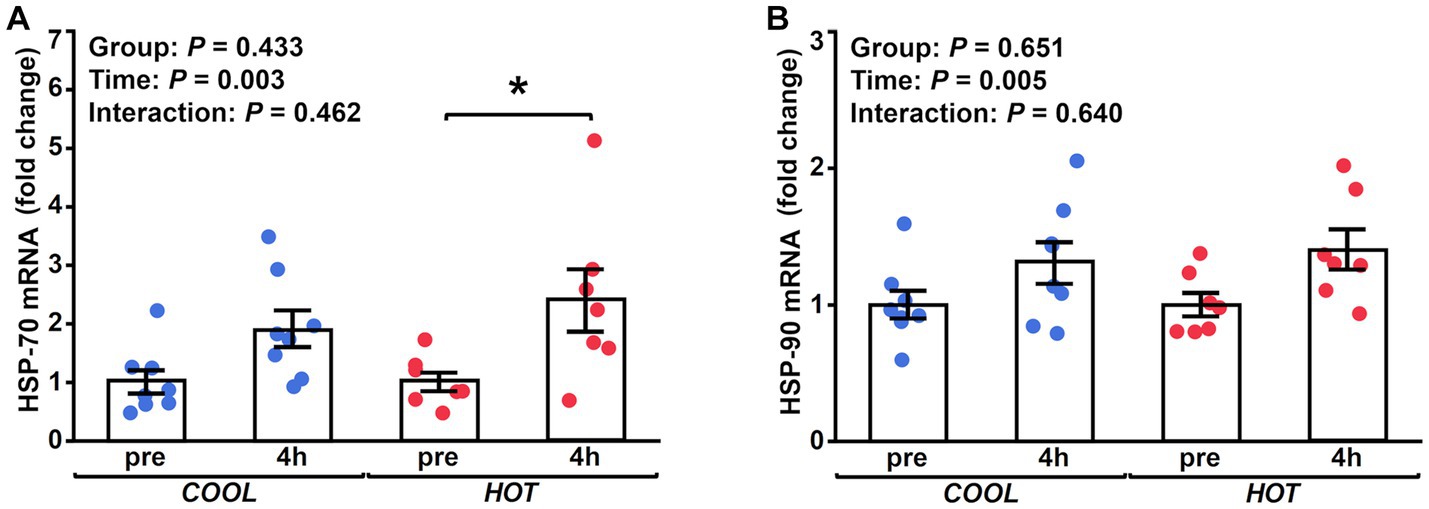
Figure 2. Fold changes in the mRNA expression of HSP-70 (A) and HSP-90 (B) at 4 h after exercise compared to before exercise (pre) in COOL (blue) and HOT (red). *Significant differences from pre (p < 0.05).
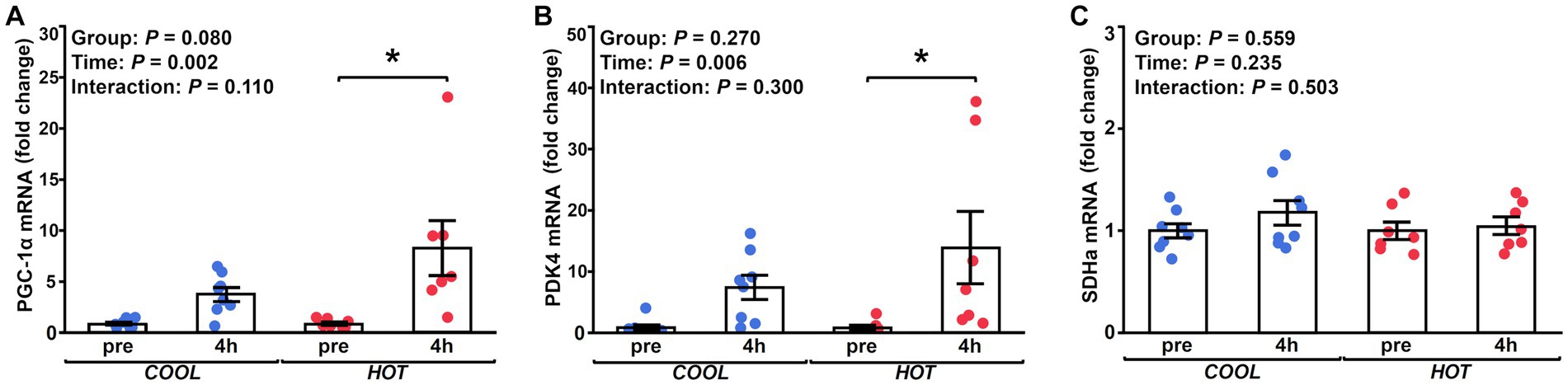
Figure 3. Fold changes in the mRNA expression of PGC-1α (A), PDK4 (B), and SDHa (C) at 4 h after exercise compared to before exercise (pre) in COOL (blue) and HOT (red). *Significant differences from pre (p < 0.05).
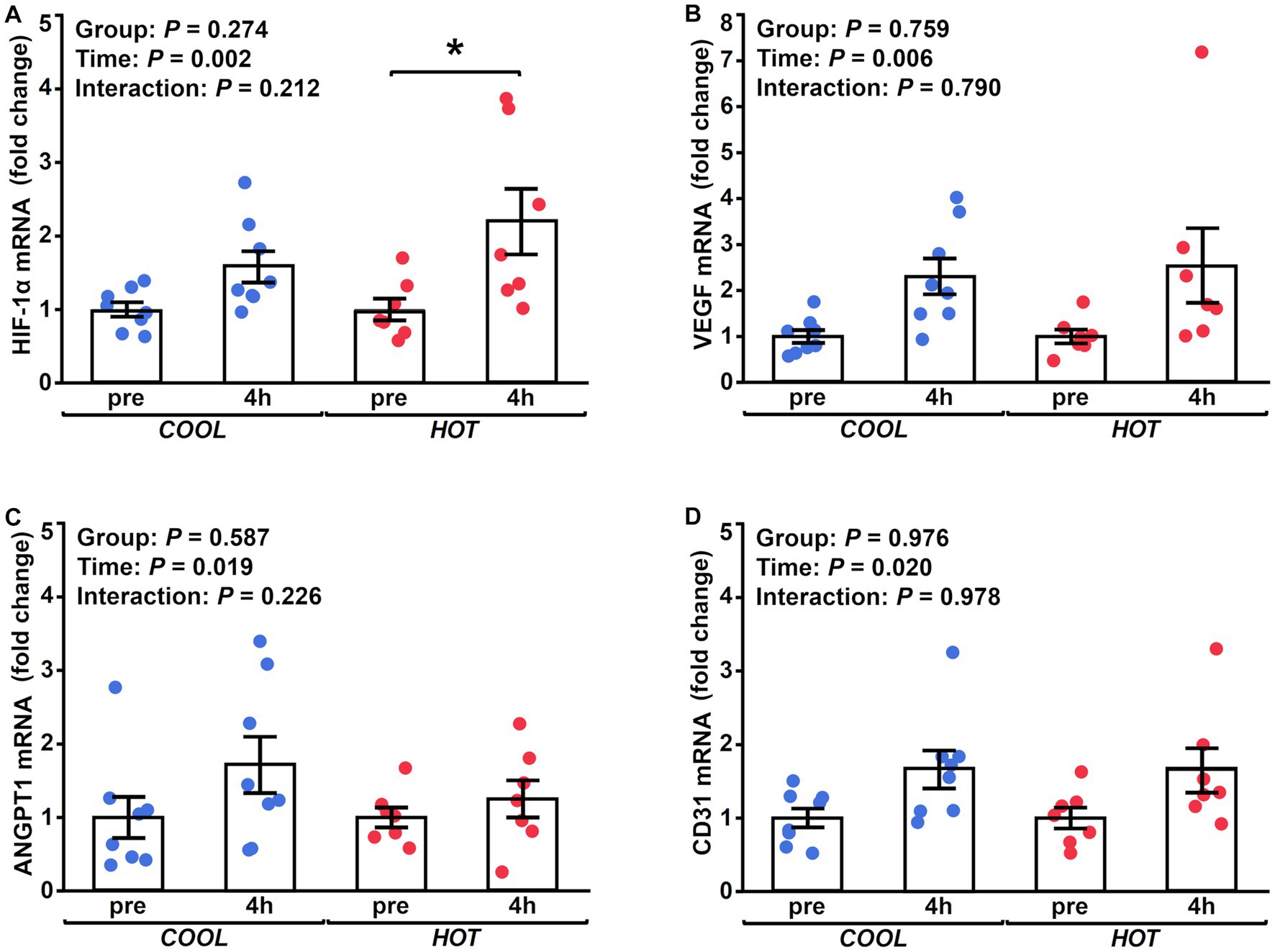
Figure 4. Changes in the mRNA expression of HIF-1α (A), VEGF (B) and ANGPT1 (C), and CD31 (D) at 4 h after exercise compared to before exercise (pre) in COOL (blue) and HOT (red). *Significant differences from pre (p < 0.05).
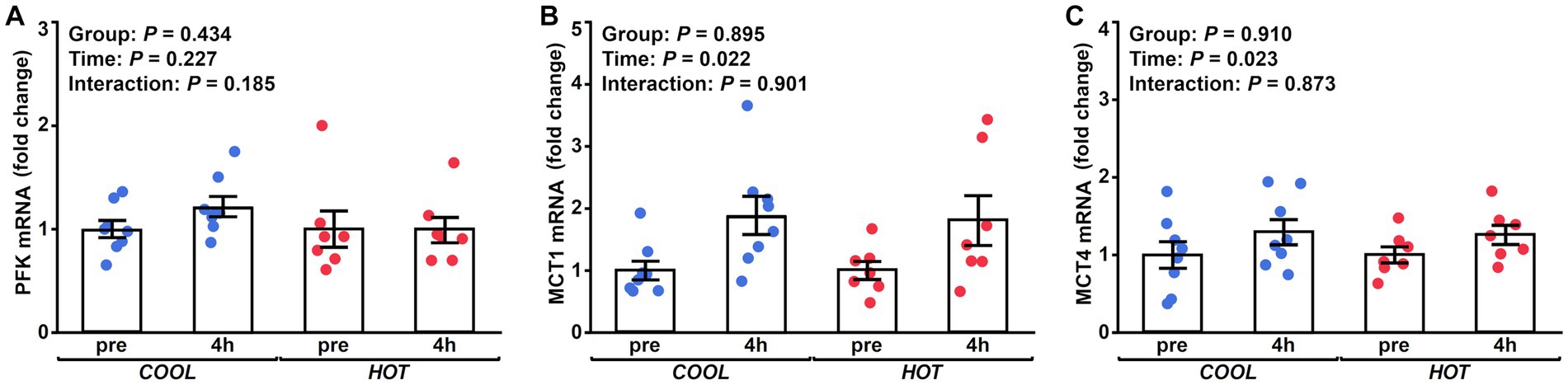
Figure 5. Changes in the mRNA expression of PFK (A), MCT1 (B), and MCT4 (C) at 4 h after exercise compared to before exercise (pre) in COOL (blue) and HOT (red).
4. Discussion
Our main finding in this study is that exercise in a hot environment upregulates the expression of HSP-70 mRNA and PGC-1α mRNA in Thoroughbred horses. These results indicate that the combination of heat exposure and exercise facilitate the activation of heat stress responses and mitochondrial adaptations in Thoroughbred horses.
HSP-70 is classified as molecular chaperone that plays a central role in the cellular stress response to hot environments and has a protective function against cell injury associated with adverse stresses (41). Previous studies have shown that acute thermal stress with an increase in body temperature enhances HSP-70 mRNA expression in various species, including humans and rodents (7, 15, 42, 43). Heat shock factor 1, which is a direct transcriptional activator that induces heat shock gene transcription following heat stress, directly activates the expression of PGC-1α, a master regulator in mitochondrial biogenesis (44, 45). Although we did not measure body core temperature during exercise in this study, previous studies have demonstrated that pulmonary artery temperature during exercise under hyperthermic conditions (WBGT 28°C) is 1–2°C higher than that under cool conditions (WBGT 15°C) in horses (25–27). Therefore, it is reasonable to assume that core and muscle temperature in HOT was also higher than that in COOL, inducing sufficient thermal stress to upregulate the expression of these genes. Furthermore, the expression of PGC-1α mRNA is enhanced in an intensity-dependent manner (46–48). In horses, several studies have demonstrated that VO2 during submaximal exercise in hot conditions is higher compared to that at the same speed in cool conditions (26, 27), and increased relative workload may also contribute the upregulation of PGC-1α mRNA. Therefore, additional increases in core and muscle temperatures with the combination of heat exposure and exercise enhance HSP-70 and PGC-1α mRNA responses, which may be beneficial for cytoprotection and mitochondrial biogenesis.
While the expression of HSP-70 mRNA increased following exercise, no significant change was observed in HSP-90 mRNA. HSP-90, similar to HSP-70, plays a crucial role in cytoprotection and acts as biochemical sensors for various stressors, including heat shock and exercise (41). The extent of HSPs induction following a single bout of exercise depends on several factors, including exercise modality, duration, intensity, and training status of the subject (41, 49). Skidmore et al. demonstrated that a combination of exercise and a hot environment increases synergistic HSP-70 induction compared to either alone in rats (15). In addition, Cuthbert et al. demonstrated that HSP-70 mRNA increases 2- to 4-fold after moderate-intensity exercise (60% VO2peak) in trained humans, while HSP-90 mRNA remains unchanged. Our findings are consistent with the results of Cuthbert et al. that indicate that the sensitivity response to stress differs depending of the type of HSP and that HSP-70 may be a more sensitive marker of heat stress compared with HSP-90 in trained Thoroughbred horses.
In the present study, we detected increased PDK4 mRNA coincident with increased PGC-1α mRNA in Thoroughbred horses training in hot conditions (21, 22). Several studies have demonstrated that muscle glycogen utilization is reduced with heat acclimation (8, 50). Thus, the improved muscle energy metabolism with heat acclimation may involve a shift in energy fuel utilization from glucose oxidation to fatty acid oxidation due to the upregulation of PDK4.
In a hot environment, cutaneous blood flow increases through peripheral vasodilation for heat dissipation in horses and humans (51, 52). During prolonged low-to-moderate intensity exercise, muscle blood flow is not reduced because an increase in cardiac output can compensate the increased skin blood flow (2, 53). However, González-Alonso and Calbet demonstrated that blood flow to exercising muscle is reduced during high-intensity exercise (cycling at 80% of peak power output) starting with high skin and core temperature in humans (54). Therefore, the blood flow demands from skin and muscle may exceed the cardiac capacity, and blood flow to skeletal muscle may have reduced during high-intensity exercise (90% VO2max) in this study. HIF-1α activates in response to hypoxia in various tissues, including skeletal muscle, and plays an integral role in regulating the various genes involved in the hypoxic response (55). Reduced blood flow to skeletal muscle during intense exercise in hot conditions may cause more severe hypoxia in skeletal muscle tissue. Therefore, exercise in a hot environment may result in cellular adaptation that counteracts the effects of reduced oxygen supply to cells by activating HIF-1α. While we observed increased HIF-1α mRNA, no changes were detected in VEGF, ANGPT1, and CD31 mRNA levels in each group. VEGF, ANGPT1, and CD31 are factors related to angiogenesis and are located downstream of HIF-1α. In contrary to our findings in this study, Gustafsson et al. demonstrated that a single bout of cycling increased VEGF mRNA in human skeletal muscle and that this increase positively correlated with the change in HIF-1α mRNA (56). Additionally, Gustafsson et al., also demonstrated a correlation between the increase in VEGF mRNA and plasma lactate concentration after exercise (56). In humans, exercise in a hot environment often enhances glycolysis in skeletal muscle, which may be probably due to reduced blood flow and oxygen delivery to working muscle (57). In this study, we observed no differences in the plasma lactate concentration between HOT and COOL. Therefore, the reduction in muscle blood flow and HIF-1α induction may be insufficient to enhance angiogenesis in this study.
We observed no changes in PFK, MCT1, and MCT4 mRNA expressions in this study. PFK is a kinase enzyme that phosphorylates fructose 6-phosphate and is the main rate-controlling enzyme of glycolysis. MCT1 and 4 are key factors in mediating lactate transport. There were no differences in the plasma lactate concentration between HOT and COOL, which is reasonable in that gene expressions related to the glycolysis pathway and lactate transport were not enhanced in HOT. Previous studies in humans and horses have showed an augmented intramuscular carbohydrate utilization and an increased blood lactate concentration when comparing prolonged submaximal exercise in a hot environment with that in a cool environment (1, 27, 58). In contrast, Maxwell et al. demonstrated no differences in the rate of muscle glycogenolysis and blood lactate accumulation during intermittent high-intensity exercise between hot and cool environments (59, 60). These results, including our results, indicate that the increased glycolysis and blood lactate accumulation may depend on several factors, including the duration and intensity of exercise, training status of subjects, environmental temperature, and the timing of sample collection.
We acknowledge there are limitations in this study. First, we obtained muscle samples only at 4 h after exercise and did not observe time-course changes of gene expressions over time. Because the time-course changes of gene expressions vary with target markers (61), we could detect more mRNA changes if we collected muscle samples at several time points. Second, we only observed changes in mRNA expression following acute exercise. In humans, 10 days or longer heat acclimation are recommended to induce optimize adaptations (62). Some reports in horses have demonstrated that heat acclimation have beneficial effects on physiological responses similar to humans (29, 63). However, these studies did not evaluate cellular adaptations in skeletal muscle. Therefore, further studies are necessary to fully investigate the long-term effects of heat acclimatization or acclimation on skeletal muscle adaptations.
In summary, we have demonstrated that HSP-70, PGC-1α, HIF-1α, and PDK4 mRNA significantly increased after high-intensity exercise in hot conditions. These increases in gene expressions may facilitate a protective response to heat stress, mitochondrial biogenesis, and fatty acid oxidation. Therefore, our results suggest that heat acclimatization or acclimation for competitions and races in hot conditions is effective in improving aerobic energy metabolism, as well as reducing heat stress. Repeated exposure to heat stress, such as training in natural hot environment or in artificial heated environment, may be effective strategy for competitions and races held in hot environments.
Data availability statement
The original contributions presented in the study are included in the article/supplementary material, further inquiries can be directed to the corresponding author.
Ethics statement
The animal study was reviewed and approved by the Animal Welfare and Ethics Committee of the Japan Racing Association’s Equine Research Institute (accession number 21-1).
Author contributions
YE, KM, YT, and HO contributed to the conception and design of the study. YE, KM, YT, TY, AK, TM, HM, and HO carried out the experiments. YE, KM, YT, TY, and HO performed data collection. YE, AK, TM, and HM analyzed the data. YE wrote the first draft of the manuscript. All authors contributed to the article and approved the submitted version.
Funding
The research was funded by the Japan Racing Association.
Acknowledgments
The authors thank Naoko Honma and Ayako Hanyu of the Equine Research Institute for their technical assistance.
Conflict of interest
YE, KM, YT, TY, and HO are employees of the Japan Racing Association.
The remaining authors declare that the research was conducted in the absence of any commercial or financial relationships that could be construed as a potential conflict of interest.
Publisher’s note
All claims expressed in this article are solely those of the authors and do not necessarily represent those of their affiliated organizations, or those of the publisher, the editors and the reviewers. Any product that may be evaluated in this article, or claim that may be made by its manufacturer, is not guaranteed or endorsed by the publisher.
References
1. Febbraio, MA, Snow, RJ, Hargreaves, M, Stathis, CG, Martin, IK, and Carey, MF. Muscle metabolism during exercise and heat stress in trained men: effect of acclimation. J Appl Physiol (1985). (1994) 76:589–97. doi: 10.1152/jappl.1994.76.2.589
2. Nielsen, B, Hales, JR, Strange, S, Christensen, NJ, Warberg, J, and Saltin, B. Human circulatory and thermoregulatory adaptations with heat acclimation and exercise in a hot, dry environment. J Physiol. (1993) 460:467–85. doi: 10.1113/jphysiol.1993.sp019482
3. Lorenzo, S, Halliwill, JR, Sawka, MN, and Minson, CT. Heat acclimation improves exercise performance. J Appl Physiol (1985). (2010) 109:1140–7. doi: 10.1152/japplphysiol.00495.2010
4. Keiser, S, Fluck, D, Huppin, F, Stravs, A, Hilty, MP, and Lundby, C. Heat training increases exercise capacity in hot but not in temperate conditions: a mechanistic counter-balanced cross-over study. Am J Physiol Heart Circ Physiol. (2015) 309:H750–61. doi: 10.1152/ajpheart.00138.2015
5. Young, AJ, Sawka, MN, Levine, L, Cadarette, BS, and Pandolf, KB. Skeletal muscle metabolism during exercise is influenced by heat acclimation. J Appl Physiol (1985). (1985) 59:1929–35. doi: 10.1152/jappl.1985.59.6.1929
6. Maunder, E, Plews, DJ, Wallis, GA, Brick, MJ, Leigh, WB, Chang, WL, et al. Temperate performance and metabolic adaptations following endurance training performed under environmental heat stress. Physiol Rep. (2021) 9:e14849. doi: 10.14814/phy2.14849
7. Tamura, Y, Matsunaga, Y, Masuda, H, Takahashi, Y, Takahashi, Y, Terada, S, et al. Postexercise whole body heat stress additively enhances endurance training-induced mitochondrial adaptations in mouse skeletal muscle. Am J Physiol Regul Integr Comp Physiol. (2014) 307:R931–43. doi: 10.1152/ajpregu.00525.2013
8. Kirwan, JP, Costill, DL, Kuipers, H, Burrell, MJ, Fink, WJ, Kovaleski, JE, et al. Substrate utilization in leg muscle of men after heat acclimation. J Appl Physiol (1985). (1987) 63:31–5. doi: 10.1152/jappl.1987.63.1.31
9. Kuhlenhoelter, AM, Kim, K, Neff, D, Nie, Y, Blaize, AN, Wong, BJ, et al. Heat therapy promotes the expression of Angiogenic regulators in human skeletal muscle. Am J Physiol Regul Integr Comp Physiol. (2016) 311:R377–91. doi: 10.1152/ajpregu.00134.2016
10. Kregel, KC. Heat shock proteins: modifying factors in physiological stress responses and acquired Thermotolerance. J Appl Physiol (1985). (2002) 92:2177–86. doi: 10.1152/japplphysiol.01267.2001
11. Moseley, PL. Heat shock proteins and heat adaptation of the whole organism. J Appl Physiol (1985). (1997) 83:1413–7. doi: 10.1152/jappl.1997.83.5.1413
12. Henstridge, DC, Bruce, CR, Drew, BG, Tory, K, Kolonics, A, Estevez, E, et al. Activating Hsp72 in rodent skeletal muscle increases mitochondrial number and oxidative capacity and decreases insulin resistance. Diabetes. (2014) 63:1881–94. doi: 10.2337/db13-0967
13. Katschinski, DM, le, L, Heinrich, D, Wagner, KF, Hofer, T, Schindler, SG, et al. Heat induction of the Unphosphorylated form of hypoxia-inducible factor-1alpha is dependent on heat shock Protein-90 activity. J Biol Chem. (2002) 277:9262–7. doi: 10.1074/jbc.M110377200
14. McClung, JP, Hasday, JD, He, JR, Montain, SJ, Cheuvront, SN, Sawka, MN, et al. Exercise-heat acclimation in humans alters baseline levels and ex vivo heat Inducibility of Hsp72 and Hsp90 in peripheral blood mononuclear cells. Am J Physiol Regul Integr Comp Physiol. (2008) 294:R185–91. doi: 10.1152/ajpregu.00532.2007
15. Skidmore, R, Gutierrez, JA, Guerriero, V Jr, and Kregel, KC. Hsp70 induction during exercise and heat stress in rats: role of internal temperature. Am J Phys. (1995) 268:R92–7. doi: 10.1152/ajpregu.1995.268.1.R92
16. Morton, JP, Holloway, K, Woods, P, Cable, NT, Burniston, J, Evans, L, et al. Exercise training-induced gender-specific heat shock protein adaptations in human skeletal muscle. Muscle Nerve. (2009) 39:230–3. doi: 10.1002/mus.21182
17. Gillum, T, Kuennen, M, Gourley, C, Dokladny, K, Schneider, S, and Moseley, P. Sex differences in heat shock protein 72 expression in peripheral blood mononuclear cells to acute exercise in the heat. Int J Endocrinol Metab. (2013) 11:e8739. doi: 10.5812/ijem.8739
18. Mee, JA, Gibson, OR, Tuttle, JA, Taylor, L, Watt, PW, Doust, J, et al. Leukocyte Hsp72 Mrna transcription does not differ between males and females during heat acclimation. Temperature (Austin). (2016) 3:549–56. doi: 10.1080/23328940.2016.1214336
19. Liu, CT, and Brooks, GA. Mild heat stress induces mitochondrial biogenesis in C2c12 Myotubes. J Appl Physiol (1985). (2012) 112:354–61. doi: 10.1152/japplphysiol.00989.2011
20. Salgado, RM, Sheard, AC, Vaughan, RA, Parker, DL, Schneider, SM, Kenefick, RW, et al. Mitochondrial efficiency and exercise economy following heat stress: a potential role of uncoupling protein 3. Physiol Rep. (2017) 5:e13054. doi: 10.14814/phy2.13054
21. Wende, AR, Huss, JM, Schaeffer, PJ, Giguere, V, and Kelly, DP. Pgc-1alpha Coactivates Pdk4 gene expression via the orphan nuclear receptor Erralpha: a mechanism for transcriptional control of muscle glucose metabolism. Mol Cell Biol. (2005) 25:10684–94. doi: 10.1128/MCB.25.24.10684-10694.2005
22. Pettersen, IKN, Tusubira, D, Ashrafi, H, Dyrstad, SE, Hansen, L, Liu, XZ, et al. Upregulated Pdk4 expression is a sensitive marker of increased fatty acid oxidation. Mitochondrion. (2019) 49:97–110. doi: 10.1016/j.mito.2019.07.009
23. Heesch, MW, Shute, RJ, Kreiling, JL, and Slivka, DR. Transcriptional control, but not subcellular location, of Pgc-1alpha is altered following exercise in a hot environment. J Appl Physiol (1985). (2016) 121:741–9. doi: 10.1152/japplphysiol.01065.2015
24. Hodgson, DR, Davis, RE, and McConaghy, FF. Thermoregulation in the horse in response to exercise. Br Vet J. (1994) 150:219–35. doi: 10.1016/S0007-1935(05)80003-X
25. Geor, RJ, McCutcheon, LJ, Ecker, GL, and Lindinger, MI. Thermal and cardiorespiratory responses of horses to submaximal exercise under hot and humid conditions. Equine Vet J Suppl. (1995) 20:125–32. doi: 10.4085/1062-6050-50.9.0710.1111/j.2042-3306.1995.tb05018.x
26. Ebisuda, Y, Mukai, K, Takahashi, Y, and Ohmura, H. Effect of high ambient temperature on physiological responses during incremental exercise in thoroughbred horses. Comparative Exercise Physiology. (2023) 19:159–67. doi: 10.3920/CEP220018
27. MARLIN, DJ, SCOTT, CM, SCHROTER, RC, MILLS, PC, HARRIS, RC, HARRIS, PA, et al. Physiological responses in nonheat acclimated horses performing treadmill exercise in cool (20°C/40%RH), hot dry (30°C/40%RH) and hot humid (30°C/80%RH) conditions. Equine Vet J Suppl. (1996) 28:70–84. doi: 10.1111/j.2042-3306.1996.tb05034.x
28. Hodgson, DR. Thermoregulation In: DR Hodgson, KH McKeever, and CM McGowan, editors. The Athletic Horse; Principles and Practice of Equine Sports Medicine. 2nd ed. St. Louis: Saunders-Elsevier (2014). 108–24.
29. MARLIN, DJ, SCOTT, CM, SCHROTER, RC, HARRIS, RC, HARRIS, PA, ROBERTS, CA, et al. Physiological responses of horses to a treadmill simulated speed and endurance test in high heat and humidity before and after humid heat acclimation. Equine Vet J. (1999) 31:31–42. doi: 10.1111/j.2042-3306.1999.tb03788.x
30. Geor, RJ, McCutcheon, LJ, Ecker, GL, and Lindinger, MI. Heat storage in horses during submaximal exercise before and after humid heat acclimation. J Appl Physiol (1985). (2000) 89:2283–93. doi: 10.1152/jappl.2000.89.6.2283
31. Poso, AR, Eklund-Uusitalo, S, Hyyppa, S, and Pirila, E. Induction of heat shock protein 72 Mrna in skeletal muscle by exercise and training. Equine Vet J Suppl. (2002) 34:214–8. doi: 10.1111/j.2042-3306.2002.tb05421.x
32. HILL, EW, EIVERS, SS, McGIVNEY, BA, FONSECA, RG, GU, J, SMITH, NA, et al. Moderate and high intensity Sprint exercise induce differential responses in Cox4i2 and Pdk4 gene expression in thoroughbred horse skeletal muscle. Equine Vet J Suppl. (2010) 42:576–81. doi: 10.1111/j.2042-3306.2010.00206.x
33. Eivers, SS, McGivney, BA, Fonseca, RG, MacHugh, DE, Menson, K, Park, SD, et al. Alterations in oxidative gene expression in equine skeletal muscle following exercise and training. Physiol Genomics. (2010) 40:83–93. doi: 10.1152/physiolgenomics.00041.2009
34. Roneus, M, Lindholm, A, and Asheim, A. Muscle characteristics in thoroughbreds of different ages and sexes. Equine Vet J. (1991) 23:207–10. doi: 10.1111/j.2042-3306.1991.tb02757.x
35. Jones, JH, Longworth, KE, Lindholm, A, Conley, KE, Karas, RH, Kayar, SR, et al. Oxygen transport during exercise in large mammals. I. Adaptive variation in oxygen demand. J Appl Physiol. (1989) 67:862–70. doi: 10.1152/jappl.1989.67.2.862
36. Birks, EK, Ohmura, H, and Jones, JH. Measuring Vo2 in hypoxic and Hyperoxic conditions using dynamic gas mixing with a flow-through indirect calorimeter. J Equine Sci. (2019) 30:87–92. doi: 10.1294/jes.30.87
37. Mukai, K, Kitaoka, Y, Takahashi, Y, Takahashi, T, Takahashi, K, and Ohmura, H. Moderate-intensity training in hypoxia improves exercise performance and glycolytic capacity of skeletal muscle in horses. Physiol Rep. (2021) 9:e15145. doi: 10.14814/phy2.15145
38. Fedak, MA, Rome, L, and Seeherman, HJ. One-step N2-dilution technique for calibrating open-circuit Vo2 measuring systems. J Appl Physiol. (1981) 51:772–6. doi: 10.4085/1062-6050-50.9.0710.1152/jappl.1981.51.3.772
39. Okabe, K, Mukai, K, Ohmura, H, Takahashi, T, and Miyata, H. Effect of acute high-intensity exercise in Normobaric hypoxia on thoroughbred skeletal muscle. J Sports Med Phys Fitness. (2017) 57:711–9. doi: 10.23736/S0022-4707.16.06154-5
40. Mahoney, DJ, Carey, K, Fu, MH, Snow, R, Cameron-Smith, D, Parise, G, et al. Real-time RT-PCR analysis of housekeeping genes in human skeletal muscle following acute exercise. Physiol Genomics. (2004) 18:226–31. doi: 10.1152/physiolgenomics.00067.2004
41. Kruger, K, Reichel, T, and Zeilinger, C. Role of heat shock proteins 70/90 in exercise physiology and exercise immunology and their diagnostic potential in sports. J Appl Physiol (1985). (2019) 126:916–27. doi: 10.1152/japplphysiol.01052.2018
42. Ihsan, M, Deldicque, L, Molphy, J, Britto, F, Cherif, A, and Racinais, S. Skeletal muscle signaling following whole-body and localized heat exposure in humans. Front Physiol. (2020) 11:839. doi: 10.3389/fphys.2020.00839
43. Cuthbert, RL, Shute, RJ, and Slivka, DR. Skeletal muscle cold shock and heat shock protein Mrna response to aerobic exercise in different environmental temperatures. Temperature (Austin). (2019) 6:77–84. doi: 10.1080/23328940.2018.1555414
44. Ma, X, Xu, L, Alberobello, AT, Gavrilova, O, Bagattin, A, Skarulis, M, et al. Celastrol protects against obesity and metabolic dysfunction through activation of a Hsf1-Pgc1alpha transcriptional Axis. Cell Metab. (2015) 22:695–708. doi: 10.1016/j.cmet.2015.08.005
45. Xu, L, Ma, X, Bagattin, A, and Mueller, E. The transcriptional coactivator Pgc1alpha protects against Hyperthermic stress via cooperation with the heat shock factor Hsf1. Cell Death Dis. (2016) 7:e2102. doi: 10.1038/cddis.2016.22
46. Nordsborg, NB, Lundby, C, Leick, L, and Pilegaard, H. Relative workload determines exercise-induced increases in Pgc-1alpha Mrna. Med Sci Sports Exerc. (2010) 42:1477–84. doi: 10.1249/MSS.0b013e3181d2d21c
47. Brandt, N, Dethlefsen, MM, Bangsbo, J, and Pilegaard, H. Pgc-1alpha and exercise intensity dependent adaptations in mouse skeletal muscle. PLoS One. (2017) 12:e0185993. doi: 10.1371/journal.pone.0185993
48. Egan, B, Carson, BP, Garcia-Roves, PM, Chibalin, AV, Sarsfield, FM, Barron, N, et al. Exercise intensity-dependent regulation of peroxisome proliferator-activated receptor Coactivator-1 Mrna abundance is associated with differential activation of upstream Signalling kinases in human skeletal muscle. J Physiol. (2010) 588:1779–90. doi: 10.1113/jphysiol.2010.188011
49. Vogt, M, Puntschart, A, Geiser, J, Zuleger, C, Billeter, R, and Hoppeler, H. Molecular adaptations in human skeletal muscle to endurance training under simulated hypoxic conditions. J Appl Physiol (1985). (2001) 91:173–82. doi: 10.1152/jappl.2001.91.1.173
50. King, DS, Costill, DL, Fink, WJ, Hargreaves, M, and Fielding, RA. Muscle metabolism during exercise in the heat in Unacclimatized and acclimatized humans. J Appl Physiol (1985). (1985) 59:1350–4. doi: 10.1152/jappl.1985.59.5.1350
51. McConaghy, FF, Hodgson, DR, Rose, RJ, and Hales, JR. Redistribution of cardiac output in response to heat exposure in the pony. Equine Vet J Suppl. (1996) 22:42–6. doi: 10.1111/j.2042-3306.1996.tb05030.x
52. Johnson, JM. Exercise in a hot environment: the skin circulation. Scand J Med Sci Sports. (2010) 20:29–39. doi: 10.1111/j.1600-0838.2010.01206.x
53. Nielsen, B, Savard, G, Richter, EA, Hargreaves, M, and Saltin, B. Muscle blood flow and muscle metabolism during exercise and heat stress. J Appl Physiol (1985). (1990) 69:1040–6. doi: 10.1152/jappl.1990.69.3.1040
54. Gonzalez-Alonso, J, and Calbet, JA. Reductions in systemic and skeletal muscle blood flow and oxygen delivery limit maximal aerobic capacity in humans. Circulation. (2003) 107:824–30. doi: 10.1161/01.cir.0000049746.29175.3f
55. Maloyan, A, Eli-Berchoer, L, Semenza, GL, Gerstenblith, G, Stern, MD, and Horowitz, M. Hif-1alpha-targeted pathways are activated by heat acclimation and contribute to acclimation-ischemic cross-tolerance in the heart. Physiol Genomics. (2005) 23:79–88. doi: 10.1152/physiolgenomics.00279.2004
56. Gustafsson, T, Puntschart, A, Kaijser, L, Jansson, E, and Sundberg, CJ. Exercise-induced expression of angiogenesis-related transcription and growth factors in human skeletal muscle. Am J Phys. (1999) 276:H679–85. doi: 10.1152/ajpheart.1999.276.2.H679
57. Fink, WJ, Costill, DL, and Van Handel, PJ. Leg muscle metabolism during exercise in the heat and cold. Eur J Appl Physiol Occup Physiol. (1975) 34:183–90. doi: 10.1007/BF00999931
58. Febbraio, MA, Snow, RJ, Stathis, CG, Hargreaves, M, and Carey, MF. Effect of heat stress on muscle energy metabolism during exercise. J Appl Physiol (1985). (1994) 77:2827–31. doi: 10.1152/jappl.1994.77.6.2827
59. Maxwell, NS, Aitchison, TC, and Nimmo, MA. The effect of climatic heat stress on intermittent supramaximal running performance in humans. Exp Physiol. (1996) 81:833–45. doi: 10.1113/expphysiol.1996.sp003980
60. Maxwell, NS, Gardner, F, and Nimmo, MA. Intermittent running: muscle metabolism in the heat and effect of Hypohydration. Med Sci Sports Exerc. (1999) 31:675–83. doi: 10.1097/00005768-199905000-00009
61. Kuang, J, McGinley, C, Lee, MJ, Saner, NJ, Garnham, A, and Bishop, DJ. Interpretation of exercise-induced changes in human skeletal muscle Mrna expression depends on the timing of the post-exercise biopsies. PeerJ. (2022) 10:e12856. doi: 10.7717/peerj.12856
62. Periard, JD, Eijsvogels, TMH, and Daanen, HAM. Exercise under heat stress: thermoregulation, hydration, performance implications, and mitigation strategies. Physiol Rev. (2021) 101:1873–979. doi: 10.1152/physrev.00038.2020
Keywords: heat stress, exercise, Thoroughbred horses, messenger RNA, skeletal muscle
Citation: Ebisuda Y, Mukai K, Takahashi Y, Yoshida T, Kawano A, Matsuhashi T, Miyata H, Kuwahara M and Ohmura H (2023) Acute exercise in a hot environment increases heat shock protein 70 and peroxisome proliferator-activated receptor γ coactivator 1α mRNA in Thoroughbred horse skeletal muscle. Front. Vet. Sci. 10:1230212. doi: 10.3389/fvets.2023.1230212
Edited by:
Morteza Hosseini-Ghaffari, University of Bonn, GermanyReviewed by:
Cesar Rosales-Nieto, Texas State University, United StatesAli Razzaghi, Ferdowsi University of Mashhad, Iran
Copyright © 2023 Ebisuda, Mukai, Takahashi, Yoshida, Kawano, Matsuhashi, Miyata, Kuwahara and Ohmura. This is an open-access article distributed under the terms of the Creative Commons Attribution License (CC BY). The use, distribution or reproduction in other forums is permitted, provided the original author(s) and the copyright owner(s) are credited and that the original publication in this journal is cited, in accordance with accepted academic practice. No use, distribution or reproduction is permitted which does not comply with these terms.
*Correspondence: Yusaku Ebisuda, ZWJpc3VkYUBlcXVpbnN0LmdvLmpw