- 1Lethbridge Research and Development Centre, Agriculture and Agri-Food Canada, Lethbridge, AB, Canada
- 2Feedlot Health Management Services, Okotoks, AB, Canada
- 3Veterinary Agri-Health Systems, Airdrie, AB, Canada
- 4Alberta Beef Health Solutions, Picture Butte, AB, Canada
- 5Faculty of Veterinary Medicine, University of Calgary, Calgary, AB, Canada
- 6Department of Mathematics and Statistics, University of Calgary, Calgary, AB, Canada
- 7Western College of Veterinary Medicine, University of Saskatchewan, Saskatoon, SK, Canada
- 8Public Health Agency of Canada, Saskatoon, SK, Canada
- 9Coaldale Veterinary Clinic Ltd., Lethbridge, AB, Canada
- 10POV Inc., Airdrie, AB, Canada
A broad, cross-sectional study of beef cattle at entry into Canadian feedlots investigated the prevalence and epidemiology of antimicrobial resistance (AMR) in Mannheimia haemolytica, Pasteurella multocida, Histophilus somni, and Mycoplasma bovis, bacterial members of the bovine respiratory disease (BRD) complex. Upon feedlot arrival and before antimicrobials were administered at the feedlot, deep nasopharyngeal swabs were collected from 2,824 feedlot cattle in southern and central Alberta, Canada. Data on the date of feedlot arrival, cattle type (beef, dairy), sex (heifer, bull, steer), weight (kg), age class (calf, yearling), source (ranch direct, auction barn, backgrounding operations), risk of developing BRD (high, low), and weather conditions at arrival (temperature, precipitation, and estimated wind speed) were obtained. Mannheimia haemolytica, P. multocida, and H. somni isolates with multidrug-resistant (MDR) profiles associated with the presence of integrative and conjugative elements were isolated more often from dairy-type than from beef-type cattle. Our results showed that beef-type cattle from backgrounding operations presented higher odds of AMR bacteria as compared to auction-derived calves. Oxytetracycline resistance was the most frequently observed resistance across all Pasteurellaceae species and cattle types. Mycoplasma bovis exhibited high macrolide minimum inhibitory concentrations in both cattle types. Whether these MDR isolates establish and persist within the feedlot environment, requires further evaluation.
Introduction
Bovine respiratory disease (BRD) continues to be a challenging and costly disease in feedlot cattle in North America (1–3), accounting for 70–80% and 40–50% of the total herd-level morbidity and mortality, respectively (4). Moreover, it has been estimated that BRD costs the North American feedlot cattle industry over $3 billion annually (5). Mannheimia haemolytica, Pasteurella multocida, Histophilus somni, and Mycoplasma bovis are considered the main bacterial species associated with BRD (6). However, viruses, host, environment, and management practices also play important roles in this multi-factorial, complex disease (7). Considerable resources have been invested in the development of technologies and management strategies to mitigate and treat BRD, but the incidence of morbidity and mortality have remained relatively constant over the last 45 years (8). Practices such as preconditioning, improved diagnostics, and new vaccines continue to be developed, investigated, and validated as alternatives to antimicrobials. However, until these alternatives are shown to be cost-effective, practically implementable, and on-par or surpass currently available practices, it is likely that antimicrobial therapy will continue to be an important tool for preventing, treating, and controlling BRD in feedlot cattle.
Previous studies have suggested that antimicrobial resistance (AMR) amongst BRD-related bacteria has been increasing in North America (9–11). However, these studies mostly collected samples from clinical BRD cases. A previous Alberta study found that 30% of M. haemolytica and 12.5% of P. multocida isolated from BRD cattle mortalities were resistant to macrolides, tetracyclines (TETs), β-lactams, fluoroquinolones (FQs), lincosamides, aminoglycosides (AMGs), and pleuromutilins (12). Resistance to macrolides is of particular concern due to the importance of these drugs in controlling BRD in high risk incoming cattle (13). Unlike clinical BRD cases, studies that targeted beef cattle at feedlot arrival, prior to antimicrobial administration found that M. haemolytica and P. multocida were susceptible to fluoroquinolones, macrolides, β-lactams, and cephalosporins (14–16). This suggests that AMR increases after feedlot placement. However, previous published studies that assessed AMR prior to administration of antimicrobials at arrival focused on M. haemolytica and not all four members of the BRD bacterial complex (14, 15).
Development of AMR threatens access and efficacy of antimicrobials, has the potential to increase antimicrobial use (AMU) (5), threaten animal health and welfare (17), increase production costs (18), and promote dissemination of antimicrobial-resistance genes (ARG) in cattle and the environment (19). Passive AMR surveillance can help to identify emerging AMR trends (20). However, for a global and non-biased vision of current AMR patterns, epidemiologically robust studies are required. The Canadian beef industry in partnership with CIPARS (Canadian Integrated Program for Antimicrobial Resistance Surveillance) has recently developed a national feedlot AMU/AMR surveillance program targeting selected respiratory nasal bacterial pathogens and enteric bacteria (21). To complement the surveillance program, this study was designed to estimate the current prevalence and describe AMR in M. haemolytica, P. multocida, H. somni, and M. bovis, and to provide insight into the epidemiology and possible risk factors for AMR prior to cattle arriving at the feedlot.
Materials and Methods
Ethics Statement and General Cattle Management
The animal study was reviewed and approved by the Lethbridge Research Centre Animal Care and Use Committee (Protocol #1641, Jan 18th, 2017) and was conducted according to the Canadian Council of Animal Care Guidelines (22).
Sampling
From August 2017 to May 2018 and from August 2018 to April 2019 (19 months), deep nasopharyngeal swabs (DNPS) were collected from feedlot cattle at processing at feedlot arrival, prior to the administration of antimicrobials. Cattle were housed in ten different commercial feedlots located across southern and central Alberta purposely selected based on their one-time holding capacity, the use of an electronic record keeping system, and willingness to collaborate in the study. Each feedlot (designated A-J) was managed by one of four veterinary practices providing services to ~80% of Alberta's 1.5 million feedlot cattle. Feedlots were selected based on their one-time holding capacity, the use of an electronic animal health record-keeping system, and their willingness to participate. Six feedlots had a one-time capacity >10,000 head; whereas four housed <10,000 head. For sampling, transport truck was considered the sampling unit. When beef-type cattle from different locations were transported in the same truck, location was also considered to define the sampling unit. For sample size calculation, the isolation percentage of BRD bacteria resistant to at least one antimicrobial was expected to by 15% based on previous pilot studies carried out in the same geographical area (JVD, personal communication). Sample size at animal level was calculated before the participating feedlots were assigned. Assuming n = pq/L2; α = 0.05 (Zα = 1.96); p = 0.15; and with a precision of L = 0.05, a total of 200 head were initially targeted per feedlot per year. A total of 10 cattle from each of the 10 participating feedlots were targeted for sampling every 2 weeks for 40 weeks (September to May) over two consecutive years. Animals were selected using cluster sampling, with cattle within a transport truck constituting a cluster. During off-loading, ten animals were randomly selected as they passed through the chute. If two or more transport trucks arrived on the same day, two trucks were randomly selected and five animals were sampled from each truck. A single DNPS was collected from each animal by a trained staff member using a guarded swab (BD CultureSwab™ Plus, Mississauga ON). Swabs were shipped on ice in Amies transport media without charcoal (BD CultureSwab™ Plus) to the Lethbridge Research and Development Centre Microbiology Laboratory. Samples were kept at 4°C for up to 7 d prior to isolation of bacteria.
Upon arrival, the electronic ear tag was scanned and the arrival date, cattle type (beef, dairy), sex (heifer, bull, steer), weight (kg), age class (calf, yearling), source (ranch direct, auction barn, backgrounding operation), weather (temperature, precipitation, estimated wind speed), and BRD risk (high, low) were recorded. Ranch-direct cattle were defined as calves that arrived directly from the birth farm immediately after weaning or following a variable feeding period. Backgrounding operation-sourced cattle arrived directly from the backgrounding feedlot where they had been since weaning and fed a forage-based diet until ready for a finishing diet. Auction-derived cattle may have arrived at auction directly from the birth farm or from a backgrounding operation; this earlier history was unknown. Risk of BRD was predicted by the feedlot as per usual practices based on algorithms that consider age class, body weight, source, comingling prior to arrival, weather, transport distance, and visual health assessment. The geographical location of each feedlot, country of origin, and the point of sale at auction were recorded. Unlike dairy-type calves, beef-type calves were not traced back to their farm of origin given that more than 2/3 of the cattle (67.7%) were auction market derived. Morbidity and mortality data were compiled for all enrolled cattle up to 120 d post-arrival. Morbid animals [e.g., displaying gauntness, inactivity, depression, elevated rectal temperature (typically over 40°C), ocular/nasal discharge, cough, and/or elevated respiration rate] were designated as having BRD by trained feedlot pen riders or animal health personnel. Information on AMU before feedlot placement was unavailable.
Bacterial Isolation and Species Identification
Upon arrival at the laboratory, swabs were individually immersed in 1 mL of brain-heart infusion (BHI) containing 20% glycerol (Dalynn Biologicals, Calgary AB) and vortexed for 1 min (14). For the isolation of M. haemolytica and P. multocida, 100 μL suspension was plated onto blood agar (TSA) with 5% sheep blood supplemented with bacitracin at 15 μg/mL (Dalynn Biologicals). An additional 100 μL aliquot was plated onto TSA blood agar (Dalynn) for the isolation of H. somni. Additionally, a 1:10 dilution of the initial BHI-glycerol suspension was plated (100 μL) on TSA blood agar plates to enable isolation of H. somni without overgrowth. Bacitracin plates were incubated in an aerobic atmosphere at 37°C for 24 h, before selecting P. multocida and M. haemolytica colonies. Histophilus somni plates were incubated at 37°C for 2 d in a 5% CO2 atmosphere. Presumptive M. haemolytica, P. multocida, or H. somni colonies were identified, and three colonies of each bacterial species were sub-cultured onto TSA blood agar. Colonies were picked from TSA and stored at −80°C in BHI supplemented with 20% glycerol. For M. bovis, a high throughput PCR-based enrichment process was used for screening for the presence of M. bovis followed by bacterial isolation from positive suspensions (23).
All bacterial isolates were confirmed using PCR species-specific primers (Table 1) using a HotStartTaq Plus Master Mix kit (Qiagen, Toronto ON). For this, 3–5 colonies of pure cultures of P. multocida, M. haemolytica, or H. somni were suspended in 100 μL of TE buffer (10 mM Tris, 1 mM EDTA, pH = 8) and heat-lysed for 5 min at 95°C. The suspension was centrifuged for 10 min, at 18,400 × g at 4°C, and 2 μL of the supernatant was used as a DNA template. Presumptive M. bovis cultures were confirmed via direct-culture-PCR using 2 μL of liquid culture directly in the PCR master mix (23). The PCR amplicons were visualized either by agarose gel or capillary electrophoresis (QIAxcel, Qiagen). One randomly selected colony per bacterial species per DNPS was selected for further characterization and analysis. Additionally, M. haemolytica isolates were further characterized as serotype A1, A2 or A6 using a multiplex PCR (Table 1) (27).
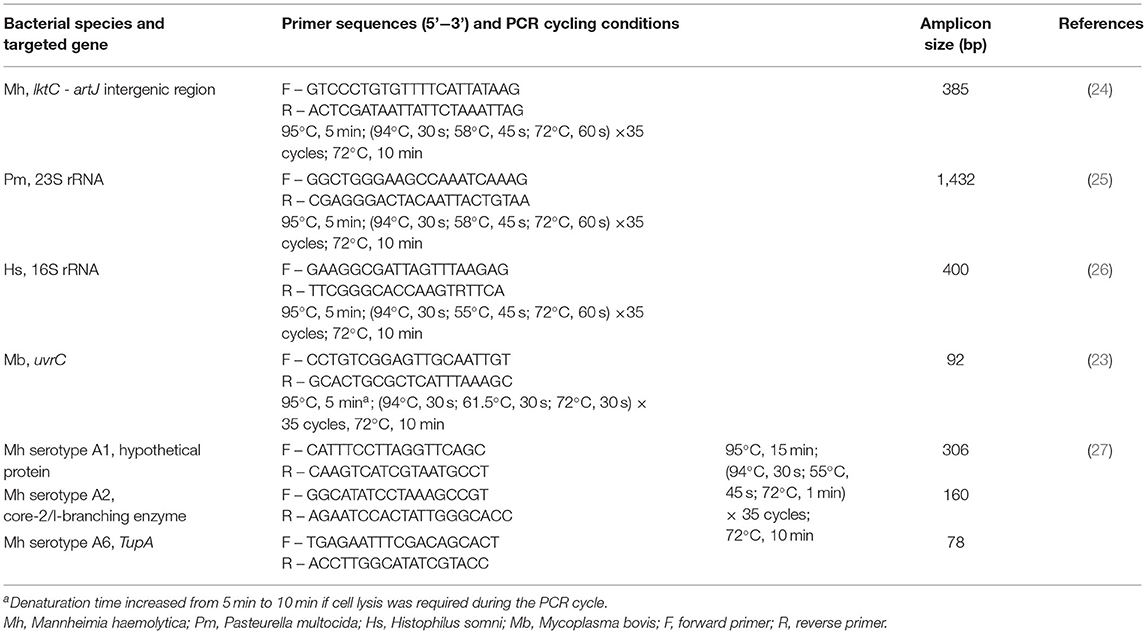
Table 1. Oligonucleotide primers, PCR protocols, and amplicon sizes for each PCR assay used in this study.
Antimicrobial Susceptibility Testing
Due to the high recovery of P. multocida and the cost of M. bovis ASTs, antimicrobial susceptibilities were only performed on a subset of P. multocida (n = 273/703 beef and n = 242/463 dairy isolates) and M. bovis (n = 122/257 beef and n = 100/198 dairy) isolates, whereas all M. haemolytica (n = 281 beef and n = 209 dairy) and H. somni (n = 68 and n = 173 dairy) isolates were tested. Pasteurella multocida and M. bovis isolates were selected based on proportional stratified random sampling (28) with consideration of feedlot, sampling month, cattle type, and country of origin. The antimicrobial susceptibility testing of M. haemolytica, P. multocida, and H. somni was carried out by broth microdilution according to Clinical and Laboratory Standards Institute (CLSI) guidelines using a final bacterial inoculum of ~5 × 105 CFU/mL (29). The Sensititre plate BOPO6F was used (Thermo Fisher Scientific, Mississauga ON), which represents a broad-spectrum of antimicrobials commonly used in beef cattle production. For M. haemolytica and P. multocida, the final per-well bacterial inoculum volume was 50 μL, whereas for H. somni, the volume was doubled to 100 μL as per manufacture's specifications. Therefore, the final antimicrobial concentrations tested for H. somni were half that of M. haemolytica and P. multocida (Supplementary Tables 1.1–1.3). Considering that the maximum spectinomycin (SPE) concentration against H. somni in BOPO6F plate was only 32 μg/mL, a custom plate with SPE ranging from 8 to 512 μg/mL was prepared for susceptibility testing. Bacterial isolates were designated as susceptible, intermediate, or resistant (SIR) according to CLSI (30). Additionally, CLSI MIC interpretive criteria for tilmicosin (TIL) in M. haemolytica was extrapolated to P. multocida and H. somni (31). Interpretive criteria for danofloxacin from M. haemolytica and P. multocida was also extrapolated to H. somni (32).
For M. bovis, recommendations for broth microdilution antimicrobial susceptibility were followed as official international guidelines are unavailable (11, 33, 34). A custom Sensititre plate (ref. no. YCML2FMBC, Thermo Fisher Scientific) was used to assess isolate sensitivities (Supplementary Table 1.4) (11). Mycoplasma bovis isolates were grown in PPLO broth and suspensions were adjusted to obtain a final concentration of 103-105 CFU/mL per well (100 μL final volume). Plates were incubated for 48 h, at 37°C, in a 5% CO2 atmosphere (35). AlamarBlue™ (Cell Viability Reagent. Thermo Fisher Scientific) at a final concentration of 10% was included in each well as indicator of cell viability and growth. Mycoplasma bovis ATCC 25523 was included as an internal standard in all susceptibility assays. Since CLSI breakpoints for M. bovis have not been developed, only MICs were reported.
Multidrug resistance (MDR) was defined as resistance to three or more antimicrobial drug classes (36). Isolates with intermediate susceptibility to an antimicrobial were not included in this definition. The 50th and 90th percentiles for MIC (MIC50 and MIC90, respectively) were calculated as the antimicrobial concentrations required to inhibit 50 and 90% of the isolates within each bacterial species. If growth was observed at the highest antimicrobial concentration tested, the MIC was assigned to the next dilution.
Antimicrobial Resistance Genetic Determinants
A subset of M. haemolytica (n = 87), P. multocida (n = 64), and H. somni (n = 24) isolates were selected for whole-genome sequencing (WGS). Isolates were selected to represent the diversity of feedlot operations, geographical origin, cattle type, sex, time of the year, and AMR phenotypes using proportional stratified random sampling. To isolate genomic DNA, bacterial cultures grown on blood agar plates were suspended in TE buffer (10 mM Tris – 1 mM EDTA, pH 8.0) to an OD600 of ~2, representing ~2 × 109 CFU/ mL. The cell suspension (1 mL) was transferred to a microcentrifuge tube and centrifuged for 2 min at 14,000 × g. Genomic DNA was extracted from the cell pellet using a DNeasy Blood and Tissue kit (Qiagen, Montreal QC). DNA quality and quantity were confirmed using a Nanodrop 2000 spectrophotometer and a fluorometer using PicoGreen (Thermo Fisher Scientific), respectively. Genomic library construction was performed using the NEB Ultra II library preparation kit (New England BioLabs, Ipswich MA). Library quality was assessed using a Bioanalyzer (Agilent, Santa Clara CA). Libraries were sequenced on an Illumina HiSeqX platform to generate 2 × 150 base paired-end reads. Library preparation and Illumina sequencing services were provided by Genome Quebec (McGill, QC). The quality of raw sequence reads was assessed using the quality control tool FastQC and reads were assembled into contigs using the Shovill pipeline (37). This pipeline included a quality trimming step with Trimmomatic (v.0.38) to remove common Illumina adapter sequences followed by de novo assembly with SPAdes (v.3.13.0) (38). The assembled contigs were annotated with Prokka (39) to identify coding genes. The assembled contigs were searched against the NCBI Bacterial Antimicrobial Resistance Reference Gene database (NCBI BioProject ID: PRJNA313047) to identify AMR genes. The genome sequencing data of isolates used in this study have been submitted to the NCBI under BioProject ID: PRJNA720670.
Data Analysis
Analyses were stratified by cattle type (beef or dairy) and included a descriptive examination of the unadjusted proportion of the following outcomes: DNPS positive for BRD bacteria, M. haemolytica serotypes, CLSI-determined resistance percentages, and BRD morbidities and mortalities (Figure 1). The unadjusted proportions of these outcomes were compared by cattle type using a chi-square or, when there were <5 samples in a stratum, the Fisher's Exact test (STATCALC, EpiInfo v.7.2.3.1). Antimicrobial susceptibility data were also presented as MIC frequency distributions and as MIC50 and MIC90 values. For M. bovis, only MIC frequency distributions and MIC50/MIC90 values were reported.
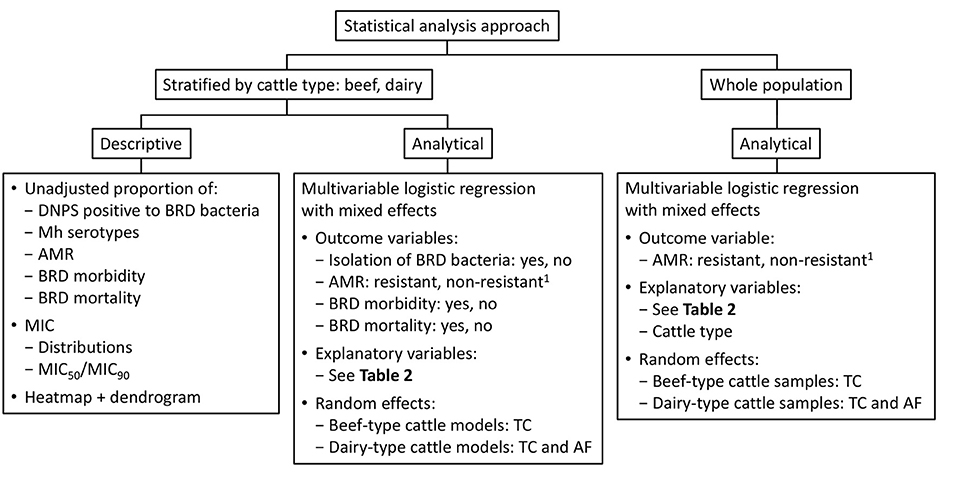
Figure 1. Statistical analyses used in the present study. 1Non-resistant category includes intermediate and susceptible categories. AF, arrived from; AMR, antimicrobial resistance; BRD, bovine respiratory disease; DNPS, deep nasopharyngeal swab; Mh, Mannheimia haemolytica; MIC, minimum inhibitory concentration; TC, truck cluster.
Multivariable logistic regression with mixed effects was used to describe the relationship between epidemiological risk factors and outcomes (Figure 1, Table 2), with control for confounding and clustered data. Outcomes included the isolation of BRD-related bacteria (yes, no), antimicrobial resistance [resistant bacteria vs. non-resistant (susceptible and intermediate) bacteria], BRD morbidity (yes, no) and BRD mortality (yes, no). Transport trailer was considered the sampling unit with animals from the same truck-load considered a cluster (TC). The majority of beef-type cattle were sourced through auction marts whereas most dairy-type cattle were farm-direct. When auction mart beef cattle were from different locations, but transported in the same truck, the random effect was nested (arrived from within TC). For dairy-type cattle models, 2 random effects were included (TC and arrived from) since the location of their origin was known. Random effects that did not account for variance within the model were removed. The variable feedlot was collinear with one-time feedlot capacity in morbidity and mortality models and as a result, it was excluded from these analyses. Based on descriptive analyses, bacterial AMR levels were consistently higher in dairy-type cattle as compared to beef-type cattle (Figure 2). Therefore, cattle type was used as an explanatory variable in logistic regression analyses in which the whole sampled population was considered to investigate associations between cattle type and AMR. The R package lme4 (v.1.1-23) was used to fit mixed effects models (RStudio v.4.0.2). Continuous variables (temperature and body weight) were scaled as it was found to improve model convergence. When models were fitted with no random effects because the variance of the RE was equal to zero, the glm function (package stats v.4.0.2) was used with logit link. A backward elimination (40) approach was used and if the Akaike information criterion (AIC) value increased substantially and/ or the β-coefficient of a variable varied more than 30% upon removal, then it was retained in the model (28). Collinearity was evaluated through the fitted model through the variance inflation factor (VIF) (car package v.3.0-7; R Studio v.3.6.3). The possibility of a non-linear relationship between the continuous explanatory variables (weight and temperature) and the probability of the outcome on the log odds (logit) scale was tested by the addition of a quadratic term that was included in the model if statistically significant.
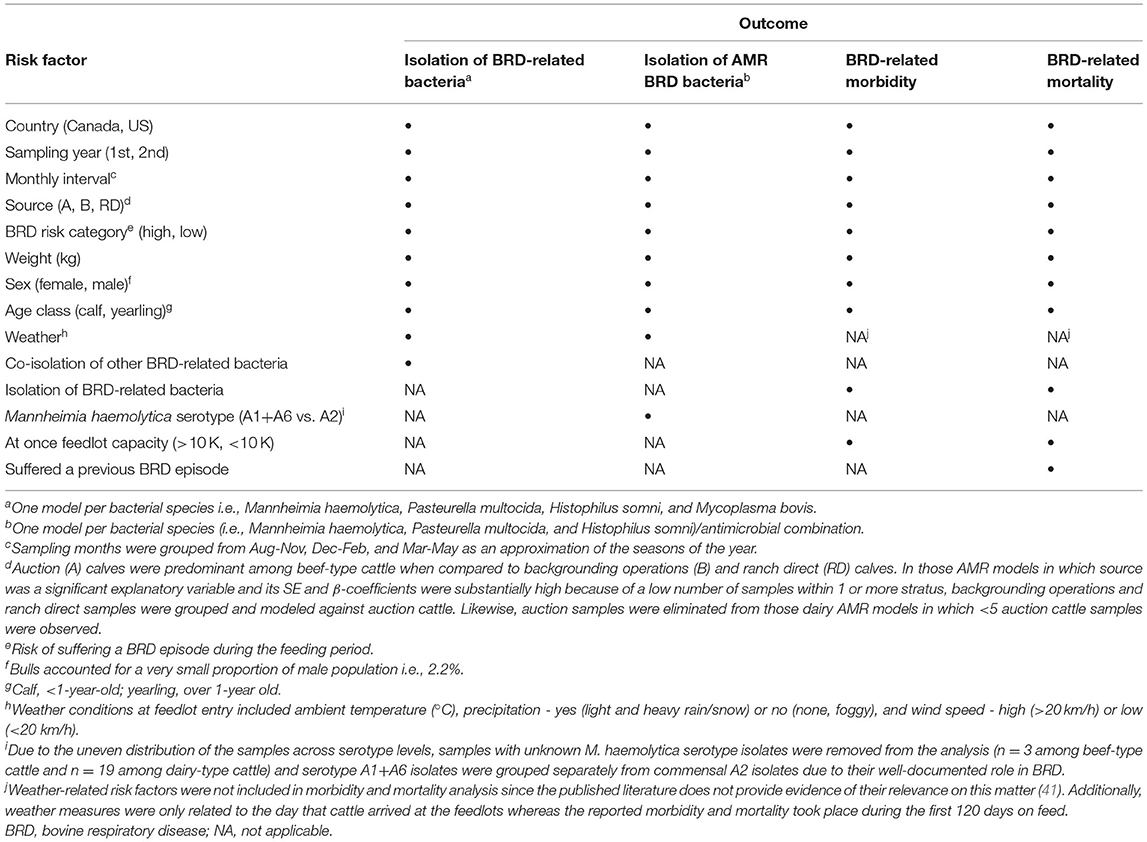
Table 2. Risk factors and outcomes investigated by multivariable logistic regression for beef and dairy-type cattle upon feedlot arrival.
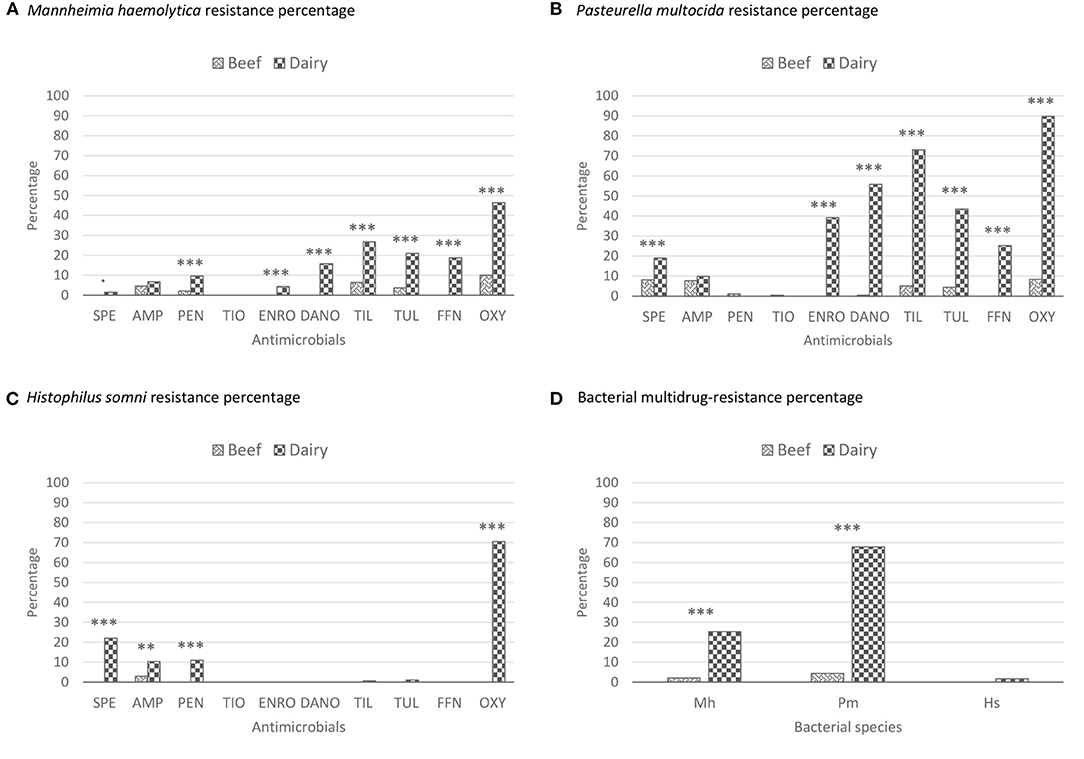
Figure 2. Antimicrobial resistance percentages of the BRD-bacterial isolates recovered from beef and dairy-type cattle upon feedlot arrival. These percentages represent unadjusted proportions. The asterisks represent the statistical test significance levels as follows: “.” 0.1, “**” 0.01, “***” 0.001. Multidrug resistance was defined as resistance to 3 or more different antimicrobial classes (36). AMP, ampicillin; BRD, bovine respiratory disease; DANO, danofloxacin; ENRO, enrofloxacin; FFN, florfenicol; Hs, Histophilus somni; Mb, Mycoplasma bovis; Mh, Mannheimia haemolytica; OXY, oxytetracycline; PEN, penicillin; Pm, Pasteurella multocida; SPE, spectinomycin; TIL, tilmicosin; TIO, ceftiofur; TUL, tulathromycin. (A) Beef SPE resistance (R): 0/281; beef AMP-R: 13/281; beef PEN-R: 6/281; beef TIO-R: 0/281; beef ENRO-R: 0/281; beef DANO-R: 0/281; beef TIL-R: 18/281; beef TUL-R: 10/281; beef FFN-R: 0/281; beef OXY-R: 28/281; dairy SPE-R: 3/209; dairy AMP-R: 14/209; dairy PEN-R: 20/209; dairy TIO-R: 0/209; dairy ENRO-R: 9/209; dairy DANO-R: 33/209; dairy TIL-R: 56/209; dairy TUL-R: 44/209; dairy FFN-R: 39/209; dairy OXY-R: 97/209. SPE X2 (1, n = 409) = 4.06, p = 0.0440; AMP X2 (1, n = 409) = 0.99, p = 0.3201; PEN X2 (1, n = 409) = 13.18, p < 0.001; ENRO X2 (1, n = 409) = 12.33, p < 0.001; DANO X2 (1, n = 409) = 47.57, p < 0.001; TIL X2 (1, n = 409) = 38.86, p < 0.001; TUL X2 (1, n = 409) = 37.41, p < 0.001; FFN X2 (1, n = 409) = 56.97, p < 0.001; OXY X2 (1, n = 409) = 83.79, p < 0.001. (B) Beef SPE-R: 22/273; beef AMP-R: 21/273; beef PEN-R: 3/273; beef TIO-R: 1/273; beef ENRO-R: 0/273; beef DANO-R: 1/273; beef TIL-R: 14/273; beef TUL-R: 12/273; beef FFN-R: 0/273; beef OXY-R: 23/273; dairy SPE-R: 46/242; dairy AMP-R: 24/242; dairy PEN-R: 0/242; dairy TIO-R: 0/242; dairy ENRO-R: 95/242; dairy DANO-R: 135/242; dairy TIL-R: 177/242; dairy TUL-R: 105/242; dairy FFN-R: 61/242; dairy OXY-R: 217/242. SPE X2 (1, n = 515) = 13.42, p < 0.001; AMP X2 (1, n = 515) = 0.80, p = 0.3722; PEN X2 (1, n = 515) = 2.67, p = 0.1482; TIO X2 (1, n = 515) = 0.89, p = 0.2650; ENRO X2 (1, n = 515) = 131.41, p < 0.001; DANO X2 (1, n = 515) = 202.73, p < 0.001; TIL X2 (1, n = 515) = 254.32, p < 0.001; TUL X2 (1, n = 515) = 111.09, p < 0.001; FFN X2 (1, n = 515) = 78.06, p < 0.001; OXY X2 (1, n = 515) = 340.27, p < 0.001. (C) Beef SPE-R: 0/68; beef AMP-R: 2/68; beef PEN-R: 0/68; beef TIO-R: 0/68; beef ENRO-R: 0/68; beef DANO-R: 0/68; beef TIL-R: 0/68; beef TUL-R: 0/68; beef FFN-R: 0/68; beef OXY-R: 0/68; dairy SPE-R: 38/173; dairy AMP-R: 23/173; dairy PEN-R: 19/173; dairy TIO-R: 0/173; dairy ENRO-R: 0/173; dairy DANO-R: 0/173; dairy TIL-R: 1/173; dairy TUL-R: 2173/; dairy FFN-R: 0/173; dairy OXY-R: 122/173. SPE X2 (1, n = 241) = 17.73, p < 0.001; AMP X2 (1, n = 241) = 5.63, p = 0.0063; PEN X2 (1, n = 241) = 8.11, p < 0.001; TIL X2 (1, n = 241) = 0.69, p = 0.3589; TUL X2 (1, n = 241) = 0.79, p = 0.2572; OXY X2 (1, n = 241) = 97.12, p < 0.001. (D) Beef Mh MDR: 6/281; beef Pm MDR: 12/274; beef Hs MDR: 0/68; dairy Mh MDR: 53/209; dairy Pm MDR: 164/242; dairy Hs MDR: 3/173. X2 (1, n = 409) = 61.04, p < 0.001; X2 (1, n = 515) = 137.84, p < 0.001; X2 (1, n = 241) = 1.19, p = 0.1810.
Clinical and Laboratory Standards Institute breakpoints for BRD bacteria have not been developed for all antimicrobials. Consequently, heatmaps with dendrograms were generated to visually explore the associations of MIC across all antimicrobials, except for trimethroprim/ sulfamethoxazole (SXT) and sulfadimethoxine which were tested at a single concentration. For these, antimicrobial MIC frequency distributions were plotted using the heatmap.2 function from the gplots package v.3.0.4 in RStudio. Dendrograms were generated to explore associations across antimicrobials and across the strata of cattle type, monthly intervals, country of origin, source, feedlot, weight range (100 kg intervals: 100–199, 200–299, 300–399, 400–499, and 500–599 kg), age class, risk category, atmospheric temperature range (14°C intervals: −27 to −14, −13 to 0, 1 to 13, and 13 to 27°C), and BRD treatment (yes/no) and BRD mortality (yes/no). Feedlot was used as a clustering factor to explore whether any of the feedlots included in this study purchased cattle from locations containing higher levels of AMR. When non-clonal bacterial populations presenting high MICs were associated with a specific feedlot, individual dendrograms were generated for bacterial species/ feedlot combination using source, arrived from within a truck load, and truck load as clustering factors. Since not all the antimicrobials were tested in the same concentration range and most MIC frequency distributions were not normally distributed, MIC observed values were normalized (the minimum value of the dataset was subtracted to each value and divided by the maximum value in the dataset) bringing the data to the 0–1 scale (42).
Results
Sampling
A total of 2,824 DNPS were collected with 1,391 (49.3%) in year 1 and 1,433 (50.7%) in year 2 (Supplementary Table 2). Six feedlots provided DNPS only from beef-type cattle (B, D, E, F, H, I), two only from dairy-type cattle (C, J), with a mixture of both cattle types from the remainder (A, G). Four feedlots provided over 300 DNPS, five provided over 200 DNPS, and one feedlot provided <200 DNPS during the study (Supplementary Table 2). To optimize sampling numbers related to seasonal inconsistency in cattle availability, more than 10 animals may have been sampled from each truck load. Additionally, a small proportion of the collected DNPS (n = 241) failed to meet the quality standards for processing and were eliminated from the study. For beef-type cattle, the range of animals sampled per truck load was 3–57 (median = 10) whereas for dairy-type cattle, the number of animals sampled per load ranged from 9 to 20 (median = 10).
Two thousand and fifty-five (72.8%) DNPS were collected from beef-type cattle and 769 (27.2%) DNPS were collected from dairy-type cattle. Compared to dairy-type cattle, beef cattle-type were heavier in incoming body weight, older upon arrival, and originated more frequently from within Canada (96.1%) (Table 3). Beef cattle-type were also more often classified as low BRD risk (60.5%) than dairy-type cattle (24.5%). Beef-type cattle were primarily sourced from auction barns (67.7%), whereas, a greater proportion of dairy-type cattle originated directly from dairy farms (88.4%). Of 2,824 cattle, 338 (12.0%) were clinically diagnosed with BRD over the 120 d feeding period (Figure 3) and 29 animals (5.6%) succumbed to BRD. Among the 29 mortalities, 19 (65.5%) were previously treated for BRD at least once.
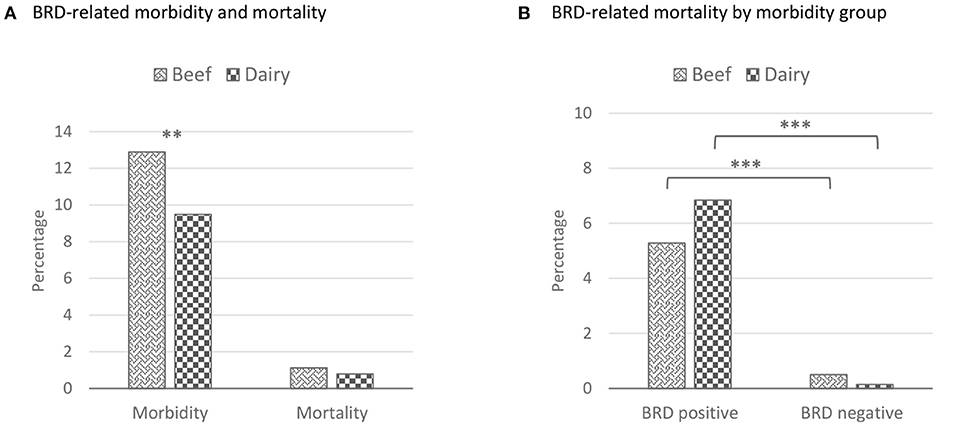
Figure 3. Proportion of cattle treated at least once for BRD and/ or succumbed to BRD during the first 120 d of the feeding period for beef and dairy-type cattle. These percentages represent unadjusted proportions. The asterisks represent the statistical test significance level as follows: “**” 0.01, “***” 0.001. BRD, bovine respiratory disease; Tx, treatment. (A) Beef morbidity proportion: 265/2,055 DNPS; beef mortality proportion: 23/2,055 DNPS; dairy morbidity proportion: 73/769 DNPS; dairy mortality proportion: 6/769 DNPS. Morbidity X2 (1, n = 2,428) = 6.15, p = 0.0132; mortality X2 (1, n = 2,428) = 0.63, p = 0.4264. (B) BRD-treated beef mortality proportion: 14/265; non-BRD treated beef mortality proportion: 9/1,790; BRD-treated dairy mortality proportion: 5/73; non-BRD treated dairy mortality proportion: 1/696. Beef X2 (1, n = 2,055) = 48.04, p < 0.001; dairy Fisher Exact (1, n = 769) = 35.27, p < 0.001.
Bacterial Isolation and Species Identification
A total of 1,646 out of 2,824 (58.3%) DNPS were positive for at least one of the bacterial species. Overall, P. multocida (41.3%) was most commonly isolated, followed by M. haemolytica (17.4%), M. bovis (16.1%), and H. somni (8.5%) (Figure 4A). A total of 492 M. haemolytica isolates were obtained from beef and dairy-type cattle (Figure 4B). For beef-type cattle, 57 isolates (20.1%) were serotype A1, 178 (62.9%) were A2, 45 (15.9%) were A6, and 3 (1.1%) were undetermined. In dairy-type cattle, 59 (28.2%), 99 (47.4%), 32 (15.3%), and 19 (9.1%) of the isolates were serotype A1, A2, A6, and undetermined, respectively.
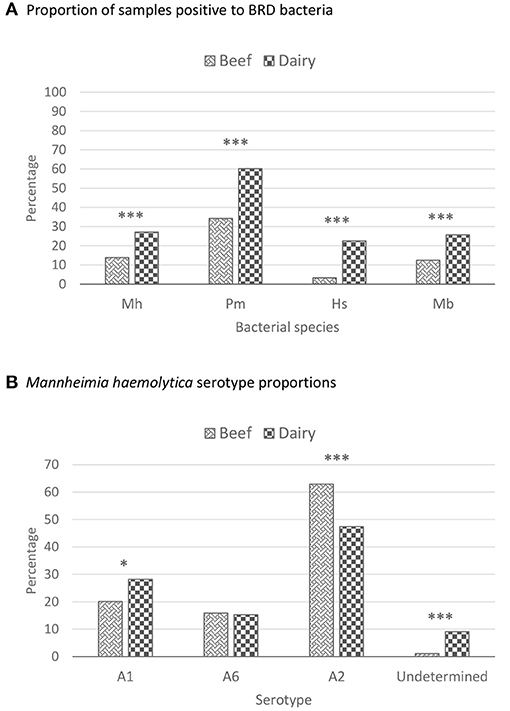
Figure 4. Proportion of deep nasopharyngeal swabs that were positive for BRD-related bacteria and Mannheimia haemolytica serotype proportions recovered from beef and dairy-type cattle upon feedlot arrival. These percentages represent unadjusted proportions. The asterisks represent the statistical test significance levels as follows: “*” 0.05, “***” 0.001. BRD, bovine respiratory disease; Hs, Histophilus somni; Mb, Mycoplasma bovis; Mh, Mannheimia haemolytica; Pm, Pasteurella multocida. (A) Beef Mh positive proportion: 283/2,055; beef Pm positive proportion: 703/2,055; beef Hs positive proportion: 68/2,055; beef Mb positive proportion: 257/2,055; dairy Mh positive proportion: 209/769; dairy Pm positive proportion: 463/769; dairy Hs positive proportion: 173/769; dairy Mb positive proportion: 198/769. Mh X2 (1, n = 2,824) = 69.91, p < 0.001; Pm X2 (1, n = 2,824) = 156.04, p < 0.001; Hs X2 (1, n = 2,824) = 263.94, p < 0.001; Mb X2 (1, n = 2,824) = 72.60, p < 0.001. (B) Beef Mh A1 positive proportion: 57/283; beef Mh A6 positive proportion: 45/283; beef Mh A2 positive proportion: 178/283; beef Mh unknown positive proportion: 3/283; dairy Mh A1 positive proportion: 59/209; dairy Mh A6 positive proportion: 32/209; dairy Mh A2 positive proportion: 99/209; dairy Mh unknown positive proportion: 19/209. Mh A1 X2 (1, n = 492) = 4.36, p = 0.0367; Mh A6 X2 (1, n = 492) = 0.03, p = 0.8587; Mh A2 X2 (1, n = 492) = 11.78, p < 0.001; Mh unknown Fisher Exact (1, n = 492) = 18.15, p < 0.001.
Antimicrobial Susceptibility Testing
Overall, the proportions of AMR-resistant M. haemolytica, P. multocida, and H. somni isolates recovered from dairy-type cattle were higher than those from beef-type cattle (Figures 2A–D). Mannheimia haemolytica isolates from dairy-type cattle had higher MIC90 for CTET and neomycin (NEO) (8 and >32 μg/mL, respectively) than those from beef-type cattle (2 and 8 μg/mL, respectively) (Supplementary Table 1.5). Likewise, P. multocida isolates from dairy-type cattle had higher MIC50/MIC90 values for CTET (4/8 μg/mL), NEO (>32/>32 μg/mL), and TYLT (>32/>32 μg/mL) than isolates from beef-type cattle (0.5/1, 16/16, and 16/32 μg/mL, respectively). Among H. somni isolates from dairy-type cattle, MIC50/MIC90 values were also higher for CTET (2/4 μg/mL) and TYLT (8/16 μg/mL) than those from beef-type cattle (0.25/0.25 and 4/4 μg/mL, respectively).
Antimicrobial susceptibility testing was completed for 490 out of 492 (99.6%) of the M. haemolytica isolates (281 from beef and 209 from dairy) (Supplementary Table 1.1). The most frequently observed resistances were OXY (10%), TIL (6.4%), and ampicillin (AMP; 4.6%) among isolates from beef-type cattle and OXY (46.4%), TIL (26.8%), and TUL (21.0%) among dairy-type cattle isolates (Figure 2A, Supplementary Figure 1.1). Interestingly, all M. haemolytica isolates exhibited a high MIC for TYLT at 32 μg/mL (1.8% of the isolates) and >32 μg/mL (98.2% of the isolates). A higher proportion of M. haemolytica serotype A1 isolates were resistant to ENRO (7.8%), DANO (24.1%), and OXY (61.2%) than serotype A2 (0, 1.5, and 18.5%) and A6 (0, 1.3, and 1.3%) isolates. In contrast, serotype A2 isolates exhibited higher proportion of resistance to penicillin (PEN; 7.6%), TUL (17.1%), and FFN (13.5%) than did A1 and A6 (Supplementary Figure 1.2). Resistance to SPE and TIL was similar between A1 and A2 isolates (1.7 and 0.4%, respectively; 19.8 and 18.5%, respectively). Of the three serotypes, A6 showed the overall lowest AMR. Among P. multocida isolates, resistance to OXY (8.4%), SPE (8.1%), and AMP (7.7%) was most frequent from beef compared to OXY (89.7%), TIL (73.1%), and DANO (55.8%) from dairy-type cattle (Figure 2B). Pasteurella multocida also presented high MICs (MIC50 and MIC90 > 32 μg/mL) for TYLT, especially in isolates from dairy-type cattle, with only one (0.2%) P. multocida isolate exhibiting resistance to ceftiofur (TIO; cephalosporin). Histophilus somni isolates from dairy-type cattle were more frequently resistant to OXY (70.5%), SPE (22%), and PEN (11.0%) compared to isolates from beef-type cattle, which only exhibited resistance to AMP (2.9%) (Figure 2C). The total percentage of MDR in all M. haemolytica, P. multocida, and Histophilus somni isolates were 12, 34.2, and 1.25%, respectively (Figure 2D).
Mycoplasma bovis MIC50/MIC90 values were consistently higher for macrolides than for other antimicrobials, regardless of cattle type, especially for TIL (>256 μg/mL both) and TIP (>128 μg/mL both) (Supplementary Table 1.5). Likewise, the MIC50/MIC90 values of GAM (128/>256 μg/mL), TUL (16/>256 μg/mL), and TYLT (32/>128 μg/mL) were also high. Enrofloxacin (dairy-type cattle isolates), GAM (beef and dairy isolates), TUL (beef and dairy isolates), and TYLT (beef and dairy isolates) MIC distributions exhibited a bimodal pattern. As previously reported, AlamarBlue™ prevented the estimation of the OXY MIC of 2 M. bovis isolates from dairy-type cattle when MIC ≥ 32 μg/mL (11).
Antimicrobial Resistance Genetic Determinants
Overall, the AMR phenotypes for M. haemolytica, P. multocida, and H. somni were corroborated with the presence of related antimicrobial resistance genes (ARG), as inferred from the WGS data (Supplementary Tables 3.1–3.3). The tet(H) gene was the most abundant determinant identified and corresponded with OXY resistance in M. haemolytica and P. multocida (Supplementary Tables 3.1, 3.2). In H. somni, tet(H) was detected in isolates with CTET MICs ranging between 1 and >4 μg/mL (Supplementary Table 3.3). Genes related to NEO and kanamycin resistance were the second most abundant determinants identified [aph(3')-Ia], coinciding with NEO MIC values > 32 μg/mL for M. haemolytica and P. multocida. Considering that the NEO concentrations were not tested beyond 16 μL/mL for H. somni, it was not possible to determine if the presence of aph(3')-Ia conferred NEO MIC > 32 μg/mL. Likewise, the ARG for GEN resistance [ant(2”)-Ia] was present in M. haemolytica when MIC values for this drug were >16 μg/mL, whereas in H. somni, aac(3)-Iva, a gentamycin (GEN) resistance gene, was associated with the GEN phenotype in only 5 of the 8 GEN resistant (MIC > 8 μg/mL) isolates. The presence of floR corresponded with FFN MIC ≥ 8 μg/mL for M. haemolytica, MIC ≥ 4 μg/mL for P. multocida, and MIC ≥ 0.5 μg/mL for H. somni. The presence of blaROB−1 was associated with AMP resistance and the detection of aadA25 (M. haemolytica) or aadA31 (P. multocida and H. somni) was associated with SPE resistance. In M. haemolytica and P. multocida, the presence of mutations in the quinolone resistance-determining region (QRDR) was related to resistance to FQs. In many cases, resistance to macrolides (TIL, TUL) was related to the presence of the erm(42) and/or the mph(E)-msr(E) operon in M. haemolytica, and erm(42) and/or the mph(E)-msr(E) operon or A2058G mutation in the 23S rRNA gene of P. multocida. No corroboration could be established between macrolide phenotype and genotype for H. somni isolates as 5 of the 24 sequenced isolates carried erm(42) and/or erm(F) genes, yet they were not resistant to macrolides (TIL and TUL MIC ranging from 2 to 4 μg/mL). Streptomycin resistance ARGs aph(3”)-Ib and aph(6)-Id frequently co-existed with NEO determinant aph(3')-Ia in the three Pasteurellaceae species. However, phenotypic streptomycin resistance was not assessed in any of the isolates.
Multivariable Logistic Regression
Recovery of BRD-Related Bacteria
Multivariable logistic regression determined that the odds of isolating a BRD pathogens was variably associated with the isolation of other BRD pathogens and the monthly arrival interval (Table 4). In the second year, the odds ratio of recovering M. bovis from both cattle types was higher than in the first year i.e., 2.3 (95% CI = 1.3–4.0, p = 0.006) and 2.5 (95% CI = 1.3–4.74, p = 0.004) in both beef and dairy-type cattle, respectively (Supplementary Tables 4.1, 4.2).
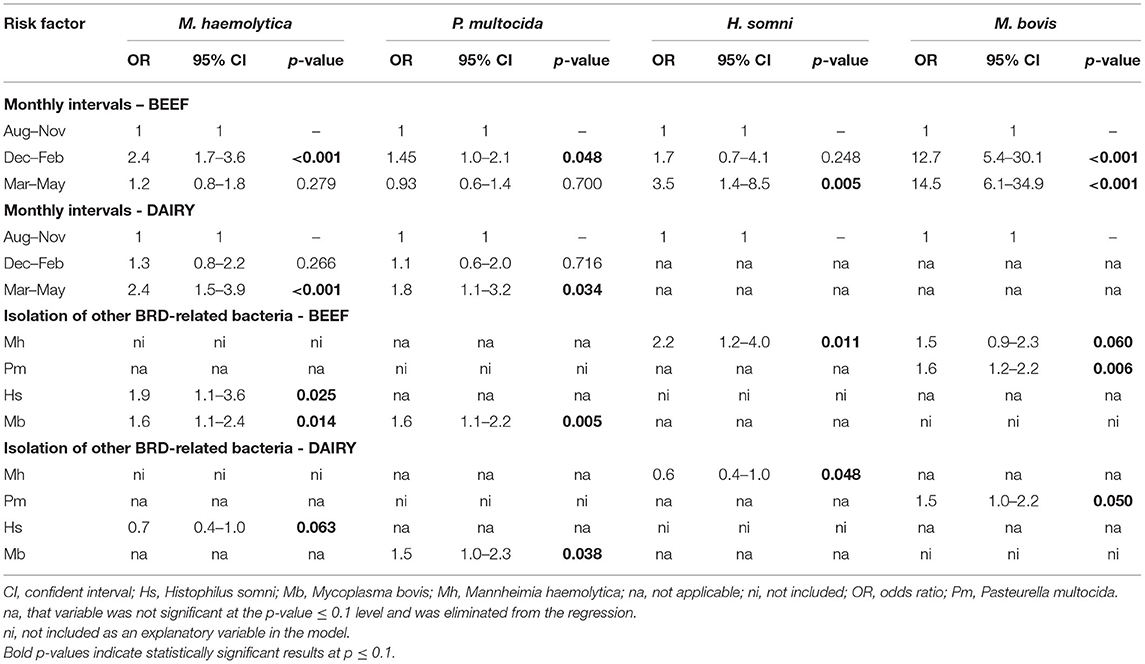
Table 4. Significant results obtained from logistic regression of recovery of bovine respiratory disease complex bacteria from beef and dairy-type cattle upon feedlot arrival.
Recovery of AMR Bacteria
Depending on the BRD bacterial species isolated from beef-type cattle, the odds of these bacteria being AMR were between 8.5 and 17.5 higher between Mar–May as compared to Aug–Nov (Tables 5, 6). In dairy-type cattle, BRD-related bacteria had lower odds of being AMR during Mar–May than Aug–Nov, and Aug–Nov as compared to Dec–Feb (Tables 7, 8). The country of origin did not affect the odds of isolating AMR BRD bacteria in beef-type cattle (Supplementary Table 4.1), whereas in dairy-type cattle it varied depending on the antimicrobial and the bacterial species (Tables 7–9). The odds of recovering AMR bacteria from backgrounded beef-type feedlot cattle were higher than for those purchased at auction (Tables 5, 6). The BRD risk category showed no effect on AMR among beef-type cattle (Supplementary Table 4.3), whereas for dairy-type cattle, it varied depending on the antimicrobial and the bacterial species (Supplementary Table 4.4). Overall, a higher ambient temperature was associated with greater odds of recovering resistant bacteria in both cattle types (Supplementary Tables 4.3, 4.4). Mannheimia haemolytica A1 and A6 serotypes were associated with higher odds of OXY resistance in beef (OR = 38.3, 95% CI = 4.8–304.1, p < 0.001) and dairy-type cattle (OR = 4.8, 95% CI = 1.9–11.9, p < 0.001) as compared to A2. The A1 and A6 serotypes, presented lower odds of being resistant to almost all antimicrobials tested among dairy-type cattle when compared to A2 (Tables 5, 6). Dairy-type cattle were associated with higher levels of AMR as compared to beef-type cattle (Supplementary Tables 4.3, 4.4).
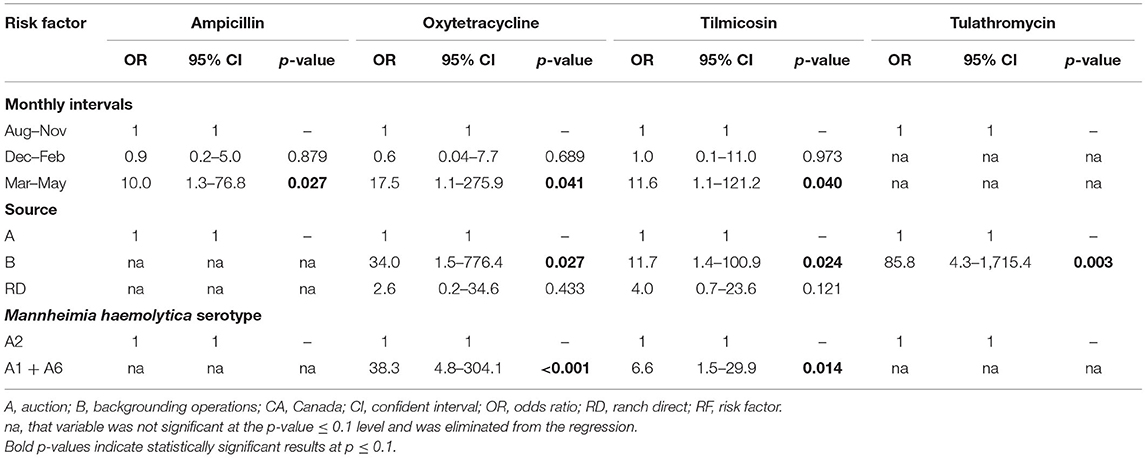
Table 5. Significant results obtained from logistic regression of antimicrobial resistant Mannheimia haemolytica from beef-type cattle upon feedlot arrival.
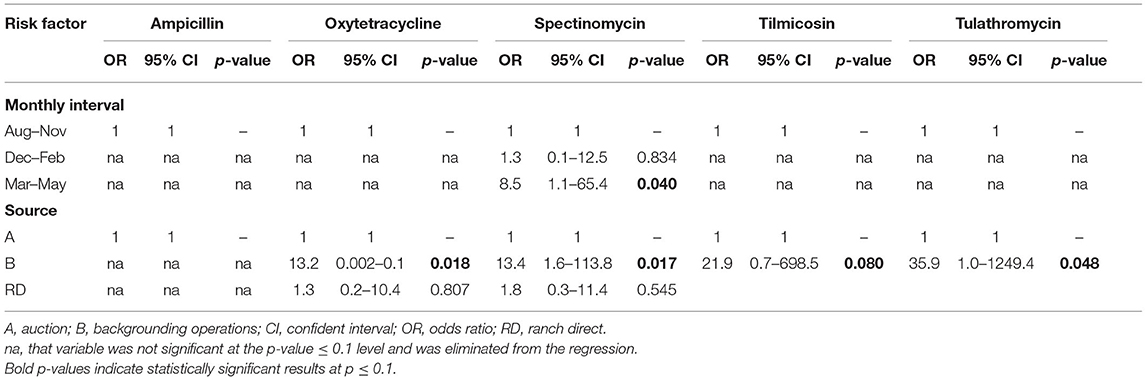
Table 6. Significant results obtained from logistic regression of antimicrobial resistant Pasteurella multocida from beef-type cattle upon feedlot arrival.
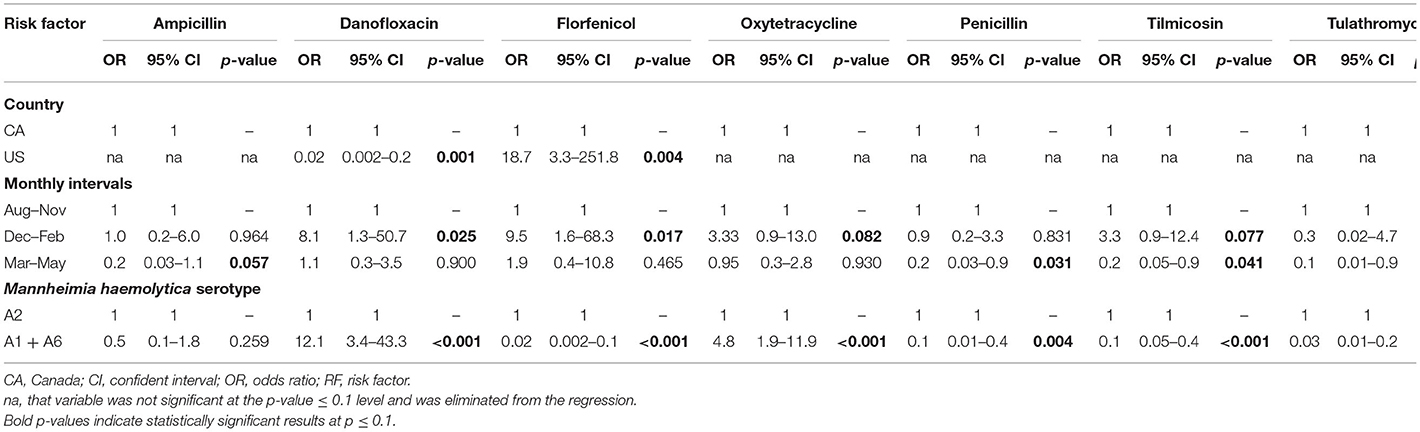
Table 7. Significant results obtained from logistic regression of antimicrobial resistant Mannheimia haemolytica from dairy-type cattle upon feedlot arrival.
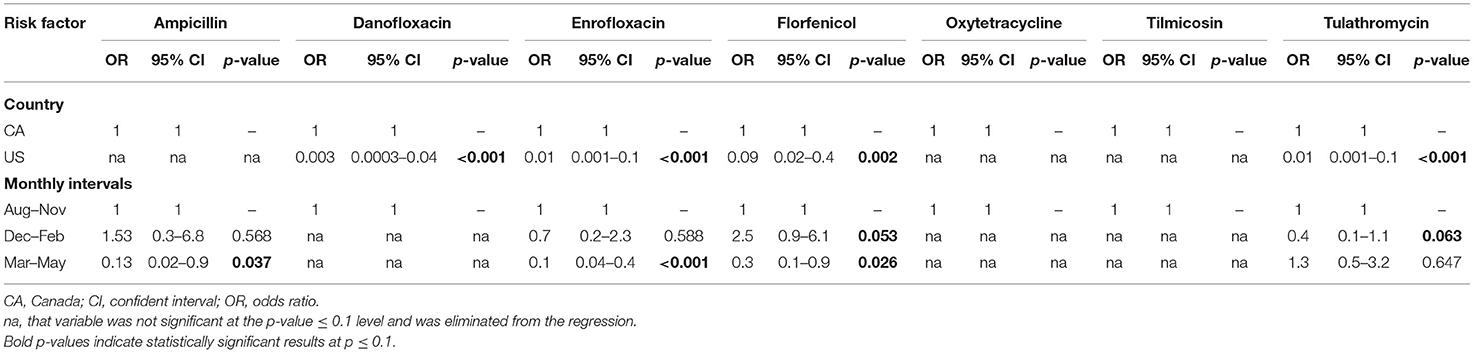
Table 8. Significant results obtained from logistic regression of antimicrobial resistant Pasteurella multocida from dairy-type cattle upon feedlot arrival.

Table 9. Significant results obtained from logistic regression of antimicrobial resistant Histophilus somni from dairy-type cattle upon feedlot arrival.
BRD-Related Morbidity and Mortality
There were no differences in the morbidity or mortality between cattle types sourced in either Canada or the US (Supplementary Table 4). The isolation of any BRD-related nasal bacteria upon feedlot arrival was not related to BRD mortalities during the feeding period. Cattle that were treated for BRD at least once were positively associated with dying from BRD. Additionally, different risk factors were associated with morbidity and/or mortality depending on cattle type i.e., BRD risk, monthly interval, weight, and age (Supplementary Tables 4.6–4.9).
Heatmaps
Antimicrobial resistant profiles of isolates from dairy-type cattle presented higher MIC values (values closer to 1), including for antimicrobials with no SIR categories such as CTET or TYLT, in M. haemolytica, P. multocida, and H. somni (Supplementary Figures 1.3.1–1.3.3). Mycoplasma bovis isolates with high macrolide MIC values were obtained from both cattle types. However, higher ENRO MICs were mainly associated with isolates from dairy-type calves as indicated by MIC50/MIC90 values (Supplementary Table 1.5, Supplementary Figure 1.3.4). Additionally, M. bovis isolates obtained from backgrounding beef-type cattle predominated among AMR profiles showing higher MICs as compared to auction and ranch direct beef-type cattle (Supplementary Figure 1.3.41). Interestingly, M. haemolytica serotypes A1 and A6 isolated from beef-type cattle were mainly distributed among isolates showing higher MICs, whereas A2 typically exhibited lower MICs (Supplementary Figure 1.3.13). Pasteurella multocida from US dairy-type cattle exhibited higher MIC values as compared to those isolated from Canadian dairy-type cattle (Supplementary Figure 1.3.17). Among dairy-type cattle, M. haemolytica and M. bovis isolates collected at feedlot C also had higher MICs than those from feedlots A, G, and J (Supplementary Figures 1.3.16, 1.3.49). Notably, feedlot C purchased dairy-type cattle mainly from different farms located in Alberta, Canada, whereas feedlots A, G, and J purchased dairy-type cattle mainly from the US (Supplementary Figures 1.3.50, 1.3.51).
Discussion
In this study, we described the prevalence of AMR in M. haemolytica, P. multocida, and H. somni in cattle at feedlot arrival, and documented that it was higher in dairy than beef-type cattle. Multidrug-resistant Pasteurellaceae bacteria have been isolated from BRD clinical cases and mortalities before (43–45), and AMR has been investigated in cattle at feedlot arrival in previous studies (14–16, 46). However, to our knowledge this study is the first in North America to collect all four species of the BRD bacterial complex from a broad cross-sectional feedlot cattle population, and investigate their antimicrobial susceptibilities at feedlot entry. One limitation of this study is the large ORs and wide CIs generated by logistic regression in some instances (Supplementary Tables 4.1–4.9) limited the precision of the conclusions obtained in this study.
The prevalence of P. multocida and H. somni in beef-type cattle was higher compared to previous studies that collected respiratory samples from feedlot cattle at arrival, but comparable for M. haemolytica (14–16). The higher prevalence of M. haemolytica serotype A2 was expected as compared to A1 and A6, as it is more frequently isolated from healthy cattle (47). Dairy-type cattle tended to harbor more members of the BRD bacterial complex than beef-type cattle. This association may be a consequence of early weaning practices in the dairy industry (17). Most dairy calves are weaned within a day of birth (17), which may limit their ingestion of colostrum. The acquisition of passive immunity at this age is limited during a critical time when the calf's immune system is still immature (48). Most beef calves are raised under extensive conditions, whereas dairy calves are often housed in confinement from birth, a practice that could promote the transmission of BRD bacteria among individuals. As reported by Griffin (49), the odds of isolating Mycoplasma bovis from both beef and dairy cattle classified as high-BRD risk were higher than those classified as low risk. However, in the current study, M. haemolytica, P. multocida, and H. somni were not associated with cattle classified as high-BRD risk.
The proportion of cattle that had BRD at least once (12%) and succumbed to it (5.6%) was comparable to those previously reported, 16.2 and 4%, respectively (50). It has been suggested that sourcing cattle directly from ranches reduces exposure to BRD-related bacteria (49), but we did not find greater odds of recovering BRD bacteria from beef-type cattle purchased at auction as compared to those from backgrounding operations or directly from ranches. Nevertheless, auction-sourced cattle did have greater odds of being treated for BRD. Auction market-derived beef-type cattle (n = 1,392) originated from 69 different locations. Frequent comingling of cattle from different locations can increase the incidence of BRD morbidity during the feeding period (51, 52). Most weaned beef calves enter the feedlot in the fall and at this time a large number of calves are comingled during sale and shipped to feedlots. During this time they are subject to handling and transport stress, resulting in an increase in BRD-morbidities and mortalities (41). Although the fall interval (Aug-Nov) was associated with more BRD morbidity in beef-type cattle, it was not associated with higher isolation of BRD bacteria at arrival. Heavier and older (yearlings) cattle were less likely to develop BRD, an observation that agrees with that of others (41). High-BRD risk beef-type cattle were not associated with greater BRD during the feeding period. It has been reported that high-BRD risk cattle are over 100 times more likely to be administrated metaphylactic macrolides than low-BRD risk cattle (53). It is well-established that the use of metaphylactic antimicrobials in moderate to high risk BRD cattle reduces morbidity and mortality (53) which could account for the lower BRD morbidity in high-BRD risk cattle. Only the isolation of P. multocida in dairy-type cattle at feedlot entry was associated with higher BRD morbidity during the feeding period (Supplementary Table 4.8). The bovine respiratory tract microbiome undergoes substantial changes in bacterial composition after feedlot placement (54). Bovine respiratory disease is also a complex disease process involving viral co-infections, and host immunity and environmental factors. Therefore, there may not be a causal link between the presence/absence of BRD bacteria in the respiratory tract of cattle upon arrival and morbidity and mortality during the feeding period. This study was limited to considering only the implications of the bacteriological component on arrival for BRD morbidities and mortalities that occurred later in the feeding period. Other factors known to contribute to this disease complex, such as immune and nutritional status, vaccination, housing, management practices, and the respiratory virome (8, 55) were not evaluated.
A higher risk of BRD morbidity has been associated with the purchase of calves in the fall (41). Aug–Nov (for dairy) and Dec–Feb (for beef and dairy) as compared to Mar–May were associated with higher AMR possibly as a result of increased AMU during this time of the year. Higher odds of AMR nasal BRD bacteria recovery were observed in beef-type cattle backgrounded in feedlots as compared to auction-derived calves. This observation most likely reflects the administration of some antimicrobials to cattle at backgrounding feedlots and the confined conditions that may promote transmission of AMR bacteria amongst cattle. However, some auction-derived cattle may also have been in a backgrounding operation prior to transfer to an auction market but were classified as auction-derived due to the impossibility of gathering such information. The odds of isolating AMR-BRD bacteria were consistently higher in dairy-type cattle than beef-type cattle (Supplementary Table 4.5). In North America, dairy calves are typically housed indoors in confinement whereas most beef calves are on pasture until weaning. Dairy calves that are fed in feedlots for meat production are moved to calf grower operations as they come out of the hutch at 1 day of age (FHMS personal communication). In calf grower operations, dairy-type calves are housed in pens which may promote the transmission of AMR bacteria as per in beef-type cattle backgrounded in feedlots. It is also possible that these dairy calves were administrated more antimicrobials than beef-type calves of a similar age to assist their immature immune system to fight bacterial infections that may take place in the growing operation.
Low levels of AMR in M. haemolytica isolated from newly arrived feedlot cattle has been previously reported, with few studies investigating AMR in P. multocida and H. somni. Mannheimia haemolytica and P. multocida resistance to TIL and TUL were very low (up to 6.4%) in beef cattle compared to dairy cattle, most likely because macrolides are less frequently administrated to ranch beef calves than dairy calves (56–58). Even though the use of TYLT is less prevalent than TIL and TUL in ranch beef calves (58), M. haemolytica and P. multocida had high TYLT MICs, a phenomenon also observed in dairy calves. Previous surveillance studies have also reported high TYLT MICs in these two bacterial species in beef cattle at arrival and during the feeding period (6, 46). Further studies are required to determine if M. haemolytica and P. multocida exhibit some degree of intrinsic resistance to TYLT as a result of up-regulation of antimicrobial efflux systems (59) or impairment of cellular uptake as per AMGs (60). Mannheimia haemolytica obtained from cattle at feedlot entry exhibited no resistance to TUL a year after it was approved for the control of BRD in Canada (46). It is possible that the slightly higher resistance (i.e., from 0 to 3.6%) observed in the present study reflects the increased use of TUL, but more isolates should be collected over a longer period of time to confirm this trend.
Macrolide resistance phenotype (TIL and TUL) appeared to be due to the presence of erm(42) and/or the macrolide efflux protein and phosphotransferase gene pair msr(E)-mph(E) in M. haemolytica and P. multocida isolates. Three macrolide resistant P. multocida isolates possessed the A2058G mutation in 23S rRNA. Macrolide resistance due to rRNA mutations is well-documented in bacteria with a homogeneous or heterogeneous presence in rrn operons (61). In H. somni, erm(42) and/or erm(F) genes were present, but this did not appear to be linked to macrolide MICs. Furthermore, TYLT did not seem to be a reliable phenotypic indicator of macrolide resistance for Pasteurellaceae species isolated in this study due to high MIC values observed (Supplementary Tables 3.1, 3.2).
As previously reported, the resistance to TIO was extremely low for P. multocida (1/515, 0.2%) and absent in M. haemolytica (16, 47) and H. somni. These results probably reflect the limited use of TIO in beef cattle (58). Third generation cephalosporins are more frequently administrated to dairy cattle than to beef cattle (56, 57), yet resistance to this drug was not observed in BRD bacteria isolated from dairy calves. The overall resistance to β-lactam antimicrobials was relatively low in beef and dairy cattle, even though this drug class is commonly used in both (56–58). In our study, all M. haemolytica isolates that had AMP MICs > 16 μg/mL and all H. somni with MICs of 1–2 μg/mL harbored the blaROB−1 gene. Furthermore, one M. haemolytica with MIC values of 0.25 μg/mL had blaROB−1, and another isolate with similar MIC value possessed blaOXA−2. In Pasteurellaceae species, blaROB−1 is typically plasmid-associated (62, 63).
Resistance to OXY was the highest resistance observed across Pasteurellaceae isolated from both cattle types (10 and 46.4% in beef and dairy cattle types, respectively), which likely reflects the frequent use of TETs (57, 58). Levels of TET-resistance in Pasteurellaceae are even high in feedlot cattle raised without the use of antimicrobials (45). Considering the importance of TETs for the treatment and prevention of histophilosis in western Canada (53), the high level of OXY resistance (70.5%) in H. somni isolates from dairy cattle is worrisome. Our results suggest that antimicrobials other than TETs would be more effective for the treatment of H. somni infections in feedlot dairy calves. The ARG tet(H) was always present in OXY-resistant isolates, a finding consistent with that of others (14, 45). Previous studies have identified tet(H) in AMR M. haemolytica from clinical BRD cases (64), and it is also frequently found in P. multocida (59) and H. somni (65).
High FQs resistance was observed in M. haemolytica and P. multocida isolates from dairy calves. Danofloxacin is not approved for use in lactating dairy cows (56, 57) but it is approved for dairy calves (66). Resistance to FQ was associated with mutations in the QRDR including DNA-gyrase encoded by the gyrA and gyrB genes and topoisomerase IV encoded by parC and parE (67–69).
Frequent administration of FFN has been reported in dairy but not in beef cattle (57, 58). Such antimicrobial usage may explain the higher FFN resistance observed in dairy M. haemolytica and P. multocida isolates, and higher MICs in H. somni isolates from dairy compared to beef cattle (Supplementary Figure 1.3.3). This resistance and/or high MIC corresponded with the presence of floR as inferred from sequenced isolates from these three species.
Aminocyclitols are infrequently used in beef or dairy cattle (56–58) yet, H. somni (dairy isolates) and P. multocida (beef and dairy isolates) exhibited 22, 8.1, and 19% resistance to SPE, respectively. Co-resistance with other antimicrobials that travel on the same MGE as SPE likely accounts for this resistant phenotype (45). Resistance to SPE was conferred by the aadA31 gene as previously described (70). In accordance with previous studies (45, 59), linkage of AMG resistance genes aph(3″)-Ib, aph(6)-Id, aph(3′)-Ia, and sulfonamide resistance gene sul2 was found in M. haemolytica, P. multocida, and H. somni. The GEN-resistance determinant aac(3)-IVa also co-occurred with aminoglycoside and sulfonamide resistance genes in H. somni depicting GEN MIC > 8 μg/mL. In the isolates harboring these groups of AMR determinants, floR and erm(42) were also frequently clustered with the AMG-associated ARGs and sul2 genes. Co-existence of these ARGs along with tet(H) is a hallmark of ICE (45, 59).
Mannheimia haemolytica serotype A2 isolates from dairy-type cattle were more frequently associated with AMR than serotype A1 and A6 isolate from beef-type cattle. The so-called “virulent M. haemolytica serotypes” A1 and A6 have been linked to higher AMR in beef cattle (14, 71). However, it is not known if there is a true association between M. haemolytica serotype and AMR or simply virulent serotypes are more frequently exposed to antimicrobials due to treatment for BRD. The results observed in this study may be the consequence of a higher prevalence of serotype A2 among healthy animals under the high selective pressure of AMU in dairy prior to entry into the feedlot environment.
Jelinski et al. (11) proposed an SIR scheme for M. bovis isolated from bovine respiratory samples. Application of these criteria to M. bovis isolates in our study indicated high levels of resistance to TIP (100% both), TIL (100% beef and 99% dairy), and GAM (97.5% beef and 97% dairy). High MIC values for macrolides in M. bovis isolates from dairy and beef have been reported in Europe, Asia, and North America (11, 72). Macrolides are not frequently administrated to ranch calves in beef cow operations (58), which contrasts to the observed high macrolide MICs for M. bovis regardless of cattle type in our study. Antimicrobial resistance in M. bovis is mediated by mutations and not genes, possibly imposing a lower fitness burden, allowing the persistence of AMR traits regardless of AMU. Based on our results, TUL, ENRO, FFN, and TETs may be more effective against M. bovis than TIP, TIL, or GAM. However, the use of TET to treat clinical disease caused by M. bovis should be undertaken with caution since other authors have proposed a possible linkage between TETs and chronic pneumonia and polyarthritis syndrome in feedlots (73, 74). Nevertheless, it is not practically known which bacteria are specifically causing BRD in any one individual animal and frequently multiple bacteria can be isolated from fatal BRD cases (75). Therefore, it is difficult for veterinary practitioners to make any specific recommendations as it is not known which pathogen(s) is causing clinical disease. The odds of isolating M. bovis from beef and dairy-type cattle were 2.52 and 2.26 times higher, respectively, in the second year as compared to the first year of our study. During the first sampling year, a commercial media for the isolation of M. bovis was used (broth and agar; cat. no. TP90 and PM80, Dalynn Biologicals). Since M. bovis colonies growing on this commercial media often did not display the typical fried-egg morphology (76), a different media was used during the second sampling year (23). As a consequence, it is not possible to know if the difference in the M. bovis recovery was a consequence of inter-year variability.
Twenty-five and 68% of M. haemolytica and P. multocida isolates from dairy-type calves were MDR. As aforementioned, AMU is greater in dairy-type cattle as compared to beef-type cattle (77). Studies have suggested that feedlots are an environment that promote/amplify AMR via various means including through metaphylaxis (78, 79), serving as a reservoir of AMR bacteria and genes, selection through antimicrobial metabolites in manure (80, 81), and manure enriching the soil microbiome in AMR bacteria (82). It is known that metaphylaxis upon feedlot arrival decreases BRD morbidity and mortality (53) but it may select for AMR bacteria (78). Bovine respiratory disease Pasteurellaceae can persist in the farm and feedlot environment (83, 84) and be transmitted amongst different herds (85). In North America, large commercial feedlots receive a constant flow of animals and pens are usually cleaned (not disinfected) of manure/bedding once or twice per year. This constant occupancy of cattle in the feedlot may promote the persistence of AMR bacteria in the environment. Some feedlots feed both beef and dairy-type cattle, resulting in cattle with significantly different AMU backgrounds being housed in close proximity. Many of the MDR Pasteurellaceae harbor ARGs on ICE and it is unknown if bacteria carrying these elements increase and spread among cattle within the feedlot, a possibility that deserves further evaluation.
Conclusion
This is the first published study in Alberta documenting AMR in four major bacterial species involved in the BRD complex isolated from beef and dairy-type cattle on feedlot arrival. Our findings show marked differences in bacterial isolation and AMR levels in bacterial members of the BRD complex between dairy and beef cattle types. Moreover, MDR M. haemolytica, P. multocida, and H. somni isolates presenting AMR phenotypes indicative of the presence of ICE were isolated more often from dairy-type than beef-type cattle. These results raise the question of whether feedlot AMU and AMR should be reported by cattle type which could help to evaluate if the higher prevalence of ICE-related AMR is linked to higher BRD treatment failure and mortality. Additionally, an association between higher AMR and feedlot-backgrounded beef-type cattle was reported. Macrolides may not be an efficacious treatment choice for M. bovis (in beef and dairy-type cattle) or H. somni (dairy) infections in feedlot cattle in Alberta. Considering that antimicrobial therapy is essential for the prevention, control, and treatment of BRD in feedlot cattle, our study highlights the continued need for AMU and AMR surveillance of BRD-related bacteria in feedlot cattle to help inform veterinarians on treatment protocol decisions that promote prudent drug use.
Data Availability Statement
The data presented in the study are deposited in the NCBI repository, accession number PRJNA720670.
Ethics Statement
The animal study was reviewed and approved by the Lethbridge Research Centre Animal Care and Use Committee (Protocol #1641, Jan 18th, 2017) and was conducted according to the Canadian Council of Animal Care Guidelines. Written informed consent for participation was not obtained from the owners because Consent was provided verbally as a result of communication between veterinary practitioners and their clients.
Author Contributions
JV, CB, SJH, SH, CD, SG, and TM: concept and design of the study. CB, CD, JV, SH, and SJH: sample collection. CL, SA-L, RH, and RZ: data acquisition. RZ, SA-L, and RD: analysis of data. RZ, SA-L, and MA: interpretation of data. SA-L: drafting the manuscript. CB, CD, CL, JV, MA, RD, RH, RZ, SG, SH, SJH, and TM: critical review of the manuscript including the final version. All authors contributed to the article and approved the submitted version.
Funding
This project was funded by Alberta Beef Producers (ABP) and Alberta Livestock and Meat Agency Ltd. (ALMA). Project number ANH.09.16.
Conflict of Interest
CB is part owner and managing partner of Feedlot Health Management Services Ltd. and Southern Alberta Veterinary Services. SJH is an employee at Feedlot Health Management Services Ltd., Okotoks, Alberta, Canada. Feedlot Health is a private company that provides expert consultation regarding production and management of feedlot cattle and calf grower calves, including developing veterinary protocols to support animal health. They also conduct in-house and contract research related to dairy calf grower and feedlot production.
The remaining authors declare that the research was conducted in the absence of any commercial or financial relationships that could be construed as a potential conflict of interest.
Acknowledgments
This work is an excellent illustration of academia and industry collaboration. Its completion could not have been possible without the contribution of all professionals who were involved in it in one way or another. We would like to thank Cheyenne Conrad, Ruth Barbieri, Brendon DeGroot, Sujeema Abeysekara, Wendi Smart (AAFC), Homayoun Zahiroddini (AAF), and Taylor Davedow (University of Manitoba) for their technical assistance in the laboratory; to all the personnel from the veterinary practices and feedlots involved in this project; to Dr. Murray Jelinski, Andrea Kinnear, and Karen Gesy (Western College of Veterinary Medicine, University of Saskatchewan) for their expertise on Mycoplasma bovis; and to Dr. Diego Nobrega (University of Guelph) for his expertise on epidemiologic analysis.
Supplementary Material
The Supplementary Material for this article can be found online at: https://www.frontiersin.org/articles/10.3389/fvets.2021.692646/full#supplementary-material
Abbreviations
A, auction; AF, arrived from; AMG, aminoglycoside; AMP, ampicillin; AMR, antimicrobial resistance; AMU, antimicrobial use; ARG, antimicrobial resistance gene; B, backgrounding; BHI, brain-heart infusion; BRD, bovine respiratory disease; CI, Confidence Interval; CLSI, Clinical an Laboratory Standards Institute; cPCR, conventional PCR; CTET, chlortetracycline; DANO, danofloxacin; DNPS, deep nasopharyngeal swab; ENRO, enrofloxacin; FFN, florfenicol; FQ, fluoroquinolone; GLMM, generalized linear mixed model; GAM, gamithromycin; GEN, gentamycin; MDR, multidrug resistance; ICE, integrative and conjugative element; MGE, mobile genetic element; MIC, minimum inhibitory concentration; NEO, neomycin; OR, odds ratio; OXY, oxytetracycline; PEN, penicillin; qPCR, quantitative PCR; QRDR, quinolone resistant determining region; RD, ranch direct; RE, random effect; RFID, radio frequency identification; SIR, susceptible, intermediate, resistant; SPE, spectinomycin; TC, truck cluster; TET, tetracycline; TIL, tilmicosin; TIO, ceftiofur; TIP, tildipirosin; TMR, total mixed ration; TUL, tulathromycin; TYLT, tylosin tartrate; WGS, whole genome sequencing.
References
1. United States Department of Agriculture. Part IV: Health and Health Management on U.S. Feedlots With a Capacity of 1,000 or More Head. Fort Collins, CO: United States Department of Agriculture (2013).
2. Lhermie G, Chiu LV, Kaniyamattam K, Tauer LW, Scott HM, Grohn YT. Antimicrobial policies in United States beef production: choosing the right instruments to reduce antimicrobial use and resistance under structural and market constraints. Front Vet Sci. (2019) 6:245. doi: 10.3389/fvets.2019.00245
3. Jim K. Impact of bovine respiratory disease (BRD) from the perspective of the Canadian beef producer. Anim Health Res Rev. (2009) 10:109–10. doi: 10.1017/S1466252309990119
4. Hilton WM. BRD in 2014: where have we been, where are we now, and where do we want to go? Anim Health Res Rev. (2014) 15:120–2. doi: 10.1017/S1466252314000115
5. Watts JL, Sweeney MT. Antimicrobial resistance in bovine respiratory disease pathogens: measures, trends, and impact on efficacy. Vet Clin North Am Food Anim Pract. (2010) 26:79–88. doi: 10.1016/j.cvfa.2009.10.009
6. Anholt RM, Klima C, Allan N, Matheson-Bird H, Schatz C, Ajitkumar P, et al. Antimicrobial susceptibility of bacteria that cause bovine respiratory disease complex in Alberta, Canada. Front Vet Sci. (2017) 4:207. doi: 10.3389/fvets.2017.00207
7. Taylor JD, Fulton RW, Lehenbauer TW, Step DL, Confer AW. The epidemiology of bovine respiratory disease: what is the evidence for preventive measures? Can Vet J. (2010) 51:1351–9.
8. Smith RA, Step DL, Woolums AR. Bovine respiratory disease: looking back and looking forward, what do we see? Vet Clin North Am Food Anim Pract. (2020) 36:239–51. doi: 10.1016/j.cvfa.2020.03.009
9. DeDonder KD, Apley MD. A literature review of antimicrobial resistance in pathogens associated with bovine respiratory disease. Anim Health Res Rev. (2015) 16:125–34. doi: 10.1017/S146625231500016X
10. Portis E, Lindeman C, Johansen L, Stoltman G. A ten-year (2000-2009) study of antimicrobial susceptibility of bacteria that cause bovine respiratory disease complex–Mannheimia haemolytica, Pasteurella multocida, and Histophilus somni–in the United States and Canada. J Vet Diagn Invest. (2012) 24:932–44. doi: 10.1177/1040638712457559
11. Jelinski M, Kinnear A, Gesy K, Andrés-Lasheras S, Zaheer R, Weese S, et al. Antimicrobial sensitivity testing of Mycoplasma bovis isolates derived from western Canadian feedlot cattle. Microorganisms. (2020) 8:124. doi: 10.3390/microorganisms8010124
12. Klima CL, Zaheer R, Cook SR, Booker CW, Hendrick S, Alexander TW, et al. Pathogens of bovine respiratory disease in North American feedlots conferring multidrug resistance via integrative conjugative elements. J Clin Microbiol. (2014) 52:438–48. doi: 10.1128/JCM.02485-13
13. Cameron A, McAllister TA. Antimicrobial usage and resistance in beef production. J Anim Sci Biotechnol. (2016) 7:68. doi: 10.1186/s40104-016-0127-3
14. Klima CL, Alexander TW, Read RR, Gow SP, Booker CW, Hannon S, et al. Genetic characterization and antimicrobial susceptibility of Mannheimia haemolytica isolated from the nasopharynx of feedlot cattle. Vet Microbiol. (2011) 149:390–8. doi: 10.1016/j.vetmic.2010.11.018
15. Noyes NR, Benedict KM, Gow SP, Booker CW, Hannon SJ, McAllister TA, et al. Mannheimia haemolytica in feedlot cattle: prevalence of recovery and associations with antimicrobial use, resistance, and health outcomes. J Vet Intern Med. (2015) 29:705–13. doi: 10.1111/jvim.12547
16. Guo Y, McMullen C, Timsit E, Hallewell J, Orsel K, van der Meer F, et al. Genetic relatedness and antimicrobial resistance in respiratory bacteria from beef calves sampled from spring processing to 40 days after feedlot entry. Vet Microbiol. (2020) 240:108478. doi: 10.1016/j.vetmic.2019.108478
17. McEwen SA, Fedorka-Cray PJ. Antimicrobial use and resistance in animals. Clin Infect Dis. (2002) 1:S93–106. doi: 10.1086/340246
18. Jim GK, Booker CW, Ribble CS, Guichon PT, Thorlakson BE. A field investigation of the economic impact of respiratory disease in feedlot calves. Can Vet J. (1993) 34:668–73.
19. Boerlin P, Reid-Smith RJ. Antimicrobial resistance: its emergence and transmission. Anim Health Res Rev. (2008) 9:115–26. doi: 10.1017/S146625230800159X
20. Mather AE, Reeve R, Mellor DJ, Matthews L, Reid-Smith RJ, Dutil L, et al. Detection of rare antimicrobial resistance profiles by active and passive surveillance approaches. PLoS ONE. (2016) 11:e0158515. doi: 10.1371/journal.pone.0158515
21. Hannon SJ, Brault SA, Otto SJG, Morley PS, McAllister TA, Booker CW, et al. Feedlot cattle antimicrobial use surveillance network: a Canadian journey. Front Vet Sci. (2020) 7:596042. doi: 10.3389/fvets.2020.596042
22. Olfert E, Cross B, McWilliam A. Guide to the Care and Use of Experimental Animals. Ottawa, ON: Canadian Council on Animal Care (1993).
23. Andres-Lasheras S, Zaheer R, Ha R, Lee C, Jelinski M, McAllister TA. A direct qPCR screening approach to improve the efficiency of Mycoplasma bovis isolation in the frame of a broad surveillance study. J Microbiol Methods. (2019) 169:105805. doi: 10.1016/j.mimet.2019.105805
24. Angen O, Thomsen J, Larsen LE, Larsen J, Kokotovic B, Heegaard PM, et al. Respiratory disease in calves: microbiological investigations on trans-tracheally aspirated bronchoalveolar fluid and acute phase protein response. Vet Microbiol. (2009) 137:165–71. doi: 10.1016/j.vetmic.2008.12.024
25. Miflin JK, Blackall PJ. Development of a 23S rRNA-based PCR assay for the identification of Pasteurella multocida. Lett Appl Microbiol. (2001) 33:216–21. doi: 10.1046/j.1472-765x.2001.00985.x
26. Angen O, Ahrens P, Tegtmeier C. Development of a PCR test for identification of Haemophilus somnus in pure and mixed cultures. Vet Microbiol. (1998) 63:39–48. doi: 10.1016/S0378-1135(98)00222-3
27. Klima CL, Zaheer R, Briggs RE, McAllister TA. A multiplex PCR assay for molecular capsular serotyping of Mannheimia haemolytica serotypes 1, 2, and 6. J Microbiol Methods. (2017) 139:155–60. doi: 10.1016/j.mimet.2017.05.010
28. Dohoo I, Martin W, Stryhn H. Veterinary Epidemiologic Research. 2nd ed. Charlottetown, Prince Edward Island, Canada: Ver Inc. (2014).
29. Institute CaLS. Performance Standards for Antimicrobial Disk and Dilution Susceptibility Tests for Bacteria Isolated From Animals; Approved Standard. 4th ed. CLSI document VET01-4. Wayne, PA: Institute CaLS (2013).
30. Institute CaLS. Performance Standards for Antimicrobial Disk and Dilution Susceptibility Tests for Bacteria Isolated From Animals. 4th ed. CLSI document VET08. Wayne, PA: Institute CaLS (2018).
31. (CLSI) CaLSI. Understanding Susceptibility Test Data as a Component of Antimicrobial Stewardship in Veterinary Settings. 1st ed. CLSI document VE09. Wayne, PA: CaLSI (2019).
32. Institute CaLS. Understanding Susceptibility Test Data as a Component of Antimicrobial Stewardship in Veterinary Settings. 1st ed. CLSI document VET09. Wayne, PA: Institute CaLS (2019).
33. Rosenbusch RF, Kinyon JM, Apley M, Funk ND, Smith S, Hoffman LJ. In vitro antimicrobial inhibition profiles of Mycoplasma bovis isolates recovered from various regions of the United States from 2002 to 2003. J Vet Diagn Invest. (2005) 17:436–41. doi: 10.1177/104063870501700505
34. Hannan PC. Guidelines and recommendations for antimicrobial minimum inhibitory concentration (MIC) testing against veterinary mycoplasma species. Vet Res. (2000) 31:373–95. doi: 10.1051/vetres:2000100
35. Ayling RD, Rosales RS, Barden G, Gosney FL. Changes in antimicrobial susceptibility of Mycoplasma bovis isolates from Great Britain. Vet Rec. (2014) 175:486. doi: 10.1136/vr.102303
36. Institute CaLS. Generation, Presentation, and Application of Antimicrobial Susceptibility Test Data for Bacteria of Animal Origin; A Report. CLSI document VET05-R. Wayne, PA: Institute CaLS (2011).
37. Seemann T. Shovill. Available online at: https://github.com/tseemann/shovill (accessed March 2021).
38. Bankevich A, Nurk S, Antipov D, Gurevich AA, Dvorkin M, Kulikov AS, et al. SPAdes: a new genome assembly algorithm and its applications to single-cell sequencing. J Comput Biol. (2012) 19:455–77. doi: 10.1089/cmb.2012.0021
39. Seemann T. Prokka: rapid prokaryotic genome annotation. Bioinformatics. (2014) 30:2068–9. doi: 10.1093/bioinformatics/btu153
40. Heinze G, Wallisch C, Dunkler D. Variable selection - a review and recommendations for the practicing statistician. Biom J. (2018) 60:431–49. doi: 10.1002/bimj.201700067
41. Taylor JD, Fulton RW, Lehenbauer TW, Step DL, Confer AW. The epidemiology of bovine respiratory disease: what is the evidence for predisposing factors? Can Vet J. (2010) 51:1095–102.
42. Galili T, O'Callaghan A. Introduction to Heatmaply. (2020). Available online at: https://cran.r-project.org/web/packages/heatmaply/vignettes/heatmaply.html (accessed August 10, 2020).
43. Eidam C, Poehlein A, Leimbach A, Michael GB, Kadlec K, Liesegang H, et al. Analysis and comparative genomics of ICEMh1, a novel integrative and conjugative element (ICE) of Mannheimia haemolytica. J Antimicrob Chemother. (2015) 70:93–7. doi: 10.1093/jac/dku361
44. Michael GB, Kadlec K, Sweeney MT, Brzuszkiewicz E, Liesegang H, Daniel R, et al. ICEPmu1, an integrative conjugative element (ICE) of Pasteurella multocida: analysis of the regions that comprise 12 antimicrobial resistance genes. J Antimicrob Chemother. (2012) 67:84–90. doi: 10.1093/jac/dkr406
45. Stanford K, Zaheer R, Klima C, McAllister T, Peters D, Niu YD, et al. Antimicrobial resistance in members of the bacterial bovine respiratory disease complex isolated from lung tissue of cattle mortalities managed with or without the use of antimicrobials. Microorganisms. (2020) 8:288. doi: 10.3390/microorganisms8020288
46. Alexander TW, Cook S, Klima CL, Topp E, McAllister TA. Susceptibility to tulathromycin in Mannheimia haemolytica isolated from feedlot cattle over a 3-year period. Front Microbiol. (2013) 4:297. doi: 10.3389/fmicb.2013.00297
47. Klima CL, Alexander TW, Hendrick S, McAllister TA. Characterization of Mannheimia haemolytica isolated from feedlot cattle that were healthy or treated for bovine respiratory disease. Can J Vet Res. (2014) 78:38–45.
48. Chase CC, Hurley DJ, Reber AJ. Neonatal immune development in the calf and its impact on vaccine response. Vet Clin North Am Food Anim Pract. (2008) 24:87–104. doi: 10.1016/j.cvfa.2007.11.001
49. Griffin D. Bovine pasteurellosis and other bacterial infections of the respiratory tract. Vet Clin North Am Food Anim Pract. (2010) 26:57–71. doi: 10.1016/j.cvfa.2009.10.010
50. Peel DS. The effect of market forces on bovine respiratory disease. Vet Clin North Am Food Anim Pract. (2020) 36:497–508. doi: 10.1016/j.cvfa.2020.03.008
51. Ribble CS, Meek AH, Shewen PE, Guichon PT, Jim GK. Effect of pretransit mixing on fatal fibrinous pneumonia in calves. J Am Vet Med Assoc. (1995) 207:616–9.
52. Stroebel C, Alexander T, Workentine ML, Timsit E. Effects of transportation to and co-mingling at an auction market on nasopharyngeal and tracheal bacterial communities of recently weaned beef cattle. Vet Microbiol. (2018) 223:126–33. doi: 10.1016/j.vetmic.2018.08.007
53. Brault SA, Hannon SJ, Gow SP, Warr BN, Withell J, Song J, et al. Antimicrobial use on 36 beef feedlots in western Canada: 2008-2012. Front Vet Sci. (2019) 6:329. doi: 10.3389/fvets.2019.00329
54. Timsit E, McMullen C, Amat S, Alexander TW. Respiratory bacterial microbiota in cattle: from development to modulation to enhance respiratory health. Vet Clin North Am Food Anim Pract. (2020) 36:297–320. doi: 10.1016/j.cvfa.2020.03.001
55. Scott MA, Woolums AR, Swiderski CE, Perkins AD, Nanduri B, Smith DR, et al. Whole blood transcriptomic analysis of beef cattle at arrival identifies potential predictive molecules and mechanisms that indicate animals that naturally resist bovine respiratory disease. PLoS ONE. (2020) 15:e0227507. doi: 10.1371/journal.pone.0227507
56. United States Department of Agriculture. Health Management Practices on U.S. Dairy Operations. Collins, CO: United States Department of Agriculture (2018).
57. Saini V, McClure JT, Leger D, Dufour S, Sheldon AG, Scholl DT, et al. Antimicrobial use on Canadian dairy farms. J Dairy Sci. (2012) 95:1209–21. doi: 10.3168/jds.2011-4527
58. Waldner CL, Parker S, Gow S, Wilson DJ, Campbell JR. Antimicrobial usage in western Canadian cow-calf herds. Can Vet J. (2019) 60:255–67.
59. Beker M, Rose S, Lykkebo CA, Douthwaite S. Integrative and conjugative elements (ICEs) in Pasteurellaceae species and their detection by multiplex PCR. Front Microbiol. (2018) 9:1329. doi: 10.3389/fmicb.2018.01329
60. Lovering A, Reeves D. Aminoglycosides and aminocyclitols. In: Clansey N, editor. Antibiotic and Chemotherapy. Elsevier Ltd. (2011). p. 145–69.
61. Vester B, Douthwaite S. Macrolide resistance conferred by base substitutions in 23S rRNA. Antimicrob Agents Chemother. (2001) 45:1–12. doi: 10.1128/AAC.45.1.1-12.2001
62. Kadlec K, Watts JL, Schwarz S, Sweeney MT. Plasmid-located extended-spectrum beta-lactamase gene blaROB-2 in Mannheimia haemolytica. J Antimicrob Chemother. (2019) 74:851–3. doi: 10.1093/jac/dky515
63. San Millan A, Escudero JA, Gutierrez B, Hidalgo L, Garcia N, Llagostera M, et al. Multiresistance in Pasteurella multocida is mediated by coexistence of small plasmids. Antimicrob Agents Chemother. (2009) 53:3399–404. doi: 10.1128/AAC.01522-08
64. Clawson ML, Murray RW, Sweeney MT, Apley MD, DeDonder KD, Capik SF, et al. Genomic signatures of Mannheimia haemolytica that associate with the lungs of cattle with respiratory disease, an integrative conjugative element, and antibiotic resistance genes. BMC Genomics. (2016) 17:982. doi: 10.1186/s12864-016-3316-8
65. Bhatt K, Timsit E, Rawlyk N, Potter A, Liljebjelke K. Integrative conjugative element ICEHs1 encodes for antimicrobial resistance and metal tolerance in Histophilus somni. Front Vet Sci. (2018) 5:153. doi: 10.3389/fvets.2018.00153
66. Sawant AA, Sordillo LM, Jayarao BM. A survey on antibiotic usage in dairy herds in Pennsylvania. J Dairy Sci. (2005) 88:2991–9. doi: 10.3168/jds.S0022-0302(05)72979-9
67. Ozawa M, Asai T, Sameshima T. Mutations in GyrA and ParC in fluoroquinolone-resistant Mannheimia haemolytica isolates from cattle in Japan. J Vet Med Sci. (2009) 71:493–4. doi: 10.1292/jvms.71.493
68. Kong LC, Gao D, Gao YH, Liu SM, Ma HX. Fluoroquinolone resistance mechanism of clinical isolates and selected mutants of Pasteurella multocida from bovine respiratory disease in China. J Vet Med Sci. (2014) 76:1655–7. doi: 10.1292/jvms.14-0240
69. Michael GB, Bosse JT, Schwarz S. Antimicrobial resistance in Pasteurellaceae of veterinary origin. Microbiol Spectr. (2018) 6:1–33. doi: 10.1128/microbiolspec.ARBA-0022-2017
70. Cameron A, Klima CL, Ha R, Gruninger RJ, Zaheer R, McAllister TA. A novel aadA aminoglycoside resistance gene in bovine and porcine pathogens. mSphere. (2018) 3:e00568–17. doi: 10.1128/mSphere.00568-17
71. Katsuda K, Kohmoto M, Mikami O. Relationship between serotype and the antimicrobial susceptibility of Mannheimia haemolytica isolates collected between 1991 and 2010. Prev Vet Med. (2013) 94:205–8. doi: 10.1016/j.rvsc.2012.09.015
72. Lysnyansky I, Ayling RD. Mycoplasma bovis: mechanisms of resistance and trends in antimicrobial susceptibility. Front Microbiol. (2016) 7:595. doi: 10.3389/fmicb.2016.00595
73. Holman DB, Yang W, Alexander TW. Antibiotic treatment in feedlot cattle: a longitudinal study of the effect of oxytetracycline and tulathromycin on the fecal and nasopharyngeal microbiota. Microbiome. (2019) 7:86. doi: 10.1186/s40168-019-0696-4
74. Hendrick SH, Bateman KG, Rosengren LB. The effect of antimicrobial treatment and preventive strategies on bovine respiratory disease and genetic relatedness and antimicrobial resistance of Mycoplasma bovis isolates in a western Canadian feedlot. Can Vet J. (2013) 54:1146–56.
75. Booker CW, Lubbers BV. Bovine respiratory disease treatment failure: impact and potential causes. Vet Clin North Am Food Anim Pract. (2020) 36:487–96. doi: 10.1016/j.cvfa.2020.03.007
76. Razin S, Oliver O. Morphogenesis of Mycoplasma and bacterial L-form colonies. J Gen Microbiol. (1961) 24:225–37. doi: 10.1099/00221287-24-2-225
77. Call DR, Davis MA, Sawant AA. Antimicrobial resistance in beef and dairy cattle production. Anim Health Res Rev. (2008) 9:159–67. doi: 10.1017/S1466252308001515
78. Snyder E, Credille B, Berghaus R, Giguère S. Prevalence of multi drug antimicrobial resistance in Mannheimia haemolytica isolated from high-risk stocker cattle at arrival and two weeks after processing. J Anim Sci. (2017) 95:1124–31. doi: 10.2527/jas.2016.1110
79. Woolums AR, Karisch BB, Frye JG, Epperson W, Smith DR, Blanton JJ, et al. Multidrug resistant Mannheimia haemolytica isolated from high-risk beef stocker cattle after antimicrobial metaphylaxis and treatment for bovine respiratory disease. Vet Microbiol. (2018) 221:143–52. doi: 10.1016/j.vetmic.2018.06.005
80. Agga GE, Schmidt JW, Arthur TM. Effects of in-feed chlortetracycline prophylaxis in beef cattle on animal health and antimicrobial-resistant Escherichia coli. Appl Environ Microbiol. (2016) 82:7197–204. doi: 10.1128/AEM.01928-16
81. Muller HC, Van Bibber-Krueger CL, Ogunrinu OJ, Amachawadi RG, Scott HM, Drouillard JS. Effects of intermittent feeding of tylosin phosphate during the finishing period on feedlot performance, carcass characteristics, antimicrobial resistance, and incidence and severity of liver abscesses in steers. J Anim Sci. (2018) 96:2877–85. doi: 10.1093/jas/sky166
82. Agga GE, Cook KL, Netthisinghe AMP, Gilfillen RA, Woosley PB, Sistani KR. Persistence of antibiotic resistance genes in beef cattle backgrounding environment over two years after cessation of operation. PLoS ONE. (2019) 14:e0212510. doi: 10.1371/journal.pone.0212510
83. Burriel AR. Isolation of Pasteurella haemolytica from grass, drinking water, and straw bedding used by sheep. Curr Microbiol. (1997) 35:316–8. doi: 10.1007/s002849900261
84. Neupane S, Nayduch D, Zurek L. House flies (Musca domestica) pose a risk of carriage and transmission of bacterial pathogens associated with bovine respiratory disease (BRD). Insects. (2019) 10:358. doi: 10.3390/insects10100358
Keywords: antimicrobial resistance, bovine respiratory disease, cross-sectional study, epidemiology, Mannheimia haemolytica, Mycoplasma bovis, Pasteurella multocida, Histophilus somni
Citation: Andrés-Lasheras S, Ha R, Zaheer R, Lee C, Booker CW, Dorin C, Van Donkersgoed J, Deardon R, Gow S, Hannon SJ, Hendrick S, Anholt M and McAllister TA (2021) Prevalence and Risk Factors Associated With Antimicrobial Resistance in Bacteria Related to Bovine Respiratory Disease—A Broad Cross-Sectional Study of Beef Cattle at Entry Into Canadian Feedlots. Front. Vet. Sci. 8:692646. doi: 10.3389/fvets.2021.692646
Received: 08 April 2021; Accepted: 31 May 2021;
Published: 01 July 2021.
Edited by:
Annamaria Pratelli, University of Bari Aldo Moro, ItalyReviewed by:
Tara G. McDaneld, United States Department of Agriculture, United StatesGetahun E. Agga, United States Department of Agriculture, United States
Copyright © 2021 Andrés-Lasheras, Ha, Zaheer, Lee, Booker, Dorin, Van Donkersgoed, Deardon, Gow, Hannon, Hendrick, Anholt and McAllister. This is an open-access article distributed under the terms of the Creative Commons Attribution License (CC BY). The use, distribution or reproduction in other forums is permitted, provided the original author(s) and the copyright owner(s) are credited and that the original publication in this journal is cited, in accordance with accepted academic practice. No use, distribution or reproduction is permitted which does not comply with these terms.
*Correspondence: Tim A. McAllister, dGltLm1jYWxsaXN0ZXJAYWdyLmdjLmNh