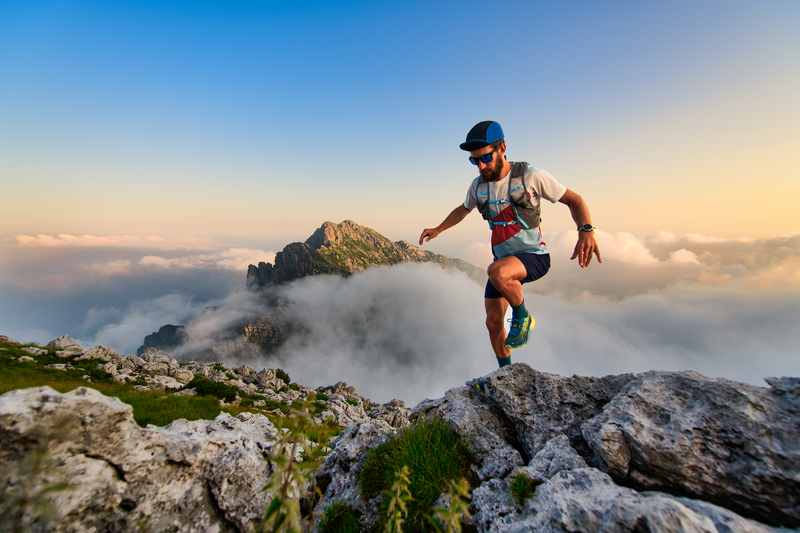
95% of researchers rate our articles as excellent or good
Learn more about the work of our research integrity team to safeguard the quality of each article we publish.
Find out more
ORIGINAL RESEARCH article
Front. Vet. Sci. , 27 April 2023
Sec. Veterinary Infectious Diseases
Volume 10 - 2023 | https://doi.org/10.3389/fvets.2023.1099255
This article is part of the Research Topic Trends For Disease Control In Aquaculture View all 5 articles
Lysine acetylation modification is a dynamic and reversible post-translational modification, which plays an important role in the metabolism and pathogenicity of pathogenic bacteria. Vibrio alginolyticus is a common pathogenic bacterium in aquaculture, and bile salt can trigger the expression of bacterial virulence. However, little is known about the function of lysine acetylation in V. alginolyticus under bile salt stress. In this study, 1,315 acetylated peptides on 689 proteins were identified in V. alginolyticus under bile salt stress by acetyl-lysine antibody enrichment and high-resolution mass spectrometry. Bioinformatics analysis found that the peptides motif ****A*Kac**** and *******Kac****A* were highly conserved, and protein lysine acetylation was involved in regulating various cellular biological processes and maintaining the normal life activities of bacteria, such as ribosome, aminoacyl-tRNA biosynthesis, fatty acid metabolism, two-component system, and bacterial secretion system. Further, 22 acetylated proteins were also found to be related to the virulence of V. alginolyticus under bile salt stress through secretion system, chemotaxis and motility, and adherence. Finally, comparing un-treated and treated with bile salt stress lysine acetylated proteins, it was found that there were 240 overlapping proteins, and found amino sugar and nucleotide sugar metabolism, beta-Lactam resistance, fatty acid degradation, carbon metabolism, and microbial metabolism in diverse environments pathways were significantly enriched in bile salt stress alone. In conclusion, this study is a holistic analysis of lysine acetylation in V. alginolyticus under bile salt stress, especially many virulence factors have also acetylated.
With the development of biotechnology development and related research, more than 200 types of post-translational modifications (PTMs), such as phosphorylation, methylation, acetylation, succinylation, and glycosylation, and a large number of PTM sites have been identified (1, 2). Acetylation is an evolutionarily conserved, abundant, and ubiquitous protein post-translational modification that plays a crucial role in the regulation of bacterial enzymatic activity, cellular physiology, metabolic processes, and virulence (3–5). Before 2008, only a few bacterial acetylated proteins had been identified and characterized, then with the rapid development of high-affinity immunoisolation using antibodies to enrich acetylated proteins/peptides from complex samples and HPLC-MS/MS technology, many research groups conducted a global screening and characterization of lysine acetylation in bacteria, such as Escherichia coli, Bacillus subtilis, and Mycobacterium tuberculosis (6–8), as well as aquatic pathogenic bacteria Aeromonas hydrophila, Vibrio mimicus, Vibrio parahaemolyticus, and Vibrio alginolyticus (9–12), etc., to provide a framework for the systematic analysis of the physiological role and regulatory mechanism of lysine acetylation in bacteria. And as the number of identified acetylation sites and proteins increases, important information such as protein interaction network, localization and gene ontology annotation can be analyzed more comprehensively (2). However, how protein acetylation in bacteria affects global cellular metabolism as well as its complex regulatory mechanisms and physiological effects are still unclear and required further research.
Vibrio alginolyticus is a halophilic Gram-negative bacterium, is widely distributed in the sea, inshore and river mouth areas. It is not only the main pathogen of mariculture economic animals, such as oysters, groupers and Penaeus vannamei (13–15), causing huge economic losses to the aquaculture industry and posing a great threat to the breeding environment, but also the key reason for acute gastroenteritis, diarrhea, and even septicemia in humans caused by exposure to contaminated seafood all over the world (16, 17). It mainly produces pathogenic effects by producing pathogenic factors that damage host somatic cells and interfere with metabolism. In V. alginolyticus common virulence factors genes including virulence expression regulatory genes (toxR), outer membrane protein (ompW, ompR, ompN), adhesion (flrA, flrB, flrC), hemolysin (thd), secretion system (tyeA, Val1686, Val1680), and so on (2, 18–22).
Bile salt is the main component of bile, which can affect cell membrane protein and phospholipid structure, thereby disrupting cell membranes, damaging nucleic acids, causing redox stress, and triggering the expression of bacterial virulence in vivo (23, 24). Many gut bacteria adapt to bile salt stress by decreasing membrane permeability, stimulating efflux pumps, inducing biofilm formation, and up regulating redox and DNA damage repair genes (23), such as the production of c-di-AMP in Clostridium difficile makes cells sensitive to osmotic stress, and then regulates bile salt stress (25), E. coli is protecting itself by the Rob-mediated upregulation of AcrAB against the harmful effects of bile salts in the intestinal tract (26). In V. parahaemolyticus, genes vtrC, vtrA and vtrB are necessary to activate type III virulence secretion system 2 in response to bile salts (27). However, the mechanism of pathogenic bacteria sensing bile salts, whether directly by binding to signaling proteins or indirectly by sensing cell damage, requires further in-depth study.
More and more studies have found that protein acetylation may be related to bacterial virulence, especially the acetylation of bacterial virulence factors. Such as acetylation of GtfB and GtfC will change the formation of biofilm of S. mutans, thus regulating its virulence and pathogenicity (28), acetylation can regulate the activity of PhoP, impairs its DNA-binding ability, and resulting in attenuated virulence of S. typhimurium (29), the reversible acetylation mediated by CobB and YfiQ can regulate the psaABCDEF operon, thus affecting the expression of Psa fimbriae protein, and ultimately affecting Y. pestis virulence (30). Acetylation acts as a fine-tuning switch that can regulate the virulence of bacteria.
Therefore, in this study, we treated V. alginolyticus with bile salt to extract the whole protein of bacteria, and identified 1,315 acetylated peptides and 689 acetylated proteins by acetyl-lysine antibody enrichment and LC-MS/MS analysis technology. The acetylated peptide analysis found that the sequence motifs ****A*Kac**** and *******Kac****A* were obviously conserved, while the acetylated proteins were mainly involved in ribosome, two-component system, aminoacyl tRNA biosynthesis, fatty acid metabolism, and bacterial secretion system processes. Through screening with VFDB database, 22 acetylated proteins were found to be related to virulence. The lysine acetylation overlapping proteins were further compared and analyzed under bile salt stress and unstressed environments. Bioinformatics analysis found that most of the overlapping acetylated proteins were involved in the regulation of ribosomes, antibiotic biosynthesis, pyrimidine metabolism, and purine metabolism. In conclusion, this study provides a theoretical basis for future research on the regulatory mechanism of bile salts in V. alginolyticus, especially in the study of bacterial physiological functions in terms of protein post-translational modification.
Vibrio alginolyticus HY9901 was isolated from Lutjanus erythopterus, and stored in our laboratory (31). Its optimum culture temperature is 28°C. Pick monoclonal colonies from TSB (Tryptone Soya Broth, Oxoid U.S.A., Inc., Columbia, Md) medium plates into TSB liquid medium for overnight culture. The next day, overnight culture transfer by 1% to a fresh TSB medium containing 0.04% bile salts (Sigma-Aldrich, St. Louis, MO) (32) and culture until the OD was about 1.0, and then collect cells were by centrifugation. The pellet was washed twice with pre-cooled PBS (Phosphate Buffered Saline) and dissolved in lysis buffer [containing 100 mM NH4HCO3 (pH 8), 6 M Urea and 0.2% SDS], followed by 5 min of ultrasonication on ice. The lysate was centrifuged at 12,000 g for 30 min at 4°C and collect the supernatant as the whole protein sample. The protein was reduced with 2 mM DTT (Thermo Fisher, Waltham, Massachusetts, USA) for 1 h at 56°C, and subsequently alkylated with sufficient Iodoacetamide (IAA, Thermo Fisher, Waltham, Massachusetts, USA) for 1 h at room temperature in the dark. Then 4 times volume of pre-cooled acetone was mixed with samples by well vortex and incubated at −20°C for at least 2 h. Sample was then centrifuged and the precipitation was collected. After washing twice with pre-cold acetone, the pellet was dissolved by dissolution buffer [6 M urea in 0.1 M triethylammonium bicarbonate (TEAB, pH 8.5), Thermo Fisher, Waltham, Massachusetts, USA]. Protein concentration was determined by Bradford protein assay.
Ten milligrams of protein was digested with Trypsin Gold (Promega, Madison, Wisconsin, USA) at 1:50 enzyme-to-substrate ratio in 50 mM TEAB buffer. After 16 h of digestion at 37°C, an equal volume of 1% formic acid solution was added and centrifuged at room temperature for 5 min at 12,000 g. Then the supernatant was desalted using a Sep-Pak Vac C18 cartridge (Thermo Fisher, Waltham, Massachusetts, USA), and washed three times with a cleaning solution containing 0.1% formic acid and 4% acetonitrile to remove the high urea. Finally, an eluent buffer containing 0.1% formic acid and 75% acetonitrile was added to elute the desalted peptide, and dried by vacuum centrifugation (Labogene, Denmark).
Lyophilized peptide segments were dissolved with MOPS IAP solution (Tris-HCl, pH 7.0) containing 50 mM MOPS, 10 mM KH2PO4 and 50 mM NaCl, and centrifuged at 4°C and 12,000 g for 5 min. The supernatant was mixed with Acetyl-Lysine Motif [Ac-K] Immunoaffinity Beads (CST #13416, Cell Signaling Technology, Danvers, Massachusetts, USA) and washed three times with 1 × PBS, followed by incubation at 4°C for 2.5 h. After incubation, the sample was centrifuged at 4°C and 3,000 g for 30 s, then the supernatant was discarded and beads were washed in precooled MOPS IAP buffer, then in H2O for 3 times, respectively. Finally, the peptides were eluted with 0.15% TFA. After mixing and incubation at room temperature for 10 min, centrifuged at 3,000 g for 30 s, and the elution was repeated 3 times. The eluted samples were pooled and centrifuged at 5,000 g for 5 min, and the supernatant were desalted using peptide desalting spin columns (Thermo Fisher, Waltham, Massachusetts, USA) and lyophilization.
Use 10 μl of 0.1% FA (formic acid) buffer to resuspend the sample, centrifuge at 10,000 rpm for 5 min at room temperature, and take 1 μg of supernatant sample for proteomics analyses using an EASY-nLCTM 1200 UHPLC (ultra-high pressure liquid chromatography) system (Thermo Fisher, Waltham, Massachusetts, USA) coupled with an Orbitrap Q Exactive HF-X mass spectrometer (Thermo Fisher, Waltham, Massachusetts, USA) operating in the DDA (data-dependent acquisition) mode. Refer to the previous description for specific details (12).
The database used is the Uniprot_Vibrio alginolyticus protein database (4,338 sequences). The raw files were directly imported into Proteome Discoverer 2.2 software for database search, peptide and protein quantification. Filtered the search results including the peptide spectrum matches (PSMs) with a reliability of more than 99%, the proteins containing at least one unique peptide, and to remove the peptides and proteins with an FDR (False discovery rate) >1%. After the database search, a series of quality control needs to be carried out, including peptide length distribution, precursor ion mass tolerance distribution, Unique peptide number distribution, protein coverage distribution, and protein molecular weight distribution. The mass spectrometry proteomics data have been deposited to the ProteomeXchange Consortium (http://proteomecentral.proteomexchange.org) via the iProX partner repository (33) with the dataset identifier PXD035599.
Sequence motifs associated with protein acetylation modification were analyzed using online software MoMo (Version 5.4.1, https://meme-suite.org/meme/index.html). Analysis of cellular components (CC), molecular functions (MF), biological processes (BP) and Kyoto Encyclopedia of Genes and Genomes (KEGG) were performed using the online software Omicsbean (http://www.omicsbean.cn/). The annotation of subcellular localization was performed using the Cell-mPLOC 2.0 (34). The COG database of NCBI (https://www.ncbi.nlm.nih.gov/COG/) was used to analyze the Cluster of Orthologous Groups of proteins (COG). STRING (https://cn.string-db.org/) combined with Cytoscape_3.1.7 software were used for protein-protein interaction analysis and graphical visualization. The Virulence Factor Database (VFDB) database (http://www.mgc.ac.cn/VFs/main.htm) was used to screen virulence factors. Use GraphPad Prism 8 software to generate the above analysis results.
The whole protein (500 μg) of V. alginolyticus under bile salt stress was incubated with specific polyclonal antibodies OmpN, OmpR, and GrpE at 4°C overnight to precipitate target proteins, as previously described (10). Briefly, the protein A/G beads were washed three times with pre-cooled PBS buffer (137 mM NaCl, 2.7 mM KCl, 4.3 mM Na2HPO4, 1.4 mM KH2PO4, pH 7.2–7.4) and then added to the lysate for incubation at 4°C for 2 h. The beads were centrifuged at 4°C and 2,500 g for 5 min to remove the supernatant, and washed five times with pre-cooled PBS buffer. In total, 50 μl loading buffer (containing 250 mM Tric-HCl pH 6.8, 10% SDS, 0.5% bromophenol blue, 50% glycerol and adding 5% β-mercaptoethanol before use) was added and boiled for 5 min, and then run SDS-PAGE and Western blot verification. After SDS-PAGE, a Trans-Blot Turbo Transfer System (Bio-Rad, Hercules, CA, USA) was used to transfer proteins to PVDF membrane (polyvinylidene fluoride, Millipore, Billerica, MA, USA). The membrane was blocked with QuickBlockTM blocking buffer (Beyotime Biotechnology Co., Ltd, Shanghai) 15 min at room temperature and probed with OmpN, OmpR, GrpE serum antibody and anti-acetyllysine mouse mAb (PTM-101, 1:5,000 diluted in QuickBlockTM blocking buffer, PTM Biolabs Inc., Hangzhou, China), then incubated at 4°C for overnight The second antibody was HRP-labeled Goat Anti Rabbit IgG or Anti Mouse IgG (1:8,000 diluted in QuickBlockTM secondary antibody dilution buffer) which is incubated at room temperature for 2 h, and then the membrane is washed four times with TBST (20 mM Tris, 137 mM NaCl, 0.1% Tween-20, pH 7.6). Finally, the membrane colored by claritytm Western ECL substrate (#1705061, Bio-rad Co., Ltd.). The automatic chemiluminescence image analysis system (TAN 5200, Tanon Science & Technology Co., Ltd, Shanghai, China) was used to take photos and record the experimental results.
In the study, immunoaffinity enrichment of lysine-acetylated peptides with Acetyl-Lysine Motif [Ac-K] Immunoaffinity Beads and LC-MS/MS analysis to profile the acetylated proteins and peptides under bile salt stress in V. alginolyticus. The analysis found that peptides and proteins with FDR < 1% had a total of 1,315 peptides and 689 proteins were acetylated under bile salt stress in V. alginolyticus (Supplementary Tables 1, S2). The modification sites in acetylated proteins are mainly between 1 and 12. The results showed that 409 proteins (59.36%) have only one modification site, 129 proteins (20.17%) have two sites, 60 proteins (8.71%) have three sites, 36 proteins (5.23%) have four sites, and 45 proteins (6.53%) have more than four modification sites, and the proteins RpoB and N646_ 0059 contain 12 modification sites (Figure 1A). Most of the acetylated-modified peptides are between 7 and 29 amino acids (94.3%), only two peptides are six amino acids, and 73 peptides (5.55%) are ≥30 amino acids (Figure 1B).
Figure 1. Outline of acetylome under bile salt stress in V. alginolyticus. (A) Site coverage of acetylated proteins, and (B) Distribution of acetylated peptide lengths.
In order to identify potential motifs in acetylated peptides under bile salt stress in V. alginolyticus, sequence motifs (amino acid sequences from the −7 to +7 positions of the identified Kac, Kac represents lysine acetylation modification site) were extracted by online software MoMo. From 1,315 unique lysine-acetylated peptides, seven sequence motif were enriched, namely *****A*Kac*******, *******Kac*****A*, *******Kac*A*****, ******DKac*******, ******EKac*I*****, *****V*Kac******* and ******AKac******* (* represents a random amino acid) (Figure 2A). Particularly, sequence motifs *****A*Kac******* (155 Kac peptides) and *******Kac*****A* (122 Kac peptides) were evidently conserved (Figure 2B). Lysine acetylation occurs preferentially in regions rich in alanine (A), such as on position −2, +6, +2, and −1. Acidic amino acid aspartate (D) and glutamate (E) residue were preferentially occupied the position −1, and valine (V) residue preferred to be on position −2 (Figure 2).
Figure 2. Analysis of motifs of lysine acetylated-peptides under bile salt stress in V. alginolyticus. (A) The sequence logos of the acetylation motifs identified by MoMo software. These motifs consist of 14 residues (seven amino acids upstream and seven amino acids downstream) surrounding the acetylated lysine, and (B) Number of acetylated peptides contained in the identified motif.
In order to better understand the function of acetylated modified proteins under bile salt stress in V. alginolyticus, we performed annotation and enrichment of GO (Gene Ontology) and KEGG functions of modified proteins. Go annotation includes three parts: biological processes, molecular functions and cell components. For biological processes, acetylated proteins are mainly involved in the regulation of small molecule metabolic process, organonitrogen compound metabolic process, organic substance metabolic process, single-organism metabolic process, cellular metabolic process, metabolic process, and single-organism process (Figure 3A). rRNA binding, ligase activity, RNA binding, small molecule binding, ion binding, binding, and catalytic activity were the largest enriched molecular functions (Figure 3B). For cellular components, the majority of acetylated proteins were found to enriched in cytoplasm, cell, intracellular, intracellular part (Figure 3C). Analysis results indicate that most lysine acetylated proteins are categorized as involved in small molecule metabolic processes, binding functions, and location of the cytoplasm.
Figure 3. GO and KEGG enrichment analysis of lysine acetylated proteins under bile salt stress in V. alginolyticus. (A) biological process, (B) molecular function, (C) cellular component, and (D) KEGG pathway.
In order to further insights into the functional correlation of acetylation in V. alginolyticus under bile salt stress, we performed KEGG pathways enrichment analysis for all identified acetylated proteins. The results revealed that the largest group of acetylated proteins was involved in metabolic pathways (20.5%), biosynthesis of antibiotics (13.3%), ribosome (5.8%), purine metabolism (3.3%), carbon metabolism (2%), beta-Lactam resistance (2.0%), and RNA degradation (1.7%) (Figure 3D). In general, these data strongly suggest that the intracellular metabolic pathway may be strictly regulated by lysine acetylation modification, so the acetylated of enzymes in the metabolic pathway is particularly important in response to bile salt stress in V. alginolyticus.
Cluster of Orthologous Groups of proteins (COG) functional annotation provides important information proteins function. Annotated acetylated proteins were categorized into 21 functional groups according to the COG function classification. Among these COG categories, a large number of acetylated proteins are enriched in translation, ribosomal structure and biogenesis, amino acid transport and metabolism, energy production and conversion, carbohydrate transport and metabolism, transcription, signal transduction mechanisms, cell wall/membrane/envelope biogenesis, general function prediction only, posttranslational modification, protein turnover, chaperones, and lipid transport and metabolism ten COG terms (Figure 4A). Our results show that a large number of translation and metabolism related proteins undergo acetylation modification.
Figure 4. Functional annotation of the lysine acetylated proteins under bile salt stress in V. alginolyticus. (A) The COG function classification analysis and (B) subcellular localization analysis of the acetylated proteins.
It is well-known that the biological cell is a highly ordered structure subdivided into multiple membrane-bound compartments with different functions. To explore the distribution of acetylated proteins among cellular compartments, we performed subcellular localization analysis of acetylated proteins using the Cell-mPLOC 2.0 website. Results showed that acetylated proteins were mainly distributed in the cytoplasm (60.96%), followed by the cell inner membrane (22.99%), periplasm (9.98%), cell outer membrane (3.48%), extracell (1.6%), and nucleoid (1.07%) (Figure 4B).
Through VFDB database, we screened 22 virulence factors in the lysine acetylome of V. alginolyticus under bile salt stress (Table 1). These 22 acetylated proteins participate in various pathogenic processes of bacteria through the secretion system (AOG25_14550, VMC_23950, K05K4_27280, AOG25_21875, secA, secD, secY, tolC, tatA, yajC, yidC, and clpB), chemotaxis and motility (AOG25_15960, cheA, cheY, frr, AOG25_15815, HUO05_08750, and N646_1361), adherence (N646_1788 and VMC_30830), and toxin (AOG25_14550). Acetylation modification of virulence factors may play an important role in the pathogenicity of V. alginolyticus.
In order to understand the cellular process regulated by lysine acetylation in V. alginolyticus under bile salt stress, based on STRING and Cytoscape_ 3.1.7 software generates the protein-protein interaction network. The results showed that 647 acetylated proteins were mapped to the protein network database, forming a highly interconnected protein network. As shown in Figure 5, ribosomes, two-component system, aminoacyl-tRNA biosynthesis, fatty acid metabolism, and bacterial secretion system formed prominent and highly connected protein clusters. In particular, the identified top clusters related to ribosomes, total of 32 network nodes (acetylated proteins) and 496 lines (interactions between proteins) constitute the interaction network (Figure 5A). Other subnetworks for two-component system, fatty acid metabolism, and bacterial secretion system were also have a relatively high density. The complex protein interaction network of lysine acetylated proteins indicates that lysine acetylation modification may regulate a variety of pathways in V. alginolyticus under bile salt stress.
Figure 5. Protein-protein interaction networks of acetylated proteins under bile salt stress in V. alginolyticus. PPI networks of acetylated proteins were analyzed using the STRING combine with Cytoscape software. (A) ribosomes, (B) two-component system, (C) aminoacyl-tRNA biosynthesis, (D) fatty acid metabolism, and (E) bacterial secretion system.
OmpN, OmpR, and GrpE three acetylated proteins, were analyzed by co-immunoprecipitation (Co-IP) and Western blotting to further prove the identified lysine-acetylated results in V. alginolyticus under bile salt stress. The OmpN, OmpR, and GrpE proteins were enriched with anti-OmpN, anti-OmpR, and anti-GrpE antibodies and visualized via Western blotting, which was performed with target proteins or anti-acetyllysine antibodies, respectively (Figure 6). The results clearly showed that OmpN, OmpR and GrpE proteins were acetylated.
Figure 6. Validation of OmpN (A), OmpR (B), and GrpE (C) by Co-IP and Western blotting. OmpN, OmpR, and GrpE proteins were captured by specific antibodies (anti-OmpN, anti-OmpR, and anti-GrpE), and validation by Western blotting with anti-OmpN, anti-OmpR and anti-GrpE (above), and anti-lysine acetylation antibodies (below).
According to our previous research report, 1,178 acetylated proteins were identified in V. alginolyticus (12), while in this study, we identified 689 proteins that were acetylated under bile salt stress. Venny analysis showed that a total of 240 proteins overlapped (Figure 7A). Go and KEGG analysis were performed on these overlapped proteins. Of the overlapped proteins, GO analysis showed that proteins were enriched into six biological processes, six molecular functions and four cell components classifications. Among them, organonitrogen compound biosynthetic process, heterocyclic compound binding and cytoplasm occupy the dominant position in the biological process, molecular function and cell component classifications (Figure 7B). Further analysis of KEGG pathway showed that most acetylated proteins were involved in the regulation of metabolic pathways, ribosome, biosynthesis of antibiotics, pyrimidine metabolism and purine metabolism (Figure 7C). KEGG analysis of treated and un-treated with bile salt stress lysine acetylation protein (449 and 938 proteins) of V. alginolyticus showed that it was enriched into 8 and 7 metabolic pathways, respectively (Figures 7D, E). The same pathways are metabolic pathways, biosynthesis of antibiotics and RNA degradation. Under bile salt stress amino sugar and nucleotide sugar metabolism, beta-Lactam resistance, fatty acid degradation, carbon metabolism, and microbial metabolism in diverse environments were significantly enriched (Figure 7D). However, biosynthesis of secondary metabolites, purine metabolism, biosynthesis of amino acids, and alanine, aspartate and glutamate metabolism were significantly enriched in the absence of bile salt stress (Figure 7E).
Figure 7. Comparison of un-treated and treated with bile salt stress lysine acetylated proteins in V. alginolyticus. (A) Overlap between un-treated and treated bile salt stress lysine acetylated proteins in V. alginolyticus, (B) GO enrichment analysis, and (C) KEGG pathway enrichment analysis of the overlapped acetylated proteins. (D, E) KEGG pathway enrichment analysis of treated and un-treated with bile salt stress lysine acetylation protein.
Acetylation is an important post-translational modification in bacteria, which is necessary to maintain cellular homeostasis, regulate transcription and translation of genes, and alter cellular metabolism based on environmental stress responses (1, 35). Bile salts can trigger the expression of bacterial virulence factors, leading to changes in various intracellular biological processes in cell (23). However, up to now, there is limited information about the role of acetylation of V. alginolyticus involved in the regulation of under bile salts stress and its regulatory mechanisms are still unclear. In this study, 1,315 unique lysine-acetylated peptides on 689 proteins [account for 15.88% (689/4,338) of the total proteins in V. alginolyticus] were identified under bile salt stress in V. alginolyticus. This ratio was higher than that of the aquatic pathogens V. mimicus (15.5%) and V. parahaemolyticus (13.6%), but lower than that of V. alginolyticus (27.1%), A. hydrophila (21.7%), and V. vulnificus (40.34%) (9–12, 36).
Many previous studies reported that chaperones changed significantly under bile salt stress. In this study, 13 chaperones were also found to be acetylated, including DanK, TorD, ClpX, YidC, Tig, HslU, GrpE, ClpA, ClpB, RimM, Hsp60, GroEL, and GroL-2. These chaperones play a key role in the accurate integrity and stability of intracellular biological molecules (such as protein and DNA) and resistance to bile salt stress (37–40). Furthermore, FrdA, FrdB, SdhB, SucB, SucC, SucD, Mdh, GltA, AceB, PdhA, AcnB, and Did 12 key enzymes of TCA cycle also undergo acetylation modification. The results show that acetylated chaperones and metabolic processes related proteins play an important role in the corresponding bile salt stress and reducing damage and energy efficiency in V. alginolyticus.
The identified acetylated proteins were then further examined using GO, KEGG pathway, COG function classification and subcellular localization analyses, and the result showed that many of the acetylated proteins contributed to complicated cellular metabolic process. For example, under bile salt stress environment, translation, ribosomal structure and biogenesis, amino acid transport and metabolism, and energy production and conversion were enriched in V. alginolyticus. Furthermore, ribosome and purine metabolism related proteins are higher tendency to be acetylated, and this result have also been reported in V. vulnificus, M. tuberculosis and V. parahaemolyticus (11, 36, 41). As well as the acetylated modified proteins are mainly distributed in the cytoplasm and are highly conserved, which is similar to the results previously obtained in Spiroplasma eriocheiris, Brenneria nigrifluens, Streptococcus pneumoniae, and Streptococcus mutans (42–45). These findings suggest that the noted proteins involved in translation, protein synthesis and energy metabolism tend to undergo acetylation modification in cytoplasm under bile salt stress.
Interesting, under bile salt stress sample, many of proteins as virulence factors were shown to be acetylated. For example, secretion system related genes secA, secD, secF, yajC, yidC and tolC, which prior to studies reported that secretion system genes played roles in the bacterial adhesion, thereby promoting the adhesion of V. alginolyticus to the host surface and causing bacterial infection (46). In Vibrio cholerae, the outer membrane protein TolC is necessary for the expression of toxR regulon and participates in an efflux dependent feedback loop to regulate the expression of virulence genes, MARTX toxin secretion and resistance to antimicrobial compounds present in the host (47). In this study, we also found that ToxR protein, a key transcription activator, is acetylated. Bile salt can promote the activation of toxR regulator and participate in the regulation of bacterial virulence genes (48, 49). Bacterial toxin factor (AOG25_14550) is a Hcp1 family type VI secretion system effector, which also acetylated under bile salt stress in V. alginolyticus. It is reported that it is related to bacterial virulence in Edwardsiella ictaluri and A. hydrophila (50, 51). Furthermore, in this study, many chemotaxis, motility and adherence related proteins are acetylated. A large number of studies have proved that bacteria can move to the favorable environment or away from the harmful environment through chemotaxis and motility, which plays an important role in infection and disease. Therefore, it can block infection and prevent disease by interfering with chemotaxis and motor signaling pathways (52, 53). Additionally, Type IV pilus, mannose-sensitive hemagglutinin A (N646_1788) and MSHA biogenesis protein MshH (VMC_30830) participate in the regulation of bacterial surface attachment and colonization by controlling the dynamic activity of pili, especially in Vibrio cholerae (54, 55). In conclusion, a variety of virulence factors in V. alginolyticus are acetylated under bile salt stress, and jointly participate in the regulation of bacterial pathogenicity.
Additionally, protein-protein interaction network plays an important role in regulating cells and their signals. In this study, the ribosome related protein network is the most complex, which is consistent with many previous research results. Advanced complex composed of ribosome related proteins was found in many prokaryotic bacteria, such as V. parahaemolyticus (45 acetylated proteins and 982 direct physical interactions), V. mimicus (36 acetylated proteins and 630 direct physical interactions), Shewanella baltica (43 acetylated proteins), and Streptomyces coelicolor (25 acetylated proteins) (10, 11, 56, 57) are mainly have a hand in protein translation. Other subnetworks of proteins related to two-component system, fatty acid metabolism, and bacterial secretion system also have a relatively high correlation, and these pathways are considered to be closely related to bacterial pathogenicity (58–60). The complex protein interaction network of lysine acetylated proteins indicates that lysine acetylation modification may regulate a variety of pathways in V. alginolyticus under bile salt stress and participate in the regulation of bacterial virulence. Protein-protein interaction network provides a better approach for understanding the functions of acetylated proteins in cells. In a word, this study reported the global acetylation proteome of V. alginolyticus under bile salt stress, which provided a basis for the future study of acetylation regulation function and molecular mechanism of V. alginolyticus under bile salt stress.
The datasets presented in this study can be found in online repositories. The names of the repository/repositories and accession number(s) can be found in the article/Supplementary material.
All animal experiments were conducted strictly based on the recommendations in the Guide for the Care and Use of Laboratory Animals set by the National Institutes of Health. The animal protocols were approved by the Animal Ethics Committee of Guangdong Ocean University (Zhanjiang, China). In this study, there was no interaction with Lutjanus erythopterus from which the Vibrio alginolyticus HY9901 was isolated during the previous study. The bacteria protocols were approved by the Biosecurity Committee of Guangdong Ocean University (Zhanjiang, China).
HP designed the study and wrote the manuscript. WL analyzed the data and wrote the manuscript. XX, YP, ZW, and SW generated experimental data. JW wrote the manuscript. NW and JJ critically reviewed the manuscript. All authors contributed to the article and approved the final version of the manuscript.
This work was supported by the National Natural Science Foundation of China (No. 32073015), Natural Science Foundation of Guangdong Province (No. 2021A1515011078), Undergraduate Innovation Team of Guangdong Ocean University (No. CCTD201802), Innovation and Entrepreneurship Training Program for College Students (No. CXXL2022005), Science and Technology Innovation Cultivation Program for College Student (No. pdjh2021b0239), and Guangdong Postgraduate Education Innovation Project.
The authors would like to thank Dr. Bingling Xu for her valuable comments on the revision of this paper.
The authors declare that the research was conducted in the absence of any commercial or financial relationships that could be construed as a potential conflict of interest.
All claims expressed in this article are solely those of the authors and do not necessarily represent those of their affiliated organizations, or those of the publisher, the editors and the reviewers. Any product that may be evaluated in this article, or claim that may be made by its manufacturer, is not guaranteed or endorsed by the publisher.
The Supplementary Material for this article can be found online at: https://www.frontiersin.org/articles/10.3389/fvets.2023.1099255/full#supplementary-material
Supplementary Table 1. Acetylated proteins and sites identified under bile salt stress in V. alginolyticus.
Supplementary Table 2. The identified peptides under bile salt stress in V. alginolyticus.
1. VanDrisse CM, Escalante-Semerena JC. Protein acetylation in bacteria. Annu Rev Microbiol. (2019) 73:111–32. doi: 10.1146/annurev-micro-020518-115526
2. Liu M, Guo LK, Fu YX, Huo MT, Qi QS, Zhao G. Bacterial protein acetylation and its role in cellular physiology and metabolic regulation. Biotechnol Adv. (2021) 53:107842. doi: 10.1016/j.biotechadv.2021.107842
3. Ren J, Sang Y, Lu J, Yao YF. Protein acetylation and its role in bacterial virulence. Trends Microbiol. (2017) 25:768–79. doi: 10.1016/j.tim.2017.04.001
4. Dash A, Modak R. Protein acetyltransferases mediate bacterial adaptation to a diverse environment. J Bacteriol. (2021) 203:e00231–00221. doi: 10.1128/JB.00231-21
5. Hu LI, Lima BP, Wolfe AJ. Bacterial protein acetylation: the dawning of a new age. Mol Microbiol. (2010) 77:15–21. doi: 10.1111/j.1365-2958.2010.07204.x
6. Zhang K, Zheng SZ, Yang JS, Chen Y, Cheng ZY. Comprehensive profiling of protein lysine acetylation in Escherichia coli. J Proteome Res. (2013) 12:844–51. doi: 10.1021/pr300912q
7. Kim D, Yu BJ, Kim JA, Lee YJ, Choi SG, Kang S, et al. The acetylproteome of Gram-positive model bacterium Bacillus subtilis. Proteomics. (2013) 13:1726–36. doi: 10.1002/pmic.201200001
8. Liu FY, Yang MK, Wang XD, Yang SS, Gu J, Zhou J, et al. Acetylome analysis reveals diverse functions of lysine acetylation in Mycobacterium tuberculosis. Mol Cell Proteom. (2014) 13:3352–66. doi: 10.1074/mcp.M114.041962
9. Sun LN, Yao ZJ, Guo Z, Zhang LS, Wang YQ, Mao RR, et al. Comprehensive analysis of the lysine acetylome in Aeromonas hydrophila reveals cross-talk between lysine acetylation and succinylation in LuxS. Emerg Microbes Infect. (2019) 8:1229–39. doi: 10.1080/22221751.2019.1656549
10. Wang JL, Pang HY, Yin LL, Zeng FY, Wang, Hoare R, et al. A comprehensive analysis of the lysine acetylome in the aquatic animals pathogenic bacterium Vibrio mimicus. Front Microbiol. (2022) 13:816968. doi: 10.3389/fmicb.2022.816968
11. Pan JY, Ye ZC, Cheng ZY, Peng XJ, Wen LY, Zhao FK. Systematic analysis of the lysine acetylome in Vibrio parahemolyticus. J Proteome Res. (2014) 13:3294–302. doi: 10.1021/pr500133t
12. Pang HY Li WX, Zhang WJ, Zhou SH, Hoare R, Monaghan SJ et al. Acetylome profiling of Vibrio alginolyticus reveals its role in bacterial virulence. J Proteom. (2020) 211:103543. doi: 10.1016/j.jprot.2019.103543
13. Mao F, Liu KN, Wong NK, Zhang XY Yi WJ, Xiang ZM, et al. Virulence of Vibrio alginolyticus accentuates apoptosis and immune rigor in the Oyster Crassostrea hongkongensis. Front Immunol. (2021) 12:746017. doi: 10.3389/fimmu.2021.746017
14. Fu YW, Yao J, He MH, Wang ZP, Chen WF, Cui M, et al. Expression analysis and tissue localization of IgZ in the grouper Epinephelus coioides after Vibrio alginolyticus infection and vaccination. J Fish Dis. (2021) 44:1647–55. doi: 10.1111/jfd.13471
15. Wang FF, Huang L, Liao MQ, Dong WN, Liu C, Liu Y, et al. Integrative analysis of the miRNA-mRNA regulation network in hemocytes of Penaeus vannamei following Vibrio alginolyticus infection. Dev Comp Immunol. (2022) 131:104390. doi: 10.1016/j.dci.2022.104390
16. Baker-Austin C, Oliver JD, Alam M, Ali A, Waldor MK, Qadri F, et al. Vibrio spp. Infections. Nat Rev Dis Primers. (2018) 4:1–19. doi: 10.1038/s41572-018-0005-8
17. Wang JX, Ding Q, Yang QK, Fan H, Yu GL, Liu FX, et al. Vibrio alginolyticus triggers inflammatory response in mouse peritoneal macrophages via activation of NLRP3 inflammasome. Front Cell Infect Microbiol. (2021) 11:769777. doi: 10.3389/fcimb.2021.769777
18. Zhou MQ, Huang YP, Zhang YX, Wang QY, Ma Y, Shao S. Roles of virulence regulator ToxR in viable but non-culturable formation by controlling reactive oxygen species resistance in pathogen Vibrio alginolyticus. Microbiol Res. (2022) 254:126900. doi: 10.1016/j.micres.2021.126900
19. Cai SH, Lu YS, Jian JC, Wang B, Huang YC, Tang JF, et al. Protection against Vibrio alginolyticus in crimson snapper Lutjanus erythropterus immunized with a DNA vaccine containing the ompW gene. Dis Aquat Organ. (2013) 106:39–47. doi: 10.3354/dao02617
20. Luo G, Huang LX, Su YQ, Qin YX, Xu XJ, Zhao LM, et al. flrA, flrB and flrC regulate adhesion by controlling the expression of critical virulence genes in Vibrio alginolyticus. Emerg Microbes Infect. (2016) 5:e85. doi: 10.1038/emi.2016.82
21. Zhou SH, Tu XT, Pang HY, Hoare R, Monaghan SJ, Luo JJ, et al. A T3SS regulator mutant of Vibrio alginolyticus affects antibiotic susceptibilities and provides significant protection to Danio rerio as a live attenuated vaccine. Front Cell Infect Microbiol. (2020) 10:183. doi: 10.3389/fcimb.2020.00183
22. Zhao Z, Liu JX, Deng YQ, Huang W, Ren CH, Call DR, et al. The Vibrio alginolyticus T3SS effectors, Val1686 and Val1680, induce cell rounding, apoptosis and lysis of fish epithelial cells. Virulence. (2018) 9:318–30. doi: 10.1080/21505594.2017.1414134
23. Rivera-Cancel G, Orth K. Biochemical basis for activation of virulence genes by bile salts in Vibrio parahaemolyticus. Gut Microbes. (2017) 8:66–373. doi: 10.1080/19490976.2017.1287655
24. Letchumanan V, Chan KG, Khan TM, Bukhari SI, Ab Mutalib NS, Goh BH, et al. Bile sensing: the activation of Vibrio parahaemolyticus virulence. Front Microbiol. (2017) 8:728. doi: 10.3389/fmicb.2017.00728
25. Oberkampf M, Hamiot A, Altamirano-Silva P, Bellés-Sancho P, Tremblay YD, DiBenedetto N, et al. c-di-AMP signaling is required for bile salt resistance, osmotolerance, and long-term host colonization by Clostridioides difficile. Sci Signal. (2022) 15:eabn8171. doi: 10.1126/scisignal.abn8171
26. Rosenberg EY, Bertenthal D, Nilles ML, Bertrand KP, Nikaido H. Bile salts and fatty acids induce the expression of Escherichia coli AcrAB multidrug efflux pump through their interaction with Rob regulatory protein. Mol Microbiol. (2003) 48:1609–19. doi: 10.1046/j.1365-2958.2003.03531.x
27. Li P, Rivera-Cancel G, Kinch LN, Salomon D, Tomchick DR, Grishin NV, et al. Bile salt receptor complex activates a pathogenic type III secretion system. Elife. (2016) 5:e15718. doi: 10.7554/eLife.15718
28. Ma QZ, Pan YY, Chen Y, Yu SX, Huang J, Liu YQ, et al. Acetylation of glucosyltransferases regulates Streptococcus mutans biofilm formation and virulence. PLoS Pathog. (2021) 17:e1010134. doi: 10.1371/journal.ppat.1010134
29. Li JH, Liu ST, Su Y, Ren J, Yu S, Ni JJ, et al. Acetylation of PhoP K88 is involved in regulating Salmonella virulence. Infect Immun. (2021) 89:e00588–20. doi: 10.1128/IAI.00588-20
30. Liu WB, Tan YF, Cao SY, Zhao HH, Fang HH, Yang XY, et al. Protein acetylation mediated by YfiQ and CobB is involved in the virulence and stress response of Yersinia pestis. Infect Immun. (2018) 86:e00224–18. doi: 10.1128/IAI.00224-18
31. Cai SH, Wu ZH, Jian JC, Lu YS. Cloning and expression of the gene encoding an extracellular alkaline serine protease from Vibrio alginolyticus strain HY9901, the causative agent of vibriosis in Lutjanus erythopterus (Bloch). J Fish Dis. (2007) 30:493–500. doi: 10.1111/j.1365-2761.2007.00835.x
32. García K, Yáñez C, Plaza N, Peña F, Sepúlveda P, Pérez-Reytor D, et al. Gene expression of Vibrio parahaemolyticus growing in laboratory isolation conditions compared to those common in its natural ocean environment. BMC Microbiol. (2017) 17:118. doi: 10.1186/s12866-017-1030-6
33. Ma J, Chen T, Wu S, Yang CY, Bai MZ, Shu KX, et al. iProX: an integrated proteome resource. Nucleic Acids Res. (2019) 47:D1211–17. doi: 10.1093/nar/gky869
34. Chou KC, Shen HB. Cell-PLoc 20: an improved package of web-servers for predicting subcellular localization of proteins in various organisms. Nat Protoc. (2010) 2:1090–103. doi: 10.4236/ns.2010.210136
35. Bernal V, Castaño-cerezo S, Gallego-jara J, Écija-Conesa A, Diego TD, Iborra JL, et al. Regulation of bacterial physiology by lysine acetylation of proteins. Nat Biotechnol. (2014) 31:586–95. doi: 10.1016/j.nbt.2014.03.002
36. Pang R, Li Y, Liao K, Guo PH Li YP, Yang XJ, et al. Genome- and proteome- wide analysis of lysine acetylation in Vibrio vulnificus Vv180806 reveals its regulatory roles in virulence and antibiotic resistance. Front Microbiol. (2020) 11:591287. doi: 10.3389/fmicb.2020.591287
37. Kaur G, Ali SA, Kumar S, Mohanty AK, Behare P. Label-free quantitative proteomic analysis of Lactobacillus fermentum NCDC 400 during bile salt exposure. J Proteom. (2017) 167:36–45. doi: 10.1016/j.jprot.2017.08.008
38. Chen MJ, Tang HY, Chiang ML. Effects of heat, cold, acid and bile salt adaptations on the stress tolerance and protein expression of kefir-isolated probiotic Lactobacillus kefiranofaciens M1. Food Microbiol. (2017) 66:20–7. doi: 10.1016/j.fm.2017.03.020
39. Lv LX, Yan R, Shi HY, Shi D, Fang DQ, Jiang HY, et al. Integrated transcriptomic and proteomic analysis of the bile stress response in probiotic Lactobacillus salivarius LI01. J Proteom. (2017) 150:216–29. doi: 10.1016/j.jprot.2016.08.021
40. Burns P, Sánchez B, Vinderola G, Ruas-Madiedo P, Ruiz L, Margolles A, et al. Inside the adaptation process of Lactobacillus delbrueckii subsp. lactis to bile. Int J Food Microbiol. (2010) 142:132–41. doi: 10.1016/j.ijfoodmicro.2010.06.013
41. Xie LX, Wang XB, Zeng J, Zhou ML, Duan XK Li QM, et al. Proteome-wide lysine acetylation profiling of the human pathogen Mycobacterium tuberculosis. Int J Biochem Cell Biol. (2015) 59:193–202. doi: 10.1016/j.biocel.2014.11.010
42. Meng QG, Liu P, Wang J, Wang YH, Hou LB, Gu W, et al. Systematic analysis of the lysine acetylome of the pathogenic bacterium Spiroplasma eriocheiris reveals acetylated proteins related to metabolism and helical structure. J Proteom. (2016) 148:159–69. doi: 10.1016/j.jprot.2016.08.001
43. Liu YT, Pan Y, Lai FB, Yin XF, Ge RG, He QY. Comprehensive analysis of the lysine acetylome and its potential regulatory roles in the virulence of Streptococcus pneumoniae. J Proteom. (2018) 176:46–55. doi: 10.1016/j.jprot.2018.01.014
44. Lei L, Zeng JM, Wang LY, Gong T, Zheng, Qiu W, et al. Quantitative acetylome analysis reveals involvement of glucosyltransferase acetylation in Streptococcus mutans biofilm formation. Environ Microbiol Rep. (2020) 13:86–97. doi: 10.1111/1758-2229.12907
45. Li Y, Xue H, Bian DR, Xu GT, Piao CG. Acetylome analysis of lysine acetylation in the plant pathogenic bacterium Brenneria nigrifluens. Microbiologyopen. (2020) 9:e00952. doi: 10.1002/mbo3.952
46. Guo LN, Huang LX, Su YQ, Qin YX, Zhao LM, Yan QP. secA, secD, secF, yajC, and yidC contribute to the adhesion regulation of Vibrio alginolyticus. Microbiologyopen. (2018) 7:e00551. doi: 10.1002/mbo3.551
47. Weng YD, Fields EG, Bina TF, Budnick JA, Kunkle DE, Bina XR, et al. Vibrio cholerae TolC is required for expression of the ToxR regulon. Infect Immun. (2021) 89:e0024221. doi: 10.1128/IAI.00242-21
48. Lembke M, Höfler T, Walter AN, Tutz S, Fengler V, Schild S, et al. Host stimuli and operator binding sites controlling protein interactions between virulence master regulator ToxR and ToxS in Vibrio cholerae. Mol Microbiol. (2020) 114:262–78. doi: 10.1111/mmi.14510
49. Bina TF, Kunkle DE, Bina XR, Mullett SJ, Wendell SG, Bina JE. Bile salts promote ToxR regulon activation during growth under virulence-inducing conditions. Infect Immun. (2021) 89:e0044121. doi: 10.1128/IAI.00441-21
50. Kalindamar S, Abdelhamed H, Kordon AO, Pinchuk LM, Karsi A. Hemolysin co-regulated family proteins Hcp1 and Hcp2 contribute to Edwardsiella ictaluri pathogenesis. Front Vet Sci. (2021) 8:681609. doi: 10.3389/fvets.2021.681609
51. Wang NN, Liu J, Pang MD, Wu YF, Awan FQ Liles MR, Lu CP, et al. Diverse roles of Hcp family proteins in the environmental fitness and pathogenicity of Aeromonas hydrophila Chinese epidemic strain NJ-35. Appl Microbiol Biotechnol. (2018) 102:7083–95. doi: 10.1007/s00253-018-9116-0
52. Matilla MA, Krell T. The effect of bacterial chemotaxis on host infection and pathogenicity. FEMS Microbiol Rev. (2018) 42:40–67. doi: 10.1093/femsre/fux052
53. Corral J, Sebastià P, Coll NS, Barbé J, Aranda J, Valls M. Twitching and swimming motility play a role in Ralstonia solanacearum pathogenicity. mSphere. (2020) 5:e00740–19. doi: 10.1128/mSphere.00740-19
54. Floyd KA, Lee CK, Xian W, Nametalla M, Valentine A, Crair B, et al. c-di-GMP modulates type IV MSHA pilus retraction and surface attachment in Vibrio cholerae. Nat Commun. (2020) 11:1549. doi: 10.1038/s41467-020-15331-8
55. Hughes HQ, Floyd KA, Hossain S, Anantharaman S, Kysela DT, Zöldi M, et al. Nitric oxide stimulates type IV MSHA pilus retraction in via activation of the phosphodiesterase CdpA. Proc Natl Acad Sci USA. (2022) 119:e2108349119. doi: 10.1073/pnas.2108349119
56. Wang YB, Wang FF, Bao XY, Fu LL. Systematic analysis of lysine acetylome reveals potential functions of lysine acetylation in Shewanella baltica, the specific spoilage organism of aquatic products. J Proteom. (2019) 205:103419. doi: 10.1016/j.jprot.2019.103419
57. Yang YJ, Zhang H, Guo ZY, Zou SW, Long F, Wu JC, et al. Global insights into lysine acylomes reveal crosstalk between lysine acetylation and succinylation in Streptomyces coelicolor metabolic pathways. Mol Cell Proteom. (2021) 20:100148. doi: 10.1016/j.mcpro.2021.100148
58. Tiwari N, Miguelromero L, Kulhankova K, Cahill MP, Tran PM, Kinney KJ, et al. The SrrAB two-component system regulates Staphylococcus aureus pathogenicity through redox sensitive cysteines. Proc Natl Acad Sci USA. (2020) 117:10989–99. doi: 10.1073/pnas.1921307117
59. DeMars Z, Bose JL. Redirection of metabolism in response to fatty acid kinase in Staphylococcus aureus. J Bacteriol. (2018) 200:e00345-18. doi: 10.1128/JB.00345-18
Keywords: Vibrio alginolyticus, bile salt, acetylome, post-translational modification, virulence
Citation: Xiao X, Li W, Pan Y, Wang J, Wei Z, Wang S, Wang N, Jian J and Pang H (2023) Holistic analysis of lysine acetylation in aquaculture pathogenic bacteria Vibrio alginolyticus under bile salt stress. Front. Vet. Sci. 10:1099255. doi: 10.3389/fvets.2023.1099255
Received: 15 November 2022; Accepted: 11 April 2023;
Published: 27 April 2023.
Edited by:
Win Surachetpong, Kasetsart University, ThailandReviewed by:
Manjun Yang, Sun Yat-sen University, ChinaCopyright © 2023 Xiao, Li, Pan, Wang, Wei, Wang, Wang, Jian and Pang. This is an open-access article distributed under the terms of the Creative Commons Attribution License (CC BY). The use, distribution or reproduction in other forums is permitted, provided the original author(s) and the copyright owner(s) are credited and that the original publication in this journal is cited, in accordance with accepted academic practice. No use, distribution or reproduction is permitted which does not comply with these terms.
*Correspondence: Huanying Pang, cGh5aW5nMTIxOEAxNjMuY29t
†These authors have contributed equally to this work and share first authorship
Disclaimer: All claims expressed in this article are solely those of the authors and do not necessarily represent those of their affiliated organizations, or those of the publisher, the editors and the reviewers. Any product that may be evaluated in this article or claim that may be made by its manufacturer is not guaranteed or endorsed by the publisher.
Research integrity at Frontiers
Learn more about the work of our research integrity team to safeguard the quality of each article we publish.