- 1URZ, INRAE, Domaine Duclos Prise d'eau, Petit-Bourg, France
- 2Departamento de Mejora Genética Animal, Instituto Nacional de Investigación y Tecnología Agraria y Alimentaria, INIA-CSIC, Madrid, Spain
- 3GenPhySE, Université de Toulouse, INRAE, INP, Castanet Tolosan, France
Heat stress (HS) affects pig performance, health and welfare, resulting in a financial burden to the pig industry. Pigs have a limited number of functional sweat glands and their thermoregulatory mechanisms used to maintain body temperature, are challenged by HS to maintain body temperature. The genetic selection of genotypes tolerant to HS is a promising long-term (adaptation) option that could be combined with other measures at the production system level. This review summarizes the current knowledge on the genetics of thermoregulation in pigs. It also discusses the different phenotypes that can be used in genetic studies, as well as the variability in thermoregulation between pig breeds and the inheritance of traits related to thermoregulation. This review also considers on-going challenges to face for improving heat tolerance in pigs.
Introduction
Non-ruminants (pigs and poultry) represent the majority of meat consumed in the world (1). Pork production is expected to increase, despite the need to change production practices due to finite natural resources (2) and the need to decrease meat consumption [particularly beef and dairy cattle products (3)] to reduce GHG emissions (4, 5) (In Europe, the objective of the European Commission is−55% GHG by 2030 compared to 1990). Heat stress (HS) impacts pig performance, health and welfare (6), resulting in a financial burden to the pig industry (7). For instance, in the USA, HS has been estimated to cost from $300 (8) to $900 (9) million annually, depending on the year and method of estimation. Climate change, with concomitant changes in the frequency and magnitude of ambient temperatures and precipitation, may accentuate animal health and welfare problems (6). Furthermore, there is growing evidence (10) that genetic selection has reduced pigs' ability to cope with HS, due to an increase of metabolic heat production with the improvement in reproductive traits and lean tissue growth rate (11, 12) at the expense of adaptive capacities (13). Unlike ruminants, pigs have a limited number of functional sweat glands to facilitate heat loss by evaporation, so their thermoregulatory mechanisms are challenged by HS when trying to maintain body temperature (14). There is a great amount of research that proposes adaptation solutions aimed at reducing the negative effects of climate change on livestock production (3, 15, 16). Several solutions are already available and implemented, such as altering the environmental (cooling options) or feeding management (changes in diet composition and/or distribution) (7). However, some of these adaptation strategies could come at a high cost, both financially and environmentally. The genetic selection of genotypes tolerant to HS is a promising long-term (adaptation) option that could be combined with other measures at the production system level (16, 17). Furthermore, in the framework of agroecology (18, 19), selection must be considered in relation to the food system and the value chain. This implies that we should no longer try to isolate animals from the fluctuations of the environment of production, but rather to favor their capacity to produce and to reproduce in less controlled environments, including in HS conditions (20). The focus of this review is to discuss our current knowledge of the genetics of thermoregulation in pigs. Deepening our understanding of the genetic variability of thermoregulation in pigs and how it can be used to select animals with better heat tolerance is essential to develop strategies to mitigate the negative effects of climate change on pig production.
The Phenome of Thermoregulation in Pigs
Thermoregulation in Pigs
Pigs are homeothermic animals as they can keep deep body temperature relatively constant, within narrow limits, despite a wide variation of the surrounding climatic environment. Thermoregulation is the physiological process allowing the balance between heat production and heat loss mechanisms (21). We assume that from an animal production point of view, physiological HS can be defined as the magnitude of environmental and metabolic loads for which the animal cannot dissipate an adequate quantity of heat to maintain homeostasis with minimal performance losses (22). Figure 1 schematically illustrates the relationship between ambient temperature, heat production and heat loss. Illustrative values of critical and rectal temperatures are also given, based on available data from the literature in lactating sows (23) and in growing pigs (10). These critical temperatures vary greatly, depending on numerous factors such as breed, body weight and composition, diet management, group size, and temperature by humidity interactions (24). Pigs can lose heat by conduction, convection and radiation (sensible heat loss), and by evaporation (latent heat loss). In the thermoneutral zone (from temperatures C to D, Figure 1), pig metabolism (and heat production) is relatively constant. When the ambient temperature increases above D, sensible heat transfer becomes ineffective due to the reduction of the temperature gradient between skin and ambient air. Pigs then rely mainly on evaporative heat loss by increasing respiratory rate to maintain a constant body temperature (25). The ability of the pig to dissipate heat is actually a combination of the effect of ambient temperature and humidity, that can be captured by the temperature humidity index (THI) (26–28). Most studies (27–29) designed to characterize the effect of heat load from the environment on livestock responses have summarized the climatic factors in a THI index. The levels of panting, metabolism, and body core temperature differ between physiological stages and animals. Animals in stages of high metabolic activities (lactation, growth) are typically more susceptible, as well as animals with a low surface/area body weight ratio. Furthermore, there are short-term responses to HS [acclimation or acclimatization (21)] that differ from long-term ones (adaptation). In addition HS varies in duration (short periods of HS, heat waves of few days' duration or chronic HS) and magnitude (moderate, high, or extreme) (30). The improvement of thermoregulation in pigs by genetic selection assumes that there is a genetic component of traits associated with thermoregulation. Hence, if thermoregulation is heritable, animals, breeds, or lines with higher C, D, E, or F values could be selected, resulting in an increased tolerance to HS (31).
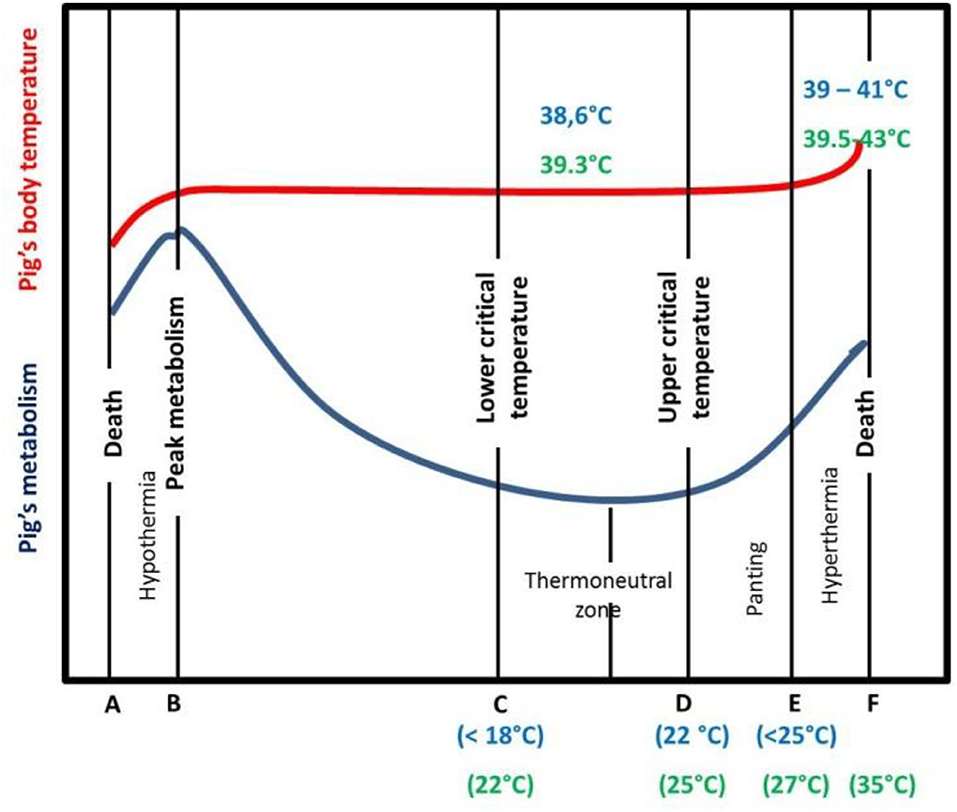
Figure 1. Diagrammatic presentation of the effect of ambient temperature on lactating sow (indicative values in blue) and growing pig (indicative values in green) metabolism and body temperature [adapted from (32)]. The lower critical temperature is the ambient temperature below which pigs must increase heat production to maintain heat balance. The upper critical temperature is the ambient temperature above which pigs must increase heat loss rate to achieve heat balance.
How to Phenotype Thermoregulation in Genetic Studies?
The regulation of body temperature is a complex physiological process involving regulation from the cell to the whole animal, therefore, the phenome of thermoregulation encompasses a large variety of physical and biochemical traits (21). The starting point of genetic studies to perform selection for more heat tolerant animals is to define heritable phenotypes associated with resistance or susceptibility to heat stress. One of the aims of such studies would be to obtain relevant genetic markers or genes for a better genomic evaluation of heat tolerance. So far, the main criterion considered in breeding programs is the maintenance of production performance under HS (33). Other traits directly related to thermoregulation should be considered as potential selection criteria. The main challenges with phenotyping trait indicators of thermoregulation capacities in genetic studies are to accurately define phenotypes. Desirable phenotypes should (i) be biologically relevant, (ii) be technically easy to measure routinely in genetic evaluations schemes at low financial and energetic cost levels, and (iii) fall within the following framework: more non-invasive phenotypic parameters to monitor is desirable, for animal welfare and for the representativeness of the measured phenotypes to the physiological reality of the animal.
As reviewed by Renaudeau et al. (32), several physiological traits (macro-phenotypes and biomarkers) that are directly or indirectly related to heat production, heat loss, and body core temperature can be measured. Rectal temperature is an indicator of body core temperature, which is the result of the whole thermoregulation process. Skin temperatures measured at several sites are indicators of sensible heat loss. More precisely, with rectal, skin and ambient temperatures or THI, a thermal circulation index can be calculated as an indicator of blood flow to the skin to promote sensible heat loss (34). Respiratory rate is an indicator of latent heat dissipation. Several blood metabolites and hormones associated with the HS response have been proposed as potential biomarkers for HS (32, 35, 36). The main biomolecules associated with the HS response in pigs that have been reported in the literature are summarized in Table 1. These studies were largely conducted using commercial breeds, that were more sensitive to HS. The hypothalamic–pituitary–adrenocortical (HPA) axis is one of the most important stress-responsive neuroendocrine systems. The activation of the HPA axis leads to the production of cortisol which is released into circulation and represents one of the principal stress hormones in livestock species (36, 37). Plasma or serum is mostly used to measure cortisol levels (37). However, there is a growing tendency to use cortisol level from saliva (38, 39), which is a non-invasive measure and correlates well with serum levels (40). In pigs, salivary cortisol increases with HS and shows a circadian pattern (39). Thyroid hormones T3 and T4 play a vital role in regulating thermogenesis and are also identified as indicators of the response to HS in livestock species (41, 42). High temperature reduces the levels of plasma thyroid hormones and the T3:T4 ratio (i.e., conversion rate of T4–T3) in pigs (43, 44), suggesting reduced metabolic rate. Another important metabolic regulator and indicator of HS is the amount of circulating non-esterified fatty acids (NEFA). Several studies showed decreased plasma or serum NEFA levels in pigs under HS, suggesting reduced adipose tissue mobilization (44–46). Moreover, heat-stressed animals, despite having lower feed intake, exhibit higher insulin levels. This paradox may be explained by the insulin's role in activating heat shock proteins (HSP) (47). The increase in circulating insulin is correlated with HSP70 expression (45) and both insulin and HSP90 response are required for successful adaptation to HS (43).
The hormonal, cellular and molecular response to HS is therefore complex and is still being unraveled. Moreover, little data is available on the heritability of these biomarkers and their correlation with other HS phenotypes and with production traits, making it difficult to postulate on the most relevant biomarkers to include in genetic studies. Because HS reduces feed intake, it can be difficult to determine whether these responses are the consequences of direct effects of HS, or of indirect effects linked to the reduced feed intake (44, 45, 48, 49). Moreover, most of these measures are obtained from blood sampling methods that are costly in production conditions and that are invasive to animal welfare. Nevertheless, understanding the metabolic and cellular response to HS is essential to eventually find proxies or alternative measurements (such as salivary measures) for these parameters that can be used in genetic studies.
Finally, the variation in traits of economic interest in response to the heat load, such as reproduction (e.g., fertility, prolificity) and production traits (e.g., growth rate, feed intake, and feed efficiency) are indicators of resilience to HS (50, 51). These traits could also be useful to identify the most interesting traits which highlight the biological response to heat. For instance, feed intake is likely to respond faster than body weight to HS, as reducing intake reduces metabolic heat production. Consequently, analysis of feeding behaviors could be a non-invasive and easy-to-measure proxy for body core temperature, as feeding behavior patterns have been shown to change significantly with temperature (52). Under HS, pigs spend less time eating and reduce meal size and duration (53, 54) and feeding rate (52), probably as a way to reduce heat production by decreasing physical and metabolic activity (55). It is important to emphasize that the quantification of criteria to characterize thermoregulation should be done in relation with the fluctuations of the climatic conditions to capture the animal's response to heat load. Several statistical models have been developed to quantify trait changes due to HS: as a slope (56) or as coefficients associated to the broken lines of the curve (22, 57), or as indicators of trait variability (50). For instance, in lactating sows, who are particularly sensitive to heat stress, due to their high metabolic heat production for milk production (58), longitudinal measures of traits associated with thermoregulation during lactation or from gestation to weaning provide more accurate information than a single measure to characterize the best moment for phenotyping both within a day and during the gestation and lactation periods. Carabaño et al. (57) and Gourdine et al. (59) reported that the most discriminating daily period (i.e., maximum range) for thermoregulatory variation is between 04:00 to 07:00 h and 19:00 to 23:00 h, in relation with the hourly feed intake and the circadian rhythm of body core temperature. At the lactation scale, other studies (60) have suggested that the dynamics of rectal temperature was directly related to the kinetic metabolic heat production related to energy and protein intake and milk synthesis. Furthermore, from a meta-analysis (23), a curvilinear relation was found between the increase in average rectal temperature of lactating sows and ambient temperature, with an increase in rectal temperature of 0.07°C per degree of ambient temperature.
Genetic and Genomic Considerations for Thermoregulation in Pigs
Is There Variability for Thermoregulation Traits Between Breed or Line?
Genetic variation between breeds in response to HS have been reported in several species such as cattle (61, 62), poultry (63), and pigs (64–66). To the best of our knowledge, little has been published on the differences in heat tolerance between tropical and temperate pig breeds, despite most of pig breeds being from tropical and subtropical areas (67). In these areas, microevolution has promoted the emergence of breeds with a high ability to cope with HS but these breeds remain poorly characterized (68). The physiological adaptation to HS of tropical breeds could be partly explained by a lower metabolic heat production due to a lower productive potential of tropical breeds than commercial breeds, but also by a higher ability of some breeds to dissipate heat than other breeds, probably related to favorable alleles. Figures 2–4 illustrate the variability of thermoregulatory responses to HS between a tropical local Creole breed (local tropical breed) and a Large White breed (European commercial breed) in lactating sows. Within the same production environment, high average THI during lactation caused greater increase of rectal temperature (Figure 2), skin temperature (Figure 3) and respiratory rate (Figure 4) in Large White than in Creole sows, suggesting better physiological adaptation of Creole sows to HS. Moreover, higher variation within Creole sows than within Large White sows illustrates the high variability of an unselected breed compared to a selected one. In growing pigs, studies comparing the same breeds (Large White vs. Creole) have shown that the effect of HS on thermoregulatory responses were higher in Large White than Creole pigs, whether under chronic HS, such as seasonal effects in indoors (54) or outdoors (69), or under short-term HS (70), showing the existence of breeds with higher critical temperatures values (e.g., in the tropical conditions of Guadeloupe, the upper critical THI for Large White sow is around 24.5°C and the corresponding values for Creole sow is >24.5°C) (59). To our knowledge, there is no data in the literature about the hormonal and metabolic response of tropical breeds to HS, and it is therefore difficult to assess how the potential biomarkers mentioned above predict how the physiological mechanisms have evolved under HS in these breeds. However, several studies showed that cortisol levels are highly genetically variable, even in highly selected pig breeds (71, 72). It would be of particular interest to characterize neuro-endocrine (cortisol), metabolic (thyroid hormones, NEFA) and cellular and molecular (HSP proteins) responses to HS in heat tolerant breeds to evaluate the relevance of these potential biomarkers for selection of heat tolerant animals.
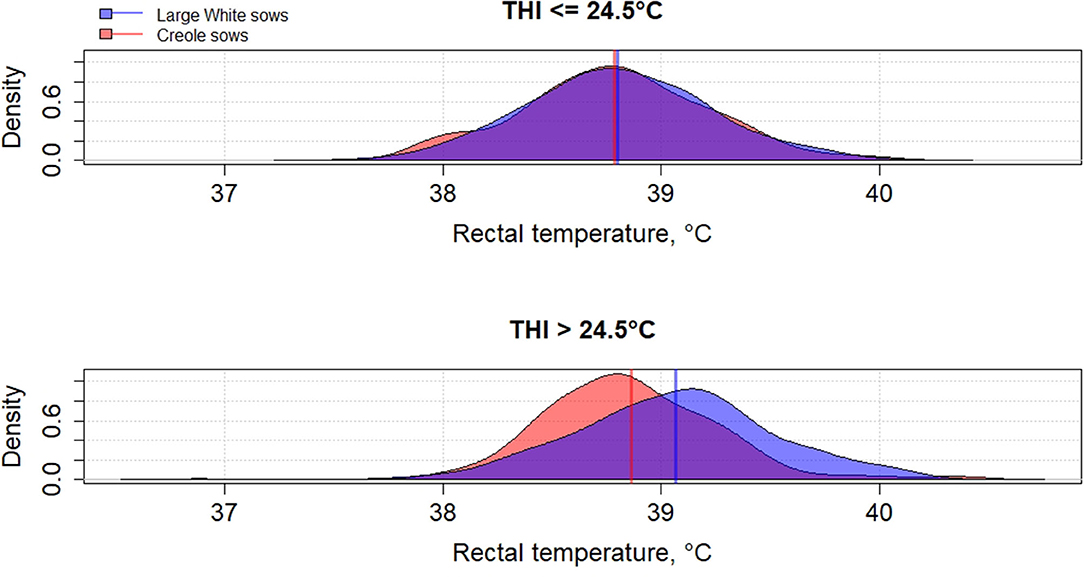
Figure 2. Density distribution of average rectal temperature of Creole and Large White lactating sows according to the average thermal-humidity index (THI) during lactation [adapted from Gourdine et al. (59)].
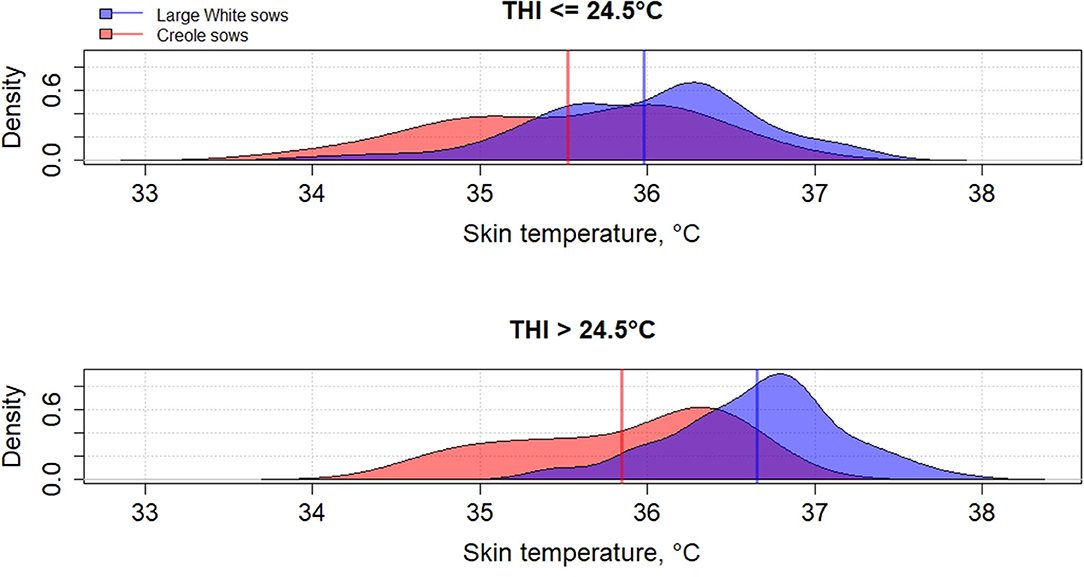
Figure 3. Density distribution of average skin temperature of Creole and Large White lactating sows according to the average thermal-humidity index (THI) during lactation [adapted from Gourdine et al. (59)].
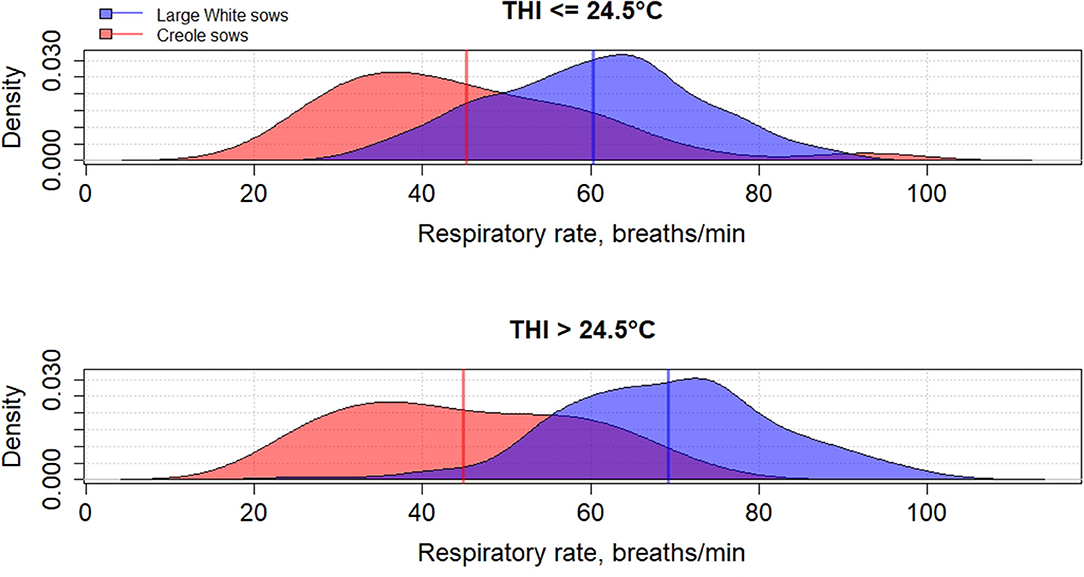
Figure 4. Density distribution of average respiratory rate of Creole and Large White lactating sows according to the average thermal-humidity index (THI) during lactation [adapted from Gourdine et al. (59)].
When comparing feeding behavior of Creole and Large White pigs under HS, both breeds had similar daily feed intake but different feeding behavior patterns, with fewer but larger meals for the Creole associated with a lower feeding rate (54). Differences in feeding behavior in response to HS have been observed in other breeds (73) and support the idea of using feeding behavior as a proxy for thermoregulation. In this context, the genetic variation responsible for the natural thermotolerance of tropical local pig breeds could be used for genetic improvement for heat tolerance of international commercial pig breeds. To our knowledge, only a few studies have dealt with this topic (50), despite the widespread use of crossbreeding schemes in the pig industry. Crossbreeding with well-heat-adapted but less productive breeds (which partly explain their better tolerance to HS) might be financially less profitable in many contexts such as large intensive operations, due to the payment grid and production costs. Indeed, crossbred pigs with tropical local pig genetics would grow slower and fatter with heterogeneous groups of pigs to manage (74), which often means penalties from the abattoir. However, when and if knowledge allows it, introgression of favorable alleles to HS from tropical pig breeds into commercial pig lines could be a promising technique to improve heat tolerance.
Are Thermoregulation Traits Heritable?
In contrast to production traits, the inheritance of traits associated to thermoregulation has been poorly described in the literature. In species such as cattle (75–77), rectal temperature is the main thermoregulation trait for which heritability was accurately estimated (Table 2). In pigs, estimation of genetic parameters of thermoregulation traits rarely exist. For instance, to our best knowledge, few studies have estimated heritabilities of rectal temperature in piglets (78), in growing pigs (79) and in sows (22, 80), essentially pointing out low to moderate genetic bases for body and skin temperatures. However, thermoregulation is a physiological homeostatic process, meaning that the steady state of the internal temperature is maintained. That raises the question of which trait should be genetically improved for a better thermoregulation. Genetic improvement of body core temperature should not target the average body core temperature (for instance increasing the mean from 38.7 to 39.7°C), but rather focus on reducing its variance, with the aim to decrease the number of heat-stressed pigs. At the animal level, that corresponds to animals with a better regulation of body temperature during heat stress.
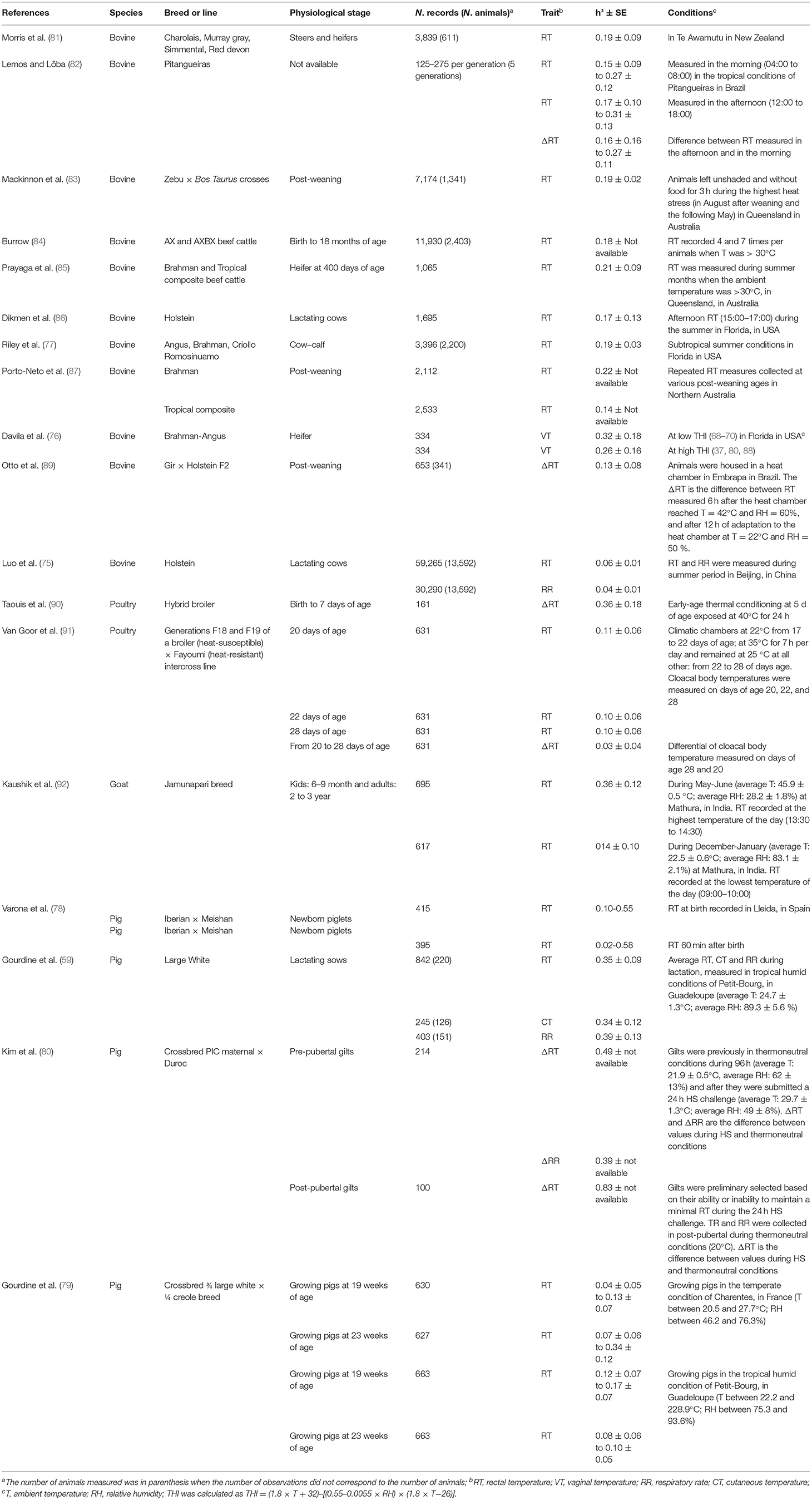
Table 2. Heritabilities (h2 ± SE) of body temperature and respiratory rate in different livestock species.
Similarly to thermoregulation traits, little data is available on the genetic parameters of potential biomarkers for HS in pigs. In pigs, cortisol levels were found to be highly heritable (h2 = 0.68) (93) and in dairy cows, NEFA was found to be moderately heritable [0.08–0.35 (94, 95)]. To our knowledge, no studies have investigated the genetic correlations between these metabolic markers and performance traits in pigs. However, higher cortisol has been shown to have positive effects on traits related to robustness and adaptation, while having negative effects on production traits (growth rate, fat/lean ratio) (37). Foury et al. (72) found that cortisol and carcass lean content were phenotypically negatively correlated (−0.46), so that ~21% of variation in fatness across breeds could be explained by variation in cortisol. These results need to be confirmed by genetic studies but suggest that it might be possible to select for cortisol levels allowing better heat tolerance without compromising production traits.
Using feeding behavior as a proxy for thermoregulation, a pangenomic study has been performed (73) to detect genomic variants associated with changes in feeding behavior under HS. The authors found that heritabilities for differences in feeding activity were low to moderate, suggesting that heat tolerance is heritable, and suggested some candidates genes, such as DPYSL2 and ADRA1A, and biological pathways (e.g., immune function) to explain the detected associations.
As reviewed by Renaudeau et al. (24), there is a moderate to strong negative phenotypic correlation between rectal temperature and production traits in many species, suggesting that thermoregulation and production traits may be partly governed by separate genomic loci. However, estimates of the association between these traits often had large standard errors due to the limited number of animals in most experimental designs and they depend on the conditions of recording (i.e., if HS occurs or not, and how HS occurs). In acute HS (e.g., 24 h of severe HS of 29.7°C), some studies have shown little or no association between HS and sow feed intake or changes in body weight (80). Like other complex traits, large numbers of genes are probably involved in thermoregulation, and the genetic correlations with other economically important traits should be quantified to investigate possible antagonisms or favorable relationships that should be accounted for in a selection index to improve heat tolerance. If loci involved in variation of production and thermoregulation traits are in linkage disequilibrium, it can be expected that favorable alleles for production traits are associated with unfavorable alleles for thermoregulation, and trade-offs will have to be found between production and regulation capacity during HS. Few studies provide “omics” information for thermoregulation in pigs. In cattle (89, 96) and in poultry (97, 98), the slick hair gene and the naked neck gene, respectively, are used by introgression or crossbreeding to improve the thermotolerance of breeds. QTL have been found associated to body temperature in the Japanese quail (98), in poultry (99), and in cattle (91). To the best of our knowledge, quantitative trait loci (QTL) associated with thermoregulation traits in response to HS were only reported in the studies of Kim et al. (80) in gilts and Riquet et al. (100) in growing pigs. These studies, either with genome-wide association analysis or linkage analysis, have detected a small number of loci (<100) with very small effect on the variability of thermoregulation traits. Increasing the sample size of the pig population to be measured and to be genotyped is therefore necessary, to detect a larger number of SNPs of interest and to decrease the confidence interval of the SNPs' position (80). The sequencing technologies show great improvement and it should be possible in the near future to directly incorporate the causal polymorphisms of the variability of thermoregulation traits instead of genomic markers (33). However, our current knowledge about the genomic variability of thermoregulation traits in pig is limited, and further research is needed.
Challenges to Face
As pointed out by several authors, the analysis of high-throughput phenotypic and genomic data to address issues related to HS, and more generally to health and animal welfare, requires the development of new methods and technologies capable of integrating diverse, heterogeneous, and large-scale data. In this context, the advent of new technologies, omic tools (101, 102) (including genomic, epigenomic, transcriptomic, metabolomic, microbiome information, and genome editing), but also Artificial Intelligence (AI) approaches (such as deep learning, machine learning, etc.) offer new and very promising avenues for analyses to address HS complex problems.
To the best of our knowledge, there is no pig breeding program including traits associated with thermoregulation. Nevertheless, it is likely that international pig breeding companies take advantage of their presence in contrasting areas, from the Northern Europe to South America, to select pigs according to the environment of production, based on the breeding values of production and reproduction traits. Implementation of traits directly associated with thermoregulation in a conventional pig breeding program is not straightforward, firstly due to the difficulty of defining heat tolerance directly in terms of measurable traits (50), secondly due to the difficulty to measure these appropriate phenotypes routinely and technically easily under HS conditions, and thirdly as far we know due to the lack of quantification of economic weights of traits associated to thermoregulation. Furthermore, the choice of breeding approaches will have to deal with the biological antagonism between production traits and thermoregulation traits (24), i.e., probably leading to reduced genetic gains on the current breeding objectives. Finally, substantial differences can be observed between pig's performance in production environments and that in selection environment, which is referred to as genotype by environment interactions (G × E) (103). Therefore, breeding programs should also consider these interactions as they can be a source of inefficiency (104, 105) to transfer the genetic progress to production farms. For instance, the best pigs for a criterion assessed in a temperate environment would not necessary be the best for the same criterion assessed in tropical conditions. To our knowledge, there are very few studies reporting G × E interactions in relation to thermoregulation or HS in pigs (65, 79), compared to available studies in broiler chickens (63), dairy cattle (62), or beef cattle (106, 107), but they show substantial G × E interactions for some production traits. This may be due to the lack of known genetic relationships between animals reared under contrasted climatic conditions and also due to the absence of a significant amount of data to infer the existence and level of G × E interactions.
In summary, the success of selection for improved response to HS may depend on interrelated factors: (i) the extent of G × E interactions; (ii) the level of antagonisms between genes involved in thermoregulation and production biological pathways, (iii) the definition of a heat tolerance index, and (iv) the ability to collect phenotypic and genomic information on a large scale in the appropriate environmental conditions.
There is no doubt that genomic innovations and precision selection (cisgenesis, transgenesis, genome editing) will be mobilized for future genetic studies on the thermoregulation of pigs (33). These studies cannot be disconnected from the complex and systemic issues related to global change and its impacts on pig production (such as the availability of crops for pig feeding, the emergence of new disease or pathogen vectors in certain regions) (108), the ethical issues related to the use of new breeding techniques, and societal questions on animal welfare and research priorities (33).
Author Contributions
J-LG, WR, HG, and NP assisted in the conception of the study and contributed to manuscript revision, read, and approved the submitted version. All authors contributed to the article and approved the submitted version.
Conflict of Interest
The authors declare that the research was conducted in the absence of any commercial or financial relationships that could be construed as a potential conflict of interest.
Publisher's Note
All claims expressed in this article are solely those of the authors and do not necessarily represent those of their affiliated organizations, or those of the publisher, the editors and the reviewers. Any product that may be evaluated in this article, or claim that may be made by its manufacturer, is not guaranteed or endorsed by the publisher.
References
1. Robinson TP, Thornton PK, Franceschini G, Kruska. Global Livestock Production Systems. Rome: Food and Agriculture Organization of the United Nations (FAO) and International Livestock Research Institute (ILRI) (2011).
2. FAO. World Livestock: Transforming the Livestock Sector Through the Sustainable Development Goals. Rome: FAO (2018).
3. Rojas-Downing MM, Nejadhashemi AP, Harrigan T, Woznicki SA. Climate change and livestock: impacts, adaptation, and mitigation. Clim Risk Manag. (2017) 16:145–63. doi: 10.1016/j.crm.2017.02.001
4. Poore J, Nemecek T. Reducing food's environmental impacts through producers and consumers. Science. (2018) 360:987–92. doi: 10.1126/science.aaq0216
5. European Commission. Communication from the Commission to the European Parliament, the Council, The European Economic and social Committee and the Committee of the Regions. Stepping up Europe's 2030 Climate Ambition. Investing in a Climate-Neutral Future for the Benefit of Our People. Brussels (2020). Available online at: https://eur-lex.europa.eu/legal-content/EN/TXT/PDF/?uri=CELEX:52020DC0562&from=EN
6. Lacetera N. Impact of climate change on animal health and welfare. Anim Front. (2019) 9:26–31. doi: 10.1093/af/vfy030
7. Mayorga EJ, Renaudeau D, Ramirez BC, Ross JW, Baumgard LH. Heat stress adaptations in pigs. Anim Front. (2019) 9:54–61. doi: 10.1093/af/vfy035
8. St-Pierre NR, Cobanov B, Schnitkey G. Economic losses from heat stress by US livestock industries. J Dairy Sci. (2003) 86:E52–77. doi: 10.3168/jds.S0022-0302(03)74040-5
9. Schieck, S,. Heat Stress in Swine Affects Production. Available online at: https://extension.umn.edu/swine-production-management/heat-stress-swine-affects-production
10. Renaudeau D, Gourdine JL, St-Pierre NR. A meta-analysis of the effects of high ambient temperature on growth performance of growing-finishing pigs. J Anim Sci. (2011) 89:2220–30. doi: 10.2527/jas.2010-3329
11. Costa FG, Silva JH, Lima RC, Oliveira CF, Rodrigues VP, Pinheiro SG. Scientific progress in the production of monogastric in the first decade of the twenty-first century. Rev Bras Zootec. (2010) 39:288–302. doi: 10.1590/S1516-35982010001300032
12. Merks JWM, Mathur PK, Knol EF. New phenotypes for new breeding goals in pigs. Animal. (2012) 6:535–43. doi: 10.1017/S1751731111002266
13. van der Waaij E. A resource allocation model describing consequences of artificial selection under metabolic stress. J Anim Sci. (2004) 82:973–81. doi: 10.2527/2004.824973x
14. Renaudeau D, Leclercq-Smekens M, Herin M. Differences in skin characteristics in European (large white) and Caribbean (Creole) growing pigs with reference to thermoregulation. Anim Res. (2006) 55:209–17. doi: 10.1051/animres:2006012
15. O'Brien D, Herron J, Andurand J, Care S, Martinez P, Migliorati L, et al. Life beef carbon: a common framework for quantifying grass and corn based beef farms' carbon footprints. Animal. (2020) 14:834–45. doi: 10.1017/S1751731119002519
16. Grossi G, Goglio P, Vitali A, Williams AG. Livestock and climate change: impact of livestock on climate and mitigation strategies. Anim Front. (2019) 9:69–76. doi: 10.1093/af/vfy034
17. Bernabucci U. Climate change: impact on livestock and how can we adapt. Anim Front. (2019) 9:3–5. doi: 10.1093/af/vfy039
18. Altieri MA, Nicholls C I. Agroecology: challenges and opportunities for farming in the anthropocene. Int J Agric Nat Resour. (2020) 47:204–15. doi: 10.7764/ijanr.v47i3.2281
19. Franzluebbers AJ, Wendroth O, Creamer NG, Feng GG. Focusing the future of farming on agroecology. Agric Environ Lett. (2020) 5:1–6. doi: 10.1002/ael2.20034
20. Phocas F, Belloc C, Bidanel J, Delaby L, Dourmad JY, Dumont B, et al. Review: Towards the agroecological management of ruminants, pigs and poultry through the development of sustainable breeding programmes: I-selection goals and criteria. Animal. (2016) 10:1749–59. doi: 10.1017/S1751731116000926
21. Collier RJ, Baumgard LH, Zimbelman RB, Xiao Y. Heat stress: physiology of acclimation and adaptation. Anim Front. (2019) 9:12–9. doi: 10.1093/af/vfy031
22. Gourdine J-L, Mandonnet N, Giorgi M, Renaudeau D. Genetic parameters for thermoregulation and production traits in lactating sows reared in tropical climate. Animal. (2017) 11:365–74. doi: 10.1017/S175173111600135X
23. Dourmad JY, Velly V, Lechartier C, Gourdine JL, Renaudeau D. Effect of ambient temperature on lactating sows, a meta-analysis and modeling approach. J Rech Porc En Fr. (2015) 47:105–10.
24. Renaudeau D, Mandonnet N, Tixier-Boichard M, Noblet J, Bidanel J. Attenuate the effects of high ambient temperature on pig performance: the genetic selection. Prod Anim. (2004) 17:93–108. doi: 10.20870/productions-animales.2004.17.1.3556
25. Huynh TTT, Aarnink AJA, Heetkamp MJW, Verstegen MWA, Kemp B. Evaporative heat loss from group-housed growing pigs at high ambient temperatures. J Therm Biol. (2007) 32:293–9. doi: 10.1016/j.jtherbio.2007.03.001
26. Xin H, Harmon JD. Livestock Industry Facilities and Environment: Heat Stress Indices for Livestock. Agriculture and Environment Extension Publications (1998). Available online at: http://lib.dr.iastate.edu/extension_ag_pubs/163 (accessed Apri 26, 2021).
27. Lallo C, Cohen J, Rankine D, Taylor M, Cambell J, Stephenson T. Characterizing heat stress on livestock using the temperature humidity index (THI)prospects for a warmer Caribbean. Reg Environ Change. (2018) 18:2329–40. doi: 10.1007/s10113-018-1359-x
28. Mutua J, Marshall K, Paul B, Notenbaert A. A methodology for mapping current and future heat stress risk in pigs. Animal. (2020) 14:1952–60. doi: 10.1017/S1751731120000865
29. Bohmanova J, Misztal I, Cole JB. Temperature-humidity indices as indicators of milk production losses due to heat stress. J Dairy Sci. (2007) 90:1947–56. doi: 10.3168/jds.2006-513
30. Thornton P, Nelson G, Mayberry D, Herrero M. Increases in extreme heat stress in domesticated livestock species during the twenty-first century. Glob Change Biol. (2021) 27:5762–72. doi: 10.1111/gcb.15825
31. Kanis E, van den Belt H, Groen A, Schakel J, de Greef K. Breeding for improved welfare in pigs: a conceptual framework and its use in practice. Anim Sci. (2004) 78:315–29. doi: 10.1017/S1357729800054102
32. Renaudeau D, Collin A, Yahav S, de Basilio V, Gourdine JL, Collier RJ. Adaptation to hot climate and strategies to alleviate heat stress in livestock production. Animal. (2012) 6:707–28. doi: 10.1017/S1751731111002448
33. Le Roy P, Ducos A, Phocas F. What performance for tomorrow's animals? INRA Prod Anim. (2019) 32:233–46. doi: 10.20870/productions-animales.2019.32.2.2466
34. Renaudeau D, Kerdoncuff M, Anais C, Gourdine JL. Effect of temperature level on thermal acclimation in large white growing pigs. Animal. (2008) 2:1619–26. doi: 10.1017/S1751731108002814
35. Dou S, Villa-Vialaneix N, Liaubet L, Billon Y, Giorgi M, Gilbert H, et al. (NMR)-N-1H-Based metabolomic profiling method to develop plasma biomarkers for sensitivity to chronic heat stress in growing pigs. PLoS ONE. (2017) 12:e0188469. doi: 10.1371/journal.pone.0188469
36. Sejian V, Bhatta R, Gaughan J, Dunshea F, Lacetera N. Review: adaptation of animals to heat stress. Animal. (2018) 12:S431–44. doi: 10.1017/S1751731118001945
37. Mormède P, Foury A, Terenina E, Knap PW. Breeding for robustness: the role of cortisol. Animal. (2011) 5:651–7. doi: 10.1017/S1751731110002168
38. Ruis M, Brake J, Engel B, Ekkel E, Buist W, Blokhuis H, et al. The circadian rhythm of salivary cortisol in growing pigs: effects of age, gender, and stress. Physiol Behav. (1997) 62:623–30. doi: 10.1016/S0031-9384(97)00177-7
39. Hillmann E, Schrader L, Mayer C, Gygax L. Effects of weight, temperature and behaviour on the circadian rhythm of salivary cortisol in growing pigs. Animal. (2008) 2:405–9. doi: 10.1017/S1751731107001279
40. Cook N, Schaefer A, Lepage P, Jones S. Salivary vs serum cortisol for the assessment of adrenal activity in swine. Can J Anim Sci. (1996) 76:329–35. doi: 10.4141/cjas96-049
41. Todini L. Thyroid hormones in small ruminants: effects of endogenous environmental and nutritional factors. Animal. (2007) 1:997–1008. doi: 10.1017/S1751731107000262
42. Silva J. Thermogenic mechanisms and their hormonal regulation. Physiol Rev. (2006) 86:435–64. doi: 10.1152/physrev.00009.2005
43. Fernandez M, Stoakes S, Abuajamieh M, Seibert J, Johnson J, Horst E, et al. Heat stress increases insulin sensitivity in pigs. Physiol Rep. (2015) 3e12478. doi: 10.14814/phy2.12478
44. Serviento A, Labussiere E, Castex M, Renaudeau D. Effect of heat stress and feeding management on growth performance and physiological responses of finishing pigs. J Anim Sci. (2020) 98:skaa387. doi: 10.1093/jas/skaa387
45. Pearce S, Gabler N, Ross J, Escobar J, Patience J, Rhoads R, et al. The effects of heat stress and plane of nutrition on metabolism in growing pigs. J Anim Sci. (2013) 91:2108–18. doi: 10.2527/jas.2012-5738
46. Fernandez M, Johnson J, Abuajamieh M, Stoakes S, Seibert J, Cox L, et al. Effects of heat stress on carbohydrate and lipid metabolism in growing pigs. Physiol Rep. (2015) 3:e12315. doi: 10.14814/phy2.12315
47. Li G, Ali I, Currie R. Insulin induces myocardial protection and Hsp70 localization to plasma membranes in rat hearts. Am J Physiol Heart Circ Physiol. (2006) 291:H1709–21. doi: 10.1152/ajpheart.00201.2006
48. Cervantes M, Cota M, Arce N, Castillo G, Avelar E, Espinoza S, et al. Effect of heat stress on performance and expression of selected amino acid and glucose transporters, HSP90, leptin and ghrelin in growing pigs. J Therm Biol. (2016) 59:69–76. doi: 10.1016/j.jtherbio.2016.04.014
49. Morales A, Grageola F, Garcia H, Arce N, Araiza B, Yanez J, et al. Performance, serum amino acid concentrations and expression of selected genes in pair-fed growing pigs exposed to high ambient temperatures. J Anim Physiol Anim Nutr. (2014) 98:928–35. doi: 10.1111/jpn.12161
50. Misztal I. Breeding and genetics symposium: resilience and lessons from studies in genetics of heat stress. J Anim Sci. (2017) 95:1780–7. doi: 10.2527/jas2016.0953
51. Berghof TVL, Poppe M, Mulder HA. Opportunities to improve resilience in animal breeding programs. Front Genet. (2019) 9:692. doi: 10.3389/fgene.2018.00692
52. Santos LSD, Pomar C, Campos PHRF, da Silva WC, Gobi JDP, Veira AM, et al. Precision feeding strategy for growing pigs under heat stress conditions. J Anim Sci. (2018) 96:4789–801. doi: 10.1093/jas/sky343
53. Quiniou N, Dubois S, Noblet J. Voluntary feed intake and feeding behaviour of group-housed growing pigs are affected by ambient temperature and body weight. Livest Prod Sci. (2000) 63:245–53. doi: 10.1016/S0301-6226(99)00135-9
54. Renaudeau D, Giorgi M, Silou F, Weisbecker J. Effect of breed (lean or fat pigs) and sex on performance and feeding behaviour of group housed growing pigs in a tropical climate. Asian Australas J Anim Sci. (2006) 19:593–600. doi: 10.5713/ajas.2006.593
55. Nienaber J, Hahn G, McDonald T, Korthals R. Feeding patterns and swine performance in hot environments. Trans ASAE. (1996) 39:195–202. doi: 10.13031/2013.27498
56. Bernabucci U, Biffani S, Buggiotti L, Vitali A, Lacetera N, Nardone A. The effects of heat stress in Italian Holstein dairy cattle. J Dairy Sci. (2014) 97:471–86. doi: 10.3168/jds.2013-6611
57. Carabaño MJ, Pineda-Quiroga C, Ugarte E, Diaz C, Ramon M. Genetic basis of thermotolerance in 2 local dairy sheep populations in the Iberian Peninsula. J Dairy Sci. (2021) 104:5755–67. doi: 10.3168/jds.2020-19503
58. Black J, Mullan B, Lorschy M, Giles L. Lactation in the sow during heat-stress. Livest Prod Sci. (1993) 35:153–70. doi: 10.1016/0301-6226(93)90188-N
59. Gourdine JL, Bidanel JP, Noblet J, Renaudeau D. Rectal temperature of lactating sows in a tropical humid climate according to breed, parity and season. Asian Australas J Anim Sci. (2007) 20:832–41. doi: 10.5713/ajas.2007.832
60. Williams AM, Safranski TJ, Spiers DE, Eichen PA, Coate EA, Lucy MC. Effects of a controlled heat stress during late gestation, lactation, and after weaning on thermoregulation, metabolism, and reproduction of primiparous sows. J Anim Sci. (2013) 91:2700–14. doi: 10.2527/jas.2012-6055
61. Burrow H, Prayaga K. Correlated responses in productive and adaptive traits and temperament following selection for growth and heat resistance in tropical beef cattle. Livest Prod Sci. (2004) 86:143–61. doi: 10.1016/j.livprodsci.2003.06.001
62. Hayes BJ, Bowman PJ, Chamberlain AJ, Savin K, van Tassell CP, Sonstegard TS, et al. A validated genome wide association study to breed cattle adapted to an environment altered by climate change. PLoS ONE. (2009) 4:e6676. doi: 10.1371/journal.pone.0006676
63. Mignon-Grasteau S, Moreri U, Narcy A, Rousseau X, Rodenburg TB, Tixier-Boichard M, et al. Robustness to chronic heat stress in laying hens: a meta-analysis. Poult Sci. (2015) 94:586–600. doi: 10.3382/ps/pev028
64. Bloemhof S, van der Waaij EH, Merks JWM, Knol EF. Sow line differences in heat stress tolerance expressed in reproductive performance traits. J Anim Sci. (2008) 86:3330–7. doi: 10.2527/jas.2008-0862
65. Bloemhof S, Mathur PK, Knol EF, van der Waaij EH. Effect of daily environmental temperature on farrowing rate and total born in dam line sows. J Anim Sci. (2013) 91:2667–79. doi: 10.2527/jas.2012-5902
66. Tiezzi F, Brito LF, Howard J, Huang YJ, Gray K, Schwab C, et al. Genomics of heat tolerance in reproductive performance investigated in four independent maternal lines of pigs. Front Genet. (2020) 11:629. doi: 10.3389/fgene.2020.00629
67. FAO. Status and Trends of Animal Genetic Resources-−2018 Intergovernmental Technical Working Group on Animal Genetic Resources for Food and Agriculture. Rome: FAO (2018).
68. Hoffmann I. Climate change and the characterization, breeding and conservation of animal genetic resources. Anim Genet. (2010) 41:32–46. doi: 10.1111/j.1365-2052.2010.02043.x
69. Gourdine J-L, Bambou JC, Giorgi M, Loranger-Merciris G, Archimede H. Performance of growing pigs reared indoors or outdoors in sweet-potato fields. Rev Elev Med Vet Pays Trop. (2018) 71:41–6. doi: 10.19182/remvt.31347
70. Renaudeau D. Effects of short-term exposure to high ambient temperature and relative humidity on thermoregulatory responses of European (large white) and caribbean (creole) restrictively-fed growing pigs. Anim Res. (2005) 54:81–93. doi: 10.1051/animres:2005005
71. Désautés C, Sarrieau A, Caritez JC, Mormède P. Behavior and pituitary-adrenal function in large white and Meishan pigs. Domest Anim Endocrinol. (1999) 16:193–205. doi: 10.1016/S0739-7240(99)00014-4
72. Foury A, Geverink NA, Gil M, Gispert M, Hortos M, Furnols MF, et al. Stress neuroendocrine profiles in five pig breeding lines and the relationship with carcass composition. Animal. (2007) 1:973–82. doi: 10.1017/S1751731107000249
73. Cross AJ, Keel BN, Brown-Brandl TM, Cassady JP, Rohrer GA. Genome-wide association of changes in swine feeding behaviour due to heat stress. Genet Sel Evol. (2018) 50:11. doi: 10.1186/s12711-018-0382-1
74. Rose R, Gilbert H, Loyau T, Giorgi M, Billon Y, Riquet J, et al. Interactions between sire family and production environment (temperate vs. tropical) on performance and thermoregulation responses in growing pigs. J Anim Sci. (2017) 95:4738–51. doi: 10.2527/jas2017.1611
75. Luo H, Brito LF, Li X, Su G, Dou J, Xu W, et al. Genetic parameters for rectal temperature, respiration rate, and drooling score in holstein cattle and their relationships with various fertility, production, body conformation, and health traits. J Dairy Sci. (2021) 104:4390–403. doi: 10.3168/jds.2020-19192
76. Davila KMS, Hamblen H, Hansen PJ, Dikmen S, Oltenacu PA, Mateescu RG. Genetic parameters for hair characteristics and core body temperature in a multibreed brahman-angus herd. J Anim Sci. (2019) 97:3246–52. doi: 10.1093/jas/skz188
77. Riley DG, Chase CC Jr, Coleman SW, Olson TA. Genetic assessment of rectal temperature and coat score in Brahman, Angus, and Romosinuano crossbred and straightbred cows and calves under subtropical summer conditions. Livest Sci. (2012) 148:109–18. doi: 10.1016/j.livsci.2012.05.017
78. Varona L, Casellas J, Piedrafita J, Sanchez A, Garcia-Casado P, Arque M, et al. Bayes factor analysis for the genetic background of physiological and vitality variables of F-2 iberian x meishan newborn piglets. J Anim Sci. (2005) 83:334–9. doi: 10.2527/2005.832334x
79. Gourdine J-L, Riquet J, Rose R, Poullet N, Giorgi M, Billon Y, et al. Genotype by environment interactions for performance and thermoregulation responses in growing pigs. J Anim Sci. (2019) 97:3699–713. doi: 10.1093/jas/skz245
80. Kim K-S, Seibert JT, Edea Z, Graves KL, Kim E-S, Keating AF, et al. Characterization of the acute heat stress response in gilts: III. Genome-wide association studies of thermotolerance traits in pigs. J Anim Sci. (2018) 96:2074–85. doi: 10.1093/jas/sky131
81. Morris C, Jones K, Wilson J. Heritability of rectal temperature and relationships with growth in young cattle in a temperate climate. N Z J Agric Res. (1989) 32:375–8. doi: 10.1080/00288233.1989.10421755
82. Lemos A, Lobo R. Effects of environment and heredity on the rectal temperature of pitangueiras cattle. Rev Bras Genet. (1990) 13:777–88.
83. Mackinnon M, Meyer K, Hetzel D. Genetic-variation and covariation for growth, parasite resistance and heat tolerance in tropical cattle. Livest Prod Sci. (1991) 27:105–22. doi: 10.1016/0301-6226(91)90090-D
84. Burrow H. Variances and covariances between productive and adaptive traits and temperament in a composite breed of tropical beef cattle. Livest Prod Sci. (2001) 70:213–33. doi: 10.1016/S0301-6226(01)00178-6
85. Prayaga K, Corbet N, Johnston D, Wolcott M, Fordyce G, Burrow H. Genetics of adaptive traits in heifers and their relationship to growth, pubertal and carcass traits in two tropical beef cattle genotypes. Anim Prod Sci. (2009) 49:413–25. doi: 10.1071/EA08247
86. Dikmen S, Cole J, Null D, Hansen P. Heritability of rectal temperature and genetic correlations with production and reproduction traits in dairy cattle. J Dairy Sci. (2012) 95:3401–5. doi: 10.3168/jds.2011-4306
87. Porto-Neto L, Reverter A, Prayaga K, Chan E, Johnston D, Hawken R, et al. The genetic architecture of climatic adaptation of tropical cattle. PLoS ONE. (2014) 9:e0113284. doi: 10.1371/journal.pone.0113284
88. Hansen P. Prospects for gene introgression or gene editing as a strategy for reduction of the impact of heat stress on production and reproduction in cattle. Theriogenology. (2020) 154:190–202. doi: 10.1016/j.theriogenology.2020.05.010
89. Otto P, Guimaraes S, Verardo L, Azevedo A, Vandenplas J, Sevillano C, et al. Genome-wide association studies for heat stress response in Bos taurus x Bos indicus crossbred cattle. J DAIRY Sci. (2019) 102:8148–58. doi: 10.3168/jds.2018-15305
90. Taouis M, Basilio V, Grasteau S, Crochet S, Bouchot C, Bigot K, et al. Early-age thermal conditioning reduces uncoupling protein messenger RNA expression in pectoral muscle of broiler chicks at seven days of age. Poult Sci. (2002) 81:1640–3. doi: 10.1093/ps/81.11.1640
91. Van Goor A, Bolek K, Ashwell C, Persia M, Rothschild M, Schmidt C, et al. Identification of quantitative trait loci for body temperature, body weight, breast yield, and digestibility in an advanced intercross line of chickens under heat stress. Genet Sel Evol. (2015) 47:96. doi: 10.1186/s12711-015-0176-7
92. Kaushik R, Goel A, Rout PK. Establishing the genetic variation in physiological response in response to heat stress in semi-arid region in jamunapari goats. Biol Rhythm Res. (2020) 51:1–14. doi: 10.1080/09291016.2018.1499218
93. Larzul C, Terenina E, Foury A, Billon Y, Louveau I, Merlot E, et al. The cortisol response to ACTH in pigs, heritability and influence of corticosteroid-binding globulin. Animal. (2015) 9:1929–34. doi: 10.1017/S1751731115001767
94. Oikonomou G, Valergakis GE, Arsenos G, Roubies N, Banos G. Genetic profile of body energy and blood metabolic traits across lactation in primiparous holstein cows. J Dairy Sci. (2008) 91:2814–22. doi: 10.3168/jds.2007-0965
95. Benedet A, Costa A, De Marchi M, Penasa M. Heritability estimates of predicted blood β-hydroxybutyrate and nonesterified fatty acids and relationships with milk traits in early-lactation holstein cows. J Dairy Sci. (2020) 103:6354–63. doi: 10.3168/jds.2019-17916
96. Ortiz-Colon G, Fain S, Pares I, Curbelo-Rodriguez J, Jimenez-Caban E, Pagan-Morales M, et al. Assessing climate vulnerabilities and adaptive strategies for resilient beef and dairy operations in the tropics. Clim Change. (2018) 146:47–58. doi: 10.1007/s10584-017-2110-1
97. Galal A, Radwan L, Rezik H, Ayoub H. Expression levels of HSP70 and CPT-1 in three local breeds of chickens reared under normal or heat stress conditions after the introduction of the naked neck gene. J Therm Biol. (2019) 80:113–8. doi: 10.1016/j.jtherbio.2018.12.018
98. Nawab A, Ibtisham F, Li G, Kieser B, Wu J, Liu W, et al. Heat stress in poultry production: mitigation strategies to overcome the future challenges facing the global poultry industry. J Therm Biol. (2018) 78:131–9. doi: 10.1016/j.jtherbio.2018.08.010
99. Minvielle F, Kayang B, Inoue-Murayama M, Miwa M, Vignal A, Gourichon D, et al. Microsatellite mapping of QTL affecting growth, feed consumption, egg production, tonic immobility and body temperature of Japanese quail. BMC Genomics. (2005) 6:87. doi: 10.1186/1471-2164-6-87
100. Riquet J, Feve K, Labrune Y, Rose R, Billon Y, Giorgi M, et al. Genetic Dissection of Mechanisms Underlying Heat Adaptation in Pigs. In: 36th Conference of the International Society for Animal Genetics (ISAG). Dublin (2017).
101. Dikmen S, Cole JB, Null DJ, Hansen PJ. Genome-Wide association mapping for identification of quantitative trait loci for rectal temperature during heat stress in holstein cattle. PLoS ONE. (2013) 8:e69202. doi: 10.1371/journal.pone.0069202
102. Clark E, Archibald A, Daetwyler H, Groenen M, Harrison P, Houston R, et al. From FAANG to fork: application of highly annotated genomes to improve farmed animal production. Genome Biol. (2020) 21:285. doi: 10.1186/s13059-020-02197-8
103. Wientjes YCJ, Calus MPL. Board invited review: the purebred-crossbred correlation in pigs: a review of theory, estimates, and implications1. J Anim Sci. (2017) 95:3467–78. doi: 10.2527/jas.2017.1669
104. Rauw WM, Gomez-Raya L. Genotype by environment interaction and breeding for robustness in livestock. Front Genet. (2015) 6:310. doi: 10.3389/fgene.2015.00310
105. Knap P, Su G. Genotype by environment interaction for litter size in pigs as quantified by reaction norms analysis. Animal. (2008) 2:1742–7. doi: 10.1017/S1751731108003145
106. Cardoso F, Tempelman R. Linear reaction norm models for genetic merit prediction of angus cattle under genotype by environment interaction. J Anim Sci. (2012) 90:2130–41. doi: 10.2527/jas.2011-4333
107. Georges M, Charlier C, Hayes B. Harnessing genomic information for livestock improvement. Nat Rev Genet. (2019) 20:135–56. doi: 10.1038/s41576-018-0082-2
Keywords: thermoregulation, pig, heat stress, genetics, selection
Citation: Gourdine J-L, Rauw WM, Gilbert H and Poullet N (2021) The Genetics of Thermoregulation in Pigs: A Review. Front. Vet. Sci. 8:770480. doi: 10.3389/fvets.2021.770480
Received: 03 September 2021; Accepted: 19 November 2021;
Published: 13 December 2021.
Edited by:
Angela Maree Lees, University of New England, AustraliaReviewed by:
Yachun Wang, China Agricultural University, ChinaJohn Gaughan, The University of Queensland, Australia
Copyright © 2021 Gourdine, Rauw, Gilbert and Poullet. This is an open-access article distributed under the terms of the Creative Commons Attribution License (CC BY). The use, distribution or reproduction in other forums is permitted, provided the original author(s) and the copyright owner(s) are credited and that the original publication in this journal is cited, in accordance with accepted academic practice. No use, distribution or reproduction is permitted which does not comply with these terms.
*Correspondence: Jean-Luc Gourdine, amVhbi1sdWMuZ291cmRpbmVAaW5yYWUuZnI=