- 1College of Animal Science and Technology, Gansu Agricultural University, Lanzhou, China
- 2State Key Laboratory of Grassland Agro-Ecosystems, College of Pastoral Agriculture Science and Technology, Lanzhou University, Lanzhou, China
This study aimed to explore the effects of early feeding strategies on the growth and rumen development of lambs from pre-weaning to the transition to fattening diets. Ninety-six newborn, male lambs with similar body weights were randomly assigned to three treatments: fed starter at 42 days old + weaned at 56 days old (Ctrl, n = 36), fed starter at 7 days old + weaned at 56 days old (ES, n = 36), and fed starter at 7 days old + weaned at 28 days old (ES + EW, n = 24). The fattening diets of all lambs were gradually replaced from 60 to 70 days of age. Six randomly selected lambs from each treatment were slaughtered at 14, 28, 42, 56, 70, and 84 days of age. The results showed that the richness and diversity of rumen microbiota of lambs in the Ctrl group were distinct from those of lambs in the other groups at 42 days of age. Moreover, transcriptome analysis revealed 407, 219, and 1,211 unique differentially expressed genes (DEGs) in the rumen tissue of ES vs. Ctrl, ES vs. ES + EW, and ES + EW vs. Ctrl groups, respectively, at 42 days of age. Different early feeding strategies resulted in differences in ruminal anatomy, morphology, and fermentation in lambs from 42 to 84 days of age (P < 0.05). Lambs in the ES + EW group had a higher average starter diet intake than those in the other groups (P < 0.05) from 28 to 56 days of age, which affected their growth performance. After 42 days of age, the body and carcass weights of lambs in the ES and ES + EW groups were higher than those in the Ctrl group (P < 0.05). These findings demonstrate that feeding lambs with a starter diet at 7 days of age and weaning them at 28 days of age can promote rumen development and improve growth performance, and this advantage persists for up to 2 weeks after transition to the fattening diet.
Introduction
Rumen development is the most critical physiological challenge in young ruminants. The ruminant forestomach has a unique structure and function that not only differentiates it from the stomach of monogastric animals morphologically but also in terms of digestion and metabolism (1). Lambs are born with a physically and metabolically underdeveloped rumen similar to that of a monogastric animal, whereby the milk enters the abomasum through the esophageal groove to be digested and is then absorbed in the small intestine to maintain and meet the nutrient requirements for growth (2, 3). After lambs start consuming solid feeds, the rumen's colonization by microorganisms, establishment of fermentation, initiation of transport and absorption, volume enlargement, and growth of papillae are all necessary as lambs shift from dependence on milk to solid feeds (4, 5). Numerous studies have confirmed that even subtle changes in early feeding styles and nutrient composition are able to substantially influence ruminal development (6–9).
The weaning age of lambs is generally determined by the production goal in the commercial lamb industry. Early weaning can shorten the breeding cycle of ewes and be a strong stressor for lambs, as separation from the dams increases lamb morbidity and mortality (10). Lactation time is an important factor in ewe-lamb contact intensity and early weaning stress (11). Furthermore, early weaning success is limited by rumen volume, the rate of functional development (12, 13), and the establishment of a functional rumen microbiome (14, 15). Studies related to the early weaning of lambs have mainly focused on growth performance (16, 17), rumen development (9), and rumen microbiota (18, 19). Therefore, no uniform standard for the early weaning of lambs has been established so far and a weaning strategy that minimizes the negative impact of early weaning while maintaining economic benefits is required.
Early supplementation has been shown to improve rumen development and function during the transition from milk to solid feeds (20, 21), preventing several issues related to weaning. Previous studies have confirmed a positive correlation between starter diet intake and rumen development (9, 22), as well as diet fermentability (23) and feed additives (24). Similarly, the levels and physicochemical characteristics of the available substrates affect rumen microbial diversity and fermentation patterns after lambs begin to consume solid feeds (25, 26). For example, feeding lambs readily fermentable carbohydrates, including starch and sugar, increases volatile fatty acids (VFAs), especially butyrate, which is responsible for ruminal epithelial development (27). Additionally, feeding forage-containing starter diets stimulates rumen muscularization and rumination, enhances rumen volume and motility, and maintains rumen wall integrity and health (28). Thus, decades of research has focused on feeding strategies to facilitate early weaning and the transition from liquid to solid feeds in lambs (29–32). Abecia et al. (33) showed that nutritional intervention in young goats during the pre-weaning period can influence the rumen microbial composition, with the effects lasting for 3 months after weaning. However, there are no published studies characterizing the long-term effects of pre-weaning feeding strategies on lambs. We hypothesized that feeding strategies during the pre-weaning period of lambs would influence rumen development, affecting the ruminal microbiota and ruminal epithelium to change the ruminal fermentation pattern and function. This would occur via changes in protein and gene expression, and the ruminal microbiota, with the effects persisting after weaning. Therefore, the present study aimed to investigate the effects of lambs' early feeding strategies on rumen development and performance from pre-weaning to transition to fattening diets.
Materials and methods
Animal management and diets
Ninety-six newborn male lambs (3.58 ± 0.66 kg) from Hu ewes with double male lambing were obtained from a commercial sheep farm in Gansu Province, China. The ewes and lambs were housed in well-ventilated sheep pens (6 m × 8 m) with eight lambs and their dams per pen. All ewes were fed three times daily according to the feeding management of the sheep farm and had ad libitum access to water. The ewes were fed a total mixed feed of 23.90% corn silage, 15.41% oat hay, 12.84% alfalfa hay, 10.35% barley straw, 6.34% oilseed rape straw, 16.10% soybean meal, 11.30% corn, and 3.75% soybean meal. When the ewes were fed, lambs were separated from their dams and housed in individual pens (2.5 m × 1.5 m); however, the lambs and their dams could see each other. Throughout the experimental period, the lambs were unable to feed on the ewes' diet.
As described in Figure 1, when the lambs were 7 days old, 36 lambs (birth weight, BW = 4.94 ± 0.80 kg) were randomly selected as the control group (Ctrl); these lambs were raised with ewes, fed on starter diet at 42 days old, and weaned at 56 days old. Other lambs (n = 60; 7 days old; BW = 4.91 ± 0.81 kg) were offered a pelleted starter diet ad libitum during the time they were separated from their dams (the starter was offered each morning and the residue was collected the next morning). Twenty-four of these lambs (28 days old; BW = 7.58 ± 1.62 kg) were weaned at 28 days of age and categorized as the early weaning group (EW + ES). The remaining lambs (n = 30; 28 days old; BW = 8.38 ± 0.96 kg) served as the early supplementary group (ES). The lambs in the Ctrl and ES groups were weaned at 56 days of age. After weaning, the lambs were housed in pens (6 m × 8 m) where they could not see the dams. The fattening diets of all lambs were gradually replaced from 60 to 70 days of age. The lambs had ad libitum access to the experimental diets and water during the experimental period. Six lambs were selected from each group and slaughtered on days 14, 28, 42, 56, 70, and 84.
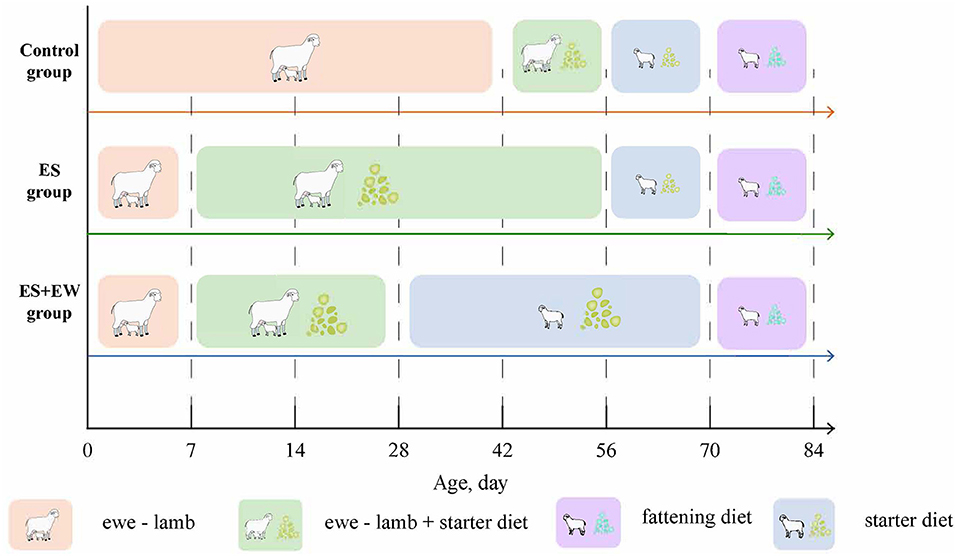
Figure 1. Experimental design. Study design showing the time frame of the experiment and the treatment groups. The ewes of control group were fed according to the sheep farm procedure. At 42, 56, 70, and 84 days of age, six lambs were randomly selected from each treatment group, weighed, and slaughtered.
The experimental diets were formulated and calculated according to the Nutrient Requirements of Small Ruminants (34). The experimental diets were provided as 2.5 mm pellets. Table 1 shows the experimental diets, including their formulation and chemical composition. The feedstuffs were analyzed for dry matter (DM, method 930.15), crude protein (CP, method 984.13), calcium (method 975.03), and phosphorous (method 965.17) according to the Association of Official Agricultural Chemists (AOAC) methods (35), while starch content was determined according to the method described by MacRae et al. (36). The determination of neutral detergent fiber (NDF) and acid detergent fiber (ADF) were based on the method of Van Soest et al. (37). All analyses were performed in triplicate. The digestive energy (DE) was computed according to the National Research Council (NRC) methodology (34) using sheep tabular values.
Sample collection
Samples were collected as described in Liu et al. (26). In brief, the body weight of each lamb was determined weekly from 7 to 84 days of age. The starter and fattening dietary intakes were measured daily for each pen. For each lamb, diarrhea was monitored and recorded daily to calculate the diarrhea rate. At 42, 56, 70, and 84 days of age, six lambs were randomly selected from each treatment group, weighed, and slaughtered in the abattoir. Immediately after slaughter, both carcass and organ weight were measured. The entire rumen content was collected, homogenized, and a subsample was transferred into a plastic bottle, snap-frozen in liquid nitrogen, and stored at −80°C for bacterial analysis. Ruminal tissues from the ventral sac region were sampled and stored at −80°C for transcriptomic analysis or washed in normal saline and stored in 10% buffered formalin solution for morphological analysis. Ruminal fluid was collected and stored at −20°C for the determination of volatile fatty acids (VFAs), ammonia nitrogen (NH3-N), and microbial crude protein (MCP) concentrations.
Measurement of physiological parameters
Immediately after slaughter, rumen pH was determined using a pH meter (Orion Star A121 portable pH meter; Thermo Fisher Scientific, Waltham, MA, USA). The concentrations of VFAs, NH3-N, and MCP in the ruminal fluid were analyzed as described previously (26, 38, 39). In brief, VFAs were measured in an Agilent 6890N gas chromatograph (Agilent Technologies Inc., Santa Clara, CA, USA) with a 30 m (0.32 mm internal diameter) fused silica column (HP-19091N-213; Agilent Technologies Inc.), as described by Liu et al. (26). The NH3-N content was measured using a SP-723 spectrophotometer (Spectrum Instruments, Ltd., Shanghai, China) according to the Berthelot reaction (phenol-hypochlorite), as described by Broderick and Kang (38). The MCP content was measured using the Folin phenol method, as described by Makkar et al. (39). Ruminal morphometric analyses were performed using hematoxylin-eosin staining, as described by Lesmeister et al. (40).
DNA extraction, 16S rRNA amplicon sequencing, and bacterial composition analysis
For microbial DNA extraction, rumen contents were collected from six lambs in each group at 42 days of age. Total DNA was extracted from rumen contents using a QIAamp DNA Stool Mini Kit (QIAGEN, Hilden, Germany), according to the manufacturer's instructions. To assess the rumen microbial profiles, the V3–V4 region of the bacterial 16S rRNA gene was amplified using the universal primers 341F (5′-CCTAYGGGRBGCASCAG-3′) and 806R (5′-GGACTACNNGGGTATCTAAT-3′) (41) with barcode sequences. The obtained amplicons libraries for all samples were sequenced on the Illumina MiSeq platform (Illumina Inc., San Diego, CA, USA) and 2 × 250 bp paired-end reads were generated.
The raw sequence data were processed using QIIME (www.qiime.org). Sequences with ≥97% similarity were assigned to the same operational taxonomic unit (OTU). All the reads were identified and classified using the UCHIME algorithm (42), compared against the reference database. To calculate the α-diversity of rumen microbiota, the following metrics were calculated: number of observed species, Chao1 index, and Shannon index (43). Principal coordinate analysis (PCoA) of weighted UniFrac distances, as described by Fomenky et al. (44), was used to visualize microbiota association patterns, i.e., β-diversity. Taxonomical annotation was performed using Mothur v 1.41.1 (43) and by referring to Silva.nr.132 (45). The number of shared genera was visualized in Venn diagrams. The linear discriminant analysis effect size (LEfSe) was used to analyze the bacterial differences at the genus level among the three treatment groups.
RNA extraction and transcriptome, gene expression, and functional analyses
Total RNA was isolated from nine rumen tissue samples of lambs at 42 days of age, using TRIzol (TransGen Biotech, Beijing, China), according to the manufacturer's instructions. The concentration and quality of total RNA were examined in a NanoDrop spectrophotometer (NanoDrop Technologies, Wilmington, DE, USA) and Bioanalyzer 2100 system (Agilent Technologies Inc.), respectively. After screening for RNA quality, a total of 1.5 μg RNA per sample was used to construct a cDNA sequencing library using the TruSeq Stranded mRNA Sample Prep Kit (Illumina Inc.), following the manufacturer's instructions (46). After library construction, all libraries were sequenced using the Illumina HiSeq 4000 platform (Illumina Inc.) at Shanghai Personalbio Science and Technology Co., Ltd. (Shanghai, China).
Low-quality reads and adapter sequences were removed using Trimmomatic (47). Subsequently, the high-quality reads were aligned to the sheep reference genome (Ovis aries v3.1) using TopHat2 (v2.0.13) and Bowtie2 (v2.3.3.1), as described previously (48, 49). Gene transcripts were assembled using String-Tie (v1.3.1) based on the reference genome model (50). Gene expression values were normalized to fragments per kilobase of transcript per million mapped (FPKM).
Differentially expressed genes (DEGs) in pair-wise comparisons (ES vs. Ctrl, ES vs. ES + EW, and ES + EW vs. Ctrl group) were identified using the DESeq R package (1.10.1) in R software (v3.5.1) (51). Adjusted P < 0.05 and absolute value of [log2 (fold change)] > 1.5 were both used as filter factors for identifying DEGs. KOBAS (v3.0) was used to identify DEGs in the Kyoto Encyclopedia of Genes and Genomes (KEGG) pathways (52).
Quantitative real-time polymerase chain reaction
Four genes (transforming growth factor, TGF; and insulin like growth factor binding proteins 3, 5, and 6, IGBP3, IGBP5, and IGBP6) associated with the phosphatidylinositol 3 kinase-protein kinase B (PI3K-Akt) and TGF-β signaling pathways were employed to assess the relationship between ruminal morphology and gene expression. Another five genes (sodium-hydrogen exchangers 2 and 3, NHE2 and NHE3; DR alpha, DRA; 3-hydroxy-3-methylglutaryl-CoA lyase; HMGCL, and monocarboxylate transporter 1; MCT1) associated with mineral absorption and carbohydrate metabolism were selected for correlation analysis with ruminal pH and total VFAs (TVFA).
Total RNA was extracted from the rumen tissues of 18 lambs at 42 days of age using TRIzol (TransGen Biotech). Absorbance at 260 and 280 nm was measured using a NanoDropⓇ ND-1000 spectrophotometer (Thermo Fisher Scientific) to assess RNA purity and yield, and reverse-transcribed into cDNA, following the manufacturer's instructions. The primers used (Table 2) were designed in Primer Premier 5.0 software (PREMIER Biosoft International, Palo Alto, CA, USA). The quantitative real-time PCR (qRT-PCR) was performed on an ABI3700 Real-Time PCR system (Applied Biosystems Inc., Foster City, CA, USA) under the following conditions: denaturation at 94 °C for 3 min; then, 40 cycles at 94 °C for 30 s, annealing (different temperature for each gene, Table 2) for 30 s, extension at 72 °C for 30 s; final extension at 72 °C for 10 min. Each 20 μL qRT-PCR reaction contained 50 ng cDNA, 10 μL of SYBR Green (TransGen Biotech), 0.4 μL of each primer, and 8.2 μL ddH2O. Beta actin was used as the internal control gene. The 2−ΔΔCT method was used to analyze the data (52).
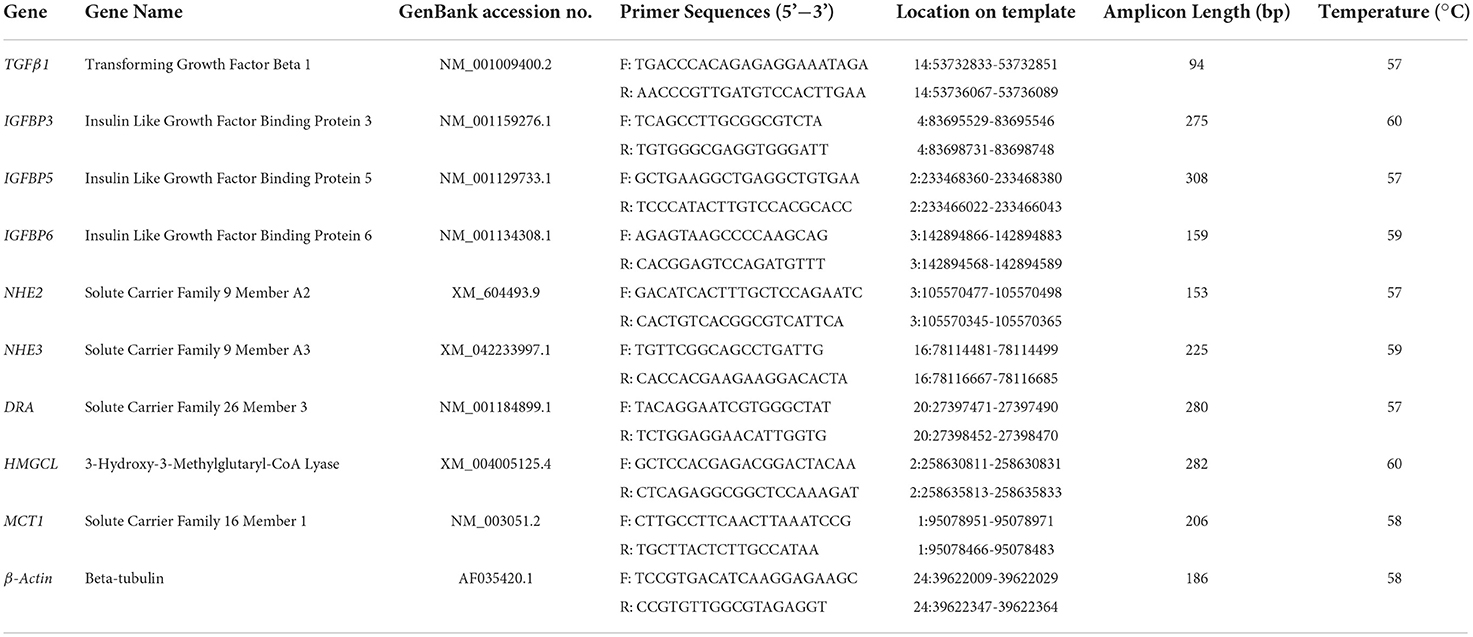
Table 2. QPCR Primers used in the validation of DEGs of rumen tissue between Hu lambs with different early feeding strategies.
Statistical analysis
Statistical analyses of growth performance, ruminal anatomy, morphology, and fermentation were performed in R (v3.5.1; Free Software Foundation, Boston, MA, USA), using the linear model:
where Yijk is the value measured in treatment i at age j of lamb k; μ is the overall mean; Ti is the fixed effect of the three treatments (Ctrl, ES, and ES + EW; i = 1, 2, and 3), Aj is the fixed effect of age over the seven periods (days 7, 14, 28, 42, 56, 70, and 84; j = 1, 2,…,7); (T:A)ij is the fixed effect of the interaction between treatment and age; μk is the random effect of different early rearing in lambs (k = 1, 2, 3,…18); BWk and DRk are the covariates “birth weight” and “diarrhea rate,” respectively; εijk is the random residual error. A significant effect of the treatment was established at P < 0.05.
Bacterial abundance and gene expression data were analyzed using one-way analysis of variance (ANOVA) in SPSS Statistics 23.0 (IBM Co. Ltd., Chicago, IL, USA). The model was the following:
where Yij is the dependent variable (j = 1, 2…,6); μ is the overall mean; Ti is the fixed effect of the three treatments (Ctrl, ES, and ES + EW; i = 1, 2, and 3); εij is the random effect. A significant effect of the treatment was established at P < 0.05.
Spearman's rank correlation was employed to assess the relationship between rumen fermentation parameters, ruminal morphology, gene expression, and relative bacterial abundance using R (v3.5.1; Free Software Foundation). A significant effect of the treatment was established at P < 0.05. All data were analyzed visually using GraphPad Prism 8.0.1 (GraphPad Software, Inc., San Diego, CA, USA) and Origin software (Origin Lab Corp., Northampton, MA, USA).
Results
Growth performance
The average starter diet intake of lambs was significantly affected by age, rearing, and their interaction (P = 0.022; Figure 2A). Lambs in the ES and ES + EW group were supplemented with starter diets until the end of the experiment (from 42 to 84 days of age), and their average starter diet intake was higher than that of lambs in the Ctrl group (P < 0.05). There was no significant interactive effect of rearing and age on the body and carcass weights of lambs (P > 0.05). After 42 days of age, the body weight of lambs in the ES + EW group was higher than that of lambs in the Ctrl group (P < 0.05; Figure 2B). The body weight of lambs in the ES group was higher than that of the lambs in the Ctrl group (P < 0.05) from 77 to 84 days of age (Figure 2B). The carcass weight of lambs in the ES and ES + EW groups was higher than that of the lambs in the Ctrl group after 56 days of age (P < 0.05, Figure 2C), except for lambs in the ES + EW group at 70 days of age (P > 0.05). Notably, the carcass weight of lambs in the Ctrl group was higher than that of lambs in the ES group (P < 0.05) at 28 days of age.
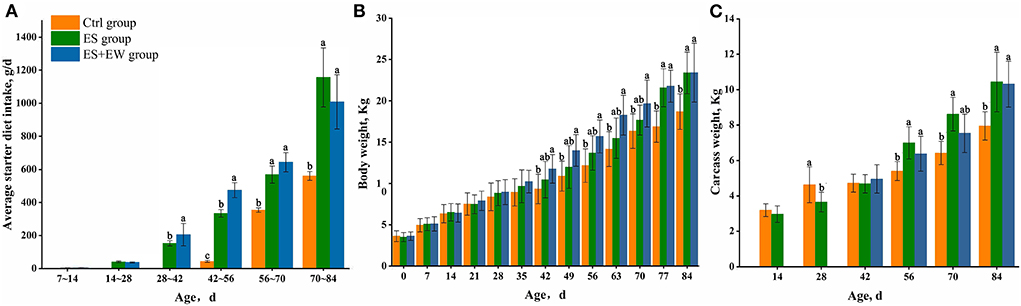
Figure 2. Average starter diet intake (A), body weight (B), and carcass weight (C) of lambs in different early feeding strategies. Columns with different letters at a single time point indicate means that differed based on the means separation (P < 0.05). Error bars indicate SEM for the treatment × age interaction.
Ruminal anatomy, morphology, and fermentation
The weights of the reticulorumen, which were expressed as a percentage of the body weight, whole gastrointestinal tract, and whole stomach were significantly affected by age, rearing, and their interaction (P < 0.001; Figure 3A). Moreover, the reticulorumen weight of lambs in the ES + EW group was higher (P < 0.05) than that of lambs in the other groups from 56 to 84 days of age. Lambs fed with starter diets had a higher reticulorumen weight, expressed as a percentage of body weight (P = 0.034) and whole stomach weight (P = 0.027) at 28 days of age. Additionally, early weaning increased the reticulorumen weight of lambs compared with lambs in the ES (P = 0.007) and Ctrl (P < 0.001) groups at 42 days of age.
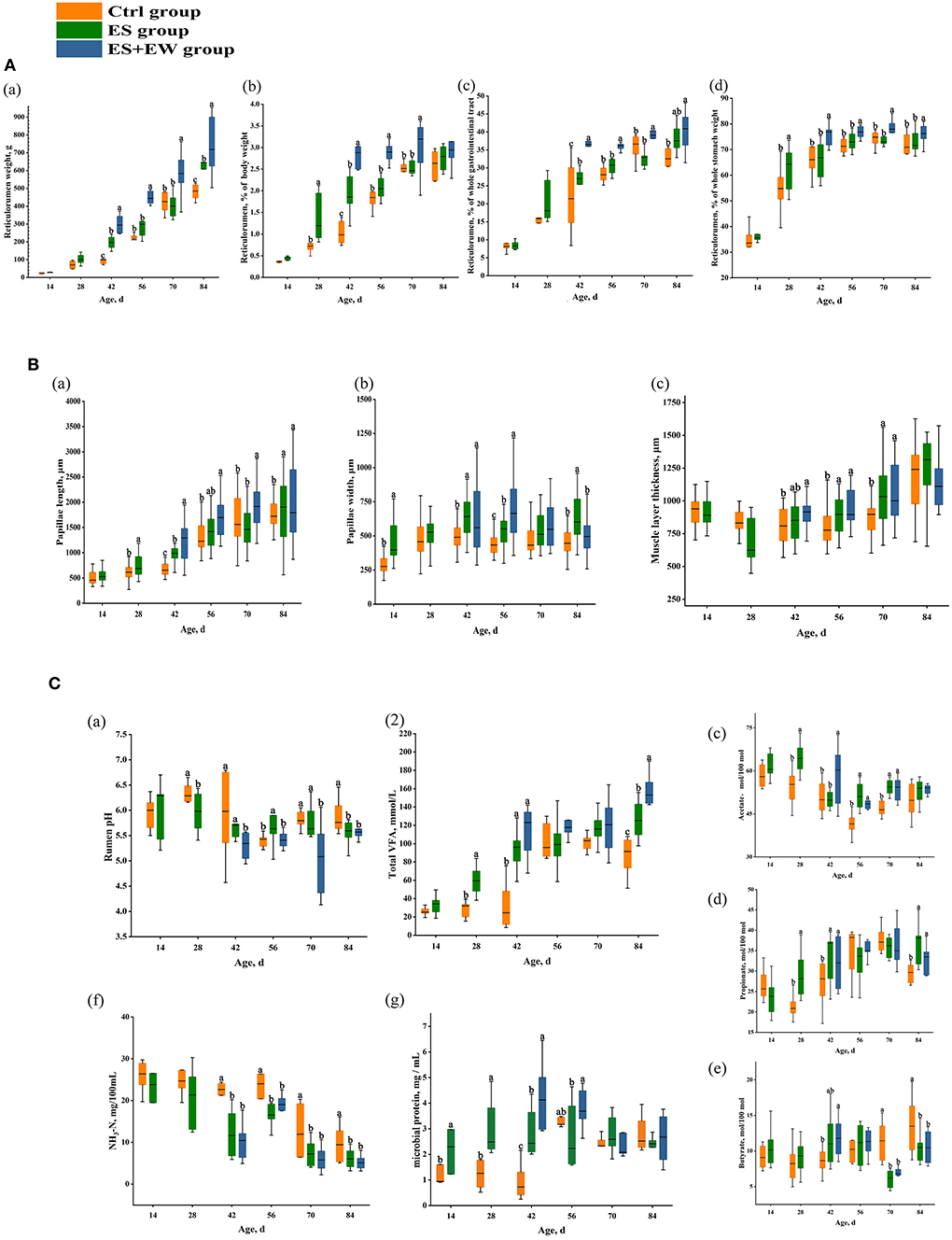
Figure 3. The development of lamb ruminal anatomy (A), morphology (B), and fermentation (C) in different early feeding strategies. Boxes with different letters at a single time point indicate means that differed based on the means separation (P < 0.05). Error bars indicate SEM for the treatment × age interaction.
Ruminal papillae length (P = 0.012; Figure 3Ba) and width (P = 0.030; Figure 3Bb), and ruminal muscle layer thickness (P < 0.001; Figure 3Bc) were all significantly affected by age, rearing, and their interaction. Lambs in the ES group had longer rumen papillae than lambs in the Ctrl group (P < 0.05) at 28, 42, and 84 days of age. The rumen papillae of lambs in the ES + EW group were longer than that of lambs in the other groups from 42 to 84 days of age (P < 0.05). Furthermore, the rumen papillae of lambs in the ES + EW group were longer than that of lambs in the Ctrl group at 28 and 42 days of age (P < 0.05). Additionally, the rumen papillae of lambs in the ES + EW group were wider than that of lambs in the Ctrl group at 42 and 56 days of age (P < 0.05). Similarly, lambs in the ES group had wider rumen papillae than lambs in the Ctrl group at 14, 42, and 56 days of age (P < 0.05). At 84 days of age, the rumen papillae of lambs in the ES group were wider than those of lambs in the other groups (P < 0.05). The rumen muscle layer of lambs in the ES and ES+EW groups was thicker than that of lambs in the Ctrl group at 56 and 70 days of age (P < 0.05). The lambs in the ES and ES + EW group had a thicker rumen muscle layer than that in the Ctrl group at 56 and 70 days of age (P < 0.05). Additionally, the rumen muscle layer of lambs in the ES + EW group was thicker than that of lambs in the Ctrl group at 42 days of age (P < 0.05).
Ruminal VFA concentrations (P < 0.001; Figure 3Ca), MCP (P < 0.001; Figure 3Cg), molar proportions of acetate (P < 0.001; Figure 3Cc), propionate (P < 0.001; Figure 3Cd), and butyrate (P < 0.001; Figure 3Ce) were all significantly affected by age, rearing, and their interaction. Conversely, neither ruminal pH (P = 0.282; Figure 3Ca) nor NH3-N (P = 0.464; Figure 3Cf) were significantly affected by age and rearing. However, the lambs in the ES and ES + EW groups had higher ruminal pH than the lambs in the Ctrl group at 28, 42, 70, and 84 days of age (P < 0.05) and the ruminal pH of lambs in the ES group was higher than that of lambs in the other groups at 56 days of age (P = 0.046). Conversely, the ruminal pH of lambs in the ES + EW group was higher than that in other groups at 84 days of age (P = 0.017). As for NH3-N concentration, lambs in the ES and ES + EW groups showed lower values than lambs in the Ctrl group from 42 to 84 days of age (P < 0.05).
Lambs in the ES group had higher ruminal VFA concentrations than lambs in the Ctrl group at 28 and 84 days of age (P < 0.05). At 42 days of age, lambs in the ES and ES + EW groups had higher ruminal VFA concentrations than that in the Ctrl group (P = 0.014). Lambs in the ES and ES + EW groups showed higher molar proportion of acetate than lambs in the Ctrl group at 28, 56, and 70 days of age (P < 0.05). At 42 days of age, the molar proportion of acetate in lambs in the ES + EW group was higher than that of lambs in the other groups (P = 0.027). The lambs in the ES and ES + EW groups showed higher molar proportions of propionate than the lambs in the Ctrl group at 28, 42, and 84 days of age (P < 0.05). At 70 and 84 days of age, lambs in the ES and ES + EW groups had lower molar proportions of butyrate than lambs in the Ctrl group (P < 0.05). The molar proportion of butyrate in lambs in the ES + EW group was higher than that of lambs in the Ctrl group (P = 0.043). Additionally, lambs in the ES + EW group had higher molar proportions of butyrate than lambs in the ES group at 56 days of age (P = 0.038). The lambs in the ES and ES + EW groups had higher MCP than the lambs in the Ctrl group from 14 to 42 days of age (P < 0.05).
Ruminal microbiota diversity and community structure
A total of 810,363 high-quality sequences were obtained from the 18 samples of rumen contents, with an average of 45,020 sequences per sample, and 4,420 OTUs were defined based on 97% similarity. At 42 days of age, the number of observed species (P = 0.006; Figure 4Aa) and the value of Chao1 (P = 0.006; Figure 4Ac) in the Ctrl group were lower than that in the other groups. Conversely, the value of Shannon (P = 0.001; Figure 4Ab) was higher in the Ctrl group than in the other groups. However, no difference in α-diversity was observed between the ES and ES + EW groups (P > 0.05).
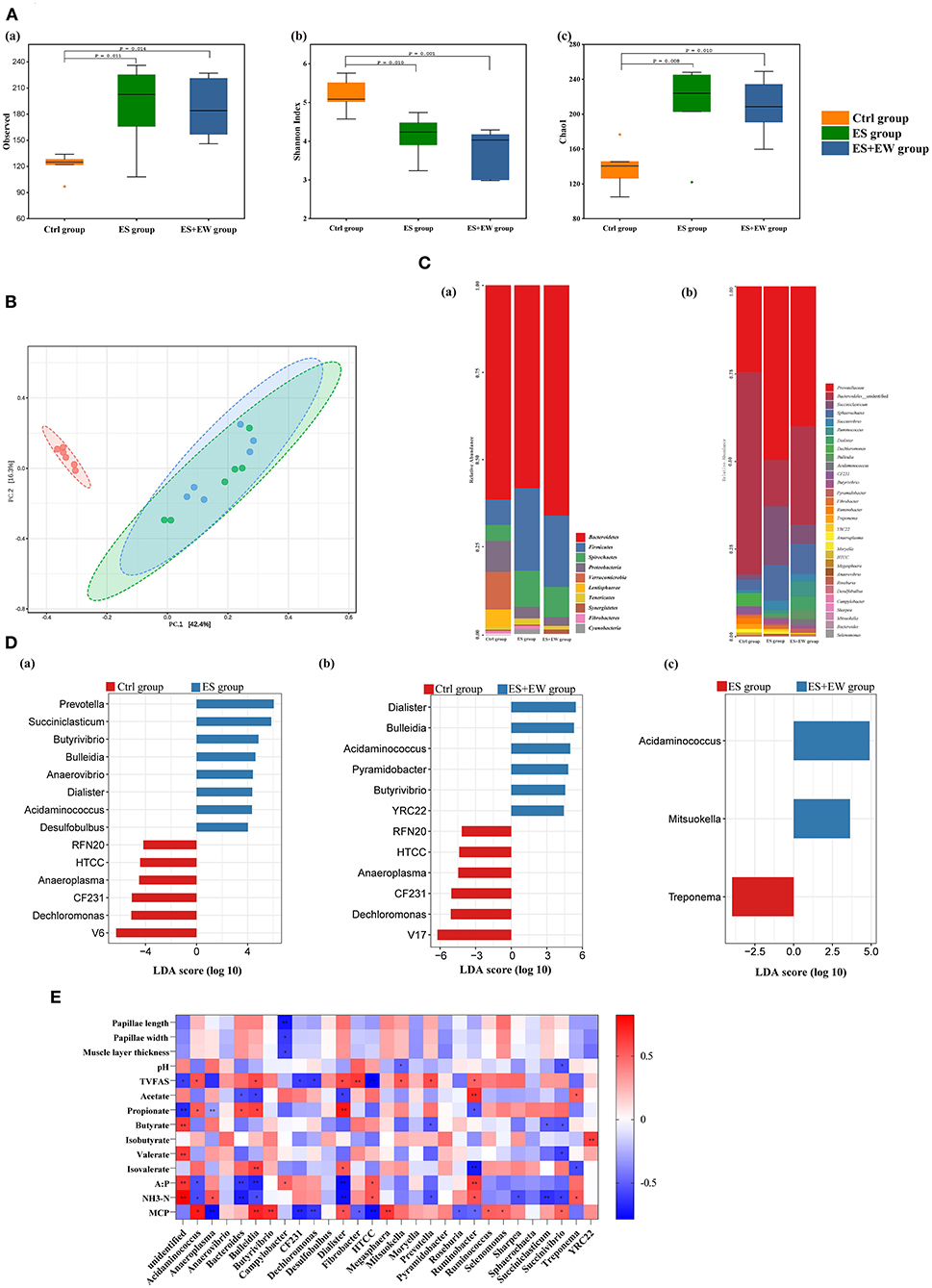
Figure 4. Ruminal microbiota diversity and community structure of lambs in different early feeding strategies at 42 days of age. (A) The alpha diversity in the rumen microbial community based on the observed species (a), Shannon index (b) and Chao1 index (c). (B) The principal coordinate analysis (PCoA) based on the unweighted Unifrac distances. (C) The composition of rumen microbiome at phylum and genus level: the composition of rumen microbiome at phylum level (a), the composition of major rumen genera (b). (D) LEfSe identified significantly different bacteria at the genus level as differentiating the two groups, including Ctrl vs. ES (a), Ctrl vs. ES+EW (b), and ES vs. ES+EW (c). Genera in this graph were statistically significant (P < 0.05) and had an LDA Score > 2.5, which was considered a significant effect size; (E) Heat maps showing the correlations between animal phenotypical variables and relative abundance of bacterial genera. The depth of the color indicates the correlation between species and environmental factors. “*” and “**” indicate the different levels at 0.05 and 0.01, respectively.
The results of the β-diversity analysis using weighted UniFrac distances revealed a clear diversification of the bacterial community between the Ctrl group and the other two groups (Figure 4B). The ANOSIM revealed differences in bacterial community composition between the Ctrl and ES groups (R = 0.572, P = 0.029) and the Ctrl and ES + EW groups (R = 0.681, P = 0.017). No significant differences were observed between the ES and ES + EW groups (R = 0.021, P = 0.641).
We identified the top ten most abundant phyla (Figure 4Ca) and the phyla with abundance > 1.00% of total sequences in at least one group. The main phylum, with the highest relative abundance across all samples, was Bacteroidetes, accounting for 58.19–66.06% of the total sequences. In the ES and ES + EW groups, it was followed by Firmicutes (23.65 and 20.45%, respectively), Spirochaetes (10.35 and 8.61%, respectively), and Proteobacteria (3.32 and 2.52%, respectively), while in the Ctrl group, it was followed by Verrucomicrobia (10.66%), Proteobacteria (8.84%), and Firmicutes (7.36%). The relative abundances of Proteobacteria (P = 0.007), Verrucomicrobia (P = 0.002), and Lentisphaerae (P = 0.014) were higher, whereas that of Firmicutes (P = 0.003) was lower in the Ctrl group than in the other groups. Bacterial genera with abundance > 0.10% are presented in Figure 4Cb. The lambs in the ES + EW group had a higher relative abundance of Acidaminococcus (P < 0.001), Dechloromonas (P = 0.021), high-throughput culture collection (HTCC) isolates (P = 0.002), Mitsuokella (P = 0.018), and Roseburia (P = 0.005) than in the other groups. Bacteroidales_unidentified (P = 0.002) and CF231 (P = 0.007) showed the highest relative abundance in the Ctrl group. The relative abundance of Butyrivibrio (P = 0.011) in the ES + EW group was higher than that in the Ctrl group. Lambs in the ES group had a higher relative abundance of Succiniclasticum (P = 0.020) than those in the Ctrl group.
To identify the differentiated bacterial taxa among the three rearing methods, LEfSe analysis was performed at the genus level. When the Ctrl and ES groups were compared (Figure 4Da), V6, Dechloromonas, CF231, Anaeroplasma, HTCC, and RFN20 were significantly enriched in the Ctrl group, whereas the biomarkers for the ES group were Prevotella, Succiniclasticum, Butyrivibrio, and Bulleidia. When the Ctrl and ES + EW groups were compared (Figure 4Db), V17, Dechloromonas, CF231, Anaeroplasma, HTCC, and RFN20 were enriched in the Ctrl group, whereas Mitsuokella, Sharpea, YRC22, Butyrivibrio, Pyramidobacter, Acidaminococcus, Bulleidia, and Dialister were biomarkers for the ES + EW group (Figure 4Db). Additionally, the rumen microbiota in lambs of the ES group had a higher relative abundance of Treponema than that of lambs in the other groups, whereas Acidaminococcus and Mitsuokella were over-represented in the EW + ES group (Figure 4Dc).
Bacterial abundance and phenotypic variables relationship
Correlation analysis showed that ruminal muscle layer thickness (R = −0.596, P = 0.025) and papillae length (R = −0.726, P = 0.003), and width (R = −0.618, P = 0.018) were negatively correlated with the relative abundance of Campylobacter (Figure 4E; n = 6). Ruminal pH was negatively correlated with the relative abundances of Mitsuokella (R = −0.485, P = 0.041) and Succinivibrio (R = −0.546, P = 0.019). TVFA was positively correlated with the relative abundances of Acidaminococcus (R = 0.562, P = 0.024), Bulleidia (R = 0.593, P = 0.015), Dialister (R = 0.596, P = 0.015), Mitsuokella (R = 0.554, P = 0.026), and Prevotella (R = 0.585, P = 0.017) but negatively correlated with that of Anaeroplasma (R = −0.647, P = 0.007), CF231(R = −0.603, P = 0.013), Dechloromonas (R = −0.620, P = 0.010), Fibrobacter (R = −0.647, P = 0.007), HTCC (R = −0.786, P < 0.001), and Ruminobacter (R = −0.510, P = 0.043). The molar proportion of acetate was positively correlated with the relative abundances of Ruminobacter (R = 0.684, P = 0.003) and Treponema (R = 0.524, P = 0.037) but negatively correlated with that of Bacteroides (R = −0.539, P = 0.031), Bulleidia (R = −0.595, P = 0.015), and Dialister (R = −0.601, P = 0.014). The molar proportion of propionate was positively correlated with the relative abundances of Acidaminococcus (R = 0.527, P = 0.036), Bacteroides (R = 0.578, P = 0.019), Bulleidia (R = 0.616, P = 0.011), and Desulfobulbus (R = 0.735, P = 0.001) but negatively correlated with that of Ruminobacter (R = −0.536, P = 0.032). The molar proportion of butyrate was negatively correlated with the relative abundances of Prevotella (R = −0.526, P = 0.036), Succiniclasticum (R = −0.503, P = 0.047), and Succinivibrio (R = −0.531, P = 0.034). The molar proportion of isobutyrate was positively correlated with the relative abundance of YRC (R = 0.625, P = 0.010) while the molar proportion of valerate was negatively correlated with the relative abundance of Succinivibrio (R = −0.599, P = 0.014). The molar proportion of isovalerate was positively correlated with the relative abundances of Bulleidia (R = 0.624, P = 0.010) and Dialister (R = 0.542, P = 0.030), but negatively correlated with that of Ruminobacter (R = −0.716, P = 0.002) and Treponema (R = −0.565, P = 0.023). The ratio of acetate to propionate was positively correlated with Campylobacter (R = 0.505, P = 0.046), HTCC (R = 0.559, P = 0.024), and Ruminobacter (R = 0.635, P = 0.008) relative abundances but negatively correlated with Acidaminococcus (R = −0.550, P = 0.027), Bacteroides (R = −0.626, P = 0.009), Bulleidia (R = −0.684, P = 0.003), and Dialister (R = −0.760, P = 0.001) relative abundances. Ruminal NH3-N was positively correlated with the relative abundances of Anaeroplasma (R = 0.561, P = 0.019), HTCC (R = 0.559, P = 0.020), Ruminobacter (R = 0.526, P = 0.019), and Treponema (R = 0.545, P = 0.024) but negatively correlated with that of Acidaminococcus (R = −0.564, P = 0.018), Bacteroides (R = −0.704, P = 0.002), Bulleidia (R = −0.559, P = 0.020), Dialister (R = −0.744, P = 0.001), Prevotella (R = −0.549, P = 0.022), Ruminobacter (R = −0.562, P = 0.019), Sharpea (R = −0.555, P = 0.021), Succiniclasticum (R = −0.640, P = 0.006), and Succinivibrio (R = −0.600, P = 0.011). Ruminal MCP concentration was positively correlated with the relative abundances of Acidaminococcus (R = 0.745, P < 0.001), Bulleidia (R = 0.715, P = 0.003), Butyrivibrio (R = 0.666, P = 0.003), Dialister (R = 0.561, P = 0.015), Megasphaera (R = 0.660, P = 0.003), Ruminococcus (R = 0.516, P = 0.029), Selenomonas (R = 0.502, P = 0.034), and Succinivibrio (R = 0.514, P = 0.029) but negatively correlated with that of Anaeroplasma (R = −0.756, P < 0.001), CF231 (R = −0.715, P = 0.001), Dechloromonas (R = −0.686, P = 0.002), Fibrobacter (R = −0.619, P = 0.006), HTCC (R = −0.504, P = 0.033), Roseburia (R = −0.754, P < 0.001), and Ruminobacter (R = −0.507, P = 0.032).
Characterization of the transcriptome in the rumen tissue
A total of 467.71 million (51.97 ± 1.77 million reads per sample) high-quality, paired reads were obtained from nine rumen tissue samples using RNA sequencing, and the alignment rate to the Ovis aries reference genome was 89.01% (from 92.19% to 86.08%). Principal component analysis (PCA) of total gene expression demonstrated marked clustering among the three groups (Figures 5A,C). When compared with the Ctrl group, there were 466 genes that were differentially expressed in the ES group, including 287 upregulated and 179 downregulated genes (Figure 5B). Compared with the ES group, there were 264 DEGs in the ES + EW group, including 238 upregulated and 26 downregulated genes (Figure 5B). Additionally, there were 1,476 DEGs in the ES + EW group compared with the ES group, including 1,161 upregulated and 315 downregulated genes (Figure 5B).
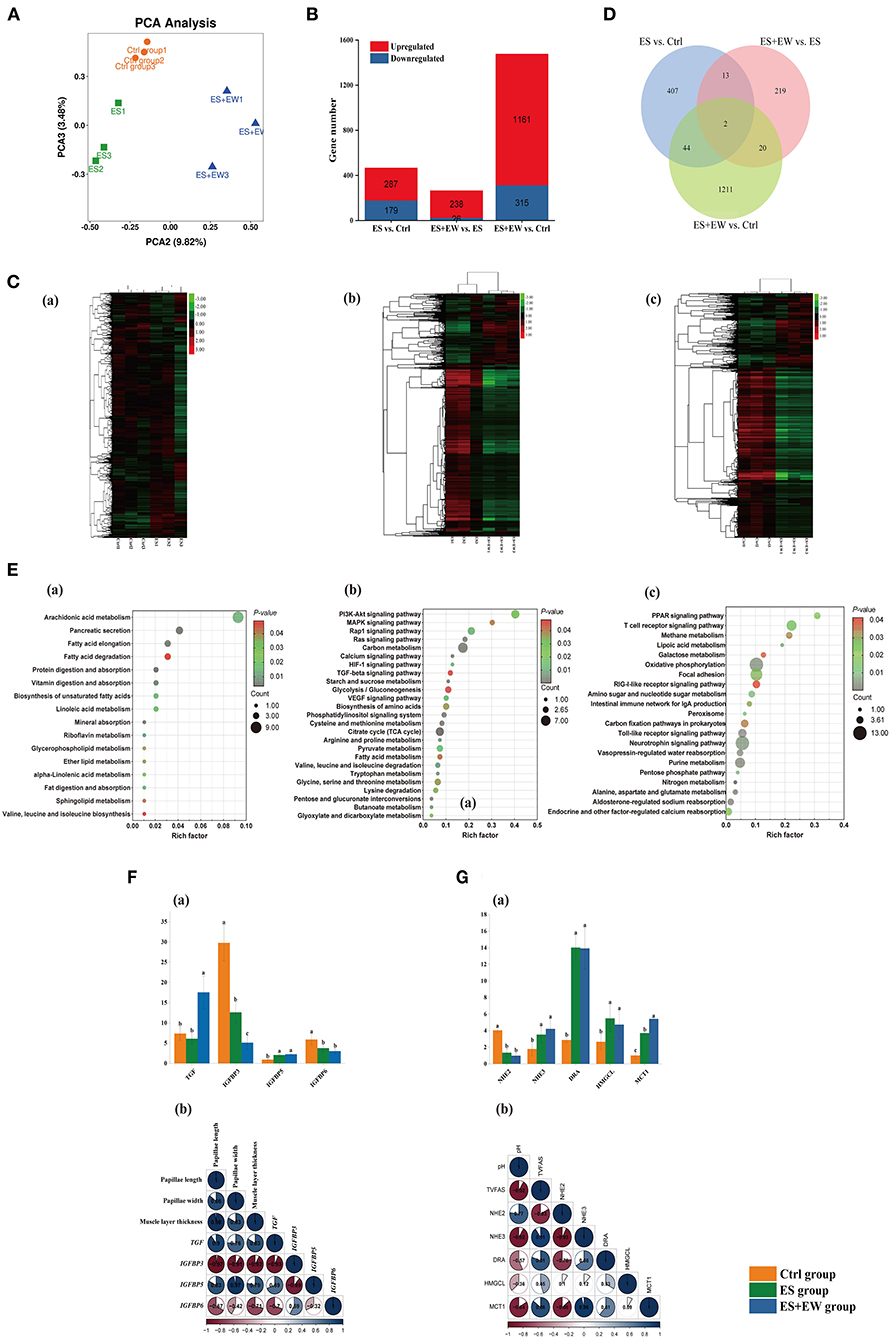
Figure 5. The PCA analysis of gene expressions, number of DEGs, KEGG pathways, and main DEGs in the rumen tissue of lambs in different early feeding strategies at 42 days of age. (A) The PCA analysis of gene expressions in the rumen tissue among three groups. (B) The number of DEGs at each comparison in the different early feeding strategies. (C) Clustering heat-map of the DEGs, including Ctrl vs. ES (a), ES vs. ES+EW (b), and Ctrl vs. ES+EW (c); (D) The Venn diagram of all the comparisons and the numbers of DEGs at different early feeding strategies. (E) The KEGG pathways significantly enriched in the unique DEGs identified in the rumen tissue between Ctrl vs. ES (a), ES vs. ES+EW (b), and Ctrl vs. ES+EW (c), and DEGs in main KEGG pathways. The significance of identified KEGG pathways was determined by P < 0.05. (F,G) The relationship between DGEs expression and phenotypic variables, including gene expression related to the development of rumen epithelial morphology of lambs in three groups, n = 6 (Fa). Correlation heatmap showing the correlation of rumen epithelial morphology and DGEs, n = 6 (Fb). Gene expression related to rumen fermentation of lambs in three groups, n = 6 (Ga); Correlation heatmap showing rumen fermentation correlated with DGEs, n = 6 (Gb). The qRT-PCR measurements of the expression of DGEs were analyzed using 2−ΔΔCT method.
Functional analysis of DEGs
Compared with the Ctrl group, 407 unique DEGs were observed in the rumen tissue of the ES group (Figure 5D). The results of the KEGG pathway analysis showed that 16 pathways were significantly enriched (Figure 5Ea, P < 0.05). Most of these pathways were associated with nutrient transport and lipid metabolism, including arachidonic acid metabolism (including genes PTGES, CBR1, ALOX12B, PLA2G, SPLA2, and AKR1C3), fatty acid elongation (including genes HADH, ELOVL7, HSD17B12, KAR, and IFA38), fatty acid degradation (including genes ACADS, ACSBG, HADH, ACSL, fadD, and ADH1_7), and mineral absorption (including genes SLC26A3, DRA, and CLCN2).
Compared with the ES + EW group, 219 unique DEGs were observed in the rumen tissue of the ES group (Figure 5D). The KEGG pathway analysis revealed 25 significantly enriched pathways (Figure 5Eb, P < 0.05), most of which associated with cell proliferation, apoptosis, and differentiation, such as the PI3K-Akt (including genes PTK2, FAK, PPP2R5, ITGB1, PPP2R1, and ITGB7), MAPK signaling (including genes RPS6KA, RSK2, PPM1B, PP2CB, and MAPK1_3), and TGF-β (including genes TFDP1 and MAPK1_3) pathways.
Additionally, compared with the Ctrl group, 1211 unique DEGs were observed in the rumen tissue of the ES + EW group (Figure 5D). The KEGG pathway analysis showed that 21 pathways were significantly enriched (Figure 5Ec, P < 0.05), including the T-cell receptor signaling pathway (including genes PPP3R, CNB, NFKB1, FOS, JUN, MAPK1_3, DLG1, and RHOA), the intestinal immune network for immunoglobulin A (IgA) production (including genes CXCR4, TNFRSF3, and LTBR), toll-like receptor signaling pathway (including genes MAPK1_3, JUN, FOS, MAP2K3, MKK3), methane metabolism (including genes ACSS, PGAM, and gpmA), and galactose metabolism (including genes UGP2, galU, galF, and GAA). These pathways are also associated with immune functions and carbohydrate metabolism.
Relationship between DEGs and phenotypic variables
Figure 5F shows the relationship between rumen morphology and DEGs (n = 6). Rumen muscle layer thickness (R = 0.831, P = 0.002; R = 0.694, P = 0.035; R = −0.920, P = 0.001; R = −0.705, P = 0.021), and papillae length (R = 0.895, P < 0.001; R = 0.826, P = 0.002; R = −0.966, P < 0.001; R = −0.673, P = 0.032) and width (R = 0.757, P = 0.001; R = 0.967, P < 0.001; R = −0.907, P = 0.001) were positively correlated with TGF and IGFBP5 expression, but negatively correlated with IGFBP3 and IGFBP6 expression. Spearman's rank correlation analysis was used to explore the relationship between rumen fermentation and DEGs (Figure 5G, n = 6). The expression of NHE3 (R = −0.920, P < 0.001; R = 0.905, P = 0.001), DRA (R = −0.573, P = 0.046; R = 0.812, P = 0.017) and MCT1 (R = −0.844, P = 0.004; R = 0.862, P = 0.003) was negatively correlated with ruminal pH but positively correlated with rumen TVFA concentration. Conversely, NHE2 (R = 0.767, P = 0.009; R = −0.826, P = 0.002) expression was positively correlated with ruminal pH but negatively correlated with rumen TVFA concentration.
Discussion
Growth performance
Rumen development in pre-weaning young ruminants influences their adaptation from liquid to solid diets and post-weaning growth performance (53). The present study showed that the average starter diet intake of lambs in the ES + EW group (weaned at 28 days of age) was higher than that in other groups from 28 to 56 days of age. Furthermore, as the lambs were provided with a starter diet at 7 days of age, there was no rapid reduction in the average starter diet intake and body weight (from 28 to 35 days of age) of lambs weaned at 28 days of age. The separation of lambs from their dams while the latter were fed (and the lambs were supplemented) might thus have helped the lambs to successfully adapt to the abrupt separation from their dams at 28 days of age. Such conditions might have favored a smooth transition from milk to solid diets and enabled the lambs to be completely dependent on a solid diet as their source of protein and carbohydrates. However, these results contradict those reported by Carballo et al. (54) and Wang et al. (10). This discrepancy might be explained by the difference in the time at which starter diets were provided to lambs, weaning age, and the composition and nutritional levels of starter diets. A sharp increase in the average starter diet intake was observed from 56 to 70 days of age in lambs in the ES group (weaned at 56 days of age). After changing the diet, lambs in the ES group had a short-term (from 70 to 84 days of age) higher average starter diet intake, body weight, and carcass weight than lambs in the other groups. These results suggest that early life stress may have a long-lasting effect on the performance of lambs (55). It is worth noting that the carcass weight of lambs in the Ctrl group was higher than that of lambs in the other groups at 28 days of age. This might be explained by young ruminants relying solely on the nutrients obtained from milk during the first four weeks of life (53).
Ruminal anatomy, morphology, and fermentation
The anatomical and physiological development of the rumen is one of the most important events during the weaning transition of a young ruminant (56). Previous studies by Baldwin et al. (29) and Diao et al. (9) on rumen development in the pre- and post-weaning stages have shown that at birth, 8 weeks, and 12–16 weeks of age the reticulorumen accounts for 38%, 61.23%, and 67% of the entire stomach weight, respectively. These observations are consistent with those of the present study. Furthermore, early weaning and provision of starter diets to lambs promoted rumen development, which is in accordance with the results of previous studies showing that providing solid feed as early as possible is beneficial to the rapid development of the rumen (20). The physical development of the rumen can be divided into two aspects: an increase in muscle mass and the growth of the papillae. Steele et al. (57) and Aschenbach et al. (58) suggested that the ruminal epithelium plays an important role in ruminal development, including absorption, transport, short-chain fatty acid metabolism, and protection. Similarly, Lesmeister et al. (40) found that ruminal epithelium length was the most important factor for evaluating rumen development, followed by ruminal epithelium width and muscle layer thickness. In the present study, feeding lambs with starter diets stimulated ruminal morphological development, and this effect was more effectively promoted by early weaning. Stimulation of ruminal physical development is determined by the nutritional composition and physical structure of the starter diets fed to lambs (17). In particular, the ruminal epithelium of lambs in the Ctrl group was shorter than that of lambs in the other groups after diet transition (from 70 to 84 days of age), further supporting that early nutritional regulation may have long-term effects on the performance of lambs.
Rumen pH is essential for rumen development, rumen environment, and even lamb health. The ruminal pH of lambs has been reported to be lower than that of adult sheep (59), which is similar to our present results. The lambs in the present study showed no signs of metabolic disorders, suggesting that lambs at this age tolerate pH levels that would be detrimental to the health of adult sheep. In addition, as lambs consumed more of the starter diet, rumen digesta pH decreased, whereas VFA concentrations gradually increased. Liquid diets limit metabolic activity and VFA absorption in the rumen epithelium (60), retarding development. It is noteworthy that early weaning contributes to rapid rumen development, the positive effect of which was maintained for up to 2 weeks after diet transition. Our study found higher molar proportions of acetate in the rumen of lambs fed with starter diets, especially in the EW + ES group. This result was similar to that reported by Terré et al. (61), and might be due to the higher starter diet intake in the EW + ES group, which promoted rumen development. The present study also showed that NH3 concentrations decreased with the increasing age of lambs, which concurs with the results of previous studies (62, 63). The lambs in the ES and EW + ES groups had higher ruminal NH3-N concentrations than lambs in the Ctrl group from 42 to 84 days of age. The subsequent decrease in rumen NH3-N concentration is possibly due to better utilization of NH3 by rumen microorganisms and to the dilution effect arising from a greater rumen volume (64). Microbial proteins play an important role in supplying protein to ruminants and provide most of the amino acids needed for the growth, maintenance, and production of the host animal (65). In the present study, lambs in the Ctrl group had lower MCP levels than lambs in the other groups, confirming that feed intake affects the rate of microbial protein synthesis (66). In summary, starter fermentability and intake play an important role in rumen development.
Rumen bacterial diversity and community structure
The α- and β-diversity of the bacterial community structure of lambs in the Ctrl group were distinct from those of lambs in the other groups. In the present study, early supplementation and weaning caused a decrease in microbial diversity, which is consistent with the results of a previous study (18). Solid diets are the main factor influencing rumen bacterial community structure. The important turning point in microbial colonization is the introduction of a solid diet to young ruminants (67). Kim et al. (22) reported that feeding Holstein calves starter diets with forage was conducive to an increase in microbial richness. As so, the results of the present study are mostly consistent with those of previous studies (18, 68, 69), with slight differences. These differences may be related to the different feeding strategies. In the present study, the rumen microbiota was dominated by Bacteroidetes, Firmicutes, and Proteobacteria, similar to the observations of previous studies (18, 70). The relative abundance of Proteobacteria in the Ctrl group was higher than that in the other groups. Yáñez-Ruiz et al. (71) concluded that the establishment of ruminal bacterial communities in lambs from birth to weaning is rapid, with Proteobacteria being gradually replaced by Bacteroidetes as the main phylum. In ruminants, Firmicutes play an important role in degrading fiber (22), while the main function of Bacteroidetes is to degrade carbohydrates and proteins, which can encourage the functional development of gastrointestinal immunity (72). In the present study, lambs in the Ctrl group had a lower abundance of Bacteroidetes than lambs in the other groups. Malmuthuge et al. (73) reported that providing a starter diet could propel rumen microbiota development to a more mature status. The abundance of Verrucomicrobia was second only to Bacteroidetes in the Ctrl group and was substantially higher than that in the other groups. The present results are also consistent with those of a previous study (74). Verrucomicrobia have been suggested to coevolve with the mammalian gut, as this phylum plays an important role in maintaining gut homeostasis. Shen et al. (75) reported that Verrucomicrobia modulate the expression of pathways related to the immune tolerance of the rumen epithelium. At the genus level, the abundance of Acidaminococcus was higher in the ES + EW group than in the other groups. Acidaminococcus is a genus in the phylum Firmicutes, and its members are anaerobic diplococci that can use amino acids as their sole energy source for growth. Acidaminococcus may be responsible for the increased metabolism of amino acids, carbohydrates, and lipids (76). According to the dietary intake analysis of the three groups, the higher intake of starter diets containing high levels of carbohydrates and amino acids after early weaning probably provided more nutrients for metabolism and growth of Acidaminococcus. Additionally, lambs in the ES + EW group had higher Mitsuokella abundance than lambs in the other groups. Zhang et al. (77) found that Mitsuokella abundance was positively correlated with dry matter intake in calves, similar to the present results. Lambs fed with solid diets before weaning had a greater abundance of Prevotella. McLoughlin et al. (78) indicate that Prevotella is driven by dietary composition and independent of weaning. Our results support previous observations showing that the inclusion of starter concentrate in the diet of pre-weaning lambs promotes rumen colonization by Succiniclasticum (79). In short, feeding pre-weaning lambs with starter diets can change the structure of the rumen bacterial community and contribute to convergence toward the microbiota profile of adult animals. Early weaning increases the starter intake of lambs, thereby stimulating rumen microbial colonization.
Molecular mechanism of early weaning and effect of early supplementation on ruminal morphology and functional development based on transcriptome analysis
Compared with the Ctrl group, 407 unique genes were differentially expressed in the ES group. The enriched KEGG pathways were mainly related to nutrient transport and metabolism in rumen tissue. Fatty acids are essential for cell proliferation in the cellular membranes (80). According to our findings, the upregulated genes HADH, HSD17B12, KAR, IFA38, ACADS, ACSL, fadD, and ADH1_7 were enriched in the fatty acid elongation and degradation pathway, which is beneficial to the proliferation and turnover of rumen epithelial cells. Previous studies have identified that these genes are associated with fatty acid synthesis and deposition (81–83). This may have contributed to the higher carcass weight of lambs in the ES group than in the Ctrl group from 56 to 84 days of age. Moreover, arachidonic acid metabolism is a significantly enriched signaling pathway in ruminal tissue of lambs fed with solid diets (84), which is consistent with our results. It is well established that arachidonic acid regulates cellular inflammation, oxidative stress, proliferation, and membrane permeability (85), which may affect rumen immune function. In the present study, the expression of SLC26A3 and DRA was increased in the ES group, and enriched in ion and mineral absorption pathways, which has been shown to be conducive to ruminal absorption of VFA (27). Taken together, these results suggest that early supplementation may enhance nutrient metabolism and transport in pre-weaned lambs.
Functional analysis of the 219 unique DEGs in the ES + EW vs. ES groups showed that early weaning affected cell proliferation, apoptosis, and differentiation. Compared with lambs in the ES group, 13 upregulated genes (PTK2, FAK, PPP2R5, ITGB1, PPP2R1, RPS6KA, RSK2, PPM1B, PP2CB, CALM, CTNNB1, TFDP1 and MAPK1_3) and one downregulated gene (ITGB7) were enriched in the PI3K-Akt, MAPK, Rap1, Ras, and TGF-β signaling pathways of ES + EW lambs. All of these genes contribute to the establishment of the rumen epithelial function and barrier (86, 87). Notably, we also identified many signaling pathways involved in amino acid metabolism. Previous studies have suggested that a comparatively low number of amino acids and peptides may be absorbed and metabolized by the rumen tissue (88). However, the development of the rumen epithelium requires increased cell and protein turnover (25, 89). In the present study, many upregulated DEGs in the ES + EW group were annotated in pathways related to amino acid metabolism, including biosynthesis of amino acids, cysteine and methionine metabolism, arginine and proline metabolism, valine, leucine, and isoleucine degradation; glycine, serine, and threonine metabolism; and lysine degradation. In summary, we hypothesize that MCP synthesis might promote protein turnover and oxidation in the rumen. These metabolites are substrates for the citric acid cycle, generate energy-containing compounds, are responsible for the activation of some biological processes, and might further promote rumen epithelium development (84). Therefore, early weaning increases the intake of solids in lambs, promoting nutrient transport, degradation (especially of nitrogenous substances), and absorption in the rumen epithelium.
Compared to the Ctrl group, 1,121 unique genes were differentially expressed in the ES + EW group. These genes were mainly enriched in the biological processes of immune function and nutrient metabolism, which play important roles in rumen development. Naeem et al. (80) suggested that the peroxisome proliferator-activated receptor (PPAR) signaling pathway could be important in promoting rumen metabolism and development. The PPAR signaling pathway was the most significantly altered pathway induced by the interaction between early supplementation and early weaning. Toll-like receptors (TLRs) play a critical role in suppressing inflammation in the gastrointestinal epithelium by reducing the production of inflammatory cytokines. Shen et al. (75) found that the carbohydrates in the starter diet induced the expansion of the rumen microbiota and promoted epithelium tolerance by enhancing the intensity of toll-like signaling, and the newly established equilibrium benefited the transport of ruminal energy substances into the blood. Similarly, during the analysis of shared DEGs in the toll-like signaling pathway, the expression of toll-like signaling pathway genes in the ES + EW group was lower than that in the Ctrl group. Taking these results together, we hypothesize that there is better resistance to inflammation and a greater ability to transport energy substances within the rumen tissue of ES + EW lambs.
Hayashi et al. (90) indicated that insulin-like growth factor 1 (IGF1) can stimulate epithelial cell proliferation and differentiation to enhance ruminal papillae development. IGF1 induces a cellular response by regulating IGFBPs. When IGFBP5 is upregulated, it potentiates IGF1 effects, stimulating the proliferation of rumen epithelium, whereas IGFBP3 regulates this process in the opposite direction (91). In the present study, early supplementation increased IGFBP5 expression and decreased IGFBP3 expression, which should promote rumen epithelial cell proliferation to facilitate the development of ruminal morphology. NHEs are apical membrane sodium ion (Na+)-hydrogen ion (H+) anti-porters involved in the regulation of intracellular pH. The expression of NHEs is upregulated by butyrate; however, the long-term regulation of NHEs is primarily accomplished by changes in transcription (92). In the present study, the mRNA expression of NHE2 was higher in the Ctrl group than in the other groups, while NHE3 expression was lower in the Ctrl group than in the other groups. NHE3 imports Na+ to the cell and exports H+ to the rumen, while NHE2 imports H+ to the cell and exports Na+ to the extracellular space (27). Therefore, the lower NHE2 expression and higher NHE3 expression may demonstrate decreased H+ recycling into the lumen and a greater net H+ uptake by the rumen epithelium to maintain a stable rumen environment. Schurmann et al. (93) proposed that DRA is responsible for neutralizing acid in the rumen by exporting bicarbonate ions from epithelial cells and importing dissociated VFAs. MCT1, which is localized in the basolateral membrane, is involved in the basolateral export of ketone bodies arising from butyrate metabolism and lactate arising from propionate metabolism (94). The expressions of DRA and MCT1 in the Ctrl group were lower than that in the other groups, which concurs with the results of Laarman et al. (95). These results may explain why early supplementation leads to an increase in VFA concentration within the rumen.
Conclusion
In summary, feeding starter diets to lambs is a turning point in rumen development, and it accelerates rumen development with increased feed intake after weaning. The present study indicated that feeding lambs with starter diets at 7 days of age and weaning them at 28 days of age can stimulate rumen morphological development, promote rumen microbial colonization, and improve rumen epithelial anti-inflammatory and nutrient transport capacities. The positive effects of this early feeding strategy on lamb development persisted for up to 2 weeks after the lambs changed to fattening diets. However, our study has only examined the short-term effects of early rearing practices after changing to fattening diets, and more work is necessary for understanding the long-term influence of early rearing on lambs.
Data availability statement
The raw sequence data obtained in this study can be found on the NCBI Sequence Read Archive under accession numbers PRJNA594822 and PRJNA317746.
Ethics statement
All experimental protocols and sample collection methods were approved by the Ethics Committee of Gansu Agriculture University under Permit No. 2012-2-159.
Author contributions
FL and WW designed the study. TL, XWa, ZM, and CL conducted the experiment and sample collection. TL, WW, XWa, and ZM performed data analysis. TL and CZ produced the initial draft of the manuscript and contributed to the revision of the manuscript. XWe revised the manuscript and provided the experimental resources. All authors reviewed and approved the final manuscript.
Funding
This study was supported by grants from the National Natural Science Foundation of China (31860656), the Youth Science and Technology Fund Program of Gansu Province (20JR10RA553), the Provincial Natural Fund of Gansu (20IR1ORA538), and the Special Talent Introduction Program of Gansu Agricultural University (GSAU-RCZX201710).
Conflict of interest
The authors declare that the research was conducted in the absence of any commercial or financial relationships that could be construed as a potential conflict of interest.
Publisher's note
All claims expressed in this article are solely those of the authors and do not necessarily represent those of their affiliated organizations, or those of the publisher, the editors and the reviewers. Any product that may be evaluated in this article, or claim that may be made by its manufacturer, is not guaranteed or endorsed by the publisher.
Supplementary material
The Supplementary Material for this article can be found online at: https://www.frontiersin.org/articles/10.3389/fvets.2022.925649/full#supplementary-material
References
1. Ramkrishna V, Tiwari GP. Histological and histochemical observations on the forestomach of goat during pre-natal life. Acta Anat (Basel). (1979) 103:292–300.
2. Bhatt RS, Tripathi MK, Verma DL, Karim SA. Effect of different feeding regimes on pre-weaning growth rumen fermentation and its influence on post-weaning performance of lambs. J Anim Physiol Anim Nutr. (2009) 93:568–76. doi: 10.1111/j.1439-0396.2008.00845.x
3. Scharrer E, Medl M, Liebich HG. Changes in the structure and function of the rumen epithelium during development. 3 effect of liquid versus solid diet on Na and Cl transport across lamb rumen epithelium. Zentralbl Veterinarmed A. (1983) 30:767–74.
4. Lane MA. Baldwin RLt, Jesse BW. Sheep rumen metabolic development in response to age and dietary treatments. J Anim Sci. (2000) 78:1990–6. doi: 10.2527/2000.7871990x
5. Morgavi DP, Rathahao-Paris E, Popova M, Boccard J, Nielsen KF, Boudra H. Rumen microbial communities influence metabolic phenotypes in lambs. Front Microbiol. (2015) 6:1060. doi: 10.3389/fmicb.2015.01060
6. Norouzian MA, Valizadeh R. Effect of forage inclusion and particle size in diets of neonatal lambs on performance and rumen development. J Anim Physiol Anim Nutr. (2014) 98:1095–101. doi: 10.1111/jpn.12183
7. Wang W, Li C, Li F, Wang X, Zhang X, Liu T, et al. Effects of early feeding on the host rumen transcriptome and bacterial diversity in lambs. Sci Rep. (2016) 6:32479. doi: 10.1038/srep32479
8. Li C, Zhang Q, Wang G, Niu X, Wang W, Li F, et al. The functional development of the rumen is influenced by weaning and associated with ruminal microbiota in lambs. Anim Biotechnol. (2020) 2020:1–17. doi: 10.1080/10495398.2020.1812618
9. Diao Q, Zhang R, Fu T. Review of strategies to promote rumen development in calves. Anim (Basel). (2019) 9:490. doi: 10.3390/ani9080490
10. Wang S, Ma T, Zhao G, Zhang N, Tu Y, Li F, et al. Effect of age and weaning on growth performance, rumen fermentation, and serum parameters in lambs fed starter with limited ewe-lamb interaction. Anim (Basel). (2019) 9:825. doi: 10.3390/ani9100825
11. Hinch G, Lynch J, Elwin R, Green GJAABS. Long-term associations between merino ewes and their offspring. Appl Anim Behav Sci. (1990) 27:93–103.
12. Campbell B, Pullin A, Pairis-Garcia M, McCutcheon J, Lowe G, Campler M, et al. The effects of alternative weaning strategies on lamb health and performance. Small Ruminant Res. (2017) 156:57–65. doi: 10.1016/j.smallrumres.2017.09.006
13. Myers S, Faulkner D, Ireland F, Berger L, Parrett DF. Production systems comparing early weaning to normal weaning with or without creep feeding for beef steers. J Anim l Sci. (1999) 77:300–10.
14. Yin X, Ji S, Duan C, Tian P, Ju S, Yan H, et al. Age-related changes in the ruminal microbiota and their relationship with rumen fermentation in lambs. Front Microbiol. (2021) 12. doi: 10.3389/fmicb.2021.679135
15. Mao H, Zhang Y, Yun Y, Ji W, Jin Z, Wang C, et al. weaning age affects the development of the ruminal bacterial and archaeal community in Hu lambs during early life. Front Microbiol. (2021) 12:636865. doi: 10.3389/fmicb.2021.636865
16. Lambertz C, Farke-Rover A, Moors E, Gauly M. Effects of castration and weaning conducted concurrently or consecutively on behaviour, blood traits and performance in beef calves. Animal. (2015) 9:122–9. doi: 10.1017/S1751731114002080
17. McCoard SA, Cristobal-Carballo O, Knol FW, Heiser A, Khan MA, Hennes N, et al. Impact of early weaning on small intestine, metabolic, immune and endocrine system development, growth and body composition in artificially reared lambs. J Anim Sci. (2020) 98:skz356. doi: 10.1093/jas/skz356
18. Wang S, Chai J, Zhao G, Zhang N, Cui K, Bi Y, et al. The temporal dynamics of rumen microbiota in early weaned lambs. Microorganisms (2022) 10:144. doi: 10.3390/microorganisms10010144
19. Li C, Wang W, Liu T, Zhang Q, Wang G, Li F, et al. Effect of early weaning on the intestinal microbiota and expression of genes related to barrier function in lambs. Front Microbiol. (2018) 9:1431. doi: 10.3389/fmicb.2018.01431
20. Yang B, Le J, Wu P, Liu J, Guan LL, Wang J. Alfalfa intervention alters rumen microbial community development in hu lambs during early life. Front Microbiol. (2018) 9:574. doi: 10.3389/fmicb.2018.00574
21. Yang B, He B, Wang SS, Liu JX, Wang JK. Early supplementation of starter pellets with alfalfa improves the performance of pre- and postweaning Hu lambs. J Anim Sci. (2015) 93:4984–94. doi: 10.2527/jas.2015-9266
22. Kim YH, Nagata R, Ohtani N, Ichijo T, Ikuta K, Sato S. Effects of dietary forage and calf starter diet on ruminal Ph and bacteria in holstein calves during weaning transition. Front Microbiol. (2016) 7:1575. doi: 10.3389/fmicb.2016.01575
23. Saeedi S, Dayani O, Tahmasbi R, Khezri A. Effect of supplementation of calf starter with fennel powder on performance, weaning age and fermentation characteristics in holstein dairy calves. J Anim Physiol Anim Nutr (Berl). (2017) 101:81–7. doi: 10.1111/jpn.12511
24. Soltani Nezhad B, Dayani O, Khezri A, Tahmasbi R. Performance and carcass characteristics in fattening lambs feed diets with different levels of pistachio by-products silage with wasted date. Small Ruminant Res. (2016) 137:177–82. doi: 10.1016/j.smallrumres.2016.03.015
25. Sun D, Mao S, Zhu W, Liu J. proteomic identification of ruminal epithelial protein expression profiles in response to starter feed supplementation in pre-weaned lambs. Anim Nutr. (2021) 7:1271–82. doi: 10.1016/j.aninu.2021.06.014
26. Liu T, Li F, Wang W, Yue X, Li F, Li C, et al. Effects of lamb early starter feeding on the expression of genes involved in volatile fatty acid transport and Ph regulation in rumen tissue. Anim Feed Sci Technol. (2016) 217:27–35. doi: 10.1016/j.anifeedsci.2016.04.006
27. Liu L, Sun D, Mao S, Zhu W, Liu J. Infusion of sodium butyrate promotes rumen papillae growth and enhances expression of genes related to rumen epithelial Vfa uptake and metabolism in neonatal twin lambs. J Anim Sci. (2019) 97:909–21. doi: 10.1093/jas/sky459
28. Montoro C, Miller-Cushon EK, DeVries TJ, Bach A. Effect of physical form of forage on performance, feeding behavior, and digestibility of Holstein calves. J Dairy Sci. (2013) 96:1117–24. doi: 10.3168/jds.2012-5731
29. Baldwin R, McLeod K, Klotz J, Heitmann RN. Rumen development, intestinal growth and hepatic metabolism in the pre-and postweaning ruminant. J Dairy Sci. (2004) 87:E55–65. doi: 10.3168/jds.S0022-0302(04)70061-2
30. Diao Q, Zhang R, Yan T. Current research progresses on calf rearing and nutrition in China. J Integrat Agric. (2017) 16:2805–14. doi: 10.1016/S2095-3119(17)61767-2
31. Liu J, Bian G, Sun D, Zhu W, Mao S. Starter feeding supplementation alters colonic mucosal bacterial communities and modulates mucosal immune homeostasis in newborn lambs. Front Microbiol. (2017) 8:429. doi: 10.3389/fmicb.2017.00429
32. Zhang Y, Choi SH, Nogoy KM, Liang S. Review: the development of the gastrointestinal tract microbiota and intervention in neonatal ruminants. Animal. (2021) 15:100316. doi: 10.1016/j.animal.2021.100316
33. Abecia L, Martin-Garcia AI, Martinez G, Newbold CJ, Yanez-Ruiz DR. Nutritional intervention in early life to manipulate rumen microbial colonization and methane output by kid goats postweaning. J Anim Sci. (2013) 91:4832–40. doi: 10.2527/jas.2012-6142
34. Council NR (NRC). Nutrient Requirements of Small Ruminants: Sheep, Goats, Cervids, and New World Camelids. Washington: National Academic Press (2007).
36. MacRae J, Smith D, McCready RM. Starch estimation in leaf tissue—a comparison of results using six methods. J Sci Food Agric. (1974) 25:1465–9.
37. Van Soest PJ, Robertson JB, Lewis BA. Methods for dietary fiber, neutral detergent fiber, and nonstarch polysaccharides in relation to animal nutrition. J Dairy Sci. (1991) 74:3583–97.
38. Broderick GA, Kang JH. Automated simultaneous determination of ammonia and total amino acids in ruminal fluid and in vitro media. J Dairy Sci. (1980) 63:64–75.
39. Makkar HP, Sharma OP, Dawra RK, Negi SS. Simple determination of microbial protein in rumen liquor. J Dairy Sci. (1982) 65:2170–3.
40. Lesmeister KE, Tozer PR, Heinrichs AJ. Development and analysis of a rumen tissue sampling procedure. J Dairy Sci. (2004) 87:1336–44. doi: 10.3168/jds.S0022-0302(04)73283-X
41. Berg J, Brandt KK, Al-Soud WA, Holm PE, Hansen LH, Sorensen SJ, et al. Selection for Cu-tolerant bacterial communities with altered composition, but unaltered richness, via long-term Cu exposure. Appl Environ Microbiol. (2012) 78:7438–46. doi: 10.1128/AEM.01071-12
42. Edgar RC, Haas BJ, Clemente JC, Quince C, Knight R. Uchime improves sensitivity and speed of chimera detection. Bioinformatics. (2011) 27:2194–200. doi: 10.1093/bioinformatics/btr381
43. Schloss PD, Westcott SL, Ryabin T, Hall JR, Hartmann M, Hollister EB, et al. Introducing mothur: open-source, platform-independent, community-supported software for describing and comparing microbial communities. Appl Environ Microbiol. (2009) 75:7537–41. doi: 10.1128/AEM.01541-09
44. Fomenky BE, Chiquette J, Bissonnette N, Talbot G, Chouinard PY, Ibeagha-Awemu EMJAFS, et al. Impact of saccharomyces Cerevisiae boulardii Cncmi-1079 and lactobacillus acidophilus Bt1386 on total lactobacilli population in the gastrointestinal tract and colon histomorphology of holstein dairy calves. Anim Feed Sci Technol. (2017) 234:151–61. doi: 10.1016/j.anifeedsci.2017.08.019
45. Quast C, Pruesse E, Yilmaz P, Gerken J, Schweer T, Yarza P, et al. The silva ribosomal RNA gene database project: improved data processing and web-based tools. Nucl Acids Res. (2013) 41:D590–6. doi: 10.1093/nar/gks1219
46. Lee C, Harris RA, Wall JK, Mayfield RD, Wilke CO. Rnaseiii and T4 polynucleotide kinase sequence biases and solutions during Rna-Seq library construction. Biol Direct. (2013) 8:16. doi: 10.1186/1745-6150-8-16
47. Bolger AM, Lohse M, Usadel B. Trimmomatic: a flexible trimmer for illumina sequence data. Bioinformatics. (2014) 30:2114–20. doi: 10.1093/bioinformatics/btu170
48. Kim D, Pertea G, Trapnell C, Pimentel H, Kelley R, Salzberg SL. Tophat2: accurate alignment of transcriptomes in the presence of insertions, deletions and gene fusions. Genome Biol. (2013) 14:R36. doi: 10.1186/gb-2013-14-4-r36
49. Langmead B, Salzberg SL. Fast gapped-read alignment with bowtie 2. Nat Methods. (2012) 9:357–9. doi: 10.1038/nmeth.1923
50. Kim D, Langmead B, Salzberg SL. Hisat: a fast spliced aligner with low memory requirements. Nat Methods. (2015) 12:357–60. doi: 10.1038/nmeth.3317
51. Robinson MD, McCarthy DJ, Smyth GK. Edger: a bioconductor package for differential expression analysis of digital gene expression data. Bioinformatics. (2010) 26:139–40. doi: 10.1093/bioinformatics/btp616
52. Xie C, Mao X, Huang J, Ding Y, Wu J, Dong S, et al. Kobas 2.0: a web server for annotation and identification of enriched pathways and diseases. Nucl Acids Res. (2011) 39:W316–22. doi: 10.1093/nar/gkr483
53. Khan MA, Bach A, Weary DM, von Keyserlingk MAG. Invited review: transitioning from milk to solid feed in dairy heifers. J Dairy Sci. (2016) 99:885–902. doi: 10.3168/jds.2015-9975
54. Carballo OC, Khan MA, Knol FW, Lewis SJ, Stevens DR, Laven RA, et al. Impact of weaning age on rumen development in artificially reared lambs1. J Anim Sci. (2019) 97:3498–510. doi: 10.1093/jas/skz148
55. Chai J, Ma T, Wang H, Qi M, Tu Y, Diao Q, et al. Effect of early weaning age on growth performance, nutrient digestibility, and serum parameters of lambs. Anim Prod Sci. (2016) 57:110–5. doi: 10.1071/AN15079
56. Niekerk JK, Middeldorp M, Guan LL, Steele MA. Preweaning to postweaning rumen papillae structural growth, ruminal fermentation characteristics, and acute-phase proteins in calves. J Dairy Sci. (2021) 104:3632–45. doi: 10.3168/jds.2020-19003
57. Steele MA, Penner GB, Chaucheyras-Durand F, Guan LL. Development and physiology of the rumen and the lower gut: targets for improving gut health. J Dairy Sci. (2016) 99:4955–66. doi: 10.3168/jds.2015-10351
58. Aschenbach JR, Zebeli Q, Patra AK, Greco G, Amasheh S, Penner GB. Symposium review: the importance of the ruminal epithelial barrier for a healthy and productive cow. J Dairy Sci. (2019) 102:1866–82. doi: 10.3168/jds.2018-15243
59. Guo J, Li P, Zhang K, Zhang L, Wang X, Li L, et al. Distinct stage changes in early-life colonization and acquisition of the gut microbiota and its correlations with volatile fatty acids in goat kids. Front Microbiol. (2020) 11:584742. doi: 10.3389/fmicb.2020.584742
60. Lesmeister KE, Heinrichs AJ. Effects of adding extra molasses to a texturized calf starter on rumen development, growth characteristics, and blood parameters in neonatal dairy calves. J Dairy Sci. (2005) 88:411–8. doi: 10.3168/jds.S0022-0302(05)72702-8
61. Terré M, Pedrals E, Dalmau A, Bach A. What do preweaned and weaned calves need in the diet: a high fiber content or a forage source? J Dairy Sci. (2013) 96:5217–25. doi: 10.3168/jds.2012-6304
62. Kazemi-Bonchenari M, Mirzaei M, Jahani-Moghadam M, Soltani A, Mahjoubi E, Patton RA. Interactions between levels of heat-treated soybean meal and prilled fat on growth, rumen fermentation, and blood metabolites of Holstein calves. J Anim Sci. (2016) 94:4267–75. doi: 10.2527/jas.2016-0514
63. Holtshausen L, Cruywagen C. The effect of age on in sacco estimates of rumen dry matter and crude protein degradability in veal calves. S Afr J Anim Sci. (2000) 30:212–9. doi: 10.4314/sajas.v30i3.3854
64. Hill T, Bateman II H, Aldrich J, Schlotterbeck R. Crude protein for diets fed to weaned dairy calves. Prof Anim Sci. (2008) 24:596–603. doi: 10.15232/S1080-7446(15)30910-4
65. Vaithiyanathan S, Bhatta R, Mishra A, Prasad R, Verma D, Singh NP. Effect of Feeding Graded Levels of Prosopis Cineraria Leaves on Rumen Ciliate Protozoa, Nitrogen Balance and Microbial Protein Supply in Lambs and Kids. Anim Feed Sci Technol. (2007) 133:177–91. doi: 10.1016/j.anifeedsci.2006.04.003
66. Direkvandi E, Mohammadabadi T, Salem AZM. Effect of microbial feed additives on growth performance, microbial protein synthesis, and rumen microbial population in growing lambs. Transl Anim Sci. (2020) 4:txaa203. doi: 10.1093/tas/txaa203
67. Rey M, Enjalbert F, Combes S, Cauquil L, Bouchez O, Monteils V. Establishment of ruminal bacterial community in dairy calves from birth to weaning is sequential. J Appl Microbiol. (2014) 116:245–57. doi: 10.1111/jam.12405
68. Cristobal-Carballo O, McCoard SA, Cookson AL, Laven RA, Ganesh S, Lewis SJ, et al. Effect of divergent feeding regimes during early life on the rumen microbiota in calves. Front Microbiol. (2021) 12:711040. doi: 10.3389/fmicb.2021.711040
69. Jami E, Israel A, Kotser A, Mizrahi I. Exploring the bovine rumen bacterial community from birth to adulthood. ISME J. (2013) 7:1069–79. doi: 10.1038/ismej.2013.2
70. Wang Y, Zhang H, Zhu L, Xu Y, Liu N, Sun X, et al. Dynamic distribution of gut microbiota in goats at different ages and health states. Front Microbiol. (2018) 9:2509. doi: 10.3389/fmicb.2018.02509
71. Yáñez-Ruiz DR, Abecia L, Newbold C. Manipulating rumen microbiome and fermentation through interventions during early life: a review. Front Microbiol. (2015) 6:1133. doi: 10.3389/fmicb.2015.01133
72. Abdul Rahman N, Parks DH, Vanwonterghem I, Morrison M, Tyson GW, Hugenholtz P, et al. Phylogenomic analysis of the bacterial phylum fibrobacteres. Front Microbiol. (2015) 6:1469. doi: 10.3389/fmicb.2015.01469
73. Malmuthuge N, Griebel PJ, Guan LL. Taxonomic identification of commensal bacteria associated with the mucosa and digesta throughout the gastrointestinal tracts of preweaned calves. Appl Environ Microbiol. (2014) 80:2021–8. doi: 10.1128/AEM.03864-13
74. Han Z, Li A, Pei L, Li K, Jin T, Li F, et al. Milk replacer supplementation ameliorates growth performance and rumen microbiota of early-weaning yimeng black goats. Front Vet Sci. (2020) 7:572064. doi: 10.3389/fvets.2020.572064
75. Shen H, Lu Z, Chen Z, Wu Y, Shen Z. Rapid fermentable substance modulates interactions between ruminal commensals and toll-like receptors in promotion of immune tolerance of goat rumen. Front Microbiol. (2016) 7:1812. doi: 10.3389/fmicb.2016.01812
76. Mayorga OL, Kingston-Smith AH, Kim EJ, Allison GG, Wilkinson TJ, Hegarty MJ, et al. Temporal metagenomic and metabolomic characterization of fresh perennial ryegrass degradation by rumen bacteria. Front Microbiol. (2016) 7:1854. doi: 10.3389/fmicb.2016.01854
77. Zhang Y, Cheng J, Zheng N, Zhang Y, Jin D. Different milk replacers alter growth performance and rumen bacterial diversity of dairy. Bull Calves Livestock Sci. (2020) 231:103862. doi: 10.1016/j.livsci.2019.103862
78. McLoughlin S, Spillane C, Claffey N, Smith PE, O'Rourke T, Diskin MG, et al. Rumen microbiome composition is altered in sheep divergent in feed efficiency. Front Microbiol. (2020) 11:1981. doi: 10.3389/fmicb.2020.01981
79. Dias J, Marcondes MI, Noronha MF, Resende RT, Machado FS, Mantovani HC, et al. Effect of pre-weaning diet on the ruminal archaeal, bacterial, and fungal communities of dairy calves. Front Microbiol. (2017) 8:1553. doi: 10.3389/fmicb.2017.01553
80. Naeem A, Drackley JK, Stamey J, Loor JJ. Role of metabolic and cellular proliferation genes in ruminal development in response to enhanced plane of nutrition in neonatal holstein calves. J Dairy Sci. (2012) 95:1807–20. doi: 10.3168/jds.2011-4709
81. Yuan Z, Ge L, Sun J, Zhang W, Wang S, Cao X, et al. Integrative analysis of Iso-Seq and Rna-Seq data reveals transcriptome complexity and differentially expressed transcripts in sheep tail fat. PeerJ. (2021) 9:e12454. doi: 10.7717/peerj.12454
82. Urrutia O, Mendizabal J, Insausti K, Soret B, Purroy A, Arana AJLS. Effect of linseed dietary supplementation on adipose tissue development, fatty acid composition, and lipogenic gene expression in lambs. Livest Sci. (2015) 178:345–56. doi: 10.1016/j.livsci.2015.05.006
83. Miao X, Luo Q, Qin X, Guo Y, Zhao HJB. Genome-wide Mrna-Seq profiling reveals predominant down-regulation of lipid metabolic processes in adipose tissues of small tail han than dorset sheep. Biochem Biophys Res Commun. (2015) 467:413–20. doi: 10.1016/j.bbrc.2015.09.129
84. Sun D, Yin Y, Guo C, Liu L, Mao S, Zhu W, et al. Transcriptomic analysis reveals the molecular mechanisms of rumen wall morphological and functional development induced by different solid diet introduction in a lamb model. J Anim Sci Biotechnol. (2021) 12:33. doi: 10.1186/s40104-021-00556-4
85. Steele MA, Dionissopoulos L, AlZahal O, Doelman J, McBride BW. Rumen epithelial adaptation to ruminal acidosis in lactating cattle involves the coordinated expression of insulin-like growth factor-binding proteins and a cholesterolgenic enzyme. J Dairy Sci. (2012) 95:318–27. doi: 10.3168/jds.2011-4465
86. Zhang R, Zhu W, Mao S. High-concentrate feeding upregulates the expression of inflammation-related genes in the ruminal epithelium of dairy cattle. J Anim Sci Biotechnol. (2016) 7:42. doi: 10.1186/s40104-016-0100-1
87. Zhang R, Liu J, Jiang L, Mao S. Effect of high-concentrate diets on microbial composition, function, and the VFAs formation process in the rumen of dairy cows. Anim Feed Sci Technol. (2020) 269:114619. doi: 10.1016/j.anifeedsci.2020.114619
88. Huntington G, Poore M, Hopkins B, Spears J. Effect of ruminal protein degradability on growth and N metabolism in growing beef steers. J Anim Sci. (2001) 79:533–41. doi: 10.2527/2001.792533x
89. Quigley JD. Symposium review: re-evaluation of national research council energy estimates in calf starters. J Dairy Sci. (2019) 102:3674–83. doi: 10.3168/jds.2018-15367
90. Hayashi K, Carpenter KD, Welsh TH, Burghardt RC, Spicer LJ, Spencer TE. The Igf system in the neonatal ovine uterus. Reproduction. (2005) 129:337–47. doi: 10.1530/rep.1.00342
91. Ferry RJ, Katz LE, Grimberg A, Cohen P, Weinzimer SA. Cellular actions of insulin-like growth factor binding proteins. Horm Metab Res. (1999) 31:192–202.
92. Malo ME, Fliegel L. Physiological role and regulation of the Na+/H+ exchanger. Can J Physiol Pharmacol. (2006) 84:1081–95. doi: 10.1139/y06-065
93. Schurmann BL, Walpole ME, Gorka P, Ching JC, Loewen ME, Penner GB. Short-term adaptation of the ruminal epithelium involves abrupt changes in sodium and short-chain fatty acid transport. Am J Physiol Regul Integr Comp Physiol. (2014) 307:R802–16. doi: 10.1152/ajpregu.00035.2014
94. Muller F, Huber K, Pfannkuche H, Aschenbach JR, Breves G, Gabel G. Transport of ketone bodies and lactate in the sheep ruminal epithelium by monocarboxylate transporter 1. Am J Physiol Gastrointest Liver Physiol. (2002) 283:G1139–46. doi: 10.1152/ajpgi.00268.2001
Keywords: early supplementation, early weaning, lamb, rumen development, growth performance
Citation: Liu T, Li F, Wang W, Wang X, Ma Z, Li C, Weng X and Zheng C (2022) Early feeding strategies in lambs affect rumen development and growth performance, with advantages persisting for two weeks after the transition to fattening diets. Front. Vet. Sci. 9:925649. doi: 10.3389/fvets.2022.925649
Received: 22 April 2022; Accepted: 04 July 2022;
Published: 28 July 2022.
Edited by:
Xuezhao Sun, Jilin Agricultural Science and Technology University, ChinaReviewed by:
Jianmin Chai, Foshan University, ChinaParniyan Goodarzi, Parniyan Goodarzi, United States
Juan Pinos-Rodriguez, Universidad Veracruzana, Mexico
Amin Khezri, Shahid Bahonar University of Kerman, Iran
Copyright © 2022 Liu, Li, Wang, Wang, Ma, Li, Weng and Zheng. This is an open-access article distributed under the terms of the Creative Commons Attribution License (CC BY). The use, distribution or reproduction in other forums is permitted, provided the original author(s) and the copyright owner(s) are credited and that the original publication in this journal is cited, in accordance with accepted academic practice. No use, distribution or reproduction is permitted which does not comply with these terms.
*Correspondence: Chen Zheng, zhengc@gsau.edu.cn