- 1Departamento de Microbiología y Genética, Universidad de Salamanca, Salamanca, Spain
- 2Instituto de Investigación Biomédica de Salamanca, Salamanca, Spain
Brucella ovis is a non-zoonotic bacterium causing contagious epididymitis and other genital lesions in rams and responsible for significant economic losses in sheep-breeding areas. It is a naturally rough (without O-chains in the lipopolysaccharide) Brucella species whose virulence mechanisms have been less explored than those of zoonotic smooth brucellae (bearing O-chains that mask other outer membrane molecules). Considering the rough nature of Brucella ovis, the influence of surface components other than O-chains on its biological properties may be greater than in smooth Brucella species. Here we describe the construction and characterization of the mucR deletion mutant of virulent B. ovis PA, which is defective in a transcriptional regulator, affecting surface properties and virulence in smooth brucellae. This mutant showed increased amounts of three proteins identified as HdeA (acid-activated chaperone), Omp25d (outer membrane protein undetectable in the parental strain), and BOV_A0299 (hypothetical protein of unknown function). This observation correlated with the enhanced transcription of the corresponding genes and constitutes the first report on this type of proteome alteration in Brucella ΔmucR mutants. The upstream regions of the three genes contained AT rich domains with T-A steps described as binding sites for MucR in the Brucella abortus 2308 babR promoter (gene also upregulated in B. ovis ΔmucR), which suggests that hdeA, omp25d, and BOV_A0299 expression could be repressed by MucR through a direct binding to their promoter regions. Relative quantification of transcripts of several other genes selected according to the transcriptome of smooth brucellae ΔmucR mutants revealed not only similarities but also relevant differences among strains, such as those detected in flagellar and virB genes. Periplasmic HdeA has been related to the resistance of B. abortus to acidic pH, conditions encountered by Brucella inside phagocytes, but the deletion of hdeA in B. ovis PA and the ΔmucR mutant did not modify any of the evaluated properties of these strains. The B. ovis PA ΔmucR and ΔmucRΔhdeA mutants had defective in vitro growth and altered surface properties and architecture, exemplified by detectable amounts of Omp25d. Moreover, they showed virulence attenuation but established persistent splenic infection in mice, which encourages their evaluation as specifical attenuated vaccines against B. ovis.
Introduction
The genus Brucella (https://lpsn.dsmz.de/genus/brucella) includes facultative intracellular Gram-negative bacterial pathogens that differ, among other phenotypic and genotypic characteristics, in pathogenicity and host preference (1, 2). Depending on the Brucella species, a variety of terrestrial and marine mammal species can be infected, but amphibians, reptiles, and fish have also been reported as hosts for the atypical Brucella strains described in the recent years (1, 2). Ovine brucellosis is mainly caused by Brucella melitensis and Brucella ovis, species that share high levels of homology at the DNA level (3) but exhibit relevant differences regarding pathogenicity. Thus, B. melitensis induces abortion in the natural host, is the most relevant zoonotic Brucella species (4), and is defined as smooth because it bears O-polysaccharide chains in the lipopolysaccharide (LPS), which are required for full virulence (5, 6). On the contrary, B. ovis is rough (lacks O-chains in the LPS), has never been reported as a human pathogen, and rarely induces abortion in sheep. However, B. ovis causes contagious epididymitis and other genital lesions in rams that originate important economic losses worldwide (7, 8) but lack a specific vaccine.
B. melitensis Rev1 is an attenuated smooth vaccine, used in vaccination campaigns against ovine brucellosis, that protects not only against homologous B. melitensis infections but also against heterologous infection by B. ovis (8, 9). However, since it induces antibodies against O-chains, which are targets for the serological diagnosis of B. melitensis infection, this vaccine is banned in regions where zoonotic B. melitensis is considered eradicated. Accordingly, ovine brucellosis caused by rough B. ovis is increasing in these regions (10), which highlights the need to develop a specific vaccine against B. ovis infection that does not interfere with the diagnosis of brucellosis caused by smooth Brucella species.
Both the development of specific attenuated vaccines and the understanding of the mechanisms underlying the differences of pathogenicity and host preference observed among the species of the genus Brucella require a better knowledge of the bacterial components needed for infection in each species. Most works analyzing Brucella virulence have been performed with smooth zoonotic species (mainly with B. melitensis and Brucella abortus), although studies with B. ovis are increasing in number in the recent years. These studies have evidenced not only similarities but also relevant differences between B. ovis and smooth brucellae. Thus, some virulence factors described in smooth brucellae, such as the type IV secretion system encoded by the virB operon, the quorum-sensing transcriptional regulator VjbR, ß-1,2 glucans, and core LPS glycosyltransferases or pyruvate phosphate dikinase, are also required for virulence in B. ovis (11–13). However, some other proteins that are essential in smooth B. melitensis or B. abortus for full virulence, such as Omp10, Omp19, BepC, SP41, BacA, or the flagellar apparatus, are not required in B. ovis (11, 14, 15), while an ATP-binding cassette transporter, absent in the main zoonotic smooth brucellae, is a virulence factor in B. ovis (16).
The surface of bacterial pathogens is a key structure connecting the cell with the surrounding environment, including host cells and defense mechanisms. The LPS O-chains are essential for virulence in smooth brucellae (5, 6), and it is known that they mask other surface components (5, 17). Considering the rough nature of B. ovis, molecules exposed on the bacterial surface and/or regulatory mechanisms affecting its structure could be relevant actors in host–pathogen interactions, targets for the development of attenuated vaccines and related to the differences in pathogenicity and host preference that exist in the genus Brucella. In fact, in addition to the presence or absence of O-chains in the LPS, the Brucella species differ in outer membrane (OM) composition (5, 6, 18) and OM-related properties (5, 6, 19).
Among the virulence factors identified in smooth brucellae, MucR is a transcriptional regulator affecting the surface properties and virulence in B. melitensis and B. abortus (20–22), but that has not been studied in rough B. ovis. In this work, we have constructed a ΔmucR mutant in virulent B. ovis PA and evaluated its growth characteristics, properties related to the bacterial surface, expression of relevant genes, and virulence in cellular and animal models. Additionally, considering the high levels of HdeA—a protein that has been related to acid stress resistance in B. abortus (23)—detected in the ΔmucR mutant (see below), we have also constructed and characterized the ΔhdeA mutant in the genetic background of parental B. ovis PA and in the isogenic ΔmucR mutant. The results are discussed in comparison with those obtained with ΔmucR mutants of other brucellae.
Materials and Methods
Cloning Vectors, Bacterial Strains, and Culture Conditions
Plasmid pGEM-T Easy (Promega, Madison, WI, USA) was used to clone PCR products, and pCVD-KanD (11)—which does not replicate in Brucella spp. and confers sucrose sensitivity and kanamycin resistance—was used to construct, as described below, the recombinant plasmids containing the inactivated genes used for mutagenesis. Plasmid pBBR1MCS-2, which replicates in Brucella spp. and confers kanamycin resistance (24), was used for genetic complementation of the ΔmucR mutant with wild-type mucR.
B. ovis PA was used as a parental strain for the construction of the mutant strains described in Table 1. B. ovis PA-derived strains were cultured on tryptic soy agar (TSA) or broth (TSB) (Pronadisa-Laboratorios Conda, Torrejón de Ardoz, Spain) supplemented with 0.3% yeast extract (YE, (Pronadisa-Laboratorios Conda, Torrejón de Ardoz, Spain) and 5% horse serum (HS) (Gibco-Life Technologies, Grand Island, NY, USA) (TSA-YE-HS or TSB-YE-HS). When required for the construction and maintenance of the genetically engineered strains, kanamycin (50 μg/ml) or sucrose (5%) was added to the medium. B. ovis mutants defective in proteins of the Omp25/Omp31 family (Table 1) were previously obtained (25, 26) and used as controls in immunoblot assays. B. ovis strains were cultured at 37°C under a 5% CO2 atmosphere.
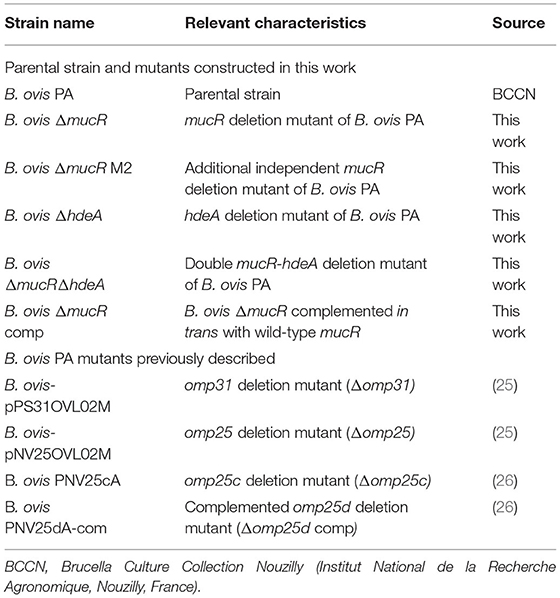
Table 1. Main B. ovis bacterial strains used in this work (intermediate bacterial strains obtained during procedures of mutagenesis are not cited).
Escherichia coli JM109 was used for the replication of pGEM-T Easy, pBBR1MCS-2, and their derived recombinant plasmids. E. coli CC118 was used for the replication of pCVD-KanD and its derived recombinant plasmids. E. coli strains were cultured at 37°C in Luria–Bertani medium that was supplemented with 50 μg/ml ampicillin or kanamycin when required. The microbiological procedures were reviewed and approved by the Biosecurity Committee of the University of Salamanca, Spain.
Primers, Analysis of DNA Sequences, and Construction and Genetic Complementation of B. ovis Mutant Strains
The primers (IDT, Leuven, Belgium) used in this work for the construction and characterization of mutant strains are listed in Table 2 and were designed according to the published genome sequence of B. ovis 63/290 (ATCC 25840), with GenBank accession numbers NC_009505 and NC_009504 for chromosomes I and II, respectively.
GenBank accession numbers were also used to retrieve the respective DNA sequences from B. melitensis 16M (AE008917 and AE008918), B. abortus 2308 (AM040264 and AM040265), and Brucella canis RM6/66 (NZ_CP007758 and NZ_CP007759). Orthologs of the analyzed genes were identified at the Kyoto Encyclopedia of Genes and Genomes (https://www.kegg.jp), and multiple nucleotide sequence alignments were performed with Clustal Omega at the European Bioinformatics Institute (https://www.ebi.ac.uk/Tools/msa/clustalo/).
For non-polar deletion of mucR in B. ovis PA, the wild-type chromosomal gene was replaced by the inactivated gene, following a procedure previously described (11, 15). Briefly, two PCR reactions were performed to amplify the 5′ and 3′ ends of mucR, together with about 700 bp located upstream or downstream the gene, respectively. Amplification of the 5′ end was performed with B. ovis PA DNA and primers mucRMUT-F and mucROVL-R, while the 3′ end was amplified with primers mucROVL-F and mucRMUT-R (Table 2). The two PCR products were fused by an overlapping PCR with primers mucRMUT-F and mucRMUT-R through the overlapping section of primers mucROVL-F and mucROVL-R (Table 2). The amplicon was cloned in plasmid pGEM-T Easy and transformed in E. coli JM109. The correct nucleotide sequence of the insert cloned in the resulting pNVmucR01 recombinant plasmid—that contains the mucR gene of B. ovis PA almost completely deleted and adjacent DNA to both sides of the gene—was verified by automated Sanger sequencing with primers Universal-F and Universal-R (Table 2) at the DNA sequencing facility of the Universidad de Salamanca, Spain. The insert of pNVmucR01 was extracted by digestion with SphI and SacI restriction enzymes and cloned into plasmid pCVDKan-D digested with the same enzymes. The resulting plasmid pNVmucR02, containing the defective mucR gene together with the sacB gene that confers sensitivity to sucrose and a kanamycin resistance cassette, was replicated in E. coli CC118 and subsequently introduced in parental B. ovis PA by electroporation. The bacteria were cultured in TSA-YE-HS plates supplemented with Kan to select an intermediate strain with the entire plasmid integrated in the chromosome after a single homologous recombination event occurred through one of the mucR ends. The intermediate strain, which is resistant to Kan and contains a copy of wild-type mucR and one copy of ΔmucR, was cultured in the presence of sucrose to select for the second homologous recombination event that leads either to a revertant strain recovering the parental genotype or to the desired ΔmucR mutant and the loss of the plasmid. Differentiation between both B. ovis PA strains was performed by PCR with specific primers annealing inside and/or outside the deleted fragment (pairs mucRMUT-F + mucRMUT-R and mucRMUT-F + mucR-R4) (Table 2). Two intermediate strains selected from two independent electroporation events were selected to obtain two independent mutant strains for confirmative studies. The ΔmucR mutant was complemented in trans with plasmid pNVmucRcom01, which is pBBR1MCS-2 bearing wild type mucR of B. ovis PA amplified with primers mucR-com1 and mucR-com2 (Table 2).
The ΔhdeA mutant of B. ovis PA was obtained with the same procedure but using the specific primers listed in Table 2. The pNVhdeA02 plasmid was electroporated in parental B. ovis PA to obtain the ΔhdeA mutant and in the ΔmucR mutant to obtain the ΔmucRΔhdeA double mutant.
Growth, Autoagglutination, and Susceptibility Assays
The growth characteristics of mutant strains were evaluated in comparison with those of the parental strain B. ovis PA. Bacterial suspensions of optical density values at 600 nm (OD600) of 0.2 were prepared in phosphate-buffered saline (PBS). Serial dilutions were plated in triplicate on TSA-YE-HS plates that were then incubated for 10 days at 37°C in a 5% CO2 atmosphere. The colony size was periodically checked, and the number of colony-forming units (CFU)/ml corresponding to the OD600 score of 0.2 was determined and used for further experiments.
Growth curves were determined in TSB-YE-HS inoculated with 2.5 × 108 CFU/ml of each B. ovis strain and incubated under agitation at 120 rpm at 37°C and 5% CO2. The OD600 scores were periodically determined, and the number of CFU/ml was evaluated at several time points by platting serial dilutions on TSA-YE-HS plates. To evaluate susceptibility to hypersaline medium, TSA-YE-HS containing 1 M NaCl was inoculated with 2.5 × 108 CFU/ml of each bacterial strain and incubated under agitation at 120 rpm at 37°C and 5% CO2 for 24 h when the number of CFU/ml was determined. Susceptibility to acid pH was evaluated similarly in TSB-YE-HS adjusted to pH 4.4 and inoculated with 5 × 108 CFU/ml of each bacterial strain. The results were presented as means ± SD of three assays.
Autoagglutination ability was evaluated as previously described (11, 26) by determining the evolution of OD600 values—in static incubation at room temperature—of bacterial suspensions in TSA-YE-HS of initial OD600 scores of 0.8. Susceptibility test to polymyxin B (10 mg/ml), sodium deoxycholate (10 mg/ml), sodium dodecyl sulfate (SDS, 10 mg/ml), Tween 20 (10%), 3-[(3-cholamidopropyl)dimethylammonio]-1-propanesulfonate (CHAPS, 100 mg/ml), 2,2′-dipyridyl (0.1 M), and hydrogen peroxide (H2O2, 7.5%) (Sigma Aldrich, St. Louis, MO, USA) was performed in a disc assay. The bacterial suspensions (100 μl containing 108 CFU) were spread on TSA-YE-HS plates. Then, a paper disc (diameter of 9 mm) was deposited on the center of the plate and soaked with 20 μl of each agent. The diameter of the growth inhibition zone was measured after 7 days of incubation at 37°C and 5% CO2 (four diameter scores were considered to establish the mean value for each plate). The results were presented as means ± SD of three assays.
Protein and Immunological Techniques
SDS-polyacrylamide gel electrophoresis (SDS-PAGE) was performed, as previously described (18), with whole-cell bacterial lysates and using pre-stained protein marker VI (Applichem-Panreac, Barcelona, Spain) as protein standard. The samples were resolved in a Protean II xi cell (Bio-Rad, Hercules, CA, United States) using 14% acrylamide/bisacrylamide gels. After the electrophoresis step, the protein bands were stained with Coomassie blue or transferred to nitrocellulose with a semidry electroblotter (Amersham, GE Healthcare, Little Chalfont, United Kingdom) for the analysis of reactivity with antibodies against surface antigens.
The proteomic analysis of protein bands excised from SDS-PAGE gels was performed by MALDI-TOF or LC–MS/MS, after trypsin in-gel digestion, in the proteomics facility of Centro de Investigación del Cáncer, Salamanca, Spain, following its standardized procedures.
Immunoblot was performed, as described before (18, 28), with rabbit antibodies raised previously against Omp31b, Omp25c, or Omp25d recombinant outer membrane proteins of Brucella spp. (18), a goat anti-rabbit IgG-peroxidase conjugate as secondary antibody, and 4-chloro-1-naphthol (Sigma Aldrich, St. Louis, MO, USA) as substrate for peroxidase. Before immunoblotting, the proteins transferred to the nitrocellulose membrane were stained with Ponceau S (Sigma Aldrich, St. Louis, MO, USA) to check the protein load (that was similar in all tested strains).
Quantitative Reverse-Transcription PCR
For relative quantification of transcripts by real-time reverse transcription-PCR (RT-qPCR), bacterial strains were incubated in TSB-YE-HS for 15 h (exponential growth phase), and RNA was extracted using the E.Z.N.A.® Bacterial RNA Kit (Omega Bio-tek Inc., Norcross, GA, USA). Contaminant DNA was removed by DNaseI, RNase-free treatment (Thermo Fisher Scientific, Vilnius, Lithuania), and cDNA synthetized from 1 μg of RNA with the NZY first-strand cDNA synthesis kit (NZYTech Lda., Lisboa, Portugal). A reaction without retrotranscriptase was also settled to be used in RT-PCR reactions as control of DNA absence. Real-time reactions were performed, in a StepOnePlusTM apparatus (Applied Biosystems, Foster City, USA), on cDNA obtained from B. ovis PA, the ΔmucR mutant, and the ΔmucR mutant complemented with wild-type mucR. The primer pairs listed in Table 2 and NZYSupreme qPCR Green Master Mix (2x) ROX plus (NZYTech Lda., Lisboa, Portugal) were used in the amplification reactions. Two independent biological samples with three technical replicates for each strain and primer pair were analyzed. Calculation of relative expression was performed with the StepOneTM software v2.3 (2−ΔΔCt method). B. ovis PA and 16S were used as reference strain and gene, respectively, and the results were expressed as mean ± SD of the log2 of the relative quantity (log2RQ).
Virulence Assays
J774A.1 murine macrophages were used to evaluate the intracellular behavior of B. ovis strains in phagocytic cells as previously described (14). Briefly, 2 × 104 macrophages per well were incubated for 24 h in 96-well plates at 37°C and 5% CO2. The macrophages were then infected with each B. ovis strain at a multiplicity of infection of 1:200. After an incubation period of 2 h, extracellular bacteria were killed with gentamycin, macrophages lysed, and the number of intracellular bacteria determined in three wells per strain by plating serial dilutions on TSA-YE-HS (t0). Intracellular bacteria were also determined at 20 and 44 h later (t20 and t44) in three wells per strain, where infected macrophages had been maintained in the presence of gentamycin. The results were expressed as means ± SD (n = 3) of the log10 CFU/well values at each time point.
Virulence in mice was evaluated in 6-week-old female BALB/c mice (Charles River Laboratories, Chatillon-sur-Chalaronne, France). Mice received a week before were inoculated intraperitoneally with 106 or 108 CFU of each bacterial strain in 0.2 ml PBS. At several post-infection (p.i.) time points, CFU were determined in spleen, in five mice per group, as described before (29). The results were expressed as means ± SD (n = 5) of the log10 CFU/spleen values at each time point. The mice experiments were designed according to the Spanish and European legislation for research with animals (RD 53/2013 and directive 2010/63/EU).
Statistical Analysis
Statistical analysis was performed by one-way ANOVA and Fisher's LSD using the GraphPad Prism 7 Software (GraphPad Software Inc., San Diego, CA, United States). A 99% confidence interval was considered for statistically significant differences (P < 0.01).
Results
HdeA, Omp25d, and BOV_A0299 Are Over-translated in B. ovis PA ΔmucR
A first proteomic analysis by SDS-PAGE was performed with parental B. ovis PA, the ΔmucR mutant and the ΔmucR mutant complemented in trans with wild-type mucR. When compared to the parental strain, three proteins of the ΔmucR mutant showed increased levels that recovered those of the parental strain after complementation with wild-type mucR (Figure 1). The results were also reproduced in a second ΔmucR mutant (ΔmucR M2) (Figure 1), which was obtained from another independent mutagenesis procedure and was included in some analysis to obtain additional confirmation of results. The three protein bands were excised from the gel and identified by the proteomics facility of Centro de Investigación del Cáncer, Salamanca, Spain. A protein of low molecular mass showed a remarkable intensity in the ΔmucR mutant (Figure 1) and was identified as the product of the gene BOV_A0312, which codes for a protein annotated as acid-activated periplasmic chaperone HdeA in the genome sequence of B. ovis 63/290 (GenBank accession number NC_009504) and that contributes to acid resistance in B. abortus 2308 (23). Another protein band matched Omp25d (BOV_0115) (Figure 1), a member of the Brucella Omp25/Omp31 family of OMPs that has never been detected in parental B. ovis PA and was only detected in a Δomp25d mutant complemented in trans that overexpresses omp25d (18). The third overproduced protein, located between Omp25d and HdeA in the SDS-PAGE gel, was identified as the product of gene BOV_A0299, which corresponds to a hypothetical protein lacking homology with proteins of known function.
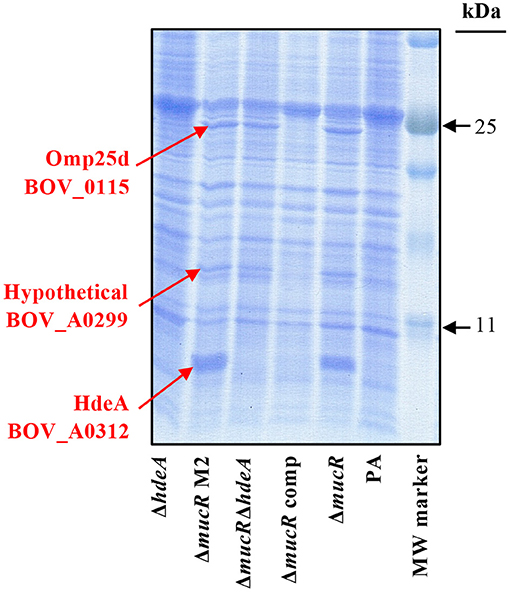
Figure 1. Protein profile in SDS-PAGE of the B. ovis PA mutants. Proteins were stained with Coomassie blue after electrophoresis. Overrepresented proteins in the ΔmucR and ΔmucRΔhdeA mutants, which were excised from the gel for identification, are highlighted in red.
Considering that brucellae are intracellular bacteria facing acidic conditions in the phagocyte (30) and that HdeA, described as chaperone activated under acidic conditions, is highly represented in the ΔmucR mutant (Figure 1) and has never been studied in B. ovis, the hdeA deletion mutant was constructed in B. ovis PA and in the ΔmucR mutant. Both single ΔhdeA and double ΔmucRΔhdeA mutants were included in further experiments.
As expected, overproduction of HdeA was not detected when hdeA was deleted from the ΔmucR mutant, but the resulting double ΔmucRΔhdeA mutant maintained the increased levels of Omp25d and BOV_0299 (Figure 1). No apparent differences in the SDS-PAGE protein profile were observed between the parental strain and the single ΔhdeA mutant, although it must be considered that a discrete HdeA band is not detected in the parental strain (Figure 1).
B. ovis PA ΔmucR and ΔmucRΔhdeA, but Not ΔhdeA, Have Defective in vitro Growth
The colony size of mutant strains and parental B. ovis PA in TSA-YE-HS solid medium was monitored daily and recorded after 6 days (Figure 2A). Colonies of the ΔmucR mutant were significantly smaller than those of B. ovis PA, and complementation of the mutant with wild-type mucR restored the parental phenotype. The ΔmucRΔhdeA double mutant had slightly bigger colonies than the ΔmucR mutant, but not as big as those of the parental strain (Figure 2A). On the contrary, the single deletion of hdeA in B. ovis PA did not have an apparent effect on colony size (Figure 2A).
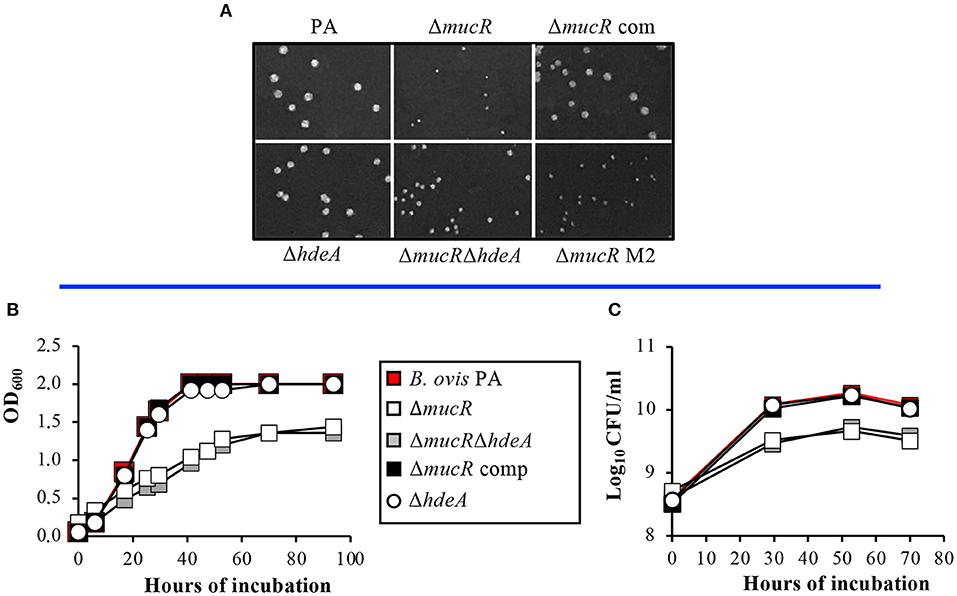
Figure 2. Growth characteristics of the B. ovis PA mutants. Colony size in TSA-YE-HS plates was monitored daily and recorded after 6 days of incubation (A). Growth in TSB-YE-HS liquid medium was evaluated by measuring the evolution over time in OD600 (B) and CFU/ml (C) values.
The growth deficiencies of the mucR mutants were also evident in TSB-YE-HS liquid medium since the ΔmucR and ΔmucRΔhdeA mutants had reduced replication rates, and their OD600 and log10 CFU values in the stationary phase were significantly lower than those obtained with the parental strain and the ΔhdeA mutant (Figures 2B,C).
B. ovis PA ΔmucR and ΔmucRΔhdeA, but Not ΔhdeA, Have Altered Outer Membrane-Related Properties
A disc assay was used to determine the susceptibility of the mutant strains derived from B. ovis PA to several compounds related to outer membrane properties and/or survival in the host. No statistically significant differences were found between parental B. ovis PA and the mutant strains regarding susceptibility to H2O2 or the high-affinity iron chelator 2,2′-dipyridyl (Table 3). On the contrary, the ΔmucR single mutant and the ΔmucRΔhdeA double mutant were more susceptible (P < 0.001) to the cationic peptide polymyxin B and the detergents sodium deoxycholate, sodium dodecyl sulfate, Tween 20, and CHAPS (Table 3). Complementation in trans of the ΔmucR mutant with wild-type mucR restored the parental phenotype (Table 3).
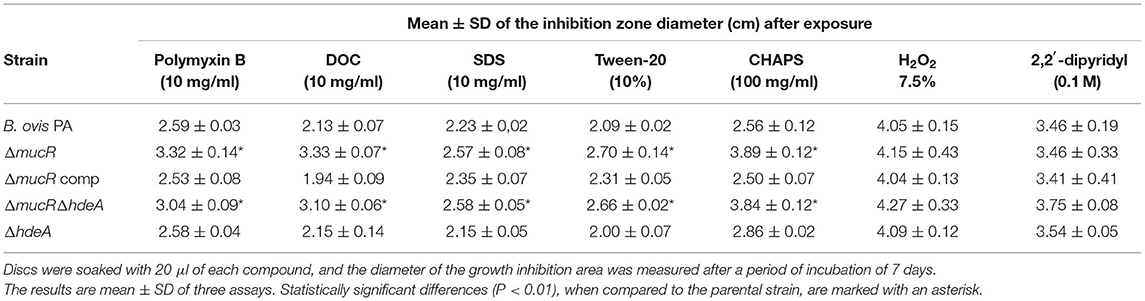
Table 3. Susceptibility pattern of the B. ovis PA mutants in a disc assay (discs—diameter of 9 mm—were deposited on the center of TSA-YE-HS plates containing 108 CFU of each bacterial strain).
Additionally, the ΔmucR single and double mutants showed autoagglutination ability indicative of surface alterations (Figure 3), but no significant differences were found among strains regarding survival to exposure for 24 h to hypersaline or acid pH conditions (Supplementary Table S1).
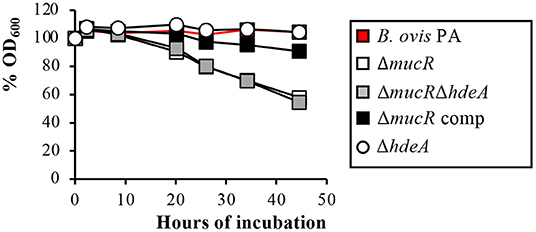
Figure 3. Autoagglutination assay with the B. ovis PA mutants. The percentages of the OD600 values with respect to the initial value of 0.8 were recorded over time and are presented in the figure.
Immunodetection of Omp25d and Major OMPs of the Omp25/Omp31 Family
Confirmation of Omp25d overproduction in the ΔmucR and ΔmucRΔhdeA mutants was obtained by immunoblot with rabbit sera raised previously against recombinant Omp25d (18). Both mutant strains developed a protein band of identical molecular mass to that detected in a B. ovis PA Δomp25d mutant complemented in trans with omp25d and that overproduces Omp25d (18) (Figure 4A). As expected according to previous reports, Omp25d was not detected by immunoblot in parental B. ovis PA (18), and this phenotype was recovered in the ΔmucR mutant complemented with mucR (Figure 4A). Omp25d was also undetectable in the ΔhdeA mutant (Figure 4A), which is in accordance with the SDS-PAGE protein profile (Figure 1).
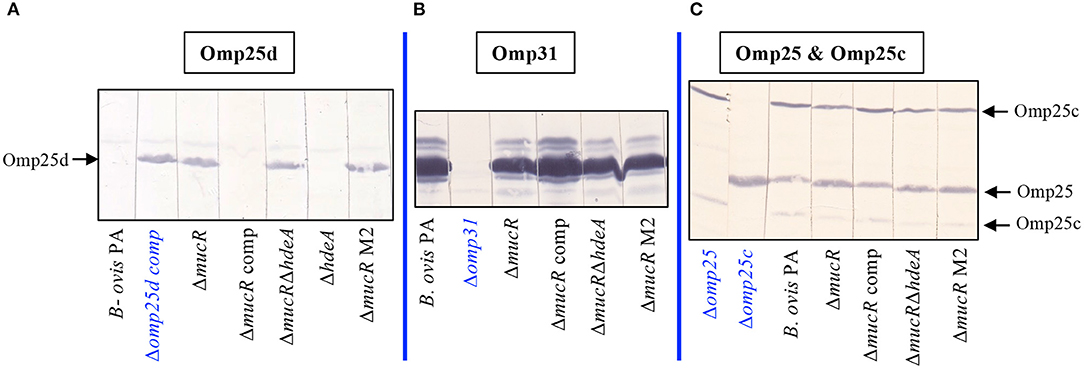
Figure 4. Detection of Omp25d and major outer membrane proteins of the Omp25/Omp31 family in the B. ovis PA mutants. Omp25d (A), Omp31 (B), Omp25, and Omp25c (C) were detected by immunoblot after SDS-PAGE resolution of whole-cell lysates. Previously characterized rabbit sera raised against recombinant proteins of the Omp25/Omp31 family (18) were used as primary antibodies. The anti-Omp25c serum allows the simultaneous detection of Omp25 and Omp25c because of cross-reactivity due to the amino acid sequence similarity between both proteins (18). Mutant B. ovis PA strains defective in Omp31, Omp25, or Omp25c obtained before (25, 26) were used as negative control strains for each protein. Since, as previously described, Omp25d is not detected in parental B. ovis PA (18), an omp25d mutant complemented in trans with wild-type omp25d and that overproduces Omp25d (18, 26) was used as the positive control strain for this protein. The omp25d mutant was not included since B. ovis PA behaves as the negative control for Omp25d.
Considering the overproduction of Omp25d detected in the ΔmucR and ΔmucRΔhdeA mutants and that members of the Brucella spp. Omp25/Omp31 seem to be tightly balanced (18), these mutants were evaluated in immunoblot (Figures 4B,C) with rabbit sera that allow the immunological detection of Omp31, Omp25, and Omp25c major OMPs (18). Except for the Δomp31 mutant of B. ovis PA used as control in this assay (25), the characteristic multiple band pattern of Omp31 was detected in all mutant strains tested, although Omp31 appeared to be more abundant in the parental strain and the complemented ΔmucR mutant (Figure 4B). Although the multiple band profile of Omp31 makes it difficult to estimate its quantity by SDS-PAGE, a lower amount of Omp31 in the ΔmucR and ΔmucRΔhdeA mutants would be in accordance with the transcriptomic results described below. Omp25c and Omp25 were also detected in the mutant strains (Figure 4C) by reactivity with an anti-Omp25c sera that cross-react with Omp25, thus allowing the detection of both proteins (18). Differentiation between Omp25 and Omp25c bands was performed according to the reactivity profile of the Δomp25 and Δomp25c control strains previously obtained. Although deletion of mucR in B. ovis PA did not lead to important alterations in the levels of Omp25 and Omp25c as visualized by immunoblot (Figure 4C), subtle differences seem to exist among strains (single and double ΔmucR mutants seem to have a more intense Omp25 band and less intense Omp25c bands) that would also correlate with the RT-qPCR results described below.
ΔmucR Mutants of B. ovis PA, B. melitensis 16M, B. abortus 2,308, and B. canis RM6/66 Display Differential Transcriptional Patterns
According to the results obtained in SDS-PAGE, HdeA and BOV_A0299 were overproduced in the ΔmucR mutant of B. ovis PA (Figure 1), suggesting that the corresponding genes are upregulated in this mutant. However, the analysis of previous works performed with smooth B. melitensis 16M and B. abortus 2308 showed that orthologs of BOV_A0299 were not listed among the genes regulated by MucR and that hdeA was not cited as over-transcribed in the ΔmucR mutant of B. abortus 2308 (Table 4). Considering these differences among species, a transcriptomic analysis of the B. ovis PA ΔmucR mutant and the complemented strain was performed in comparison with the parental strain. A panel of 19 genes was selected according to the protein profile detected in SDS-PAGE and immunoblot in the B. ovis PA mutants (Figures 1, 4) and to the transcriptome of ΔmucR mutants analyzed in smooth Brucella species (20, 31). Data of the ΔmucR mutant of B. canis RM6/66 described during the preparation of this manuscript (32) were also included in the interspecies comparative analysis summarized in Table 4.
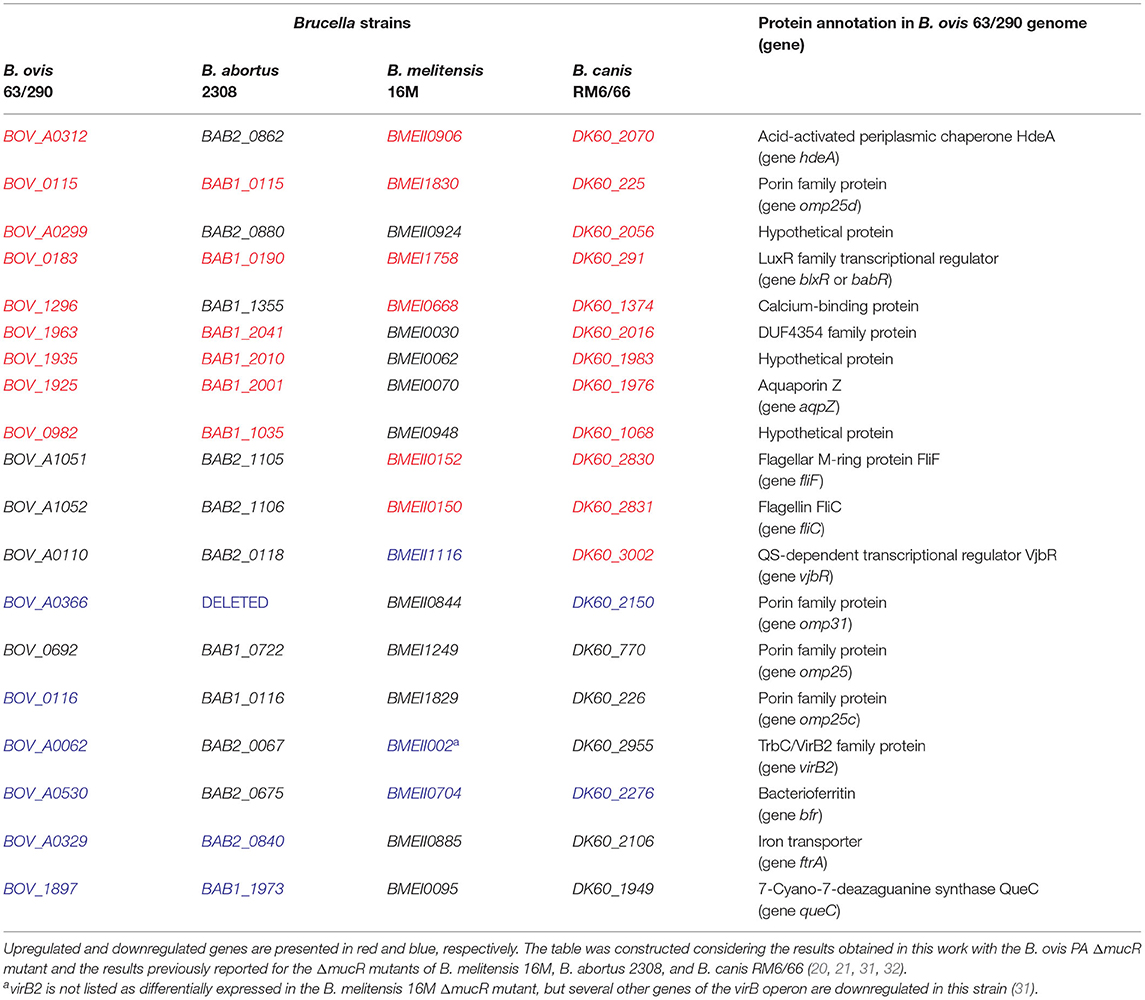
Table 4. Genes of B. ovis analyzed in this work and their orthologs in Brucella strains with available ΔmucR mutants.
In accordance with the proteomic results (Figure 1), transcripts for genes hdeA, omp25d, and BOV_A0299 were overrepresented in the ΔmucR mutant of B. ovis PA when compared to the parental strain and the complemented mutant (Figure 5); the results were also reproduced in B. canis RM6/66 (Table 4). Considering the results described in B. abortus 2308 (20, 33), genes BOV_0982 and BOV_0183, the latter coding for the LuxR family quorum sensing transcriptional regulator blxR (34) also named babR (35), were also included in the transcriptomic analysis. In B. abortus 2308, overexpression of the corresponding orthologs BAB1_0190 and BAB1_1035 in the ΔmucR mutant has been associated to the demonstrated direct binding of MucR to their promoter region (20, 33). Both genes were also upregulated in the ΔmucR mutant of B. ovis PA (Figure 5) and in B. canis RM6/66 (Table 4). However, the B. melitensis 16M ortholog of BOV_0982 (BMEI0948) does not appear among the upregulated genes in the ΔmucR mutant (31) (Table 4).
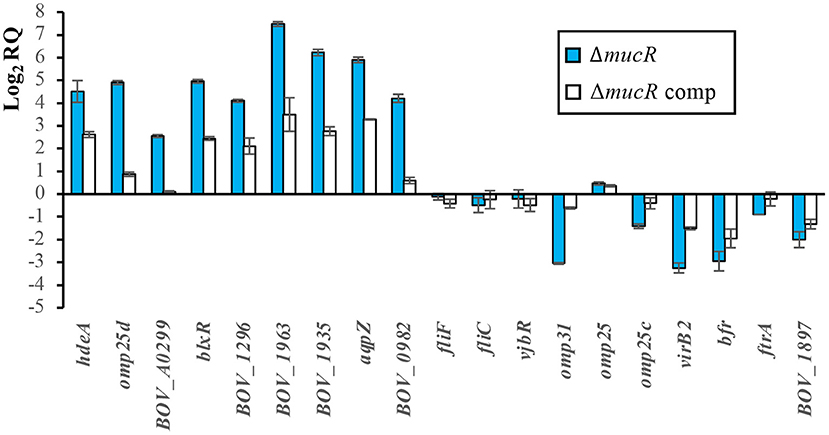
Figure 5. Relative quantification of transcripts of selected genes by RT-qPCR. The relative expression of each gene was evaluated by the 2−ΔΔCt method with the StepOneTM software v2.3 and using B. ovis PA and 16S gene as reference strain and gene, respectively. The results are presented as means ± SD of the log2 of the relative quantities (log2RQ) of two biological samples with three technical replicates each (genes of parental B. ovis PA, which was used as reference strain for relative quantification of transcripts, have a log2RQ value of 0).
Four other genes (orthologs of genes BOV_1296, BOV_1963, BOV_1935, and BOV_1925) upregulated in either B. melitensis or B. abortus ΔmucR mutants (but not simultaneously in both strains) were also found upregulated in the ΔmucR mutant of B. ovis (Figure 5) and B. canis (32) (Table 4). On the contrary, flagellar genes fliF and fliC, which are upregulated in the ΔmucR mutants of B. melitensis 16M and B. canis, maintain the transcription level of the parental strain in the B. ovis PA mutant (Figure 5), and no flagellar genes are listed among the upregulated genes in the B. abortus mutant (20) (Table 4). More interspecies differences in the transcriptome of Brucella ΔmucR mutants were observed regarding vjbR, which codes for a quorum sensing-related regulator acting as activator of flagellar and virB genes in B. melitensis (36) and that is required for virulence in Brucella spp. (11, 36, 37). Expression patterns dependent on Brucella species were also detected for genes encoding surface antigens of the Omp25/Omp31 family, for queC, and for virulence genes reported as downregulated in ΔmucR mutants of smooth B. melitensis 16M or B. abortus 2308 (20, 31), such as the virB operon encoding the type IV secretion system or iron homeostasis genes bfr and ftrA (Figure 5, Table 4).
B. ovis PA ΔmucR and ΔmucRΔhdeA, but Not ΔhdeA, Have Attenuated Virulence in Macrophages and Mice
Murine J774A.1 macrophages were used to determine the ability of the B. ovis PA mutants obtained in this work to internalize, survive, and replicate within phagocytic cells. While the ΔhdeA mutant behaved as the parental strain, the ΔmucR and ΔmucRΔhdeA mutants internalized similarly to the parental strain but showed increased killing at t20. Both mutants were able to replicate thereafter, but intracellular counts at t44 were in the order of 1 log unit lower than those obtained with the parental strain (Figure 6A).
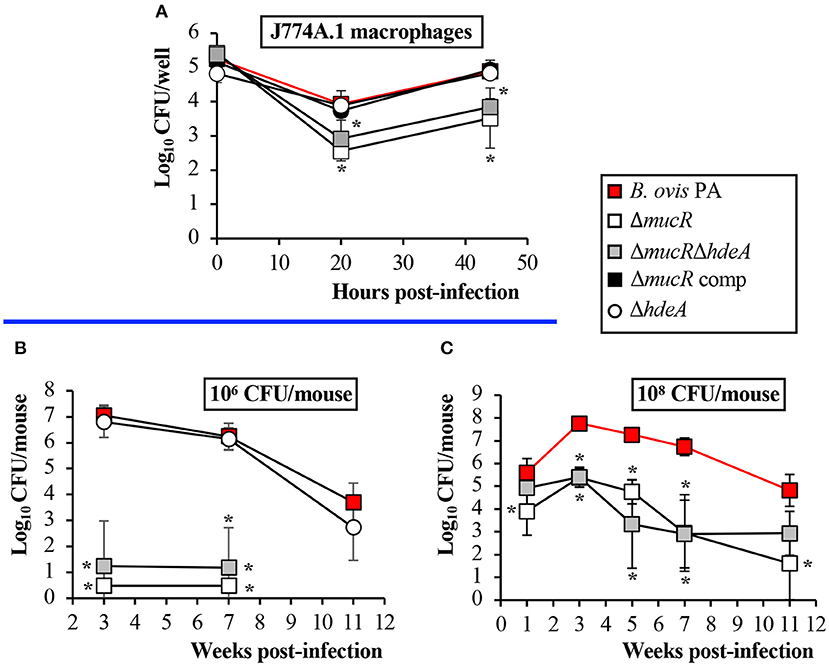
Figure 6. Virulence of the B. ovis PA mutants in J774A.1 macrophages (A) and mice inoculated intraperitoneally with 106 CFU (B) or 108 CFU (C). Results in the macrophages are expressed as means ± SD (n = 3) of the log10 CFU/well at each time point. For evaluation of virulence in mice, bacterial numbers were determined in spleen at several time points. The results are presented as means ± SD (n = 5) of the log10 CFU/spleen. Statistically significant differences (P < 0.01) with the parental strain are marked with an asterisk.
For a first evaluation in the mouse model, the mice were inoculated with 106 CFU of the parental strain or the isogenic mutants obtained in this work. Bacterial counts in the spleen were determined at weeks 3 and 7 p.i., time points that correspond to the peak of infection in mice for B. ovis PA (acute phase of infection) and to the plateau of the chronic phase (25). The ΔhdeA mutant did not show statistically significant differences with the parental strain even at week 11 p.i., an additional point of analysis included for this mutant (Figure 6B). On the contrary, spleens of mice inoculated with the ΔmucR and ΔmucRΔhdeA mutants were free of infection, except for one mouse at each sampling point of the group inoculated with B. ovis PA ΔmucRΔhdeA (Figure 6B). A second experiment was performed in mice inoculated with 108 CFU—a dose usually employed for protection experiments with B. ovis attenuated vaccines (13, 25, 29)—in which bacterial splenic colonization was monitored from week 1 to 11 p.i. In these conditions, the ΔmucR mutants produced a persistent infection but with bacterial splenic colonization levels that were significantly lower than those observed with the parental strain (Figure 6C).
Discussion
In the last decade, the regulatory network of the MucR transcriptional regulator has been depicted in B. melitensis 16M and B. abortus 2308 (20, 21, 31) and very recently also in B. canis RM6/66 (32). Several upregulated or downregulated genes at the transcription level were found in the corresponding ΔmucR mutants, but apart from some flagellar proteins in B. melitensis 16M (21), no reports exist regarding whether the modification of the transcription rate of affected genes effectively leads to increased or reduced levels of the encoded proteins. In this work, we demonstrate that deletion of mucR in B. ovis PA leads to increased levels of transcripts for hdeA, omp25d, and BOV_A0299 (Figure 5), resulting in increased translation of their respective encoded proteins, which were easily detected after SDS-PAGE, followed by Coomassie blue staining (Figure 1).
HdeA has been described in enteric bacteria as a periplasmic chaperone with activity at low pH and required for acid resistance (38, 39), and its ortholog in B. abortus 2308 was found to be involved in survival to in vitro acid stress exposure but dispensable for virulence in macrophages and mice (23). Considering its high overproduction in the ΔmucR mutant of B. ovis PA and its described role in resistance to acid pH, a condition encountered by Brucella inside phagocytes, we considered it interesting to analyze the relevance of HdeA for the in vitro and in vivo behavior of B. ovis PA and the isogenic ΔmucR mutant. However, no differences between the parental strain and the B. ovis PA ΔhdeA mutant were found in any of the tests performed, including survival at acid pH in the conditions assayed (Supplementary Table S1) and virulence (Figure 6). Accordingly, HdeA is not essential, at least in B. abortus 2308 and B. ovis PA, to survive under the acidic conditions that Brucella encounters inside phagocytes or to follow a normal infectious process in mice inoculated intraperitoneally. Nevertheless, HdeA of pathogenic enteric bacteria has exclusive activity at stomach pH ranges (pH values below 3)—conditions that have not been analyzed with Brucella ΔhdeA mutants—and is essential for bacterial resistance to this acidic environment (38, 39). Accordingly, although the intestinal mucosa is not considered a relevant port of entry for Brucella (40), HdeA could have a role if some degree of invasion by this route occurs. Moreover, contribution of HdeA to Brucella virulence in the natural host cannot be discarded. Although other characteristics not evaluated in this work could be affected, overproduction of HdeA in the B. ovis PA ΔmucR mutant (Figure 1) was not responsible for any of its observed defective characteristics (i.e., growth, OM-related properties, and virulence) nor provided beneficial characteristics to the ΔmucR mutant since the behavior of the ΔmucRΔhdeA double mutant was undistinguishable from that of the ΔmucR single mutant in all tests performed.
Omp25d is a member of the Omp25/Omp31 family, which is constituted by seven homologous outer membrane proteins with a different occurrence and distribution pattern depending on the Brucella species (18). Detection of Omp25d in wild-type brucellae has not been reported, and this protein could only be detected in a B. ovis Δomp25d mutant complemented in trans with omp25d and that overexpresses omp25d (18). The protein has been linked to the virulence of B. ovis PA (26), and its overproduction could be somehow involved in the attenuation of the ΔmucR mutant (Figure 6). In this respect, proteins of the Omp25/Omp31 family seem to be finely tuned (18), as it is also suggested by the lower transcription levels of omp31 and omp25c—which encode two major OMPs of the family (18)—that were observed in the Omp25d-overproducing ΔmucR mutant (Figure 5) and that also seem to correlate with lower protein levels (Figure 4). According to these results, which include the lack of detection of Omp25d in B. ovis PA under standard culture conditions (Figures 1, 4A), MucR could modulate the levels of surface proteins of the Omp25/Omp31 family in response to environmental stimuli within the host to build an optimal surface architecture for the establishment of infection.
Regarding BOV_A0299, the third overproduced protein detected in the ΔmucR mutant of B. ovis PA (Figure 1), no homology with proteins of known function has been evidenced, but the protein is highly conserved in the genus Brucella, at least in the classical species (data not shown). According to the attenuation of the ΔmucR mutant and the degree of conservation of BOV_A0299 in the genus Brucella, this protein emerges as a new candidate to be further studied regarding its role in the biology of the bacterium.
The DNA region located upstream of babR (or blxR), a gene that is upregulated in the four available Brucella ΔmucR mutants (Table 4), has been previously analyzed in B. abortus 2308 while searching for the target site for MucR binding (33). Multiple binding sites of MucR to the promoter region of babR were identified, and it was determined that AT-rich regions containing T-A steps were involved in the MucR–promoter interaction resulting in transcriptional repression (33). Considering these observations, we have analyzed the DNA region located upstream of the genes coding for the three proteins overproduced in the B. ovis PA ΔmucR mutant (HdeA, Omp25d, and BOV_A0299). The three genes presented AT-rich regions containing T-A steps (Supplementary Figure S1) that could be targets for MucR binding, which suggests that MucR also acts as a direct repressor of these genes.
Despite the homology at the DNA level shared by the classical Brucella species (3), the ΔmucR available mutants show not only common transcriptomic characteristics but also differences in the expression of several genes (Table 4). Among the first genes that were shown to be regulated by MucR, several flagellar genes of B. melitensis 16M locus I (fliF, fliC, ftcR, and flgE) and that are required for virulence (41) can be mentioned (21). It was proposed that the downregulation of flagellar genes mediated by MucR was due to its repressor activity upstream of ftcR, which encodes a master regulator of flagellar expression (21). On the contrary, in B. ovis PA no MucR-mediated positive or negative regulation of fliC and fliF flagellar genes has been evidenced (Figure 5, Table 4). Nevertheless, this observation is not in disagreement with the proposed role of MucR as repressor of ftcR since the predicted binding site of FtcR upstream of fliF (42) is missing in B. ovis due to two independent deletion events accounting for 283 bp (11). Even if there was a MucR-mediated regulation of flagellar gene expression in B. ovis PA, deletion of mucR would not have a relevant impact in flagellum-dependent bacterial properties since it has been demonstrated that the entire three main flagellar loci are dispensable for B. ovis PA virulence, and their deletion does not modify any of the evaluated bacterial characteristics (15). Upregulation of flagellar genes detected in B. canis RM6/66 ΔmucR (32) was also in accordance with the results described for the B. melitensis 16M mutant (Table 4), but the expression of flagellar genes in B. abortus 2308 does not seem to be dependent on MucR (20) (Table 4). These differences cannot be explained according to distinctive traits in DNA regions located upstream of fliC, fliF, or ftcR since they are almost identical between B. melitensis 16M and B. abortus 2308 (data not shown). However, in addition to MucR and FtcR, other actors affecting flagellar gene expression have been identified in B. melitensis 16M (e.g., VjbR, BlxR, RpoE1, RpoH2, BdpA, and YbeY), which suggest a complex regulation network for flagellar gene expression (21). Therefore, the differences in flagellar gene expression detected among Brucella species in ΔmucR mutants could be related to different effects on the expression of the other regulators of the network, although the influence of the experimental conditions used in each work cannot be discarded.
Another recognized virulence factor in Brucella, including B. ovis PA, is the type IV secretion system whose components are encoded by the virB operon (11, 30). Although binding of MucR upstream of virB1 has been evidenced in B. abortus 2308 (33), this interaction had a weaker affinity than that observed with the babR promoter and was considered to have little impact on the expression of virB genes in this strain (33). These observations corroborate previous results where no virB genes were listed among the differentially expressed genes in the ΔmucR mutant of B. abortus 2308 (20) and are in accordance with results obtained with the B. canis RM6/66 mutant. On the contrary, several virB genes were downregulated in the ΔmucR mutant of B. melitensis 16M (31), and reduced virB2 transcription was detected in the B. ovis PA ΔmucR mutant (Figure 5, Table 4). Considering the low affinity of MucR by the virB promoter (33) and that DNA regions located upstream of virB1 and virB2 are almost identical in the four species (data not shown), differences among species regarding the role of MucR in virB expression are probably not related to a direct binding of MucR to the promoter region but to an indirect effect on other known or unknown regulators of this operon that is controlled by a complex regulatory network of repressors and activators responding to different environmental stimuli (30). BabR and VjbR, listed in Table 4, are two quorum-sensing-related transcriptional regulators known to affect virB expression, but several other proteins have been involved in its regulation (30). In this respect, the reported differences among Brucella strains regarding the production of VirB proteins under several culture conditions provide evidence of a differential regulation of virB expression within the genus Brucella (43).
The differences among Brucella spp. ΔmucR mutants were not exclusive to the transcriptomic pattern but also extended to phenotypic characteristics. A surprising observation was that, while the deletion of mucR caused in vitro growth defects in B. melitensis 16M (21), B. abortus 2308 (20), and B. ovis PA (Figure 2)—although they were less evident in B. melitensis 16M (21, 31)—the growth characteristics of the ΔmucR mutant of B. canis RM6/66 were identical to those of the parental strain (32). Also remarkable is the fact that, contrary to what was described in smooth Brucella ΔmucR mutants, B. ovis PA ΔmucR or ΔmucRΔhdeA did not show higher susceptibility than the parental strain to oxidative, acid, or hypersaline stresses in the conditions assayed (Table 3, Supplementary Table S1). Additionally, B. ovis PA ΔmucR did not show the increased susceptibility to iron restriction (Table 3) reported for mutants of the three other Brucella species (20, 31, 32) despite the downregulation observed by the RT-qPCR of genes ftrA and bfr that are related to iron homeostasis (Figure 5). Susceptibility to detergents and the cationic peptide polymyxin B, which is related to properties of the bacterial cell envelope (5, 19), has been evaluated in the Brucella spp. ΔmucR mutants, except that of B. canis. While susceptibility to detergents is shared by the Brucella spp. ΔmucR mutants (20, 21) (Table 3), deletion of mucR increases the susceptibility of B. melitensis 16M and B. ovis PA (21) (Table 3), but not that of B. abortus 2308 (20) to polymyxin B. Considering the influence of MucR in the modulation of the bacterial surface architecture (clearly exemplified in this work by the increased levels of Omp25d in the B. ovis PA ΔmucR mutant), the differences observed among the Brucella spp. ΔmucR mutants might be related, at least in part, to the distinctive OM-related properties reported for each Brucella species (5, 19) and contribute to the differences of pathogenicity, host preference and tissue tropism that exist within the genus Brucella. Some of these distinctive properties seem to be associated to the smooth or rough phenotype, but some others seem to be dependent on differences in other cell envelope components (19). In this respect, a distinctive pattern for each Brucella species regarding members of the Omp25/Omp31 family, at least Omp25d being controlled by MucR (Figure 1), has been described (5, 18).
Despite the differences detailed above, all Brucella spp. ΔmucR mutants shared the virulence attenuation in cellular models and mice as a common trait (20–22, 32) (Figure 6). Although the B. ovis PA ΔmucR and ΔmucRΔhdeA mutants have important in vitro growth defects (Figure 2), which would be a drawback for their industrial production, the persistent infection that they establish in mice (Figure 6) is an interesting trait that makes them potential candidates to be evaluated as specific attenuated vaccines against ovine brucellosis caused by B. ovis. The good protective activity against B. melitensis infection described for B. melitensis 16M ΔmucR (44) also encourages further evaluation of the B. ovis PA mutant as vaccine.
Data Availability Statement
The raw data supporting the conclusions of this article will be made available by the authors, without undue reservation.
Ethics Statement
The animal study was reviewed and approved by Bioethics Committee of the University of Salamanca and competent authority of Junta de Castilla y León, Spain.
Author Contributions
RS-M and NV conceived the study. BT-C and NV wrote the manuscript, and all authors participated in the experimental work, the discussion of the results, and the revision of the manuscript. All authors read and approved the final version of the manuscript.
Funding
This work is part of projects AGL2014-58795-C4-4-R, co-financed with FEDER funds, and PID2019-107601RB-C33, financed by MCIN/AEI/10.13039/501100011033. Financial support to BT-C was provided by project PID2019-107601RB-C33, financed by MCIN/AEI/10.13039/501100011033. The projects were awarded by Ministerio de Ciencia e Innovación, Spain.
Conflict of Interest
The authors declare that the research was conducted in the absence of any commercial or financial relationships that could be construed as a potential conflict of interest.
Publisher's Note
All claims expressed in this article are solely those of the authors and do not necessarily represent those of their affiliated organizations, or those of the publisher, the editors and the reviewers. Any product that may be evaluated in this article, or claim that may be made by its manufacturer, is not guaranteed or endorsed by the publisher.
Acknowledgments
We thank the staff of the DNA sequencing and animal experimentation facilities of the University of Salamanca for their efficient collaboration.
Supplementary Material
The Supplementary Material for this article can be found online at: https://www.frontiersin.org/articles/10.3389/fvets.2021.814752/full#supplementary-material
References
1. Whatmore AM, Foster JT. Emerging diversity and ongoing expansion of the genus Brucella. Infect Genet Evol. (2021) 92:104865. doi: 10.1016/j.meegid.2021.104865
2. Moreno E. The one hundred year journey of the genus Brucella (Meyer and Shaw 1920). FEMS Microbiol Rev. (2021) 45:1–22. doi: 10.1093/femsre/fuaa045
3. Verger JM, Grimont F, Grimont PA, Grayon M. Brucella, a monospecific genus as shown by deoxyribonucleic-acid hybridization. Int J Syst Bacteriol. (1985) 35:292–5. doi: 10.1099/00207713-35-3-292
4. OIE. Chapter 3.1.4. Brucellosis (infection with B. abortus, B. melitensis and B. suis). Manual of Diagnostic Tests and Vaccines for Terrestrial Animals. (2021). Available online at: https://www.oie.int/fileadmin/Home/eng/Health_standards/tahm/3.01.04_BRUCELLOSIS.pdf.
5. Vizcaíno N, Cloeckaert A. Biology and genetics of the Brucella outer membrane. In: López-Goñi I, O'Callaghan D, editors. Brucella Molecular Microbiology and Genomics. Norfolk, UK: Caister Academic Press (2012). p. 133–61.
6. Stranahan LW, Arenas-Gamboa AM. When the going gets rough: the significance of Brucella lipopolysaccharide phenotype in host-pathogen interactions. Front Microbiol. (2021) 12:713157. doi: 10.3389/fmicb.2021.713157
7. Burgess GW. Ovine contagious epididymitis: a review. Vet Microbiol. (1982) 7:551–75. doi: 10.1016/0378-1135(82)90049-9
8. OIE. Chapter 3.8.7. Ovine epididymitis (Brucella ovis). Manual of Diagnostic Tests and Vaccines for Terrestrial Animals. (2021). Available online at: https://www.oie.int/fileadmin/Home/eng/Health_standards/tahm/3.08.07._OVINE_EPID.pdf.
9. Blasco JM, Marín CM, Barberán M, Moriyón I, Díaz R. Immunization with Brucella melitensis Rev 1 against Brucella ovis infection of rams. Vet Microbiol. (1987) 14:381–92. doi: 10.1016/0378-1135(87)90029-0
10. Picard-Hagen N, Berthelot X, Champion JL, Eon L, Lyazrhi F, Marois M, et al. Contagious epididymitis due to Brucella ovis: relationship between sexual function, serology and bacterial shedding in semen. BMC Vet Res. (2015) 11:125. doi: 10.1186/s12917-015-0440-7
11. Martín-Martín AI, Sancho P, de Miguel MJ, Fernández-Lago L, Vizcaíno N. Quorum-sensing and BvrR/BvrS regulation, the type IV secretion system, cyclic glucans, and BacA in the virulence of Brucella ovis: similarities to and differences from smooth brucellae. Infect Immun. (2012) 80:1783–93. doi: 10.1128/IAI.06257-11
12. Vizcaíno N, Pérez-Etayo L, Conde-Álvarez R, Iriarte M, Moriyón I, Zúñiga-Ripa A. Disruption of pyruvate phosphate dikinase in Brucella ovis PA CO[[sb]]2[[/s]]-dependent and independent strains generates attenuation in the mouse model. Vet Res. (2020) 51:101. doi: 10.1186/s13567-020-00824-7
13. Soler-Llorens P, Gil-Ramirez Y, Zabalza-Barangua A, Iriarte M, Conde-Alvarez R, Zuniga-Ripa A, et al. Mutants in the lipopolysaccharide of Brucella ovis are attenuated and protect against B. ovis infection in mice. Vet Res. (2014) 45:72. doi: 10.1186/s13567-014-0072-0
14. Sidhu-Muñoz RS, Sancho P, Vizcaíno N. Brucella ovis PA mutants for outer membrane proteins Omp10, Omp19, SP41, and BepC are not altered in their virulence and outer membrane properties. Vet Microbiol. (2016) 186:59–66. doi: 10.1016/j.vetmic.2016.02.010
15. Sidhu-Muñoz RS, Tejedor C, Vizcaíno N. The three flagellar loci of Brucella ovis PA are dispensable for virulence in cellular models and mice. Front Vet Sci. (2020) 7:441. doi: 10.3389/fvets.2020.00441
16. Silva TM, Paixão TA, Costa EA, Xavier MN, Sá JC, Moustacas VS, et al. Putative ATP-binding cassette transporter is essential for Brucella ovis pathogenesis in mice. Infect Immun. (2011) 79:1706–17. doi: 10.1128/IAI.01109-10
17. Bowden RA, Cloeckaert A, Zygmunt MS, Bernard S, Dubray G. Surface exposure of outer membrane protein and lipopolysaccharide epitopes in Brucella species studied by enzyme-linked immunosorbent assay and flow cytometry. Infect Immun. (1995) 63:3945–52. doi: 10.1128/iai.63.10.3945-3952.1995
18. Martín-Martín AI, Caro-Hernández P, Sancho P, Tejedor C, Cloeckaert A, Fernández-Lago L, et al. Analysis of the occurrence and distribution of the Omp25/Omp31 family of surface proteins in the six classical Brucella species. Vet Microbiol. (2009) 137:74–82. doi: 10.1016/j.vetmic.2008.12.003
19. Martín-Martín AI, Sancho P, Tejedor C, Fernández-Lago L, Vizcaíno N. Differences in the outer membrane-related properties of the six classical Brucella species. Vet J. (2011) 189:103–5. doi: 10.1016/j.tvjl.2010.05.021
20. Caswell CC, Elhassanny AE, Planchin EE, Roux CM, Weeks-Gorospe JN, Ficht TA, et al. Diverse genetic regulon of the virulence-associated transcriptional regulator MucR in Brucella abortus 2308. Infect Immun. (2013) 81:1040–51. doi: 10.1128/IAI.01097-12
21. Mirabella A, Terwagne M, Zygmunt MS, Cloeckaert A, De Bolle X, Letesson JJ. Brucella melitensis MucR, an orthologue of Sinorhizobium meliloti MucR, is involved in resistance to oxidative, detergent, and saline stresses and cell envelope modifications. J Bacteriol. (2013) 195:453–65. doi: 10.1128/JB.01336-12
22. Wu Q, Pei J, Turse C, Ficht TA. Mariner mutagenesis of Brucella melitensis reveals genes with previously uncharacterized roles in virulence and survival. BMC Microbiol. (2006) 6:102. doi: 10.1186/1471-2180-6-102
23. Valderas MW, Alcantara RB, Baumgartner JE, Bellaire BH, Robertson GT, Ng WL, et al. Role of HdeA in acid resistance and virulence in Brucella abortus 2308. Vet Microbiol. (2005) 107:307–12. doi: 10.1016/j.vetmic.2005.01.018
24. Kovach ME, Elzer PH, Hill DS, Robertson GT, Farris MA, Roop RM II, et al. Four new derivatives of the broad-host-range cloning vector pBBR1MCS, carrying different antibiotic-resistance cassettes. Gene. (1995) 166:175–6. doi: 10.1016/0378-1119(95)00584-1
25. Sidhu-Muñoz RS, Sancho P, Cloeckaert A, Zygmunt MS, de Miguel MJ, Tejedor C, et al. Characterization of cell envelope multiple mutants of Brucella ovis and assessment in mice of their vaccine potential. Frontiers Microbiol. (2018) 9:2230. doi: 10.3389/fmicb.2018.02230
26. Caro-Hernández P, Fernández-Lago L, de Miguel MJ, Martín-Martín AI, Cloeckaert A, Grilló MJ, et al. Role of the Omp25/Omp31 family in outer membrane properties and virulence of Brucella ovis. Infect Immun. (2007) 75:4050–61. doi: 10.1128/IAI.00486-07
27. Elhassanny AE, Anderson ES, Menscher EA, Roop RM II. The ferrous iron transporter FtrABCD is required for the virulence of Brucella abortus 2308 in mice. Mol Microbiol. (2013) 88:1070–82. doi: 10.1111/mmi.12242
28. Vizcaíno N, Kittelberger R, Cloeckaert A, Marín CM, Fernández-Lago L. Minor nucleotide substitutions in the omp31 gene of Brucella ovis result in antigenic differences in the major outer membrane protein that it encodes compared to those of the other Brucella species. Infect Immun. (2001) 69:7020–8. doi: 10.1128/IAI.69.11.7020-7028.2001
29. Sancho P, Tejedor C, Sidhu-Muñoz RS, Fernández-Lago L, Vizcaíno N. Evaluation in mice of Brucella ovis attenuated mutants for use as live vaccines against B. ovis infection. Vet Res. (2014) 45:61. doi: 10.1186/1297-9716-45-61
30. Lacerda TL, Salcedo SP, Gorvel JP. Brucella T4SS: the VIP pass inside host cells. Curr Opin Microbiol. (2013) 16:45–51. doi: 10.1016/j.mib.2012.11.005
31. Dong H, Liu W, Peng X, Jing Z, Wu Q. The effects of MucR on expression of type IV secretion system, quorum sensing system and stress responses in Brucella melitensis. Vet Microbiol. (2013) 166:535–42. doi: 10.1016/j.vetmic.2013.06.023
32. Sun J, Dong H, Peng X, Liu Y, Jiang H, Feng Y, et al. Deletion of the transcriptional regulator MucR in Brucella canis affects stress responses and bacterial virulence. Front Vet Sci. (2021) 8:650942. doi: 10.3389/fvets.2021.650942
33. Borriello G, Russo V, Paradiso R, Riccardi MG, Criscuolo D, Verde G, et al. Different Impacts of MucR Binding to the babR and virB Promoters on Gene Expression in Brucella abortus 2308. Biomolecules. (2020) 10:788. doi: 10.3390/biom10050788
34. Rambow-Larsen AA, Rajashekara G, Petersen E, Splitter G. Putative quorum-sensing regulator BlxR of Brucella melitensis regulates virulence factors including the type IV secretion system and flagella. J Bacteriol. (2008) 190:3274–82. doi: 10.1128/JB.01915-07
35. Uzureau S, Lemaire J, Delaive E, Dieu M, Gaigneaux A, Raes M, et al. Global analysis of quorum sensing targets in the intracellular pathogen Brucella melitensis 16 M. J Proteome Res. (2010) 9:3200–17. doi: 10.1021/pr100068p
36. Delrue RM, Deschamps C, Leonard S, Nijskens C, Danese I, Schaus JM, et al. A quorum-sensing regulator controls expression of both the type IV secretion system and the flagellar apparatus of Brucella melitensis. Cell Microbiol. (2005) 7:1151–61. doi: 10.1111/j.1462-5822.2005.00543.x
37. Liu Y, Sun J, Peng X, Dong H, Qin Y, Shen Q, et al. Deletion of the LuxR-type regulator VjbR in Brucella canis affects expression of type IV secretion system and bacterial virulence, and the mutant strain confers protection against Brucella canis challenge in mice. Microb Pathog. (2020) 139:103865. doi: 10.1016/j.micpath.2019.103865
38. Gajiwala KS, Burley SK, HDEA. a periplasmic protein that supports acid resistance in pathogenic enteric bacteria. J Mol Biol. (2000) 295:605–12. doi: 10.1006/jmbi.1999.3347
39. Hong W, Jiao W, Hu J, Zhang J, Liu C, Fu X, et al. Periplasmic protein HdeA exhibits chaperone-like activity exclusively within stomach pH range by transforming into disordered conformation. J Biol Chem. (2005) 280:27029–34. doi: 10.1074/jbc.M503934200
40. Gorvel JP, Moreno E, Moriyón I. Is Brucella an enteric pathogen? Nat Rev Microbiol. (2009) 7:250. doi: 10.1038/nrmicro2012-c1
41. Fretin D, Fauconnier A, Köhler S, Halling S, Léonard S, Nijskens C, et al. The sheathed flagellum of Brucella melitensis is involved in persistence in a murine model of infection. Cell Microbiol. (2005) 7:687–98. doi: 10.1111/j.1462-5822.2005.00502.x
42. Léonard S, Ferooz J, Haine V, Danese I, Fretin D, Tibor A, et al. FtcR is a new master regulator of the flagellar system of Brucella melitensis 16M with homologs in Rhizobiaceae. J Bacteriol. (2007) 189:131–41. doi: 10.1128/JB.00712-06
43. Rouot B, Alvarez-Martinez MT, Marius C, Menanteau P, Guilloteau L, Boigegrain RA, et al. Production of the type IV secretion system differs among Brucella species as revealed with VirB5- and VirB8-specific antisera. Infect Immun. (2003) 71:1075–82. doi: 10.1128/IAI.71.3.1075-1082.2003
Keywords: Brucella ovis, MucR, HdeA, virulence, Omp25d, transcriptional regulation, acid stress, deletion mutant
Citation: Tartilán-Choya B, Sidhu-Muñoz RS and Vizcaíno N (2022) The Transcriptional Regulator MucR, but Not Its Controlled Acid-Activated Chaperone HdeA, Is Essential for Virulence and Modulates Surface Architecture and Properties in Brucella ovis PA. Front. Vet. Sci. 8:814752. doi: 10.3389/fvets.2021.814752
Received: 14 November 2021; Accepted: 16 December 2021;
Published: 31 January 2022.
Edited by:
Changyong Cheng, Zhejiang A&F University, ChinaReviewed by:
Hao Dong, China Animal Disease Control Center (CADC), ChinaClayton Caswell, Virginia Tech, United States
Copyright © 2022 Tartilán-Choya, Sidhu-Muñoz and Vizcaíno. This is an open-access article distributed under the terms of the Creative Commons Attribution License (CC BY). The use, distribution or reproduction in other forums is permitted, provided the original author(s) and the copyright owner(s) are credited and that the original publication in this journal is cited, in accordance with accepted academic practice. No use, distribution or reproduction is permitted which does not comply with these terms.
*Correspondence: Nieves Vizcaíno, vizcaino@usal.es
†Present address: Rebeca S. Sidhu-Muñoz, Group of Translational Research on Renal and Cardiovascular Diseases, Salamanca, Spain