- 1The Innovation Centre of Ruminant Precision Nutrition and Smart and Ecological Farming, Jilin Agricultural Science and Technology University, Jilin, China
- 2Jilin Inter-Regional Cooperation Centre for the Scientific and Technological Innovation of Ruminant Precision Nutrition and Smart and Ecological Farming, Jilin, China
- 3Grasslands Research Centre, AgResearch Limited, Palmerston North, New Zealand
- 4Faculty of Veterinary and Agricultural Sciences, The University of Melbourne, Melbourne, VIC, Australia
Carbohydrates are the major component of most ruminant feeds. The digestion of carbohydrates in the rumen provides energy to the ruminants but also contributes to enteric methane (CH4) emissions. Fresh forage is the main feed for grazing ruminants in temperate regions. Therefore, this review explored how dietary carbohydrate type and digestion affect ruminant CH4 emissions, with a focus on fresh forage grown in temperate regions. Carbohydrates include monosaccharides, disaccharides, oligosaccharides, and polysaccharides. Rhamnose is the only monosaccharide that results in low CH4 emissions. However, rhamnose is a minor component in most plants. Among polysaccharides, pectic polysaccharides lead to greater CH4 production due to the conversion of methyl groups to methanol and finally to CH4. Thus, the degree of methyl esterification of pectic polysaccharides is an important structural characteristic to better understand CH4 emissions. Apart from pectic polysaccharides, the chemical structure of other polysaccharides per se does not seem to affect CH4 formation. However, rumen physiological parameters and fermentation types resulting from digestion in the rumen of polysaccharides differing in the rate and extent of degradation do affect CH4 emissions. For example, low rumen pH resulting from the rapid degradation of readily fermentable carbohydrates decreases and inhibits the activities of methanogens and further reduces CH4 emissions. When a large quantity of starch is supplemented or the rate of starch degradation is low, some starch may escape from the rumen and the escaped starch will not yield CH4. Similar bypass from rumen digestion applies to other polysaccharides and needs to be quantified to facilitate the interpretation of animal experiments in which CH4 emissions are measured. Rumen bypass carbohydrates may occur in ruminants fed fresh forage, especially when the passage rate is high, which could be a result of high feed intake or high water intake. The type of carbohydrates affects the concentration of dissolved hydrogen, which consequently alters fermentation pathways and finally results in differences in CH4 emissions. We recommend that the degree of methyl esterification of pectic polysaccharides is needed for pectin-rich forage. The fermentation type of carbohydrates and rumen bypass carbohydrates should be determined in the assessment of mitigation potential.
Introduction
Global warming resulting from the emissions of greenhouse gases (GHG) is a worldwide issue. Greenhouse gases emitted from anthropomorphic activities, including industrial and agricultural production, are the main driving force of global warming (1). A target for net zero emissions was set to achieve a balance between emission and removal of anthropogenic GHG by 2050 (2, 3). Methane (CH4) is an important gas causing greenhouse effects on the earth, and its atmospheric warming potential is 28 times that of carbon dioxide (CO2) (4). The global annual emissions of CH4 into the atmosphere are 500–600 million tonnes, and the half-life of CH4 in the atmosphere is 8.6 years (5). In November 2021, over 110 countries pledged to reduce global CH4 emissions by at least 30% in 2030 compared with 2020 (https://www.globalmethanepledge.org/). Agriculture accounts for 62% of global CH4 emissions from human activities, of which ruminants account for 58% of agricultural emissions (6). Enteric fermentation represents about 30–32% of total anthropogenic CH4 emissions in the world (7). Thus, ruminant production industries are an important source of CH4 emissions. Methane emissions from ruminants not only aggravate the global greenhouse effect but also cause an energy loss in livestock, accounting for 3.9–10.7% of ingested metabolic energy (8).
Although ruminants can produce CH4 in the hindgut, most CH4 is formed in the rumen (9). The feed ingested by ruminants is degraded and fermented by microorganisms in the rumen to produce products such as short-chain fatty acids (SCFA), CO2 and metabolic hydrogen (H2) (10). Short-chain fatty acids are absorbed through the rumen wall to provide energy. Metabolic H2 and CO2 are used by methanogens to produce CH4. In this process, CO2 is the carbon source, and H2 is the main electron donor. Four moles of hydrogen (H2) can produce 1 mole of CH4 (11). Methanogenesis is the main biochemical pathway to remove metabolic H2 for maintaining a very low concentration of H2 in the rumen (about 1 μM dissolved H2). If the H2 concentration increases, the feed degradation rate decreases. The CH4 emissions can be reduced by inhibiting the formation of H2 from fermentation or promoting alternative pathways of H2. A few species of methanogens use alcohols as electron donors to participate in the formation of CH4, but most methanogens use H2 and formate as electron donors (11). Although there are other methanogenic microorganisms in the rumen, methanogens are absolutely dominant. Methanogens belong to Archaea (12). There are 107-109 cells of methanogenic archaea per mL of rumen fluid (13). There are three main types of Archaea in the rumen. Cluster A is the most important, mainly including Methanobrevibacters; Cluster B mainly Methanosphaera and Cluster C the remainder. Clusters A and B account for 84% of the total number of Archaea in the rumen (12). The pH range suitable for the growth of methanogens is 6.0–7.5, with the bottom limit of 5.5–6.5. Other microorganisms in the rumen, such as protozoa, have an indirect impact on CH4 emissions. Protozoa use starch, cellulose, hemicellulose, pectin and soluble sugar to produce SCFA and metabolic H2. The metabolic H2 is converted to CH4 by methanogens attached to the surface of protozoa (14).
In temperate countries with animal agriculture based on pastoral systems, pastures are dominated by perennial ryegrass, but mixtures of ryegrass with clover or herbs and grazing forage crop monocultures have become more common to improve animal performance, fill pasture feed gaps, and reduce environmental impact (15, 16). These alternative pasture species contain a wide range of non-structural carbohydrates (NFC) and generally have a greater ratio of NFC to structural carbohydrates than ryegrass pasture. We postulate that the reported differences in CH4 emissions between ryegrass and alternative forages (15, 17, 18) might be due to their difference in carbohydrate composition and rate of digestion, as it is known that carbohydrates affect CH4 emissions (19).
The hydrolysis of di-, oligo- and poly-saccharides in the rumen into monosaccharides is a complex process with a large number of enzymes involved. However, it is interesting to note that no H2 is produced, and thus, no CH4 is formed during this process (11). On the other hand, the fermentation of monosaccharides to pyruvate and then into SCFA such as acetate and butyrate results in H2 formation, which is mainly converted into CH4 by ruminal methanogens. The type of SCFA produced from the fermentation of pyruvate depends on the ruminal microbial community structure and ruminal environment (11, 20, 21), which can affect CH4 emissions (21, 22). The dissolved H2 concentration in the rumen is a key factor determining the SCFA formation pathways and end-products (20). Feeding different dietary carbohydrate fractions often results in different ruminal fermentation and passage rates (23), ruminal pH, rumen buffering capacity, and different (acetate + butyrate)/propionate ratios (21, 24). Therefore, changing forage carbohydrate composition might be an effective way to mitigate CH4 emissions. However, to the best of our knowledge, few systematic reviews are performed in this area although some reviews have more or less touched upon carbohydrates [e.g., (10, 25–28)], despite many study results being published over the last few decades. Therefore, the aim of this review was to summarise published data on carbohydrate types and their molecular structure present in ruminant feeds, with an emphasis on fresh forages and their role in enteric CH4 emissions. The literature for this review was searched using SCOPS with methane, ruminant (or rumen, methanogen) and carbohydrate (including terms in this category such as monosaccharide, disaccharide, polysaccharide, pectin, starch, fructan, etc.) and with methane and temperate forage (including the common or scientific names of temperate forages) as keywords and the date range set between 2000 and 2022.
Fresh Forage Carbohydrate Composition and CH4 Emissions From Ruminants
Improved pastures in pastoral animal production systems are generally dominated by perennial ryegrass (Lolium perenne L.) (29). Perennial ryegrass pasture composition was previously found to have only minor effects on CH4 emissions from sheep and cattle (29–31). However, mixing pastures with clover or herbs or grazing forage crop monocultures is increasing. These alternative forage species have a much wider range of carbohydrate types and compositions than perennial ryegrass. A series of trials with sheep fed fresh alternative forages vs. perennial ryegrass have been performed (15, 32), and the detailed carbohydrate composition of the forage eaten was analysed by conventional gravimetric and spectrophotometric methods (Table 1). Carbohydrate fractions analysed included water soluble carbohydrates (WSC) after hot-water extraction (consists of mono-, di-, and oligo- saccharides), pectin using meta-hydroxydiphenyl (44), and fibre residue after neutral detergent (NDF, consists of hemicellulose, cellulose and lignin) and acid detergent (ADF, consists of cellulose and lignin) extraction (45). Hemicellulose concentration was estimated as NDF – ADF, cellulose concentration as ADF – the residue left after sulphuric acid extraction of ADF, and total NFC as 1 – ash – crude protein – lipids – NDF. Starch concentration was assumed to be negligible in these fresh forages. The studies showed that only sheep fed forage brassicas had consistently lower CH4 emissions than sheep fed perennial ryegrass pasture (15, 35, 37, 46), while sheep fed forage chicory (33, 34) or white clover (17) had similar CH4 emission to sheep fed perennial ryegrass pasture (Table 1). Although there was a moderate negative relationship (R2 = 0.38) between CH4 yield [g/kg dry matter intake (DMI)] and WSC: NDF ratio (Figure 1), the forage carbohydrate composition did not fully explain differences in CH4 emissions (32, 47). Therefore, other potential mechanisms behind lower CH4 emissions in sheep fed forage brassicas were investigated, including chemical and digestive parameters like hydrogen sinks (nitrate and sulphur), plant secondary compounds (S-methyl cysteine sulfoxide and glucosinolates), rumen fermentation profiles (i.e., SCFA profile), solid and liquid rumen turnover rates, total tract digestibility and free triiodothyronine in blood (related to digesta fractional passage rate) (35, 37, 46), but none of these individual variables had a strong relationship with CH4 emissions (47). Therefore, the full mechanisms for the CH4 mitigation properties of forage brassicas remain unclear to date. Sun (48) proposed a new hypothesis based on a literature review to explain the low enteric CH4 emission observed from ruminants fed forage brassica. According to the hypothesis, glucosinolates, a secondary metabolite widely present in forage brassica or their breakdown products, can stimulate the secretion of free triiodothyronine in ruminants. This may change rumen physiology by reducing the mean retention time of digesta in the rumen, ultimately reducing enteric CH4 emissions.
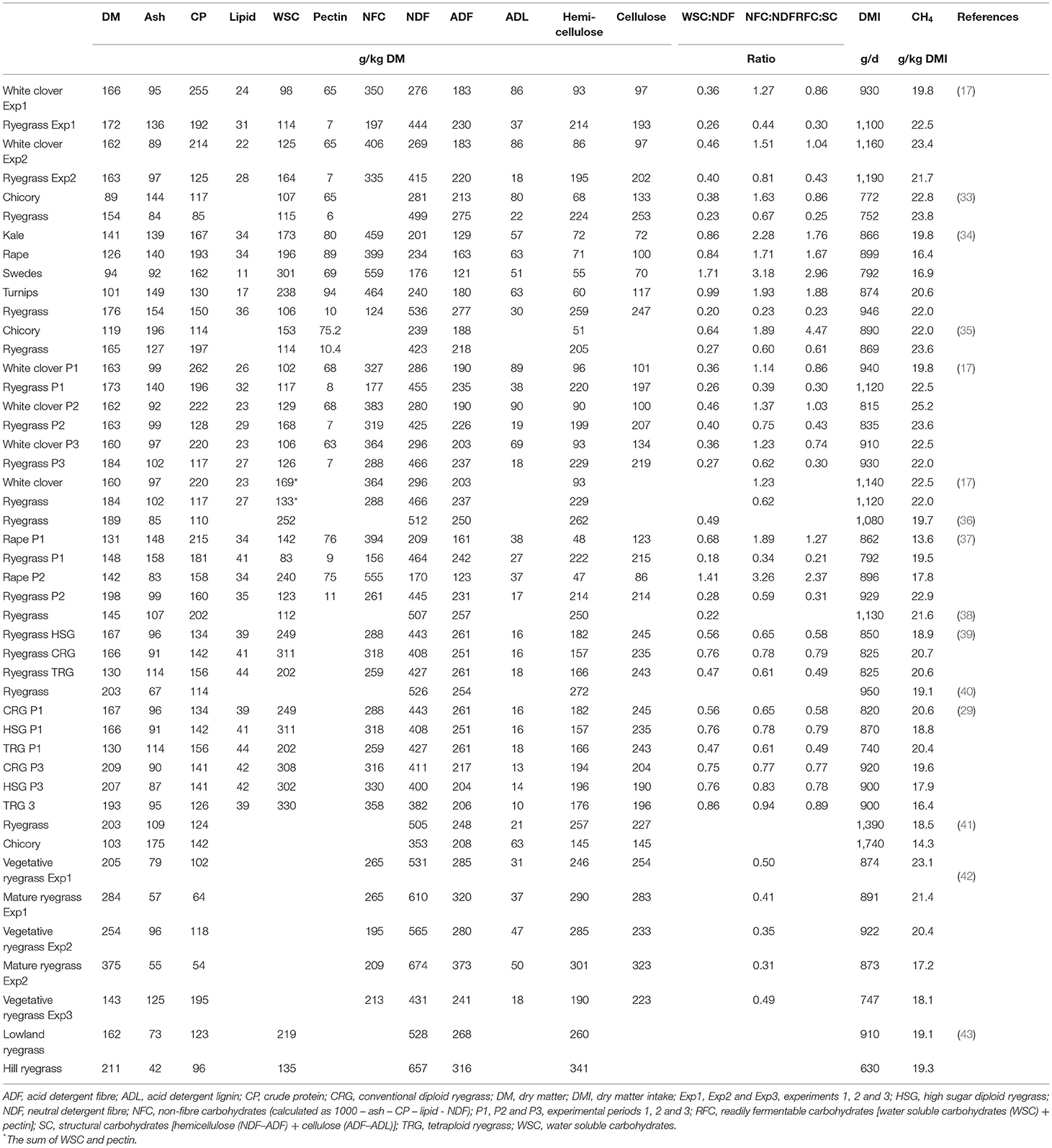
Table 1. Summary of methane measurements (measured using respiration chambers) in sheep fed different fresh temperate forages of varying chemical composition and crude carbohydrate fractions.
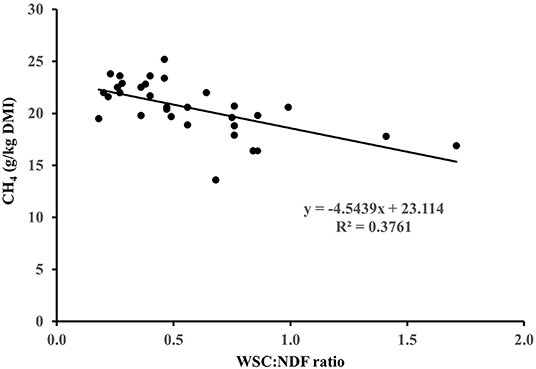
Figure 1. Relationship between CH4 yield (g/kg dry matter intake; DMI) and forage water soluble carbohydrate (WSC): neutral detergent fibre (NDF) ratio in sheep fed different fresh forages.
The forage effects of NFC on ruminant performance and CH4 emissions have been studied extensively over the last two decades. For example, high levels of NFC in the diet can be delivered by feeding high sugar grasses (HSG). In a study by Kim et al. (49), lambs fed fresh HSG emitted 17% less CH4 than lambs fed conventional perennial ryegrass (19.3 vs. 23.3 g/kg DMI) and when these ryegrasses were mixed with white clover, such CH4 emission differences remained (18.6 vs. 21.2 g/kg DMI). In New Zealand, sheep fed an HSG cultivar or a tetraploid ryegrass cultivar were found to have a lower CH4 yield than sheep fed a conventional diploid ryegrass cultivar (Table 1) (29, 39). However, Staerfl et al. (50) found similar CH4 yield (19.4 and 20.3 g CH4/kg DMI, respectively) in lactating Holstein-Friesian dairy cows fed HSG or conventional ryegrass hay [492 vs. 491 g NDF, 224 vs. 101 g NDF, 193 vs. 103 g WSC, and 129 vs. 82 g ethanol soluble carbohydrates per kg of dry matter (DM), respectively]. Therefore, the effect of increasing dietary NFC concentration in ryegrass or by feeding alternative forages, on CH4 emissions has been variable.
Carbohydrate Compositions
The chemical characterisation of carbohydrates in feeds/forages in the previous sections was performed using conventional gravimetric and spectrophotometric methods, which do not provide a detailed characterisation of the types (Figure 2) and molecular structure of plant carbohydrates. In vitro studies have provided evidence that different types of carbohydrates change CH4 emissions (52–55). Carbohydrates in plants are present as mono-, di-, oligo- and- poly-saccharides (56) and can be linked with other compounds in the plant such as protein and lignin to form the plant cell wall structures.
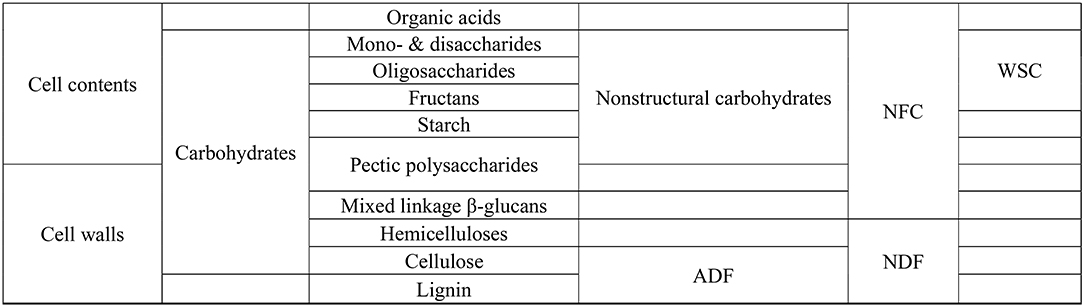
Figure 2. Plant carbohydrates. ADF, acid detergent fibre; NDF, neutral detergent fibre; NFC, non-fibre carbohydrates; WSC, water soluble carbohydrates. Adapted from Hall et al. (51).
Free Monosaccharides
Glucose and fructose are present as free monosaccharides in plants (57, 58) at concentrations generally below 10 g/kg DM, although they can be up to 61 g/kg DM in spring grass (57). Glucose can account for 20–90% of total non-structural carbohydrates in the leaves of tropical grasses (59) and 11–36 g/kg DM (60) and 10–20 g/kg DM (58) in some cool-season grasses. Fructose is not detectable or is only present in very small amounts in the leaves of tropical grasses (59) and between 8 and 22 g/kg DM (60) and 5–12 g/kg DM (58) in the leaves of cool-season grasses. Free sugars are estimated to degrade in the rumen at a rate of 40–60%/h (61).
Monosaccharides in Di-, Oligo-, and Poly-Saccharides
Monosaccharides are the basic units of disaccharides, oligosaccharides and polysaccharides, and in plants include pentoses (5-carbon sugars) such as xylose and arabinose, and hexoses such as glucose, galactose, rhamnose, mannose, fructose, glucuronic acid, and galacturonic acid. Many of these can occur in a variety of different polysaccharides. For example, in lignified secondary cell walls of dicotyledons, xylose occurs mostly in xylans, but in primary walls of dicotyledons, it occurs mostly in xyloglucans, with small amounts in pectic polysaccharides. Similarly, in primary cell walls of dicotyledons arabinose, galactose, rhamnose, and galacturonic acid occur mostly in pectic polysaccharides. These associations result in different rates and extent of digestion of monosaccharides from the polysaccharides. The ratio of xylose to arabinose has been suggested as an indicator of the potential digestion of forage cell walls (62). This would apply particularly to dicotyledonous forages, but even in grasses where heteroxylans containing arabinose occur in both primary and lignified secondary cell walls, the proportion of arabinose in these polysaccharides is greater in primary cell walls, which also contain pectic polysaccharides containing arabinose (44). Therefore, it is important to know the monosaccharide composition of carbohydrates in forages.
Di- and- oligo-saccharides, non-fibre polysaccharides and soluble fibre are part of NFC, which are rapidly degraded in the rumen (20–60%/h) and to a large extent (61) but vary in concentrations, monosaccharide composition and structure (see next section), which might affect CH4 emissions differently.
Di- and- Oligo-Saccharides
The main disaccharide present in forages and feeds is sucrose, and the main oligosaccharides are raffinose, stachyose and verbascose, but the contents of sucrose (<40 g/kg DM) and oligosaccharides (<40 g/kg DM) are generally low across a range of feeds including whole plant cereals (57). However, the concentration of sucrose in grasses reached up to 62 g/kg DM in the leaves of Festuca arundinacea, between 24 and 42 g/kg DM in Agropyron (red), L. perenne, Dactvlis glomerata, Phleum pratense, and Bromus inermis, but was <9 g/kg DM in Bromus tectorum (60), 8–18 g/kg DM in F. arundinacea, Lolium multiflorum × F. arundinaceae, Thinopyrum ponticum, Thinopyrum intermedium, and B. inermis (58) and 24–42 g/kg DM in P. pratense (63). The simple sugars in fresh fodder beet underground storage organs and leaves consist of 15–20% sucrose (64).
Fructans
Fructans are present in the vegetative parts of temperate grasses ranging from 19 to 383 g/kg DM, typically 25–50 g/kg DM (58). Fructans have a linear chain of β-2,6-fructosyl-fructose residues and can have a branched structure by both β2-1 and β2-6 linkages in some plant species. The side and branch chains of fructans have a α-1,2-linked residue as a terminal unit in most cases, which is a non-reducing end (65, 66). Fructans with a low degree of polymerisation can be extracted with boiling 80% ethanol, and fructans with a high degree of polymerisation can be extracted with boiling water (67). Fructans are rapidly and completely fermented in the rumen (68). In the 1970s, a high fructan content was found in perennial ryegrass plants of specific populations, leading to the breeding of cultivars called generically “high sugar grass” (69), which have received considerable attention for their putative benefits to animal production (29, 69, 70). Inulin is also a fructan, sometimes called inulin-type fructan, but it is a linear β 2-1 fructan with a basic unit of α-D-glucose linked to 1-2 β-D-fructose β (1, 5) bond (66). Inulin is widely present in other forage crops like the tubers of Jerusalem artichoke (Helianthus tuberosus) (71) and the roots of chicory (66).
Starch
Starch is a carbohydrate composed of glycosidically linked glucose units and composed of two types of polysaccharides: linear amylose and branched amylopectin. Amylose has a linear chain only, and the length of the chain is several hundred glucose residues (72). Amylose occasionally contains α-1,6 linked glucose. Amylopectin has a chain of α1,4-linked glucose residues with side chains linked by α1,4,6-linked glucose residues (72). The side chain sometimes has terminal glucose which is a reducing sugar (72, 73). A large number of the macromolecular chains in starch granules are organised in crystalline structures, with three patterns of crystalline structures occurring: A-type pattern common in cereal starches, B-type pattern present in some tuber starches and cereal starches, and C-type pattern is common in legume starches. B-type crystalline starches with larger granules are more resistant to enzymatic breakdown than A-type crystalline starch with small granules (74). Starch is rapidly degraded in the rumen (20–40%/h; 51), with the physical structure surrounding starch granules being the main barrier to degradation (73). Starch concentration in vegetative parts of forages is very low, typically ranging from 10 to 33 g/kg DM (58), with 40 g/kg DM in the leaves of Lolium temulentum (75), 34 g/kg DM in lucerne leaves, 3 g/kg DM in lucerne stems, 4 g/kg DM in timothy hay (76), 2–3 g/kg DM in timothy leaves (63) and 5–17 g/kg DM in forage brassicas (Sun et al., unpublished data). Starch is the main storage polysaccharide in grains and some tubers such as potatoes and sweet potatoes, but not in the underground storage organs of swedes, turnips, and fodder beet.
Pectic Polysaccharides
Pectic polysaccharides are present in the primary cell walls of all forage plants and are located particularly in the middle lamella (77). The pectic polysaccharides in the primary walls of grasses (family Poaceae) have a similar structure to those in dicotyledonous primary walls (78), but they occur in much lower proportions in Poaceae (Table 1). The principal monosaccharide components of pectins are galacturonic acid, rhamnose, arabinose and galactose (79). There are three main types of pectic polysaccharide domains: homogalacturonan (HG), rhamnogalacturonan I (RG I), and rhamnogalacturonan II (RG II) (80) and a small amount of a fourth domain, xylogalacturonan (XGA), occurs in some walls (44, 78). The XGA is composed of a backbone of 4-linked α-D-galactosyluronic acid residues to which xylose residues are attached at the C(O)3 position of about half of the galacturonic acid residues (78).
The HG is a linear homopolymer of 4-linked α-D-galactosyluronic acid residues. Methyl-esterification of galactosyluronic acid residues often occurs at the C-6 carboxyl group; acetyl groups are also present at C-2 and/or C-3 in some plants (79). Two HG chains with blocks of more than 10 unesterified galacturonic acid (GalA) residues can form a dimer via Ca2+-cross-linking (80). RG I consists of a backbone of alternating (1, 7)-linked α-D-galacturonic acid and (1, 5)-linked α-L-rhamnose residues and has several different side-chains attached to the C4 of rhamnose. These side-chains are mainly composed of arabinose and galactose chains (arabinans and galactans, respectively) linked by (1, 8)-linkages and by (1, 7)-linkages, respectively (81). Type I arabinogalactan side-chains have (1, 7)-β-galactan chains with limited substitution by short arabinose-containing oligomers (82). RG II is quantitatively a minor constituent of primary cell walls in dicotyledons (83), and it has been isolated from the primary cell walls of the Poaceae. It is released as low-molecular-weight polysaccharides, containing about 30 different glycosidic linkages with at least 12 different glycosyl residues, after treating with endo-polygalacturonase (78).
Cellulose
Cellulose, a simple linear polymer of (1, 7)-linked β-D-glucosyl residues, is the most abundant plant polysaccharide and forms the basic structure of all plant cell walls (84). Cellulose occurs as crystalline microfibrils about 3 nm in diameter containing 18 cellulose molecules aligned side to side (85, 86). In secondary lignified cell walls, some of the cellulose microfibrils may aggregate into structures known as macrofibrils (87). Primary cell walls contain ~20–30% cellulose, and lignified secondary walls contain ~40–60% cellulose (88). Cellulose typically accounts for about 35~50% of plant dry matter (89).
Other Non-Cellulosic Polysaccharides (“Hemicelluloses”)
Other non-cellulosic polysaccharides have traditionally been called hemicelluloses and comprise a group of polysaccharides that are extracted from cell walls with alkaline solution after initial treatments with water and chelating reagents (90). They include xyloglucans, heteroxylans, heteromannans, and β-glucans and are bound to cellulose by hydrogen bonds. Their structures vary greatly in the walls of different cell types and different plant species. Xyloglucans (XGs) are the principal other non-cellulosic polysaccharides of dicotyledonous primary cell walls (91), accounting for up to 20–25% of the primary cell walls (92). The main backbone is composed of 4-linked β-D-glucosyl residues, most of which are linked to α-D-xylosyl residues of side chains with α-(1, 9)-glycosidic linkage and these xylosyl residues can be linked to fucose, galactose, and less commonly arabinose (92).
Fucogalactoxyloglucans have been found in the primary walls of most dicotyledonous plants except for those of solanaceous plants (e.g., potato), which contain arabinoxyloglucans (93). In the walls of the monocotyledon family Poaceae (grasses), the xyloglucans do not contain arabinose and fucose (94). The content of xyloglucans in members of the Poaceae family is small, accounting for only 2–5% of the walls (95).
Heteroxylans include arabinoxylans, glucuronoxylans, and glucurono-arabinoxylans. They have a backbone of 4-linked xylose residues with short side chains of arabinose, glucuronic acid and 4-O-methyl-glucuronic acid residues (96). The xylose residues in the backbone may be O-acetylated, and in the Poaceae the arabinose residues may be esterified with ferulic or p-coumaric acid (96, 97). Heteroxylans are present in small proportions in the primary walls of dicotyledons, but they are abundant in the Poaceae. Cellulose microfibrils in the walls of Poaceae may be interlocked mainly by glucuronoarabinoxylans (GAXs). Unbranched GAXs are cross-linked with each other or to cellulose via hydrogen bonds. Branched GAXs are unable to form crosslinking between two GAXs or GAX to cellulose since arabinose and glucuronic acid side groups prevent the formation of hydrogen bonds (95). Galactoarabinoxylans have 4-linked xylan backbone with α-L-arabinofuranose residues and/or short chains containing arabinose and galactose attached at C3- or C2- positions. They are found in perennial ryegrass (98, 99) and cocksfoot grass (Dactylis glomerata) leaves (98).
Heteromannans galactoglucomannans (GGM) are found widely in a small amount, and they have a backbone of (1, 7)-linked β-D-mannose and β-D-glucose residues.
(1,3:1,4)-β-Glucans or written as(1 → 3), (1 → 4)-β-glucans are also known as mixed-linked glucans or β-glucans and consist of unbranched and unsubstituted chains of (1,3)- and (1,4)-β-glucosyl residues with varying ratio of (1,4)-β-D-glucosyl residues to 1,3)-β-D-glucosyl residues (100). They have been found in the primary cell walls of Poaceae, and contain about 30% 3-linked residues and 70% 4-linked residues (101). In cereal grains, they occur particularly in the walls of the aleurone, and starchy endosperm and their contents vary markedly, largely depending on species (102), and these polysaccharides are particularly abundant in barley (103). (1,3:1,4)-β-Glucans are thought to form a gel-like matrix in cell walls between the reinforcing cellulose microfibrils (102).
Effects of Carbohydrate Types on Methane Emissions From Ruminants
Monosaccharides
Czerkawski and Breckenridge (52) conducted in vitro rumen simulation technique (RUSITEC) incubations with 26 carbohydrates. These carbohydrates resulted in similar CH4 production per unit of carbohydrate fermented, except for rhamnose. Rhamnose resulted in distinguishable CH4 production, but this monosaccharide is only a minor component in plants (44). However, carbohydrates differed in their extent and rate of fermentation: glucose, fructose and sucrose were fermented rapidly; L-arabinose, xylose galactose, and mannose were fermented at an intermediate rate; and glucuronic acid, galacturonic acid, and fucose fermented at a slow rate (52). Carbohydrates with a slow fermentation rate have a higher probability of escaping the rumen without being fermented (104), which could result in lower CH4 emissions. Pacheco et al. (105) speculated that the escape of soluble cell contents could explain why CH4 produced per unit of digestible organic matter was less in forages with a greater content of water.
Disaccharides
Sucrose concentration is generally low in forages, but it can be high in some feeds, for example, as the main storage carbohydrate in some roots like those of fodder beet. Its fractional fermentation rate in the rumen was about 1,200–1,404%/h (106), which is much higher than for starch [e.g., 30%/h for barley starch (107)]. In a sheep trial conducted by Huhtanen and Robertson (108), with 400 g/kg DM of sugar-beet pulp replaced by sucrose, maize starch or xylose, CH4 emissions were similar for sheep supplemented with sucrose, maize starch or xylose [6.6% of gross energy (GE)], but lower than those fed sugar-beet pulp (7.1% of GE intake). Sucrose was more rapidly fermented than steam-flaked maize starch in vitro at two pre-determined pH levels (pH 6 and 7) (109). Methane emissions per unit of degraded organic matter (OM) were similar for sucrose and starch at pH 6, but more CH4 was produced from sucrose than from starch at a pH of 7. At pH 6, sucrose led to a higher molar proportion of propionate and less butyrate than starch, while at pH 7, the fermentation profile was similar for both sucrose and starch. At both levels of pH, sucrose produced more CH4 and more SCFA (109). These findings suggest that pH in the rumen will interact with carbohydrate type leading to varying levels of CH4 production in response to carbohydrate supplementation.
Golder et al. (110) found that supplementation of the diet with crushed triticale grain decreased rumen pH and increased total SCFA, acetate, butyrate and propionate concentrations compared with the control and these changes were even more extreme when triticale grain plus fructose were supplemented. Although CH4 emissions were not measured, the large drop in rumen pH suggests a reduction in CH4 emissions could be expected.
Pectic Polysaccharides
Pectic polysaccharides present in forage are methyl esterified to varying degrees. The hydrolysis of methyl esters from pectic polysaccharides produces methanol (CH3OH), which is stoichiometrically converted to CH4 as 4CH3OH → 3CH4 + CO2 + 2H2O. The theoretical molar ratio of CH4 produced from methanol (CH4/CH3OH) is 0.75. When pectin or methanol was infused into the rumen of sheep, the measured conversion ratio was 0.77, suggesting all methanol infused was converted to CH4 (111). Thus, forages containing large amounts of highly methyl-esterified pectic polysaccharides might result in higher CH4 emissions.
Poulsen et al. (54) reported no differences in CH4 production among sugar beet pectin, maize starch, wheat starch and chicory inulin incubated in vitro (batch culture), but the extent of fermentation may change the final CH4 production. Nevertheless, pectin resulted in a higher molar proportion of acetate, and a lower proportion of butyrate and inulin lowered acetate and increased butyrate, compared with starch from either maize or wheat. These differences in SCFA suggested that different amounts of hydrogen were produced and consequently would have led to different amounts of CH4 released per unit of fermented substrates. In the RUSITEC-based study by Zhao et al. (112), CH4 emissions were lower with inulin than with starch, and inulin resulted in a reduced molar proportion of acetate and acetate: propionate ratio and increased butyrate proportion, which suggests a potential for reduced CH4 emissions by feeding inulin. Consisted with this result, rumen fermentation of fructose, which is a product of inulin degradation in the rumen, resulted in less acetate and more butyrate compared with starch in steers (113).
In pectic polysaccharides, HG is a major component and has a backbone of galacturonic acid residues esterified with methyl groups to different degrees. The cleavage of methyl groups from HG may release methanol which is used for CH4 formation by some microbes (111). Geerkens et al. (114) compared mango peels rich in pectin, de-pectinised mango peels, apple pectin and citrus pectin in vitro (batch culture) mixed with hay (Table 2). Mango peels produced more CH4 than hay when expressed as either CH4/gas production (GP) or CH4/SCFA, while de-pectinisation of mango peels resulted in reduced CH4, suggesting that pectin may cause an increase in CH4 production. However, CH4/GP dropped, and CH4/SCFA was similar or dropped when citrus or apple pectin was incubated compared with hay. This suggests that the structure of pectic polysaccharides affects CH4 formation. This result is different from the finding of Czerkawski and Breckenridge (52) in which the degree of methyl esterification was not considered. When high and low degrees of methyl esterification were compared, low esterified pectin produced less CH4 than high esterified pectin for both apple and citrus pectins (114). This supports the hypothesis that methyl group cleavage from pectin leads to increased CH4 formation, with methanol as an intermediary.
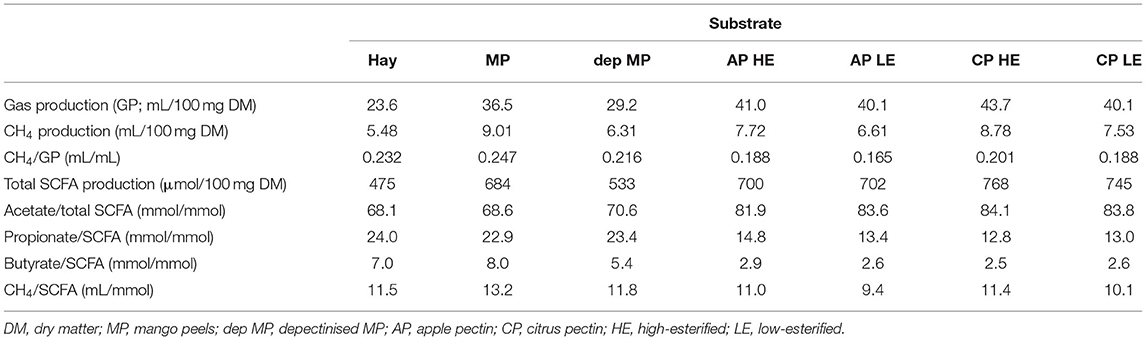
Table 2. Total gas, methane (CH4) and short-chain fatty acid (SCFA) productions of pectic substrates after 24 h in vitro incubation [adapted from Geerkens et al. (114)].
Starch
Between 22 and 94% of dietary starch eaten is digested in the rumen depending on total starch intake and starch source (115). The size and structure of starch granules and the pattern of enzyme-resistant crystalline starches may affect their degradation rate in the rumen (73). For example, starch from potatoes is degraded faster than that from barley and oats (115).
Dairy cows fed diets containing lucerne hay and concentrate (45:55 w/w) based on wheat grain had lower CH4 yield (11.1 g CH4/kg DMI) compared with cows fed concentrates based on maize grain (19.5 g/kg DMI) (116). Average daily ruminal pH was similar between cows fed either type of grain, but the duration when ruminal pH was below 6 was twice as long for wheat grain supplemented cows compared with maize grain supplemented cows. Wheat and maize have different starch structures, leading to faster degradation for wheat starch than for maize starch with values of 103.8%/h for wheat grain and 8.1%/h for maize grain reported by Moharrery et al. (115). The faster degradation rate of wheat starch might result in a longer duration of pH below 6, which in itself may inhibit methanogen activity and therefore contribute to lower CH4 emissions in cows fed wheat grain as found by Moate et al. (116). Lactating dairy cows fed diets based on grass-clover/maize silage (60% diet DM) and either maize cob silage, highly rumen digestible rolled barley grain or sodium hydroxide wheat grain, with a high rumen escape starch (at 25% of diet DM), had similar CH4 yield and total tract starch digestibility (117).
Besides starch type, grain processing also affects the degradation rate of starch in the rumen (73), which might alter the rumen fermentation profile and rumen pH and could therefore change CH4 emissions. Lactating dairy cows fed diets with 60% grass silage and 40% concentrate containing either slowly degradable native maize grain (5%/h) or rapidly degradable gelatinised maize grain (16%/h) at either 270 or 530 g/kg concentrate had similar CH4 yield and rumen pH but an increased rate of starch fermentation and increased level of starch reduced CH4 produced per unit of estimated rumen-fermentable organic matter (118).
Thus, the amount of starch digested in the rumen and the rate of digestion will affect the rumen fermentation pattern and rumen pH, whilst starch escaped from the rumen has little chance to be converted to CH4 (Figure 3). The proportion of starch degraded in the rumen, the rate of degradation in the rumen, and rumen escaped starch need to be considered when determining the CH4 mitigation potential. The complex interactions between the rate of rumen fermentation, pH and the passage rate of starch from the rumen could have resulted in inconsistent results reported in the literature attempting to link the supplementation level of concentrates with the amount of CH4 emissions. Rumen passage rate is affected by many factors including supplement concentrates in the diet, whereas it could result in a shift of dissolved hydrogen and consequently rumen fermentation pattern and CH4 emissions (20). Another aspect to consider is the effect of feeding concentrates on fibre digestion. Supplementation of large amounts of grain suppresses the degradation of fibre, which will result in less hydrogen produced and, therefore, less CH4. For instance, a recent study conducted by Bougouin et al. (119) showed that cows fed with starch-rich diets based on grass silage has comparatively lower enteric CH4 emission than fibre-rich diets based on grass silage. The main reasons for reduced methanogenesis may be a reduction of the rumen protozoa population (by 36%) and a shift in rumen fermentation toward propionate at the expense of butyrate. However, increasing the level of starch should be carefully applied as a high amount of starch feeding can lead to ruminal acidosis. According to Nozière et al. (120), the chemical treatment of grains can be used to prevent rumen acidosis by reducing the digestibility of the starch inside the rumen. Furthermore, introducing buffers (e.g., sodium bicarbonate or plant buffering capacity) to starch-based diets reduces the prevalence of rumen acidosis by stabilising rumen pH and improving feed digestion (121, 122).
Supplementing Feeds Differing in Main Carbohydrates
Hindrichsen et al. (53) compared feeds with different major carbohydrates represented by different substrates including oat hulls (lignified fibre), soybean hulls (non-lignified fibre), apple pulp (pectin), sugar beet pulp (hemicelluloses and pectin), guar gum (galactomannan), Jerusalem artichoke tubers (fructan), molasses (sucrose) and wheat (starch). They were incubated in RUSITEC fermenters mixed with forage (a mixture of maize silage, grass silage and hay) at a ratio of 1:1, on a DM basis, and CH4 emissions (mmol CH4/g degraded OM) were lower for oat hulls, guar gum and wheat than for molasses, with other supplements intermediate (Table 3). Multiple-regression analysis of CH4 emission data with chemical composition revealed a negative correlation with lignin and a positive correlation with total sugars (53). The same diets, except sugar beet pulp and guar gum, were fed to 12 Brown Swiss dairy cows with forage: concentrate ratios of 1: 1 (123). Methane emissions per unit of DMI were only lower for cows fed oat hulls (Table 3), which might have resulted from a lower digestibility due to the high degree of lignification of fibre in oaten hulls. Additionally, CH4 emissions per unit of DM were numerically 10% lower for cows fed the Jerusalem artichoke tubers than cows fed wheat. When emissions were expressed as per unit of digested OM, the difference among diets disappeared (123).
Methane emissions were 16% higher for sheep fed 1.0 kg molassed sugar-beet pulp (containing 166 g sugars and 166 g cellulose) than for sheep fed 1.0 kg chopped hay (125 g sugars and 302 g cellulose) (124). Methane emissions were 8% higher for lactating dairy cows fed grass-clover silage based diet supplemented with molasses (g/kg diet DM, 250 g starch, 32 g sugar, 300 g NDF) vs. wheat grain (g/kg diet DM, 5 g starch, 240 g sugar, 278 g NDF) (125). Giger-Reverdin and Sauvant (126) analysed a CH4 emission dataset from male sheep fed 22 different feed classes, which resulted in the separation of feeds into four CH4 yield groups being: high—peas and faba beans; medium—grains, sugar beet pulp and soybean meal; low—silages, hays and straw; very low—distillers grains. However, there was substantial variation in CH4 yield within feed class, and the proportion of the test feed in the diet and type of basal forage diet were not mentioned.
A recent study conducted by Jonker et al. (127) found that dry cows grazing fodder beet (Beta vulgaris) supplemented with perennial ryegrass based pasture silage (6 kg DM/cow/day) produced 28% lower CH4 yield (g/kg DM intake) than cows that grazed forage kale (Brassica oleracea) supplemented with barley straw (Hordeum vulgare) (3 kg DM/cow/day). Fodder beet has a very high concentration of readily fermentable carbohydrates, which may affect rumen fermentation and thereby reduce the CH4 emission. Børsting et al. (122) found that feeding sugar beet molasses instead of wheat increased enteric CH4 production and yield. This might have been because a high sugar concentration in sugar beet molasses increased the proportion of butyrate in rumen liquid leading to greater H2 formation, which will increase the enteric CH4 production (20) when the rumen pH is maintained at the normal level (109).
Conclusions and Recommendations
The different carbohydrates present in different plants may impact the quantity of CH4 emissions from ruminants consuming these plants. The detailed compositions and structures of carbohydrates in plants fed to ruminants have not been extensively studied. So far, most carbohydrate information on forage and feeds is based on analysis using the detergent system, which only provides a crude classification of structural and non-structural carbohydrates. Forage brassicas, chicory, and white clover have more readily fermentable carbohydrates (all low starch) and less structural carbohydrates than ryegrass, but the type of carbohydrates in these forages are largely unknown. It is recommended that a comprehensive carbohydrate study is carried out on these forages as these data may help explain variation in CH4 emissions observed in animals fed different forages.
Based on evidence from the in vitro studies, all carbohydrates, except rhamnose and pectins, may have the same efficiency of being converted to CH4. However, rhamnose is generally a minor component in plants. Methyl groups in pectic polysaccharides can be converted to methanol and further converted to CH4 in the rumen. Although some in vitro studies have suggested that CH4 emissions may be lower for some types of carbohydrates, in vivo studies have not clearly supported that conclusion. The inconsistent in vivo results with soluble sugars make it difficult to speculate if the soluble sugars in forage brassicas contribute to low CH4 emissions from sheep fed these forages. Nevertheless, brassicas, chicory, and white clover all contain a large amount of pectic polysaccharides. Compared to ryegrass pastures, brassicas have reduced CH4 emissions, while chicory and white clover do not. Since methyl groups in pectic polysaccharides increase CH4 emissions, the degree of methyl esterification might make the difference and needs to be elucidated for these forages. How high pectin containing diets and the concentration of methyl group in the diets affect CH4 emissions should be studied in the future.
Supplementation of concentrate is considered to reduce CH4 emissions due to the associated shift of rumen fermentation. However, this is mainly observed for diets with a high proportion of concentrates (>80%). There are several possible mechanisms for carbohydrates to affect CH4 emissions. The fermentation of starch in the rumen produces short-chain fatty acids, which may reduce rumen pH if the rate of absorption and escapement from the rumen is less than the rate of production and subsequently alter the structure of rumen microbial communities. The degradation of starch and structural carbohydrates in the rumen results in different ratios of acetate, butyrate,and propionate. In general, starch promotes the formation of propionate, although sometimes butyrate, which is associated with less CH4 production. Structural carbohydrates promote the formation of acetate, which provides hydrogen for CH4 production. Diets containing high amounts of fermentable carbohydrates fed at a high feeding level can increase rumen passage rate, which may increase the escape of substrates for microbes to produce hydrogen and lead to lower CH4 emissions. The degradation rate of starch and the level of starch intake affect the amount of starch escaping from the rumen. Similar bypass from rumen digestion applies to other polysaccharides and rumen bypass nutrients may occur in ruminants fed fresh forage, especially when the passage rate is high, which could be a result of high levels of feed or water intake. It is recommended that studies are conducted on the sites of digestion of carbohydrates in forages such as brassicas to determine if changes in the sites of digestion are responsible for the mitigation effects measured from this group of forage crops.
Author Contributions
XS and DP: conceptualisation. XS, AJ, and SM: writing—original draft preparation. XS, LC, AJ, and DP: writing—review and editing. All the authors approved the final version of the manuscript.
Funding
This work was financially supported by the Pastoral Greenhouse Gas Research Consortium (PGgRc), Wellington, New Zealand, under the Low GHG Feed programme, and by the Department of Science and Technology of Jilin Province, Changchun, China, with a grant (No. 20200602016ZP).
Conflict of Interest
The authors declare that the research was conducted in the absence of any commercial or financial relationships that could be construed as a potential conflict of interest.
Publisher's Note
All claims expressed in this article are solely those of the authors and do not necessarily represent those of their affiliated organizations, or those of the publisher, the editors and the reviewers. Any product that may be evaluated in this article, or claim that may be made by its manufacturer, is not guaranteed or endorsed by the publisher.
Acknowledgments
The authors thank emeritus professor Philip Harris at the University of Auckland for valuable comments and suggestions.
References
1. Arora NK, Fatima T, Mishra I, Verma M, Mishra J, Mishra V. Environmental sustainability: challenges and viable solutions. Environ Sustain. (2018) 1:309–40. doi: 10.1007/s42398-018-00038-w
2. IPCC. Summary for policymakers. In: Climate Change 2021: The Physical Science Basis. In: Masson-Delmotte V, Zhai P, Pirani A, Connors SL, Péan C, Berger S, . editors. Contribution of Working Group I to the Sixth Assessment Report of the Intergovernmental Panel on Climate Change. Cambridge: Cambridge University Press (2021). p. 1–31.
3. Net Zero Climate. Net Zero Climate (2021). Available online at: https://netzeroclimate.org/what-is-net zero/Nguyen QV, M.
4. IPCC. Climate Change 2014: Synthesis Report. Contribution of Working Groups I, II, III to the Fifth Assessment Report of the Intergovernmental Panel on Climate Change, Paachauri RK, Meyer LA, editors. IPCC: Geneva, Switzerland (2014).
5. Muller RA, Muller EA. Fugitive methane and the role of atmospheric half-life. Geoinfor Geostat. (2017) 5:3. doi: 10.4172/2327-4581.1000162
6. Kirschke S, Bousquet P, Ciais P, Saunois M, Canadell JG, Dlugokencky EJ, et al. Three decades of global methane sources and sinks. Nat Geosci. (2013) 6:813–23. doi: 10.1038/ngeo1955
7. Gerber PJ, Steinfeld H, Henderson B, Mottet A, Opio C, Dijkman J, et al. Tackling Climate Change Through Livestock: A Global Assessment of Emissions and Mitigation Opportunities. Rome: Food and Agriculture Organization of the United Nations (FAO) (2013).
8. Appuhamy JA, France J, Kebreab E. Models for predicting enteric methane emissions from dairy cows in North America, Europe, and Australia and New Zealand. Glob Change Biol. (2016) 22:3039–56. doi: 10.1111/gcb.13339
9. Immig I. The rumen and hindgut as source of ruminant methanogenesis. Environ Monit Assess. (1996) 42:57–72. doi: 10.1007/BF00394042
10. Vasta V, Daghio M, Cappucci A, Buccioni A, Serra A, Viti C, et al. Invited review: Plant polyphenols and rumen microbiota responsible for fatty acid biohydrogenation, fiber digestion, and methane emission: experimental evidence and methodological approaches. J Dairy Sci. (2019) 102:3781–804. doi: 10.3168/jds.2018-14985
11. Pereira AM, de Lurdes Nunes Enes Dapkevicius M, Borba AES. Alternative pathways for hydrogen sink originated from the ruminal fermentation of carbohydrates: Which microorganisms are involved in lowering methane emission? Anim Microbiome. (2022) 4:5. doi: 10.1186/s42523-021-00153-w
12. Janssen PH, Kirs M. Structure of the archaeal community of the rumen. Appl Environ Microbiol. (2008) 74:3619–25. doi: 10.1128/AEM.02812-07
14. Tapio I, Snelling TJ, Strozzi F, Wallace RJ. The ruminal microbiome associated with methane emissions from ruminant livestock. J Anim Sci Biotechnol. (2017) 8:7. doi: 10.1186/s40104-017-0141-0
15. Sun XZ, Pacheco D, Luo DW. Forage brassica: a feed to mitigate enteric methane emissions? Anim Prod Sci. (2016) 56:451–6. doi: 10.1071/AN15516
16. Cheng L, Judson HG, Bryant RH, Mowat H, Guinot L, Hague H, et al. The effects of feeding cut plantain and perennial ryegrass-white clover pasture on dairy heifer feed and water intake, apparent nutrient digestibility and nitrogen excretion in urine. Anim Feed Sci Technol. (2017) 229:43–6. doi: 10.1016/j.anifeedsci.2017.04.023
17. Hammond KJ, Burke JL, Koolaard JP, Muetzel S, Pinares-Patiño CS, Waghorn GC. Effects of feed intake on enteric methane emissions from sheep fed fresh white clover (Trifolium repens) and perennial ryegrass (Lolium perenne) forages. Anim Feed Sci Technol. (2013) 179:121–32. doi: 10.1016/j.anifeedsci.2012.11.004
18. Pacheco D, Muetzel S, Lewis S, Dalley D, Bryant M, Waghorn GC. Rumen digesta and products of fermentation in cows fed varying proportions of fodder beet (Beta vulgaris) with fresh pasture or silage or straw. Anim Prod Sci. (2020) 60:524–34. doi: 10.1071/AN18002
19. Moe PW, Tyrrell HF. Methane production in dairy cows. J Dairy Sci. (1979) 62:1583–6. doi: 10.3168/jds.S0022-0302(79)83465-7
20. Janssen PH. Influence of hydrogen on rumen methane formation and fermentation balances through microbial growth kinetics and fermentation thermodynamics. Anim Feed Sci Technol. (2010) 160:1–22. doi: 10.1016/j.anifeedsci.2010.07.002
21. Ungerfeld EM. Metabolic hydrogen flows in rumen fermentation: principles and possibilities of interventions. Front Microbiol. (2020) 11:589. doi: 10.3389/fmicb.2020.00589
22. Kittelmann S, Pinares-Patiño CS, Seedorf H, Kirk MR, Ganesh S, McEwan JC, et al. Two different bacterial community types are linked with the low-methane emission trait in sheep. PLoS ONE. (2014) 9:e103171. doi: 10.1371/journal.pone.0103171
23. Van Amburgh ME, Russomanno KL, Higgs RA, Chase LE. Invited review: modifications to the cornell net carbohydrate and protein system related to environmental issues—Capability to evaluate nitrogen and phosphorus excretion and enteric carbon dioxide and methane emissions at the animal level. Appl Anim Sci. (2019) 35:101–13. doi: 10.15232/aas.2018-01783
24. Bannink A, Kogut J, Dijkstra J, France J, Kebreab E, Van Vuuren AM, et al. Estimation of the stoichiometry of volatile fatty acid production in the rumen of lactating cows. J Theor Biol. (2006) 238:36–51. doi: 10.1016/j.jtbi.2005.05.026
25. Beauchemin KA, Kreuzer M, O'Mara F, McAllister TA. Nutritional management for enteric methane abatement: A review. Aust J Exp Agric. (2008) 48:21–7. doi: 10.1071/EA07199
26. Knapp JR, Laur GL, Vadas PA, Weiss WP, Tricarico JM. Invited review: Enteric methane in dairy cattle production: quantifying the opportunities and impact of reducing emissions. J Dairy Sci. (2014) 97:3231–61. doi: 10.3168/jds.2013-7234
27. Beauchemin KA, Ungerfeld EM, Eckard RJ, Wang M. Review: Fifty years of research on rumen methanogenesis: lessons learned and future challenges for mitigation. Animal. (2020) 14:s2–16. doi: 10.1017/S1751731119003100
28. Ku-Vera JC, Jiménez-Ocampo R, Valencia-Salazar SS, Montoya-Flores MD, Molina-Botero IC, Arango J, et al. Role of secondary plant metabolites on enteric methane mitigation in ruminants. Front Vet Sci. (2020) 7:584. doi: 10.3389/fvets.2020.00584
29. Jonker A, Molano G, Sandoval E, Taylor PS, Antwi C, Olinga S, et al. Methane emissions differ between sheep offered a conventional diploid, a high-sugar diploid or a tetraploid perennial ryegrass cultivar at two allowances at three times of the year. Anim Prod Sci. (2018) 58:1043–8. doi: 10.1071/AN15597
30. Hammond KJ, Muetzel S, Waghorn GC, Pinares-Patiño CS, Burke JL, Hoskin SO. The variation in methane emissions from sheep and cattle is not explained by the chemical composition of ryegrass. Proc N Z Soc Anim Prod. (2009) 69:174–8. Available online at: https://www.nzsap.org/system/files/proceedings/2009/ab09043.pdf
31. Muetzel S, Clark H. Methane emissions from sheep fed fresh pasture. N Z J Agric Res. (2015) 58:472–89. doi: 10.1080/00288233.2015.1090460
32. van Lingen HJ, Jonker A, Kebreab E, Pacheco D. Quantitative joint evaluation of sheep enteric methane emissions and faecal dry matter and nitrogen excretion. Agric Ecosyst Environ. (2021) 305:107116. doi: 10.1016/j.agee.2020.107116
33. Sun XZ, Hoskin SO, Muetzel S, Molano G, Clark H. Effects of forage chicory (Cichorium intybus) and perennial ryegrass (Lolium perenne) on methane emissions in vitro and from sheep. Anim Feed Sci Technol. (2011) 166–167, 391–397. doi: 10.1016/j.anifeedsci.2011.04.027
34. Sun XZ, Hoskin SO, Zhang GG, Molano G, Muetzel S, Pinares-Patiño CS, et al. Sheep fed forage chicory (Cichorium intybus) or perennial ryegrass (Lolium perenne) have similar methane emissions. Anim Feed Sci Technol. (2012) 172:217–25. doi: 10.1016/j.anifeedsci.2011.11.007
35. Sun XZ, Waghorn GC, Hoskin SO, Harrison SJ, Muetzel S, Pacheco D. Methane emissions from sheep fed fresh brassicas (Brassica) compared to perennial ryegrass (Lolium perenne). Anim Feed Sci Technol. (2012) 176:107–16. doi: 10.1016/j.anifeedsci.2012.07.013
36. Moorby JM, Fleming HR, Theobald VJ, Fraser MD. Can live weight be used as a proxy for enteric methane emissions from pasture-fed sheep? Sci Rep. (2015) 5:17915. doi: 10.1038/srep17915
37. Sun X, Henderson G, Cox F, Molano G, Harrison SJ, Luo D, et al. Lambs fed fresh winter forage rape (Brassica napus L.) emit less methane than those fed perennial ryegrass (Lolium perenne L.), and possible mechanisms behind the difference. PLoS ONE. (2015) 10:e0119697. doi: 10.1371/journal.pone.0119697
38. Zhao YG, Aubry A, O'Connell NE, Annett R, Yan T. Effects of breed, sex, and concentrate supplementation on digestibility, enteric methane emissions, and nitrogen utilization efficiency in growing lambs offered fresh grass. J Anim Sci. (2015) 93:5764–73. doi: 10.2527/jas.2015-9515
39. Jonker A, Molano G, Sandoval E, Taylor P, Antwi C, Olinga S, et al. Methane emissions differ between sheep offered a conventional diploid, a high sugar diploid or a tetraploid perennial ryegrass cultivar at two allowances at three times of the year. Anim Prod Sci. (2016) 58:1043–8.
40. Zhao YG, Aubry A, O'Connell NE, Annett R, Yan T. Enteric methane emissions and nitrogen utilisation efficiency for two genotype of hill hoggets offered fresh, ensiled and pelleted ryegrass. Livestock Sci. (2016) 188:1–8. doi: 10.1016/j.livsci.2016.03.016
41. Niderkorn V, Martin C, Bernard M, Le Morvan A, Rochette Y, Baumont R. Effect of increasing the proportion of chicory in forage-based diets on intake and digestion by sheep. Animal. (2019) 13:718–26. doi: 10.1017/S1751731118002185
42. Ramírez-Restrepo CA, Waghorn GC, Gillespie H, Clark H. Partition of dietary energy by sheep fed fresh ryegrass (Lolium perenne) with a wide-ranging composition and quality. Anim Prod Sci. (2020) 60:1008–17. doi: 10.1071/AN19285
43. Zhao YG, Annett R, Yan T. Effects of forage types on digestibility, methane emissions, and nitrogen utilization efficiency in two genotypes of hill ewes. J Anim Sci. (2017) 95:3762–71. doi: 10.2527/jas.2017.1598
44. Harris PJ. Diversity in plant cell walls. In: RJ Henry, editor. Plant Diversity Evolution: Genotypic Phenotypic Variation in Higher Plants. Wallingford: CAB International (2005). p. 201–27. doi: 10.1079/9780851999043.0201
45. Robertson JB, Van Soest PJ. The detergent system of analysis. In: James WPT, Theander O, editor. The Analysis of Dietary Fibre in Food. New York, NY: Marcel Dekker (1981). p. 123–58.
46. Sun XZ, Sandoval E, Pacheco D. Effects of forage rape inclusion level in the diet on methane emissions from sheep. Proc N Z Soc Anim Prod. (2015) 75:64–6. Available online at: https://www.nzsap.org/system/files/proceedings/Sun%20et%20al.%20Forage%20rape%20reduces%20sheep%20methane.pdf
47. He Y, Sun X, You P. Animal, feed and rumen fermentation attributes associated with methane emissions from sheep fed brassica crops. J Anim Physiol Anim Nutr. (2021) 105:210–8. doi: 10.1111/jpn.13460
48. Sun XZ. Invited review: Glucosinolates might result in low methane emissions from ruminants fed brassica forages. Front Vet Sci. (2020) 7:588051. doi: 10.3389/fvets.2020.588051
49. Kim EJ, Newbold CJ, Scollan ND. Effect of water-soluble carbohydrate in fresh forage on growth and methane production by growing lambs. Adv Anim Biosci. (2011) 2:270.
50. Staerfl SM, Amelchanka SL, Kälber T, Soliva CR, Kreuzer M, Zeitz JO. Effect of feeding dried high-sugar ryegrass (‘AberMagic') on methane and urinary nitrogen emissions of primiparous cows. Livestock Sci. (2012) 150:293–301. doi: 10.1016/j.livsci.2012.09.019
51. Hall MB, Eastridge ML. Invited review: Carbohydrate and fat: Considerations for energy and more. Prof Anim Sci. (2014) 30:140–9. doi: 10.15232/S1080-7446(15)30101-7
52. Czerkawski JW, Breckenridge G. Fermentation of various soluble carbohydrates by rumen micro-organisms with particular reference to methane production. Br J Nutr. (1969) 23:925–37. doi: 10.1079/BJN19690104
53. Hindrichsen IK, Wettstein HR, Machmüller A, Soliva CR, Bach Knudsen KE, Madsen J, et al. Effects of feed carbohydrates with contrasting properties on rumen fermentation and methane release in vitro. Can J Anim Sci. (2004) 84:265–76. doi: 10.4141/A03-095
54. Poulsen M, Jensen BB, Engberg RM. The effect of pectin, corn and wheat starch, inulin and pH on in vitro production of methane, short chain fatty acids and on the microbial community composition in rumen fluid. Anaerobe. (2012) 18:83–90. doi: 10.1016/j.anaerobe.2011.12.009
55. Purcell PJ, Boland TM, O'Kiely P. The effect of water-soluble carbohydrate concentration and type on in vitro rumen methane output of perennial ryegrass determined using a 24-hour batch-culture gas production technique. Ir J Agric Food Res. (2014) 53:21–36. Available online at: https://www.jstor.org/stable/24369733
56. Klevenhusen F, Zebeli Q. A review on the potentials of using feeds rich in water-soluble carbohydrates to enhance rumen health and sustainability of dairy cattle production. J Sci Food Agric. (2021) 101:5737–46. doi: 10.1002/jsfa.11358
57. Bach Knudsen KE. Carbohydrate and lignin contents of plant materials used in animal feeding. Anim Feed Sci Technol. (1997) 67:319–38. doi: 10.1016/S0377-8401(97)00009-6
58. Zhao D, MacKown CT, Starks PJ, Kindiger BK. Interspecies variation of forage nutritive value and nonstructural carbohydrates in perennial cool-season grasses. Agron J. (2008) 100:837–44. doi: 10.2134/agronj2007.0178
59. Moraes MG, Chatterton NJ, Harrison PA, Filgueiras TS, Figueiredo-Ribeiro RCL. Diversity of non-structural carbohydrates in grasses (Poaceae) from Brazil. Grass Forage Sci. (2013) 68:165–77. doi: 10.1111/j.1365-2494.2012.00883.x
60. Chatterton NJ, Harrison PA, Thornley WR, Draper EA. Oligosaccharides in foliage of Agropyron, Bromus, Dactylis, Festuca, Lolium and Phleum. N. Phytol. (1990) 114:167–71. doi: 10.1111/j.1469-8137.1990.tb00387.x
61. Van Amburgh ME, Collao-Saenz EA, Higgs RJ, Ross DA, Recktenwald EB, Raffrenato E, et al. The Cornell Net Carbohydrate and Protein System: updates to the model and evaluation of version 6.5. J Dairy Sci. (2015) 98:6361–80. doi: 10.3168/jds.2015-9378
62. Südekum KH. Monosaccharide composition of cell-wall carbohydrates. Digestion and absorption. Livestock Prod Sci. (1994) 39:71–9. doi: 10.1016/0301-6226(94)90155-4
63. Ould-Ahmed M, Decau ML, Morvan-Bertrand A, Prud'homme MP, Lafrenière C, Drouin P. Fructan, sucrose and related enzyme activities are preserved in timothy (Phleum pratense L.) during wilting. Grass Forage Sci. (2017) 72:64–79. doi: 10.1111/gfs.12209
64. Fasahat P, Aghaeezadeh M, Jabbari L, Sadeghzadeh Hemayati S, Townson P. Sucrose accumulation in sugar beet: From fodder beet selection to genomic selection. Sugar Tech. (2018) 20:635–44. doi: 10.1007/s12355-018-0617-z
65. Gallagher JA, Cairns AJ, Turner LB. Fructan in temperate forage grasses; Agronomy, physiology, molecular biology. In: Shiomi N, Benkeblia N, editors. Onodera Recent Advances in Fructooligosaccharides Research (Kerala S: Research Signpost), 15–46.
66. Yoshida M. Fructan structure and metabolism in overwintering plants. Plants. (2021) 10:933. doi: 10.3390/plants10050933
67. Pavis N, Chatterton NJ, Harrison PA, Baumgartner S, Praznik W, Boucaud J, et al. Structure of fructans in roots and leaf tissues of Lolium perenne. N. Phytol. (2001) 150:83–95. doi: 10.1046/j.1469-8137.2001.00069.x
68. Hall MB, Weimer PJ. Divergent utilization patterns of grass fructan, inulin, and other nonfiber carbohydrates by ruminal microbes. J Dairy Sci. (2016) 99:245–57. doi: 10.3168/jds.2015-10417
69. Rasmussen S, Thornley JH, Parsons AJ, Harrison SJ. Mathematical model of fructan biosynthesis and polymer length distribution in plants. Ann Bot. (2013) 111:1219–31. doi: 10.1093/aob/mct087
70. Carswell AM, Gongadze K, Misselbrook TH, Wu L. Impact of transition from permanent pasture to new swards on the nitrogen use efficiency, nitrogen and carbon budgets of beef and sheep production. Agric Ecosyst Environ. (2019) 283:106572. doi: 10.1016/j.agee.2019.106572
71. Wang Y, Zhao Y, Xue F, Nan X, Wang H, Hua D, et al. Nutritional value, bioactivity, and application potential of Jerusalem artichoke (Helianthus tuberosus L.) as a neotype feed resource. Anim Nutr. (2020) 6:429–37. doi: 10.1016/j.aninu.2020.09.001
72. Apriyanto A, Compart J, Fettke J. A review of starch, a unique biopolymer – Structure, metabolism and in planta modifications. Plant Sci. (2022) 318:111223. doi: 10.1016/j.plantsci.2022.111223
73. Owens FN, Basalan M. Ruminal fermentation. In: Millen DD, De Beni M, Arrigoni RD, Pacheco L, editors. Rumenology. (2016). p. 63–102. doi: 10.1007/978-3-319-30533-2_3
74. Pérez S, Baldwin PM, Gallant DJ. Structural features of starch granules I. In: BeMiller JN, Whistler RL, editors. Starch: Chemistry Technology, 3rd Edn. San Diego, CA: Elsevier Science & Technology. (2009). p. 149–92. doi: 10.1016/B978-0-12-746275-2.00005-7
75. Cairns AJ, Begley P, Sims IM. The structure of starch from seeds and leaves of the fructan-accumulating ryegrass, Lolium temulentum L. J Plant Physiol. (2002) 159:221–30. doi: 10.1078/0176-1617-00685
76. Hall MB, Hoover WH, Jennings JP, Miller Webster TK. A method for partitioning neutral detergent-soluble carbohydrates. J Sci Food Agric. (1999) 79:2079–86. doi: 10.1002/(SICI)1097-0010(199912)79:15<207-9::AID-JSFA502>3.0.CO;2-Z
77. Cosgrove DJ. Growth of the plant cell wall. Nat Rev Mol Cell Biol. (2005) 6:850–61. doi: 10.1038/nrm1746
78. Mohnen D. Pectin structure and biosynthesis. Curr Opin Plant Biol. (2008) 11:266–77. doi: 10.1016/j.pbi.2008.03.006
79. Hocq L, Pelloux J, Lefebvre V. Connecting homogalacturonan-type pectin remodeling to acid growth. Trends Plant Sci. (2017) 22:20–9. doi: 10.1016/j.tplants.2016.10.009
80. Yapo BM, Gnakri D. Pectic polysaccharides their functional properties. In: Ramawat KG, Mérillon JM, editors. Polysaccharides: Bioactivity Biotechnology, Cham: Springer International Publishing (2015). p. 1729–49. doi: 10.1007/978-3-319-16298-0_62
81. Kaczmarska A, Pieczywek PM, Cybulska J, Zdunek A. Structure and functionality of Rhamnogalacturonan I in the cell wall and in solution: A review. Carbohydr Polym. (2022) 278:118909. doi: 10.1016/j.carbpol.2021.118909
82. Øbro J, Harholt J, Scheller HV, Orfila C. Rhamnogalacturonan I in Solanum tuberosum tubers contains complex arabinogalactan structures. Phytochemistry. (2004) 65:1429–38. doi: 10.1016/j.phytochem.2004.05.002
83. Onuh AF, Miwa K. Regulation, diversity and evolution of boron transporters in plants. Plant Cell Physiol. (2021) 62:590–9. doi: 10.1093/pcp/pcab025
84. Polko JK, Kieber JJ. The regulation of cellulose biosynthesis in plants. Plant Cell. (2019) 31:282–96. doi: 10.1105/tpc.18.00760
85. Jarvis MC. Cellulose biosynthesis: counting the chains. Plant Physiol. (2013) 163:1485–6. doi: 10.1104/pp.113.231092
86. Newman RH, Hill SJ, Harris PJ. Wide-angle x-ray scattering and solid-state nuclear magnetic resonance data combined to test models for cellulose microfibrils in mung bean cell walls. Plant Physiol. (2013) 163:1558–67. doi: 10.1104/pp.113.228262
87. Lyczakowski JJ, Bourdon M, Terrett OM, Helariutta Y, Wightman R, Dupree P. Structural imaging of native cryo-preserved secondary cell walls reveals the presence of macrofibrils and their formation requires normal cellulose, lignin and xylan biosynthesis. Front Plant Sci. (2019) 10:1398. doi: 10.3389/fpls.2019.01398
88. Albersheim P, Darvill A, Roberts K, Sederoff R, Staehelin A. Plant Cell Walls. From Chemistry to Biology. New York, NY: Garland Science (2010).
89. Lynd LR, Weimer PJ, Van Zyl WH, Pretorius IS. Microbial cellulose utilization: Fundamentals and biotechnology. Microbiol Mol Biol Rev. (2002) 66:506–77. doi: 10.1128/MMBR.66.3.506-577.2002
90. Scheller HV, Ulvskov P. Hemicelluloses. Annu Rev Plant Biol. (2010) 61:263–89. doi: 10.1146/annurev-arplant-042809-112315
91. Park YB, Cosgrove DJ. Xyloglucan and its interactions with other components of the growing cell wall. Plant Cell Physiol. (2015) 56:180–94. doi: 10.1093/pcp/pcu204
92. Pauly M, Keegstra K. Biosynthesis of the plant cell wall matrix polysaccharide xyloglucan. Annu Rev Plant Biol. (2016) 67:235–59. doi: 10.1146/annurev-arplant-043015-112222
93. Pauly M, Gille S, Liu L, Mansoori N, de Souza A, Schultink A, et al. Hemicellulose biosynthesis. Planta. (2013) 238:627–42. doi: 10.1007/s00425-013-1921-1
94. Hsieh YS, Harris PJ. Xyloglucans of monocotyledons have diverse structures. Mol Plant. (2009) 2:943–65. doi: 10.1093/mp/ssp061
95. Held MA, Jiang N, Basu D, Showalter AM, Faik A. Plant cell wall polysaccharides: Structure biosynthesis. In: Ramawat KG, Mérillon JM editors. Polysaccharides: Bioactivity Biotechnology. Cham: Springer International Publishing (2015). p. 3–54. doi: 10.1007/978-3-319-16298-0_73
96. Ebringerovd A, Heinze T. Xylan and xylan derivatives - biopolymers with valuable properties, 1 - Naturally occurring xylans structures, isolation procedures and properties. Macromol Rapid Commun. (2000) 21:542–56. doi: 10.1002/1521-3927(20000601)21:9%3C542::AID-MARC542%3E3.0.CO;2-7
97. Harris PJ, Trethewey JAK. The distribution of ester-linked ferulic acid in the cell walls of angiosperms. Phytochem Rev. (2010) 9:19–33. doi: 10.1007/s11101-009-9146-4
98. Xu F, Geng ZC, Sun JX, Liu CF, Ren JL, Sun RC, et al. Fractional and structural characterization of hemicelluloses from perennial ryegrass (Lolium perenne) and cocksfoot grass (Dactylis glomerata). Carbohydr Res. (2006) 341:2073–82. doi: 10.1016/j.carres.2006.04.033
99. Xu F, Sun JX, Geng ZC, Liu CF, Ren JL, Sun RC, et al. Comparative study of water-soluble and alkali-soluble hemicelluloses from perennial ryegrass leaves (Lolium peree). Carbohydr Polym. (2007) 67:56–65. doi: 10.1016/j.carbpol.2006.04.014
100. Ross P, Farrell MP. The road to structurally defined β-glucans. Chem Rec. (2021) 21:3178–93. doi: 10.1002/tcr.202100059
101. Nor NNM, Marikkar N, Manaf YN, Mustafa S. Chemical characteristics, physical and functional properties of some β-bonded polysaccharides: A review. Int J Chem Stud. (2021) 9:17–28. doi: 10.22271/chemi.2021.v9.i2a.11991
102. Burton RA, Fincher GB. (1,3;1,4)-beta-D-glucans in cell walls of the poaceae, lower plants, and fungi: a tale of two linkages. Mol Plant. (2009) 2:873–82. doi: 10.1093/mp/ssp063
103. Trethewey JAK, Harris PJ. Location of (1 → 3)- and (1 → 3), (1 → 4)-β-D-glucans in vegetative cell walls of barley (Hordeum vulgare) using immunogold labelling. N. Phytol. (2002) 154:347–58. doi: 10.1046/j.1469-8137.2002.00383.x
104. Lanzas C, Sniffen CJ, Seo S, Tedeschi LO, Fox DG. A revised CNCPS feed carbohydrate fractionation scheme for formulating rations for ruminants. Anim Feed Sci Technol. (2007) 136:167–90. doi: 10.1016/j.anifeedsci.2006.08.025
105. Pacheco D, Waghorn GC, Janssen PH. Decreasing methane emissions from ruminants grazing forages: A fit with productive and financial realities? Anim Prod Sci. (2014) 54:1141–54. doi: 10.1071/AN14437
106. Weisbjerg MR, Hvelplund T, Bibby BM. Hydrolysis and fermentation rate of glucose, sucrose and lactose in the rumen. Acta Agric Scand A Anim Sci. (1998) 48:12–8. doi: 10.1080/09064709809362398
107. Tóthi R, Lund P, Weisbjerg MR, Hvelplund T. Effect of expander processing on fractional rate of maize and barley starch degradation in the rumen of dairy cows estimated using rumen evacuation and in situ techniques. Anim Feed Sci Technol. (2003) 104:71–94. doi: 10.1016/S0377-8401(02)00292-4
108. Huhtanen P, Robertson S. The Effect of dietary inclusion of starch, sucrose and xylose on the utilization of dietary energy in sheep. Anim Feed Sci Technol. (1988) 21:11–21. doi: 10.1016/0377-8401(88)90015-6
109. Hindrichsen IK, Kreuzer M. High methanogenic potential of sucrose compared with starch at high ruminal pH. J Anim Physiol Anim Nutr. (2009) 93:61–5. doi: 10.1111/j.1439-0396.2007.00779.x
110. Golder HM, Celi P, Rabiee A, Heuer C, Bramley E, Miller DW, et al. Effects of grain, fructose, and histidine on ruminal pH and fermentation products during an induced subacute acidosis protocol. J Dairy Sci. (2012) 95:1971–82. doi: 10.3168/jds.2011-4671
111. Pol A, Demeyer DI. Fermentation of methanol in the sheep rumen. Appl Environ Microbiol. (1988) 54:832–4. doi: 10.1128/aem.54.3.832-834.1988
112. Zhao XH, Gong JM, Zhou S, Liu CJ, Qu MR. The effect of starch, inulin, and degradable protein on ruminal fermentation and microbial growth in rumen simulation technique. Ital J Anim Sci. (2014) 13:189–95. doi: 10.4081/ijas.2014.3121
113. Heldt JS, Cochran RC, Stokka GL, Farmer C, Mathis CP, Titgemeyer EC, et al. Effects of different supplemental sugars and starch fed in combination with degradable intake protein on low-quality forage use by beef steers. J Anim Sci. (1999) 77:2793–802. doi: 10.2527/1999.77102793x
114. Geerkens CH, Schweiggert RM, Steingass H, Boguhn J, Rodehutscord M, Carle R. Influence of apple and citrus pectins, processed mango peels, a phenolic mango peel extract, and gallic acid as potential feed supplements on in vitro total gas production and rumen methanogenesis. J Agric Food Chem. (2013) 61:5727–37. doi: 10.1021/jf401544v
115. Moharrery A, Larsen M, Weisbjerg MR. Starch digestion in the rumen, small intestine, and hind gut of dairy cows - a meta-analysis. Anim Feed Sci Technol. (2014) 192:1–14. doi: 10.1016/j.anifeedsci.2014.03.001
116. Moate PJ, Deighton MH, Jacobs J, Ribaux BE, Morris GL, Hannah MC, et al. Influence of proportion of wheat in a pasture-based diet on milk yield, methane emissions, methane yield, and ruminal protozoa of dairy cows. J Dairy Sci. (2020) 103:2373–86. doi: 10.3168/jds.2019-17514
117. Hymøller L, Hellwing ALF, Lund P, Weisbjerg MR. Milk production is unaffected by replacing barley or sodium hydroxide wheat with maize cob silage in rations for dairy cows. Animal. (2014) 8:738–47. doi: 10.1017/S1751731114000329
118. Hatew B, Podesta SC, Van Laar H, Pellikaan WF, Ellis JL, Dijkstra J, et al. Effects of dietary starch content and rate of fermentation on methane production in lactating dairy cows. J Dairy Sci. (2015) 98:486–99. doi: 10.3168/jds.2014-8427
119. Bougouin A, Martin C, Doreau M, Ferlay A. Effects of starch-rich or lipid-supplemented diets that induce milk fat depression on rumen biohydrogenation of fatty acids and methanogenesis in lactating dairy cows. Animal. (2019) 13:1421–31. doi: 10.1017/S1751731118003154
120. Nozière P, Ortigues-Marty I, Loncke C, Sauvant D. Carbohydrate quantitative digestion and absorption in ruminants: from feed starch and fibre to nutrients available for tissues. Animal. (2010) 4:1057–74. doi: 10.1017/S1751731110000844
121. Kang S, Wanapat M, Cherdthorng A. Effect of banana flower powder supplementation as a rumen buffer on rumen fermentation efficiency and nutrient digestibility in dairy steers fed a high-concentrate diet. Anim Feed Sci Technol. (2014) 196:32–41. doi: 10.1016/j.anifeedsci.2014.07.003
122. Børsting CF, Brask M, Hellwing ALF, Weisbjerg MR, Lund P. Enteric methane emission and digestion in dairy cows fed wheat or molasses. J Dairy Sci. (2020) 103:1448–62. doi: 10.3168/jds.2019-16655
123. Hindrichsen IK, Wettstein HR, Machmüller A, Jörg B, Kreuzer M. Effect of the carbohydrate composition of feed concentratates on methane emission from dairy cows and their slurry. Environ Monit Assess. (2005) 107:329–50. doi: 10.1007/s10661-005-3008-3
124. Clapperton JL, Czerkawski JW. Methane production and soluble carbohydrates in the rumen of sheep in relation to the time of feeding and the effects of short-term intraruminal infusions of unsaturated fatty acids. Br J Nutr. (1969) 23:813–26. doi: 10.1079/BJN19690092
125. Hellwing ALF, Brask M, Lund P, Weisbjerg MR. Effect of carbohydrate source rumen pH on enteric methane from dairy cows. In: MG Hassouna, edItors. Emissions of Gas Dust from Livestock (INRA N, Rennes, France, and IFIP, Le Rheu, France) (2012). p. 206–9.
126. Giger-Reverdin S, Sauvant D. Methane production in sheep in relation to concentrate feed composition from bibliographic data. In: Morand-Fehr LI, editors. Sheep Goat Nutrition: Intake, Digestion, Quality of Products Rangelands. Zaragoza P, Spain: CIHEAM (2000). p. 43–46.
Keywords: fibre, greenhouse gas, livestock, feed, rumen, degradation
Citation: Sun X, Cheng L, Jonker A, Munidasa S and Pacheco D (2022) A Review: Plant Carbohydrate Types—The Potential Impact on Ruminant Methane Emissions. Front. Vet. Sci. 9:880115. doi: 10.3389/fvets.2022.880115
Received: 21 February 2022; Accepted: 16 May 2022;
Published: 17 June 2022.
Edited by:
Paula Macarena Toro-Mujica, Universidad de O'Higgins, ChileReviewed by:
Anusorn Cherdthong, Khon Kaen University, ThailandJacobo Arango, International Center for Tropical Agriculture (CIAT), Colombia
Copyright © 2022 Sun, Cheng, Jonker, Munidasa and Pacheco. This is an open-access article distributed under the terms of the Creative Commons Attribution License (CC BY). The use, distribution or reproduction in other forums is permitted, provided the original author(s) and the copyright owner(s) are credited and that the original publication in this journal is cited, in accordance with accepted academic practice. No use, distribution or reproduction is permitted which does not comply with these terms.
*Correspondence: Xuezhao Sun, eHVlemhhb3NAaG90bWFpbC5jb20=; David Pacheco, ZGF2aWQucGFjaGVjb0BhZ3Jlc2VhcmNoLmNvLm56