- Xiangya School of Pharmaceutical Sciences, Central South University, Changsha, Hunan, China
Tissue and organ transplantation continues to be an effective measure for saving the lives of certain critically ill patients. The organ preservation methods that are commonly utilized in clinical practice are presently only capable of achieving short-term storage, which is insufficient for meeting the demand for organ transplantation. Ultra-low temperature storage techniques have garnered significant attention due to their capacity for achieving long-term, high-quality preservation of tissues and organs. However, the experience of cryopreserving cells cannot be readily extrapolated to the cryopreservation of complex tissues and organs, and the latter still confronts numerous challenges in its clinical application. This article summarizes the current research progress in the cryogenic preservation of tissues and organs, discusses the limitations of existing studies and the main obstacles facing the cryopreservation of complex tissues and organs, and finally introduces potential directions for future research efforts.
1. Introduction
Currently, the transplantation of human tissues and organs is recognized as an effective treatment for certain serious diseases. However, according to conservative estimates by the World Health Organization, <10% of the global demand for organ transplants is met, and the COVID-19 pandemic has exacerbated the supply-demand imbalance for human organs (1–3). One significant reason for the shortage of organs is the short safe storage time of donated organs, typically 4–36 h, causing a large number of healthy organs being discarded after exceeding the preservation time (4, 5). At present, the main methods used clinically to extend organ preservation time are static cold storage (SCS) (6) and machine perfusion (MP) (7–9). The preservation of most organs involves immersion in a preservation solution at a temperature range of 0–4°C, which reduces organ metabolism (10). For instance, SCS can typically preserve the heart for ~4 h and the liver for 12 h (11, 12). But metabolic activity does not completely cease during SCS, and the accumulation of toxic metabolites over time can cause organ damage. Recently, machine perfusion has attracted great interest due to its ability to provide oxygen and nutrients for cellular metabolism and remove toxic metabolites that can lead to ROS production, thereby reducing ischemia-reperfusion injury (9, 13). For short-term preservation, methods such as SCS and MP have made some progress compared to the previous ones, but still do not meet the requirements for long-term storage of tissues and organs.
According to the Arrhenius equation, the rate of cellular metabolism slows down with decreasing temperature. It is generally believed that tissues and organs can be stored for extended periods at temperatures below −130°C. Cryopreservation involves maintaining the physiological function of cells, tissues, and organs at ultra-low temperatures (−80°C or −196°C), at which point cellular activities nearly cease. Cryopreservation can effectively halt biological time and overcome the limitations of existing low-temperature storage technologies, making it a powerful tool for achieving long-term storage of tissues and organs (14–16).
The implementation of cryopreservation is not without challenges. For cells, the primary cause of damage during cryoprotective processes is the formation of ice crystals (17, 18). During slow cooling, ice crystals form outside the cells and gradually grow, causing mechanical damage to cells. Simultaneously, water inside the cells will extrude, leading to an increase in intracellular solute concentration and causing osmotic injury. While rapid cooling results in the dominant form of intracellular ice due to insufficient time for the water inside the cells to escape. This leads to mechanical damage to cell organelles, nuclei, and other structures (19, 20). Additionally, during the rewarming process, the recrystallization process can generate large ice crystals, which causes even more severe mechanical damage to the cells (18). So various cryoprotective agents (CPAs), such as glycerol (21), DMSO (22), and trehalose (23), have been used to reduce the damage caused by ice crystals.
At present, cryopreservation has been applied in clinical settings for certain types of cells, but the damage factors and mechanisms during cryopreservation of larger tissues and organs are more complex (24). In the cryopreservation of tissues and organs, it is not only crucial to consider the survival of individual cells, but also the preservation of the ability of cell-to-cell interactions, which makes it challenging for most complex tissues and organs to meet the clinical application requirements after cryopreservation (8, 25). This paper provides an overview of the current state of research on the cryopreservation of tissues and organs, covering commonly used techniques and their potential for development. We also describe the challenges encountered in cryopreserving complex tissues and organs, like thermal stress damage resulting from complicated heat and mass transfer, and discuss possible future directions (as shown in Figure 1).
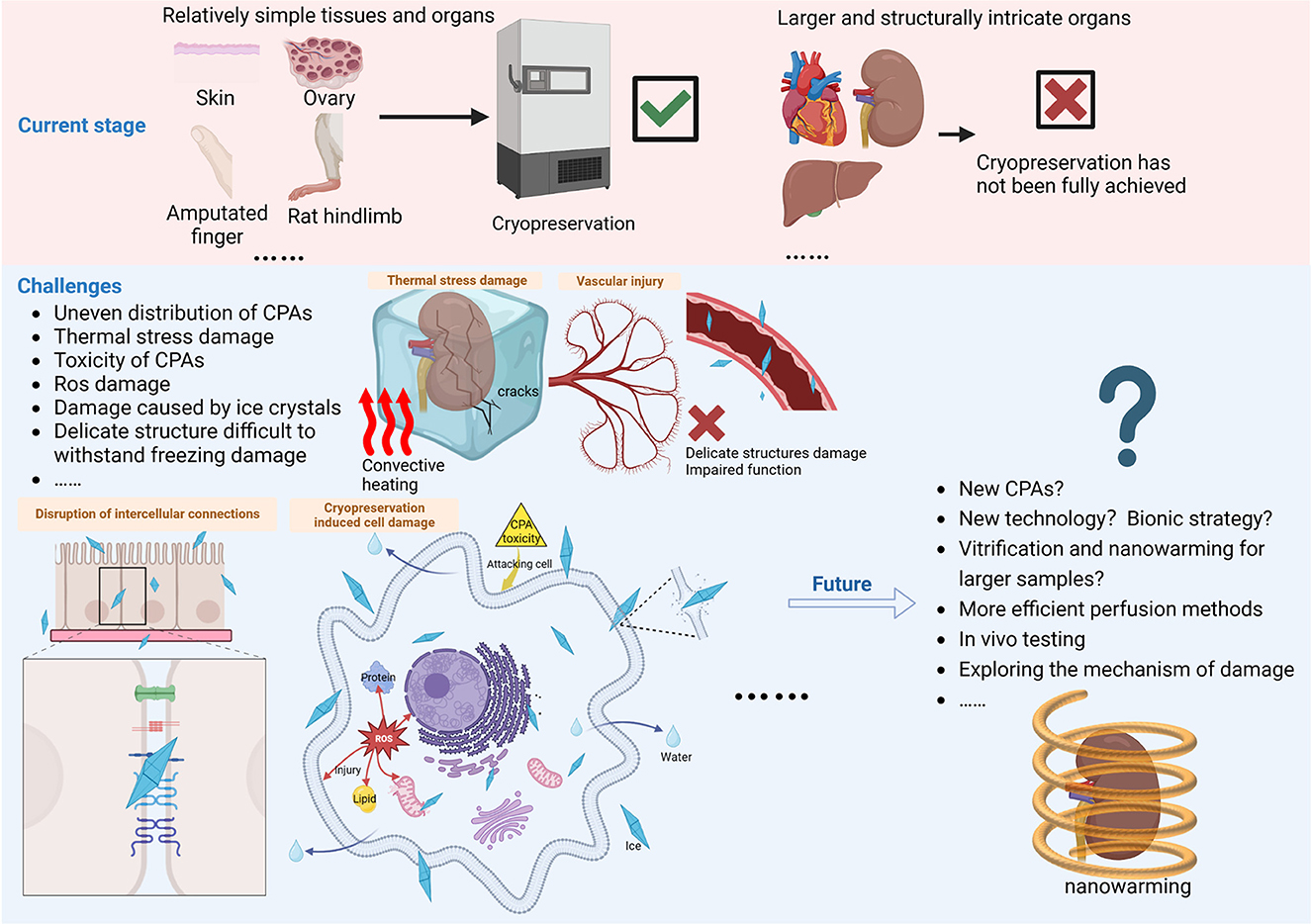
Figure 1. A few tissues and organs have been cryopreserved and transplanted, but cryopreservation of larger and more complex tissues and organs has not yet achieved clinical application. It faces challenges posed by inhomogeneous heat and mass transfer, thermal stress, CPA toxicity, reactive oxygen species, and ice crystals, requiring comprehensive protection measures in terms of macroscopic volume, fine structure, cellular connectivity, and individual cells. Vitrification and nanowarming has made a breakthrough in the volume of cryopreserved biological samples, applying it to the cryopreservation of larger tissues and organs and performing in vivo experiments to evaluate the recovery effect is the direction of future efforts. In addition, the mechanism of damage during cryopreservation needs to be further elucidated in order to explore new CPAs and new technical strategies to accelerate the development of cryopreservation technology. Created with BioRender.com.
2. Current status of tissue and organ cryopreservation
The history of cryopreservation of mammalian organs may be traced back to Gonzales and Luyet's attempt to freeze chick embryo hearts by vitrification in 1950 (26). Over the decades, people have never stopped exploring and have tried to cryopreserve a variety of tissues and organs (as shown in Table 1), and the most commonly used cryopreservation techniques include conventional slow freezing, vitrification, and directional freezing (14). A comparison of their strengths and weaknesses is in the Supplementary Table S1. At present, the technically mature programmable slow freezing method has established standard protocols for cryopreserving various types of cells (such as red blood cells and reproductive cells) (27, 28) and has been developed for clinical cryopreservation of some tissues [such as ovaries (29) and skin (30)]. Conventional slow freezing protocols typically employ a cooling rate of 1°C/min and 1.5 M CPA for cell freezing, the whole process allowing relatively free formation and growth of ice crystals, which is usually fatal for cryopreservation of large-volume tissues and organs.
For this, scientists have used the technique of directional freezing to regulate the growth of ice crystals, where the sample moves through a temperature gradient at a constant velocity to limit the gradual growth of ice crystals across that gradient. The controlled ice crystals appear as lamellae, with cells trapped between the lamellae to reduce mechanical damage (31). Additionally, tight contact between the sample and the highly heat-conductive metal block enables efficient heat dissipation, avoiding the damage to cells caused by freeze-thaw cycles (32). Directional freezing can be used for both slow and rapid cooling, and has been extended to directional vitrification (33). Directed freezing technology demonstrates the capability to achieve uniform cooling rates in tissues and organs, and has been applied in cryopreservation experiments of various types of tissue and organs, such as ovaries (34), rat hindlimbs (35), and pig livers (36). This is a promising approach, but some research results seem to lack sufficient conviction and more efforts are still needed to advance its development.
Vitrification is the rapid freezing of a liquid to allow it to jump through the crystallization zone and form an amorphous or non-crystalline solid state (37). To achieve vitrification, cooling rates faster than the critical cooling rate (CCR) are required to prevent ice crystal formation, and it has been achieved after perfusion of small organs with CPAs (38). Similarly, a very high warming rate is required during the recovery process to avoid devitrification (39, 40). Although using CPAs can decrease both CCR and critical warming rate (CWR), the CWR is usually an order of magnitude higher than the CCR (41), and traditional convective warming is difficult to achieve such a rate. Recently reported magnetic nanoparticle-induced heating technology effectively prevents devitrification and thermal stress during the rewarming process. Ideally, organs would be uniformly loaded with CPAs and magnetic nanoparticles through optimized hypothermic machine perfusion, and then rapidly heated uniformly in a radiofrequency-induced magnetic field after vitrification. Nanowarming has been demonstrated to be feasible in the cryopreservation of various tissues and organs, such as porcine arteries, rat hearts, rat kidneys, and rat livers, although issues such as CPA toxicity, uniform perfusion and washout of the magnetic nanoparticles remain to be addressed. Nonetheless, it is undeniable that this technology has propelled the field of vitrification of organs toward new frontiers (38, 40, 42–46).
So far, the majority of tissues and organs that have been successfully cryopreserved and transplanted are small or structurally simple, such as skin (47), corneas (48), and osteochondral tissue (49). However, successful cases of complex organs are few and far between. To our knowledge, the reported successful cases include ovaries, rat hindlimbs, and human fingers. Ovarian tissue can recover its function after cryopreservation, and transplant recipients [such as sheep (50) and humans (51)] have successfully given birth to offspring. In addition, cryopreserved rat hindlimbs can survive after transplantation (35, 52), although the function of some hindlimbs may not recover. Human amputated fingers can be successfully reimplanted back into the patient's hand and restore function after 30 days of cryopreservation (53, 54). Cryopreservation of small limbs with low content of complex muscle tissue is relatively easy, but larger tissues and organs with more complex structures and functions have not yet been fully achieved, possibly because some structures are too delicate and complex to withstand the adverse effects of the cryopreservation process (53, 55). For example, the delicate capillary network of frozen kidneys can be damaged after nanowarming, with consequent impairment of organ function (56). Functional recovery after cryopreservation of vital organs such as the heart and kidneys has not been accurately assessed, as many researches fail to sufficiently assess biological characteristics and function, and in vivo testing is absent.
3. The challenges of tissue and organ cryopreservation
3.1. Troubles caused by ice crystals
During the cooling and rewarming processes in cryopreservation, uncontrolled ice crystal formation and growth can be fatal to tissue and organ preservation (57). For programmable slow freezing, ice crystals are difficult to avoid even with the best preservation protocols (17, 20), which can cause damage to the intercellular connection and blood vessels in tissues and organs. However, it is often difficult to achieve the optimal cryopreservation conditions for multiple cell types in an organ simultaneously (57, 58). Additionally, the phase transition process of ice crystal formation is accompanied by heat release, which can cause serious damage to tissue cells beyond a certain limit (24). It is worth noting that necessary cell-cell and cell-matrix interactions in tissues are related to intracellular ice formation (IIF), which is inseparable from the low survival rate of tissues after cryopreservation (59–61).
3.2. Toxicity due to high concentration of CPAs
Except for rare reports of cell CPA-free cryopreservation (62), CPAs are indispensable for both tissue and organ cryopreservation. The conventional cryoprotectant DMSO has been shown to have multiple toxic damages to cells, such as damage to proteins (63), mitochondria (64), and extracellular structures (65). Since the function of tissues and organs depends on intercellular junctions (57), damage to cell structures can result in impaired or lost function of tissues and organs after cryopreservation. Cell vitrification typically uses high concentrations of CPAs at 4–8 M, and similarly, organ cryopreservation also requires loading high concentrations of CPAs to inhibit ice crystal formation. For example, Fahy et al. (66) used a cryoprotective solution as high as 9.3 M to perfuse rabbit kidneys for vitrification. In large organs, the time of perfusing protective solution can reach several hours, and high concentrations of CPA can cause damage to cells through osmotically induced mechanical stress, and prolonged exposure can also make CPA toxicity a dominant factor in injury (4, 65). It is crucial to find a balance between the toxicity of CPA and its ability to inhibit ice formation in the cryopreservation of large tissue organs.
3.3. Heat and mass transfer problems in complex structures
Compared to cell suspensions, the processes of heat and mass transfer in tissues and organs appear to be much more complex. In terms of mass transfer, the loading and removal of CPAs and the distribution of water are the main considerations (67). The mass transfer process within the organ becomes complicated due to the different types and arrangements of cells, as well as the interactions between cells. This limits the diffusion of water and CPAs, resulting in difficulties achieving uniform distribution of CPAs in organs. Moreover, the excessive macroscopic volume also hinders the uniform distribution of CPAs in the surface and interior of the organ. For example, during organ perfusion, vascular cells that first come into contact with CPAs are subject to greater toxicity and injury (67–69). A similar problem to mass transfer is faced in heat transfer, which is limited by the macroscopic volume and thermal conductivity of the organ, making it difficult to achieve uniform cooling and rewarming (44, 70). As a result, organs experience thermal stress damage during cryopreservation, which often leads to the rupture of biological samples. The cracks also promote ice crystal formation, especially during the rewarming process (42, 71). The uneven distribution of CPA tends to lead to the appearance of temperature gradients and aggravates the thermal stress damage (72). The complex heat and mass transfer problems seriously hinder the tissue and organ cryopreservation, while the gold standard convective warming cannot meet the need for rapid and uniform heating of large biological samples. Urgent measures are needed to address these pressing problems.
3.4. Oxidative damage
Under normal physiological conditions, cells maintain a balance between oxidation and reduction reactions. However, this balance is disrupted during cryopreservation by decreased enzyme activity, ATP deficiency, and Ca2+ accumulation, leading to oxidative stress and the production of a large number of reactive oxygen species (ROS) (73, 74). Excessive ROS can induce a multitude of deleterious effects, including but not limited to DNA damage, protein oxidation, and lipid peroxidation (75). The adverse effects of ROS are further aggravated by the fact that mitochondria, the source of ROS, are also attacked by ROS. Damaged mitochondria produce less ATP and more ROS, which further exacerbates cellular oxidative damage (76, 77). The series of damages caused by oxidative stress can impair cellular functions and decrease vitality post-cryopreservation, accelerating cell apoptosis and necrosis in conjunction with other types of damage (78, 79). An additional problem that organs face during ex vivo cryopreservation is ischemia-reperfusion injury, which is caused by redox imbalance. Research has shown that a substantial amount of ROS is generated during ischemia (hypoxia) and reperfusion (reoxygenation) (80, 81), leading to tissue damage and compromising the quality of the transplantable organs.
4. Possible research directions for tissue and organ cryopreservation
4.1. Reducing the toxicity caused by CPAs
The toxicity of conventional CPAs has been a persistent obstacle to the development of cryopreservation, prompting researchers to explore solutions. Substantial research has been devoted to the development of high-efficiency and low-toxicity CPAs, such as antifreeze proteins (82), macromolecular polymers (83, 84), and nanomaterials (85–87). Besides, combining different CPAs in specific molar ratios as a CPA cocktail can reduce toxicity. Examples of such cocktails include improved VS41A, Natural Deep Eutectic Systems (NADES), and others used for vitrification of biological samples (88–91). Notably, mathematical models have been used in the development of low-toxicity vitrification solutions (66, 92, 93), a form similar to computer simulation of drug development or a trend in the development of new CPAs. And the method of hypothermic machine perfusion with multiple steps of loading/unloading CPAs can reduce the toxicity and osmotic damage to organs (94). In vitrification, toxicity damage may have become the main challenge (45), and identifying more favorable perfusion schemes and ideal CPAs will strongly promote the development of cryopreservation.
4.2. Finding new methods of cryopreservation
Tissue and organ cryopreservation is a huge challenge that requires cross-disciplinary participation and more effective new technologies to break existing barriers. According to recent studies, strategies such as nanoparticle-mediated intracellular delivery of trehalose (95), microfluidics-controlled hydrogel for cell encapsulation (96), and sand-mediated ice nucleation (97) have achieved good results in cell cryopreservation. Physical fields have also played a huge role in cryopreservation, such as magnetic fields (45) and laser fields (98), which can mediate rapid nanowarming to avoid ice crystals during the thawing process. And the appropriate use of additives has been found to improve the recovery of cells after cryopreservation, such as antioxidants and caspase inhibitors (99–101). In addition, the biochemical perspective has given researchers much insight. Dou et al. significantly enhanced cold tolerance by feeding L-proline to Japanese carpenter ants, innovatively linking genetic variation to cold resistance in ants through exogenous feeding of CPA (102). This indicates that a bionic strategy of learning from nature can provide new techniques and ideas for tissue and organ cryopreservation.
4.3. Exploring the mechanism of damage in cryopreservation
The current understanding of the damage mechanisms during cryopreservation mainly focuses on the dispersed cellular level, while the mechanisms targeting the damage to the multi-level structure and physiological functions of tissues and organs are still unclear. Research on the cryopreservation of tissues and organs mainly focuses on the integrity of macroscopic structures, and there is a lack of in-depth exploration at the cellular and molecular levels within the organs. For example, recent research on vitrification and nanowarming of rat hearts showed that the macroscopic structure remained intact, but the cause of functional damage was not clear and it was speculated that it may be due to the toxicity of the CPA or perfusion injury (103). However, the toxic mechanism of the vitrification solution on tissue organs has not been elucidated, and the mechanism of toxic damage still needs to be demonstrated at the microscopic molecular level (65, 88). Without further explanation of the damage mechanism of tissue and organ during cryopreservation, it will be difficult to apply targeted methods to improve the effectiveness of cryoprotection.
5. Conclusion
Cryopreservation of a small number of tissues and organs has been clinically adopted, but complex organs like the heart may fail to recover due to damage to their highly refined structure. During cryopreservation, tissues and organs are primarily subjected to damage from ice crystal formation, thermal stress, reactive oxygen species, and toxicity of CPAs, and the biological features and functional testing of the organs after recovery are relatively lacking. The combination of vitrification and nanowarming technology has shown remarkable performance in maintaining the structural integrity of small animal organs, and expanding this technique to larger tissue and organs with in vivo functional tests after recovery is a promising future direction. In-depth exploration at the cellular and molecular levels within organs is beneficial for elucidating the specific mechanisms of damage in cryopreservation, thereby providing new research perspectives. Optimizing the vitrification solution and machine perfusion protocols can reduce toxic damage during cryopreservation, while searching new efficient and low-toxic CPAs remains an important means of solving this problem. Furthermore, interdisciplinary new technologies are an important direction for future development, which may bring unexpected breakthroughs in improving the quality of tissue and organ preservation.
Author contributions
JC has made substantial contributions to the conception and design of this work. XL, YH, and XC have took part in revising work critically for important intellectual content. ST has revised work and approved the final version to be published. All authors contributed significantly to the writing of the manuscript.
Conflict of interest
The authors declare that the research was conducted in the absence of any commercial or financial relationships that could be construed as a potential conflict of interest.
Publisher's note
All claims expressed in this article are solely those of the authors and do not necessarily represent those of their affiliated organizations, or those of the publisher, the editors and the reviewers. Any product that may be evaluated in this article, or claim that may be made by its manufacturer, is not guaranteed or endorsed by the publisher.
Supplementary material
The Supplementary Material for this article can be found online at: https://www.frontiersin.org/articles/10.3389/fvets.2023.1201794/full#supplementary-material
References
1. Jones B, Bes M. Keeping kidneys. Bull World Health Organ. (2012) 90:718–9. doi: 10.2471/BLT.12.021012
2. Giwa S, Lewis JK, Alvarez L, Langer R, Roth AE, Church GM, et al. The promise of organ and tissue preservation to transform medicine. Nat Biotechnol. (2017) 35:530–42. doi: 10.1038/nbt.3889
3. Aubert O, Yoo D, Zielinski D, Cozzi E, Cardillo M, Dürr M, et al. COVID-19 pandemic and worldwide organ transplantation: a population-based study. Lancet Public Health. (2021) 6:e709–19. doi: 10.1016/S2468-2667(21)00200-0
4. Lewis JK, Bischof JC, Braslavsky I, Brockbank KG, Fahy GM, Fuller BJ, et al. The grand challenges of organ banking: proceedings from the first global summit on complex tissue cryopreservation. Cryobiology. (2016) 72:169–82. doi: 10.1016/j.cryobiol.2015.12.001
5. Tingle SJ, Figueiredo RS, Moir JAG, Goodfellow M, Talbot D, Wilson CH. Machine perfusion preservation versus static cold storage for deceased donor kidney transplantation. Cochrane Database Syst Rev. (2019) 3:CD011671. doi: 10.1002/14651858.CD011671.pub2
6. Karakoyun R, Romano A, Nordström J, Ericzon B-G, Nowak G. Type of preservation solution, UW or HTK, has an impact on the incidence of biliary stricture following liver transplantation: a retrospective study. J Transplant. (2019) 2019:8150736. doi: 10.1155/2019/8150736
7. Henry SD, Guarrera JV. Protective effects of hypothermic ex vivo perfusion on ischemia/reperfusion injury and transplant outcomes. Transplant Rev. (2012) 26:163–75. doi: 10.1016/j.trre.2011.09.001
8. Friend PJ. Strategies in organ preservation-a new golden age. Transplantation. (2020) 104:1753–5. doi: 10.1097/TP.0000000000003397
9. Amin A, Panayotova G, Guarrera JV. Hypothermic machine perfusion for liver graft preservation. Curr Opin Organ Transplant. (2022) 27:98–105. doi: 10.1097/MOT.0000000000000973
10. Jing L, Yao L, Zhao M, Peng LP, Liu M. Organ preservation: from the past to the future. Acta Pharmacol Sin. (2018) 39:845–57. doi: 10.1038/aps.2017.182
11. Ferng AS, Schipper D, Connell AM, Marsh KM, Knapp S, Khalpey Z. Novel vs clinical organ preservation solutions: improved cardiac mitochondrial protection. J Cardiothorac Surg. (2017) 12:7. doi: 10.1186/s13019-017-0564-x
12. de Vries RJ, Tessier SN, Banik PD, Nagpal S, Cronin SEJ, Ozer S, et al. Subzero non-frozen preservation of human livers in the supercooled state. Nat Protoc. (2020) 15:2024–40. doi: 10.1038/s41596-020-0319-3
13. de Vries RJ, Tessier SN, Banik PD, Nagpal S, Cronin SEJ, Ozer S, et al. Supercooling extends preservation time of human livers. Nat Biotechnol. (2019) 37:1131–6. doi: 10.1038/s41587-019-0223-y
14. Maffei S, Brevini TAL, Gandolfi F. Freezing and freeze-drying: the future perspective of organ and cell preservation. In:Brevini TAL, , editor. Stem Cells in Animal Species: From Pre-clinic to Biodiversity. Cham: Springer International Publishing (2014), p. 167–84. doi: 10.1007/978-3-319-03572-7_9
15. He B, Su S, Yuan G, Duan J, Zhu Z, Wang Z. Clinical guideline for vascularized composite tissue cryopreservation. J Tissue Eng Regen Med. (2021) 15:527–33. doi: 10.1002/term.3190
16. Liu X, Hu Y, Zhang W, Yang D, Pan Y, Ekpo MD, et al. Tricine as a novel cryoprotectant with osmotic regulation, ice recrystallization inhibition and antioxidant properties for cryopreservation of red blood cells. Int J Mol Sci. (2022). doi: 10.3390/ijms23158462
17. Mazur P. Cryobiology: the freezing of biological systems. Science. (1970) 168:939–49. doi: 10.1126/science.168.3934.939
18. Chang T, Zhao G. Ice inhibition for cryopreservation: materials, strategies, and challenges. Adv Sci. (2021) 8:2002425. doi: 10.1002/advs.202002425
19. Mazur P, Leibo SP, Chu EH. A two-factor hypothesis of freezing injury. Evidence from Chinese hamster tissue-culture cells. Exp Cell Res. (1972) 71:345–55. doi: 10.1016/0014-4827(72)90303-5
20. Mazur P. Freezing of living cells: mechanisms and implications. Am J Physiol. (1984). 247(3 Pt 1):C125–42. doi: 10.1152/ajpcell.1984.247.3.C125
21. Zhang PQ, Tan PC, Gao YM, Zhang XJ, Xie Y, Zheng DN, et al. The effect of glycerol as a cryoprotective agent in the cryopreservation of adipose tissue. Stem Cell Res Ther. (2022) 13:152. doi: 10.1186/s13287-022-02817-z
22. Egli RJ, Sckell A, Fraitzl CR, Felix R, Ganz R, Hofstetter W, et al. Cryopreservation with dimethyl sulfoxide sustains partially the biological function of osteochondral tissue. Bone. (2003) 33:352–61. doi: 10.1016/S8756-3282(03)00192-3
23. Cheng Y, Yu Y, Zhang Y, Zhao G, Zhao Y. Cold-responsive nanocapsules enable the sole-cryoprotectant-trehalose cryopreservation of β cell-laden hydrogels for diabetes treatment. Small. (2019) 15:e201904290. doi: 10.1002/smll.201904290
24. Liu D, Pan F. Advances in cryopreservation of organs. J Huazhong Univ Sci Technolog Med Sci. (2016) 36:153–61. doi: 10.1007/s11596-016-1559-x
25. Liu Z, Zheng X, Wang J. Bioinspired Ice-binding materials for tissue and organ cryopreservation. J Am Chem Soc. (2022) 144:5685–701. doi: 10.1021/jacs.2c00203
26. Fahy G. The history of organ cryopreservation research. Cryobiology. (2020) 97:268. doi: 10.1016/j.cryobiol.2020.10.077
27. Dolmans MM, Donnez J, Cacciottola L. Fertility preservation: the challenge of freezing and transplanting ovarian tissue. Trends Mol Med. (2021) 27:777–91. doi: 10.1016/j.molmed.2020.11.003
28. Shen L, Guo X, Ouyang X, Huang Y, Gao D, Zhao G. Fine-tuned dehydration by trehalose enables the cryopreservation of RBCs with unusually low concentrations of glycerol. J Mater Chem B. (2021) 9:295–306. doi: 10.1039/D0TB02426K
29. Hovatta O. Methods for cryopreservation of human ovarian tissue. Reprod Biomed Online. (2005) 10:729–34. doi: 10.1016/S1472-6483(10)61116-9
30. Xu Q, Zhu L, Wang G, Sun Y, Wang J, Lin J, et al. Application of cryopreserved autologous skin replantation in the treatment of degloving injury of limbs. J Plast Reconstr Aesthet Surg. (2022) 75:2387–440. doi: 10.1016/j.bjps.2022.04.006
31. Saragusty J. Directional freezing for large volume cryopreservation. Methods Mol Biol. (2015) 1257:381–97. doi: 10.1007/978-1-4939-2193-5_19
32. Arav A, Patrizio P. Techniques of cryopreservation for ovarian tissue and whole ovary. Clin Med Insights Reprod Health. (2019) 13:1179558119884945. doi: 10.1177/1179558119884945
33. Arav A, Natan D. Directional freezing of reproductive cells and organs. Reprod Domest Anim. (2012) 47 Suppl 4:193–6. doi: 10.1111/j.1439-0531.2012.02075.x
34. Arav A, Gavish Z, Elami A, Natan Y, Revel A, Silber S, et al. Ovarian function 6 years after cryopreservation and transplantation of whole sheep ovaries. Reprod Biomed Online. (2010) 20:48–52. doi: 10.1016/j.rbmo.2009.10.019
35. Arav A, Friedman O, Natan Y, Gur E, Shani N. Rat hindlimb cryopreservation and transplantation: a step toward “organ banking”. Am J Transplant. (2017) 17:2820–8. doi: 10.1111/ajt.14320
36. Gavish Z, Ben-Haim M, Arav A. Cryopreservation of whole murine and porcine livers. Rejuvenation Res. (2008) 11:765–72. doi: 10.1089/rej.2008.0706
37. Arav A. Cryopreservation by directional freezing and vitrification focusing on large tissues and organs. Cells. (2022) 11:1072. doi: 10.3390/cells11071072
38. Manuchehrabadi N, Gao Z, Zhang J, Ring HL, Shao Q, Liu F, et al. Improved tissue cryopreservation using inductive heating of magnetic nanoparticles. Sci Transl Med. (2017) 9:eaah4586. doi: 10.1126/scitranslmed.aah4586
39. Fahy GM, MacFarlane DR, Angell CA, Meryman HT. Vitrification as an approach to cryopreservation. Cryobiology. (1984) 21:407–26. doi: 10.1016/0011-2240(84)90079-8
40. Etheridge ML, Xu Y, Rott L, Choi J, Glasmacher B, Bischof JC. RF heating of magnetic nanoparticles improves the thawing of cryopreserved biomaterials. Technology. (2014) 02:229–42. doi: 10.1142/S2339547814500204
41. Han Z, Bishop JC. Perspective: Critical cooling and warming rates as a function of CPA concentration. Cryo Letters. (2020) 41:185–93.
42. Fahy GM, Wowk B. Principles of cryopreservation by vitrification. Methods Mol Biol. (2015) 1257:21–82. doi: 10.1007/978-1-4939-2193-5_2
43. Finger EB, Bischof JC. Cryopreservation by vitrification: a promising approach for transplant organ banking. Curr Opin Organ Transplant. (2018) 23:353–60. doi: 10.1097/MOT.0000000000000534
44. Chiu-Lam A, Staples E, Pepine CJ, Rinaldi C. Perfusion, cryopreservation, and nanowarming of whole hearts using colloidally stable magnetic cryopreservation agent solutions. Sci Adv. (2021) 7:eabe3005. doi: 10.1126/sciadv.abe3005
45. Sharma A, Rao JS, Han Z, Gangwar L, Namsrai B, Gao Z, et al. Vitrification and nanowarming of kidneys. Adv Sci. (2021) 8:e2101691. doi: 10.1002/advs.202101691
46. Sharma A, Lee CY, Namsrai BE, Han Z, Tobolt D, Rao JS, et al. Cryopreservation of whole rat livers by vitrification and nanowarming. Ann Biomed Eng. (2023) 51:566–77. doi: 10.1007/s10439-022-03064-2
47. Martinez-Flores F, Chacon-Gomez M, Madinaveitia-Villanueva JA, Barrera-Lopez A, Aguirre-Cruz L, Querevalu-Murillo W. [The clinical use of cryopreserved human skin allografts for transplantation]. Cir Cir. (2015) 83:485–91. doi: 10.1016/j.circen.2015.11.003
48. Burgos-Blasco B, Vidal-Villegas B, Collado-Vincueria I, Soria-García AM, Cuiña-Sardiña R, Mendez-Fernandez R, et al. Clinical outcomes of long-term corneas preserved frozen in Eusol-C used in emergency tectonic grafts. Cell Tissue Bank. (2022) 1–6. doi: 10.1007/s10561-022-10037-1
49. Vangsness CT Jr, Higgs G, Hoffman JK, Farr J, Davidson PA, Milstein F, et al. Implantation of a novel cryopreserved viable osteochondral allograft for articular cartilage repair in the knee. J Knee Surg. (2018) 31:528–35. doi: 10.1055/s-0037-1604138
50. Campbell BK, Hernandez-Medrano J, Onions V, Pincott-Allen C, Aljaser F, Fisher J, et al. Restoration of ovarian function and natural fertility following the cryopreservation and autotransplantation of whole adult sheep ovaries. Hum Reprod. (2014) 29:1749–63. doi: 10.1093/humrep/deu144
51. Donnez J, Dolmans MM, Demylle D, Jadoul P, Pirard C, Squifflet J, et al. Livebirth after orthotopic transplantation of cryopreserved ovarian tissue. Lancet. (2004) 364:1405–10. doi: 10.1016/S0140-6736(04)17222-X
52. Wang Z, He B, Duan Y, Shen Y, Zhu L, Zhu X, et al. Cryopreservation and replantation of amputated rat hind limbs. Eur J Med Res. (2014) 19:28. doi: 10.1186/2047-783X-19-28
53. Wang ZT, Zhu L, Kou W, Sun WH, He B, Wang CX, et al. Replantation of cryopreserved fingers: an “organ banking” breakthrough. Plast Reconstr Surg. (2019) 144:679–83. doi: 10.1097/PRS.0000000000005979
54. Wang J, Lin J, Pei Y, Xu Q, Zhu L. Cryopreservation and transplantation of amputated finger. Cryobiology. (2020) 92:235–40. doi: 10.1016/j.cryobiol.2020.01.017
55. de Vries RJ, Yarmush M, Uygun K. Systems engineering the organ preservation process for transplantation. Curr Opin Biotechnol. (2019) 58:192–201. doi: 10.1016/j.copbio.2019.05.015
56. Zhan TJ, Liu K, Yang JM, Dang HY, Chen L, Xu Y. Fe3O4 nanoparticles with carboxylic acid functionality for improving the structural integrity of whole vitrified rat kidneys. Acs Applied Nano Materials. (2021) 4:13552–61. doi: 10.1021/acsanm.1c03014
57. Pegg DE. The role of vitrification techniques of cryopreservation in reproductive medicine. Hum Fertil. (2005) 8:231–9. doi: 10.1080/14647270500054803
58. Kilbride P, Lamb S, Milne S, Gibbons S, Erro E, Bundy J, et al. Spatial considerations during cryopreservation of a large volume sample. Cryobiology. (2016) 73:47–54. doi: 10.1016/j.cryobiol.2016.05.013
59. Acker JP, Larese A, Yang H, Petrenko A, McGann LE. Intracellular ice formation is affected by cell interactions. Cryobiology. (1999) 38:363–71. doi: 10.1006/cryo.1999.2179
60. Higgins AZ, Karlsson JO. Effects of intercellular junction protein expression on intracellular ice formation in mouse insulinoma cells. Biophys J. (2013) 105:2006–15. doi: 10.1016/j.bpj.2013.09.028
61. Taylor MJ, Weegman BP, Baicu SC, Giwa SE. New approaches to cryopreservation of cells, tissues, and organs. Transfus Med Hemother. (2019) 46:197–215. doi: 10.1159/000499453
62. Akiyama Y, Shinose M, Watanabe H, Yamada S, Kanda Y. Cryoprotectant-free cryopreservation of mammalian cells by superflash freezing. Proc Natl Acad Sci U S A. (2019) 116:7738–43. doi: 10.1073/pnas.1808645116
63. Giugliarelli A, Urbanelli L, Ricci M, Paolantoni M, Emiliani C, Saccardi R, et al. Evidence of DMSO-induced protein aggregation in cells. J Phys Chem A. (2016) 120:5065–70. doi: 10.1021/acs.jpca.6b00178
64. Ma L, Dong JX, Fu WR, Li XY, Chen J, Liu Y. Mitochondrial morphology and function impaired by dimethyl sulfoxide and dimethyl formamide. J Bioenerg Biomembr. (2018) 50:297–305. doi: 10.1007/s10863-018-9759-7
65. Best BP. Cryoprotectant toxicity: facts, issues, and questions. Rejuvenation Res. (2015) 18:422–36. doi: 10.1089/rej.2014.1656
66. Fahy GM, Wowk B, Pagotan R, Chang A, Phan J, Thomson B, et al. Physical and biological aspects of renal vitrification. Organogenesis. (2009) 5:167–75. doi: 10.4161/org.5.3.9974
67. Karlsson JO, Toner M. Long-term storage of tissues by cryopreservation: critical issues. Biomaterials. (1996) 17:243–56. doi: 10.1016/0142-9612(96)85562-1
68. Acker J. 20. The complexity of tissue and organ cryopreservation: a call for interdisciplinary research. Cryobiology. (2015) 71:170. doi: 10.1016/j.cryobiol.2015.05.026
69. Warner RM, Shuttleworth R, Benson JD, Eroglu A, Higgins AZ. General tissue mass transfer model for cryopreservation applications. Biophys J. (2021) 120:4980–91. doi: 10.1016/j.bpj.2021.10.014
70. Balasubramanian SK, Coger RN. Heat and mass transfer during the cryopreservation of a bioartificial liver device: a computational model. ASAIO J. (2005) 51:184–93. doi: 10.1097/01.MAT.0000161079.35897.7D
71. Eisenberg DP, Steif PS, Rabin Y. On the effects of thermal history on the development and relaxation of thermo-mechanical stress in cryopreservation. Cryogenics. (2014) 64:86–94. doi: 10.1016/j.cryogenics.2014.09.005
72. Gallardo M, Paulini F, Corral A, Balcerzyk M, Lucci CM, Ambroise J, et al. Evaluation of a new freezing protocol containing 20% dimethyl sulphoxide concentration to cryopreserve human ovarian tissue. Reprod Biomed Online. (2018) 37:653–65. doi: 10.1016/j.rbmo.2018.09.012
73. Brinkkoetter PT, Song H, Losel R, Schnetzke U, Gottmann U, Feng Y, et al. Hypothermic injury: the mitochondrial calcium, ATP and ROS love-hate triangle out of balance. Cell Physiol Biochem. (2008) 22:195–204. doi: 10.1159/000149797
74. Catalán J, Yánez-Ortiz I, Tvarijonaviciute A, González-Aróstegui LG, Rubio CP, Barranco I, et al. Seminal plasma antioxidants are related to sperm cryotolerance in the horse. Antioxidants. (2022) 11:1279. doi: 10.3390/antiox11071279
75. Len JS, Koh WSD, Tan S-X. The roles of reactive oxygen species and antioxidants in cryopreservation. Biosci Rep. (2019) 39:BSR20191601. doi: 10.1042/BSR20191601
76. Bisht S, Faiq M, Tolahunase M, Dada R. Oxidative stress and male infertility. Nat Rev Urol. (2017) 14:470–85. doi: 10.1038/nrurol.2017.69
77. Fan P, Xie XH, Chen CH, Peng X, Zhang P, Yang C, et al. Molecular regulation mechanisms and interactions between reactive oxygen species and mitophagy. DNA Cell Biol. (2019) 38:10–22. doi: 10.1089/dna.2018.4348
78. Heng BC, Clement MV, Cao T. Caspase inhibitor Z-VAD-FMK enhances the freeze-thaw survival rate of human embryonic stem cells. Biosci Rep. (2007) 27:257–64. doi: 10.1007/s10540-007-9051-2
79. Banihani S, Agarwal A, Sharma R, Bayachou M. Cryoprotective effect of L-carnitine on motility, vitality and DNA oxidation of human spermatozoa. Andrologia. (2014) 46:637–41. doi: 10.1111/and.12130
80. Valko M, Leibfritz D, Moncol J, Cronin MT, Mazur M, Telser J. Free radicals and antioxidants in normal physiological functions and human disease. Int J Biochem Cell Biol. (2007) 39:44–84. doi: 10.1016/j.biocel.2006.07.001
81. Akhtar MZ, Henderson T, Sutherland A, Vogel T, Friend PJ. Novel approaches to preventing ischemia-reperfusion injury during liver transplantation. Transplant Proc. (2013) 45:2083–92. doi: 10.1016/j.transproceed.2013.04.004
82. He Z, Liu K, Wang J. Bioinspired materials for controlling ice nucleation, growth, and recrystallization. Acc Chem Res. (2018) 51:1082–91. doi: 10.1021/acs.accounts.7b00528
83. Matsumura K, Hyon SH. Polyampholytes as low toxic efficient cryoprotective agents with antifreeze protein properties. Biomaterials. (2009) 30:4842–9. doi: 10.1016/j.biomaterials.2009.05.025
84. Deller RC, Vatish M, Mitchell DA, Gibson MI. Synthetic polymers enable non-vitreous cellular cryopreservation by reducing ice crystal growth during thawing. Nat Commun. (2014) 5:3244. doi: 10.1038/ncomms4244
85. Bai G, Gao D, Liu Z, Zhou X, Wang J. Probing the critical nucleus size for ice formation with graphene oxide nanosheets. Nature. (2019) 576:437–41. doi: 10.1038/s41586-019-1827-6
86. Zhu W, Guo J, Agola JO, Croissant JG, Wang Z, Shang J, et al. Metal-organic framework nanoparticle-assisted cryopreservation of red blood cells. J Am Chem Soc. (2019) 141:7789–96. doi: 10.1021/jacs.9b00992
87. Lee C, Lee Y, Jung WH, Kim T-Y, Kim T, Kim D-N, et al. Peptide-DNA origami as a cryoprotectant for cell preservation. Sci Adv. (2022) 8:eadd0185. doi: 10.1126/sciadv.add0185
88. Fahy GM, Wowk B, Wu J, Paynter S. Improved vitrification solutions based on the predictability of vitrification solution toxicity. Cryobiology. (2004) 48:22–35. doi: 10.1016/j.cryobiol.2003.11.004
89. Fahy GM. Cryoprotectant toxicity neutralization. Cryobiology. (2010) 60(3 Suppl):S45–53. doi: 10.1016/j.cryobiol.2009.05.005
90. Kilbride P, McIntyre R, Manuchehrabadi N, Sandlin RD. 56. Poly-vitrification – a new approach to organ preservation. Cryobiology. (2015) 71:179. doi: 10.1016/j.cryobiol.2015.05.062
91. Jesus AR, Duarte ARC, Paiva A. Use of natural deep eutectic systems as new cryoprotectant agents in the vitrification of mammalian cells. Sci Rep. (2022) 12:8095. doi: 10.21203/rs.3.rs-1338804/v1
92. Brockbank KG, Chen Z, Greene ED, Campbell LH. Vitrification of heart valve tissues. Methods Mol Biol. (2015) 1257:399–421. doi: 10.1007/978-1-4939-2193-5_20
93. Clark S, Jomha NM, Elliott JAW. Modeling the simultaneous transport of multiple cryoprotectants into articular cartilage using a triphasic model. J Phys Chem B. (2022) 126:9566–79. doi: 10.1021/acs.jpcb.2c05736
94. Taylor MJ, Song YC, Kheirabadi BS, Lightfoot FG, Brockbank KGM. Vitrification fulfills its promise as an approach to reducing freeze-induced injury in a multicellular tissue. In: ASME 1999 International Mechanical Engineering Congress and Exposition. Nashville, TN: American Society of Mechanical Engineers (1999). p. 93–102. doi: 10.1115/IMECE1999-0588
95. Zhang Y, Wang H, Stewart S, Jiang B, Ou W, Zhao G, et al. Cold-responsive nanoparticle enables intracellular delivery and rapid release of trehalose for organic-solvent-free cryopreservation. Nano Lett. (2019) 19:9051–61. doi: 10.1021/acs.nanolett.9b04109
96. Huang H, Choi JK, Rao W, Zhao S, Agarwal P, Zhao G, et al. Alginate hydrogel microencapsulation inhibits devitrification and enables large-volume low-CPA cell vitrification. Adv Funct Mater. (2015) 25:6839–50. doi: 10.1002/adfm.201503047
97. Jiang B, Li W, Stewart S, Ou W, Liu B, Comizzoli P, et al. Sand-mediated ice seeding enables serum-free low-cryoprotectant cryopreservation of human induced pluripotent stem cells. Bioact Mater. (2021) 6:4377–88. doi: 10.1016/j.bioactmat.2021.04.025
98. Hou Y, Lu C, Dou M, Zhang C, Chang H, Liu J, et al. Soft liquid metal nanoparticles achieve reduced crystal nucleation and ultrarapid rewarming for human bone marrow stromal cell and blood vessel cryopreservation. Acta Biomater. (2020) 102:403–15. doi: 10.1016/j.actbio.2019.11.023
99. Yagi T, Hardin JA, Valenzuela YM, Miyoshi H, Gores GJ, Nyberg SL. Caspase inhibition reduces apoptotic death of cryopreserved porcine hepatocytes. Hepatology. (2001) 33:1432–40. doi: 10.1053/jhep.2001.24560
100. Deng SL, Sun TC, Yu K, Wang ZP, Zhang BL, Zhang Y, et al. Melatonin reduces oxidative damage and upregulates heat shock protein 90 expression in cryopreserved human semen. Free Radic Biol Med. (2017) 113:347–54. doi: 10.1016/j.freeradbiomed.2017.10.342
101. Murray KA, Gibson MI. Chemical approaches to cryopreservation. Nat Rev Chem. (2022) 6:579–93. doi: 10.1038/s41570-022-00407-4
102. Dou M, Li Y, Sun Z, Li L, Rao W. L-proline feeding for augmented freeze tolerance of Camponotus japonicus Mayr. Sci Bull. (2019) 64:1795–804. doi: 10.1016/j.scib.2019.09.028
103. Gao Z, Namsrai B, Han Z, Joshi P, Rao JS, Ravikumar V, et al. Vitrification and rewarming of magnetic nanoparticle-loaded rat hearts. Adv Mater Technol. (2022) 7:2100873. doi: 10.1002/admt.202100873
104. Offerijns FG, Krijnen HW. The preservation of the rat heart in the frozen state. Cryobiology. (1972) 9:289–95. doi: 10.1016/0011-2240(72)90050-8
105. Wang X, Chen H, Yin H, Kim SS, Lin Tan S, Gosden RG. Fertility after intact ovary transplantation. Nature. (2002) 415:385. doi: 10.1038/415385a
106. Song YC, Khirabadi BS, Lightfoot F, Brockbank KG, Taylor MJ. Vitreous cryopreservation maintains the function of vascular grafts. Nat Biotechnol. (2000) 18:296–9. doi: 10.1038/73737
107. Hughes SM, Ferre AL, Yandura SE, Shetler C, Baker CAR, Calienes F, et al. Cryopreservation of human mucosal tissues. PLoS ONE. (2018) 13:e0200653. doi: 10.1371/journal.pone.0200653
108. Zhan L, Rao JS, Sethia N, Slama MQ, Han Z, Tobolt D, et al. Pancreatic islet cryopreservation by vitrification achieves high viability, function, recovery and clinical scalability for transplantation. Nat Med. (2022) 28:798–808. doi: 10.1038/s41591-022-01718-1
109. Canatelli-Mallat M, Lascaray F, Entraigues-Abramson M, Portiansky EL, Blamaceda N, Morel GR, et al. Cryopreservation of a human brain and its experimental correlate in rats. Rejuvenation Res. (2020) 23:516–25. doi: 10.1089/rej.2019.2245
Keywords: cryopreservation, tissue and organ, cryoprotectants, vitrification, organ transplantation
Citation: Chen J, Liu X, Hu Y, Chen X and Tan S (2023) Cryopreservation of tissues and organs: present, bottlenecks, and future. Front. Vet. Sci. 10:1201794. doi: 10.3389/fvets.2023.1201794
Received: 07 April 2023; Accepted: 09 May 2023;
Published: 25 May 2023.
Edited by:
Cristina Soriano-Úbeda, Universidad de León, SpainReviewed by:
Thirumala Rao Talluri, ICAR- National Research Centre, IndiaCopyright © 2023 Chen, Liu, Hu, Chen and Tan. This is an open-access article distributed under the terms of the Creative Commons Attribution License (CC BY). The use, distribution or reproduction in other forums is permitted, provided the original author(s) and the copyright owner(s) are credited and that the original publication in this journal is cited, in accordance with accepted academic practice. No use, distribution or reproduction is permitted which does not comply with these terms.
*Correspondence: Songwen Tan, c29uZ3dlbi50YW4mI3gwMDA0MDtjc3UuZWR1LmNu