- 1Institute of Goats and Sheep Science, Nanjing Agricultural University, Nanjing, Jiangsu, China
- 2Agricultural and Rural Science & Technology Service Center, and Enterprise Graduate Workstation, Taicang, China
- 3Jiangsu Qianbao Animal Husbandry Co., Ltd, Yancheng, Jiangsu, China
Introduction: This study aims to investigate the long-term effects of spirulina supplementation in a high-fat diet (HFD) on rumen morphology, rumen fermentation, and the composition of rumen microbiota in lambs. Spirulina is a blue-green microalgae that has been shown to have high nutritional value for livestock.
Methods: Fifty-four lambs were randomly divided into three groups: a normal chow diet (NCD) group, a high-fat diet (HFD) group, and a high-fat diet supplemented with 3% spirulina (HFD+S) group. Rumen morphology, rumen fermentation, and rumen microbiota were analyzed at the end of the study.
Results: Spirulina supplementation improved the concentration of volatile fatty acids and rumen papilla length. Additionally, there was a tendency for an increase in rumen weight and an upregulation of the genes Claudin-1, Claudin-4, and Occludin in the HFD+S group. Pyrosequencing of the 16S ribosomal RNA gene also showed that spirulina supplementation significantly changed the rumen microbiota composition in the HFD group, with a decrease in richness and diversity. Specifically, the relative abundance of Prevotella 9 and Megasphaera was significantly increased in the HFD group compared to the NCD group, while spirulina supplementation reversed these changes.
Discussion: This study suggests that 3% spirulina supplementation can improve rumen development and fermentation, and effectively relieve rumen microbe disorders in lambs caused by a high-fat diet. However, further research is needed to confirm the findings and to examine the long-term effects of spirulina supplementation in different types of livestock and under different dietary conditions.
Introduction
Spirulina is a functional additive that contains several active components, such as phenolic acids, beta-carotene, vitamins, minerals, tocopherols, fatty acids, and gamma-linolenic acid (1, 2). It belongs to the Oscillatoriaceae family. It is also rich in antioxidants, including essential amino acids (3–5). Spirulina also reduce the blood lipid content through its rich content of gamma-linolenic acid (6). Based on these nutritional benefits, spirulina is now used as a food supplement for both humans and livestock. Spirulina can be used as an amino acid supplement in poultry and piglet diets and is effective in relieving sows from nutritional metabolism disorders due to gestation (7–9). A previous study showed that it was beneficial to lamb growth when they were fed 20% spirulina supplementation (10). In addition, our previous study found that adding 3% spirulina to a high-energy diet could improve the immune and antioxidant capacity of sheep and alleviate lipid metabolism disorder (6). Researchers have also considered using spirulina to reduce obesity-associated chronic inflammatory states (11–13). However, the effects of spirulina in the diet on rumen microbiota remain unknown.
The rumen development of lambs can be divided into three stages: the non-rumination stage from birth to 3 weeks of age, the transition stage from 3 to 8 weeks of age, and the rumination stage after 8 weeks of age (14). The morphological development of rumen epithelium in ruminants may be accompanied by molecular adaptations of nutrient absorption and metabolism (15). The diversity of the rumen microbial community is one of the main ways to understand rumen function (16). Microbes are important for animal productivity because they help degrade carbohydrates, which are then turned into volatile fatty acids (VFA) to supply energy for metabolic functions (17). The rumen microbiota also plays a vital role in the metabolism of fatty acids in dietary fat (18, 19).
According to the previous results of our team, high fat diet has significant effect on growth performance in Hu sheep. High fat diet obviously downregulated average daily feed intake and feed/gain ratio whereas upregulated the GR value (6). Some studies have connected a high-fat diet to the development of gastrointestinal diseases (20, 21). However, whether spirulina supplementation can ameliorate the negative effects of rumen morphology and the ruminal bacteria imbalance caused by consuming high-fat diets remains poorly understood. Therefore, the effects of dietary spirulina supplementation on rumen morphology, fermentation, and microbiota composition were examined in Hu sheep fed an HFD diet.
Materials and methods
The management of animals and experimental design
All animal experimental procedures were approved by the Ethics Committee of Nanjing Agricultural University, China (Approval ID: SYXK2011-0036). A total of 54 male lambs without castration (27.5 ± 1.78 kg) at 3 month of age were raised at Qidong Ruipeng Animal Husbandry, Jiangsu Province, China. The lambs were placed into three random groups: a normal chow diet (NCD), high-fat diet (HFD), and high-fat diet supplemented with 3% spirulina (HFD + S). There were three replicates per group and six lambs per replicate. Three percent fat was added to the high-fat diet to increase the energy level. The floors, walls, and fences of the lamb house were disinfected. Vaccination, parasites, and other prophylactic measures were carried out during the prefeeding to make sure that the lambs were healthy. The experiment continued for 74 days, including a 14 days adaptation period. The feed formulation described by Liang et al. (6) was carried out and is presented in Table 1. Powdered spirulina was purchased from a commercial supplier (Ordos Mengjian Spirulina Co., Ltd., Inner Mongolia, China).
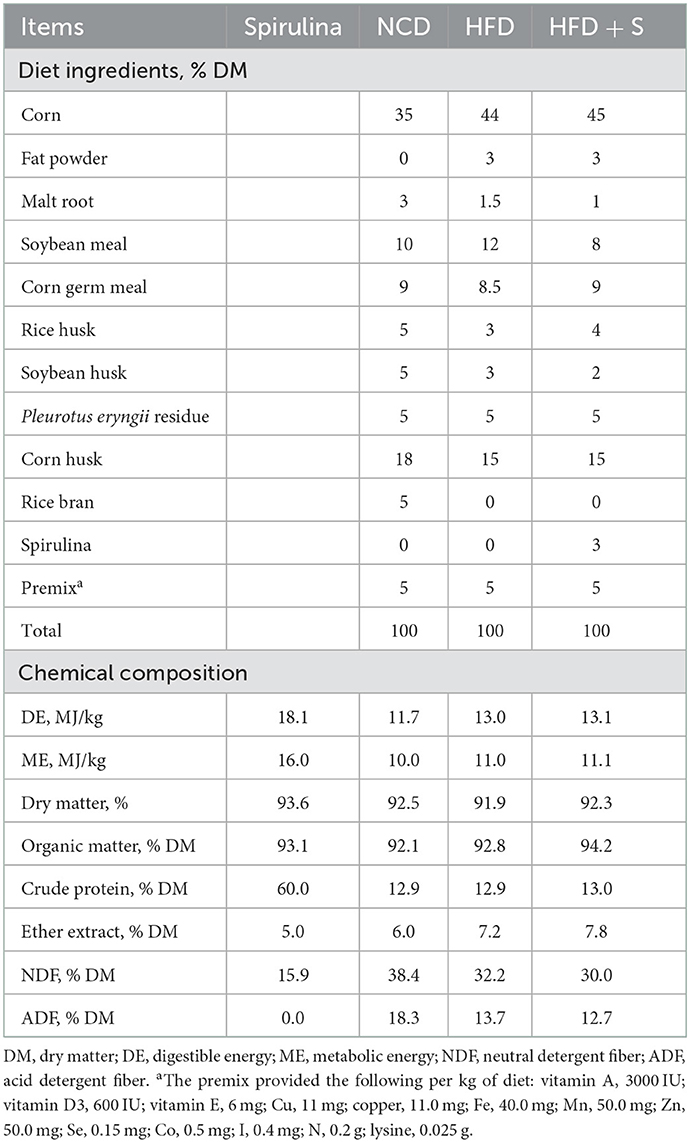
Table 1. Diet ingredients and chemical composition from Hu sheep fed standard (ST) and high-energy (HE) diets divided in supplemented subgroups: control and spirulina experimental diets.
Sample collection
After 2 months of feeding, five Hu sheep with similar body weights and body conditions were randomly selected from each group and slaughtered without feeding. Immediately after slaughter, a representative sample of rumen digestive fluid (at least 200 ml) was collected to determine the pH value of rumen fluid by pH meter (Code: fc230b, Hanna, Italy). Then the rumen fluid was filtered through four layers of cheesecloth and stored at −20°C for VFA concentration analysis (22). Rumen tissues were collected and fixed with 4% paraformaldehyde for histomorphological analysis. At the same time, the rumen digesta samples were thoroughly mixed well, collected into a 5 ml cryopreservation tube, and stored at −80°C for further analysis.
Analysis of rumen tissue morphology and rumen fermentation parameters
The VFA concentration in the rumen fluid was analyzed according to the method reported by Feng and Gao (23). Gas chromatography (GC-14B; Shimadzu, Japan; capillary column film thickness: 30 m × 0.32 mm × 0.25 μm; column temperature = 130°C; injector temperature = 180°C; detector temperature = 180°C) was used to determine VFA, which has been used in prior experiments (24). Crotonic acid was used as the internal standard.
The morphology of the rumen was assessed using the method described by Ye et al. (25). Rumen tissues were washed in phosphate buffer saline (PBS) and fixed in 4% formaldehyde before being embedded in paraffin and stained with hematoxylin and eosin. With 40 × objective lens, measurements of lesions were taken. Each lamb sample in one group received five slides, with each slide capturing two photographs. We measured the predefined criteria described earlier, using Image Pro Plus software (Media Cybernetics, Bethesda, MD, USA) (26). During the analysis, three extreme samples were removed from each group, and there were altogether 36 samples.
DNA extraction and high-throughput sequencing
The lamb rumen content sample was collected for microbial profile analysis and stored at −80°C until analysis. The DNA was isolated using MN NucleoSpin 96 Soi (MN-MACHEREY-NAGEL, Germany). With the primers 338F (5′ ACTCCTACGGGAGGCAGCA-3′); 806R (5′-GGACTACHVGGGTWTCTAAT-3′) and the cycling settings used by Hu et al. (22), the V3–V4 regions of the bacterial 16S RNA gene were amplified. PCR products were sequenced on the Illumina MiSeq platform by high-throughput pyrosequencing at Biomarker Technologies Co., Ltd. (Beijing, China). Trimmomatic V0.33 software filtered the raw reads obtained by sequencing. Cutadapt 1.9.1 software was used to identify and remove primer sequences, and clean reads without primer sequences were obtained. To acquire the final effective readings, they were grouped into operational taxonomic units with 97% similarity (OTU). The chimeric sequences were identified and deleted using the UCHIME V4.2 program.
RNA isolation, cDNA synthesis and qPCR
The Trizol method described by Liang was used to extract total RNA from ruminal tissue (6). The RNA concentration was then quantified using a NanoDrop 2000 Spectrophotometer (Thermo Scientific, Waltham, MA, USA). The absorption ratio (260/280 nm) of all samples was between 1.8 and 2.1, indicating high RNA purity. Total RNA was used for reverse transcription using a PrimeScript® RT reagent kit with gDNA Eraser (Takara Bio, Otsu, Japan). The expression of the target genes was determined using the QuantStudio 5 Real-time PCR Instrument (Applied Biosystems, Foster, California, USA) with fluorescence detection of AceQ qPCR SYBR Green Master Mix (Vazyme Biotech, Nanjing, China) under the standard program. The data of the gene expression were normalized by the housekeeping gene (actin beta, ACTB) using the 2−ΔΔCT method. The primers and amplicon sizes of the genes are shown in Supplementary Table S1.
Statistical analysis
The basic data (rumen papillae, thickness, total volatile fatty acid content and pH) were subjected to one-way analyses of variance (ANOVA) run using Duncan's test. The data were processed as mean values ± SEM. P < 0.05 was considered to be significantly different. The analysis was performed using SPSS 25.0 (IBM Corp., Armonk, NY, USA).
Results
Effect of spirulina supplementation on rumen development of Hu sheep fed with HFD
The rumen development characteristics of Hu sheep fed different diets are shown in Figure 1 and Table 2. Compared with the HFD group, there was no significant difference in rumen weight (emptied rumen weight and relative weight) between the NCD group and the HFD + S group, but there was an increasing trend (P > 0.05). However, the ruminal papilla length (P < 0.05), the thickness of total epithelial (P < 0.05), and stratum corneum (SC, P < 0.05) in the HFD group were significantly lower than those in the other two groups. The thickness of stratum granulosum (SG, P < 0.001) in the NCD group were significantly higher than other two groups, but the thickness of stratum spinosum and basale were not significantly different among the three groups (SS + SB, P > 0.05; Table 2).
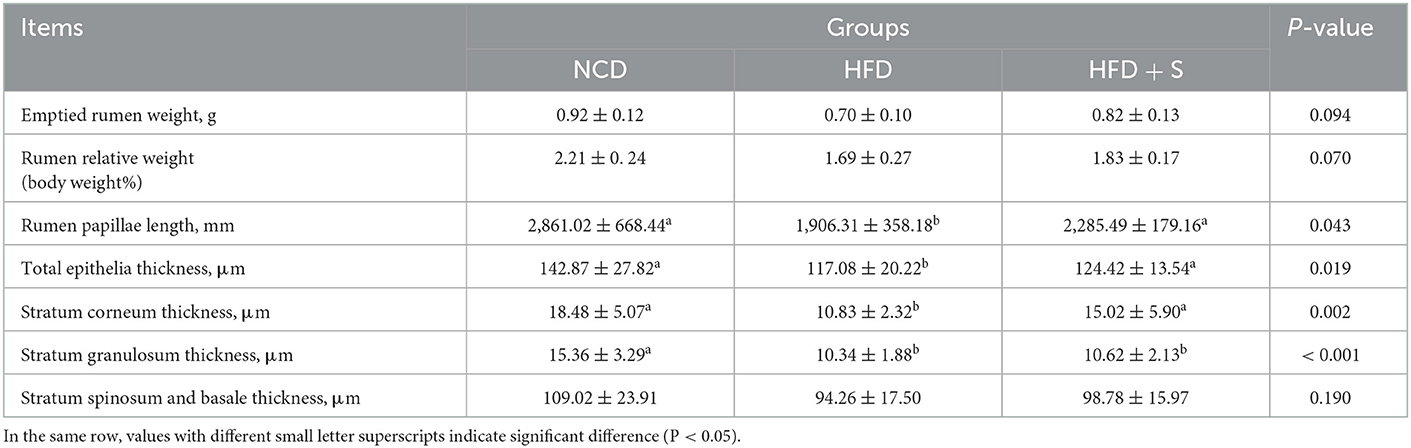
Table 2. Effect of spirulina supplementation on the growth and development of rumen and rumen papillae of Hu sheep fed HDF.
Effect of spirulina supplementation on rumen fermentation of Hu sheep fed with HFD
The phenotypic characteristics of rumen fermentation in Hu sheep in the three groups are shown in Table 3. Compared whith the NCD group, HFD treatment significantly decreased overall VFA, acetate, propionate, and butyrate concentrations while significantly increasing the acetate to propionate ratio (P < 0.05). Furthermore, spirulina supplementation could ameliorate the effect of an HFD on rumen fermentation. There were no significant variations in the pH value or the concentrations of isobutyrate, valerate, and isovalerate among the three groups (P > 0.05).
Effect of spirulina supplementation on rumen microbiota diversity of Hu sheep fed with HFD
To examine the effect of spirulina supplementation on ruminal microbiota, ruminal bacterial communities were determined by Illumina HiSeq sequencing of the 16S RNA V3–V4 region. As shown in Supplementary Table S2, 1,198,763 raw reads were obtained from the high-throughput sequencing library. Clean reads were obtained through quality filtering using QIIME1, 1,178,220. A total of the effective read clustered into operational taxonomic units (OTUs), and OTUs were formed at the 97% similarity level. All the rarefaction curves tended to approach the plateau (Supplementary Figure S1). Notably, the analysis of OTUs number (P = 0.0149), ACE (P = 0.0011) and Chao1 (P = 0.0001) indicated that rumen bacterial diversity was reduced in both HFD and HFD + S groups (Figure 2A). Furthermore, based on the analysis of Bray-Curits Metric and Principal coordinates (R = 0.296, P = 0.002), the difference in rumen microbiota composition was found among the three groups (Figure 2B). Furthermore, a Venn diagram analysis showed 411 common core OTUs in the three groups, as well as 31 and 6 unique OTUs in the NCD and HFD groups, respectively (Figure 2C). These findings showed that HFD feeding affected the microbiological composition of rumen contents, but HFD + S had no effect on their species (P > 0.05).
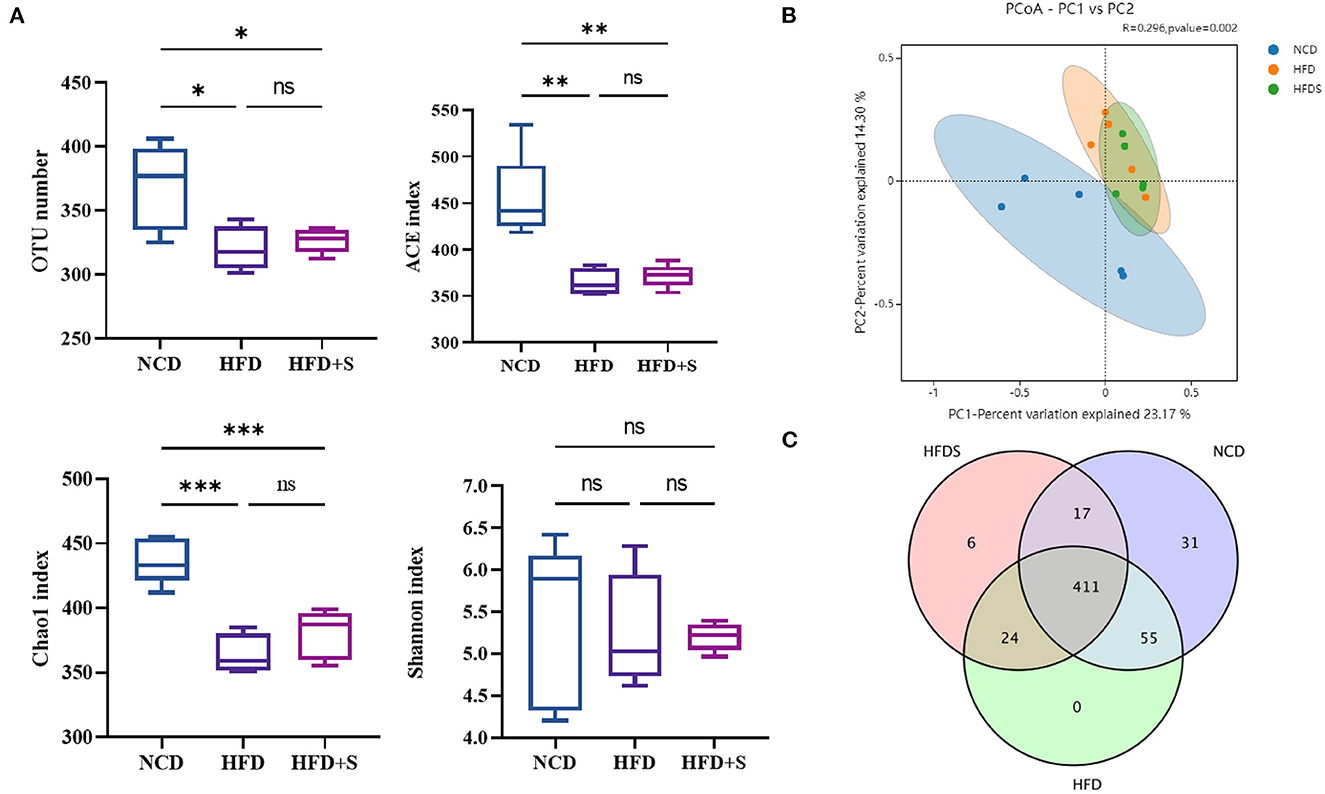
Figure 2. Effect of high-fat diet and spirulina supplementation on the rumen bacterial communities' diversity. (A) Comparison of the OUT number, ACE, Chao1 and Shannon of the α-diversity in NCD, HFD and HFD + S groups. (B) Bacterial communities PCoA based on the OUT level. (C) Based on the OUT level, Venn diagrams. “*” represents a significant correlation (P < 0.05), “**” represents an extremely significant correlation (P < 0.01), “***” represents an extremely significant correlation (P < 0.001).
Effect of spirulina supplementation on the bacterial composition of Hu sheep fed HFD
A total of 18 phyla and 158 genera were found in the rumen microbiota. As shown in Figure 3A, at the phylum level, four major dominant phyla were identified in three groups: Firmicutes, Bacteroidetes, Proteobacteria and Actinobacteria. The phylum level analysis indicated that HFD and HFD+S feeding obviously increased the relative abundance of Proteobacteria and decreased Firmicutes level (P < 0.05), while spirulina supplementation significantly ameliorated the influences of these bacteria caused by HFD treatment (P < 0.05). To further identify the differences among each group, a genus-level analysis was performed. As observed in Figure 3B, the relative abundance of Prevotella_9 and Megasphaera significantly increased in the HFD group compared with the NCD and HFD + S groups (P < 0.05).
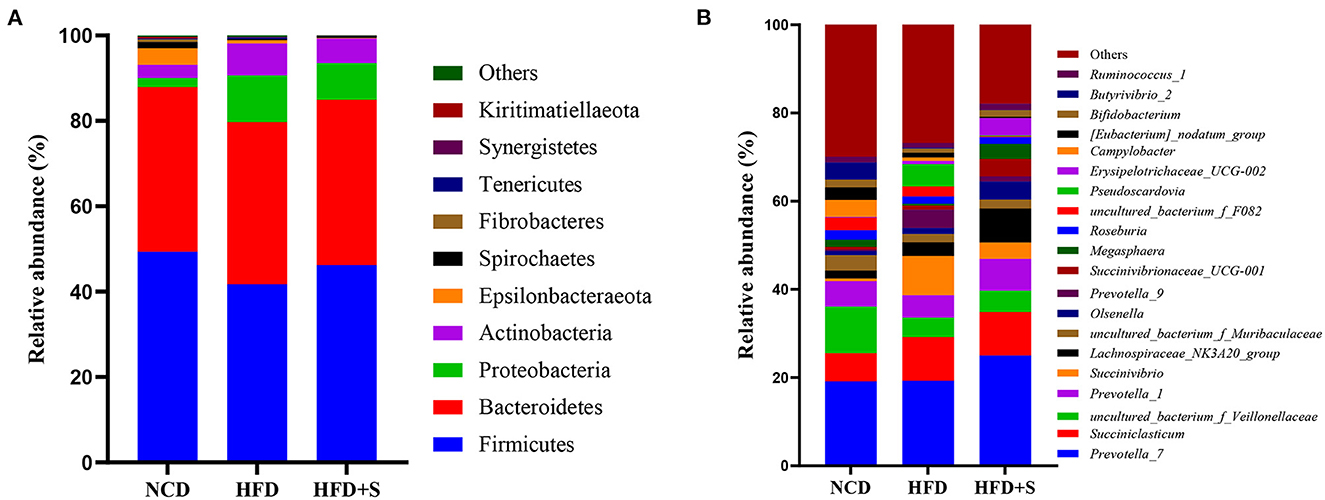
Figure 3. The taxonomic profiles for the relative abundance in the ruminal digesta of each group. (A) The relative phylum-level abundance in the ruminal digesta. (B) The relative genus-level abundance in the ruminal digesta.
Effect of spirulina supplementation on rumen microbiota's key phylotypesbacteria composition in HFD-fed sheep
The OTUs of each group were compared to identify the significantly abundant bacterial groups under different diet treatments. Biomarker detection was performed using linear discriminant analysis (LDA) LEfSe with a 3.5-threshold value at the genus level (Figures 4A, B). The relative abundance of Butyrivibrio_2, Lachnospiraceae_UCG_008, Fibrobacter, and Saccharofermentans were significantly increased in the NCD group; Succinivibrio, CAG_352, and Pseudoscardovia were significantly increased in the HFD group; Erysipelotrichaceae_UCG_002, Dialister, and Mitsuokella were significantly increased in the HFD + S group (Figures 4C–J).
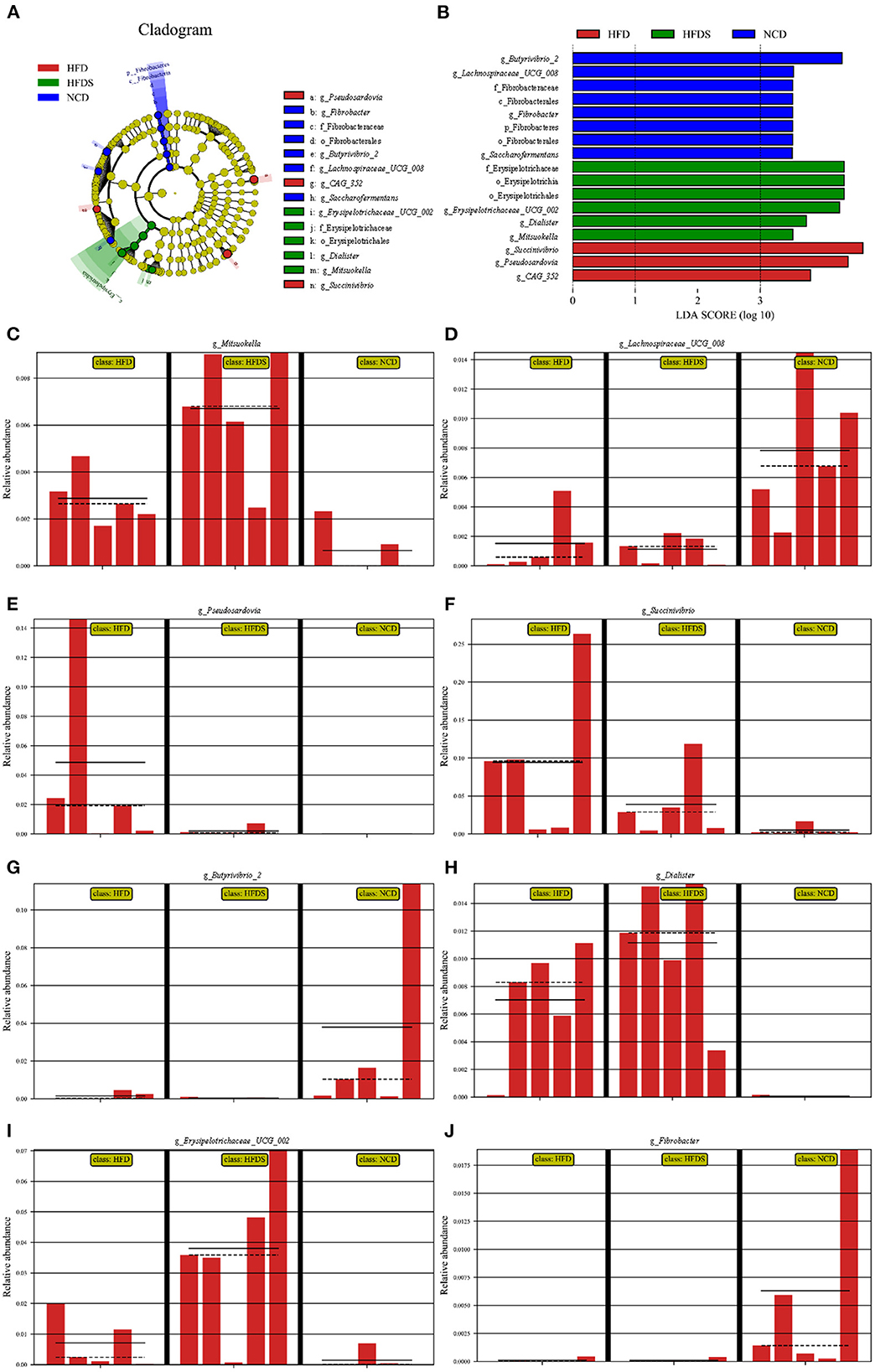
Figure 4. High-fat feeding and spirulina supplementation are associated with multiple bacteria. (A) The taxonomic cladogram was obtained from LEfSe analysis. (B) Linear discriminant (LDA) and effect size (LEfSe) analysis of the three groups. (C–J) The relative abundance of bacterial obtained in rumen microbiota from the LefSe results.
The relationship between the ruminal bacterial community and fermentation parameters in Hu sheep
The concentrations of acetate, propionate, isobutyrate, butyrate, isovalerate, valerate, total VFA, and the acetate to propionate ratio in the rumen liquid were measured to assess the relationship between the top 15 relative abundance ruminal bacterial communities and the composition of VFA in the rumen liquid. We found five significant positive correlations, four significant negative correlations, and three highly significant negative correlations in the correlation analysis between the ruminal bacterial community and the fermentation parameters. Prevotella_1 had a significantly negative correlation with valerate content (P < 0.05); Succinivbrio had a negative correlation with total VFA, acetate, and propionate concentrations (P < 0.05); Megasphaera and Erysipelotrichaceae_UCG-002 had a significantly positive correlation with isovalerate concentration (P < 0.05); Ruminococcus_1 had a negative correlation with butyrate and valerate concentrations (Figure 5).
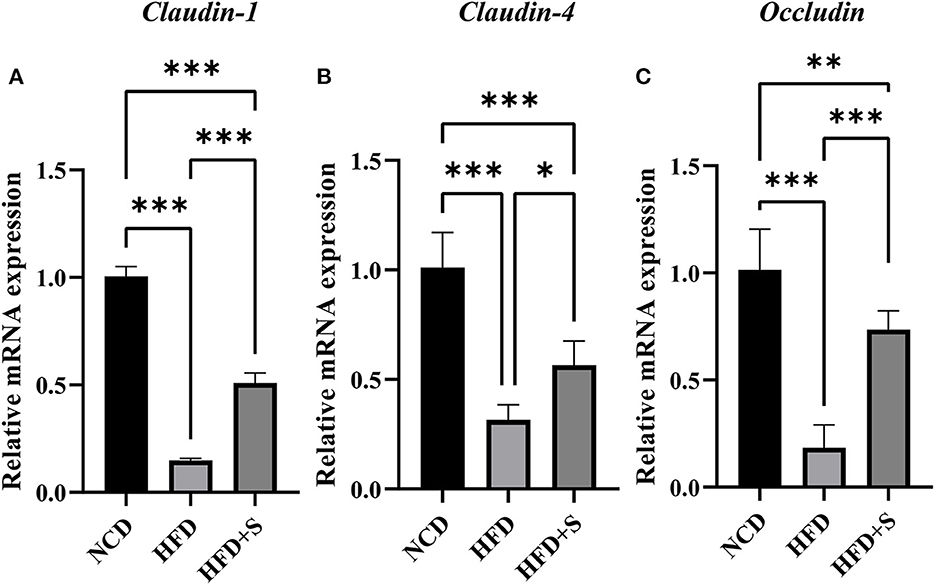
Figure 5. (A–C) Relationship between changes of microbial community and fermentation parameters of the ruminal digesta. “*” represents a significant correlation (P < 0.05), “**” represents an extremely significant correlation (P < 0.01), “***” represents an extremely significant correlation (P < 0.001).
Effect of spirulina supplementation on rumen tight junction proteins of Hu sheep fed HFD
As shown in Figure 6, the mRNA expression of tight junction proteins Claudin-1, Claudin-4 and Occludin in rumen were significantly decreased (P < 0.01) by high-fat diets compared with the NCD, while these were significantly increased by spirulina supplementation in the HFD + S group (P < 0.05).
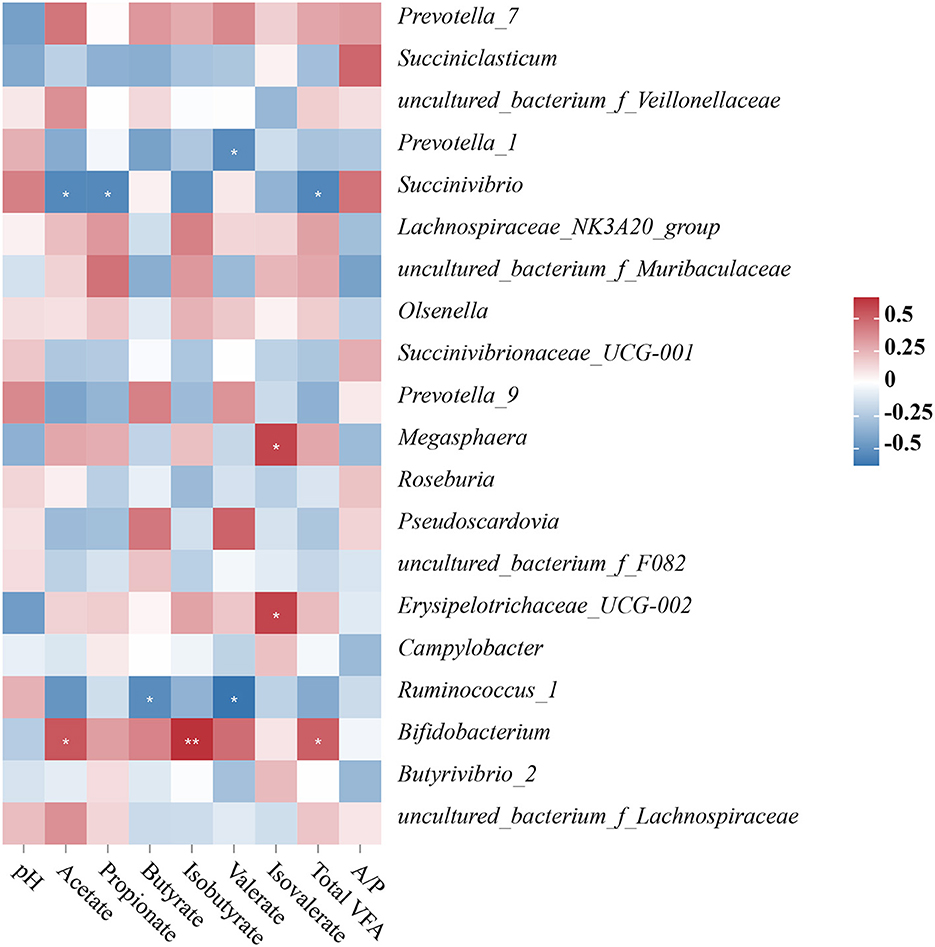
Figure 6. Changes in relative mRNA expression of genes related to the tight junction of ruminal tissue in sheep with normal chow diet (NCD), high-fat diet (HFD) and HFD diet supplemented in spirulina (HFS + S). “*” represents a significant correlation (P < 0.05), “**” represents an extremely significant correlation (P < 0.01).
Discussion
The rumen's development and morphology have an impact on lambs' digestibility and growth performance and could be measured by rumen weight and rumen papilla length (27, 28). There are several reports on nutrient levels or the composition of feeds that can affect the rumen histological morphology of ruminants (29–31). In the present study, compared with the control group, the ruminal papilla length, thickness of total epithelial, stratum corneum, and stratum granulosum were significantly lower in the HFD group, while the rumen's histological morphology showed no significant difference in the NCD and HFD + S groups, which indicated that 3% spirulina supplementation could ameliorate rumen disorder caused by an HFD diet. This result is also similar to a previous study in which HFD leads to damage to the digestive tract (32). Rumen pH and VFA are both important pointers of rumen fermentation in ruminants, which can reflect rumen wall function and regulate the internal environment acid–base balance (33, 34). In this study, there were no significant variations in pH across the three groups. This is most likely because the rumen wall has a restricted capacity to affect VFA transfer as well as the ability of ruminant saliva to dilute pH. Previous studies have shown that lipid supplementation did not affect the pH in the rumen, which might indicate that fiber digestion cellulolytic processes were unaffected or that microbes had adapted to the diet (35, 36). VFA played an important role in promoting rumen development (37). Thus, we analyzed the VFA concentrations in ruminal digesta. The results showed that HFD decreased the total VFA, acetate, propionate, butyrate and valerate concentrations in the rumen, while they were increased by spirulina supplementation. Moreover, it has been recognized that VFA, a major product of microbial fermentation, has a wide range of effects on host physiology (38). Previous studies indicated that the type of feed, time of weaning, and microorganisms all have a role in the rumen growth process (39–41). The results of rumen weight, rumen papillae length, and thickness of ruminal epithelia showed that HFD inhibited rumen development in lambs and that spirulina supplementation into the HFD diet could alleviate it, which were consistent with previous studies (39, 40, 42, 43).
This research used 16S rRNA V3–V4 high-throughput sequencing technology to assess the microorganism diversity in high-fat diets supplemented with spirulina, which not only obtained relatively complete bacteria information but also reduced their separation and cloning error (27). In the present study, HFD changed the richness of rumen bacteria, which was confirmed by community richness estimates according to ACE and Chao1, while community diversity markers like Shannon were not affected. Meanwhile, the OTUs results suggested that HFD and spirulina supplementation could reduce the richness and maintain the relatively mature structure of the microbiota in the rumen of lambs. The Venn group figures and OTUs unweighted UniFrac PCoA further indicated differences in bacterial communities among the three groups, showing that HFD changed the composition of the ruminal digesta bacterial community. This change may be caused by a decrease in total VFA, leading to changes in the ruminal digesta bacterial community. Lipid supplementation altered the concentration of all quantified VFA in the rumen, which was triggered by a larger intake of total fatty acid (44).
The effect of hydrogenated fat on rumen microbiota is controversial. Some studies reported that the addition of saturated fatty acids had no significant effect on rumen microbiota (45–47), while some studies believed that excessive lipid supplementation would have adverse effects on rumen (19, 48). This may be due to the toxicity of large amounts of unsaturated fatty acids produced by the decomposition of excess fat in the rumen to microbiota especially cellulolytic bacteria. In this study, excessive addition of fat powder had a negative effect on rumen microbiota. It is worth noting that in this study, in order to ensure the same level of other nutrients (crude protein), more corn was added to the diet of the high-fat group. In our results, the adverse effects of the high-fat group on the rumen microbiota may also be caused by this reason. Previous studies have also reported that high-concentrate diet will damage the structure of the rumen microbiota and affect the rumen function (49, 50).
Studies have shown that in mammals, especially in ruminants, Firmicutes, Bacteroidetes, Actinobacteria, and Proteobacteria are the dominant bacteria (51, 52). The dominant bacteria analyzed were also similar in our study with other previous reports (53, 54). The level of Firmicutes in the NCD group was significantly higher, while the level of Proteobacteria was significantly lower than in the HFD and HFD + S groups. Firmicutes are the main bacteria that decompose fiber, including many bacteria that can decompose cellulose, and Proteobacteria is a sign of intestinal bacteria imbalance (55, 56). This result indicated that HFD might have a negative influence on rumen fiber digestion and ruminal microbiota balance. The relative abundances of Megasphaera and Prevotella_9 rose at the genus level in HFD-fed sheep. Several processes, including protein metabolism, carbohydrate metabolism, and lipid metabolism, were shown to be favorably linked with several Prevotella strains (57). Increases in energy content generated by lipid supplementation in the dietary treatments may be one explanation for the rise in Prevotella percentage in the HFD and HFD+S groups in this study. Megasphaera was well-known for being a powerful lactate utilizer in the rumen and for helping to avoid lactic acidosis (58–61). In addition, LEfSe analysis showed that the increase in the abundance of Megasphaera in the HFD and HFD+S groups further explained why feeding on a high-fat diet did not decrease ruminal pH.
This study performed a correlation analysis between the ruminal bacterial community and the fermentation parameters at the genus level. For example, Succinivibrio was adversely linked to total VFA, acetate, and propionate levels. Succinivibrio, as the producer of succinate and acetate, can be converted to propionate, which promotes the formation of bacterial proteins (27, 62, 63). Succinivibrio is a member of the Succinivibrionaceae family, and its main constituent is succinate, which is a precursor to propionate and acetate (64). Moreover, propionate is an important precursor of gluconeogenesis. A small amount of propionate absorbed by rumen epithelium is converted into lactic acid, and the rest enters the liver to generate glucose through gluconeogenesis or enters the tricarboxylic acid cycle for oxidation. A recent study reported that rumen epithelium development may be improved by propionate as a signaling molecule (65). In this study, the content of propionate in the rumen was consistent with the development trend of the rumen. Also, extensive studies have shown that dietary additives can effectively change rumen fermentation mode, promote propionic acid production, and inhibit methanogenesis (66, 67). According to other studies, spirulina is high in gamma-linolenic acid, and adding it to diet may effectively decrease methane production while increasing propionate synthesis (6, 68). In this study, the addition of spirulina significantly reduced acetate and propionate, but the relationship between spirulina and methane production needs to be further explored. Erysipelotrichaceae_UCG-002 and Megasphaera were positively correlated with the concentration of isovalerate. Previous studies found that members of the Erysipelotrichaceae family had a strong positive correlation with host cholesterol metabolites and high-fat or Western diet–feeding mice (18, 69–71). Bifidobacterium is a key probiotic for maintaining intestinal microbial equilibrium, forming a healthy gut barrier and lowering lipopolysaccharide levels (72, 73). Consistent with previous reports, Bifidobacterium was inversely associated with a high-fat diet (74–77). Furthermore, Bifidobacterium was positively connected to isobutyrate, total VFA, and acetate concentrations in the current investigation. These findings were consistent with prior research that indicated a link between Bifidobacterium and VFA (78, 79). Numerous studies have shown that VFAs are an important energy source for ruminants (80, 81). As a result, it was critical to investigate VFA metabolism, especially butyrate, which is also recognized as a rumen development stimulator (82, 83). Also, a previous study reported that rumen papillae width and length are the most significant pointers for rating rumen development (84). This study's results showed that HFD feeding reduced the content of butyrate in the rumen and inhibited the growth of the rumen papilla, but spirulina supplementation effectively alleviated this phenotype. Another study showed that butyrate could also promote the increase of rumen weight (85). In this study, the difference in rumen weight was not significant among the three groups. However, compared with the HFD group, rumen weight in the HFD+S group showed an upward trend.
Our findings also demonstrated that the HFD reduced Claudin-1, Claudin-4, and Occludin mRNA expression. Previous research has shown that VFA can increase the expression of Claudin-1, Claudin-4, and Occludin in the rumen epithelium, encouraging rumen papilla growth (86). These findings also support the deleterious effects of the HFD on rumen function and the beneficial benefits of spirulina supplementation. As a result of these observations, the HFD group's tight junction impairment might be due to rumen bacteria structural disorder, decreased fermentation function, and lower VFA concentration.
In conclusion, this study showed that HFD decreased the length of rumen papillae and total ruminal VFA concentrations in sheep and that spirulina supplementation could effectively alleviate these negative effects. This might be associated with the change in ruminal microbiota composition in sheep including the significant change in the relative abundance of Megasphaera and Prevotella_9. These results suggest beneficial effects of 3% spirulina supplementation on altering the diversity of ruminal microbiota. This study is meaningful for further exploring the regulation of rumen development and microbiota by spirulina supplementation in an HFD-fed diet.
Data availability statement
The original contributions presented in the study are publicly available. This data can be found at: NCBI, PRJNA513129 and SRP354618.
Ethics statement
The animal study was reviewed and approved by Ethics Committee of Nanjing Agricultural University, China. Written informed consent was obtained from the owners for the participation of their animals in this study.
Author contributions
ZWa and YL conceived and designed the experiments and wrote the paper. ZWa, YB, XY, and JL performed the experiments. ZWa analyzed the data. YZ and FW helped perform the analysis and with con-strictive discussions. ZWe and DW helped conducting the experiments. All authors read and approved the final manuscript.
Funding
This research was funded by Jiangsu Agricultural Industry Technology System (JATS [2022]478) and Project of Seed Industry Revitalization in Jiangsu Province (JBGS [2021]113).
Conflict of interest
DW was employed by Jiangsu Qianbao Animal Husbandry Co., Ltd.
The remaining authors declare that the research was conducted in the absence of any commercial or financial relationships that could be construed as a potential conflict of interest.
Publisher's note
All claims expressed in this article are solely those of the authors and do not necessarily represent those of their affiliated organizations, or those of the publisher, the editors and the reviewers. Any product that may be evaluated in this article, or claim that may be made by its manufacturer, is not guaranteed or endorsed by the publisher.
Supplementary material
The Supplementary Material for this article can be found online at: https://www.frontiersin.org/articles/10.3389/fvets.2023.1001621/full#supplementary-material
References
1. Yu T, Wang Y, Chen X, Xiong W, Tang Y, Lin L, et al. Spirulina platensis alleviates chronic inflammation with modulation of gut microbiota and intestinal permeability in rats fed a high-fat diet. J Cell Mol Med. (2020) 24:8603–13. doi: 10.1111/jcmm.15489
2. Wu Q, Liu L, Miron A, Klímová B, Wan D, Kuča K, et al. The antioxidant, immunomodulatory, and anti-inflammatory activities of Spirulina: an overview. Arch Toxicol. (2016) 90:1817–40. doi: 10.1007/s00204-016-1744-5
3. Khan Z, Bhadouria P, Bisen PS. Nutritional and therapeutic potential of Spirulina. Curr Pharm Biotechnol. (2005) 6:373–9. doi: 10.2174/138920105774370607
4. Abdelkhalek NKM, Ghazy EW, Abdel-Daim MM. Pharmacodynamic interaction of Spirulina platensis and deltamethrin in freshwater fish Nile tilapia, Oreochromis niloticus: impact on lipid peroxidation and oxidative stress. Environ Sci Pollut Res. (2015) 22:3023–31. doi: 10.1007/s11356-014-3578-0
5. Khalil SR, Reda RM, Awad A. Efficacy of Spirulina platensis diet supplements on disease resistance and immune-related gene expression in Cyprinus carpio L. exposed to herbicide atrazine Fish Shellfish Immunol. (2017) 67:119–28. doi: 10.1016/j.fsi.2017.05.065
6. Liang Y, Bao Y, Gao X, Deng K, An S, Wang Z, et al. Effects of spirulina supplementation on lipid metabolism disorder, oxidative stress caused by high-energy dietary in Hu sheep. Meat Sci. (2020) 164:108094. doi: 10.1016/j.meatsci.2020.108094
7. Lugarà R, Renner S, Wolf E, Liesegang A, Bruckmaier R, Giller K, et al. Crossbred sows fed a western diet during pre-gestation, gestation, lactation, and post-lactation periods develop signs of lean metabolic syndrome that are partially attenuated by spirulina supplementation. Nutrients. (2022) 14:3574. doi: 10.3390/nu14173574
8. Neumann C, Velten S, Liebert F. The graded inclusion of algae (Spirulina platensis) or insect (Hermetia illucens) meal as a soybean meal substitute in meat type chicken diets impacts on growth, nutrient deposition and dietary protein quality depending on the extent of amino acid supplementation. Open J Anim Sci. (2018) 8:163–83. doi: 10.4236/ojas.2018.82012
9. Neumann C, Velten S, Liebert F. N balance studies emphasize the superior protein quality of pig diets at high inclusion level of algae meal (Spirulina platensis) or insect meal (Hermetia illucens) when adequate amino acid supplementation is ensured. Animals. (2018) 8:172. doi: 10.3390/ani8100172
10. Kashani A, Holman BW, Nichols PD, Malau-Aduli AE. Effect of dietary supplementation with Spirulina on the expressions of AANAT, ADRB3, BTG2 and FASN genes in the subcutaneous adipose and Longissimus dorsi muscle tissues of purebred and crossbred Australian sheep. J Anim Sci Technol. (2015) 57:8. doi: 10.1186/s40781-015-0047-3
11. Li TT, Tong AJ, Liu YY, Huang ZR, Wan XZ, Pan YY, et al. Polyunsaturated fatty acids from microalgae Spirulina platensis modulates lipid metabolism disorders and gut microbiota in high-fat diet rats. Food Chem Toxicol. (2019) 131:110558. doi: 10.1016/j.fct.2019.06.005
12. Hua P, Yu Z, Xiong Y, Liu B, Zhao L. Regulatory efficacy of spirulina platensis protease hydrolyzate on lipid metabolism and gut microbiota in high-fat diet-fed rats. Int J Mol Sci. (2018) 19:4023. doi: 10.3390/ijms19124023
13. Li TT, Liu YY, Wan XZ, Huang ZR, Liu B, Zhao C, et al. Regulatory efficacy of the polyunsaturated fatty acids from microalgae spirulina platensis on lipid metabolism and gut microbiota in high-fat diet rats. Int J Mol Sci. (2018) 19:3075. doi: 10.3390/ijms19103075
14. Wardrop ID, Coombe JB. The post-natal growth of the visceral organs of the lamb I. The growth of the visceral organs of the grazing lamb from birth to sixteen weeks of age. J Agric Sci. (1960) 54:140–3. doi: 10.1017/S0021859600021523
15. Liu L, Sun D, Mao S, Zhu W, Liu J. Infusion of sodium butyrate promotes rumen papillae growth and enhances expression of genes related to rumen epithelial VFA uptake and metabolism in neonatal twin lambs. J Anim Sci. (2019) 97:909–21. doi: 10.1093/jas/sky459
16. Matthews C, Crispie F, Lewis E, Reid M, O'Toole PW, Cotter PD, et al. The rumen microbiome: a crucial consideration when optimising milk and meat production and nitrogen utilisation efficiency. Gut Microbes. (2019) 10:115–32. doi: 10.1080/19490976.2018.1505176
17. Wang M, Wang R, Xie TY, Janssen PH, Sun XZ, Beauchemin KA, et al. Shifts in rumen fermentation and microbiota are associated with dissolved ruminal hydrogen concentrations in lactating dairy cows fed different types of carbohydrates. J Nutr. (2016) 146:1714–21. doi: 10.3945/jn.116.232462
18. Fleissner CK, Huebel N, Abd El-Bary MM, Loh G, Klaus S, Blaut M, et al. Absence of intestinal microbiota does not protect mice from diet-induced obesity. Br J Nutr. (2010) 104:919–29. doi: 10.1017/S0007114510001303
19. Maia MRG, Chaudhary LC, Figueres L, Wallace RJ. Metabolism of polyunsaturated fatty acids and their toxicity to the microflora of the rumen. Antonie Van Leeuwenhoek. (2007) 91:303–14. doi: 10.1007/s10482-006-9118-2
20. Fan-Jiang PY, Lee PS, Nagabhushanam K, Ho CT, Pan MH. Pterostilbene attenuates high-fat diet and dextran sulfate sodium-induced colitis via suppressing inflammation and intestinal fibrosis in mice. J Agric Food Chem. (2021). doi: 10.1021/acs.jafc.1c02783
21. Hong W, Xu D, Song X, Niu B, Zhuang Z, Lu Y, et al. A and retinoic acid accelerate the attenuation of intestinal adaptability upon feeding induced by high-fat diet in mice. J Nutr Biochem. (2021) 97:108803. doi: 10.1016/j.jnutbio.2021.108803
22. Hu F, Xue Y, Guo C, Liu J, Mao S. The response of ruminal fermentation, epithelium-associated microbiota, and epithelial barrier function to severe feed restriction in pregnant ewes. J Anim Sci. (2018) 96:4293–305. doi: 10.1093/jas/sky306
23. Feng Z, Gao M. An improved method for determination of ammonia nitrogen in tummy juice by colorimetry. Anim Husbandry Feed Sci. (2010) 31:37.
24. Qin W. Determination of rumen volatile fatty acids by means of gas chromatography. J Nanjing Agric Coll. (1982) 4:110–6.
25. Ye H, Liu J, Feng P, Zhu W, Mao S. Grain-rich diets altered the colonic fermentation and mucosa-associated bacterial communities and induced mucosal injuries in goats. Sci Rep. (2016) 6:20329. doi: 10.1038/srep20329
26. Steele MA, Croom J, Kahler M, AlZahal O, Hook SE, Plaizier K, et al. Bovine rumen epithelium undergoes rapid structural adaptations during grain-induced subacute ruminal acidosis. Am J Physiol Regul Integr Comp Physiol. (2011) 300:R1515–23. doi: 10.1152/ajpregu.00120.2010
27. Lv F, Wang X, Pang X, Liu G. Effects of supplementary feeding on the rumen morphology and bacterial diversity in lambs. PeerJ. (2020) 8:e9353. doi: 10.7717/peerj.9353
28. Lesmeister KE, Heinrichs AJ, Gabler MT. Effects of supplemental yeast (Saccharomyces cerevisiae) culture on rumen development, growth characteristics, and blood parameters in neonatal dairy calves. J Dairy Sci. (2004) 87:1832–9. doi: 10.3168/jds.S0022-0302(04)73340-8
29. Álvarez-Rodríguez J, Monleón E, Sanz A, Badiola JJ, Joy M. Rumen fermentation and histology in light lambs as affected by forage supply and lactation length. Res Vet Sci. (2012) 92:247–53. doi: 10.1016/j.rvsc.2011.03.010
30. Wang L, Qi W, Mao S, Zhu W, Liu J. Effects of whole corn high-grain diet feeding on ruminal bacterial community and epithelial gene expression related to VFA absorption and metabolism in fattening lambs. J Anim Sci. (2022) 100:skac056. doi: 10.1093/jas/skac056
31. Sun D, Yin Y, Guo C, Liu L, Mao S, Zhu W, et al. Transcriptomic analysis reveals the molecular mechanisms of rumen wall morphological and functional development induced by different solid diet introduction in a lamb model. J Anim Sci Biotechnol. (2021) 12:33. doi: 10.1186/s40104-021-00556-4
32. Ding Q, Zhang Z, Li Y, Liu H, Hao Q, Yang Y, et al. Propionate induces intestinal oxidative stress via Sod2 propionylation in zebrafish. iScience. (2021) 24:102515. doi: 10.1016/j.isci.2021.102515
33. Seifter JL. Body fluid compartments, cell membrane ion transport, electrolyte concentrations, and acid-base balance. Semin Nephrol. (2019) 39:368–79. doi: 10.1016/j.semnephrol.2019.04.006
34. Yohe TT, Schramm H, White RR, Hanigan MD, Parsons CLM, Tucker HLM, et al. Form of calf diet and the rumen. II: impact on volatile fatty acid absorption. J Dairy Sci. (2019) 102:8502–12. doi: 10.3168/jds.2019-16450
35. Cancino-Padilla N, Catalán N, Siu-Ting K, Creevey CJ, Huws SA, Romero J, et al. Long-term effects of dietary supplementation with olive oil and hydrogenated vegetable oil on the rumen microbiome of dairy cows. Microorganisms. (2021) 9:1121. doi: 10.3390/microorganisms9061121
36. Nur Atikah I, Alimon AR, Yaakub H, Abdullah N, Jahromi MF, Ivan M, et al. Profiling of rumen fermentation, microbial population and digestibility in goats fed with dietary oils containing different fatty acids. BMC Vet Res. (2018) 14:344. doi: 10.1186/s12917-018-1672-0
37. Baldwin RL, McLeod KR, Klotz JL, Heitmann RN. Rumen development, intestinal growth and hepatic metabolism in the pre- and postweaning ruminant. J Dairy Sci. (2004) 87:E55–65. doi: 10.3168/jds.S0022-0302(04)70061-2
38. Baxter NT, Schmidt AW, Venkataraman A, Kim KS, Waldron C, Schmidt TM, et al. Dynamics of human gut microbiota and short-chain fatty acids in response to dietary interventions with three fermentable fibers. MBio. (2019) 10:e02566–18. doi: 10.1128/mBio.02566-18
39. Jiao J, Li X, Beauchemin KA, Tan Z, Tang S, Zhou C, et al. Rumen development process in goats as affected by supplemental feeding v. grazing: age-related anatomic development, functional achievement and microbial colonisation. Br J Nutr. (2015) 113:888–900. doi: 10.1017/S0007114514004413
40. Malmuthuge N, Liang G, Guan LL. Regulation of rumen development in neonatal ruminants through microbial metagenomes and host transcriptomes. Genome Biol. (2019) 20:172–172. doi: 10.1186/s13059-019-1786-0
41. Stobo IJ, Roy JH, Gaston HJ. Rumen development in the calf. 2 The effect of diets containing different proportions of concentrates to hay on digestive efficiency. Br J Nutr. (1966) 20:189–215. doi: 10.1079/BJN19660022
42. Beiranvand H, Ghorbani GR, Khorvash M, Nabipour A, Dehghan-Banadaky M, Homayouni A, et al. Interactions of alfalfa hay and sodium propionate on dairy calf performance and rumen development. J Dairy Sci. (2014) 97:2270–80. doi: 10.3168/jds.2012-6332
43. Sun DM, Mao SY, Zhu WY, Liu JH. Effect of starter diet supplementation on rumen epithelial morphology and expression of genes involved in cell proliferation and metabolism in pre-weaned lambs. Animal. (2018) 12:2274–83. doi: 10.1017/S1751731118000290
44. Gomez-Insuasti AS, Granja-Salcedo YT, Rossi LG, Ramalho Vieira B, Berchielli TT. Effect of soybean oil availabilities on rumen biohydrogenation and duodenal flow of fatty acids in beef cattle fed a diet with crude glycerine. Arch Anim Nutr. (2018) 72:308–20. doi: 10.1080/1745039X.2018.1492805
45. Tapio I, Shingfield KJ, McKain N, Bonin A, Fischer D, Bayat AR, et al. Oral samples as non-invasive proxies for assessing the composition of the rumen microbial community. PLoS ONE. (2016) 11:e0151220. doi: 10.1371/journal.pone.0151220
46. Bayat AR, Tapio I, Vilkki J, Shingfield KJ, Leskinen H. Plant oil supplements reduce methane emissions and improve milk fatty acid composition in dairy cows fed grass silage-based diets without affecting milk yield. J Dairy Sci. (2018) 101:1136–51. doi: 10.3168/jds.2017-13545
47. Granja-Salcedo YT, Duarte Messana J, Carneiro V, de Souza AV, Lino Dias, Takeshi Kishi L, et al. Effects of partial replacement of maize in the diet with crude glycerin and/or soyabean oil on ruminal fermentation and microbial population in Nellore steers. Br J Nutr. (2017) 118:651–60. doi: 10.1017/S0007114517002689
48. Fiorentini G, Carvalho IP, Messana JD, Canesin RC, Castagnino PS, Lage JF, et al. Effect of lipid sources with different fatty acid profiles on intake, nutrient digestion and ruminal fermentation of feedlot nellore steers. Asian-Austr J Anim Sci. (2015) 28:1583–91. doi: 10.5713/ajas.15.0130
49. Liu JH, Bian GR, Zhu WY, Mao SY. High-grain feeding causes strong shifts in ruminal epithelial bacterial community and expression of Toll-like receptor genes in goats. Front Microbiol. (2015) 6:167. doi: 10.3389/fmicb.2015.00167
50. Liu J, Xue C, Sun D, Zhu W, Mao S. Impact of high-grain diet feeding on mucosa-associated bacterial community and gene expression of tight junction proteins in the small intestine of goats. Microbiologyopen. (2019) 8:e00745. doi: 10.1002/mbo3.745
51. Mao H, Zhang Y, Yun Y, Ji W, Jin Z, Wang C, et al. Weaning age affects the development of the ruminal bacterial and archaeal community in hu lambs during early life. Front Microbiol. (2021) 12:636865–636865. doi: 10.3389/fmicb.2021.636865
52. Ley RE, Lozupone CA, Hamady M, Knight R, Gordon JI. Worlds within worlds: evolution of the vertebrate gut microbiota. Nat Rev Microbiol. (2008) 6:776–88. doi: 10.1038/nrmicro1978
53. Mizrahi I, Wallace RJ, Moraïs S. The rumen microbiome: balancing food security and environmental impacts. Nat Rev Microbiol. (2021). doi: 10.1038/s41579-021-00543-6
54. Stewart RD, Auffret MD, Warr A, Walker AW, Roehe R, Watson M, et al. Compendium of 4,941 rumen metagenome-assembled genomes for rumen microbiome biology and enzyme discovery. Nat Biotechnol. (2019) 37:953–61. doi: 10.1038/s41587-019-0202-3
55. Matsui H, Ogata K, Tajima K, Nakamura M, Nagamine T, Aminov RI, et al. Phenotypic characterization of polysaccharidases produced by four Prevotella type strains. Curr Microbiol. (2000) 41:45–9. doi: 10.1007/s002840010089
56. Li ZT, Hu GA, Zhu L, Zhao ZC, Yun J, Gao MJ, et al. In vitro digestion and fecal fermentation of highly resistant starch rice and its effect on the gut microbiota. Food Chem. (2021) 361:130095. doi: 10.1016/j.foodchem.2021.130095
57. Huang S, Ji S, Yan H, Hao Y, Zhang J, Wang Y, et al. The day-to-day stability of the ruminal and fecal microbiota in lactating dairy cows. Microbiolo Open. (2020) 9:e990. doi: 10.1002/mbo3.990
58. Shabat SK, Sasson G, Doron-Faigenboim A, Durman T, Yaacoby S, Berg Miller ME, et al. Specific microbiome-dependent mechanisms underlie the energy harvest efficiency of ruminants. ISME J. (2016) 10:2958–72. doi: 10.1038/ismej.2016.62
59. Kamke J, Kittelmann S, Soni P, Li Y, Tavendale M, Ganesh S, et al. Rumen metagenome and metatranscriptome analyses of low methane yield sheep reveals a Sharpea-enriched microbiome characterised by lactic acid formation and utilisation. Microbiome. (2016) 4:56. doi: 10.1186/s40168-016-0201-2
60. Plaizier JC, Li S, Tun HM, Khafipour E. Nutritional models of experimentally-induced subacute ruminal acidosis (SARA) differ in their impact on rumen and hindgut bacterial communities in dairy cows. Front Microbiol. (2016) 7:2128. doi: 10.3389/fmicb.2016.02128
61. Klieve AV, Hennessy D, Ouwerkerk D, Forster RJ, Mackie RI, Attwood GT, et al. Establishing populations of Megasphaera elsdenii YE 34 and Butyrivibrio fibrisolvens YE 44 in the rumen of cattle fed high grain diets. J Appl Microbiol. (2003) 95:621–30. doi: 10.1046/j.1365-2672.2003.02024.x
62. Pope PB, Smith W, Denman SE, Tringe SG, Barry K, Hugenholtz P, et al. Isolation of succinivibrionaceae implicated in low methane emissions from tammar wallabies. Science. (2011) 333:646–8. doi: 10.1126/science.1205760
63. Wang Z, Fu H, Zhou Y, Yan M, Chen D, Yang M, et al. Identification of the gut microbiota biomarkers associated with heat cycle and failure to enter oestrus in gilts. Microb Biotechnol. (2020). doi: 10.1111/1751-7915.13695
64. Blackburn TH, Hungate RE. Succinic acid turnover and propionate production in the bovine rumen. Appl Microbiol. (1963) 11:132–5. doi: 10.1128/am.11.2.132-135.1963
65. Zhang XZ, Chen WB, Wu X, Zhang YW, Jiang YM, Meng QX, et al. Calcium propionate supplementation improves development of rumen epithelium in calves via stimulating G protein-coupled receptors. Animal. (2018) 12:2284–91. doi: 10.1017/S1751731118000289
66. Iwamoto M, Asanuma N, Hino T. Effects of nitrate combined with fumarate on methanogenesis, fermentation, and cellulose digestion by mixed ruminal microbes in vitro. Nihon Chikusan Gakkaiho. (1999) 70:471–8. doi: 10.2508/chikusan.70.471
67. Lopez S, McIntosh F, Wallace R, Newbold C. Effect of adding acetogenic bacteria on methane production by mixed rumen microorganisms. Anim Feed Sci Technol. (1999) 78:1–9. doi: 10.1016/S0377-8401(98)00273-9
68. Li XZ, Park BK, Shin JS, Choi SH, Smith SB, Yan CG, et al. Effects of dietary linseed oil and propionate precursors on ruminal microbial community, composition, and diversity in yanbian yellow cattle. PLoS ONE. (2015) 10:e0126473. doi: 10.1371/journal.pone.0126473
69. Kaakoush NO. Insights into the Role of Erysipelotrichaceae in the Human Host. Front Cell Infect Microbiol. (2015) 5:84. doi: 10.3389/fcimb.2015.00084
70. Martínez I, Wallace G, Zhang C, Legge R, Benson AK, Carr TP, et al. Diet-induced metabolic improvements in a hamster model of hypercholesterolemia are strongly linked to alterations of the gut microbiota. Appl Environ Microbiol. (2009) 75:4175–84. doi: 10.1128/AEM.00380-09
71. Xiong F, Zheng Z, Xiao L, Su C, Chen J, Gu X, et al. Soyasaponin A(2) alleviates steatohepatitis possibly through regulating bile acids and gut microbiota in the methionine and choline deficient (MCD) diet-induced non-alcoholic steatohepatitis (NASH) mice. Mol Nutr Food Res. (2021) 65:e2100067. doi: 10.1002/mnfr.202100067
72. Li J, Zhao F, Wang Y, Chen J, Tao J, Tian G, et al. Gut microbiota dysbiosis contributes to the development of hypertension. Microbiome. (2017) 5:14. doi: 10.1186/s40168-016-0222-x
73. Guo M, Wang H, Xu S, Zhuang Y, An J, Su C, et al. Alteration in gut microbiota is associated with dysregulation of cytokines and glucocorticoid therapy in systemic lupus erythematosus. Gut Microbes. (2020) 11:1758–73. doi: 10.1080/19490976.2020.1768644
74. Zheng Z, Lyu W, Ren Y, Li X, Zhao S, Yang H, et al. Allobaculum involves in the modulation of intestinal ANGPTLT4 expression in mice treated by high-fat diet. Front Nutr. (2021) 8:690138. doi: 10.3389/fnut.2021.690138
75. Hsu YJ, Chiu CC, Lee MC, Huang WC. Combination of treadmill aerobic exercise with Bifidobacterium longum OLP-01 supplementation for treatment of high-fat diet-induced obese murine model. Obes Facts. (2021) 14:306–19. doi: 10.1159/000516865
76. Stanislawski MA, Dabelea D, Wagner BD, Iszatt N, Dahl C, Sontag MK, et al. Gut microbiota in the first 2 years of life and the association with body mass index at age 12 in a norwegian birth cohort. MBio. (2018) 9:e01751–18. doi: 10.1128/mBio.01751-18
77. Chen J, Wang Y, Zhu T, Yang S, Cao J, Li X, et al. Beneficial regulatory effects of polymethoxyflavone-rich fraction from Ougan (Citrus reticulata cv. suavissima) fruit on gut microbiota and identification of its intestinal metabolites in mice. Antioxidants. (2020) 9:831. doi: 10.3390/antiox9090831
78. Logtenberg MJ, Akkerman R, Hobe R, Donners KMH, Van Leeuwen SS, Hermes GDA, et al. Structure-specific fermentation of galacto-oligosaccharides, isomalto-oligosaccharides and isomalto/malto-polysaccharides by infant fecal microbiota and impact on dendritic cell cytokine responses. Mol Nutr Food Res. (2021) 65:e2001077. doi: 10.1002/mnfr.202001077
79. Kiarie EG, Mohammadigheisar M, Kakhki RAM, Madsen MH. Impact of feeding modified soy protein concentrate in the starter phase on growth performance and gastrointestinal responses in broiler chickens through to day 42 of age. Poult Sci. (2021) 100:101147. doi: 10.1016/j.psj.2021.101147
80. Ma X, Zhou W, Guo T, Li F, Li F, Ran T, et al. Effects of Dietary barley starch contents on the performance, nutrient digestion, rumen fermentation, and bacterial community of fattening Hu sheep. Front Nutr. (2021) 8:797801. doi: 10.3389/fnut.2021.797801
81. Guo T, Wang ZL, Guo L, Li F, Li. Effects of supplementation of nonforage fiber source in diets with different starch levels on growth performance, rumen fermentation, nutrient digestion, and microbial flora of Hu lambs. Transl Anim Sci. (2021) 5:txab065. doi: 10.1093/tas/txab065
82. Gorka P, Kowalski ZM, Pietrzak P, Kotunia A, Kiljanczyk R, Flaga J, et al. Effect of sodium butyrate supplementation in milk replacer and starter diet on rumen development in calves. J Physiol Pharmacol. (2009) 60 Suppl 3:47–53.
83. Górka P, Kowalski ZM, Pietrzak P, Kotunia A, Jagusiak W, Holst JJ, et al. Effect of method of delivery of sodium butyrate on rumen development in newborn calves. J Dairy Sci. (2011) 94:5578–88. doi: 10.3168/jds.2011-4166
84. Lesmeister KE, Tozer PR, Heinrichs AJ. Development and analysis of a rumen tissue sampling procedure. J Dairy Sci. (2004) 87:1336–44. doi: 10.3168/jds.S0022-0302(04)73283-X
85. Yang W, Shen Z, Martens H. An energy-rich diet enhances expression of Na(+)/H(+) exchanger isoform 1 and 3 messenger RNA in rumen epithelium of goat. J Anim Sci. (2012) 90:307–17. doi: 10.2527/jas.2011-3854
Keywords: rumen microbiota, Hu sheep, rumen morphology, spirulina supplementation, rumen development and fermentation
Citation: Wang Z, Liang Y, Lu J, Wei Z, Bao Y, Yao X, Fan Y, Wang F, Wang D and Zhang Y (2023) Dietary spirulina supplementation modifies rumen development, fermentation and bacteria composition in Hu sheep when consuming high-fat dietary. Front. Vet. Sci. 10:1001621. doi: 10.3389/fvets.2023.1001621
Received: 03 September 2022; Accepted: 05 January 2023;
Published: 30 January 2023.
Edited by:
Bo Wang, China Agricultural University, ChinaReviewed by:
Zhibin Ji, Shandong Agricultural University, ChinaYang He, China Agricultural University, China
Copyright © 2023 Wang, Liang, Lu, Wei, Bao, Yao, Fan, Wang, Wang and Zhang. This is an open-access article distributed under the terms of the Creative Commons Attribution License (CC BY). The use, distribution or reproduction in other forums is permitted, provided the original author(s) and the copyright owner(s) are credited and that the original publication in this journal is cited, in accordance with accepted academic practice. No use, distribution or reproduction is permitted which does not comply with these terms.
*Correspondence: Yanli Zhang, emhhbmd5YW5saUBuamF1LmVkdS5jbg==