- Laboratory of Veterinary Internal Medicine, Department of Clinical Veterinary Science, College of Veterinary Medicine, Seoul National University, Seoul, Republic of Korea
Background: Mesenchymal stem cells (MSCs) are useful agents in the treatment of various inflammatory diseases. The immunomodulatory effects of MSCs are largely related to their secretory properties. mRNA engineering emerged as a safe alternative to enhance the secretory function of MSCs. Optimization of the untranslated region (UTR) sequence is important for enhancing the translational efficiency of exogenous mRNAs. However, research on the optimization of UTR in canine MSCs has not yet been conducted.
Objectives: We aimed to identify the UTR sequence related to the expression efficiency of in vitro transcription (IVT) mRNA in canine MSCs and investigate whether mRNA-engineered MSCs that overexpress TSG-6 exhibit enhanced anti-inflammatory effects.
Methods: Canine adipose tissue-derived (cAT)-MSCs were transfected with green fluorescence protein (GFP) mRNA with three different UTRs: canine hemoglobin subunit alpha-like 1 (HBA1), HBA2, and hemoglobin subunit beta-like (HBB). The translation efficacy of each mRNA was evaluated using relative fluorescence. TSG-6 mRNA was produced with the UTR optimized according to relative fluorescence results. cAT-MSCs were transfected with TSG-6 mRNA (MSCTSG-6), and TSG-6 expression was analyzed using real-time quantitative PCR, ELISA, and western blotting. To evaluate the anti-inflammatory effects of MSCsTSG-6, DH82 cells were co-cultured with MSCsTSG-6 or treated with dexamethasone, and changes in the expression of inflammatory cytokines were analyzed using qPCR.
Results: The highest fluorescence level was observed in the HBA1 UTR at 24 h post-transfection. TSG-6 mRNA transfection yielded high levels of TSG-6 in the cAT-MSCs. In DH82 cells co-cultured with MSCsTSG-6, the expression of inflammatory cytokines decreased compared to that in co-culturing with naïve MSCs and dexamethasone treatment.
Conclusions: Optimization of the HBA1 UTR improved the translation efficiency of IVT mRNA in canine MSCs. cAT-MSCs engineered with TSG-6 mRNA effectively enhanced the anti-inflammatory effects of the MSCs when co-cultured with LPS-activated DH82 cells.
1. Introduction
mRNA is a promising nucleic acid-based therapeutic agent with a wide range of applications, including vaccination and protein replacement therapies (1). Unlike DNA therapeutics, mRNA does not require nuclear entry because translation occurs in the cytoplasm and presents no risk of insertional mutagenesis because it does not integrate into the genome (2). mRNA is degraded via normal physiological pathways, resulting in low toxicity. Moreover, the production of mRNA through in vitro transcription (IVT) is rapid and inexpensive; therefore, mRNA-based therapeutics are considered a promising new drug class (3).
Despite these advantages, the application of exogenous mRNA is limited by instability and a short half-life. Modification of the structural elements of mRNA could resolve these issues, one approach being the optimization of the mRNA untranslated region (UTR) sequence (4). There are several regions in mRNA that affect translation efficacy and degradation by nuclease, including the 5′ cap, 5′- and 3′-UTRs, open reading frame (ORF), and poly (A) tail. Among these, 5′- and 3′-UTRs promote mRNA stability and translation efficacy (5). Several studies have shown that mRNA stability can be improved by modifying the UTR sequence of proteins: modification with human α- and β-globin UTR sequences promoted the amount and duration of protein production (6–8). mRNA-based therapeutics have since gained widespread acceptance, from vaccine and protein replacement therapies to cancer immunotherapy and stem cell genome engineering (9).
Because of their ability to modulate inflammatory responses, mesenchymal stem cells (MSCs) are promising treatment agents in various inflammatory diseases, such as inflammatory bowel disease (10), pancreatitis (11), rheumatoid arthritis (12), and peritonitis (13). MSCs exert their anti-inflammatory effects by secreting various paracrine soluble factors, among which tumor necrosis factor (TNF)-α-induced gene/protein 6 (TSG-6) is a major factor regulating inflammation in pancreatitis (14), lung injury (15, 16), inflammatory bowel disease (17), myocardial infarction (18), corneal injury (19), and skin wounds (20). One of the mechanisms underlying the immunomodulation of TSG-6 is its effect on macrophage polarization. Macrophages in the inflammatory context can be classified into two subsets: M1 and M2. Activated M1 macrophages promote inflammation by secreting inflammatory cytokines, such as interleukin (IL)-1β and TNF-α, whereas M2 macrophages secrete cytokines, such as IL-10, inducing an anti-inflammatory response (21). Recent studies have shown that MSCs alleviate inflammation by switching macrophages from the M1 to M2 phenotype via TSG-6 secretion (22, 23).
The clinical application of MSC therapies is limited by their insufficient secretion capacity. One solution is to genetically modify MSCs to overexpress specific therapeutic factors. Although viral-based gene delivery is commonly used, its clinical application is limited by safety concerns, such as genome insertion; therefore, mRNA transfection systems have emerged as a safe and effective alternative (24). MSCs engineered with mRNA to overexpress therapeutic factors, such as insulin growth factor-1 (IGF-1) and IL-10, showed enhanced anti-inflammatory action in osteoarthritis, acute graft-vs.-host disease, and traumatic brain injury models (25–27). Yang et al. (28) also improved the therapeutic effect of MSCs in a rat periodontitis model by overexpressing TSG-6 through viral-based engineering. However, application of an mRNA transfection system to increase TSG-6 expression in MSCs has not yet been reported for any animal model. Moreover, given the lack of research on UTR sequences for efficient mRNA expression in animal cell lines, including dogs, studies on mRNA-based therapeutics in veterinary medicine are urgently needed.
In this study, we aimed to (1) identify the UTR sequence regulating the expression efficiency of IVT mRNA in canine cell lines and (2) investigate whether mRNA-engineered MSCs that overexpress TSG-6 exhibit enhanced anti-inflammatory effects.
2. Materials and methods
2.1. DNA template design
The template of IVT mRNA consisted of the T7 promoter (5′-TAATACGACTCACTATAGGG-3′), 5′-UTR, ORF, and 3′-UTR. Three canine globin 5′- and 3′-UTRs were used to identify the UTR sequence that most effectively stabilized mRNA: canine hemoglobin subunit alpha-like 1 (HBA1), HBA2, and hemoglobin subunit beta-like (HBB). The ORF was designed to encode green fluorescence protein (GFP) to evaluate expression efficiency with relative fluorescence, and a stop codon was placed downstream of the GFP-encoding sequence.
To produce TSG-6 mRNA, the 5′- and 3′-UTRs of HBA1 were used based on the relative fluorescence results. The designed DNA templates were synthesized using the Gene in Fragment form synthesis service provided by BIONICS (Korea). All the UTRs and ORF sequences were obtained from the National Center for Biotechnology Information (NCBI, USA).
2.2. In vitro transcription
Fragment DNA (0.2 μg) was used as a template for IVT. mRNA was produced using an mMESSAGE mMACHINE T7 ULTRA Transcription Kit (Life Technologies, Carlsbad, CA, USA) according to the manufacturer's instructions. Briefly, linear template DNA was transcribed with T7 enzyme and 5′ capped with Anti-Reverse Cap Analog (ARCA) during transcription; DNase I treatment and poly (A) tailing were then performed. The synthesized mRNA was purified using the lithium chloride (LiCl) precipitation method and quantified using a Nanophotometer Pearl (Implen, München, Germany).
2.3. Cell culture
Canine adipose tissue-derived (cAT)-MSCs were cultured in Dulbecco's modified Eagle's medium (DMEM; Welgene, Gyeongsan-si, Korea), supplemented with 10% fetal bovine serum (FBS; Gibco, Billings, MT, USA) and 1% penicillin/streptomycin (PS; PAN-Biotech, Aidenbach, Germany). DH82, a canine macrophage cell line, was purchased from the American Type Culture Collection (ATCC number: CRL-10389; Manassas, VA, USA) and maintained in DMEM with 15% FBS and 1% PS. All cells were incubated at 37°C and 5% CO2. The medium was changed every 2–3 days, and the cells were subcultured when they reached 70–80% confluence. cAT-MSCs from the third passage were used for all subsequent experiments.
2.4. Isolation and characterization of cAT-MSCs
Canine adipose tissues were obtained from three healthy adult female dogs undergoing ovariohysterectomy at the Seoul National University Veterinary Medical Teaching Hospital (SNU VMTH) under a protocol approved by the Institutional Animal Care and Use Committee (IACUC) of SNU (protocol no. SNU-220818-1). MSC isolation and culture were performed as previously described (29). Adipose tissue samples were washed with Dulbecco's phosphate-buffered saline (DPBS; Welgene) supplemented with 1% PS, disintegrated using sterilized scissors, treated with collagenase type IA (0.1%; Gibco), and incubated at 37°C for 1 h. After incubation, tissue sample neutralization was performed using DMEM supplemented with 10% FBS. The neutralized samples were centrifuged at 1,200 × g for 5 min, and the pellet was passed through a 70-μm cell strainer (Thermo Fisher Scientific, Waltham, MA, USA) and again centrifuged at 1,200 × g for 10 min. To remove the remaining red blood cells, the pellet was resuspended in RBC lysis buffer (Sigma-Aldrich, St. Louis, MO, USA), incubated at room temperature for 5 min, washed with DPBS, and centrifuged at 1,200 × g for 5 min. After removing the supernatant, the pellets were resuspended in DMEM with 10% FBS and 1% PS and seeded at a density of 3,000 cells/cm2 in 100 mm cell culture dishes. Cells were maintained at 37°C in 5% CO2 and the medium was replaced every 2–3 days. Subculture was performed when cells exhibiting fibroblast-like morphology reached 70–80% confluence.
To assess their differentiation capacities, cAT-MSCs were cultured using three differentiation media (StemPro Adipogenesis Differentiation, Stem Pro Osteogenesis Differentiation, and StemPro Chondrogenesis Differentiation kits; Gibco). Differentiation into adipocytes, osteocytes, and chondrocytes was identified by staining the cells with Oil Red O, 1% alizarin red, and alcian blue (Sigma-Aldrich), respectively. Additionally, the expression of specific surface markers was evaluated with flow cytometry using FACS Aria II system (BD Biosciences). Cells were stained with monoclonal antibodies against CD34-PE conjugated, CD45-FITC conjugated, CD29-FITC conjugated, and CD90-PE conjugated (BD Biosciences, San Jose, CA, USA), and the resulting fluorescence was analyzed using Flowjo software (Tree Star, Woodburn, OR, USA).
2.5. mRNA transfection in cAT-MSCs and effective UTR selection
We compared the translation efficacy of the GFP mRNAs with the UTRs HBA1, HBA2, and HBB in transfected cAT-MSCs. Cells (1 × 105) were seeded in confocal dishes and transfected with 0.5 μg of each mRNA for 24 h with Lipofectamine MessengerMAX Reagent (Thermo Fisher Scientific) and Opti-MEM I Reduced Serum Medium (Gibco) according to the manufacturers' instructions. The fluorescence levels of transfected cells were measured using a confocal laser scanning microscope (LSM710; Zeiss, Germany) at 6, 12, 24, 36, 48, 60, 72, 84, and 96 h post-transfection. At each time point, three images per confocal dish were randomly acquired. The relative fluorescence was calculated using ImageJ software (National Institutes of Health, Bethesda, MD, USA). Briefly, we evaluated the area and intensity of green fluorescence using the ImageJ as the cells were evenly seeded. The fluorescence area was relatively calculated by assuming the total area as 1.
2.6. RNA extraction, cDNA synthesis, and real-time quantitative PCR (qPCR)
Total RNA of TSG-6 mRNA-transfected cAT-MSCs and co-cultured DH82 cells was extracted using an Easy-Blue total RNA extraction kit (iNtRON Biotechnology, Seongnam, Korea). The concentration and the purity of samples were measured using a Nanophotometer Pearl (Implen). CellScript All-in-One 5 × 1st cDNA Strand Synthesis Master Mix (CellSafe, Bucheon-si, Korea) was used for cDNA synthesis. qPCR was conducted in duplicate using AMPIGENE qPCR Green Mix Hi-Rox with SYBR green dye (Enzo Life Sciences, Farmingdale, NY, USA), 400 nM forward and reverse primers, and 1 μL of cDNA. Thermocycler settings were 95°C for 2 min, followed by 40 cycles at 95°C for 5 s and 60°C for 25 s. Expression levels were analyzed using the 2−ΔΔ/Cts method and normalized to that of Glyceraldehyde 3-phosphate dehydrogenase (GAPDH). Primer sequences used in this study are listed in Table 1.
2.7. Protein extraction and western blot analysis
Total protein of TSG-6 mRNA-transfected cAT-MSCs was extracted using Pro-Prep protein extraction solution (iNtRON Biotechnology). The concentration of extracted protein was quantified using a DC Protein Assay Kit (Bio-Rad Laboratories, Hercules, CA, USA). We separated 20 μg of protein by SDS-PAGE, which was then transferred to polyvinylidene difluoride membranes (EMD Millipore, Burlington, MA, USA). The membranes were blocked with 5% non-fat dry milk and exposed to primary antibodies against TSG-6 (1:500, Santa Cruz Biotechnology, Santa Cruz, CA, USA) and β-actin (1:1,000, Santa Cruz Biotechnology) at 4°C for 24 h. Membranes were washed and then incubated with secondary antibodies at room temperature for 1 h. We detected the immunoreactive bands of the proteins using a chemiluminescence kit (Advansta, San Jose, CA, USA); the protein expression levels were normalized to that of β-actin.
2.8. Enzyme-linked immunosorbent assay (ELISA)
We measured TSG-6 content in the cell culture supernatant of TSG-6 mRNA-transfected cAT-MSCs using a commercial TSG-6 ELISA kit (MyBioSource, San Diego, CA, USA) according to the manufacturer's instructions.
2.9. Co-culture with DH82 and TSG-6 mRNA transfected cAT-MSCs (MSCTSG−6)
cAT-MSCs (4 × 105) were seeded onto 0.4 μM pore-sized Transwell inserts (SPL Life Sciences, Pocheon-si, Korea) and transfected with 2.5 μg TSG-6 mRNA per well for 24 h (or without, as a control), using Lipofectamine MessengerMAX Reagent and Opti-MEM I Reduced Serum Medium. After 24 h, the inserts were washed twice with DPBS and the medium was changed to DMEM supplemented with 10% FBS and 1% PS.
DH82 cells were seeded in 6-well plates at a density of 1 × 106 cells/well and incubated with or without 200 ng/mL lipopolysaccharide (LPS; Sigma-Aldrich) for 24 h. After changing the medium to DMEM with 10% FBS and 1% PS, DH82 macrophages were co-cultured with TSG-6 mRNA-transfected cAT-MSCs, naïve cAT-MSCs, or treated with 10 μM dexamethasone (a well-known anti-inflammatory drug) for 24 h. This concentration of dexamethasone (24-h treatment) was selected because it effectively reduced inflammatory cytokine (IL-1β and IL-6) expression in LPS-stimulated DH82 cells without affecting viability, based on a Cell Counting Kit-8 (CCK-8) assay (Donginbio, Seoul, Korea) and qPCR (Supplementary Figure 1).
2.10. Statistical analysis
Each experiment was conducted at least three times. Data are shown as the mean ± standard deviation. Mean values from different groups were compared using the Mann–Whitney t-test and one-way analysis of variance using GraphPad Prism v.7.01 software (GraphPad Software, La Jolla, CA, USA). Statistical significance was set at P < 0.05.
3. Results
3.1. Characterization of cAT-MSCs
We evaluated the stem cell identity of the cAT-MSCs using differentiation assays and flow cytometry. The cells were successfully differentiated into adipocytes, osteocytes, and chondrocytes (Figures 1A–C). Flow cytometry analysis showed high expression of the stem cell markers CD29 and CD90, while no expression of the hematopoietic markers CD34 and CD25 was detected (Figures 1D–G).
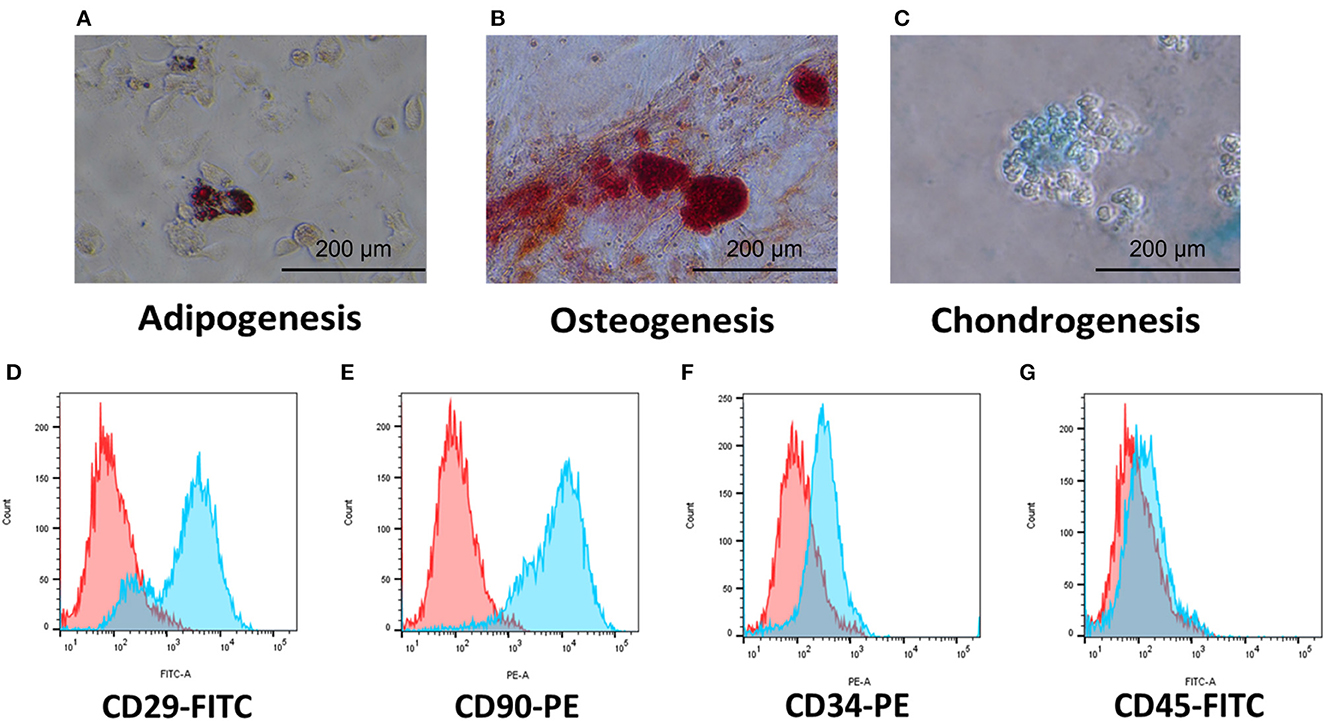
Figure 1. Characterization of cAT-MSCs isolated from canine adipose tissue. (A–C) Trilineage potential of cAT-MSCs. Differentiation into adipocyte, osteocyte, and chondrocyte was confirmed via Oil Red O, Alizarin Red, and Alcian Blue staining, respectively. (D–G) Flow cytometry analysis of cell surface markers. cAT-MSCs expressed CD29 and CD90 and lacked CD34 and CD45. (Blue: stained marker, red: unstained negative control).
3.2. Efficient UTR selection in GFP mRNA-transfected cAT-MSCs
cAT-MSCs were transfected with GFP mRNA containing three different UTRs (HBA1, HBA2, and HBB) and monitored for 96 h (Figures 2A, B). We observed significantly different fluorescence levels depending on the UTR, indicating variation in the translation efficacy of each mRNA. HBA1-, HBA2-, and HBB-GFP mRNA reached a peak fluorescence level at 24, 12, and 72 h, respectively, of which HBA1 had the highest fluorescence level and HBB had the lowest (Figure 2C). At 24 h post-transfection, HBA1 showed a significantly higher level of fluorescence than HBA2 and HBB did. HBA1 maintained a higher fluorescence level than the other two mRNAs for about 36 h. Fluorescence expression of HBA1 was observed up to 96 h post-transfection (Figure 3). Overall, HBA1 most effectively enhanced mRNA stability and translation efficacy.
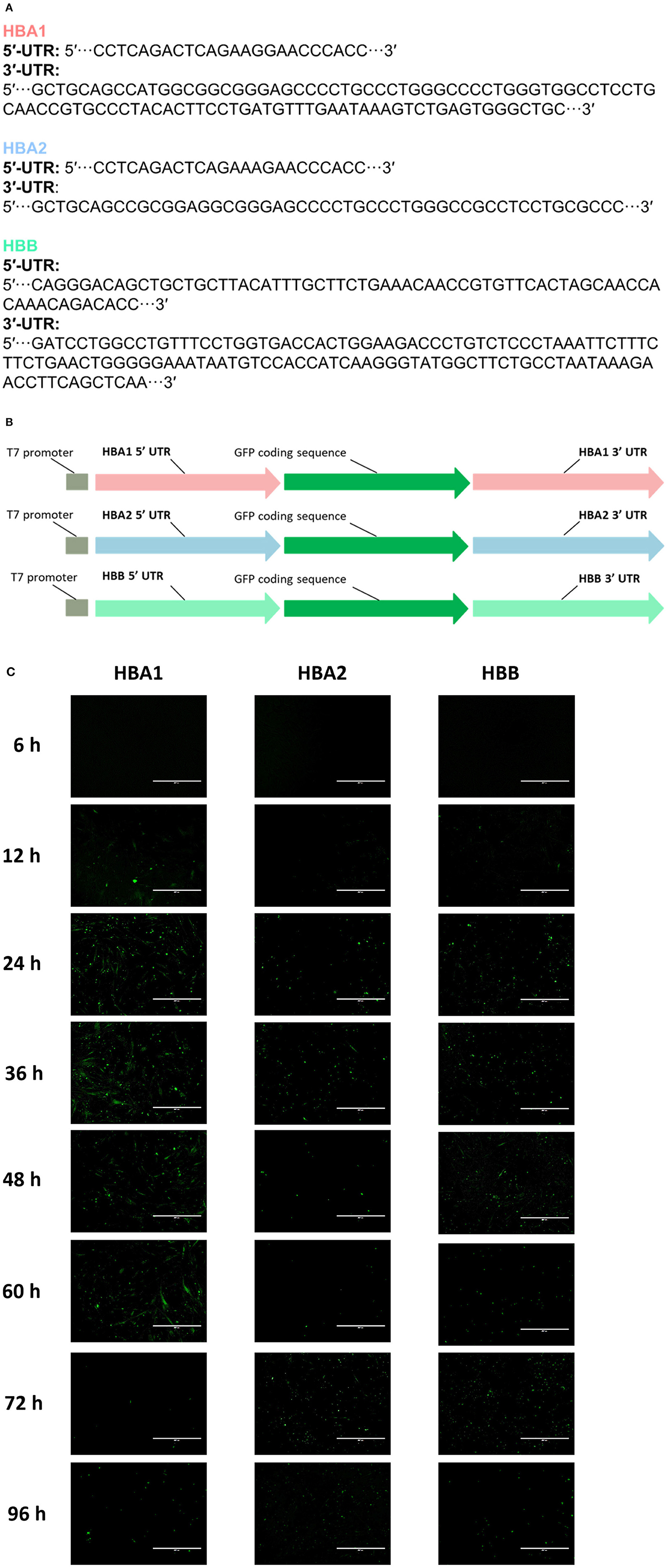
Figure 2. cAT-MSCs expressing GFP with three different UTRs. (A) Sequences of HBA1, HBA2, and HBB UTR regions. (B) Schematic structure of each template DNA, consisting of a T7 promoter, a 5′-UTR, a GFP coding region, and a 3′-UTR. (C) The highest fluorescence level was observed with HBA1 24 h post-transfection. HBA2 and HBB reached peak fluorescence levels at 12 and 72 h post-transfection, both at lower levels than those of HBA1. Fluorescence was observed up to 96 h post-transfection. Scale bar, 400 μm (HBA1: GFP mRNA with UTR of canine hemoglobin subunit alpha-like 1; HBA2: GFP mRNA with UTR of canine hemoglobin subunit alpha-like 2; HBB: GFP mRNA with UTR of canine hemoglobin subunit beta-like).
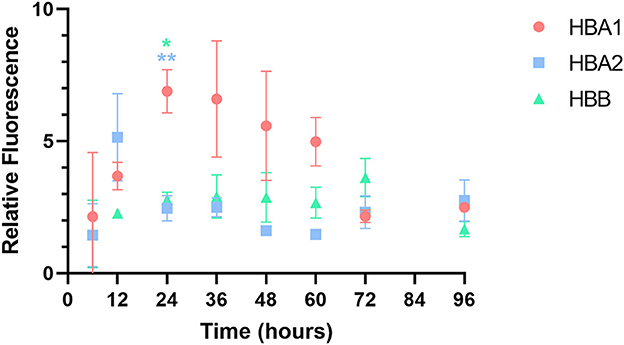
Figure 3. Relative fluorescence of each GFP mRNA over time. Among the three GFP mRNAs, HBA1-GFP mRNA showed the highest fluorescence level 24 h after transfection. Results are shown as the mean ± standard deviation. *P < 0.05, **P < 0.01 vs. HBA2 and HBB at the same time point. (HBA1: GFP mRNA with UTR of canine hemoglobin subunit alpha-like 1; HBA2: GFP mRNA with UTR of canine hemoglobin subunit alpha-like 2; HBB: GFP mRNA with UTR of canine hemoglobin subunit beta-like).
3.3. Enhancement of TSG-6 expression in cAT-MSCTSG−6
TSG-6 mRNA was produced with the HBA1 UTR and transfected into cAT-MSCs for 24 h. The coding sequence of TSG-6 mRNA is described in Supplementary Data 1. The expression of TSG-6 mRNA was more than 10,000-times higher in the MSCTSG-6 than in the MSC group, indicating the successful transfection of TSG-6 mRNA (Figure 4A, Supplementary Tables 1, 2). Western blot confirmed that the expression of TSG-6 protein was significantly higher in the MSCTSG-6 group than that in the MSC group (Figure 4B). TSG-6 ELISA also confirmed that the secretion of TSG-6 was enhanced in the MSCTSG-6 group (Figure 4C). These results demonstrate that TSG-6 mRNA transfection could produce high levels of TSG-6 in cAT-MSCs.
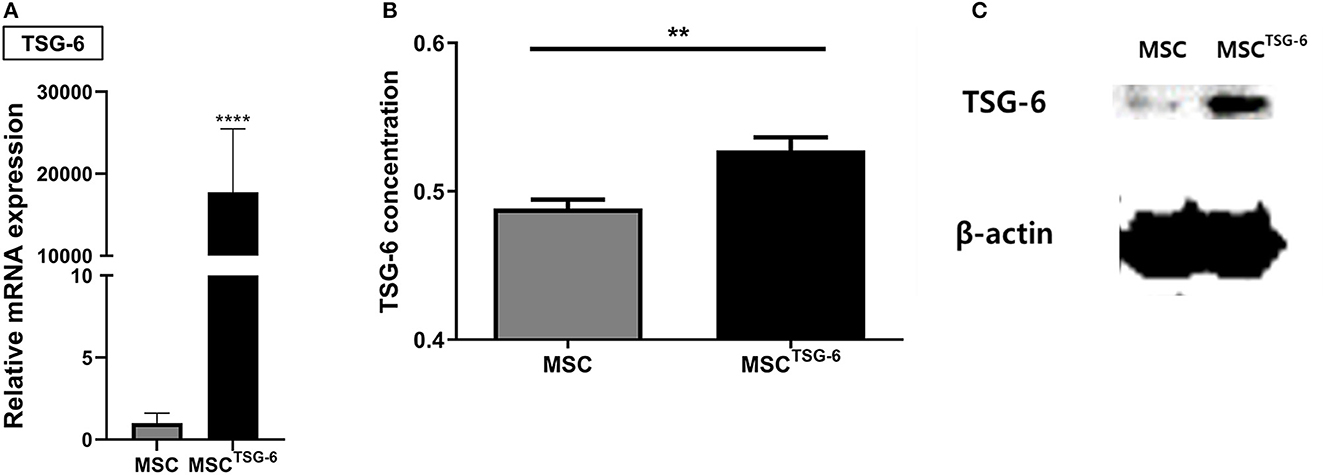
Figure 4. TSG-6 mRNA transfection increased expression of TSG-6 in cAT-MSCs. (A) TSG-6 mRNA level was increased in cAT-MSCs transfected with TSG-6 mRNA (MSCTSG-6). (B) TSG-6 protein level was increased in MSCsTSG-6. (C) Secretion of TSG-6 was also increased in MSCsTSG-6. Results are shown as the mean ± standard deviation. **P < 0.01, ****P < 0.0001.
3.4. cAT-MSCTSG−6 co-culture decreases the macrophage-related expression of inflammatory cytokines
We evaluated the immunomodulatory effect of MSCTSG-6 in the co-culture system with DH82 macrophages (Figure 5A). In LPS-stimulated DH82 cells, the mRNA expression of inflammatory cytokines demonstrating the M1 polarization significantly increased compared to the naïve group. In contrast, in DH82 cells co-cultured with MSCs or MSCsTSG-6, the expression of inflammatory cytokines was significantly decreased compared with that in the LPS-stimulated group. Compared to the MSC-co-cultured group, the mRNA expression levels of all inflammatory cytokines were significantly lower in the MSCTSG-6-co-cultured group (Figures 5B–D, Supplementary Tables 3–6).
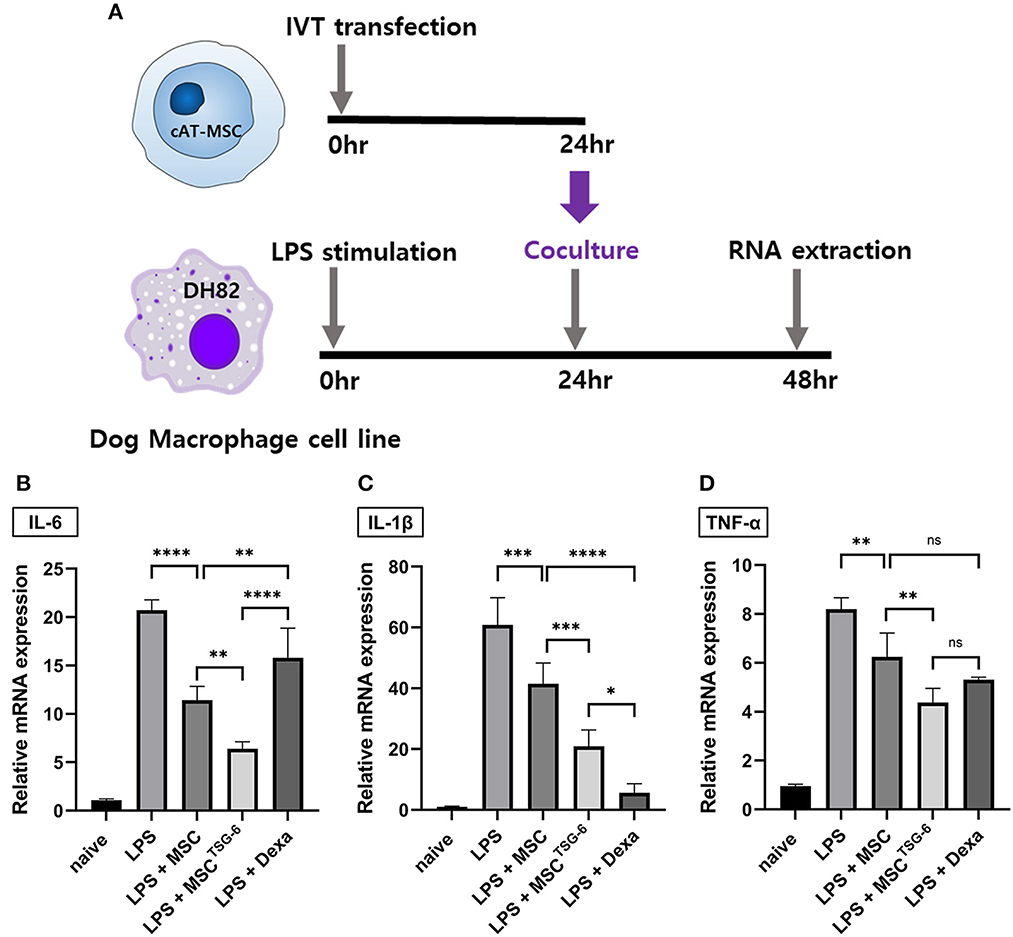
Figure 5. MSCTSG-6 co-culture altered the phenotype of DH82 macrophages. (A) Schematic diagram of co-culture with DH82 cells and MSCsTSG-6. (B–D) LPS treatment-induced M1 polarization of DH82 cells. IL-6, IL-1β, and TNF-α were used as M1 phase markers. The mRNA expression of these markers increased significantly in LPS-stimulated DH82 cells. Co-culture of these cells with naïve MSCs or MSCsTSG-6 reduced the mRNA level of M1 markers. MSCsTSG-6 more effectively reduced the expression of M1 markers than naïve MSCs did. (B) MSCsTSG-6 more effectively reduced IL-6 expression than dexamethasone did. (C) mRNA level of IL-1β showed a greater decrease with dexamethasone than MSCsTSG-6. (D) MSCsTSG-6 and dexamethasone reduced TNF-α mRNA expression to a comparable degree. Results are shown as the mean ± standard deviation obtained in three independent experiments. *P < 0.05, **P < 0.01, ***P < 0.001, ****P < 0.0001. ns, not significant.
The anti-inflammatory effect of MSCTSG-6 was compared with that of dexamethasone. We compared the expression levels of inflammatory cytokines between LPS-stimulated DH82 cells treated with 10 μM dexamethasone to those co-cultured with MSCs or MSCsTSG-6. The concentration of IL-6 was higher in the dexamethasone-treated group than in the MSC- and MSCTSG-6-co-cultured groups (Figure 5B). In contrast, the mRNA expression level of IL-1β was lower in the dexamethasone-treated group than in the MSC- and MSCTSG-6-co-cultured groups (Figure 5C). TNF-α mRNA expression levels did not vary between groups (Figure 5D).
4. Discussion
Through their secretion of immunomodulatory factors, such as TSG-6, MSCs show promising therapeutic effects against various inflammatory diseases (32). However, challenges related to the survival and secretion efficacy of MSCs have hindered their clinical application. Varied strategies, such as biochemical priming and genetic engineering, have been applied to enhance the secretory functions of MSCs (33). In this study, we investigated whether the immunomodulatory effects of canine MSCs can be enhanced by TSG-6 mRNA transfection.
First, we generated three different GFP-encoding mRNAs using the UTRs of canine HBA1, HBA2, and HBB, and evaluated their expression efficacy to identify the UTR optimized for canine MSCs. The highest peak fluorescence intensity level was observed with HBA1-GFP mRNA, and its relative fluorescence intensity was higher than that for HBA2- and HBB-GFP for ~36 h. Fluorescence expression of HBA1-GFP mRNA was observed up to 96 h post-transfection. Given that the half-life of wild-type GFP is 26 h (34), our findings indicated that UTR optimization increased mRNA stability.
This is the first study to attempt UTR optimization to improve IVT mRNA translation efficiency in canine MSCs. Holtkamp et al. (35) demonstrated that the effect of the UTR sequence on mRNA translation efficiency depends on the cell type. This indicates that UTR optimization should be designed according to the target cells. In human cells, the UTR of globin, with a half-life of 16–48 h, is commonly used for UTR optimization to increase mRNA stability (36). The mechanism of mRNA stabilization is related to the low G-C content (~44%) of the 5′-UTR of human β-globin, which reduces the likelihood that a secondary structure will be formed and inhibit protein translation (37). In addition, the pyrimidine-rich element (PRE) in the 3′-UTR of human β-globin is a recognition site for RNA-binding proteins that regulate mRNA stability (38). In the present study, the canine HBA1 UTR improved the stability of mRNA and expression of TSG-6. Although further studies are needed to determine whether canine globin UTR enhances mRNA stability by the same mechanism as human globin, our findings can inform the design of suitable templates to increase the translation efficiency of IVT mRNA in canine MSCs.
Pro-inflammatory cytokine treatment and viral engineering have also been used to modify the secretion of anti-inflammatory factors by MSCs. Several studies have demonstrated that priming MSCs with pro-inflammatory cytokines, such as TNF-α and IFN-γ, effectively increases their immunosuppressive properties (39). However, preconditioning with pro-inflammatory cytokines carries a risk of toxicity, even at low concentrations (40). In addition, priming MSCs with pro-inflammatory cytokines induces non-specific increase in the secretion of overall factors. Depending on the disease context, some factors secreted may be helpful, but some factors may also be detrimental (39). Viral engineering of MSCs can induce expression more accurately and stably than other preconditioning methods but harbors the risk of genetic mutation and tumorigenicity (41). In contrast, engineering MSCs with mRNA only induces overexpression of specific therapeutic factor and does not have any risk of genetic mutation. Therefore, our study presents a reduced-risk genetic engineering method to effectively modulate TSG-6 secretion and anti-inflammatory effects.
We produced TSG-6-overexpressing canine MSCs by transfecting cAT-MSCs with TSG-6 mRNA and found that, compared to naïve MSCs, the MSCsTSG-6 caused a greater reduction in inflammatory cytokine expression in LPS-stimulated DH82 cells. We also compared the anti-inflammatory effects of MSCTSG-6 with those of dexamethasone. Although 10 μM dexamethasone was a higher concentration than that reported to effectively lower the expression of inflammatory cytokines in macrophage cells (42, 43), the degree of decrease in the expression of IL-6 and TNF-α in the MSCTSG-6 co-culture group was higher or similar to that with 10 μM dexamethasone. These results indicate that TSG-6, induced by MSC mRNA engineering, has an anti-inflammatory effect comparable to that of dexamethasone, which is consistent with the findings of previous studies of topically administered TSG-6 vs. systemic dexamethasone (44, 45). Dexamethasone is a potent anti-inflammatory and immunosuppressive drug that is widely used in the treatment of various inflammatory and autoimmune diseases, but its long-term use is limited due to its systemic side effects on endocrine and metabolic functions (46). However, MSC engineering through mRNA transfection induces relatively transient target protein overexpression, so the risk of long-term side effects is low (26). In this respect, our results that MSCTSG-6 showed a similar anti-inflammatory effect to dexamethasone could be noteworthy. For future clinical application, evaluating the long-term therapeutic efficacy and side effects of dexamethasone and MSCTSG-6 in various inflammatory disease models is necessary.
We acknowledge the limitations of our study. First, UTR optimization was performed using the ORF of GFP. It is not clear whether this optimized UTR can be applied to other ORFs, as different ORFs can affect the secondary structure and the resulting translation efficiency of mRNA (4). In addition, an in vivo study to evaluate the therapeutic efficacy of TSG-6 mRNA-engineered MSC in inflammatory disorders is needed. Nevertheless, our study is the first to successfully optimize the UTR of canine MSCs and genetically engineer canine MSCs via mRNA transfection. Our study provides an empirical basis for mRNA-based therapeutics, including MSC engineering, in veterinary medicine, and our framework could be applied to designing IVT mRNA-encoding therapeutic factors other than TSG-6.
Data availability statement
The original contributions presented in the study are included in the article/Supplementary material, further inquiries can be directed to the corresponding author.
Ethics statement
The animal study was reviewed and approved by Institutional Animal Care and Use Committee (IACUC) of Seoul National University (SNU). Written informed consent was obtained from the owners for the participation of their animals in this study.
Author contributions
G-HY and S-MP contributed to conception and design of the study, organization of data, statistical analysis, and manuscript writing. G-HL performed the organization of data and statistical analysis. K-WS supervised and reviewed the manuscript. H-YY designed and supervised the study. All authors contributed to manuscript revision, read, and approved the submitted version.
Acknowledgments
We are grateful to the Research Institute for Veterinary Science, Seoul National University. We would like to thank Editage (www.editage.co.kr) for English language editing.
Conflict of interest
The authors declare that the research was conducted in the absence of any commercial or financial relationships that could be construed as a potential conflict of interest.
Publisher's note
All claims expressed in this article are solely those of the authors and do not necessarily represent those of their affiliated organizations, or those of the publisher, the editors and the reviewers. Any product that may be evaluated in this article, or claim that may be made by its manufacturer, is not guaranteed or endorsed by the publisher.
Supplementary material
The Supplementary Material for this article can be found online at: https://www.frontiersin.org/articles/10.3389/fvets.2023.1134185/full#supplementary-material
References
1. Weng Y, Li C, Yang T, Hu B, Zhang M, Guo S, et al. The challenge and prospect of mRNA therapeutics landscape. Biotechnol Adv. (2020) 40:107534. doi: 10.1016/j.biotechadv.2020.107534
2. Guan S, Rosenecker J. Nanotechnologies in delivery of mRNA therapeutics using nonviral vector-based delivery systems. Gene Ther. (2017) 24:133–43. doi: 10.1038/gt.2017.5
3. Sahin U, Karikó K, Türeci Ö. mRNA-based therapeutics—developing a new class of drugs. Nat Rev Drug Discov. (2014) 13:759–80. doi: 10.1038/nrd4278
4. Asrani KH, Farelli JD, Stahley MR, Miller RL, Cheng CJ, Subramanian RR, et al. Optimization of mRNA untranslated regions for improved expression of therapeutic mRNA. RNA Biol. (2018) 15:756–62. doi: 10.1080/15476286.2018.1450054
5. Zarghampoor F, Azarpira N, Khatami SR, Behzad-Behbahani A, Foroughmand AM. Improved translation efficiency of therapeutic mRNA. Gene. (2019) 707:231–8. doi: 10.1016/j.gene.2019.05.008
6. Volloch V, Housman D. Stability of globin mRNA in terminally differentiating murine erythroleukemia cells. Cell. (1981) 23:509–14. doi: 10.1016/0092-8674(81)90146-X
7. Karikó K, Kuo A, Barnathan E. Overexpression of urokinase receptor in mammalian cells following administration of the in vitro transcribed encoding mRNA. Gene Ther. (1999) 6:1092–100. doi: 10.1038/sj.gt.3300930
8. Ross J, Sullivan TD. Half-lives of beta and gamma globin messenger RNAs and of protein synthetic capacity in cultured human reticulocytes. Blood. (1985) 66:1149–54. doi: 10.1182/blood.V66.5.1149.1149
9. Trepotec Z, Lichtenegger E, Plank C, Aneja MK, Rudolph C. Delivery of mRNA therapeutics for the treatment of hepatic diseases. Mol Ther. (2019) 27:794–802. doi: 10.1016/j.ymthe.2018.12.012
10. González MA, Gonzalez-Rey E, Rico L, Büscher D, Delgado M. Adipose-derived mesenchymal stem cells alleviate experimental colitis by inhibiting inflammatory and autoimmune responses. Gastroenterology. (2009) 136:978–89. doi: 10.1053/j.gastro.2008.11.041
11. Jung KH, Song SU Yi T, Jeon MS, Hong SW, Zheng HM, et al. Human bone marrow-derived clonal mesenchymal stem cells inhibit inflammation and reduce acute pancreatitis in rats. Gastroenterology. (2011) 140:998–1008. doi: 10.1053/j.gastro.2010.11.047
12. Shin TH, Kim HS, Kang TW, Lee BC, Lee HY, Kim YJ, et al. Human umbilical cord blood-stem cells direct macrophage polarization and block inflammasome activation to alleviate rheumatoid arthritis. Cell Death Dis. (2016) 7:e2524. doi: 10.1038/cddis.2016.442
13. Choi H, Lee RH, Bazhanov N, Oh JY, Prockop DJ. Anti-inflammatory protein TSG-6 secreted by activated MSCs attenuates zymosan-induced mouse peritonitis by decreasing TLR2/NF-κB signaling in resident macrophages. Blood. (2011) 118:330–8. doi: 10.1182/blood-2010-12-327353
14. Li Q, Song WJ Ryu MO, Nam A, An JH, Ahn JO, et al. TSG-6 secreted by human adipose tissue-derived mesenchymal stem cells ameliorates severe acute pancreatitis via ER stress downregulation in mice. Stem Cell Res Ther. (2018) 9:255. doi: 10.1186/s13287-018-1009-8
15. Lee JW, Fang X, Krasnodembskaya A, Howard JP, Matthay MA. Concise review: mesenchymal stem cells for acute lung injury: role of paracrine soluble factors. Stem Cells. (2011) 29:913–9. doi: 10.1002/stem.643
16. Danchuk S, Ylostalo JH, Hossain F, Sorge R, Ramsey A, Bonvillain RW, et al. Human multipotent stromal cells attenuate lipopolysaccharide-induced acute lung injury in mice via secretion of tumor necrosis factor-alpha-induced protein 6. Stem Cell Res Ther. (2011) 2:27. doi: 10.1186/scrt68
17. Sala E, Genua M, Petti L, Anselmo A, Arena V, Cibella J, et al. Mesenchymal stem cells reduce colitis in mice via release of TSG6, independently of their localization to the intestine. Gastroenterology. (2015) 149:163–176.e20. doi: 10.1053/j.gastro.2015.03.013
18. Lee RH, Pulin AA, Seo MJ, KOTA DJ, Ylostalo J, Larson BL, et al. Intravenous hMSCs improve myocardial infarction in mice because cells embolized in lung are activated to secrete the anti-inflammatory protein TSG-6. Cell Stem Cell. (2009) 5:54–63. doi: 10.1016/j.stem.2009.05.003
19. Roddy GW, Oh JY, Lee RH, Bartosh TJ, Ylostalo J, Coble K, et al. Action at a distance: systemically administered adult stem/progenitor cells (MSCs) reduce inflammatory damage to the cornea without engraftment and primarily by secretion of TNF-alpha stimulated gene/protein 6. Stem Cells. (2011) 29:1572–9. doi: 10.1002/stem.708
20. Qi Y, Jiang D, Sindrilaru A, Stegemann A, Schatz S, Treiber N, et al. TSG-6 released from intradermally injected mesenchymal stem cells accelerates wound healing and reduces tissue fibrosis in murine full-thickness skin wounds. J Invest Dermatol. (2014) 134:526–37. doi: 10.1038/jid.2013.328
21. Murray PJ, Wynn TA. Protective and pathogenic functions of macrophage subsets. Nat Rev Immunol. (2011) 11:723–37. doi: 10.1038/nri3073
22. Song WJ Li Q, Ryu MO, Ahn JO, Bhang DH, Jung YC, Youn HY. TSG-6 released from intraperitoneally injected canine adipose tissue-derived mesenchymal stem cells ameliorate inflammatory bowel disease by inducing M2 macrophage switch in mice. Stem Cell Res Ther. (2018) 9:91. doi: 10.1186/s13287-018-0841-1
23. Song WJ Li Q, Ryu MO, Ahn JO, Ha Bhang D, Chan Jung Y, Youn HY. TSG-6 secreted by human adipose tissue-derived mesenchymal stem cells ameliorates DSS-induced colitis by inducing M2 macrophage polarization in mice. Sci Rep. (2017) 7:5187. doi: 10.1038/s41598-017-04766-7
24. Ullah M, Liu DD, Thakor AS. Mesenchymal stromal cell homing: mechanisms and strategies for improvement. iScience. (2019) 15:421–38. doi: 10.1016/j.isci.2019.05.004
25. Peruzzaro ST, Andrews MMM, Al-Gharaibeh A, Pupiec O, Resk M, Story D, et al. Transplantation of mesenchymal stem cells genetically engineered to overexpress interleukin-10 promotes alternative inflammatory response in rat model of traumatic brain injury. J Neuroinflammation. (2019) 16:2. doi: 10.1186/s12974-018-1383-2
26. Zhang C, Delawary M, Huang P, Korchak JA, Suda K, Zubair AC. IL-10 mRNA engineered MSCs demonstrate enhanced anti-inflammation in an acute GvHD model. Cells. (2021) 10:101. doi: 10.3390/cells10113101
27. Wu H, Peng Z, Xu Y, Sheng Z, Liu Y, Liao Y, et al. Engineered adipose-derived stem cells with IGF-1-modified mRNA ameliorates osteoarthritis development. Stem Cell Res Ther. (2022) 13:19. doi: 10.1186/s13287-021-02695-x
28. Yang H, Aprecio RM, Zhou X, Wang Q, Zhang W, Ding Y, et al. Therapeutic effect of TSG-6 engineered iPSC-derived MSCs on experimental periodontitis in rats: a pilot study. PLoS ONE. (2014) 9:e100285. doi: 10.1371/journal.pone.0100285
29. An JH Li Q, Ryu MO, Nam AR, Bhang DH, Jung YC, et al. TSG-6 in extracellular vesicles from canine mesenchymal stem/stromal is a major factor in relieving DSS-induced colitis. PLoS ONE. (2020) 15:e0220756. doi: 10.1371/journal.pone.0220756
30. Yi Z, Stunz LL, Bishop GA. CD40-mediated maintenance of immune homeostasis in the adipose tissue microenvironment. Diabetes. (2014) 63:2751–60. doi: 10.2337/db13-1657
31. Manning K, Rachakonda PS, Rai MF, Schmidt MF. Co-expression of insulin-like growth factor-1 and interleukin-4 in an in vitro inflammatory model. Cytokine. (2010) 50:297–305. doi: 10.1016/j.cyto.2010.01.010
32. Spaggiari GM, Capobianco A, Abdelrazik H, Becchetti F, Mingari MC, Moretta L. Mesenchymal stem cells inhibit natural killer-cell proliferation, cytotoxicity, and cytokine production: role of indoleamine 2,3-dioxygenase and prostaglandin E2. Blood. (2008) 111:1327–33. doi: 10.1182/blood-2007-02-074997
33. Park JS, Suryaprakash S, Lao YH, Leong KW. Engineering mesenchymal stem cells for regenerative medicine and drug delivery. Methods. (2015) 84:3–16. doi: 10.1016/j.ymeth.2015.03.002
34. Li X, Zhao X, Fang Y, Jiang X, Duong T, Fan C, et al. Generation of destabilized green fluorescent protein as a transcription reporter. J Biol Chem. (1998) 273:34970–5. doi: 10.1074/jbc.273.52.34970
35. Holtkamp S, Kreiter S, Selmi A, Simon P, Koslowski M, Huber C, et al. Modification of antigen-encoding RNA increases stability, translational efficacy, and T-cell stimulatory capacity of dendritic cells. Blood. (2006) 108:4009–17. doi: 10.1182/blood-2006-04-015024
36. Chen CY, Ezzeddine N, Shyu AB. Messenger RNA half-life measurements in mammalian cells. Methods Enzymol. (2008) 448:335–57. doi: 10.1016/S0076-6879(08)02617-7
37. Sgourou A, Routledge S, Antoniou M, Papachatzopoulou A, Psiouri L, Athanassiadou A. Thalassaemia mutations within the 5′UTR of the human beta-globin gene disrupt transcription. Br J Haematol. (2004) 124:828–35. doi: 10.1111/j.1365-2141.2004.04835.x
38. Russell JE, Liebhaber SA. The stability of human beta-globin mRNA is dependent on structural determinants positioned within its 3' untranslated region. Blood. (1996) 87:5314–23. doi: 10.1182/blood.V87.12.5314.bloodjournal87125314
39. Wechsler ME, Rao VV, Borelli AN, Anseth KS. Engineering the MSC secretome: a hydrogel focused approach. Adv Healthc Mater. (2021) 10:e2001948. doi: 10.1002/adhm.202001948
40. Park SM, Li Q, Ryu MO, Nam A, An JH, Yang JI, et al. Preconditioning of canine adipose tissue-derived mesenchymal stem cells with deferoxamine potentiates anti-inflammatory effects by directing/reprogramming M2 macrophage polarization. Vet Immunol Immunopathol. (2020) 219:109973. doi: 10.1016/j.vetimm.2019.109973
41. Zhao L, Hu C, Zhang P, Jiang H, Chen J. Melatonin preconditioning is an effective strategy for mesenchymal stem cell-based therapy for kidney disease. J Cell Mol Med. (2020) 24:25–33. doi: 10.1111/jcmm.14769
42. Nakamura Y, Murai T, Ogawa Y. Effect of in vitro and in vivo administration of dexamethasone on rat macrophage functions: comparison between alveolar and peritoneal macrophages. Eur Respir J. (1996) 9:301–6. doi: 10.1183/09031936.96.09020301
43. Jeon YJ, Han SH, Lee YW, Lee M, Yang KH, Kim HM. Dexamethasone inhibits IL-1β gene expression in LPS-stimulated RAW 2647 cells by blocking NF-κB/Rel and AP-1 activation. Immunopharmacology. (2000) 48:173–83. doi: 10.1016/S0162-3109(00)00199-5
44. Wisniewski HG, Hua JC, Poppers DM, Naime D, Vilcek J, Cronstein BN. TNF/IL-1-inducible protein TSG-6 potentiates plasmin inhibition by inter-alpha-inhibitor and exerts a strong anti-inflammatory effect in vivo. J Immunol. (1996) 156:1609–15. doi: 10.4049/jimmunol.156.4.1609
45. Kim YJ, Ryu JS, Park SY, Lee HJ, Ko JH, Kim MK, et al. Comparison of topical application of TSG-6, cyclosporine, and prednisolone for treating dry eye. Cornea. (2016) 35:536–42. doi: 10.1097/ICO.0000000000000756
Keywords: canine adipose tissue, mesenchymal stem cell, immunomodulation, TSG-6, in vitro transcription, mRNA, genetic engineering
Citation: Yun G-H, Park S-M, Lim G-H, Seo K-W and Youn H-Y (2023) Canine adipose tissue-derived MSCs engineered with mRNA to overexpress TSG-6 and enhance the anti-inflammatory effects in canine macrophages. Front. Vet. Sci. 10:1134185. doi: 10.3389/fvets.2023.1134185
Received: 30 December 2022; Accepted: 17 March 2023;
Published: 06 April 2023.
Edited by:
Hesam Dehghani, Ferdowsi University of Mashhad, IranReviewed by:
Marina I. Garin, Health Research Institute Foundation Jimenez Diaz (IIS-FJD), SpainTereza Cristina Cardoso, Universidade Estadual de São Paulo, Brazil
Copyright © 2023 Yun, Park, Lim, Seo and Youn. This is an open-access article distributed under the terms of the Creative Commons Attribution License (CC BY). The use, distribution or reproduction in other forums is permitted, provided the original author(s) and the copyright owner(s) are credited and that the original publication in this journal is cited, in accordance with accepted academic practice. No use, distribution or reproduction is permitted which does not comply with these terms.
*Correspondence: Hwa-Young Youn, aHl5b3VuQHNudS5hYy5rcg==
†These authors have contributed equally to this work and share first authorship