- 1Jiangsu Key Laboratory of Immunity and Metabolism, Department of Pathogen Biology and Immunology, Xuzhou Medical University, Xuzhou, China
- 2National Experimental Teaching Demonstration Center of Basic Medicine, Xuzhou Medical University, Xuzhou, China
- 3School of Life Sciences, Xuzhou Medical University, Xuzhou, China
- 4The First Clinical Medical College, Xuzhou Medical University, Xuzhou, China
- 5The Second Clinical Medical College, Xuzhou Medical University, Xuzhou, China
The deposition of Schistosoma japonicum (S. japonicum) eggs commonly induces inflammation, fibrosis, hyperplasia, ulceration, and polyposis in the colon, which poses a serious threat to human health. However, the underlying mechanism is largely neglected. Recently, the disorder of glucose and lipid metabolism was reported to participate in the liver fibrosis induced by the parasite, which provides a novel clue for studying the underlying mechanism of the intestinal pathology of the disease. This study focused on the metabolic reprogramming profiles of glucose and lipid in the colon of mice infected by S. japonicum. We found that S. japonicum infection shortened the colonic length, impaired intestinal integrity, induced egg-granuloma formation, and increased colonic inflammation. The expression of key enzymes involved in the pathways regulating glucose and lipid metabolism was upregulated in the colon of infected mice. Conversely, phosphatase and tensin homolog deleted on chromosome ten (PTEN) and its downstream signaling targets were significantly inhibited after infection. In line with these results, in vitro stimulation with soluble egg antigens (SEA) downregulated the expression of PTEN in CT-26 cells and induced metabolic alterations similar to that observed under in vivo results. Moreover, PTEN over-expression prevented the reprogramming of glucose and lipid metabolism induced by SEA in CT-26 cells. Overall, the present study showed that S. japonicum infection induces the reprogramming of glucose and lipid metabolism in the colon of mice, and PTEN may play a vital role in mediating this metabolic reprogramming. These findings provide a novel insight into the pathogenicity of S. japonicum in hosts.
Introduction
Schistosomiasis, a global neglected tropical disease (NTDS) caused by the organisms belonging to the genus Schistosoma, including S. japonicum, S. mansoni, and S. haematobium, is considered the second-most socially and economically devastating parasitic disease (1). As the most problematic of human helminthiases, schistosomiasis threatens more than 800 million people and affects more than 250 million people worldwide (2).
Migrating parasite eggs are regarded as the major pathogenic factor of schistosomiasis, contributing to the formation of tissue lesions, particularly in the intestinal tract (3). A large number of schistosome eggs trapped in the intestinal wall lead to the formation of egg granulomas and result in symptoms such as diarrhea, inflammation, fibrosis, hyperplasia, and polyposis (4). The immune mechanism of intestinal lesions in schistosomiasis has been studied previously (5). Polarized T-helper 2 (Th2)-mediated immune responses are responsible for the development of chronic intestinal pathologies induced by schistosome eggs and soluble egg antigens (SEA) (6). Many cell types, such as basophils, B cells, regulatory T cells (Treg), and dendritic cells (DCs), have been implicated in the induction or maintenance of Th2 responses against eggs and SEA (7–9). Furthermore, some independent studies in humans and rodents have demonstrated that fecal microbiome differences linked to host immune-regulation or inflammation are associated with parasitic infection (5, 10, 11).
Recently, many studies have focused on the reverse effect of schistosome infection on metabolism-related diseases and found that chronic S. japonicum infection or treatment with SEA could regulate host metabolism via Th2 responses (12, 13). As an important organ for digestion, absorption, and metabolism of dietary nutrients, the glucose and lipid metabolism in the intestinal tract is important for host health. However, the potential effect of schistosome infection on intestinal glucose and lipid metabolism remains unclear.
Phosphatase and tensin homolog deleted on chromosome ten (PTEN) is an important member of the protein tyrosine phosphatase (PTP) gene family (14). PTEN is a tumor suppressor gene and regulates cell metabolism (15). For instance, PTEN overexpression could inhibit glycolysis in tumor cells (16). A previous study showed that hepatic PTEN-knockout increased the triglyceride (TG) content, reduced apolipoprotein B (ApoB) protein mass, and promoted fatty liver development (17). We previously reported that the decreased PTEN expression is associated with the metabolic reprogramming events in hepatic fibrosis induced by S. japonicum (18). However, whether PTEN and its downstream metabolic pathway participate in the formation of intestinal lesions induced by S. japonicum remains elusive.
In the present study, a murine model of S. japonicum cercaria infection and an in vitro model of SEA treated CT-26 cells were used to explore the possible role of schistosome infection in intestinal glucose and lipid metabolism. We found that S. japonicum infection induced the reprogramming of glucose and lipid metabolism in the intestine of mice, which accompanied with the inactivation of PTEN pathway. Consistent with this, SEA treatment could mimic similar metabolic alterations in CT-26 cells that were inhibited by PTEN overexpression. These findings might provide a novel insight on the formation of intestinal lesions and developing a metabolism-targeted strategy for the treatment of schistosomiasis.
Materials and Methods
Parasite Preparation and Animal Studies
The cercariae of S. japonicum (Chinese strain) were obtained from freshwater snails (Oncomelania hupensis) that were provided by the National Institute of Parasitic Diseases, Chinese Center for Disease Control and Prevention (Shanghai, China). Female BALB/c mice were obtained from Shanghai Laboratory Animal Center (SLAC, Shanghai, China) and housed and maintained with a 12 h light/dark photoperiod and ad libitum access to water and food. Then, mice were randomly divided into 2 groups: control group and model group. In the model group, mice were percutaneously infected with 20 cercariae of S. japonicum. At 9 weeks post-infection, mice were euthanized by cervical dislocation. The intestinal tissues were collected and stored at−80°C for further analyses.
Preparation of SEA
Eggs were obtained from the livers of mice infected by S. japonicum for 9 weeks, and SEA were prepared as previously described (19). Briefly, the livers of infected mice were collected and cut into a number of pieces. Subsequently, the pieces were homogenized in LPS-free PBS on ice, filtered, washed, and centrifuged at 12,000 rpm for 15 min. Purified eggs were suspended in pre-cooled PBS containing 1 mM PMSF (Roche Diagnostics) and 2 μg/mL Leupeptin (Sigma) and homogenized on ice using a homogenizer (VirTis Co). The suspension was frozen/thawed several times and centrifuged at 12,000 rpm for 30 min at 4°C. A 0.22 μm filter was used to pass the supernatant and obtain SEA. The protein concentration of SEA was determined using the BCA Protein Assay Kit (Beyotime Biotech, Beijing, China).
Histopathological Analysis
For histopathological analysis, intestinal tissues were fixed in 4% paraformaldehyde, embedded in paraffin, and divided into sections of 5 μm thickness. Then, the sections were stained with hematoxylin and eosin (H&E) according to the standard procedure. All sections were imaged under a microscope (OLYMPUS IX51), and digital photographs were captured for further analysis.
Cell Lines and in vitro Treatment
Mouse colon cancer cell line CT-26 was purchased from Shanghai Cell Bank, Chinese Academy of Sciences and maintained in our lab. Cells (1 × 106/mL) were seeded in 6-well plates in DMEM (glutamine, high glucose) supplemented with penicillin (100 units/mL), streptomycin (100 μg/mL) and 10% heat-inactivated FBS at 37°C with 5% CO 2 in a humidified atmosphere. For treatment with SEA, cells were incubated with SEA (10 μg/mL) or PBS for 24 h. For co-treatment experiments, 24 h after transfection with PTEN plasmid, cells were incubated with SEA (10 μg/mL) or PBS for another 24 h. Cells were then harvested for further analysis.
Cell Transfection
To overexpress PTEN, the CT-26 cells were transfected with Flag-PTEN using Lipofectamine™ 2000 (Invitrogen) according to the manufacturer's instructions. The Flag-tagged full-length mouse PTEN gene complementary DNA (cDNA) in pECMV-Myc-Flag vector (Shanghai, China) was used for transfection with a plasmid encoding an Ampicillin gene. Cells transfected with only the pECMV-Myc-Flag plasmid were used as control. After 24 h of culture, the CT-26 cells were respectively transfected with 2 μg of plasmids for 24 h.
Western Blot Analysis
Western blot assays were performed as described previously (20). Total proteins were extracted from the pulverized intestine or cultured CT-26 cells in cell lysis buffer, and the concentration was determined using a BCA protein concentration assay kit (Beyotime Biotech, Beijing, China). A total of 40–80 μg of protein was resolved using 10 % SDS-PAGE gel, and separated proteins were electrotransferred to polyvinylidene difluoride membranes using a Bio-Rad electrophoresis system (Hercules, CA, USA). Western blot assays were performed using indicated primary antibodies specific for Occludin-2 (#48120, Cell Signaling Technology, USA, 1: 1000 dilution), ZO-1 (#8193, Cell Signaling Technology, USA, 1: 1000 dilution), the p85α subunit of Phosphatidylinositol 3-kinase (PI3K-p85α) (ab191606, abcam, UK, 1: 1000 dilution), p-AKT(ser473) (ab81283, abcam, UK, 1: 1000 dilution), p-AKT(ser129) (ab133458, abcam, UK, 1: 1000-1:10000 dilution), Total AKT(ab179463, abcam, UK, 1: 10000 dilution), β-actin (ab119716, abcam, UK, 1: 5000/1: 2000 dilution), PKM2 (#4053, Cell Signaling Technology, USA, 1: 1000 dilution), PGC-1α (ab54481, abcam, UK, 1: 1000 dilution), PPARα (WL00978, wanleibio, China, 1: 500-1: 1000 dilution), CPT-1a (#12252, Cell Signaling Technology, USA, 1: 1000 dilution) and SREBP (WL02093, wanleibio, China, 1: 1000-1: 1500 dilution). The immune reaction was performed using Clarity™ ECL western blot substrate (Bio-Rad, USA) and visualized using ChemiDoc Touch imaging system (Bio-Rad, USA).
Quantitative Real-Time PCR (qPCR)
Total RNA was extracted with TRIzol (Termo Fisher Scientifc, USA) from the pulverized colon or cultured CT-26 cells. Then 1 μg RNA for each sample was reverse-transcripted to cDNA using a high-capacity cDNA reverse transcription kit (Takara, Japan). qPCR was performed using the LightCycler® 480II detection system (Roche Applied Science, Penzberg, Germany). All primers are listed in Table 1. All experiments were performed in triplicate, and the relative expression of related genes was indicated by comparative cycling threshold (Ct) value normalized against an endogenous reference (β-actin) using the 2−ΔΔCt method.
Statistical Analysis
Data were analyzed using GraphPad Prism software 5.0 and are presented as mean ± SEM. Statistical significance was determined using the unpaired 2-tailed Student's t-test for a single variable and one-way analysis of variance (ANOVA) followed by the post hoc Tukey test for multiple comparisons. Values with p < 0.05 were considered statistically significant.
Results
Comparison of the Colonic Pathology in S. japonicum-Infected Mice vs. Uninfected Control Mice
To investigate the intestinal pathological changes after S. japonicum infection, 15 BALB/c mice (Model) were infected with 20 S. japonicum cercariae, while 15 uninfected gender and age-matched mice were used as controls. The colonic lesions were evaluated after 9 weeks post-infection. As shown in Figure 1A, compared to the control mice, the length of the colon in the infected mice was significantly shortened (p < 0.05). H&E staining showed that atypical changes such as irregular colonic glands, decreased goblet cells in the mucosa, and the presence of egg granulomas in the submucosa of infected mice indicated significant damage of the intestinal function (Figure 1B). In line with these changes, the colonic expression of occludin-2 and Zonula occludens 1 (ZO-1), the tight junction proteins crucial for the maintenance of intestinal barrier integrity, was decreased in infected mice (both p < 0.05, Figure 1C). Furthermore, we observed that S. japonicum infection increased the mRNA levels of proinflammatory cytokines TNF-α, IL-6, IL-1β, and Monocyte chemotactic protein-1 (MCP-1) along with anti-inflammatory cytokines Transforming growth factor-β (TGF-β) and IL-10 (both p < 0.01, Figure 1D) in the colon. These results suggest that S. japonicum infection could cause colonic injury in mice.
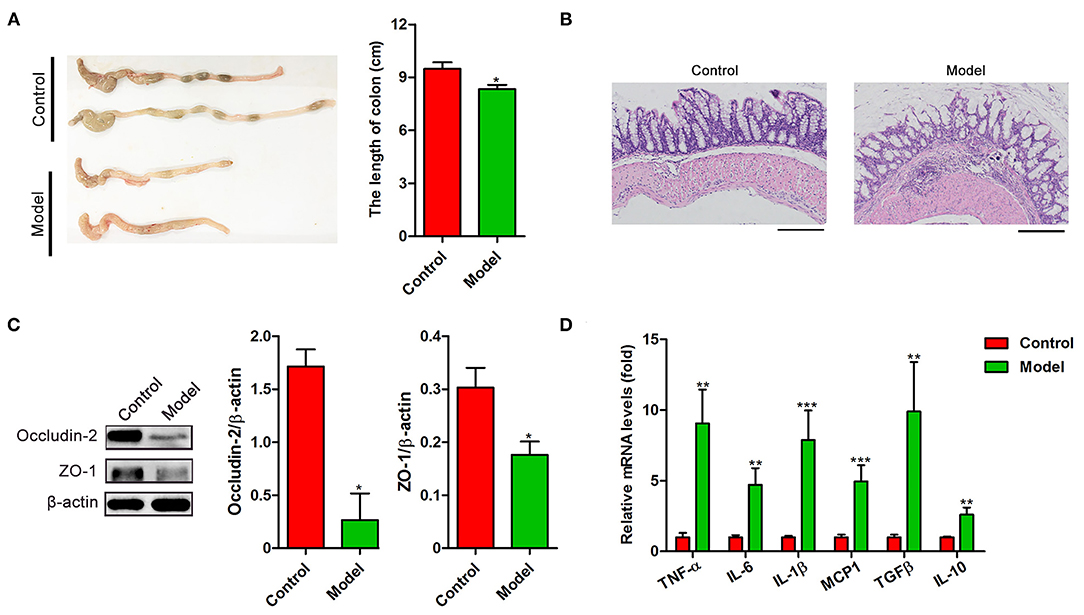
Figure 1. The intestinal pathological analysis and the cytokines expression of BALB/c mice infected with S. japonicum. Intestinal tissues were obtained from control mice or mice infected with S. japonicum for 9 weeks. (A) Representative photographs of colons (Left) and the length comparison of colon (right, n = 15). (B) Representative images of H&E stained colon. (C) The protein expression levels of occludin-2 and ZO-1(n = 4). Data are expressed as mean ± standard error of means. Similar results were observed in three independent experiments. (D) The expression of genes related inflammatory (TNF-α, IL-6, IL-1β, and MCP1) and anti-inflammatory cytokines (TGF-β and IL-10) (n = 4). Values are mean ± standard error of means. (Student's t-test: *p < 0.05, **p < 0.01, ***p < 0.001).
Expression Profile of Colonic Genes Regulating Glucose and Lipid Metabolism During S. japonicum Infection
Recently, several studies have demonstrated that S. japonicum infection could regulate hepatic glycolipid metabolism and inverse metabolism-related diseases (12, 20). Here, we profiled the changes in the expression of colonic genes involved in glucose and lipid metabolism caused by S. japonicum infection. As shown in Figure 2A, compared with the control mice, S. japonicum infected mice showed a higher expression of glycolytic pathway related proteins (Hypoxia-inducible factor 1α, HIF-1α; Pyruvate kinase isozyme type M2, PKM2), gluconeogenesis genes (Phosphoenolpyruvate carboxykinase 1, Pck1, and Glucose-6 phosphatase, G6pc), tricarboxylic acid cycle (TCA) enzymes (Citrate synthase, CS; Succinate dehydrogenase complex flavoprotein subunit A, SDHA), and glucose transporter 4 (GLUT4) (All p < 0.05). Other genes associated with these signaling pathways, such as Peroxisome proliferator-activated receptor-γ coactivator-1α (PGC-1α), Isocitrate Dehydrogenase 3 (IDH3G), and Glucose transporter 2 (GLUT2), did not demonstrate any remarkable changes. Consistent with these results, the protein expression of PKM2 was significantly upregulated in infected mice, and the PGC-1α protein expression was similar between control and S. japonicum infected mice (Figures 2B,C). Besides, S. japonicum infection increased the mRNA expression of fatty acid oxidation genes (Peroxisome proliferator-activated receptor alpha, PPARα; Carnitine palmitoyl transferase 1a, CTP-1a; Medium-chain acyl-CoA Dehydrogenase, MCAD; Cytochrome P450 proteins 4A10, CYP4A10 and Liver-fatty acid binding protein, L-FABP) and lipogenesis-related genes (Fatty acid synthase, FAS; Acetyl coenzyme A carboxylase 1, ACC1 and Stearoyl-CoA desaturase 1, SCD1) in the colon (All p < 0.05, Figure 3A). In line with this, the colonic protein expressions of CTP-1a and Sterol regulatory element-binding protein-1c (SREBP-1c) were significantly upregulated after S. japonicum infection (Figures 3B,C). However, S. japonicum infection did not affect PPARα colonic protein expression (Figures 3B,C). Together, these results indicate that S. japonicum infection promotes the expression of genes involved in glucose and lipid metabolism in the colon.
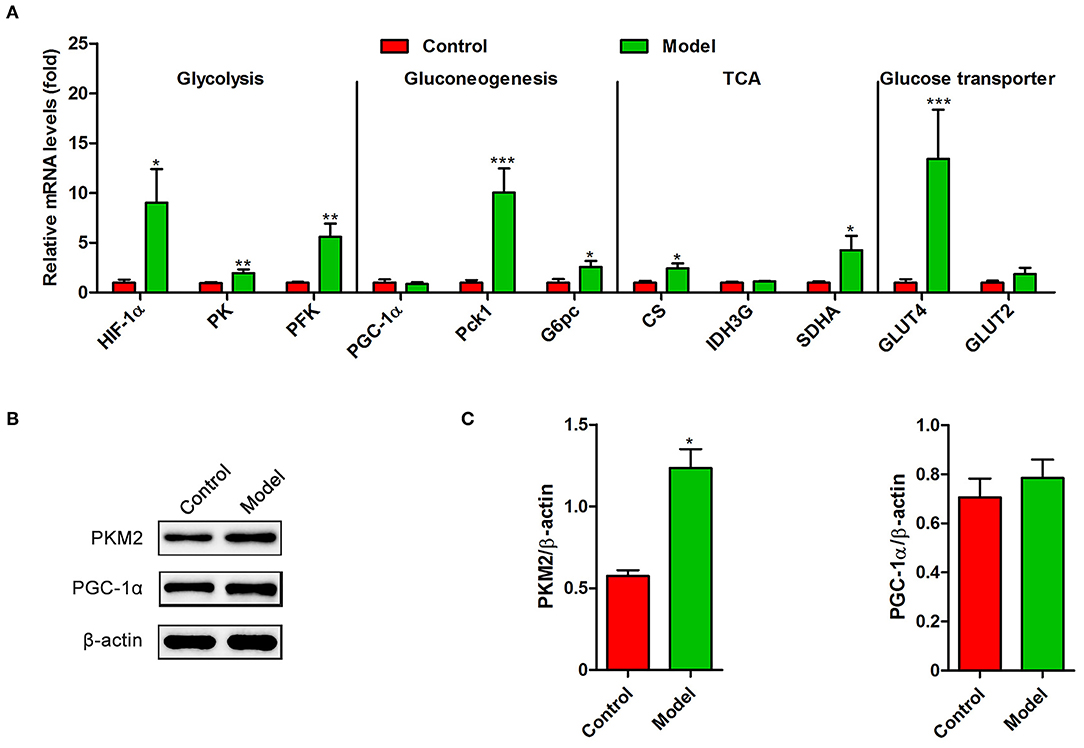
Figure 2. The expression profiling of glucose metabolism-related genes in the colon of S. japonicum-infected mice. Intestinal tissues were obtained from control mice or mice infected with S. japonicum for 9 weeks. (A) The mRNA levels of glycolysis-related genes (HIF-1α, PK and PFKFB3), gluconeogenesis-related genes (PGC-1α, Phosphoenolpyruvate carboxykinase 1 and G6pc), TCA-related genes (CS, SDHA, and IDH3G) and Glucose transporter-related genes (GLUT4 and GLUT2). (B,C) The protein expression level of PKM2 and PGC-1α. Data are expressed as mean ± standard error of means (n = 4). Similar results were observed in three independent experiments. (Student's t-test: *p < 0.05, **p < 0.01, ***p < 0.001).
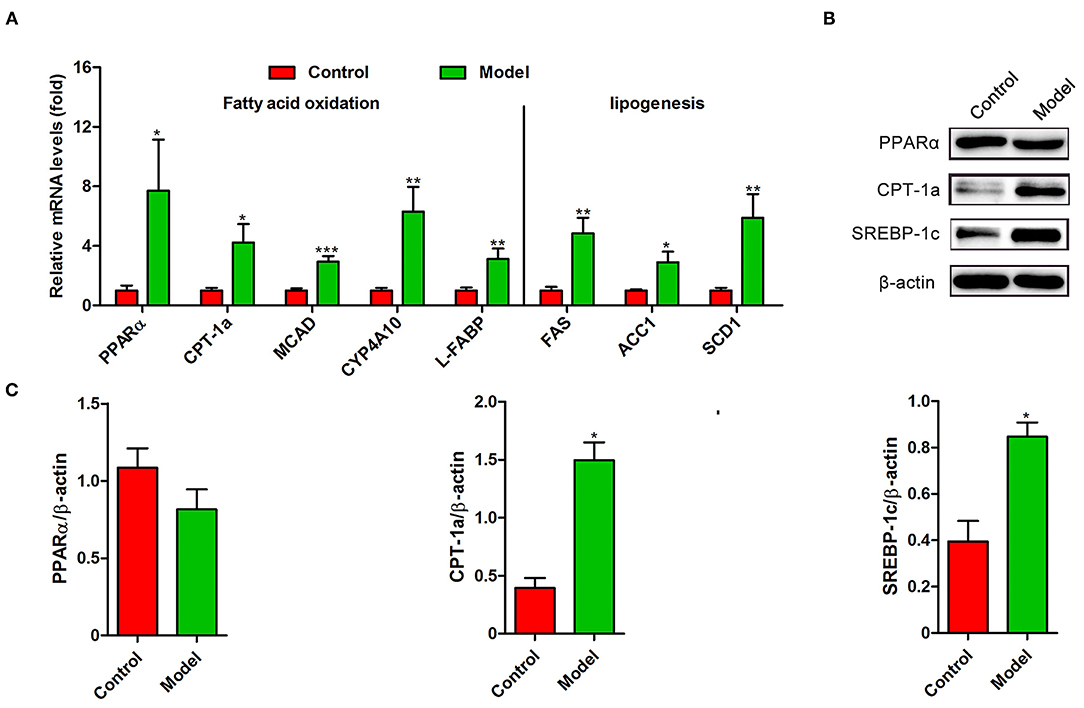
Figure 3. The expression profiling of lipid metabolism-related genes in the colon of S. japonicum-infected mice. (A) The mRNA levels of genes related to fatty acid (FA) oxidation (PPARα, CTP-1a, MCAD, CYP4A10 and L-FABP) and FA synthesis (FAS, ACC1 and SCD1) (n = 4). (B,C) The protein expression levels of PPARα, CTP-1a and SREBP-1c (n = 4). Data are expressed as mean ± standard error of means. Similar results were observed in three independent experiments. (Student's t-test: *p < 0.05, **p < 0.01, ***p < 0.001).
Inhibition of PTEN/PI3K/AKT Pathway in the Intestine Mediated by S. japonicum
We next investigated whether PTEN participates in the formation of intestinal lesion induced by S. japonicum. A decrease in the levels of PTEN was observed in the colon after S. japonicum infection (Figure 4). The expression of PI3K (p85α subunit) and the phosphorylated (Ser129) form of AKT (Figures 4A,B), which are important downstream signaling targets of PTEN, were significantly downregulated. However, no remarkable differences were observed in the phosphorylated (Ser473) form of AKT and total AKT protein levels in the colon between control mice and S. japonicum infected mice. Overall, these results indicate that the colonic PTEN/PI3K/AKT pathway was inhibited by S. japonicum infection.
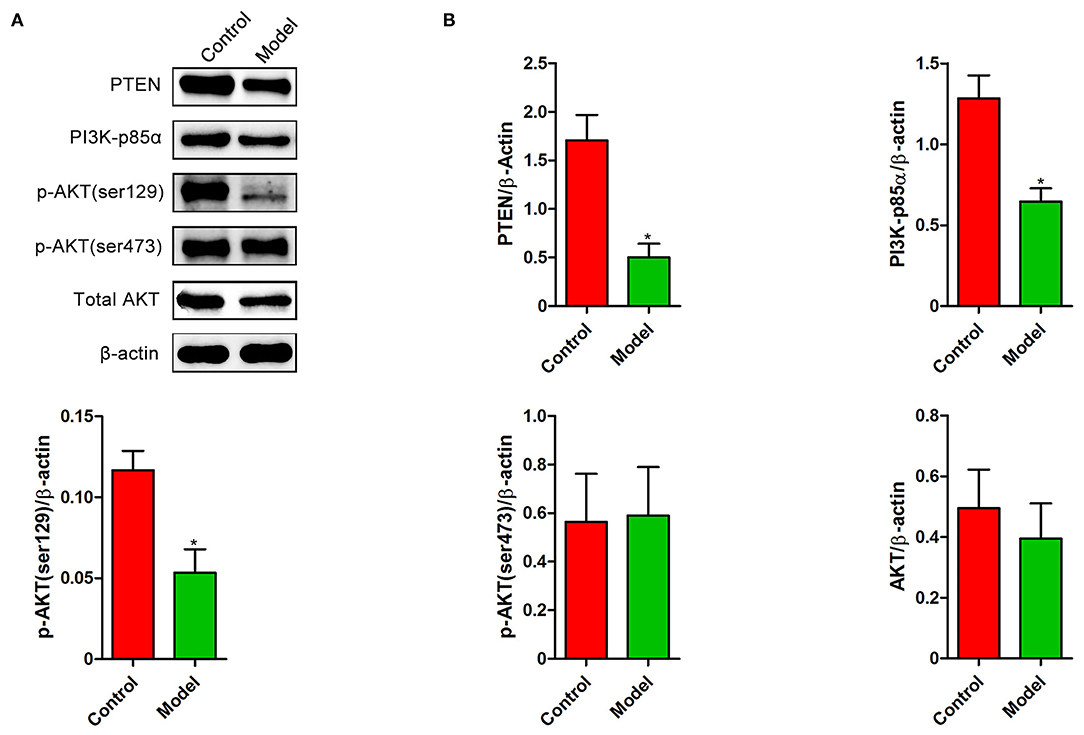
Figure 4. The expression changes of PTEN/PI3K/AKT pathway in the colon of S. japonicum-infected mice. Intestinal tissues were obtained from control mice or mice infected with S. japonicum for 9 weeks. (A,B) The protein expression levels of PTEN, PI3K (p85α subunits), p-AKT (ser129), p-AKT (ser473), and total AKT (n = 4). Data are expressed as mean ± standard error of means. Similar results were observed in three independent experiments. (Student's t-test: *p < 0.05).
The effect of SEA and PTEN Overexpression on Glucose and Lipid Metabolism in vitro
SEA secreted by the eggs are the primary pathogenic components of S. japonicum infection (20). We next stimulated CT-26 cells with SEA in vitro to detect the mechanisms underlying the aforementioned phenotypes. As shown in Figure 5, the SEA stimulation was associated with a decrease in the expression of PTEN (p < 0.05, Figures 5A,B), in accordance with the results observed under in vivo conditions. Meanwhile, the expression of PK, PFK, G6pc, CS, GLUT4, and Carnitine palmitoyl transferase 1a (CPT-1a) was significantly upregulated after exposure to SEA compared to that observed in the control group (all p < 0.05), which was consistent with our results observed under in vivo conditions. Importantly, overexpression of PTEN prevented SEA-mediated increase in the mRNA levels of aforementioned glucose and lipid metabolic genes, except GLUT4 (all p < 0.05, Figure 5C). Collectively, these results indicate that SEA may participate in the regulation of intestinal glucose and lipid metabolism after S. japonicum infection, and these effects can be attributed to the inhibition of PTEN.
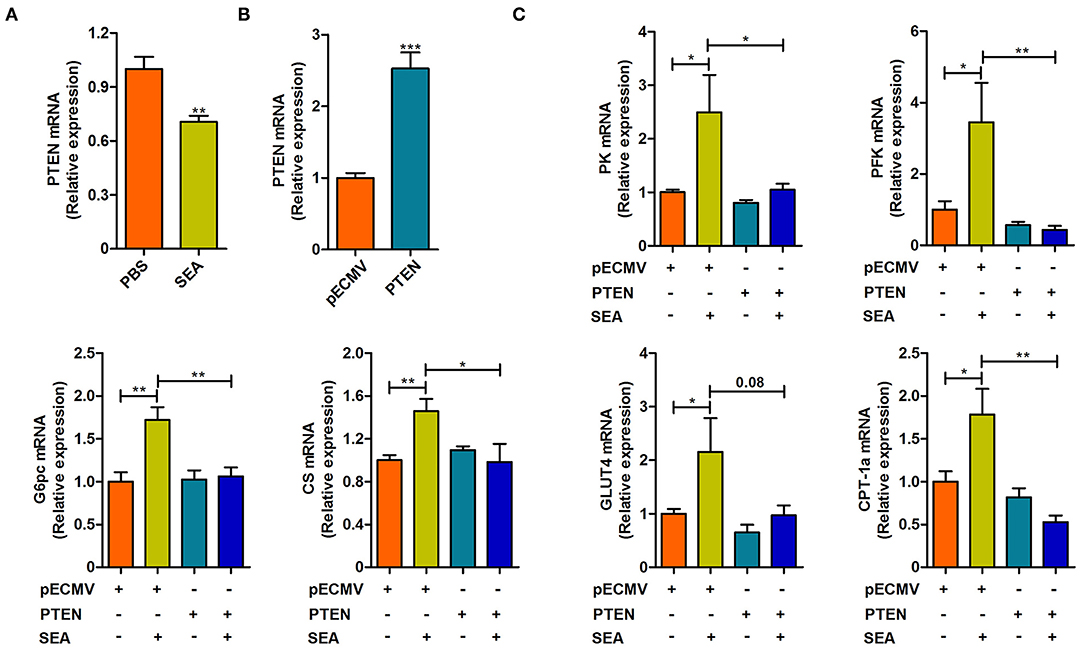
Figure 5. The effect of SEA treatment and PTEN overexpression on the reprogramming of glucose and lipid metabolism in CT-26 cells. (A) The mRNA level of PTEN in CT-26 cells stimulated with SEA (10 μg/ml) for 24 h prior to harvest. (B) The mRNA level of PTEN in CT-26 cells transfected with PTEN plasmid for 24 h prior to harvest. (C) the mRNA levels of metabolic genes in CT-26 cells transfected with indicated plasmids for 24 h, followed by treatment with SEA (10 μg/ml) for 24 h prior to harvest. Data are mean ± standard error of means. (one-way ANOVA: *p < 0.05, **p < 0.01, ***p < 0.001).
Discussion
The present study demonstrated that S. japonicum infection could impair colonic integrity, increase colonic inflammation, and induce the reprogramming of glucose and lipid metabolism in the colon of mice. The mechanism of metabolic reprogramming was demonstrated in CT-26 cells with SEA stimulation, which was mediated by PTEN inhibition. To our knowledge, this is the first study to investigate metabolic reprogramming in the intestine of mice infected with S. japonicum, which may provide a new perspective for understanding the formation of intestinal lesions induced by S. japonicum infection.
Schistosomiasis is a widely distributed chronic disease that threatens human health and social and economic development. The formation of intestinal lesions caused by the migration of parasite eggs is one of the main pathological characteristics of this disease (4). In the present study, we observed that the length was shorter and was accompanied by colonic epithelial edema. In response to these changes, we observed intestinal granulomas, impaired intestinal integrity, and increased production of pro-inflammatory cytokines in infected mice. A previous study showed that schistosomiasis could stimulate type 2 immune response (8, 21). Our results showed that the expression of anti-inflammatory cytokines (TGF-β and IL-10) in infected mice was higher than that in control mice, in agreement with previous reports. All of these results indicated that S. japonicum infection damaged the intestinal structure.
As an emerging field, immunometabolism investigates the interface between the metabolism and immune response and explores how metabolic pathways, especially glucose and lipid metabolism, govern the phenotype of the immune response. So far, many metabolic processes, as such glycolysis, the Krebs cycle, and fatty acid metabolism have been found to play a vital role in immune response (22). Activating the glycolytic pathway could shift immune cells (macrophages, Treg cells, B cells, and DC cells) from an anti-inflammatory status to a pro-inflammatory state. A shift in cell metabolism leading to fatty acid oxidation and oxidative phosphorylation demonstrates an opposite effect (23–25). The previously mentioned immune cells are involved in the induction of immune responses against schistosomiasis (7–9, 20). In accordance with the upregulation of intestinal pro-inflammatory and anti-inflammatory cytokines, the glycolysis, TCA, and fatty acid oxidation pathways were significantly activated in infected mice.
Recently, the reverse relationship between schistosome infection and metabolic syndrome has received attention (12, 20). An epidemiological study revealed that schistosome infection is negatively correlated with metabolic diseases in humans (26). Meanwhile, animal studies confirmed that chronic S. japonicum infection could improve hepatic insulin sensitivity and glucose metabolism in mice (13). The levels of lipoproteins were markedly suppressed in the serum of infected mice (27). Acute S. mansoni infection impaired lipid absorption in the gut of mice, and chronic S. mansoni infection reduced fat mass in obese mice (28). Our previous study showed that S. japonicum infection decreased the levels of serum glucose and triacylglycerol in mice and regulated hepatic glucose and lipid metabolism (18). The intestine is an important organ for digestion, absorption, and metabolism of dietary nutrients, whose metabolism is important for host health (19). Besides the glycolysis, TCA, and fatty acid oxidation pathways, we also detected alterations in gluconeogenesis, glucose transport, and fatty acid synthesis in the intestine. All of these pathways involved in glucose and lipid metabolism were enhanced based on the expression of rate-limiting enzymes in the infected group. These changes may be attributed to the compensatory effect of liver and intestine damage. Liver fibrosis induced by S. japonicum infection impaired its metabolic function (29). To meet the energy needs of the host, the intestinal function was enhanced to compensate for the damage of the liver. The enhanced metabolism may boost the intestinal immunity.
SEA released by S. japonicum eggs can recruit inflammatory and immune cells to the lesion region, causing egg granuloma formation and worsening the pathology of schistosomiasis (30). SEA treatment can improve insulin sensitivity and reduce fat mass in obese mice model developed by the administration of a high-fat diet, similar to the effects of chronic S. japonicum infection (31). In vitro stimulation by SEA caused the reprogramming of glycolipid metabolism in macrophages, which could significantly reduce the expression of Acc mRNA in hepatocytes (20). Our previous study showed that SEA stimulation directly upregulated the expression of GLUT4, MCAD, ACC1, FAS, CYP4A10, and CS in hepatocytes. Therefore, in some respects, SEA may be a crucial contributor to the reprogramming of glucose and lipid metabolism in lesions induced by S. japonicum. In line with this hypothesis, we observed that SEA treatment directly increased the mRNA expression of genes associated with glucose and lipid metabolism in CT-26 cells.
PTEN is an anti-oncogene that has a close relationship with liver fibrosis (32) and plays an essential role in immunity (33). According to our previous study, the downregulation of PTEN was linked with the changes of glucose and lipid metabolism in S. japonicum-induced liver fibrosis (18), and treatment with polyene phosphatidylcholine (PTEN agonist) showed the reversal of the alterations in glycolysis and fatty acid oxidation pathways induced by SEA. Therefore, PTEN may play an essential role in the reprogramming of metabolism in S. japonicum-induced tissue lesions. In the present study, we observed that the PTEN/PI3K/AKT pathway was inhibited in the infected group, and SEA stimulation decreased the mRNA levels of PTEN in CT-26 cells. Moreover, overexpression of PTEN reversed the changes in genes involved in the glucose and lipid metabolism in CT26 cells. These results suggested that the PTEN/PI3K/AKT pathway may play a role in the intestinal pathological metabolic changes in schistosomiasis.
Conclusions
In summary, the present study identified the expression of genes involved in the intestinal glucose and lipid metabolism after S. japonicum infection and verified that SEA initiated a cellular metabolic response via the inhibition of PTEN in enterocytes. Our findings provide a novel insight into the pathogenesis of S. japonicum with respect to the formation of intestine lesions, and investigation of PTEN and its role in schistosomiasis may be a new direction for understanding metabolic reprogramming in schistosomiasis.
Data Availability Statement
The original contributions generated for the study are included in the article, further inquiries can be directed to the corresponding author/s.
Ethics Statement
The animal study was reviewed and approved by The Ethics Committee of Xuzhou Medical University (Xuzhou, China, SCXK (FS) 2015-0009).
Author Contributions
XY, WP, and FS: conceived and designed the experiments. WD, XQ, XZ, QC, YL, and JW: performed the experiments. XY, WD, and XQ: analyzed the data. XY, WP, FS, XL, and ZP: contributed reagents, materials, and analysis tools. WD, XQ, PJ, and XY: wrote the manuscript. All authors have read and approved the manuscript.
Funding
This work was funded by the National Natural Science Foundation of China (Nos. 81871670 and 81800718), the Natural Science Foundation of the Jiangsu Higher Education Institutions of China (No. 18KJB310015), the Jiangsu Planned Projects for Postdoctoral Research Funds (No. 2019K063), the Natural Science Foundation of Jiangsu Province (No. BK20201459), the Priority Academic Program Development of Jiangsu Higher Education Institutions, the Jiangsu Shuangchuang Program, the Training Programs of Innovation and Entrepreneurship for College Students in Jiangsu Province (Nos. 201810313063X and 202010313035Z), and the Starting Foundation for Talents of Xuzhou Medical University (No. D2018006). The funders had no role in study design, data collection and analysis, decision to publish, or preparation of the manuscript.
Conflict of Interest
The authors declare that the research was conducted in the absence of any commercial or financial relationships that could be construed as a potential conflict of interest.
References
1. Deol AK, Fleming FM, Calvo-Urbano B, Walker M, Bucumi V, Gnandou I, et al. Schistosomiasis - assessing progress toward the 2020 and 2025 global goals. N Engl J Med. (2019) 381:2519–28. doi: 10.1056/NEJMoa1812165
2. Colley DG, Bustinduy AL, Secor WE, King CH. Human schistosomiasis. Lancet. (2014) 383:2253–64. doi: 10.1016/s0140-6736(13)61949-2
3. Warren KS. The pathology, pathobiology and pathogenesis of schistosomiasis. Nature. (1978) 273:609–12. doi: 10.1038/273609a0
4. Schwartz C, Fallon PG. Schistosoma “Eggs-Iting” the host: granuloma formation and egg excretion. Front Immunol. (2018) 9:2492. doi: 10.3389/fimmu.2018.02492
5. Zhao Y, Yang S, Li B, Li W, Wang J, Chen Z, et al. Alterations of the mice gut microbiome via schistosoma japonicum ova-induced granuloma. Front Microbiol. (2019) 10:352. doi: 10.3389/fmicb.2019.00352
6. Grencis RK. Immunity to helminths: resistance, regulation, and susceptibility to gastrointestinal nematodes. Annu Rev Immunol. (2015) 33:201–25. doi: 10.1146/annurev-immunol-032713-120218
7. Haeberlein S, Obieglo K, Ozir-Fazalalikhan A, Chayé MM, Veninga H, et al. Schistosome egg antigens, including the glycoprotein IPSE/alpha-1, trigger the development of regulatory B cells. PLoS Pathog. (2017) 13:e1006539. doi: 10.1371/journal.ppat.1006539
8. Mayer JU, Demiri M, Agace WW, Macdonald AS, Svensson-Frej M, Milling SW. Different populations of CD11b(+) dendritic cells drive Th2 responses in the small intestine and colon. Nat Commun. (2017) 8:15820. doi: 10.1038/ncomms15820
9. Perrigoue JG, Saenz SA, Siracusa MC, Allenspach EJ, Taylor BC, Giacomin PR, et al. MHC class II-dependent basophil-CD4+ T cell interactions promote T(H)2 cytokine-dependent immunity. Nat Immunol. (2009) 10:697–705. doi: 10.1038/ni.1740
10. Kay GL, Millard A, Sergeant MJ, Midzi N, Gwisai R, Mduluza T, et al. Differences in the faecal microbiome in schistosoma haematobium infected children vs. uninfected children. PLoS Negl Trop Dis. (2015) 9:e0003861. doi: 10.1371/journal.pntd.0003861
11. Jenkins TP, Peachey LE, Ajami NJ, Macdonald AS, Hsieh MH, Brindley PJ, et al. Schistosoma mansoni infection is associated with quantitative and qualitative modifications of the mammalian intestinal microbiota. Sci Rep. (2018) 8:12072. doi: 10.1038/s41598-018-30412-x
12. Shen SW, Lu Y, Li F, Shen ZH, Xu M, Yao WF, et al. The potential long-term effect of previous schistosome infection reduces the risk of metabolic syndrome among Chinese men. Parasite Immunol. (2015) 37:333–339. doi: 10.1111/pim.12187
13. Luo X, Zhu Y, Liu R, Song J, Zhang F, Zhang W, et al. Praziquantel treatment after Schistosoma japonicum infection maintains hepatic insulin sensitivity and improves glucose metabolism in mice. Parasit Vectors. (2017) 10:453. doi: 10.1186/s13071-017-2400-5
14. Hendriks WJ, Pulido R. Protein tyrosine phosphatase variants in human hereditary disorders and disease susceptibilities. Biochim Biophys Acta. (2013) 1832:1673–1696. doi: 10.1016/j.bbadis.2013.05.022
15. Chen CY, Chen J, He L, Stiles BL. PTEN: tumor suppressor and metabolic regulator. Front Endocrinol. (2018) 9:338. doi: 10.3389/fendo.2018.00338
16. Qian X, Li X, Shi Z, Xia Y, Cai Q, Xu D, et al. PTEN suppresses glycolysis by dephosphorylating and inhibiting autophosphorylated PGK1. Mol Cell. (2019) 76:516–527.e517. doi: 10.1016/j.molcel.2019.08.006
17. Qiu W, Federico L, Naples M, Avramoglu RK, Meshkani R, Zhang J, et al. Phosphatase and tensin homolog (PTEN) regulates hepatic lipogenesis, microsomal triglyceride transfer protein, and the secretion of apolipoprotein B-containing lipoproteins. Hepatology. (2008) 48:1799–809. doi: 10.1002/hep.22565
18. Qian XY, Ding WM, Chen QQ, Zhang X, Jiang WQ, Sun FF, et al. The metabolic reprogramming profiles in the liver fibrosis of mice infected with Schistosoma japonicum. Inflammation. (2020) 43:731–43. doi: 10.1007/s10753-019-01160-5
19. Zhu J, Xu Z, Chen X, Zhou S, Zhang W, Chi Y, et al. Parasitic antigens alter macrophage polarization during Schistosoma japonicum infection in mice. Parasit Vectors. (2014) 7:122. doi: 10.1186/1756-3305-7-122
20. Xu ZP, Chang H, Ni YY, Li C, Chen L, Hou M, et al. Schistosoma japonicum infection causes a reprogramming of glycolipid metabolism in the liver. Parasit Vectors. (2019) 12:388. doi: 10.1186/s13071-019-3621-6
21. Klaver EJ, Kuijk LM, Lindhorst TK, Cummings RD, Van Die I. Schistosoma mansoni soluble egg antigens induce expression of the negative regulators SOCS1 and SHP1 in human dendritic cells via interaction with the mannose receptor. PLoS ONE. (2015) 10:e0124089. doi: 10.1371/journal.pone.0124089
22. O'neill LA, Kishton RJ, Rathmell J. A guide to immunometabolism for immunologists. Nat Rev Immunol. (2016) 16:553–565. doi: 10.1038/nri.2016.70
23. Deng J, Lü S, Liu H, Liu B, Jiang C, Xu Q, et al. Homocysteine activates b cells via regulating PKM2-dependent metabolic reprogramming. J Immunol. (2017) 198:170–83. doi: 10.4049/jimmunol.1600613
24. Basit F, De Vries IJM. Dendritic cells require PINK1-mediated phosphorylation of BCKDE1α to promote fatty acid oxidation for immune function. Front Immunol. (2019) 10:2386. doi: 10.3389/fimmu.2019.02386
25. Mahon OR, Kelly DJ, Mccarthy GM, Dunne A. Osteoarthritis-associated basic calcium phosphate crystals alter immune cell metabolism and promote M1 macrophage polarization. Osteoarthritis Cartilage. (2020) 28:603–612. doi: 10.1016/j.joca.2019.10.010
26. Wolde M, Berhe N, Medhin G, Chala F, Van Die I, Tsegaye A. Inverse associations of Schistosoma mansoni infection and metabolic syndromes in humans: a cross-sectional study in Northeast Ethiopia. Microbiol Insights. (2019) 12:11. doi: 10.1177/1178636119849934
27. Wu J, Xu W, Ming Z, Dong H, Tang H, Wang Y. Metabolic changes reveal the development of schistosomiasis in mice. PLoS Negl Trop Dis. (2010) 4. doi: 10.1371/journal.pntd.0000807
28. Van Den Berg SM, Van Dam AD, Kusters PJH, Beckers L, Den Toom M, Van Der Velden S, et al. Helminth antigens counteract a rapid high-fat diet-induced decrease in adipose tissue eosinophils. J Mol Endocrinol. (2017) 59:245–55. doi: 10.1530/jme-17-0112
29. Duan Q, Xiong L, Liao C, Liu Z, Xiao Y, Huang R, et al. Population based and animal study on the effects of Schistosoma japonicum infection in the regulation of host glucose homeostasis. Acta Trop. (2018) 180:33–41. doi: 10.1016/j.actatropica.2018.01.002
30. Hams E, Aviello G, Fallon PG. The schistosoma granuloma: friend or foe? Front Immunol. (2013) 4:89. doi: 10.3389/fimmu.2013.00089
31. Hussaarts L, García-Tardón N, Van Beek L, Heemskerk MM, Haeberlein S, Van Der Zon GC, et al. Chronic helminth infection and helminth-derived egg antigens promote adipose tissue M2 macrophages and improve insulin sensitivity in obese mice. Faseb J. (2015) 29:3027–39. doi: 10.1096/fj.14-266239
32. Kumar P, Raeman R, Chopyk DM, Smith T, Verma K, Liu Y, et al. Adiponectin inhibits hepatic stellate cell activation by targeting the PTEN/AKT pathway. Biochim Biophys Acta Mol Basis Dis. (2018) 1864:3537–45. doi: 10.1016/j.bbadis.2018.08.012
Keywords: Schistosoma japonicum, colon, soluble egg antigens, glucose and lipid metabolism, PTEN
Citation: Yang X, Ding W, Qian X, Jiang P, Chen Q, Zhang X, Lu Y, Wu J, Sun F, Pan Z, Li X and Pan W (2021) Schistosoma japonicum Infection Leads to the Reprogramming of Glucose and Lipid Metabolism in the Colon of Mice. Front. Vet. Sci. 8:645807. doi: 10.3389/fvets.2021.645807
Received: 24 December 2020; Accepted: 17 February 2021;
Published: 10 March 2021.
Edited by:
Yadong Zheng, Lanzhou Institute of Veterinary Research (CAAS), ChinaReviewed by:
Si-Yang Huang, Yangzhou University, ChinaColin Matthew Fitzsimmons, University of Cambridge, United Kingdom
Copyright © 2021 Yang, Ding, Qian, Jiang, Chen, Zhang, Lu, Wu, Sun, Pan, Li and Pan. This is an open-access article distributed under the terms of the Creative Commons Attribution License (CC BY). The use, distribution or reproduction in other forums is permitted, provided the original author(s) and the copyright owner(s) are credited and that the original publication in this journal is cited, in accordance with accepted academic practice. No use, distribution or reproduction is permitted which does not comply with these terms.
*Correspondence: Wei Pan, panwei525@126.com
†These authors have contributed equally to this work and share first authorship