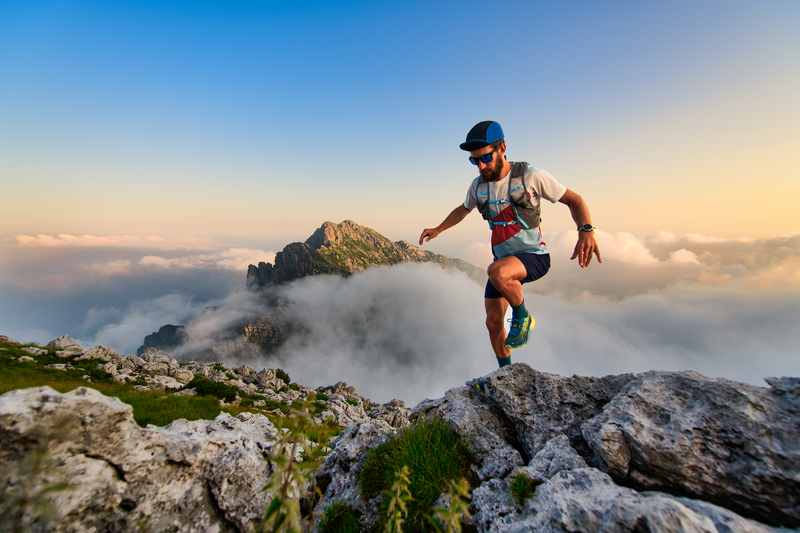
95% of researchers rate our articles as excellent or good
Learn more about the work of our research integrity team to safeguard the quality of each article we publish.
Find out more
ORIGINAL RESEARCH article
Front. Vet. Sci. , 23 March 2023
Sec. Veterinary Pharmacology and Toxicology
Volume 10 - 2023 | https://doi.org/10.3389/fvets.2023.1148766
This article is part of the Research Topic Inflammation and Immunomodulation in Veterinary Medicine View all 11 articles
Oxidative stress is due to an unbalance between pro-oxidants, such as reactive oxygen (ROS) and nitrogen (RNS) species, and antioxidants/antioxidant system. Under physiological conditions these species are involved in different cellular processes such as cellular homeostasis and immune response, while an excessive production of ROS/RNS has been linked to the development of various diseases such as cancer, diabetes, and Alzheimer's disease. In this context, the naturally occurring dipeptide carnosine has shown the ability to scavenge ROS, counteract lipid peroxidation, and inhibit proteins oxidation. Titanium dioxide nanoparticles (TiO2-NPs) have been widely used to produce cosmetics, in wastewater treatment, in food industry, and in healthcare product. As consequence, these NPs are often released into aquatic environments. The Danio rerio (commonly called zebrafish) embryos exposure to TiO2-NPs did not affect the hatching rate, but induced oxidative stress. According to this scenario, in the present study, we first investigated the effects of carnosine exposure and of a sub-toxic administration of TiO2-NPs on the development and survival of zebrafish embryos/larvae measured through the acute embryo toxicity test (FET-Test). Zebrafish larvae represent a useful model to study oxidative stress-linked disorders and to test antioxidant molecules, while carnosine was selected based on its well-known multimodal mechanism of action that includes a strong antioxidant activity. Once the basal effects of carnosine were assessed, we then evaluated its effects on TiO2-NPs-induced oxidative stress in zebrafish larvae, measured in terms of total ROS production (measured with 2,7-dichlorodihydrofluorescein diacetate probe) and protein expression by immunohistochemistry of two cellular stress markers, 70 kDa-heat shock protein (Hsp70) and metallothioneins (MTs). We demonstrated that carnosine did not alter the phenotypes of both embryos and larvae of zebrafish at different hours post fertilization. Carnosine was instead able to significantly decrease the enhancement of ROS levels in zebrafish larvae exposed to TiO2-NPs and its antioxidant effect was paralleled by the rescue of the protein expression levels of Hsp70 and MTs. Our results suggest a therapeutic potential of carnosine as a new pharmacological tool in the context of pathologies characterized by oxidative stress such as neurodegenerative disorders.
Carnosine is a naturally occurring peptide composed of β-alanine and L-histidine and synthesized through an ATP-dependent reaction by carnosine synthetase 1 (CARNS1) enzyme. This peptide can be found at very high concentrations in mammalian tissues, particularly in the brain, where it reaches concentrations ranging between 0.7 and 2.0 mM (up to 5 mM in some areas), as well as in cardiac and skeletal muscles (up to 20 mM), and also in the tissues of some species of invertebrates (1, 2). Different studies have also shown the presence of carnosine in numerous other classes of Vertebrates including fish (3, 4), while plants do not contain it at all (5).
Many beneficial activities have been attributed to carnosine, such as pH-buffering activity (6), heavy metal chelating activity (7), antioxidant, anti-glycating, and ion-chelating capacity (8), along with the ability to scavenge free-radicals (9). When the synthesis of reactive and pro-oxidant species overtakes the antioxidant defense system, oxidative stress occurs (10, 11). In this context, carnosine has shown the ability to scavenge reactive oxygen species (ROS) and alpha–beta-unsaturated aldehydes formed from peroxidation of cell membrane fatty acids during oxidative stress (12), also inhibiting the oxidative modifications of proteins exposed to hydroxyl radicals (13, 14).
The over-production of ROS and the related oxidative stress have been shown to disrupt cellular proteins (15), requiring their subsequent refolding; in this context, the activity exerted by heat shock proteins (HSPs) represents the most effectively protective mechanism (16). HSPs are a group of molecular chaperones able to revert or inhibit denaturation or unfolding of cellular proteins in response to stress and/or high temperature. Recent studies have been focused on the regulatory role of HSPs in several redox processes as well as on their protection of antioxidant mediators (17). In particular, the 70 kDa-HSP (Hsp70) family promotes the proteolytic removal of oxidatively damaged proteins by the proteasome (18) and closely interact with the nitric oxide generation systems (19). Hsp70 is represents a marker of oxidative response in different experimental models, including Danio rerio (commonly called zebrafish) (20–22).
Metallothioneins (MTs) are a group of low molecular weight metal-binding proteins with high affinity for divalent metal ions. MTs have been widely considered for their protective role that is mediated by their ability to exert metal detoxification (23) and counteract oxidative stress-induced damage (24).
Among xenobiotic, nanoparticles (NPs) could have a significant role in the development of oxidative stress phenomena owing to their small surface-area-to-volume ratio, physical size, and relatively high biopersistence. In particular, metal oxide NPs lead to ROS generation directly or indirectly, with an extent that depends on dose, metal speciation, and exposure route (25). The NPs-induced ROS formation, and in general ROS over-production, has been linked to lipid and protein peroxidation as well as to DNA fragmentation and reduced antioxidant ability (26, 27). Titanium dioxide NPs (TiO2−NPs) are widely used to produce cosmetics, wastewater treatment, food, and healthcare products. It has been demonstrated that exposure to TiO2-NPs does not affect the hatching rate of zebrafish embryos, and does not cause malformation on the larvae (28), even if it can lead to oxidative stress in embryos (29). Thus, considering the oxidative capacity of TiO2-NPs and the multimodal mechanism of action of carnosine (30), including the well-known antioxidant activity also demonstrated in zebrafish embryos (31), we investigated whether carnosine could inhibit the oxidative stress induced by TiO2-NPs in this model.
Zebrafish is a teleost fish widely used in translational research (32), allowing to investigate the molecular mechanisms related to numerous diseases including neurodegenerative and immune diseases (33–36); in particular, this animal model is considered of utmost importance in the case of (eco)toxicological studies. Zebrafish possesses several advantages over rodent models in the study of vertebrate development and disease, including high fecundity rate (37, 38). Zebrafish eggs are transparent, allowing the observation of morphogenetic changes and organogenesis in real time (39). In particular, embryos have been shown to represent a valuable tool to study both pro-oxidant (40) and pro-inflammatory (41) phenomena, as well as to investigate the therapeutic potential of nutraceuticals (42) and natural products (43). Additionally, embryos have rapid development with embryogenesis being complete by 72 hours post fertilization (hpf) and most organs fully developed by 96 hpf.
Zebrafish model represents the perfect bridge in preclinical toxicology between in vitro assays and mammalian in vivo studies (44). It has also been established as a valuable animal model to study oxidative stress phenomena in different diseases, including diabetic retinopathy and nephropathy (45, 46). In this context, carnosine treatment has shown to be able to rescue pancreas disruption (31) and microvascular alterations (46). With regard to zebrafish development, carnosine has been linked to olfactory and visual function (47), while the effects of carnosine exposure during zebrafish embryonic development have not yet been completely elucidated.
In the present study, we first investigated the effects of carnosine exposure and of a sub-toxic administration of TiO2-NPs on the development and survival of zebrafish embryos/larvae. Once the safety of carnosine was assessed, we investigated its effects on the TiO2-NPs-induced oxidative stress in zebrafish, measured in terms of total ROS production and protein expression of two well-known markers of cellular stress, Hsp70 and MTs.
All chemicals were of analytical grade and purchased from Sigma-Aldrich (St. Louis, MO, USA) or Thermo Fisher Scientific Inc. (Pittsburgh, PA, USA) unless specified otherwise.
A stock solution of carnosine at the concentration of 1 M was prepared by dissolving the powder in osmosis water, which is optimal for housing zebrafish (48). Working solutions of 0.1, 1, 10, and 20 mM were prepared by diluting the stock solution in osmosis water. TiO2-NPs, kindly supplied by the CNR-IMM of Catania, characterized by a crystalline phase mix of anatase (86%) and rutile (14%), and with an average size of about 50 nm, were diluted to 0.1 mg/mL (working solution) in osmosis water. In order to avoid the formation of NP aggregates, it was necessary to carry out 4 cycles of 10 min (with 3 min of break) of sonication by using a probe sonicator (Sonopuls, De Marco S.r.l., Milan, Italy), with a frequency of 40 kHz (49).
Fertilized eggs from the same spawning event and collected within 4 hpf were provided by the Fish Pathology and Experimental Center of Sicily (CISS) of the Department of Veterinary Science (University of Messina). Only eggs at the blastula stage were used, while the infertile eggs or eggs with alterations on the chorion were discarded. According to OECD Test Guidelines for Chemicals (50) we used the 24-well plates to distribute the eggs (1 embryo/well, 2 mL solution/well).
The first set of experiments was carried out to investigate the effects of increasing concentrations of carnosine (0.1, 1, 10, and 20 mM) as well as of TiO2-NPs (0.1 mg/mL) on zebrafish embryonic development. We set up 24-well plates for each concentration of carnosine selected and TiO2-NPs (0.1 mg/mL). We performed three replicates for each multi-well plates prepared.
In particular, in all 24-well plates set up, 20 embryos were exposed to test concentration and 4 embryos were exposed to dilution water as internal plate control. A total number of 60 embryos were used for each experimental condition. As recommended by the protocol procedure, 24-well plates of positive controls and negative controls have been made; for the negative controls, embryos were exposed to water dilution, while in positive controls, embryos were exposed to 3,4-dichloroaniline (DCA) at the concentration of 0.004 mg/mL in water (51).
During the experiments, the temperature within the wells was maintained constant (26 ± 1°C) and the solution in each well was replaced every 24 h (semi-static renewal) (50).
Since neither carnosine nor TiO2-NPs induced toxic effects, we selected a starting concentration of 20 mM carnosine which represents the highest concentration of carnosine found at the tissue level. The 24-well plates of 20 mM carnosine solutions were prepared as a pre-treatment for embryos (1 h of pre-treatment), subsequently TiO2-NPs solution was added until the end of the test. Three replicates were performed, therefore a total number of 60 embryos was used.
A binocular microscope (E200 MV-R LED, Nikon Instruments S.p.A., Florence, Italy) equipped with a camera (CMOS, Nikon Instruments S.p.A.) was used to observe and photograph the embryos every 24 h (until the end of the test: 96 hpf).
The acute toxicological endpoints (coagulated embryos, lack of somite formation, non-detachment of the tail, and lack of heartbeat) were assessed and quantified as “observed” or “not observed”. A DanioScope™ software (Noldus Information Technology bv, Wageningen, Netherlands) was used to evaluate heartbeat, body length of larvae, and possible malformations.
According to OECD, the acute embryo toxicity test (FET-Test; Fish Embryo Toxicity-Test) was considered valid only if overall survival of embryos was ≥90% in the negative control (dilution-water) and ≤ 70% (minimum mortality of 30%) in the positive control (0.004 mg/mL of DCA for zebrafish) until the end of the 96 h exposure. After hatching, the larvae of each experimental group were used to evaluate intracellular ROS and protein expression of Hsp70 and MTs through immunohistochemical analysis.
2,7-dichlorodihydrofluorescein diacetate (H2DCFDA) was used to detect intracellular ROS content. At the end of the exposure, all larvae, including controls, were stained with a ROS detection solution. The ROS detection solution (5 μM) was prepared in Hanks' balanced salt solution (HBSS). Larvae were washed twice with HBSS for 2 min, then incubated with the ROS detection solution for a total of 15 min at 28°C. At the end of the incubation, the ROS detection solution was discarded, the larvae were washed with HBSS (twice for 2 min), and placed on a glass slide. As a last step, larvae were examined by using a fluorescence microscope (NIKON ECLIPSE Ci, Nikon Instruments S.p.A.), equipped with a NIKON DS-Qi2 camera (Nikon Instruments S.p.A.). Image J software was used to measure fluorescence signals (52).
Immunohistochemical analysis was performed to evaluate the expression of Hsp70 or MTs in the whole larvae as previously described by Pecoraro et al. (51). Briefly, the larvae collected from each experimental group were washed in phosphate buffered saline (PBS) and fixed with 4% (w/v) paraformaldehyde. Next, the fixative was discarded and larvae were washed twice in PBS. The permeabilization of larvae, to improve antibody penetration, was obtained by using a solution of PBS-Triton X-100 (for 15 min). A blocking solution containing bovine serum albumin was used to block non-specific antibody binding. Larvae were placed on a glass slide and incubated overnight at 4°C with primary antibodies: anti-HSP70 polyclonal antibody (GeneTex, 1:1,000; Cat. No. GTX112963) or anti-MTs (GeneTex, 1:1,000; Cat. No. GTX12228). Larvae were then washed twice with PBS-Tween 20 to remove the excess of primary antibodies and incubated for 1 h at 4°C in the dark with TRITC-conjugated anti-rabbit (1:1,000) or FITC-conjugated anti-mouse (1:1,000) secondary antibodies. Following a washing step in PBS-Tween 20, the larvae were dehydrated employing increasing alcoholic solutions (70°, 80°, and 95°) and air dried. The larvae were mounted with 4′,6-diamidino-2-phenylindole (DAPI) (Abcam, Cambridge, UK) and sealed with rubber cement to be examined with a fluorescence microscope (NIKON ECLIPSE Ci) equipped with a NIKON DS-Qi2 camera. TRITC-conjugated anti-rabbit secondary antibody exhibited a red fluorescence, while FITC-conjugated anti-mouse secondary antibody exhibited a green fluorescence. The images obtained by fluorescence microscope have been processed with Image J software (52).
Statistical analysis was performed by using Graphpad Prism software, version 8.0 (Graphpad software, San Diego, CA, USA). Data are always reported as the mean ± standard deviation (SD). One-way analysis of variance (ANOVA), followed by Tukey's post hoc test, was used for multiple comparisons. The assumptions for performing the parametric tests were confirmed by applying the Brown–Forsythe test. Only p-values of < 0.05 were considered statistically significant.
The first set of experiments was carried out to investigate the effects of increasing concentrations of carnosine (0.1, 1, 10, and 20 mM) on zebrafish embryonic development. As shown in Figure 1, none of the carnosine concentrations tested altered the development of zebrafish larvae compared to CTRL (untreated zebrafish larvae). We also performed a DanioScope™ analysis showing no alterations in the heartbeat of the embryos/larvae (Figure 2) as well as in the body length of the larvae (Figure 3). Beats per minute (BPM) values ranged from 161.7 to 167.9, with the highest values observed in embryos exposed to 20 mM carnosine. All the measured BPM values are to be considered physiological; in fact, in zebrafish the physiological heart rate is about 120–180 BPM (53, 54). With regard to the body length of the larvae, the measured values ranged from 3,433 to 3,653 μm. Once again, the highest values were observed in the case of embryos exposed to 20 mM carnosine. As in the case of BPM measurements, the body length of the larvae are to be considered physiological (39). As previously mentioned, it has been demonstrated that exposure to TiO2-NPs does not affect the hatching rate of embryos and does not cause malformation on the larvae, even if it can lead to oxidative stress in embryos. In order to confirm the absence of acute toxicity, the effect of TiO2-NPs treatment (0.1 mg/mL) on the phenotypes of both embryos and larvae at different hpf was also evaluated. As expected, the embryos completed their embryonic development, thus no toxicological endpoints have been recorded (Figure 4). The hatching rate was not altered, neither statistically significant differences in the survival rate were observed in the experimental groups compared to the control group. Hatching occurred 72 hpf in all experimental groups, and the larvae exhibited a good shape of body.
Figure 1. Effects of increasing concentrations of carnosine (0.1, 1, 10, and 20 mM) on the phenotypes of both embryos and larvae at different (4, 24, 48, and 72) hpf. Car, carnosine.
Figure 2. Beats per minute (BPM) of unexposed larvae and larvae exposed to increasing concentrations of carnosine (0.1, 1, 10, and 20 mM). SD is represented by vertical bars. Car, carnosine; n.s., not significant.
Figure 3. Body length (μm) of unexposed larvae and larvae exposed to increasing concentrations of carnosine (0.1, 1, 10, and 20 mM). SD is represented by vertical bars. Car, carnosine; n.s., not significant.
Figure 4. Effects of TiO2-NPs on the phenotypes of both embryos and larvae at different (4, 24, 48, and 72) hpf.
With regard to the evaluation of ROS production under our experimental conditions, it was found a significant increase of these reactive species in TiO2-NPs-treated larvae compared to CTRL (p < 0.001) (Figure 5). The pre-treatment with carnosine at the concentration of 20 mM was able to completely prevent the production of ROS compared to TiO2-NPs-treated larvae (p < 0.001), giving fluorescence values comparable to that observed in untreated larvae. Despite a trend of decrease observed in TiO2-NPs-treated larvae in the presence of carnosine compared to untreated larvae, the difference was not statistically significant.
Figure 5. Total ROS production in (A) untreated larvae, (B) TiO2-NPs-treated larvae, and (C) TiO2-NPs-treated larvae in the presence of carnosine 20 mM (1 h pre-treatment). Carnosine was kept during the exposure to TiO2-NPs. The average fluorescence intensity (AU) of at least 5 values for fixed larva is reported in (D). SD is represented by vertical bars. ***Significantly different, p < 0.001. n.s., not significant.
Immunohistochemical analysis suggests the ability of TiO2-NPs to induce Hsp70 protein expression in the yolk sac and at the beginning of the tail (p < 0.001 vs. CTRL and p < 0.001 vs. TiO2-NPs + Carnosine) (Figure 6). Of note, despite the ability of carnosine to significantly decrease the inductive effect of TiO2-NPs, a value still higher compared to untreated larvae was measured. A similar trend was observed when analyzing MTs expression levels. As depicted in Figure 7, the exposure of larvae to TiO2-NPs led to a significant (p < 0.01) increase in MTs expression levels compared to untreated larvae. Again, the pre-treatment of larvae with carnosine led to MTs expression levels significantly lower (p < 0.05) if compared larvae exposed to TiO2-NPs only. It is worth mentioning that carnosine treatment led to fluorescence vales comparable to that measured in untreated larvae, representing the negative controls.
Figure 6. Hsp70 expression levels in (A) untreated larvae, (B) TiO2-NPs-treated larvae, and (C) TiO2-NPs-treated larvae in the presence of carnosine 20 mM (1 h pre-treatment). Carnosine was kept during the exposure to TiO2-NPs. The average fluorescence intensity (AU) of at least 5 values for fixed larva is reported in (D). SD is represented by vertical bars. ***Significantly different, p < 0.001.
Figure 7. MTs expression levels in (A) untreated larvae, (B) TiO2-NPs-treated larvae, and (C) TiO2-NPs-treated larvae in the presence of carnosine 20 mM (1 h pre-treatment). Carnosine was kept during the exposure to TiO2-NPs. The average fluorescence intensity (AU) of at least 4 values for fixed larva is reported in (D). SD is represented by vertical bars. *Significantly different, p < 0.05; **Significantly different, p < 0.01; n.s., not significant.
Oxidative stress is due to an unbalance occurring between the production of ROS and reactive nitrogen species (RNS) and the diminished cellular antioxidant defenses (55, 56). It is well-known that these reactive species, under basal/physiological conditions, are involved in different cellular processes such as cellular homeostasis and metabolism, signaling and redox state, being also extremely important in the immune response and pathogen clearance (57). On the other hand, the excess of ROS/RNS production can lead to the damage of fundamental macromolecules including DNA and proteins and has been linked to the development of various diseases such as cancer, cardiovascular disease, diabetes, PD, and AD (58–61).
ROS can have an adverse impact on the embryo and fetal development (62) and also play a key role in the pathophysiology of different adverse effects, from cardiotoxicity to neurotoxicity (63). Therefore, current studies in molecular toxicology and nanotoxicology are directed to better identify the preclinical toxicity of new drugs and/or NPs in order to prevent toxicity in humans. Recent studies with zebrafish focused on new drug discovery toxicology suggest that this tiny vertebrate represents the ideal tool to better understand disease biology and drug toxicity (44, 64). This becomes particularly relevant when considering the field of NPs and their relevance for human toxicology (65).
Currently, the use of NPs in industry, biology, and medicine is attracting a lot of attention. Numerous studies have been aimed at evaluating their biosafety, toxicity, and/or possible side effects both in vitro and in vivo (66, 67). Among thousands of different NPs, metal oxide NPs, and in particular TiO2-NPs, have shown different applications, including the production of solar cells, food wraps, pharmaceuticals, lacquers, medical devices, gas sensing, photocatalyst, and cosmetic, just to name a few (68). Despite this enormous potential, in vitro cells' studies have shown how these NPs could induce oxidative stress (69), inhibit cell cycle (70), lead to inflammatory responses (71) and dysregulated autophagy (72). In vivo studies have highlighted how TiO2-NPs-induced oxidative stress contributes to organ dysfunction (73). Additionally, as a consequence of their intensive applications, TiO2-NPs are often released into aquatic environments (74), increasing the exposure of humans and ecosystems to NPs (75).
According to this scenario, in the present study we first investigated the effects of carnosine or TiO2-NPs exposure on the development and survival of zebrafish embryos/larvae. Zebrafish, as discussed above, represents an excellent tool in drug discovery toxicology to study oxidative stress-linked disorders (45, 46, 76) and to test new drugs endowed with an antioxidant activity (77, 78), while the selection of carnosine is related to its well-known multimodal mechanism of action in neurodegenerative disorders (30), including a strong direct and indirect antioxidant activity (79). With specific regards to zebrafish development, carnosine-like peptides have been linked to olfactory and visual functions (47), while the effects of carnosine exposure during zebrafish embryonic development have not been fully elucidated.
In our study, carnosine did not alter, at none of the concentrations considered, the development of zebrafish larvae (Figure 1). The absence of toxic effects was expected since both preclinical (80) and clinical (81, 82) studies have clearly demonstrated that this dipeptide is essentially non-toxic and well-tolerated, without known drug interactions and severe adverse effects (83, 84). It is also worth mentioning that despite the absence of significant deleterious effects of chronic exposure to carnosine (0.01 μM to 10 mM) on embryonic development, treatment with 100 mM carnosine can result in developmental delay and compromised larval survival (47).
According to previous studies carried out on zebrafish showing that TiO2-NPs did not cause any toxicity to zebrafish embryos and larvae (85), and confirmed by our results (Figure 4), the exposure to TiO2-NPs did not affect the hatching rate of zebrafish embryos and did not cause malformation on the larvae, despite the well-recognized ability of these NPs to cause oxidative stress phenomena (28).
Starting from this evidence, the subsequent experiments described in the present study were purposely designed in order to determine whether a non-toxic amount of TiO2-NPs is capable to increase oxidative stress, measured in terms of total ROS production and protein expression of two well-known markers of cellular stress (represented by Hsp70 and MTs) in zebrafish larvae, as well as the therapeutic potential of carnosine in counteracting the pro-oxidant effects of NPs.
When evaluating the production of ROS under our experimental conditions, it was found a massive increase of these reactive species in larvae exposed to TiO2-NPs (Figure 5). Notably, the presence of carnosine completely abolished the pro-oxidant effects of NPs, giving ROS levels superimposable to that measured in untreated larvae. These results are in agreement with a multitude of in vitro and in vivo studies showing carnosine antioxidant activity and its preclinical potential as an antidote. In particular, the observed results could be related to the ability of carnosine to directly interact with and decrease different reactive species such as superoxide anion and hydroxyl radicals (86, 87), nitric oxide (88), cytotoxic carbonyl species (89), and aldehydes (1). Carnosine has also been able to act indirectly through the enhancement of the endogenous antioxidant machinery (90–93). As reported by Chan et al., the dietary supplementation with carnosine was able to decrease the formation of thiobarbituric acid reactive substances in rats (94). The ability of carnosine to counteract oxidative stress has also been demonstrated in astrocytes, oligodendrocytes, and neuronal cultures (95–98). It is worth pointing out that recent studies have shown carnosine ability to decrease NPs-induced ROS formation in lung and microglial cells (99), while this dipeptide was able to rescue 4-hydroxynonenal (HNE)-induced retinal phenotype in aldh3a1 zebrafish larvae mutants (31).
In the present study, we also demonstrated for the first time that the ability of carnosine to counteract oxidative stress was, at least in part, related to its modulatory activity on Hsp70 and MTs, two well-known biomarkers in translational medicine of oxidative stress response (100, 101). The enhanced Hsp70 and MTs protein expression levels were detected in zebrafish larvae following the treatment with TiO2-NPs (Figures 6, 7), in accordance with previous studies showing the increased expression of these markers after the exposure to metals (102–104), or other pro-oxidant/pro-inflammatory stimuli such as ionizing radiation (105), intracellular β-amyloid (106), lipopolysaccharides (107), and interferons (108). Notably, Hsp70 and MTs protein expression levels were down-regulated by the administration of carnosine to zebrafish larvae. This negative regulation of Hsp70 and MTs could be attributable to an indirect activity of carnosine able to decrease ROS and then oxidative stress, an event strictly connected to the over-expression of these proteins (17, 109–111) and that can significantly contribute to the preclinical efficacy and the therapeutic potential of carnosine as an antidote in human toxicology.
In the present study we demonstrated that carnosine, when used at physiological concentrations ranging from 0.1 to 20 mM, does not alter the phenotypes of both embryos and larvae of zebrafish at different hpf in terms of coagulated embryos, lack of somite formation, non-detachment of the tail, and lack of heartbeat. When tested in a well-known oxidative stress model represented by zebrafish larvae challenged with TiO2-NPs, carnosine significantly decreased ROS levels and this antioxidant activity was paralleled by its ability to rescue the protein expression levels of two well-recognized oxidative stress response markers represented by Hsp70 and MTs. Our results suggest a therapeutic potential of carnosine as a new pharmacological tool in the context of pathologies characterized by oxidative stress such as PD and AD.
The original contributions presented in the study are included in the article/Supplementary material, further inquiries can be directed to the corresponding author.
Ethical review and approval were not required for the animal study because no one authorization is required for experiments with larvae, before yolk sac resorption.
Conceptualization/design of the study and supervision: GC and MB. Methodology: ES, RP, and ET. Software analysis: GC, ES, RP, VC, and AP. Validation, resources, and funding acquisition: GC, FCar, and MB. Formal analysis and investigation: GC, ES, RP, VC, AP, ET, and FCap. Data curation: GC, RJ, and AS. Writing—original draft preparation: GC, ES, VC, and AP. Writing, review, and editing: GC, ES, RP, VC, AP, ET, FCap, RJ, AS, FCar, and MB. Visualization: GC, RJ, AS, FCar, and MB. Project administration: GC. All authors have read and agreed to the submitted version of the manuscript.
GC is a researcher at the University of Catania within the EU-funded PON REACT project (Azione IV.4—Dottorati e contratti di ricerca su tematiche dell'innovazione, nuovo Asse IV del PON Ricerca e Innovazione 2014–2020 Istruzione e ricerca per il recupero—REACT—EU; Progetto Identificazione e validazione di nuovi target farmacologici nella malattia di Alzheimer attraverso l'utilizzo della microfluidica, CUP E65F21002640005).
AP would like to thank the International PhD Program in Neuroscience at University of Catania (Italy).
The authors declare that the research was conducted in the absence of any commercial or financial relationships that could be construed as a potential conflict of interest.
All claims expressed in this article are solely those of the authors and do not necessarily represent those of their affiliated organizations, or those of the publisher, the editors and the reviewers. Any product that may be evaluated in this article, or claim that may be made by its manufacturer, is not guaranteed or endorsed by the publisher.
The Supplementary Material for this article can be found online at: https://www.frontiersin.org/articles/10.3389/fvets.2023.1148766/full#supplementary-material
Supplementary Video 1. CTRL 48 hpf.
Supplementary Video 2. CTRL 72 hpf.
Supplementary Video 3. Car 0.1 mM 48 hpf.
Supplementary Video 4. Car 0.1 mM 72 hpf.
Supplementary Video 5. Car 1 mM 48 hpf.
Supplementary Video 6. Car 1 mM 72 hpf.
Supplementary Video 7. Car 10 mM 48 hpf.
Supplementary Video 8. Car 10 mM 72 hpf.
Supplementary Video 9. Car 20 mM 48 hpf.
Supplementary Video 10. Car 20 mM 72 hpf.
1. Hipkiss AR, Preston JE, Himsworth DT, Worthington VC, Keown M, Michaelis J, et al. Pluripotent protective effects of carnosine, a naturally occurring dipeptide. Ann N Y Acad Sci. (1998) 854:37–53. doi: 10.1111/j.1749-6632.1998.tb09890.x
2. Drozak JM, Veiga-da-Cunha D, Vertommen V, Stroobant D, Van Schaftingen E. Molecular identification of carnosine synthase as ATP-grasp domain-containing protein 1 (ATPGD1). J Biol Chem. (2010) 285:9346–56. doi: 10.1074/jbc.M109.095505
3. Crush KG. Carnosine and related substances in animal tissues. Comp Biochem Physiol. (1970) 34:3–30. doi: 10.1016/0010-406X(70)90049-6
4. Margolis FL, Grillo M. Carnosine, homocarnosine and anserine in vertebrate retinas. Neurochem Int. (1984) 6:207–9. doi: 10.1016/0197-0186(84)90094-9
5. Boldyrev AA, Aldini G, Derave W. Physiology and pathophysiology of carnosine. Physiol Rev. (2013) 93:1803–45. doi: 10.1152/physrev.00039.2012
6. Vistoli G, Straniero V, Pedretti A, Fumagalli L, Bolchi C, Pallavicini M, et al. Predicting the physicochemical profile of diastereoisomeric histidine-containing dipeptides by property space analysis. Chirality. (2012) 24:566–76. doi: 10.1002/chir.22056
7. Torreggiani A, Tamba M, Fini G. Binding of copper(II) to carnosine: Raman and IR spectroscopic study. Biopolymers. (2000) 57:149–59. doi: 10.1002/(SICI)1097-0282(2000)57:3<149::AID-BIP3>3.0.CO;2-G
8. Ghodsi R, Kheirouri S. Carnosine and advanced glycation end products: a systematic review. Amino Acids. (2018) 50:1177–86. doi: 10.1007/s00726-018-2592-9
9. Bellia F, Vecchio G, Rizzarelli E. Carnosine and cognitive deficits. Diet Nutr Dement Cogn Decline. (2014) 159, 973–82. doi: 10.1016/B978-0-12-407824-6.00090-2
10. Gunasekara DB, Siegel JM, Caruso G, Hulvey MK, Lunte SM. Microchip electrophoresis with amperometric detection method for profiling cellular nitrosative stress markers. Analyst. (2014) 139:3265–73. doi: 10.1039/C4AN00185K
11. De Campos RPS, Lunte SM. Indirect detection of superoxide in RAW 264.7 macrophage cells using microchip electrophoresis coupled to laser-induced fluorescence. Anal Bioanal Chem. (2015) 407:7003–7012. doi: 10.1007/s00216-015-8865-1
12. Szabadfi K, Pinter E, Reglodi D, Gabriel R. Neuropeptides, trophic factors, and other substances providing morphofunctional and metabolic protection in experimental models of diabetic retinopathy. Int Rev Cell Mol Biol. (2014) 311:1–121. doi: 10.1016/B978-0-12-800179-0.00001-5
13. Kim MY, Kim EJ, Kim YN, Choi C, Lee BH. Effects of alpha-lipoic acid and L-carnosine supplementation on antioxidant activities and lipid profiles in rats. Nutr Res Pract. (2011) 5:421–8. doi: 10.4162/nrp.2011.5.5.421
14. Caruso G, Spampinato SF, Cardaci V, Caraci F, Sortino MA, Merlo S, et al. β-amyloid and oxidative stress: perspectives in drug development. Curr Pharm Des. (2019) 25:4771–81. doi: 10.2174/1381612825666191209115431
15. Lazzarino G, Listorti I, Bilotta G, Capozzolo T, Amorini AM, Longo S, et al. Water- and fat-soluble antioxidants in human seminal plasma and serum of fertile males. Antioxidants. (2019) 8:96. doi: 10.3390/antiox8040096
16. Ikwegbue PC, Masamba P, Oyinloye BE, Kappo AP. Roles of heat shock proteins in apoptosis, oxidative stress, human inflammatory diseases, and cancer. Pharmaceuticals. (2017) 11:2. doi: 10.3390/ph11010002
17. Szyller J, Bil-Lula I. Heat shock proteins in oxidative stress and ischemia/reperfusion injury and benefits from physical exercises: a review to the current knowledge. Oxid Med Cell Longev. (2021) 2021:6678457. doi: 10.1155/2021/6678457
18. Reeg S, Jung T, Castro JP, Davies KJA, Henze A, Grune T. The molecular chaperone Hsp70 promotes the proteolytic removal of oxidatively damaged proteins by the proteasome. Free Radic Biol Med. (2016) 99:153–66. doi: 10.1016/j.freeradbiomed.2016.08.002
19. Molina MN, Ferder L, Manucha W. Emerging role of nitric oxide and heat shock proteins in insulin resistance. Curr Hypertens Rep. (2016) 18:1. doi: 10.1007/s11906-015-0615-4
20. Moraes ACN, Shah S, Magalhaes VF, Habibi HR. Cylindrospermopsin impairs zebrafish (Danio rerio) embryo development. Mar Environ Res. (2022) 175:105567. doi: 10.1016/j.marenvres.2022.105567
21. Xu H, Miao XM, Wang WB, Wang G, Li Y. Transcriptome analysis reveals the early resistance of zebrafish larvae to oxidative stress. Fish Physiol Biochem. (2022) 48:1075–89. doi: 10.1007/s10695-022-01100-5
22. Martin-Folgar R, Torres-Ruiz M, de Alba M, Cañas-Portilla AI, González MC, Morales M. Molecular effects of polystyrene nanoplastics toxicity in zebrafish embryos (Daniorerio). Chemosphere. (2023) 312:137077. doi: 10.1016/j.chemosphere.2022.137077
23. Chen L, Ma L, Bai Q, Zhu X, Zhang J, Wei Q, et al. Heavy metal-induced metallothionein expression is regulated by specific protein phosphatase 2A complexes. J Biol Chem. (2014) 289:22413–26. doi: 10.1074/jbc.M114.548677
24. Juarez-Rebollar D, Rios C, Nava-Ruiz C, Mendez-Armenta M. Metallothionein in brain disorders. Oxid Med Cell Longev. (2017) 2017:5828056. doi: 10.1155/2017/5828056
25. Kessler A, Hedberg J, Blomberg E, Odnevall I. Reactive oxygen species formed by metal and metal oxide nanoparticles in physiological mediaandmdash;a review of reactions of importance to nanotoxicity and proposal for categorization. Nanomaterials. (2022) 12:1922. doi: 10.3390/nano12111922
26. Nosaka Y, Nosaka AY. Generation and detection of reactive oxygen species in photocatalysis. Chem Rev. (2017) 117:11302–36. doi: 10.1021/acs.chemrev.7b00161
27. Wang Y, Cai R, Chen C. The nano-bio interactions of nanomedicines: understanding the biochemical driving forces and redox reactions. Acc Chem Res. (2019) 52:1507–18. doi: 10.1021/acs.accounts.9b00126
28. Tang T, Zhang Z, Zhu X. Toxic effects of TiO2 NPs on zebrafish. Int J Environ Res Public Health. (2019) 16:523. doi: 10.3390/ijerph16040523
29. Chen J, Lei L, Mo W, Dong H, Li J, Bai C, et al. Developmental titanium dioxide nanoparticle exposure induces oxidative stress and neurobehavioral changes in zebrafish. Aquat Toxicol. (2021) 240:105990. doi: 10.1016/j.aquatox.2021.105990
30. Caruso G, Caraci F, Jolivet RB. Pivotal role of carnosine in the modulation of brain cells activity: Multimodal mechanism of action and therapeutic potential in neurodegenerative disorders. Prog Neurobiol. (2019) 175:35–53. doi: 10.1016/j.pneurobio.2018.12.004
31. Lou B, Boger M, Bennewitz K, Sticht C, Kopf S, Morgenstern J, et al. Elevated 4-hydroxynonenal induces hyperglycaemia via Aldh3a1 loss in zebrafish and associates with diabetes progression in humans. Redox Biol. (2020) 37:101723. doi: 10.1016/j.redox.2020.101723
32. Bailone RL, Fukushima HCS, Ventura Fernandes BH, Aguiar LK, De Correa T, Janke H, et al. Zebrafish as an alternative animal model in human and animal vaccination research. Lab Anim Res. (2020) 36:13. doi: 10.1186/s42826-020-00042-4
33. Wilkinson RN, van Eeden FJ. The zebrafish as a model of vascular development and disease. Prog Mol Biol Transl Sci. (2014) 124:93–122. doi: 10.1016/B978-0-12-386930-2.00005-7
34. Gore AV, Pillay LM, Venero Galanternik M, Weinstein BM. The zebrafish: A fintastic model for hematopoietic development and disease. Wiley Interdiscip Rev Dev Biol. (2018) 7:e312. doi: 10.1002/wdev.312
35. Calvo-Ochoa E, Byrd-Jacobs CA. The olfactory system of zebrafish as a model for the study of neurotoxicity and injury: implications for neuroplasticity and disease. Int J Mol Sci. (2019) 20:1639. doi: 10.3390/ijms20071639
36. Garcia-Moreno D, Tyrkalska SD, Valera-Perez A, Gomez-Abenza E, Perez-Oliva AB, Mulero V. The zebrafish: a research model to understand the evolution of vertebrate immunity. Fish Shellfish Immunol. (2019) 90:215–22. doi: 10.1016/j.fsi.2019.04.067
37. Westerfield M. The Zebrafish Book: A Guide for the Laboratory use of Zebrafish (danio rerio). Princeton: Princeton University (1995).
38. Ribas L, Piferrer F. The zebrafish (Danio rerio) as a model organism, with emphasis on applications for finfish aquaculture research. Rev Aquacult. (2014) 6:209–40. doi: 10.1111/raq.12041
39. Kimmel CB, Ballard WW, Kimmel SR, Ullmann B, Schilling TF. Stages of embryonic development of the zebrafish. Dev Dyn. (1995) 203:253–310. doi: 10.1002/aja.1002030302
40. Mugoni V, Camporeale A, Santoro MM. Analysis of oxidative stress in zebrafish embryos. J Vis Exp. (2014) 89:51328. doi: 10.3791/51328
41. Di Paola D. Assessment of 2-Pentadecyl-2-oxazoline role on lipopolysaccharide-induced inflammation on early stage development of zebrafish (Danio rerio). Life. (2022) 12:128. doi: 10.3390/life12010128
42. Licitra R, Marchese M, Brogi L, Fronte B, Pitto L, Santorelli FM. Nutraceutical screening in a zebrafish model of muscular dystrophy: gingerol as a possible food aid. Nutrients. (2021) 13:998. doi: 10.3390/nu13030998
43. Crawford AD, Liekens S, Kamuhabwa AR, Maes J, Munck S, Busson R, et al. Zebrafish bioassay-guided natural product discovery: isolation of angiogenesis inhibitors from East African medicinal plants. PLoS ONE. (2011) 6:e14694. doi: 10.1371/journal.pone.0014694
44. Cassar S, Adatto I, Freeman JL, Gamse JT, Iturria I, Lawrence C, et al. Use of Zebrafish in Drug Discovery Toxicology. Chem Res Toxicol. (2020) 33:95–118. doi: 10.1021/acs.chemrestox.9b00335
45. Schmohl F, Peters V, Schmitt CP, Poschet G, Buttner M, Li X, et al. CNDP1 knockout in zebrafish alters the amino acid metabolism, restrains weight gain, but does not protect from diabetic complications. Cell Mol Life Sci. (2019) 76:4551–68. doi: 10.1007/s00018-019-03127-z
46. Qi H, Schmohl F, Li X, Qian X, Tabler CT, Bennewitz K, et al. Reduced acrolein detoxification in akr1a1a zebrafish mutants causes impaired insulin receptor signaling and microvascular alterations. Adv Sci. (2021) 8:e2101281. doi: 10.1002/advs.202101281
47. Senut MC, Azher S, Margolis FL, Patel K, Mousa A, Majid A. Distribution of carnosine-like peptides in the nervous system of developing and adult zebrafish (Danio rerio) and embryonic effects of chronic carnosine exposure. Cell Tissue Res. (2009) 337:45–61. doi: 10.1007/s00441-009-0796-8
48. Nüsslein-Volhard C, Dahm R, Dahm R, Nüsslein-Volhard C. Zebrafish: a practical approach. Oxford, Oxford University Press. (2002).
49. Pecoraro R, Marino F, Salvaggio A, Capparucci F, Caro GDi, Iaria C, et al. Evaluation of chronic nanosilver toxicity to Adult Zebrafish. Front Physiol. (2017) 8:1011. doi: 10.3389/fphys.2017.01011
50. OECD (2013). Test No. 236: Fish Embryo Acute Toxicity (FET) Test, OECD Guidelines for the Testing of Chemicals, Paris: OECD Publishing. doi: 10.1787/9789264203709-en
51. Pecoraro R, Salvaggio A, Marino F, Caro GD, Capparucci F, Lombardo BM, et al. Metallic nano-composite toxicity evaluation by zebrafish embryo toxicity test with identification of specific exposure biomarkers. Curr Protoc Toxicol. (2017) 74:14 11–11 14 13. doi: 10.1002/cptx.34
53. Gu J, Wang H, Zhou L, Fan D, Shi L, Ji G, et al. Oxidative stress in bisphenol AF-induced cardiotoxicity in zebrafish and the protective role of N-acetyl N-cysteine. Sci Total Environ. (2020) 731:139190. doi: 10.1016/j.scitotenv.2020.139190
54. Wei Y, Meng Y, Huang Y, Liu Z, Zhong K, Ma J, et al. Development toxicity and cardiotoxicity in zebrafish from exposure to iprodione. Chemosphere. (2021) 263:127860. doi: 10.1016/j.chemosphere.2020.127860
55. Lazzarino G, Listorti I, Muzii L, Amorini AM, Longo S, Stasio EDi, et al. Low-molecular weight compounds in human seminal plasma as potential biomarkers of male infertility. Hum Reprod. (2018) 33:1817–28. doi: 10.1093/humrep/dey279
56. Mahmoud AM, Wilkinson FL, Sandhu MA, Lightfoot AP. The interplay of oxidative stress and inflammation: mechanistic insights and therapeutic potential of antioxidants, Hindawi. Oxidative Med. Cellular Longevity. (2021) 2021:1–4. doi: 10.1155/2021/9851914
57. Li H, Zhou X, Huang Y, Liao B, Cheng L, Ren B. Corrigendum: reactive oxygen species in pathogen clearance: the killing mechanisms, the adaption response, and the side effects. Front Microbiol. (2021) 12:685133. doi: 10.3389/fmicb.2021.685133
58. Chen X, Guo C, Kong J. Oxidative stress in neurodegenerative diseases. Neural Regen Res. (2012) 7:376–85. doi: 10.3969/j.issn.1673-5374.2012.05.009
59. Alhakamy NA, Badr-Eldin SM, Fahmy U, Alruwaili NK, Awan ZA, Caruso G, et al. Thymoquinone-loaded soy-phospholipid-based phytosomes exhibit anticancer potential against human lung cancer cells. Pharmaceutics. (2020) 12:761. doi: 10.3390/pharmaceutics12080761
60. Caruso G, Fresta CG, Grasso M, Santangelo R, Lazzarino G, Lunte SM, et al. (2020). “Inflammation as the Common Biological Link Between Depression and Cardiovascular Diseases: Can Carnosine Exert a Protective Role?” Curr Med Chem. 27:1782–800. doi: 10.2174/0929867326666190712091515
61. Caruso G, Torrisi SA, Mogavero MP, Currenti W, Castellano S, Godos J, et al. Polyphenols and neuroprotection: therapeutic implications for cognitive decline. Pharmacol Ther. (2022) 232:108013. doi: 10.1016/j.pharmthera.2021.108013
62. Swain N, Moharana AK, Jena SR, Samanta L. Impact of oxidative stress on embryogenesis and fetal development. Adv Exp Med Biol. (2022) 1391:221–41. doi: 10.1007/978-3-031-12966-7_13
63. Caruso G, Privitera A, Antunes BM, Lazzarino G, Lunte SM, Aldini G, et al. The Therapeutic potential of carnosine as an antidote against drug-induced cardiotoxicity and neurotoxicity: focus on Nrf2 pathway. Molecules. (2022) 27. doi: 10.3390/molecules27144452
64. Das BC, McCormick L, Thapa P, Karki R, Evans T. Use of zebrafish in chemical biology and drug discovery. Future Med Chem. (2013) 5:2103–16. doi: 10.4155/fmc.13.170
65. Lin Z, Aryal S, Cheng YH, Gesquiere AJ. Integration of in vitro and in vivo models to predict cellular and tissue dosimetry of nanomaterials using physiologically based pharmacokinetic modeling. ACS Nano. (2022) 16:19722–54. doi: 10.1021/acsnano.2c07312
66. Kornberg TG, Stueckle TA, Antonini JM, Rojanasakul Y, Castranova V, Yang Y, et al. Potential toxicity and underlying mechanisms associated with pulmonary exposure to iron oxide nanoparticles: conflicting literature and unclear risk. Nanomaterials. (2017) 7:307. doi: 10.3390/nano7100307
67. Attarilar S, Yang J, Ebrahimi M, Wang Q, Liu J, Tang Y, et al. The toxicity phenomenon and the related occurrence in metal and metal oxide nanoparticles: a brief review from the biomedical perspective. Front Bioeng Biotechnol. (2020) 8:822. doi: 10.3389/fbioe.2020.00822
68. Waghmode MS, Gunjal AB, Mulla JA, Patil NN, Nawani NN. Studies on the titanium dioxide nanoparticles: Biosynthesis, applications and remediation. SN Appl. Sci. (2019) 1:1–9. doi: 10.1007/s42452-019-0337-3
69. Hu H, Guo Q, Wang C, Ma X, He H, Oh Y, et al. Titanium dioxide nanoparticles increase plasma glucose via reactive oxygen species-induced insulin resistance in mice. J Appl Toxicol. (2015) 35:1122–32. doi: 10.1002/jat.3150
70. Kansara K, Patel P, Shah D, Shukla RK, Singh S, Kumar A, et al. TiO2 nanoparticles induce DNA double strand breaks and cell cycle arrest in human alveolar cells. Environ Mol Mutagen. (2015) 56:204–17. doi: 10.1002/em.21925
71. Giovanni M, Yue J, Zhang L, Xie J, Ong CN, Leong DT. Pro-inflammatory responses of RAW264.7 macrophages when treated with ultralow concentrations of silver, titanium dioxide, and zinc oxide nanoparticles. J Hazard Mater. (2015) 297:146–152. doi: 10.1016/j.jhazmat.2015.04.081
72. Lopes VR, Loitto V, Audinot JN, Bayat N, Gutleb AC, Cristobal S. Dose-dependent autophagic effect of titanium dioxide nanoparticles in human HaCaT cells at non-cytotoxic levels. J Nanobiotechnol. (2016) 14:22. doi: 10.1186/s12951-016-0174-0
73. Song B, Zhou T, Yang W, Liu J, Shao L. Contribution of oxidative stress to TiO nanoparticle-induced toxicity. Environ Toxicol Pharmacol. (2016) 48:130–40. doi: 10.1016/j.etap.2016.10.013
74. Daughton CG, Ternes TA. Pharmaceuticals and personal care products in the environment: agents of subtle change? Environ Health Perspect. (1999) 107:907–938. doi: 10.1289/ehp.99107s6907
75. Nowack B, Bucheli TD. Occurrence, behavior and effects of nanoparticles in the environment. Environ Pollut. (2007) 150:5–22. doi: 10.1016/j.envpol.2007.06.006
76. Abbate F, Maugeri A, Laurà R, Levanti M, Navarra M, Cirmi S, et al. Zebrafish as a useful model to study oxidative stress-linked disorders: focus on flavonoids. Antioxidants. (2021) 10:668. doi: 10.3390/antiox10050668
77. Manjunathan T, Guru A, Arokiaraj J, Gopinath P. 6-gingerol and semisynthetic 6-gingerdione counteract oxidative stress induced by ROS in zebrafish. Chem Biodivers. (2021) 18:e2100650. doi: 10.1002/cbdv.202100650
78. Di Paola D. Intestinal disorder in zebrafish larvae (Danio rerio): The protective action of N-palmitoylethanolamide-oxazoline. Life. (2022) 12:125. doi: 10.3390/life12010125
79. Solana-Manrique C, Sanz FJ, Martínez-Carrión G, Paricio N. Antioxidant and neuroprotective effects of carnosine: therapeutic implications in neurodegenerative diseases. Antioxidants. (2022) 11:848. doi: 10.3390/antiox11050848
80. Boldyrev AA. Carnosine and Oxidative Stress in Cells and Tissues. Hauppauge: Nova Publishers (2007).
81. Gardner ML, Illingworth KM, Kelleher J, Wood D. Intestinal absorption of the intact peptide carnosine in man, and comparison with intestinal permeability to lactulose. J Physiol. (1991) 439:411–22. doi: 10.1113/jphysiol.1991.sp018673
82. Goto K, Maemura H, Takamatsu K, Ishii N. Hormonal responses to resistance exercise after ingestion of carnosine and anserine. J Strength Condition Res. (2011) 25:398–405. doi: 10.1519/JSC.0b013e3181bac43c
83. Caruso G, Godos J, Castellano S, Micek A, Murabito P, Galvano F, et al. The therapeutic potential of carnosine/anserine supplementation against cognitive decline: a systematic review with meta-analysis. Biomedicines. (2021) 9:253. doi: 10.3390/biomedicines9030253
84. Caruso G. Unveiling the hidden therapeutic potential of carnosine, a molecule with a multimodal mechanism of action: a position paper. Molecules. (2022) 27:3303. doi: 10.3390/molecules27103303
85. Zhu X, Zhu L, Duan Z, Qi R, Li Y, Lang Y. Comparative toxicity of several metal oxide nanoparticle aqueous suspensions to Zebrafish (Danio rerio) early developmental stage. J Environ Sci Health A Tox Hazard Subst Environ Eng. (2008) 43:278–84. doi: 10.1080/10934520701792779
86. Gorbunov NV, Erin AN. Mechanism of antioxidant action of carnosine. Biull Eksp Biol Med. (1991) 111:477–8. doi: 10.1007/BF00840997
87. Caruso G, Fresta CG, Siegel JM, Wijesinghe MB, Lunte SM. Microchip electrophoresis with laser-induced fluorescence detection for the determination of the ratio of nitric oxide to superoxide production in macrophages during inflammation. Anal Bioanal Chem. (2017) 409:4529–38. doi: 10.1007/s00216-017-0401-z
88. Caruso G, Fresta CG, Martinez-Becerra F, Antonio L, Johnson RT, de Campos RP, et al. Carnosine modulates nitric oxide in stimulated murine RAW 264.7 macrophages. Mol Cell Biochem. (2017) 431:197–210. doi: 10.1007/s11010-017-2991-3
89. Aldini G, Facino RM, Beretta G, Carini M. Carnosine and related dipeptides as quenchers of reactive carbonyl species: from structural studies to therapeutic perspectives. Biofactors. (2005) 24:77–87. doi: 10.1002/biof.5520240109
90. Choi SY, Kwon HY, Kwon OB, Kang JH. Hydrogen peroxide-mediated Cu,Zn-superoxide dismutase fragmentation: protection by carnosine, homocarnosine and anserine. Biochim Biophys Acta. (1999) 1472:651–7. doi: 10.1016/S0304-4165(99)00189-0
91. Ukeda H, Hasegawa Y, Harada Y, Sawamura M. Effect of carnosine and related compounds on the inactivation of human Cu,Zn-superoxide dismutase by modification of fructose and glycolaldehyde. Biosci Biotechnol Biochem. (2002) 66:36–43. doi: 10.1271/bbb.66.36
92. Fresta CG, Hogard ML, Caruso G, Costa EE, Lazzarino G, Lunte SM, et al. Monitoring carnosine uptake by RAW 264.7 macrophage cells using microchip electrophoresis with fluorescence detection. Anal Methods. (2017) 9:402–08. doi: 10.1039/C6AY03009B
93. Fresta CG, Fidilio A, Lazzarino G, Musso N, Grasso M, Merlo S, et al. Modulation of pro-oxidant and pro-inflammatory activities of m1 macrophages by the natural dipeptide carnosine. Int J Mol Sci. (2020) 21:776. doi: 10.3390/ijms21030776
94. Chan WK, Decker EA, Chow CK, Boissonneault GA. Effect of dietary carnosine on plasma and tissue antioxidant concentrations and on lipid oxidation in rat skeletal muscle. Lipids. (1994) 29:461–6. doi: 10.1007/BF02578242
95. Horning MS, Blakemore LJ, Trombley PQ. Endogenous mechanisms of neuroprotection: role of zinc, copper, and carnosine. Brain Res. (2000) 852:56–61. doi: 10.1016/S0006-8993(99)02215-5
96. Boldyrev A, Bulygina E, Leinsoo T, Petrushanko I, Tsubone S, Abe H. Protection of neuronal cells against reactive oxygen species by carnosine and related compounds. Comp Biochem Physiol B Biochem Mol Biol. (2004) 137:81–8. doi: 10.1016/j.cbpc.2003.10.008
97. Calabrese V, Colombrita C, Guagliano E, Sapienza M, Ravagna A, Cardile V, et al. Protective effect of carnosine during nitrosative stress in astroglial cell cultures. Neurochem Res. (2005) 30:797–807. doi: 10.1007/s11064-005-6874-8
98. Spina-Purrello V, Giliberto S, Barresi V, Nicoletti VG, Giuffrida Stella AM, Rizzarelli E. Modulation of PARP-1 and PARP-2 expression by L-carnosine and trehalose after LPS and INFgamma-induced oxidative stress. Neurochem Res. (2010) 35:2144–53. doi: 10.1007/s11064-010-0297-x
99. Fresta CG, Chakraborty A, Wijesinghe MB, Amorini AM, Lazzarino G, Lazzarino G, et al. Non-toxic engineered carbon nanodiamond concentrations induce oxidative/nitrosative stress, imbalance of energy metabolism, and mitochondrial dysfunction in microglial and alveolar basal epithelial cells. Cell Death Dis. (2018) 9:245. doi: 10.1038/s41419-018-0280-z
100. El Golli-Bennour E, Bacha H. Hsp70 expression as biomarkers of oxidative stress: mycotoxins' exploration. Toxicology. (2011) 287:1–7. doi: 10.1016/j.tox.2011.06.002
101. Figueira E, Branco D, Antunes SC, Gonçalves F, Freitas R. Are metallothioneins equally good biomarkers of metal and oxidative stress?. Ecotoxicol Environ Saf. (2012) 84:185–90. doi: 10.1016/j.ecoenv.2012.07.012
102. Luther EM, Schmidt MM, Diendorf J, Epple M, Dringen R. Upregulation of metallothioneins after exposure of cultured primary astrocytes to silver nanoparticles. Neurochem Res. (2012) 37:1639–48. doi: 10.1007/s11064-012-0767-4
103. Mazzei V, Giannetto A, Brundo MV, Maisano M, Ferrante M, Copat C, et al. Metallothioneins and heat shock proteins 70 in Armadillidium vulgare (Isopoda, Oniscidea) exposed to cadmium and lead. Ecotoxicol Environ Saf. (2015) 116:99–106. doi: 10.1016/j.ecoenv.2015.03.007
104. Xin L, Wang J, Wu Y, Guo S, Tong J. Increased oxidative stress and activated heat shock proteins in human cell lines by silver nanoparticles. Hum Exp Toxicol. (2015) 34:315–23. doi: 10.1177/0960327114538988
105. Calini V, Urani C, Camatini M. Overexpression of HSP70 is induced by ionizing radiation in C3H 10T1/2 cells and protects from DNA damage. Toxicol In Vitro. (2003) 17:561–6. doi: 10.1016/S0887-2333(03)00116-4
106. Magrané J, Smith RC, Walsh K, Querfurth HW. Heat shock protein 70 participates in the neuroprotective response to intracellularly expressed beta-amyloid in neurons. J Neurosci. (2004) 24:1700–6. doi: 10.1523/JNEUROSCI.4330-03.2004
107. Dai H, Wang L, Li L, Huang Z, Ye L. Metallothionein 1: a new spotlight on inflammatory diseases. Front Immunol. (2021) 12:739918. doi: 10.3389/fimmu.2021.739918
108. Nagamine T, Suzuki K, Kondo T, Nakazato K, Kakizaki S, Takagi H, et al. Interferon-alpha-induced changes in metallothionein expression in liver biopsies from patients with chronic hepatitis C. Can J Gastroenterol. (2005) 19:481–6. doi: 10.1155/2005/262597
109. Chiaverini N, De Ley M. Protective effect of metallothionein on oxidative stress-induced DNA damage. Free Radic Res. (2010) 44:605–13. doi: 10.3109/10715761003692511
110. Stetler RA, Gan Y, Zhang W, Liou AK, Gao Y, Cao G, et al. Heat shock proteins: cellular and molecular mechanisms in the central nervous system. Prog Neurobiol. (2010) 92:184–211. doi: 10.1016/j.pneurobio.2010.05.002
Keywords: carnosine, oxidative stress, reactive oxygen species, heat shock proteins, metallothioneins, zebrafish
Citation: Caruso G, Scalisi EM, Pecoraro R, Cardaci V, Privitera A, Truglio E, Capparucci F, Jarosova R, Salvaggio A, Caraci F and Brundo MV (2023) Effects of carnosine on the embryonic development and TiO2 nanoparticles-induced oxidative stress on Zebrafish. Front. Vet. Sci. 10:1148766. doi: 10.3389/fvets.2023.1148766
Received: 20 January 2023; Accepted: 07 March 2023;
Published: 23 March 2023.
Edited by:
Cristian Piras, Magna Græcia University, ItalyReviewed by:
Carmine Merola, University of Teramo, ItalyCopyright © 2023 Caruso, Scalisi, Pecoraro, Cardaci, Privitera, Truglio, Capparucci, Jarosova, Salvaggio, Caraci and Brundo. This is an open-access article distributed under the terms of the Creative Commons Attribution License (CC BY). The use, distribution or reproduction in other forums is permitted, provided the original author(s) and the copyright owner(s) are credited and that the original publication in this journal is cited, in accordance with accepted academic practice. No use, distribution or reproduction is permitted which does not comply with these terms.
*Correspondence: Giuseppe Caruso, Z2l1c2VwcGUuY2FydXNvMkB1bmljdC5pdA==
†These authors have contributed equally to this work and share senior authorship
Disclaimer: All claims expressed in this article are solely those of the authors and do not necessarily represent those of their affiliated organizations, or those of the publisher, the editors and the reviewers. Any product that may be evaluated in this article or claim that may be made by its manufacturer is not guaranteed or endorsed by the publisher.
Research integrity at Frontiers
Learn more about the work of our research integrity team to safeguard the quality of each article we publish.