- 1Institute of Microbiology, Guangxi Academy of Agricultural Sciences, Nanning, China
- 2Guangxi Veterinary Research Institute, Nanning, China
- 3Yulin Institute of Microbiology, Yulin, China
- 4Guangxi Crop Genetic Improvement and Biotechnology Laboratory, Guangxi Academy of Agricultural Sciences, Nanning, China
Introduction: The experiment was conducted to evaluate the effects of Ganoderma lingzhi culture (GLC) as a fermented feed on growth performance, serum biochemical profile, meat quality, and intestinal morphology and microbiota in Sanhuang broilers. In addition, the association between gut bacteria and metabolites was investigated via untargeted metabolomic analysis.
Methods: A total of 192 Sanhuang broilers (112 days old) with an initial body weight of 1.62 ± 0.19 kg were randomly allocated to four treatments, six replicate pens per treatment with 8 broilers per pen. The four treatments contain a control diet (corn-soybean meal basal diet, CON), a positive control diet (basal diet + 75 mg/kg chlortetracycline, PCON), and the experimental diets supplemented with 1.5 and 3% of GLC, respectively. The trial includes phase 1 (day 1–28) and phase 2 (day 29–56).
Results: The results showed that broilers in PCON and GLC-added treatments showed a lower FCR (P < 0.05) in phase 2 and overall period and a higher ADG (P < 0.05) in phase 2. On day 56, the concentrations of serum SOD (P < 0.05), and HDL (P < 0.05) and cecal SCFA contents (P < 0.05) were increased in broilers fed GLC diets. Broilers fed GLC also showed a higher microbiota diversity and an elevated abundance of SCFA-related bacteria in the caecum. The association between intestinal bacteria and metabolites was investigated via correlation analysis. The differential metabolites in the caecum, such as L-beta-aspartyl-L-aspartic acid and nicotinamide riboside, were identified.
Conclusion: In summary, dietary GCL supplementation could increase growth performance to some extent. Moreover, GLC might benefit broilers' health by improving serum HDL content, antioxidant status, SCFAs contents, bacterial diversity, and probiotic proliferation in the caecum.
Introduction
Animal-source foods are central to maintaining global food security, contributing 25% of protein and 18% of calorie consumption worldwide. A rapid-growing population has led to projected increases of 48 and 57% for milk and meat, respectively, in global demand between 2005 and 2050. With the increasing demand for meat products, the livestock feedstuff shortage is inevitable, as the expected expansion of feed supply will be required to improve from 6.0 to 7.3 billion (1). In commercial poultry, feed costs contribute ~70% of the total production cost. One possible way to reduce the cost is to use unconventional or alternative feed ingredients in the poultry industry. Previous studies have revealed that fermentation treatment could decrease crude fiber content but increase crude protein content in unconventional feed ingredients (2). In terms of cost efficiencies, replacing expensive ingredients such as corn might encourage the utilization of cheaper fermented unconventional feedstuffs in animal diets (3). Studies have shown increasing interest in supplementing unconventional fermented ingredients to take advantage of their positive effect, especially on production parameters and intestinal health (2, 4). It could effectively alleviate feedstuff shortage by developing new feed resources or increasing the utilization rate of conventional feed ingredients.
Ganoderma lingzhi (GL), also named G. lucidum, has been collected, cultivated, and utilized as a traditional edible-medicinal fungus in Asian countries for centuries (5). The primary bioactive substances in GL were polysaccharides, triterpenoids, alkaloids, sterols, peptides, amino acids, and mineral elements, among which polysaccharides and triterpenoids were identified as the most effective ingredients (6). Researches demonstrated that GL showed strong bioactivities, such as antioxidation, immunomodulation, anticancer action, and hepatoprotection (7, 8).
The Ganoderma lingzhi culture (GLC) is produced via solid-state fermentation with GL strain. It possesses abundant nutrients and bioactive substances, such as protein, polysaccharides, and triterpenoids. We hypothesized that GLC could be an effective feed ingredient that may positively affect the growth and health of broilers. Therefore, the study aimed to evaluate the effects of GLC addition on growth performance, serum biochemical parameters, intestinal function (morphology, microbiota, and metabolite), and meat quality in Sanhuang broilers.
Materials and methods
GLC production, animals, experimental design, and sample collection
A sterilized culture media (mainly wheat bran) was inoculated with GL strain and proceeded via solid-state fermentation for 35 days to produce GLC. Subsequently, the fermented mixed culture containing 60% moisture was dried at 60°C. The analyzed nutrient of GLC is listed in Table 1.
A total of 192 16-week-old Sanhuang broilers with an initial body weight of 1.62 ± 0.19 kg were purchased from a local commercial market. All the experimental birds were randomly assigned to four treatments containing six replicates of eight birds per replicate. Four treatment diets included the control diet (corn-soybean meal basal diet), the positive control diet (basal diet + 75 mg/kg chlortetracycline), and the control diet supplemented with 1.5 and 3% GLC, respectively. The composition of experimental diets is presented in Table 2. All nutrient levels met or exceeded the requirements of the yellow-feathered broiler recommended by the Ministry of Agriculture of China (NY/T 3645-2020). The trial lasted for 8 weeks which were divided into two periods. The starter period was from 1 to 28 days, and the finisher period was from 29 to 56 days of age. All broilers were raised in wire-floored cages in an environmentally controlled room with continuous light (10–20 lux). The ambient temperature was maintained at 28–32°C, and the relative humidity was maintained at 65–75%. Indoor ventilation was controlled by industrial fans. Mashed diets and water were provided ad libitum.
The individual body weight (BW) were weighed on day 0, 28, and 56, and the amount of feed was recorded daily to calculate the average daily gain (ADG), average daily feed intake (ADFI), and feed conversion ratio (FCR). On day 56, the 24 broilers (one bird from each pen) were selected by the BW close to the average BW in every pen. The wing vein blood samples (about 5 mL) were acquired using 10 mL vacuum blood collection tube. The serum sample was then obtained at 3,000 rpm for 15 min and stored at −20°C. These same broilers were then stunned and euthanized by immersing in CO2, subsequently, slaughtered for sample collection. The ileum sections (about 5 cm) and breast meat samples (about 100 g) were collected. For further morphology analysis, the ileum fragments were stored in the sterile centrifuge tube (50 mL) containing 30 mL 4% paraformaldehyde at 4°C. The breast meat samples were stored at 4°C for meat quality determination. Cecal digesta samples from each bird (one bird from each pen) were collected and kept in liquid nitrogen for further short chain fatty acid (SCFA), microbiome bioinformatics, and untargeted metabolomic analysis.
Chemical analysis
The GLC sample was assessed for dry matter (DM), crude protein (CP), ether extract (EE), and ash (9). The concentrations of neutral detergent fiber (NDF) and acid detergent fiber (ADF) were measured using fiber analyzer equipment (2010, FOSS, Denmark). The gross energy (GE) value was determined by an oxygen bomb calorimeter (C2000, IKA, DE.). The total triterpenoid content was measured by ultraviolet spectrophotometry (2550, Shimadzu, Japan). The crude polysaccharide content was detected using the phenol-sulfuric acid method (10).
Analysis of serum indices
The contents of superoxide dismutase (SOD), catalase (CAT), malondialdehyde (MDA), total antioxidant capacity (T-AOC), and glutathione peroxidase (GSH-Px) were measured by assay kits (Jiancheng Institute of Biological Technology, Nanjing, China) and determined using automatic biochemical analyzer (model 7170, Hitachi Corp, Tokyo, Japan). The concentrations of total triglyceride (TG), total cholesterol (TC), glucose (GLU), high-density Lipoprotein (HDL), and low-density Lipoprotein (LDL) were measured by assay kits (Jiancheng Institute of Biological Technology, Nanjing, China) according to the manufacturer's instructions and determined at the wavelength of 450 nm.
Measurement of intestinal morphology
Intestinal morphology was measured following the method described by Abdelqader and Al-Fataftah (11). In brief, the ileum samples were cleaned by physiological saline and then embedded in paraffin wax. After staining with hematoxylin and eosin, paraffin sections were prepared. Crypt depth (CD) and villus height (VH) were measured using a light microscope with the morphometric system (Nikon Eclipse E100, Japan). Then, the villus height to crypt depth ratio (V/C) was calculated.
Analysis of intestinal SCFAs
The cecal content was collected on day 56 and used for SCFAs concentration analysis (12). In brief, 1 g of digesta was dissolved in purified water. The samples were shaken violently and centrifuged at 12,000 rpm for 15 min. The supernatant was collected and mixed with 25% (m/v) phosphoric acid. The mixture was placed on ice for 40 min before filtering into special injection bottles for testing. The SCFAs concentrations were measured by ion chromatography (model ICS3000, Thermo Crop, Sunnyvale, USA).
Determination of meat quality
On day 56, breast meat samples (n = 6) were collected to analyze meat quality, including color, pH, and shear force. Meat color was expressed as lightness (L*), redness (a*), and yellowness (b*) and was measured by Chroma Meter (NR, Mingao company, China). The pH values at 45 min and 24 h were determined by a pH meter (IS400, SP company, USA). The pH and meat color were measured in triplicate at three different locations. The shear force was measured following the method described by Ciobanu et al. (13). Briefly, breast meat samples were cooked at 70°C for 20 min. On each piece of meat,10 cylindrical samples (diameter 10 mm × height 10 mm) were cut along the meat fiber direction and measured using a muscle tenderness meter (MAQC-12, Mingao company, China).
16S rRNA sequencing and bioinformatic analysis
Total bacterial DNA from cecal contents was extracted according to the method described by instruction of the E.Z.N.A.® Soil DNA Kit (Omega Bio-Tek, Norcross, GA, USA). The quality of the DNA extract was checked using 1% agarose gel electrophoresis, and the concentration of DNA was determined by spectrophotometer (NanoDrop2000, Thermo Fisher Scientific, USA).
The V3-V4 region of the 16S was amplified using bacterial primers 338F (ACTCCTACGGGAGGCAGCAG) and 806R (GGACTACHVGGGTWTCTAAT). The PCR amplification of each sample was conducted with a 20 μL reaction mixture containing 5 × FastPfu Buffer (4 μL), 2.5 mM dNTPs (2 μL), FastPfu DNA Polymerase (0.4 μL, TransGen, Beijing, China), BSA (0.2 μL), template DNA (10 ng), and each primer (0.8 μL). The procedure consisted of an initial denaturation at 95°C for 3 min, the following 30 cycles of temperature gradient (95°C for 30 s, 55°C for 30 s, and 72°C for 45 s), and finally, an extension step at 72°C for 10 min using a thermal cycler (9700, ABI GeneAmp, USA). Each sample was performed in triplicate. The triplicate amplicons were pooled, electrophoresed in an agarose gel (2%), and recovered by a DNA Gel Extraction Kit (AxyPrep, Axygen Biosciences, USA).
The purified amplicons were quantified by a Fluorometer (Quantus, Promega, USA). The sequencing libraries were constructed using combining equimolar ratios of amplicons. The resulting libraries for paired-end sequencing were determined on an Illumina MiSeq PE 300 platform by Majorbio Technology Inc (Shanghai, China). The original sequence was analyzed using FASTP (https://github.com/OpenGene/fastp, version 0.20.0) for quality control, and the FLASH (http://www.cbcb.umd.edu/software/flash, version 1.2.7) was used for stitching. The OUT clustering was performed on sequences according to 97% similarity using UPARSE (http://drive5.com/uparse/, version 7.1). The silva 16S rRNA gene database (v138) was compared by RDP classifier (http://rdp.cme.msu.edu/, version 2.11) for taxonomic annotation of OTU species. The 16S function prediction analysis was determined by PICRUSt2 (version 2.2.0). All the data were analyzed through the free online platform of majorbio cloud platform (cloud.majorbio.com).
Untargeted caecal metabolomic analysis
Metabolites of cecal contents (50 mg) were extracted by 400 ml 80% methanol (aqueous solution) with 0.02 mg/mL L-2-chlorophenylalanin as internal standard. The mixture was treated with a high-throughput tissue crusher Wonbio-96c (Shanghai wanbo biotechnology co., LTD) at 50 Hz for 6 min at −10°C, then ultrasound was performed at 40 kHz for 30 min at 5°C. The sample was placed at −20°C for 30 min to precipitate the protein. The supernatant was then carefully transferred to the sample bottles for LC-MS/MS analysis after centrifugation at 13,000 × g, 4°C for 15 min. UHPLC-MS/MS analysis was performed by a UHPLC-Q Exactive system of Thermo Fisher Scientific. The chromatographic conditions were as follows: 2 μL of the sample was entered into mass spectrometry detection after being separated by HSS T3 column (100 mm × 2.1 mm i.d., 1.8 μm). The mobile phases consisted of solvent A [0.1% formic acid in water: acetonitrile (95 : 5, v/v)] and solvent B [0.1% formic acid in acetonitrile: isopropanol: water (47.5: 47.5:5, v/v)]. The separation gradient was as follows: 0–0.1 min, solvent B from 0 to 5%; 0.1–2 min, solvent B from 5 to 25%; 2–9 min, solvent B from 25 to 100%; 9–13 min, the linearity of solvent B was maintained at 100%; from 13.0 to 13.1 min, the linearity of solvent B was decreased from 100 to 0%, and from 13.1 to 16 min, the linearity of solvent B was maintained at 0%. The flow rate was 0.4 mL/min. The column temperature was kept at 40°C, and all these samples were stored at 4°C during the analysis period.
The data of mass spectrometric was collected by UHPLC-Q Exactive Mass Spectrometer equipped with an electrospray ionization source. The detection mass range was 70–1,050 m/z. Full MS resolution was 70,000, and MS/MS resolution was 17,500. The conditions were performed as follows: Capillary temperature, 320°C; heater temperature, 400°C; Aux gas flow rate, 10 arb; sheath gas flow rate, 40 arb; ion-spray voltage floating (ISVF), 3,500 V in positive mode and −2,800 V in negative mode respectively; Normalized collision energy, 20-40-60 V rolling for MS/MS.
Statistical analysis
All the data were checked for the normality of equal variances and residuals by the UNIVERIATE of SAS (Version 9.2, SAD Inst. Inc., USA), and were subjected to ANOVA by the GLM procedure of SAS. The LSMEANS was used to separate group means with Turkey's test for adjustment. The value of P < 0.05 was determined as significance, and a value of 0.05 ≤ P < 0.10 was determined as a tendency for significance.
Results
Growth performance and serum biochemical indices
As shown in Table 3, GLC diets had no effect on ADG, ADFI, and FCR from day 1 to 28, compared to the control diet. Broilers in the PCON treatment showed the highest ADG among different treatments. In the finisher period, we observed that dietary GLC supplementation resulted in increased ADG (P < 0.05) and decreased FCR (P < 0.05) and showed a tendency toward a reduction of ADFI (P < 0.10). During the overall period, the GLC-fed broilers showed a lower FCR (P < 0.05) compared to those fed control diets. A decreasing trend was found on ADFI (P < 0.10) for broilers fed GLC diets from day 1 to 56. There was no difference between ADG and FCR to broilers in GLC and PCON treatments during the overall period.
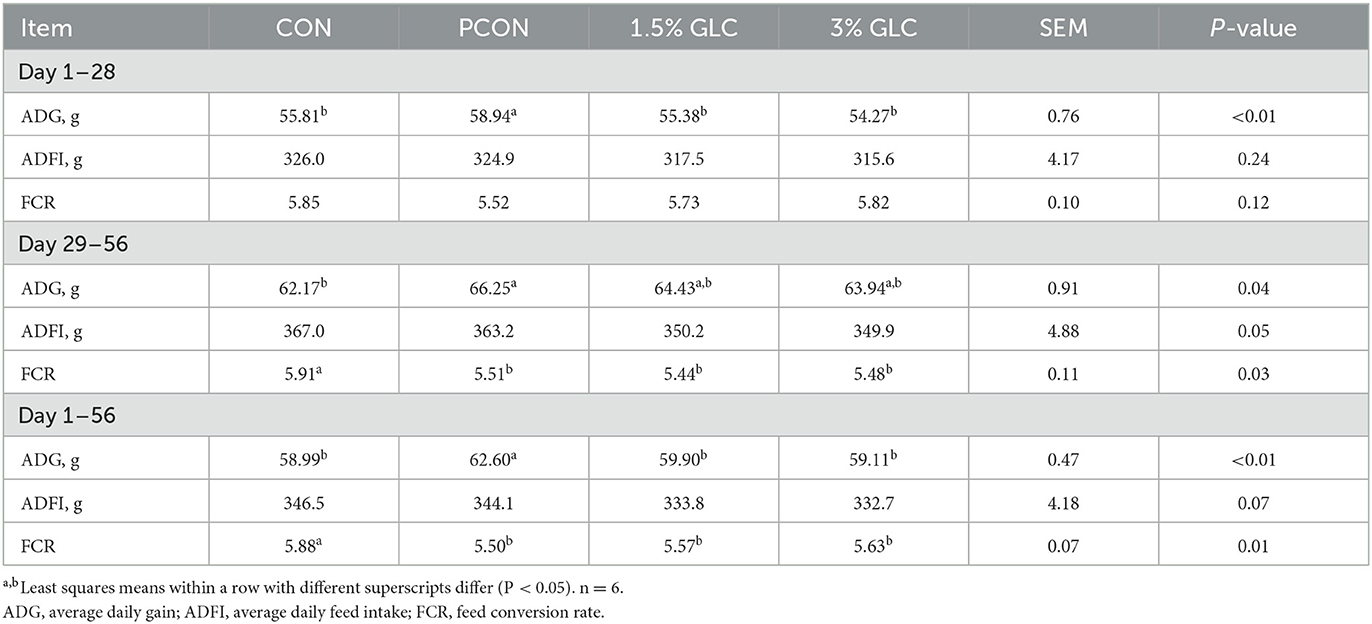
Table 3. Effects of Ganoderma lingzhi culture (GLC) supplementation on growth performance of Sanhuang Chicken during day 1–56.
As shown in Table 4, broilers fed 1.5 or 3% GLC had higher HDL (P < 0.05) and SOD (P < 0.05) concentrations in serum compared to those fed the control and positive control diets. A decreasing trend of LDL content (P < 0.10) was also observed after GLC addition.
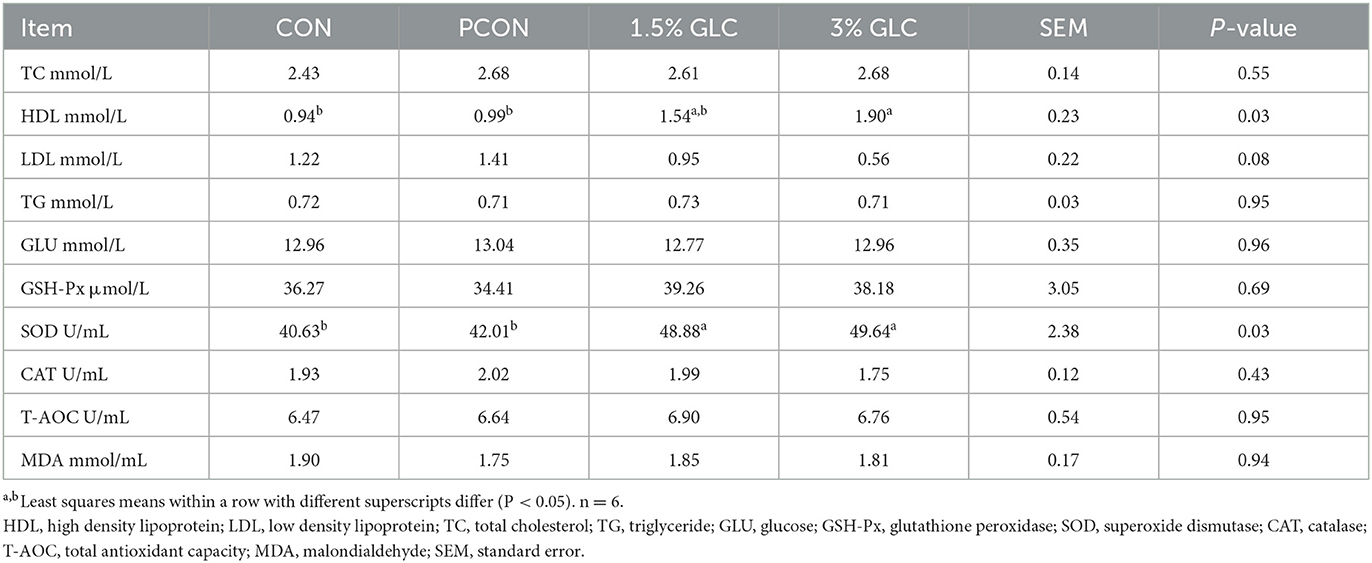
Table 4. Effects of dietary Ganoderma lingzhi culture (GLC) supplementation on serum profile of Sanhuang Chicken on day 56.
Intestinal morphology and SCFAs contents
As shown in Table 5 and Figure 1, GLC supplementation showed no effect on gut morphology in broilers. Dietary GLC addition showed no effect on the acetate, propionate, or butyrate concentrations. However, increased total SCFA contents (P < 0.05) were revealed after GLC supplementation (Table 6).

Table 5. Effects of dietary Ganoderma lingzhi culture (GLC) supplementation on the ileum morphology of Sanhuang broilers on day 56.
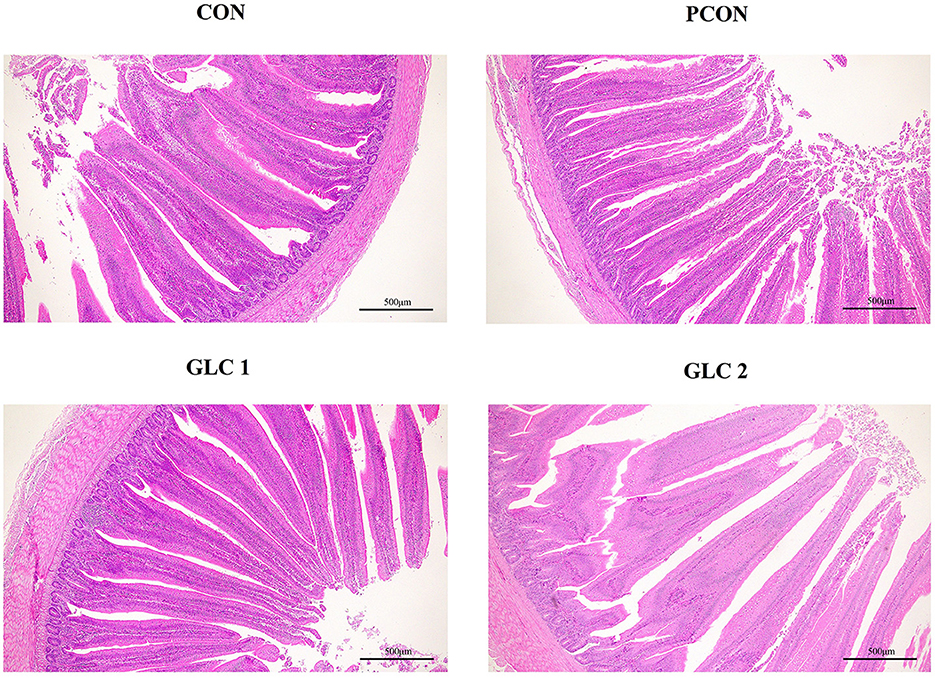
Figure 1. Effect of Ganoderma lingzhi culture (GLC) supplementation on the ileum morphology of Sanhuang broilers (n = 6; CON, control diet; PCON, positive control diet; GLC 1: 1.5% GCL diet; GLC 2: 3% GCL diet).
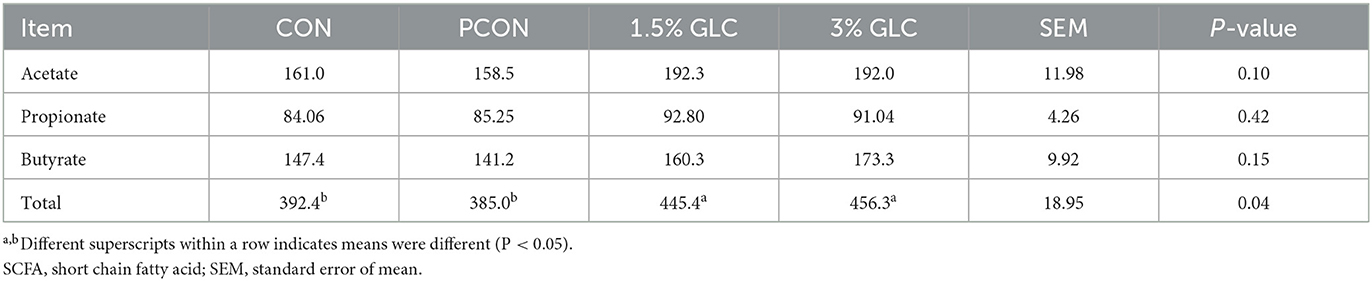
Table 6. Effects of dietary Ganoderma lingzhi culture (GLC) supplementation on SCFA concentrations in cecum of Sanhuang broilers on day 56.
Meat quality
As shown in Table 7, GLC supplementation revealed an increased trend in pH value at 45 min (P < 0.10) and did not affect pHat 24 h, meat color, and shear force.
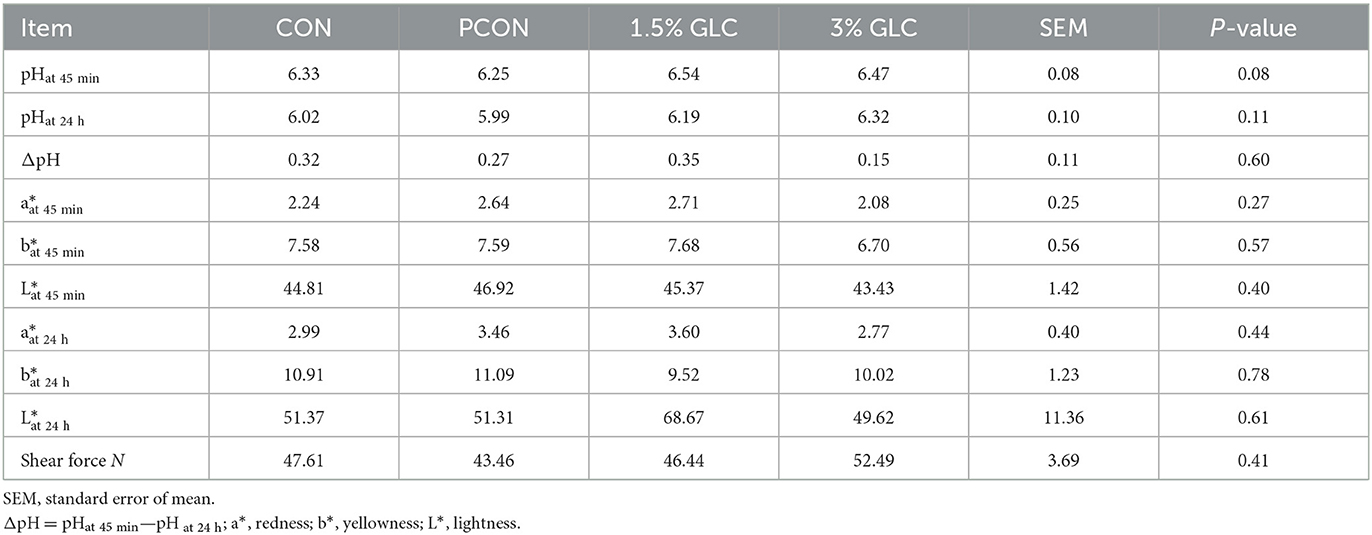
Table 7. Effects of dietary Ganoderma lingzhi culture (GLC) supplementation on meat quality of Sanhuang broilers.
Effect of GLC on the caecal microbiota
As shown in Figure 2, the similarity and overlap of operational taxonomic units (OTUs) among four treatments were summarized in the Venn diagram. The results revealed that all treatments shared 714 OTUs, while certain OTUs were unique to specific treatment (30 for the CON treatment, 12 for the PCON treatment, 23 for the 1.5% GLC treatment, and 30 for the 3% GLC treatment, respectively), indicating that the intervention of Chlortetracycline Hydrochloride and GLC exerted little influence on the OTUs composition of caecal bacteria in chicken. Furthermore, PCA exhibited a clear separation between CON, PCON, and GLC treatments in species-level composition (Figure 3).
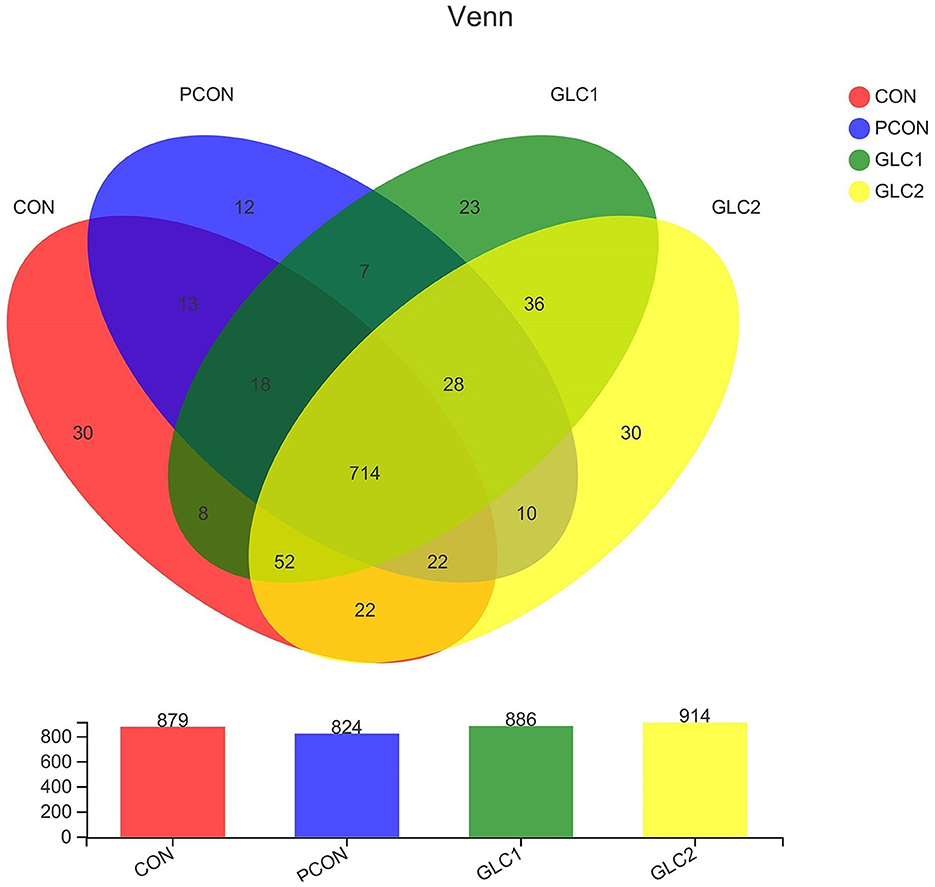
Figure 2. Effect of Ganoderma lingzhi culture (GLC) supplementation on Venn diagram analysis of the caecal microbiota (n = 6; CON, control diet; PCON, positive control diet; GLC 1: 1.5% GCL diet; GLC 2: 3% GCL diet).
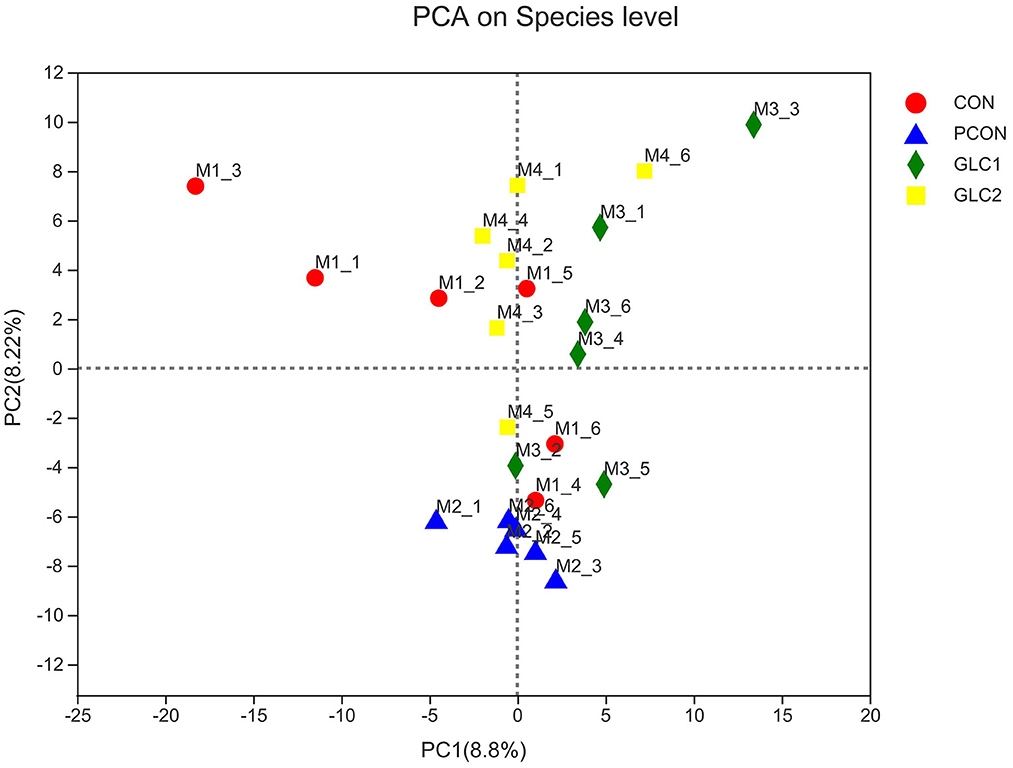
Figure 3. Effect of Ganoderma lingzhi culture (GLC) supplementation on PCA analysis of the caecal microbiota (n = 6; CON, control diet; PCON, positive control diet; GLC 1: 1.5% GCL diet; GLC 2: 3% GCL diet).
As shown in Figure 4, the Coverage, Ace, and Chao indices of the broilers fed 3% GLC were significantly higher than those of the control and positive control treatments. The results implied that the bacterial diversity and richness of the broilers fed 3% GLC changed remarkably compared with CON and PCON treatments. As shown in Figure 5, Firmicutes and Bacteroidota hold most of the community abundance at the phylum level. Compared with CON and PCON treatment, broilers fed GLC showed a higher relative abundance of Bacteroidaceae, and a lower relative abundance of Lactobacillaceae and Barnesiellaceae at the family level.
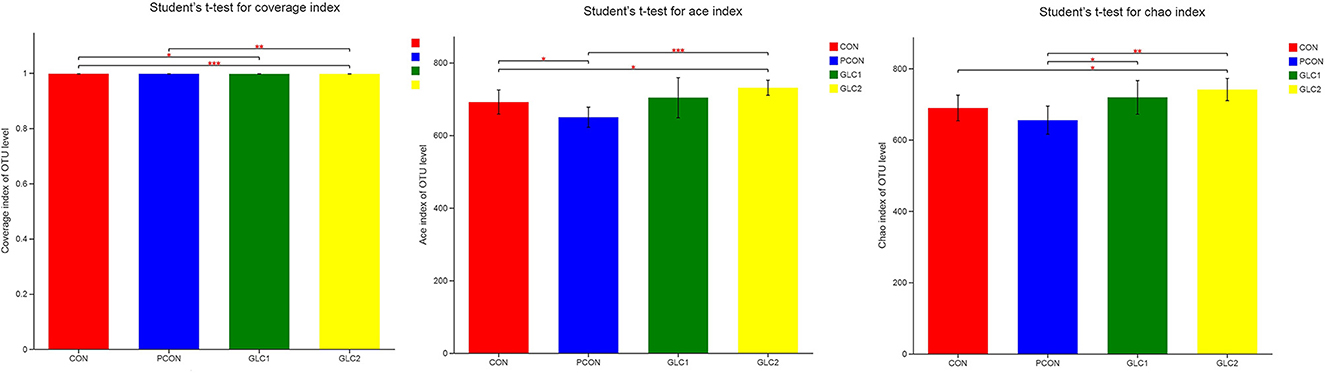
Figure 4. Effect of Ganoderma lingzhi culture (GLC) supplementation on alpha diversity of the caecal microbiota (n = 6; CON, control diet; PCON, positive control diet; GLC 1: 1.5% GCL diet; GLC 2: 3% GCL diet). *p < 0.05, **p < 0.01, ***p < 0.001.
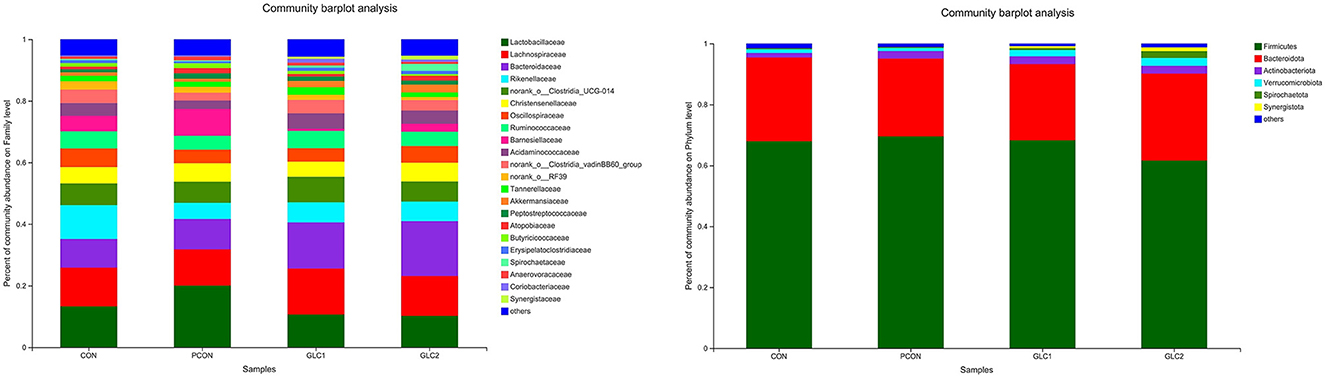
Figure 5. Effect of Ganoderma lingzhi culture (GLC) supplementation on alterations in caecal microbiota at phylum and family level (n = 6; CON, control diet; PCON, positive control diet; GLC 1: 1.5% GCL diet; GLC 2: 3% GCL diet).
As illustrated in Figure 6, the LDA score>2 means the difference was statistically significant. Compared with the CON treatment, the relative abundance of g__unclassified_f__Coriobacteriaceae, g__Marvinbryantia, g__Coprobacter, g__NK4A214_group, g__Christensenella, g__Faecalicoccus, g__GCA-900066575, g__Lachnospiraceae_NK4A136_group, g__Bifidobacterium, g__Anaerofilum, g__Pseudoflavonifractor, f__unclassified_o__Oscillospirales, and g__unclassified_o__Oscillospirales was increased significantly after GLC supplementation. In the cecum, an increase in the Synergistota populations on the phylum level was found in broilers fed the GLC diet (Figure 7). Moreover, on the Family level, the relative abundance of Butyricicoccaceae and norank_o__Rhodospirillales decreased while the relative abundance of Synergistaceae increased in the GLC treatments, compared to CON treatment.
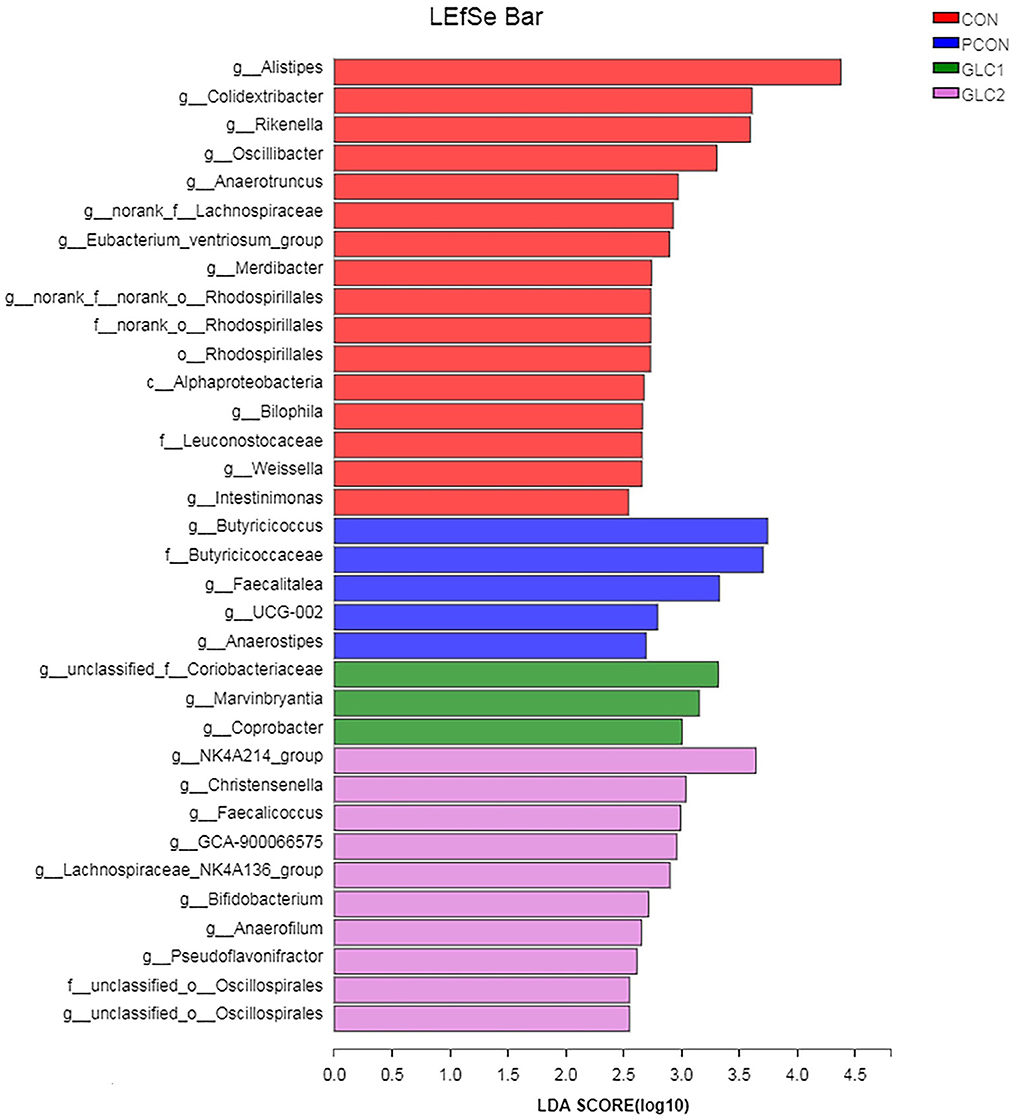
Figure 6. Effect of Ganoderma lingzhi culture (GLC) supplementation on LEfSe analysis of the caecal microbiota (n = 6; CON, control diet; PCON, positive control diet; GLC 1: 1.5% GCL diet; GLC 2: 3% GCL diet).
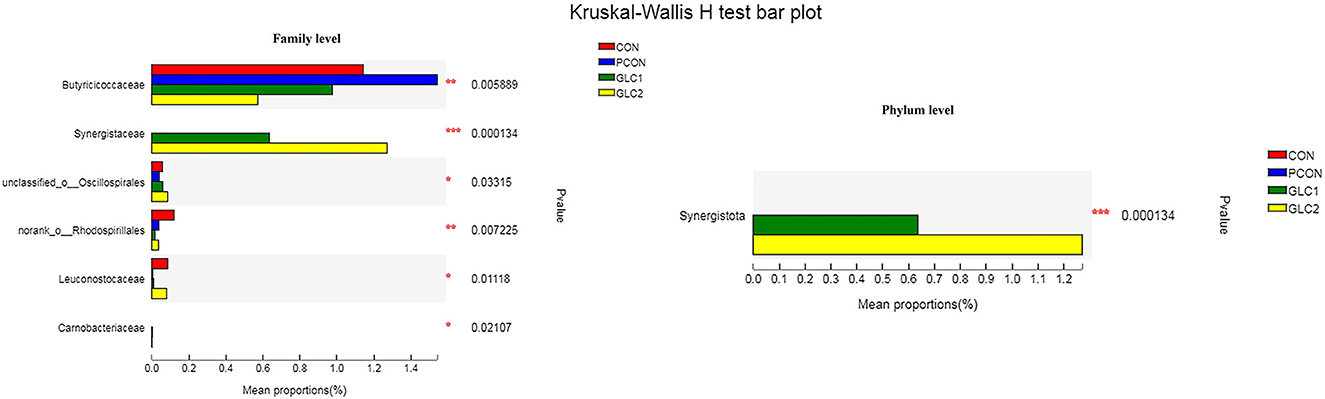
Figure 7. Effect of Ganoderma lingzhi culture (GLC) supplementation on Kruskal-Wallis H-test bar plot at phylum and family level of the caecal microbiota (n = 6; CON, control diet; PCON, positive control diet; GLC 1: 1.5% GCL diet; GLC 2: 3% GCL diet). *p < 0.05, **p < 0.01, ***p < 0.001.
Untargeted metabolomic analysis of caecal digesta
Screening of metabolic differences
The results have shown that the caecal microbiota profiling of broilers changes significantly after supplementation of 3% GLC. Therefore, metabolomics was employed to ascertain the changes in microbiota metabolites, further revealing the underlying mechanism of GLC improving growth to broilers. Metabolic changes of CON and 3% GLC samples were estimated by PCA (an unsupervised principle component analysis, Figure 8). The QC samples were gathered closely, indicating that UHPLC-MS/MS system showed good stability. Meanwhile, the samples of 3% GLC treatments were not significantly separated from the CON treatment, which demonstrated that the metabolome of the samples was mostly similar. The PLS-DA was used to generate the two-dimensional score plots. The CON and 3% GLC treatments have partly overlapping distributions of data points, suggesting that the metabolome of samples in CON and 3% GLC treatments are similar.
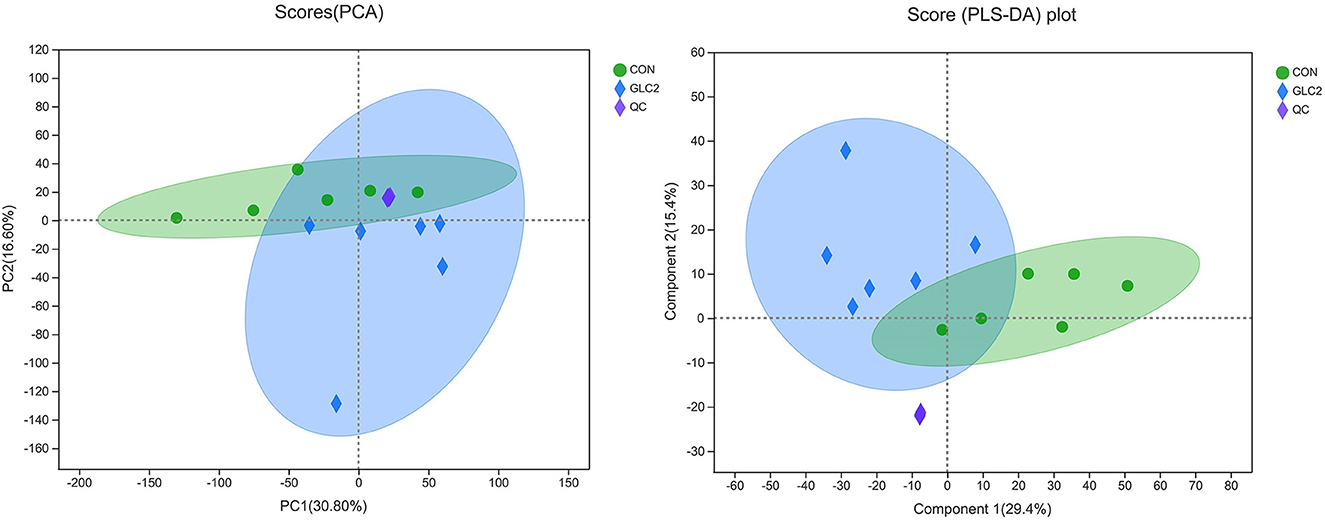
Figure 8. Effect of Ganoderma lingzhi culture (GLC) supplementation on two-dimensional PCA score of the caecal metabolites (n = 6; CON, control diet; GLC 2: 3% GCL diet; QC, quality control).
Furthermore, the results of differential metabolite analysis between CON and 3% GLC treatments showed (VIP > 1 and P < 0.01) that 170 metabolites with significant differences were identified between CON and 3% GLC treatments. As shown in Supplementary Table 1, the top 50 metabolites most likely to be potential biomarkers were screened. The data have shown that, compared with CON treatment, the levels of 17 kinds of organic acids and derivatives [L-beta-aspartyl-L-aspartic acid, 2-Amino-4- [(2-hydroxy-1-oxopropyl) amino] butanoic acid, methionyl-Proline, etc.], two kinds of organic oxygen compounds (nicotinamide riboside, 5,6-Dihydrouridine), eight kinds of lipids and lipid-like molecules (3-carboxy-4-methyl-5-pentyl-2-furanpropanoic acid, menthone 1,2-glyceryl ketal, 8-Deoxy-11-hydroxy-13-chlorogrosheimin, etc.), two kinds of benzenoids (epinephrine, 5-(3′,4′-Dihydroxyphenyl)-gamma-valerolactone), three kinds of nucleotides and analogs (2′,3′-Dideoxy-3′-fluorouridine, 5-Methyldeoxycytidine, cotinine glucuronide), three kinds of organoheterocyclic compounds (pyridoxine, 2′,3′-Didehydro-2′,3′-dideoxyguanosine, gravacridonediol), two kinds of phenylpropanoids and polyketides (3-Hydroxycoumarin, liquiritigenin), one kind of alkaloids and derivative (1,2,3,4-Tetrahydro-b-carboline-1,3-dicarboxylic acid), and seven other metabolites increased. While levels of 3-alpha,20-alpha-Dihydroxy-5-beta-pregnane 3-glucuronide, 3-(3,4,5-Trimethoxyphenyl) propanoic acid, 18R-hydroxy-5Z,8Z,11Z,14Z,16E-eicosapentaenoic acid, (3S,5S)-3, 5-Diaminohexanoate, and 1 other metabolite decreased.
As shown in Figure 9, the volcano map was constructed to visualize the potential biomarkers between CON and 3% GLC treatments. The ordinate was -log 10 (P-value), and the abscissa was log 2 (fold change). Each metabolite was represented by a point. The L-beta-aspartyl-L-aspartic acid, nicotinamide riboside, 2-amino-4-[(2-hydroxy-1-oxopropyl) amino] butanoic acid, methionyl-proline, topaquinone, and 8-azaadenosine were selected as biomarkers between the CON and 3% GLC treatments. All metabolites obtained from level-two identification were assigned to the HMDB (Human Urine Metabolome Database) database, 326 of which were matched and classified into 10 HMDB super classes. Meanwhile, “organic acids and derivatives,” which contained 101 metabolites, was the first class (30.98%), followed by “lipids and lipid-like molecules” (96 metabolites, 29.45%) and “organoheterocyclic compounds” (47 metabolites, 14.42%; Figure 10).
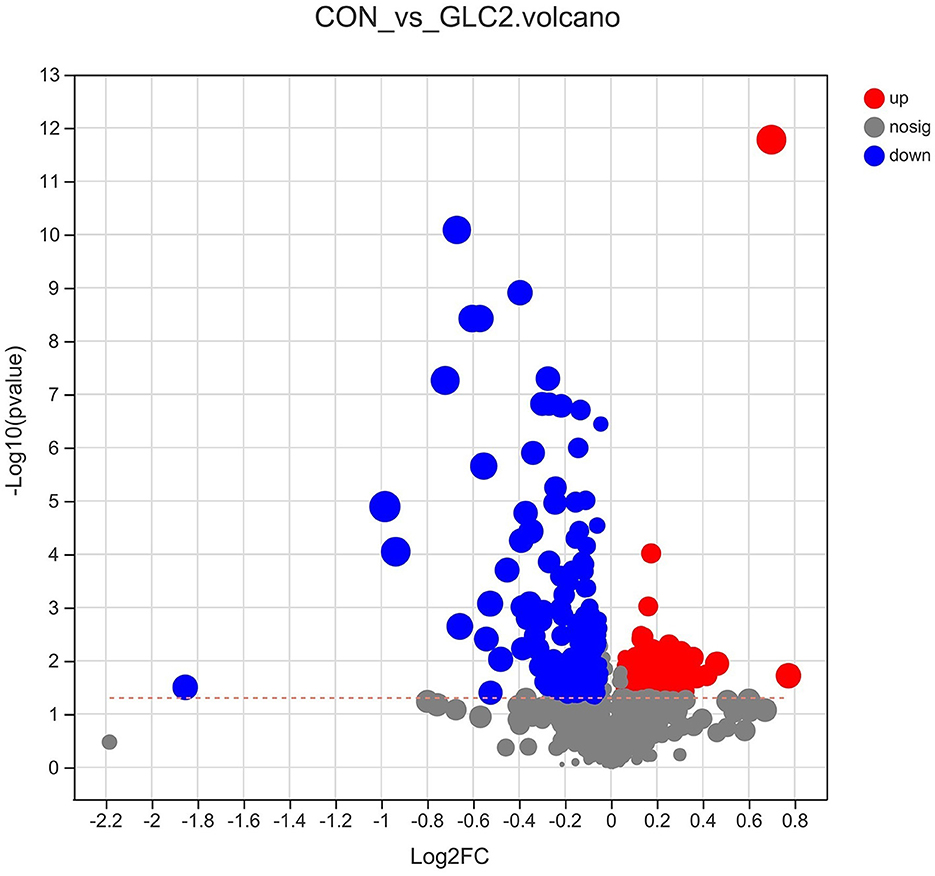
Figure 9. Effect of Ganoderma lingzhi culture (GLC) supplementation on volcano plot analysis of the caecal metabolites (n = 6; CON, control diet; GLC 2: 3% GCL diet).
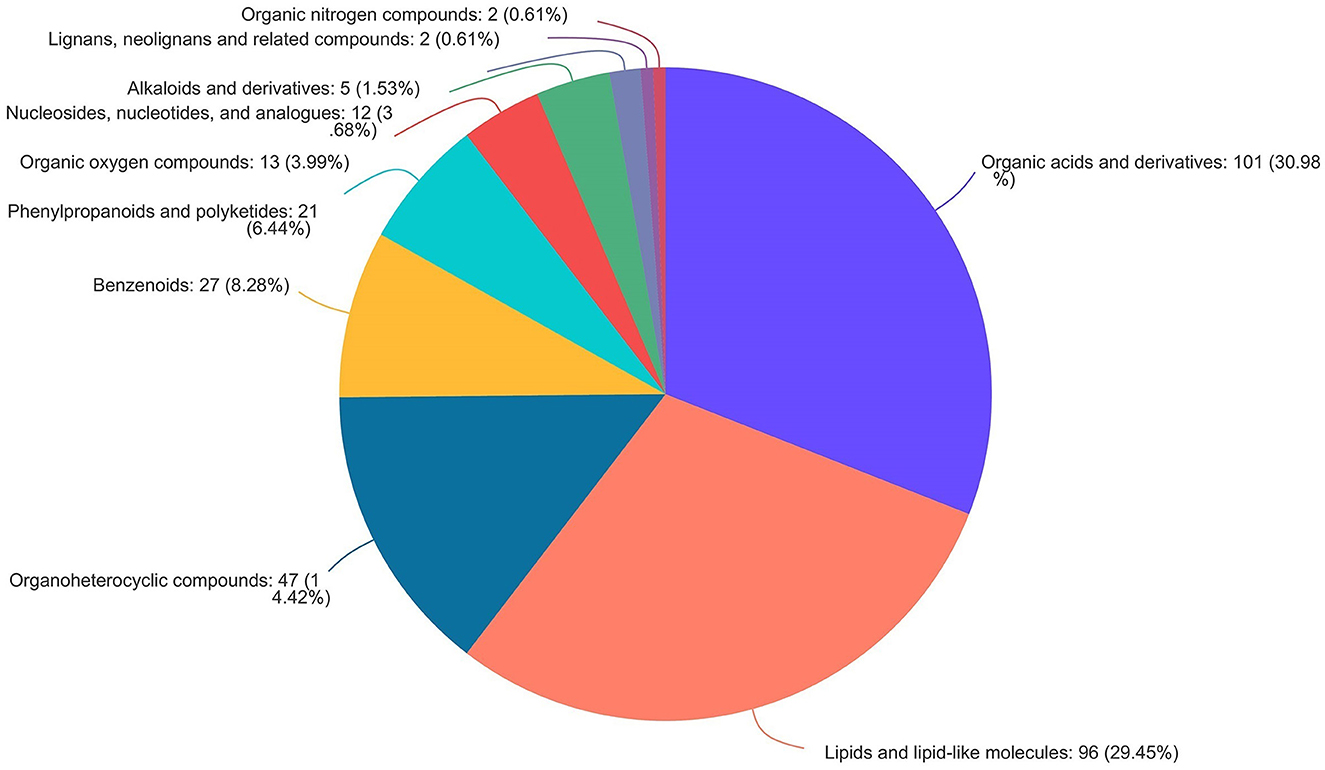
Figure 10. Pie chart of HMDB chemical taxonomy (super class) analysis of the caecal metabolites (n = 6).
Correlation analysis between microbiota and metabolites
As shown in Figure 11, to further investigate the potential functional connection between microorganisms and metabolites, a correlation heatmap was established according to Pearson's correlation analysis at the phylum and family level. The relative abundance of f__Synergistaceae was positively correlated with hydroxyprolylhydroxyproline, pyridoxine, aspartyl-leucine, and L-N-(3-Carboxypropyl) glutamine. The hydroxyprolylhydroxyproline and aspartyl-leucine were negatively correlated with the abundances of f__Butyricicoccaceae, f__Rikenellaceae, and f__norank_o__Rhodospirillales. The L-N-(3-Carboxypropyl) glutamine was positively correlated with the relative abundance of f__Synergistaceae, and negatively correlated with the relative abundance of f__norank_o__Rhodospirillales.
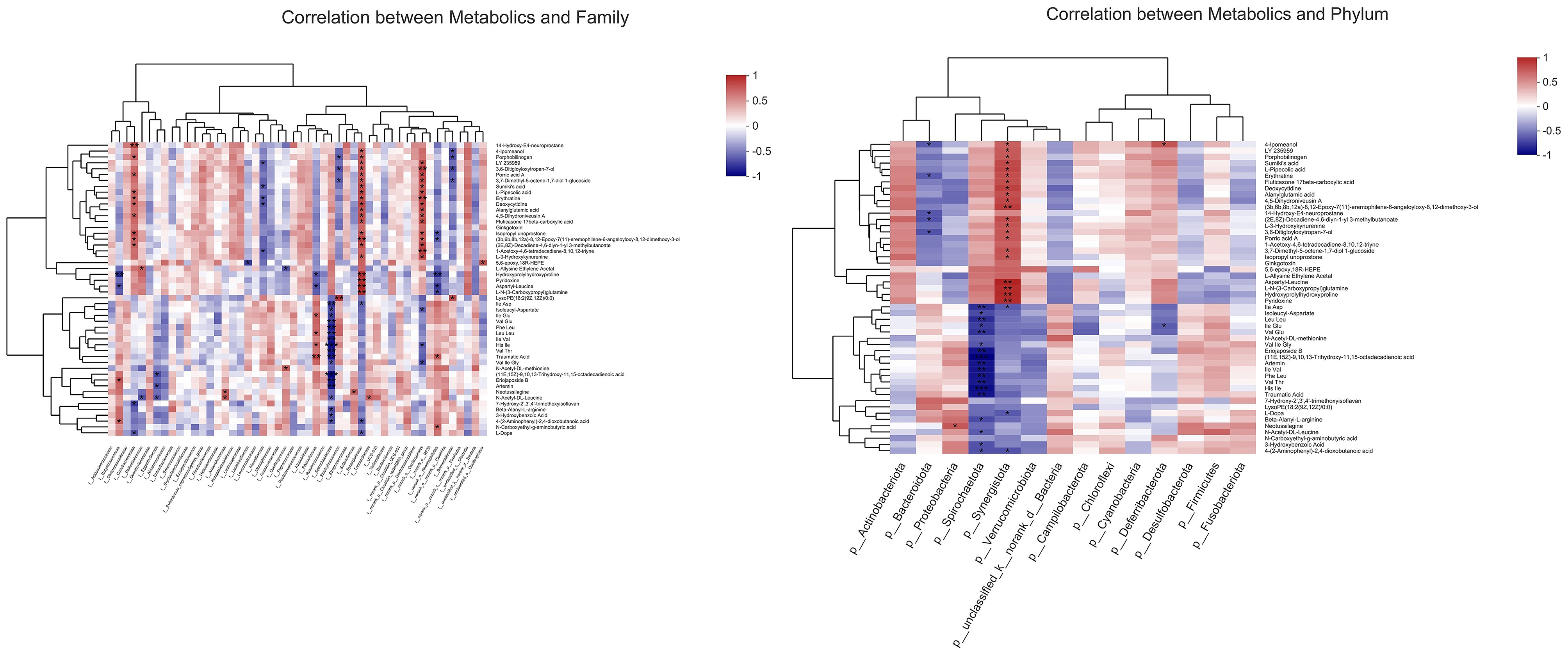
Figure 11. Correlation analysis between metabolites and microorganisms at phylum and family level (n = 6). *p < 0.05, **p < 0.01, ***p < 0.001.
Discussion
The current study detected a notable decrease in FCR of birds-fed GLC during the finisher and overall period, compared to the control diet. Similar studies have shown that the bioactive substance contained in GL could remarkably improve the growth performance of broilers. Dietary supplementation of 200 mg/kg GL spores to aflatoxin B1-contaminated diets could counteract its adverse effects on the growth performance of broilers during the overall period (6). Moreover, broilers fed diets supplemented with GL spores revealed an increased ADG and decreased FCR during the finisher period (14).
The mechanism by which GLC reduces FCR has yet to be fully elucidated. It could be partially attributed to the antioxidative characteristics of GLC. During the finisher period, growing broilers produce enhanced oxidative free radicals and endogenous calories due to body weight-correlated changes in endocrine function, body composition, and metabolic rate (14). We observed an increased antioxidant enzyme activity after GLC supplementation. The previous study showed that the GL polysaccharides could improve serum GSH-PX and SOD and reduce MDA in mice with chronic pancreatitis (15). The spores of GL might remarkably mitigate the oxidative stress level in diabetic mice and promote the recovery of body weight loss under stress (16).
We also found that broilers fed GLC showed a decreasing trend on ADFI. We deduced that the higher dietary fiber content in GLC (NDF: 45.00% and ADF 13.90%) might negatively affect the ADFI of broilers. Other studies reported that broilers fed higher fiber diets had a lower feed intake than control diets (17, 18).
We observed that GLC supplementation could increase serum HDL and show a decreased trend in LDL levels. The previous study found that GL polysaccharide complex could significantly down-regulate the low-density lipoprotein cholesterol (LDL-C) in the serum of pre-diabetic mice (19). Reactive oxygen species (ROS) and free radicals, as side-products of metabolic processes, might seriously injure cells via oxidation processes. We found that GLC could improve SOD levels in broilers, which is probably due to the polysaccharides and triterpenoids in GLC. Evaluating the antioxidant activity of GL polysaccharides or triterpenoids has been done via different methods in vitro or in vivo. All polysaccharides or triterpenoids derived from different parts of GL have confirmed the ROS scavenging abilities (20). A study showed that triterpenoids obtained from GL could improve the activities of SOD, CAT, and antioxidant enzymes in mouse splenic lymphocytes in vitro (21). The polysaccharides from GL have been demonstrated the capacity to induce the synthesis of SOD and CAT, scavenging on DPPH, and reducing lipid peroxidation (22). In another study, a GL polysaccharide showed a potent antioxidant effect via improving SOD, GSH-Px, and CAT activities in the liver of type 2 diabetic rats (23).
We found the GLC addition could promote total SCFAs content in the cecum of broilers, and the dietary fiber (especially β-glucan) in GLC might play a vital role. The dietary fiber, such as β-glucan and pectin, could not be digested directly in the upper gastrointestinal tract. The SCFAs are the primary metabolites produced by microbiota via fermenting dietary fiber in the large intestine. Xie et al. (24) found that the GL polysaccharides consist of β-1,3/1,6-glucan and some other carbohydrates, in which β-1,3-glucan was the main active ingredient. The GL polysaccharides consumption could produce more SCFAs (acetate, propionate, and butyrate) by increasing the SCFA-producing bacteria population, such as Ruminococcus_1, in the cecum of the rat. In the study of Zhu et al. (23), dietary supplementation of polysaccharides isolated from GL could significantly increase the SCFAs contents in serum, liver, and feces of diabetic rats after treatment for 4 weeks.
As is well-known, meat pH value is associated with muscle lactic acid content derived from anaerobic glycolysis (25). A higher meat pH value always correlates with decreased drip loss (higher water-holding capacity), darker color, and improved firmness. Higher ultimate pH meat would produce more acceptable quality by reducing the risk for higher drip loss and pale, soft, exudative (PSE) meat (26). Interestingly, we observed that GLC supplementation showed a trend to improve breast meat pH value at 45 min. That is, GLC addition could slow down glycolysis, thus, decreasing lactic acid concentration to some extent. However, the underlying mechanism of its protective actions remains to be explored.
The intestinal microorganisms are complex communities and have established a close symbiotic relationship with their host that contributes to the maturation of the immune system, prevention of pathogen colonization, synthesis of some vitamins, and metabolism of indigestible components (27). We assumed an underlying relation exists between the decreased FCR and higher microbiota diversity after 3% GLC addition. Previous studies suggested that higher microbial diversity is associated with more productive and stable ecological communities and stronger resistance to invasive species, leading to more efficient dietary utilization (28, 29). Zhou et al. have revealed that the intestinal microbiota is strongly linked to chicken health and phenotypes, such as feed efficiency (30).
In the current study, the relative abundance of Firmicute and the ratio of Firmicutes to Bacteroidetes (F/B) decreased in the 3% GLC treatment, compared to the CON treatment (2.84–2.41). Krajmalnik-Brown et al. (31) have proposed that the Firmicutes were more efficient in extracting energy from diets, compared to Bacteroidetes, resulting in higher absorption of calories and subsequent weight gain, inconsistent with our results. Interestingly, as mentioned above, the increased bacterial diversity caused by GLC addition could be linked to improved feed efficiency. We assumed that feed efficiency is affected by many factors, such as digestion and absorption in the gastrointestinal tract and microbial fermentation in the hindgut. This complexity could lead to a discrepancy. In the study of Chang et al. (32), they revealed that a water extract of Ganoderma mycelium (WGM) could modulate intestinal microbiota composition, mainly by decreasing the F/B ratio in mice.
In addition, the GLC supplementation could increase the relative abundance of the phylum Synergistota, in which the bacteria were mainly anaerobic microorganisms (33). Few studies about Synergistota were found, and the effect of the improved community of Synergistota on broilers needs further investigation. We also found that broilers fed GLC had a higher abundance of Bacteroidaceae. Wang et al. also observed that the β-glucan is fermented in the lower gastrointestinal tract, resulting in a microbiota composition shift, especially the improved Bacteroidota and reduced Firmicutes (34). The species of Bacteroidaceae are common intestinal bacteria members in all warm-blooded animals and could be considered probiotics (35).
A previous study indicated that the polysaccharides extracted from GL could increase the relative abundance of Lachnospiraceae, which is in accordance with our findings (36). Lachnospiraceae, as a beneficial bacterium, could participate in carbohydrate fermentation into gases (CO2 and H2) and SCFAs (37). The suppression of fermentation-related bacteria might lead to a decline in SCFA production, resulting in a higher colonic pH value and increased ammonia production and absorption in the intestine. Thus, Lachnospiraceae was also supposed to be a good indicator shown the gut state (36, 38).
We observed that 3% GLC could increase Bifidobacterium communities. The Bifidobacterium mainly acquires energy from indigestible food debris, especially the complex dietary fiber (39). As reported, the polysaccharides from GL are potential prebiotics, and their supplementation could improve the relative abundance of Bifidobacterium (40, 41). According to LDA Effect Size, Lachnospiraceae, and Bifidobacterium were selected as biomarkers with remarkable differences between treatments, which improved significantly after 3% GLC supplementation. Previous studies have shown that Lachnospiraceae and Bifidobacterium could promote SCFA production to maintain intestinal homeostasis to a certain degree (37, 42).
The metabolites of intestinal bacteria are relatively small molecules and could intensively influence tissue metabolism. The metabolites could penetrate the gut wall into the blood and are delivered to tissues and organs via blood circulation (43). In the current study, the L-beta-aspartyl-L-aspartic acid, nicotinamide riboside, 2-amino-4-[(2-hydroxy-1-oxopropyl) amino] butanoic acid, methionyl-proline, topaquinone, and 8-azaadenosine were selected as biomarkers after screening the differential metabolites by volcanic map analysis.
L-beta-aspartyl-L-aspartic acid or β-aspartylaspartic acid is a sort of dipeptides and are derivatives of asparagine (44). Nicotinamide riboside (NR) serves as a precursor of hepatic nicotinamide adenine dinucleotide phosphate (NADP+) and NADPH, which are vital for resistance to oxidative stress (45, 46). The 2-amino-4-[(2-hydroxy-1-oxopropyl) amino] butanoic acid is a sort of alpha amino acids, and have the L-configuration of the alpha-carbon atom. It also known as 2-amino-4-(lactoyl amino) butyric acid, and belongs to organic compounds. To our knowledge, only a few studies have been published on it (HMDB). Methionyl-proline is a dipeptide composed of proline and methionine, which is an incomplete breakdown product of protein catabolism or protein digestion. Some dipeptides are proven to have cell-signaling or physiological effects (HMDB). Topaquinone, also known as the quinone of 2,4,5-trihydroxyphenylalanine, is the cofactor in most copper-containing amine oxidases (47).
The changes in gut microbiota elicited by GLC digestion will inevitably trigger alterations in microbial metabolites. The correlation heat map was established to clarify the potential association between microorganisms and metabolites in broilers treated with 3% GLC. The data showed that the family increased after 3% GLC addition, such as f__Coriobacteriaceae, f__Synergistaceae, and f__norank_o__Oscillospirales were positively correlated with porric acid A, L-pipecolic acid, and deoxycytidine (Figure 11). The porric acids, is a tricyclic triterpenoid. As a fungal metabolite, it is also found to exhibit antifungal activity (48). The L-Pipecolic acid is a cyclin amino acid. It is derived from L-lysine and an intermediate of the catabolism of D, L-lysine. It participates in various physiological processes in animals, plants, and microorganisms, such as the interactions between organisms, and acts as a precursor of natural bioactive molecules (49).
The Coriobacteriaceae family is strictly anaerobic, gram-positive bacteria. The Coriobacteriaceae might play an essential role in regulating glucose homeostasis in animals, and it might be beneficial to host glucose metabolism. The previous studies showed that Coriobacteriaceae had a strong negative correlation between hepatic glucose and glycogen levels. Moreover, positive correlations were found between Coriobacteriaceae and hepatic triglycerides levels in mice and non-high-density lipoprotein cholesterol in the plasma of hamsters (50, 51).
We observed that GLC supplementation could increase the relative abundance of f-Synergistaceae. The previous study revealed that most cultured bacteria from the family Synergistaceae could ferment amino acids into SCFAs. Certain species might also degrade SCFAs with methanogens via syntrophic relationships during anaerobic digestion (52).
The Oscillospirales, a butyrate producer (53), is significantly correlated with metabolites, such as LY 235959, 3,6-Ditigloyloxytropan-7-ol, porric acid A, 3,7-dimethyl-5-octene-1,7-diol 1-glucoside, Sumiki's acid, L-pipecolic acid, after 3% GLC supplementation, compared to CON treatment. The Rikenellaceae, belonging to the Bacteroidetes phylum, might contribute to decreased visceral fat mass. It might act as an adiposity modulator by affecting the production of acetate and propionate (54).
Based on the results above, we proposed that the elevated SCFAs contents in GLC-treated broilers might be positively correlated with the increased SCFAs-related bacteria, such as Synergistota, Lachnospiraceae, Bifidobacterium, Synergistaceae, Oscillospirales, and Rikenellaceae. Namely, the GLC could influence host health by promoting the relative abundance of SCFAs-related microbe. Despite various links among the metabolism, gut microbiota, and metabolites, this effort only occupied a part portion of the entire metabolic system of the host. Thus, the underlying mechanism of the effects of GLC on broiler's growth and health still needs to be further explored.
Conclusion
This study determined the effects of GLC supplementation on growth performance, serum biochemical indices, meat quality, intestinal morphology, microbiota, and metabolites. The results revealed that GLC could reduce FCR, increase serum SOD and HDL levels, and enhance cecal SCFA contents. The study also confirmed that GLC could increase bacteria diversity and promote the proliferation of SCFAs-generated bacteria in the gut. Differential metabolites, such as L-beta-aspartyl-L-aspartic acid and nicotinamide riboside, were identified. Overall, GLC could positively affect the health of Sanhuang broilers, and its utilization provides a new target for developing biological feed via edible and medicinal fungi fermentation.
Data availability statement
The data presented in the study are deposited in the Genome Sequence Archive in National Genomics Data Center, accession number CRA010095.
Ethics statement
The animal study was reviewed and approved by Institutional Animal Care and Use Committee of Guangxi Academy of Agricultural Sciences.
Author contributions
XL designed the study and analyzed the data. LH, YS, XW, YLuo, SW, YQ, YLu, WZ, and YJ performed the animal experiment and analyzed the tissue samples. YY and YLi revised the manuscript and had primary responsibility for the final content. All authors read and approved the final manuscript.
Funding
This research was financially supported by the Guangxi key R&D plan program (AB22035030), the Achievement Transformation Project of Guangxi Academy of Agricultural Sciences (NZC202209), the China Agriculture Research System (CARS20), the Special Fund for Basic Scientific Research of Guangxi Academy of Agricultural Sciences (2023YM92), the Science and Technology Pioneer of Edible Fungi Industry (GNKM202108), and the Science and Technology Development Fund of Guangxi Academy of Agricultural Sciences (2022JM07 and 2023YM103).
Conflict of interest
The authors declare that the research was conducted in the absence of any commercial or financial relationships that could be construed as a potential conflict of interest.
Publisher's note
All claims expressed in this article are solely those of the authors and do not necessarily represent those of their affiliated organizations, or those of the publisher, the editors and the reviewers. Any product that may be evaluated in this article, or claim that may be made by its manufacturer, is not guaranteed or endorsed by the publisher.
Supplementary material
The Supplementary Material for this article can be found online at: https://www.frontiersin.org/articles/10.3389/fvets.2023.1143649/full#supplementary-material
References
1. Kim SW, Less JF, Wang L, Yan T, Kiron V, Kaushik SJ, et al. Meeting global feed protein demand: Challenge, opportunity, and strategy. Annu Rev Anim Biosci. (2019) 7:221–43. doi: 10.1146/annurev-animal-030117-014838
2. Sugiharto S, Ranjitkar S. Recent advances in fermented feeds towards improved broiler chicken performance, gastrointestinal tract microecology and immune responses: A review. Anim Nutr. (2019) 5:1–10. doi: 10.1016/j.aninu.2018.11.001
3. Supriyati Haryati T, Susanti T, Susana IWR. Nutritional value of rice bran fermented by Bacillus amyloliquefaciens and humic substances and its utilization as a feed ingredient for broiler chickens. Asian Australas J Anim Sci. (2015) 28:231–8. doi: 10.5713/ajas.14.0039
4. Alshelmani MI, Loh TC, Foo HL, Sazili AQ, Lau WH. Effect of feeding different levels of palm kernel cake fermented by Paenibacilluspolymyxa ATCC 842 on nutrient digestibility, intestinal morphology, and gut microflora in broiler chickens. Anim Feed Sci Technol. (2016) 216:216–24. doi: 10.1016/j.anifeedsci.2016.03.019
5. Dong Q, Wang Y, Shi L, Yao J, Li J, Ma F, et al. A novel water-soluble β-D-glucan isolated from the spores of Ganoderma lucidum. Carbohydr Res. (2012) 353:100–5. doi: 10.1016/j.carres.2012.02.029
6. Liu T, Ma Q, Zhao L, Jia R, Zhang J, Ji C, et al. Protective effects of sporoderm-broken spores of Ganderma lucidumon growth performance, antioxidant capacity and immune function of broiler chickens exposed to low level of aflatoxin B1. Toxins. (2016) 8:278. doi: 10.3390/toxins8100278
7. Pan D, Zhang D, Wu JS, Chen C, Xu Z, Yang H, et al. Antidiabetic, antihyperlipidemic and antioxidant activities of a novel proteoglycan from Ganoderma lucidum fruiting bodies on db/db mice and the possible mechanism. PLoS ONE. (2013) 8:e68332. doi: 10.1371/journal.pone.0068332
8. Dong C, Han Q., Ganoderma lucidum (lingzhi, Ganoderma). In: Y Liu, Z Wang, J Zhang, editors, Dietary Chinese Herbs Chemistry: Pharmacology and Clinical Evidence. London: Springer (2015). p. 759–65. doi: 10.1007/978-3-211-99448-1_85
9. Association of Official Analytical Chemists. Official Methods of Analysis of AOAC International. 20th ed. Arlington, VA: AOAC (2006). p. 223.
10. Nielsen SS. Phenol-sulfuric acid method for total carbohydrates. In: Food Analysis Laboratory Manual; Food Science Texts Series. New York, NY: Springer (2003). p. 39–44. doi: 10.1007/978-1-4757-5250-2_6
11. Abdelqader A, Al-Fataftah AR. Effect of dietary butyric acid on performance, intestinal morphology, microflora composition and intestinal recovery of heat-stressed broilers. Livest Sci. (2016) 183:78–83. doi: 10.1016/j.livsci.2015.11.026
12. Zhao J, Liu P, Huang C, Liu L, Li EK, Zhang G, et al. Effect of wheat bran on apparent total tract digestibility, growth performance, fecal microbiota and their metabolites in growing pigs. Anim Feed Sci Technol. (2018) 239:14–26. doi: 10.1016/j.anifeedsci.2018.02.013
13. Ciobanu DC, Bastiaansen JWM, Lonergan SM, Thomsen H, Dekkers JCM, Plastow GS, et al. New alleles in calpastatin gene are associated with meat quality traits in pigs. J Anim Sci. (2004) 82:2829–39. doi: 10.2527/2004.82102829x
14. Liu T, Zhou J, Li W, Rong X, Gao Y, Zhao L, et al. Effects of sporoderm-broken spores of Ganoderma lucidum on growth performance, antioxidant function and immune response of broilers. Anim Nutr. (2020) 6:39–46. doi: 10.1016/j.aninu.2019.11.005
15. Li K, Yu M, Hu Y, Ren G, Zang T, Xu X, et al. Three kinds of Ganoderma lucidum polysaccharides attenuate DDC-induced chronic pancreatitis in mice. Chem Biol Interact. (2016) 247:30–8. doi: 10.1016/j.cbi.2016.01.013
16. Wang F, Zhou Z, Ren X, Wang Y, Yang R, Luo J, et al. Effect of Ganoderma lucidum spores intervention on glucose and lipid metabolism gene expression profiles in type 2 diabetic rats. Lipids Health Dis. (2015) 14:1–9. doi: 10.1186/s12944-015-0045-y
17. Sadeghi A, Toghyani M, Gheisari A. Effect of various fiber types and choice feeding of fiber on performance, gut development, humoral immunity, and fiber preference in broiler chicks. Poult Sci. (2015) 94:2734–43. doi: 10.3382/ps/pev292
18. Tejeda OJ, Kim WK. Effects of fiber type, particle size, and inclusion level on the growth performance, digestive organ growth, intestinal morphology, intestinal viscosity, and gene expression of broilers. Poult Sci. (2021) 100:101397. doi: 10.1016/j.psj.2021.101397
19. Li L, Xu J-X, Cao Y-J, Lin Y-C, Guo W-L, Liu J-Y, et al. Preparation of Ganoderma lucidum polysaccharide-chromium (III) complex and its hypoglycemic and hypolipidemic activities in high-fat and high-fructose diet-induced pre-diabetic mice. Int J Biol Macromol. (2019) 140:782–93. doi: 10.1016/j.ijbiomac.2019.08.072
20. Cör D, Knez Ž, Knez Hrnčič M. Antitumour, antimicrobial, antioxidant and antiacetylcholinesterase effect of Ganoderma Lucidum terpenoids and polysaccharides: A review. Molecules. (2018) 23:649. doi: 10.3390/molecules23030649
21. Smina TP, De S, Devasagayam TPA, Adhikari S, Janardhanan KK. Ganoderma lucidum total triterpenes prevent radiation-induced DNA damage and apoptosis in splenic lymphocytes in vitro. Mutat Res. (2011) 726:188–94. doi: 10.1016/j.mrgentox.2011.09.005
22. Zhang Y, Feng Y, Wang W, Jia L, Zhang J. Characterization and hepatoprotections of Ganoderma lucidum polysaccharides against multiple organ dysfunction syndrome in mice. Oxid Med Cell Longev. (2021) 2021:9703682. doi: 10.1155/2021/9703682
23. Zhu KX, Nie SP, Tan LH, Li C, Gong DM, Xie MY. A Polysaccharide from Ganoderma atrum improves liver function in type 2 diabetic rats via antioxidant action and short-chain fatty acids excretion. J Agric Food Chem. (2016) 64:1938–44. doi: 10.1021/acs.jafc.5b06103
24. Xie J, Liu Y, Chen B, Zhang G, Ou S, Luo J, et al. Ganoderma lucidum polysaccharide improves rat DSS-induced colitis by altering cecal microbiota and gene expression of colonic epithelial cells. Food Nutr Res. (2019) 12:63. doi: 10.29219/fnr.v63.1559
25. Gonzalez-Rivas PA, Chauhan SS, Ha M, Fegan N, Dunshea FR, Warner RD. Effects of heat stress on animal physiology, metabolism, and meat quality: A review. Meat Sci. (2020) 162:108025. doi: 10.1016/j.meatsci.2019.108025
26. Laack R. Determinants of ultimate pH of meat and poultry. In: 53rd Annual Reciprocal Meat Conference. Missouri, MI: American Meat Science Association (2001). p. 74–5.
27. Jandhyala SM, Talukdar R, Subramanyam C, Vuyyuru H, Sasikala M, Reddy DN. Role of the normal gut microbiota. World J Gastroenterol. (2015) 21:8787–803. doi: 10.3748/wjg.v21.i29.8787
28. Fargione JE, Tilman D. Diversity decreases invasion via both sampling and complementarity effects: Diversity causes invader underyielding. Ecol Lett. (2005) 8:604–11. doi: 10.1111/j.1461-0248.2005.00753.x
29. Karkman A, Lehtimaki J, Ruokolainen L. The ecology of human microbiota: Dynamics and diversity in health and disease. Ann N Y Acad Sci. (2017) 1399:78–92. doi: 10.1111/nyas.13326
30. Zhou Q, Lan F, Li X, Yan W, Sun C, Li J, et al. The spatial and temporal characterization of gut microbiota in broilers. Front Vet Sci. (2021) 8:712226. doi: 10.3389/fvets.2021.712226
31. Krajmalnik-Brown R, Ilhan Z-E, Kang D-W, DiBaise JK. Effects of gut microbes on nutrient absorption and energy regulation. Nutr Clin Pract. (2012) 27:201–14. doi: 10.1177/0884533611436116
32. Chang CJ, Lin CS, Lu CC, Martel J, Ko YF, Ojcius DM, et al. Ganoderma lucidum reduces obesity in mice by modulating the composition of the gut microbiota. Nat Commun. (2015) 6:7489. doi: 10.1038/ncomms8489
33. Kursa O, Tomczyk G, Sawicka-Durkalec A, Giza A, Słomiany-Szwarc M. Bacterial communities of the upper respiratory tract of turkeys. Sci Rep. (2021) 11:2544. doi: 10.1038/s41598-021-81984-0
34. Wang Y, Ames NP, Tun HM, Tosh SM, Jones PJ, Khafipour E. High molecular weight barley β-glucan alters gut microbiota toward reduced cardiovascular disease risk. Front Microbiol. (2016) 7:129. doi: 10.3389/fmicb.2016.00129
35. Kollarcikova M, Faldynova M, Matiasovicova J, Jahodarova E, Kubasova T, Seidlerova Z, et al. Different bacteroides species colonise human and chicken intestinal tract. Microorganisms. (2020) 8:1483. doi: 10.3390/microorganisms8101483
36. Li K, Zhuo C, Teng C, Yu S, Wang X, Hu Y, et al. Effects of Ganoderma lucidum polysaccharides on chronic pancreatitis and intestinal microbiota in mice. Int J Biol Macromol. (2016) 93:904–12. doi: 10.1016/j.ijbiomac.2016.09.029
37. Duncan SH, Louis P, Flint HJ. Cultivable bacterial diversity from the human colon. Lett Appl Microbiol. (2007) 44:343–50. doi: 10.1111/j.1472-765X.2007.02129.x
38. Vince AJ, McNeil NI, Wager JD, Wrong OM. The effect of lactulose, pectin, arabinogalactan and cellulose on the production of organic acids and metabolism of ammonia by intestinal bacteria in a faecal incubation system. Br J Nutr. (1990) 63:17–26. doi: 10.1079/BJN19900088
39. Luo J, Li Y, Xie J, Gao L, Liu L, Ou S, et al. The primary biological network of Bifidobacterium in the gut. FEMS Microbiol Lett. (2018) 365:fny057. doi: 10.1093/femsle/fny057
40. Yao C, Wang Z, Jiang H, Yan R, Huang Q, Wang Y, et al. Ganoderma lucidum promotes sleep through a gut microbiota-dependent and serotonin-involved pathway in mice. Sci Rep. (2021) 11:13660. doi: 10.1038/s41598-021-92913-6
41. Zheng M, Pi X, Li H, Cheng S, Su Y, Zhang Y, et al. Ganoderma spp. polysaccharides are potential prebiotics: A review. Crit Rev Food Sci Nutr. (2022) 18:1–19. doi: 10.1080/10408398.2022.2110035
42. Li YJ, Chen X, Kwan TK, Loh YW, Singer J, Liu Y, et al. Dietary fiber protects against diabetic nephropathy through short-chain fatty acid-mediated activation of G protein-coupled receptors GPR43 and GPR109A. J Am Soc Nephrol. (2020) 31:1267–81. doi: 10.1681/ASN.2019101029
43. Jiang W, Zhu H, Liu C, Hu B, Guo Y, Cheng Y, et al. In-depth investigation of the mechanisms of Echinacea purpurea polysaccharide mitigating alcoholic liver injury in mice via gut microbiota informatics and liver metabolomics. Int J Biol Macromol. (2022) 209:1327–38. doi: 10.1016/j.ijbiomac.2022.04.131
44. Kasai T, Hirakuri Y, Sakamura S. Aspartyl and glutamyl peptides and the acidic cysteine derivatives in Asparagus (Asparagus officinalis) shoots. Agric Biol Chem. (1981) 45:433–7. doi: 10.1271/bbb1961.45.433
45. Bogan KL, Brenner C. Nicotinic acid, nicotinamide, and nicotinamide riboside: A molecular evaluation of NAD+ precursor vitamins in human nutrition. Annu Rev Nutr. (2008) 28:115–30. doi: 10.1146/annurev.nutr.28.061807.155443
46. Houstis N, Rosen ED, Lander ES. Reactive oxygen species have a causal role in multiple forms of insulin resistance. Nature. (2006) 440:944–8. doi: 10.1038/nature04634
47. Dooley DM. Structure and biogenesis of topaquinone and related cofactors. J Biol Inorg Chem. (1999) 4:1–11. doi: 10.1007/s007750050283
48. Carotenutoa A, Fattorusso E, Lanzottib V, Magno S. Porric acids A-C-new antifungal dibenzofurans from the Bulbs of Allium porrum L. Eur J Org Chem. (1998) 4:661–3. doi: 10.1002/(SICI)1099-0690(199804)1998:4<661::AID-EJOC661>3.0.CO;2-V
49. Pérez-García F, Brito LF, Wendisch VF. Function of L-pipecolic acid as compatible solute in Corynebacterium glutamicum as basis for its production under hyperosmolar conditions. Front Microbiol. (2019) 10:340. doi: 10.3389/fmicb.2019.00340
50. Claus SP, Ellero SL, Berger B, Krause L, Bruttin A, Molina J, et al. Colonization-induced host-gut microbial metabolic interaction. MBio. (2011) 2:271–10. doi: 10.1128/mBio.00271-10
51. Martínez I, Perdicaro DJ, Brown AW, Hammons S, Carden TJ, Carr TP, et al. Diet-induced alterations of host cholesterol metabolism are likely to affect the gut microbiota composition in hamsters. Appl Environ Microbiol. (2013) 79:516–24. doi: 10.1128/AEM.03046-12
52. Yi Y, Wang H, Chen Y, Gou M, Xia Z, Hu B, et al. Identification of novel butyrate- and acetate-oxidizing bacteria in butyrate-fed mesophilic anaerobic chemostats by DNA-based stable isotope probing. Microb Ecol. (2020) 79:285–98. doi: 10.1007/s00248-019-01400-z
53. Ahrens AP, Culpepper T, Saldivar B, Anton S, Stoll S, Handberg EM, et al. A Six-Day, Lifestyle-based immersion program mitigates cardiovascular risk factors and induces shifts in gut microbiota, specifically Lachnospiraceae, Ruminococcaceae, Faecalibacterium prausnitzii: A pilot study. Nutrients. (2021) 13:3459. doi: 10.3390/nu13103459
54. Tavella T, Rampelli S, Guidarelli G, Bazzocchi A, Gasperini C, Pujos-Guillot E, et al. Elevated gut microbiome abundance of Christensenellaceae, Porphyromonadaceae and Rikenellaceae is associated with reduced visceral adipose tissue and healthier metabolic profile in Italian elderly. Gut Microbes. (2021) 13:1–19. doi: 10.1080/19490976.2021.1880221
Keywords: Ganoderma lingzhi culture, Sanhuang broilers, fermented feeds, intestinal bacteria, metabolomics
Citation: Liu X, Huang L, Shi Y, Wang X, Luo Y, Wei S, Qin Y, Lu Y, Zhang W, Ju Y, Yan Y and Liao Y (2023) Ganoderma lingzhi culture enhance growth performance via improvement of antioxidant activity and gut probiotic proliferation in Sanhuang broilers. Front. Vet. Sci. 10:1143649. doi: 10.3389/fvets.2023.1143649
Received: 13 January 2023; Accepted: 27 March 2023;
Published: 17 April 2023.
Edited by:
Domenico Pietro Lo Fiego, University of Modena and Reggio Emilia, ItalyReviewed by:
Giacomo Biagi, University of Bologna, ItalyMonika Proszkowiec-Weglarz, Agricultural Research Service (USDA), United States
Copyright © 2023 Liu, Huang, Shi, Wang, Luo, Wei, Qin, Lu, Zhang, Ju, Yan and Liao. This is an open-access article distributed under the terms of the Creative Commons Attribution License (CC BY). The use, distribution or reproduction in other forums is permitted, provided the original author(s) and the copyright owner(s) are credited and that the original publication in this journal is cited, in accordance with accepted academic practice. No use, distribution or reproduction is permitted which does not comply with these terms.
*Correspondence: Yong Yan, aW5mdW5naUAxMjYuY29t; Yuying Liao, MzE1OTUxNjEwQHFxLmNvbQ==
†These authors have contributed equally to this work
‡ORCID: Xuzhou Liu orcid.org/0000-0002-3412-3303