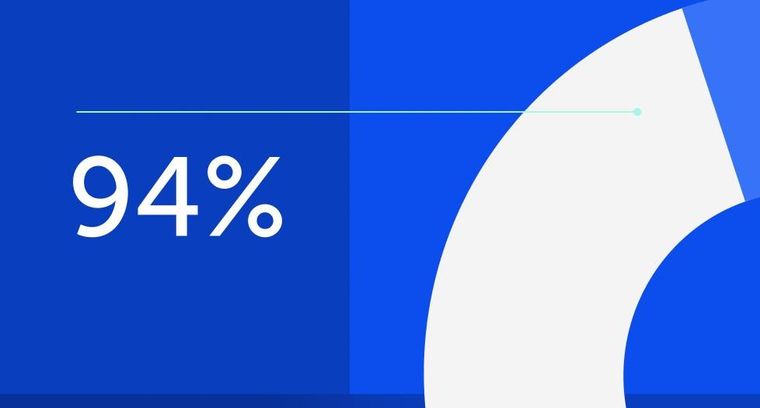
94% of researchers rate our articles as excellent or good
Learn more about the work of our research integrity team to safeguard the quality of each article we publish.
Find out more
REVIEW article
Front. Vet. Sci., 13 May 2021
Sec. Comparative and Clinical Medicine
Volume 8 - 2021 | https://doi.org/10.3389/fvets.2021.625708
This article is part of the Research TopicFluid Therapy in AnimalsView all 26 articles
Fluid selection and administration during shock is typically guided by consideration of macrovascular abnormalities and resuscitative targets (perfusion parameters, heart rate, blood pressure, cardiac output). However, the microcirculatory unit (comprised of arterioles, true capillaries, and venules) is vital for the effective delivery of oxygen and nutrients to cells and removal of waste products from the tissue beds. Given that the microcirculation is subject to both systemic and local control, there is potential for functional changes and impacts on tissue perfusion that are not reflected by macrocirculatory parameters. This chapter will present an overview of the structure, function and regulation of the microcirculation and endothelial surface layer in health and shock states such as trauma, hemorrhage and sepsis. This will set the stage for consideration of how these microcirculatory characteristics, and the potential disconnect between micro- and macrovascular perfusion, may affect decisions related to acute fluid therapy (fluid type, amount, and rate) and monitoring of resuscitative efforts. Available evidence for the impact of various fluids and resuscitative strategies on the microcirculation will also be reviewed.
Given the complex and multi-faceted nature of emergent and critical disease processes, determination of optimal approaches to fluid resuscitation continues to be a challenge for both human and veterinary medicine. Further complicating the ideal approach is determining how best to initially assess the severity of systemic compromise and gauge response to therapy. Traditionally, monitoring of a patient's hemodynamic state has been conducted at the macrovascular level by monitoring heart rate, respiratory rate, blood pressure, oxygen content and lactate, among other parameters. While these parameters may reflect systemic cardiovascular regulation, they do not necessarily indicate what is occurring at the level of the microvasculature. As the microcirculation is ultimately the conduit for delivery of oxygen and nutrients to tissues, it represents a relatively uncharted avenue of exploration to help enhance our understanding of disease processes like trauma and sepsis, its impact on tissue perfusion, as well as new ways to monitor response to therapy.
The goal of this manuscript will be to explore the structure, function, and regulation of the microcirculation, both in health and in response to disease processes like traumatic hemorrhage (representing hypovolemia and systemic vasoconstriction) and sepsis (representing a vasodilatory and maldistributive process). Finally, there will be consideration of modalities for assessing the microcirculation and tissue perfusion.
The microcirculatory unit is comprised of arterioles feeding into a capillary bed that is drained by venules (Figure 1). The feeder arterioles are highly muscular throughout their length, while the terminal metarterioles have intermittent bands of smooth muscle. True capillaries have walls that lack musculature and are one endothelial cell thick and attached to a basement membrane. A precapillary sphincter is located between the arteriole and the capillary bed. Arterioles and venules in the microcirculation generally have a diameter <100 microns, while the capillaries are <10 microns in diameter (1). There are also shunt vessels that allow arterial blood to completely bypass the associated microcirculatory unit, as dictated by arteriolar and precapillary sphincter tone (1). The vascular endothelial surface layer (ESL) also plays a pivotal role in health and disease; this is the intimal surface of blood vessels containing the endothelial glycocalyx and associated components from the endothelial cells and plasma (2, 3). The ESL ranges from 200 nm to 2 μm in thickness and comprises up to 25% of the vascular space (4). The glycocalyx is a complex carbohydrate rich gel-like layer that serves as a barrier between the vessel wall and the blood (2, 5, 6) and has an overall negative charge. It contains membrane-bound proteoglycans, secreted glycosaminoglycans, sialic acid-containing glycoproteins, and glycolipids that are associated with the vascular endothelial surface (7). Proteins within the plasma, such as albumin and antithrombin, are also contained within the glycocalyx (7–9). The primary goals of the ESL are to maintain the vascular permeability barrier, modulate nitric oxide produced in response to shear stress, retain protective enzymes such as superoxide dismutase, contain factors that inhibit coagulation such as antithrombin, tissue factor pathway inhibitor and protein C, assist with mechanotransduction, and prevent leukocyte adhesion and binding of ligands to control local inflammation (3, 10). Recent discoveries in the ESL have contributed to the revised version of the Starling principle of transvascular fluid flux (11). Further details about the ESL are described in “Resuscitative Fluid Therapy and the Endothelial Surface Layer” found elsewhere in this “Fluid Therapy in Small Animals” series.
Figure 1. Schematic of the microcirculation. Boxes on the left represent ranges of vessel diameters at varying levels of the microcirculation. Boxes throughout the diagram represent the average interstitial (tissue) oxygen tension (PtO2). Arrows represent direction of blood flow across the microcirculatory unit.
Altogether, the microcirculation represents the largest vascular surface area in the body and is vital for the effective delivery of nutrients to the cells and removal of waste products from the tissue beds (1). Both systemic and local regulation of blood flow through these units, as well as maintenance of the ESL, are essential to maintain adequate perfusion and match metabolic demand to oxygen/nutrient delivery.
Vascular tone can be influenced by numerous chemical mediators in the circulation, such as catecholamines, endothelin and thromboxane (Table 1). Catecholamines are important (predominantly) vasoconstrictor agents released in large amounts when the sympathetic nervous system is activated. This occurs via central nervous system control in response to baroreceptors and chemoreceptors in the aortic arch and carotid sinuses (12). Baroreceptors respond to changes in vessel wall distension and chemoreceptors respond to hypoxia, hypercapnia or acidemia with associated modulation in vascular tone. These effects are most important once the systemic arterial pressure drops below 80 mmHg; they attempt to maintain perfusion in individual capillary beds despite decreased systemic blood pressure (12).
It is important to note sympathetic innervation exists for the arterial tree and much of the venous system but is not present down to the level of the capillaries. The absence of innervation and musculature in the true capillaries means flow through each capillary bed is regulated by the hemodynamic pressures generated between the precapillary sphincter and the post capillary venules. A single capillary bed can be fed by multiple arterioles, a situation that allows flow through the capillary bed to increase by 200–500% without any significant change in the overall arteriolar pressure (13). This can help to preserve microcirculatory flow to specific tissue beds during periods of transient systemic hypotension.
Maintenance of vascular tone is not the only factor influencing arterial blood pressure and tissue perfusion. Vascular volume also contributes to maintenance of appropriate blood pressure and tissue perfusion. In normal animals, the renin-angiotensin-aldosterone system is integral in maintaining appropriate blood volume, as well as contributing to systemic vascular tone (4). Angiotensin II acts on vascular smooth muscle to cause vasoconstriction and in the proximal tubules it causes sodium and water retention, leading to increased blood volume and arterial pressure (14). Angiotensin also causes the release of aldosterone and vasopressin, thus promoting vasoconstriction and water retention (14).
Systemically acting vasodilators include the kinins, adrenomedullin and atrial natriuretic peptide (ANP). The kinins include bradykinin and l-lysyl-bradykinin and are activated by the kallikreins. They typically regulate blood flow at the tissue level but can also be found circulating in the bloodstream. In addition to causing arteriolar dilation, bradykinin increases capillary permeability, thereby increasing the delivery of nutrients to the tissue bed (15). Histamine, which is released when tissues are damaged or in allergic reactions, also acts as a vasodilator and increases capillary permeability. Adrenomedullin increases the production of NO while ANP acts to antagonize numerous vasoconstrictor agents, both exerting their influence on the vascular tone indirectly (15).
These systemic regulators are responsible for controlling delivery of blood to the precapillary sphincter across different tissue beds. Once blood arrives at the capillary bed, local regulatory mechanisms act to maintain flow through the capillary bed, sometimes independent of systemic changes to perfusion (15).
Basal tissue bed requirements vary based on their metabolic rate, nutrient availability and accumulation of waste products. Given that each capillary bed has unique requirements that may change independent of nearby capillary beds or systemic tissue needs, there are many local regulators of microcirculatory flow (Table 1). Typically, these changes in perfusion occur at the level of the precapillary sphincter. Independent regulation of flow based on local tissue needs results in selective capillary perfusion and the potential for microcirculatory shunting. Given the expansive nature of the capillary circulation, cardiac output would be insufficient to maintain forward flow if every capillary bed were to open simultaneously. Thus, the ability to adjust capillary perfusion through local and systemic changes is essential for modulating cardiac workload (15).
Rapid control of microcirculatory flow is mediated at a local level through autoregulation. One major mechanism is flow autoregulation, which allows maintenance of consistent capillary blood flow over a wide range of arterial perfusion pressure. This is achieved through vascular stretch receptors which respond to changes in peripheral pressure. Increases in vascular pressure trigger increased tone of the precapillary sphincter to mute transmission of that pressure though the capillary circuit (16). The opposite happens with a fall in peripheral pressure. This mechanism is effective for maintaining consistent flow over a perfusion pressure (mean arterial pressure) of 60–160 mm Hg and occurs independent of any neurohormonal input (Figure 2).
Figure 2. Curve reflecting tissue autoregulation for maintaining consistent blood flow across varying systemic perfusion pressures.
Changes in local metabolic demand can also have a significant impact on local control of blood flow. Understandably, by-products of increased metabolic activity, such as carbon dioxide, lactate, and hydrogen ion will trigger vasodilation to enhance local blood flow and improve oxygen/nutrient delivery. While these metabolites are produced downstream, the countercurrent flow between arterioles and venules allows them to be “sensed” at the level of the precapillary sphincter and alter inward flow (16). In addition, inter-cell and local neural pathways allow conduction of signals from capillary endothelium and venular smooth muscle in response to these signals (16).
Local levels of oxygen tension will also affect microcirculatory regulation. Under normal circumstances, capillary blood has a significantly lower PO2 (5–12 mm Hg) compared to arteriolar blood (~60 mm Hg). This occurs because of early off-loading in precapillary tissues, as well as endothelial consumption and countercurrent exchange with venous flow (16). An increase in precapillary tissue PO2 will promote vasoconstriction, whereas a decrease will result in vasodilation, largely through release of nitric oxide (NO). In addition to this role, NO has an impact on microvascular tone in a number of other circumstances (16). Normally, the constitutive form of nitric oxide synthetase (cNOS) is responsible for maintaining a basal level of NO and modulating vascular tone. Further, an increase in blood viscosity, which occurs in diseases such as polycythemia vera or severe hemoconcentration, results in elevated shear stress on the vascular endothelium. Through mechanotransduction shear stress serves to increase cNOS activity, resulting in increased release of NO, and associated vasodilation (Figure 3). The opposite would occur in severe anemia/hemodilution with significant reduction in red cell mass and blood viscosity (17). The inducible form of nitric oxide synthetase (iNOS) can be produced by the endothelial cells when triggered by the inflammatory cascade. Prostacyclin-induced vasodilation may also play a role in the normal response to hypoxia, especially when NO is blocked there is shear stress (18).
Figure 3. Diagram representing the impact of shear force generated against the endothelium. Through mechanotransduction, intracellular calcium is increased leading to increased nitric oxide (NO) production from stimulation of constitutive nitric oxide synthetase (cNOS). NO diffuses into surrounding smooth muscle cell causing activation of guanylyl cyclase (GC) and conversion of guanosine triphosphate (GTP) into cyclic guanosine monophosphate (cGMP). The resulting decrease in cytosolic calcium causes relaxation of vascular smooth muscle and vasodilation.
Given the complex nature of the microcirculation and its multifactorial regulation, it stands to reason that microvascular perfusion will be altered in varying ways in response to disease states. In the face of tissue injury, hypotension, and impaired oxygen delivery, it can be expected that there will be activation of both systemic and local compensatory and pathological mechanisms. While some of this response has already been described in a general sense, what follows is more specifically related to microvascular changes secondary to trauma and hemorrhage.
The initial systemic response to tissue trauma, pain, and hemorrhage is largely driven by the sympathetic nervous system leading to release of epinephrine and norepinephrine. The resulting influx of catecholamines will promote vasoconstriction, particularly in the large arterioles (70–150 μm) supplying skeletal muscle (19). Smaller arterioles (10–25 μm) have a more varied response, with constriction to some beds and dilation in others. This next level allows for finer regulation of microcirculatory flow based on metabolic demand and the “essential nature” of the associated organ/tissue bed (e.g., heart and brain).
Local factors caused by trauma and hemorrhage will also cause changes at the level of the small arterioles. Decreased oxygen delivery and tissue hypoxia will tend to cause vasodilation. However, this is offset by inhibition of eNOS (and thereby release of NO) in the early stages of trauma/hemorrhage. With time, however, there is upregulation of iNOS (from both tissue damage and inflammatory mediators) and more tendency for vasodilation (20). In addition, tissue injury and the inflammatory response will promote release of assorted vasoactive mediators with mixed effects. Progressive acidosis and accumulation of cellular metabolites (particularly in the terminal stages of shock) will tend to promote vasodilation and serve to undo systemic vasoconstrictive efforts. Despite the vasodilation, the decrease in driving pressure in the face of hypotension ultimately leads to stagnation of blood flow.
It is also important to consider the potential role of the venous circulation on microvascular perfusion. In the initial response to trauma and hemorrhage, catecholamine-induced venoconstriction, albeit limited, serves to decrease venous capacitance and encourage return of blood to the heart. However, as shock progresses there will be a general relaxation (through similar mechanisms described above), and pooling of blood in venous circulation. This downstream stagnation can then negatively impact blood flow across the capillary bed.
There are several other factors that can contribute to impaired microcirculatory flow. With trauma, inflammation, and shock there can be significant swelling of vascular endothelial cells secondary to increased membrane permeability, acidosis, and impaired electrolyte transport from failure of ATP-dependent channels (21). Given capillary luminal size, further reduction from endothelial swelling can have a significant negative impact on capillary blood flow. Endothelial edema causes decreased release of prostacyclin and NO and increased release of endothelin and thromboxane; the net effect of which is upstream vasoconstriction and a further reduction in capillary flow. Another contributing factor can be decreased red blood cell (RBC) deformability secondary to oxidative injury, ATP depletion, cell membrane injury, and cellular dehydration (21). As RBCs are typically slightly larger than the capillary lumen, folding is necessary for them to effectively move through the microcirculation. Impaired deformability, along with aggregation, can lead to capillary plugging, or shearing injury/destruction of RBCs. Along similar lines, increased leukocyte rigidity, activation, and endothelial adherence can also result in arteriolar and capillary plugging. Lastly, microthrombi formed secondary to tissue/endothelial injury, inflammatory response and hypercoagulability can lodge at various levels of the microcirculation and impede downstream flow.
These upstream and downstream effects will ultimately serve to impact capillary flow and delivery of oxygen and nutrients. Vasoconstriction will lead to shunting of blood away from capillary circulation (decrease vessel number) and hypotension, vasodilation, and obstruction will lead to stagnation (decreased flow). In addition, these abnormalities can persist for an extended period after resuscitation has been achieved, even when macrovascular parameters have been normalized (21, 22).
Shedding of the endothelial glycocalyx has been seen in experimental rodent models of non-traumatic hemorrhagic shock, although the changes are independent of increased vascular endothelial permeability (23–25).
The pathophysiological syndrome of sepsis characterized by the progression of illness severity and potentially culminating in septic shock (26). Septic shock is described in human medicine as the presence of refractory hypotension, hyperlactatemia, and organ dysfunction that persists despite aggressive fluid resuscitation (27). The rapid progression from sepsis to septic shock and organ dysfunction is poorly understood; profound changes in the macro- and microcirculation are believed to contribute to the development of organ failure and subsequent death (28–31). Microcirculatory alterations include a decreased microvascular density and perfusion along with increased capillary flow heterogeneity (Figure 4C and Supplementary Video 3) (28, 29, 32). These changes have been found to precede macrocirculatory changes in septic humans and microcirculatory improvement has also been correlated with improved survival (28, 29, 32, 33). Early microcirculatory changes were a stronger predictor of outcome than any macrocirculatory variable in septic human patients (34).
Figure 4. Representative images from a sidestream dark-field microscopy device from a healthy dog (A), dog in hemorrhagic shock (B), and dog in septic shock (C). Note the decreased density of capillaries in patients with shock.
Various factors likely contribute to the microcirculatory derangements in septic patients, including hypovolemia, endothelial cell dysfunction secondary to adhesion molecule expression, increased white blood cell adhesion, degradation of the endothelial glycocalyx, connexin uncoupling, vascular hyperpermeability, formation and deposition of microthrombi, loss of vasomotor autoregulation and reactivity, changes in local perfusion pressure and flow, and shunting of oxygen to hyperperfused capillary beds (35).
The sublingual microcirculatory derangements appear to correlate with microcirculatory changes in other organs such as the intestines and kidneys in experimental and clinical studies (36–38). Microcirculatory changes in healthy, anesthetized dogs correlate with macrocirculatory measurements of perfusion, although dogs with hemorrhagic shock do not maintain this hemodynamic coherence (39–41). Correlations in septic dogs has not yet been published.
The degraded glycocalyx layer becomes thin and sparse during sepsis, thus enabling plasma proteins and fluid to move across the vascular wall and into the interstitium (42, 43). Degradation of the glycocalyx likely occurs secondary to inflammation and increased circulating “sheddases” such as metalloproteinases, heparinase, and hyaluronidase which are activated by reactive oxygen species and proinflammatory cytokines (44). Specific changes to the endothelial glycocalyx of the lung in septic rodent models include peeling away from the endothelial surface and form spherical bodies that are visualized at the site of damage (45). An decrease in the thickness of the endothelial surface layer was seen in human patients suffering from sepsis and correlated with severity of critical illness. However, there was not an association between this thickness and microcirculatory parameters such as flow index and the proportion of perfused vessels (46).
There is mounting evidence in human medicine to suggest that goal directed therapy aimed at maximizing macrocirculatory parameters may not improve outcome (47–49). Despite preservation of total blood flow to organs, heterogeneity of microcirculatory flow can lead to hypoxic zones (29, 50). Several techniques have now been developed to assess the microcirculation to investigate its role as a diagnostic, prognostic, and monitoring tool, including laser Doppler, near-infrared spectroscopy, and videomicroscopy (51). As mentioned above, macrocirculatory hypoperfusion is associated with microcirculatory derangements; however, dysfunction of the microcirculation may occur despite normal macrocirculatory indices. This loss of hemodynamic coherence can lead to hyperlactatemia and acidemia despite normal perfusion parameters (also known as cryptic shock) (52). When patients are in shock, normalization of cardiovascular parameters may not equate to improvements in microcirculatory perfusion, as evidenced in both clinical and experimental studies (22, 29, 32, 34, 53). There are four possible mechanisms for this loss of hemodynamic coherence: (1) increased microcirculatory heterogeneity of perfusion (i.e., secondary to inflammatory cytokines), (2) hemodilution from non-oxygen carrying intravenous fluids, thus decreasing hematocrit and oxygen content, (3) microcirculatory vasoconstriction or tamponade from endogenous/exogenous vasopressors and/or increased venous pressure, ad (4) interstitial edema of tissues secondary to endothelial and glycocalyx damage (i.e., secondary to inflammation or excessive fluid therapy) (54). In this regard, direct assessment of microcirculatory flow, such as with videomicroscopy, could be better suited to determine the presence of derangements, as well as response to fluid resuscitation.
Techniques like side stream dark field (SDF) and incident dark field (IDF) microscopy allow direct imaging of the microcirculation with the ability to quantify vessel density as well as assess quality of flow (55). Both of these technologies involve a hand-held device that emits green light (530 nm) which is absorbed by the hemoglobin of erythrocytes. These illuminated red blood cells show up as a dark density flowing through the microcirculation, resulting in a real-time magnified video with resolution to allow detection of true capillaries (Figures 4A–C, Supplementary Videos 1–3). Where they differ is how the light is emitted and received by the device, with reported differences in image quality and magnification (55). Analysis of the resulting videos generates parameters including total vessel density (TVD), proportion of perfused vessels (PPV), perfused vessel density (PVD), and microvascular flow index (MFI) which reflect the quantity and quality of microvascular flow. In order to allow a more consistent approach to microvascular analysis, consensus criteria for the acquisition and analysis of microcirculatory images using have been established (56).
Despite the availability of direct microvascular imaging for two decades and some evidence in veterinary medicine, (39, 40) it has still not become a standard in monitoring response to fluid therapy. Largely this has to do with some of the limitations of these technologies. The camera is expensive and not widely available. Because of the need for light to transmit and reflect back, only mucosal or serosal surfaces can be used for visualization, and areas with keratinized epithelium or significant pigmentation cannot be examined with this technique. In order to generate diagnostic quality videos for analysis, sufficient pressure has to be applied to the tissue, while avoiding excessive compression of the microvasculature (56). While significant steps have been made toward automating the vascular analysis (previously it was largely a manual effort taking over 1 h per video), accuracy of vessel assignment used for automated calculations remains a challenge. This can result in inconsistency and variability of results. Therefore, while microvascular imaging has significant potential to aid in guiding response to fluid therapy, further refinement of automated analysis and increased availability is needed before its use can become more commonplace.
There are two primary methods for assessing the integrity of the endothelial glycocalyx: measurement of shed glycocalyx components in the plasma or serum (i.e., glycosaminoglycans such as hyaluronan and proteoglycan ectodomains such as syndecan-1) and use of imaging techniques that can be done in vivo or ex vivo. Damage to the ESL and shedding of the glycocalyx has been shown to occur in a multitude of serious illnesses; the degree of shedding is associated with poor outcomes (57). Potential therapeutic strategies to support repair of a damaged ESL, including fluid therapy prescriptions, is the focus of ongoing research.
The use of circulating biomarkers as an indicator of glycocalyx damage has limitations. These primarily include the variable methodology itself, potential for other sources of the markers since they are not unique to the glycocalyx, and upregulation of some markers with diseases such as inflammation. Further details on glycocalyx biomarkers can be found in “Resuscitative Fluid Therapy and the Endothelial Surface Layer” found elsewhere in this “Fluid Therapy in Small Animals” series.
Imaging of the ESL in vivo, ex vivo, and in vitro has been performed using multiple methods. These include transmission electron microscopy, (58–60) intravital microscopy, (61, 62) microparticle image velocimetry, (63) confocal laser scanning microscopy or atomic force microscopy, (10) two-photon laser scanning microscopy, (64) and videomicroscopy using handheld devices and various imaging technologies (i.e., orthogonal polarization spectroscopy, sidestream dark-field imaging incident dark-field imaging) (56). More recently, in vivo indirect assessments of the glycocalyx have been performed in humans and small animals using the sidestream dark-field microscopic technique in conjunction with specialized, proprietary software that measures the width of the vessel lumen available for red blood cell movement as an indirect assessment of glycocalyx thickness (also known as the perfused boundary region or PBR) (65–68). If there is damage to the ESL, RBC are able to penetrate further toward the endothelium and the PBR increases (69).
The nature of traumatic injury and hemorrhage presents significant challenges regarding how to optimize fluid therapy and volume replacement, particularly in the animal with active bleeding. In addition to loss of circulating volume, there are also aspects of progressive anemia, protein loss and coagulopathy. While administration of blood products over crystalloid or colloid is generally recommended in human medicine, (70) limited availability in veterinary medicine can result in greater use of crystalloids or consideration of synthetic colloids. Experimental hemorrhagic shock studies in rodent models suggest a benefit of balanced crystalloids, fresh frozen plasma or concentrated albumin over normal saline for restoration of the endothelial glycocalyx (71, 72). Results have not been consistent, however, Further, albumin and fresh frozen plasma appear to be more protective in most studies when compared to synthetic colloids (73). In addition to the type of fluid, volume and rate of administration are also a subject of debate; there is concern that aggressive fluid administration could serve to exacerbate ongoing hemorrhage, worsen coagulopathy, and damage the endothelial glycocalyx (74, 75). Of further consideration are the implications that fluid type and rate of administration might have on the microcirculation. As previously indicated, improving macrovascular parameters does not always correlate to restoration of microvascular perfusion.
Numerous experimental models and preclinical studies have attempted to assess the impact of various fluids on the microcirculation in patients with hemorrhagic shock. A systematic review of many of these studies, totaling 71 articles between 1990 and 2015, evaluated an assortment of fluid comparisons including blood products, hemoglobin-based oxygen carriers, crystalloids and colloids (76). Major findings of this analysis suggested that improved microcirculation was found with solutions containing hemoglobin vs. those without, those that were hyperoncotic vs. those that were not, and those that were hyperviscous vs. those that were not (76). In fitting with this, a recent study in sheep comparing hydroxyethyl starch (HES) and saline found improved hemodynamic coherence with HES, whereas saline only improved macrovascular parameters (77). However, concerns regarding the potential for acute kidney injury with synthetic colloids has served to significantly reduce their use in both human and veterinary medicine. What remains to be determined is whether use of a limited acute volume expansion carries a similar risk. In a human clinical study of patients with traumatic hemorrhagic shock, it was shown that the presence of microvascular derangement at the time of presentation that persisted after resuscitative efforts was more predictive of progression to multiple organ dysfunction syndrome than other more traditional parameters (like lactate and blood pressure) (78). This was true regardless of the type of resuscitative fluid used.
In hemorrhagic shock and trauma, there is potentially even greater interest in specifically looking at the impact of blood transfusion on restoring or maintaining microvascular perfusion. One study in human patients showed that administration of packed red blood cells helped to improve patients with microvascular parameters that were initially decreased, however there was no change or reduction in patients that started with normal values (79). Another pilot study showed improvement in microvascular parameters with red blood cell transfusion of one unit despite no change in macrovascular parameters or hemoglobin levels (80). Interestingly, these changes were negatively correlated with pre-transfusion microvascular parameters, again suggesting that the worse the microvascular impairment, the more significant the improvement. However, duration of red blood cell storage could alter the impact of red cell transfusion on microvascular perfusion. It has been demonstrated that aged red cell units may have increased levels of free hemoglobin which can serve to scavenge NO and worsen microvascular blood flow (81). Future studies evaluating the use of plasma for resuscitation from trauma are underway; initial data suggests that plasma therapy may play a beneficial role in ameliorating immunomodulatory dysfunction and trauma-induced endotheliopathy (82).
Intravenous fluid therapy has been one of the cornerstones of treatment for sepsis spanning many decades. Although fluid resuscitation may improve microvascular perfusion, this effect is not predictable and likely depends in part on the timing of administration; microvascular perfusion is improved if fluids are given within 12–24 h of diagnosing sepsis but may be less effective or even deleterious when given at later stages according to one study (83). However, additional studies found that bolus fluid therapy worsened survival in people with sepsis (84, 85). Humans with abdominal sepsis displayed an increase in mean arterial blood pressure, cardiac index, and sublingual microcirculatory red blood cell velocity following a fluid challenge; however, the intestinal microcirculatory indices did not change (86). The role of fluids in the augmentation of septic endothelial dysfunction and glycocalyx damage is the focus of current investigation (87). Adverse outcomes have also been linked to fluid therapy and fluid balance, increasing the need to determine the pathogenesis of these findings (88–93). There is evidence to suggest that intravenous crystalloid and colloid fluid administration promotes endothelial glycocalyx degradation in endotoxemic sheep (94) and humans with sepsis (74, 87). Although the exact mechanism is still unknown, there are several possible etiologies: (1) acute vascular stretching along with inflammatory mediators could stimulate endothelial expression of glycocalyx-shedding matrix metalloproteinase, (95) (2) oscillatory shear stress-induced increases in cathepsin L activation (an enzyme that may be involved in post-translational activation of endothelial heparinase), (96) (3) direct activation of circulating leukocytes and trigger neutrophil-elastase glycocalyx destruction, (97–99) and (4) atrial natriuretic peptide which has been found to induce glycocalyx damage in some human studies (26, 100, 101).
The effect of the type of fluid administered may also play a role in causing glycocalyx damage with sepsis. Both clinical and preclinical data suggest that balanced crystalloids, albumin, fresh frozen plasma, and synthetic colloids may be less injurious compared to isotonic saline (102–104). It is possible that albumin preserves the glycocalyx and may be more beneficial compared to isotonic crystalloids in experimental studies, (105, 106) but results of glycocalyx evaluation following albumin therapy in septic patients are not yet available. Albumin therapy in the form of concentrated albumin or plasma products has sparked interest for its potential ability to protect the glycocalyx due to its ability to carry erythrocyte-derived sphingosine-1-phosphate to the endothelium; this may mediate glycocalyx recovery by suppressing metalloproteinase activity (107–109). It has even been suggested that individual patients should receive tailored fluid treatment plans based on admission markers of endothelial glycocalyx damage to avoid the deleterious consequences of fluid administration to patients with high risk for vascular leakage and subsequent organ dysfunction (109).
In conclusion, the hemodynamic coherence between the macro- and microcirculation is often poor in shock states such as hemorrhage or sepsis. Various diseases lead to vascular changes that may not be readily apparent with current monitoring strategies. Therefore, intravenous fluid resuscitation strategies must take into account not only microcirculatory parameters such as systemic arterial blood pressure, but also downstream measures and/or microcirculatory assessments of the patient's response to treatment. Continued research focusing on the effects or different fluid therapy prescriptions on the macro- and microcirculation/endothelial surface layer in various disease states, the goals and timing of its administration, and ultimately outcome of the patients will likely change fluid therapy in the future.
Both authors listed have made a substantial, direct and intellectual contribution to the work, and approved it for publication.
The authors declare that the research was conducted in the absence of any commercial or financial relationships that could be construed as a potential conflict of interest.
The Supplementary Material for this article can be found online at: https://www.frontiersin.org/articles/10.3389/fvets.2021.625708/full#supplementary-material
Supplementary Video 1. Sidestream dark-field microscopy from a healthy dog.
Supplementary Video 2. Sidestream dark-field microscopy from a dog with hemorrhagic shock.
Supplementary Video 3. Sidestream dark-field microscopy from a dog with septic peritonitis.
1. Boulpaep E. The microcirculation. In: Boron WF, Boulpaep EL, , editors. Medical Physiology, 1st ed. Philadelphia, PA: Saunders Elsevier (2009). p. 463–82. doi: 10.1016/B978-1-4160-3115-4.50023-8
2. Sieve I, Münster-Kühnel AK, Hilfiker-Kleiner D. Regulation and function of endothelial glycocalyx layer in vascular diseases. Vascul Pharmacol. (2018) 100:26–33. doi: 10.1016/j.vph.2017.09.002
3. Reines BP, Ninham BW. Structure and function of the endothelial surface layer: unraveling the nanoarchitecture of biological surfaces. Q Rev Biophys. (2019) 52:e13. doi: 10.1017/S0033583519000118
4. Iba T, Levy JH. Derangement of the endothelial glycocalyx in sepsis. J Thromb Haemost. (2019) 17:283–94. doi: 10.1111/jth.14371
5. Weinbaum S, Tarbell JM, Damiano ER. The structure and function of the endothelial glycocalyx layer. Annu Rev Biomed Eng. (2007) 9:121–67. doi: 10.1146/annurev.bioeng.9.060906.151959
6. Alphonsus CS, Rodseth RN. The endothelial glycocalyx: a review of the vascular barrier. Anaesthesia. (2014) 69:777–84. doi: 10.1111/anae.12661
7. Becker BF, Chappell D, Jacob M. Endothelial glycocalyx and coronary vascular permeability: the fringe beneft. Basic Res Cardiol. (2010) 105:687–701. doi: 10.1007/s00395-010-0118-z
8. Esko JD, Kimata K, Lindahl U. Chapter 16. Proteoglycans and sulfated glycosaminoglycans. In: Varki A, Cummings RD, Esko JD, Freeze HH, Stanley P, Bertozzi CR, Hart GW, Etzler ME, , editors. Essentials of Glycobiology. Cold Spring Harbor, NY: Cold Spring Harbor Laboratory Press (2009). p. 145–60.
9. Broekhuizen LN, Mooij HL, Kastelein JJ, Stroes ESG, Vink H, Nieuwdorp M. Endothelial glycocalyx as potential diagnostic and therapeutic target in cardiovascular disease. Curr Opin Lipidol. (2009) 20:57–62. doi: 10.1097/MOL.0b013e328321b587
10. Reitsma S, Slaaf DW, Vink H, Nieuwdorp M. The endothelial glycocalyx: composition, functions, and visualization. Pflug Arch. (2007) 454:345–59. doi: 10.1007/s00424-007-0212-8
11. Woodcock TE, Woodcock TM. Revised Starling equation and the glycocalyx model of transvascular fluid exchange: an improved paradigm for prescribing intravenous fluid therapy. Br J Anaesth. (2012) 3:384–94. doi: 10.1093/bja/aer515
12. Guyton A. Nervous regulation of the circulation, and rapid control of arterial pressure. In: Hall JD, , editor. Textbook of Medical Physiology, 13th ed. Philadelphia, PA: Elsevier Inc. (2016). p. 215–26.
13. Dinnar U. Metabolic and mechanical control of the microcirculation. In: Sideman S, Beyar R, , editors. Interactive Phenomena in the Cardiac System. New York, NY: Plenum Press (2019). p. 243–54. doi: 10.1007/978-1-4615-2946-0_23
14. Guyton A. Dominant role of the kidney in long-term regulation of arterial pressure and in hypertension: the integrated system for pressure control. In: Hall JE, , editor. Textbook of Medical Physiology, 13th ed. Philadelphia, PA: Elsevier Inc. (2016). p. 227–44.
15. Ganong W. Cardiovascular regulatory mechanisms. In: Barrett KE, Barman SM, Brooks HL, Yuan J, , editors. Review of Medical Physiology, 26th ed. New York, NY: Lange- McGraw-Hill (2019). p. 575–88.
16. Segal S. Regulation of blood flow in the microcirculation. Microcirculation. (2005) 12:33–45. doi: 10.1080/10739680590895028
17. Cabrales P, Intaglietta M, Tsai AG. Increase plasma viscosity sustains microcirculation after resuscitation from hemorrhagic shock and continuous bleeding. Shock. (2005) 23:549–55.
18. Dinenno FA. Skeletal muscle vasodilation during systemic hypoxia in humans. J Appl Physiol. (2016) 120:216–25. doi: 10.1152/japplphysiol.00256.2015
19. Zakaria R, Tsakadze NL, Garrison RN. Hypertonic saline resuscitation improves intestinal microcirculation in a rat model of hemorrhagic shock. Surgery. (2006) 140:579–87. doi: 10.1016/j.surg.2006.05.015
20. Szabo C, Thiemermann C. Invited opinion: role of nitric oxide in hemorrhagic, traumatic, and anaphylactic shock and thermal injury. Shock. (1994) 2:145–55. doi: 10.1097/00024382-199408000-00011
21. Szopinski J, Kusza K, Semionow M. Microcirculatory responses to hypovolemic shock. J Trauma. (2011) 71:1779–88. doi: 10.1097/TA.0b013e31823a05b5
22. Tachon G, Harrois A, Tanaka S, Kato H, Huet O, Pottecher J, et al. Microcirculatory alterations in traumatic hemorrhagic shock. Crit Care Med. (2014) 42:1433–41. doi: 10.1097/CCM.0000000000000223
23. Kozar RA, Peng Z, Zhang R, Holcomb J, Pati S, Park P, et al. Plasma restoration of endothelial glycocalyx in a rodent model of hemorrhagic shock. Anesth Analg. (2011) 112:1289–95. doi: 10.1213/ANE.0b013e318210385c
24. TorresFilho I, Torres LN, Sondeen JL, Polykratis A, Dubick M. In vivo evaluation of venular glycocalyx during hemorrhagic shock in rats using intravital microscopy. Microvasc Res. (2013) 85:128–33. doi: 10.1016/j.mvr.2012.11.005
25. Guerci P, Ergin B, Uz Z, Ince Y, Westphal M, Heger M, et al. Glycocalyx degradation is independent of vascular barrier permeability increase in nontraumatic hemorrhagic shock in rats. Anesth Analg. (2019) 129:598–607. doi: 10.1213/ANE.0000000000003918
26. Singer M, Deutschman CS, Seymour CW, Shankar-Hari M, Annane D, Bauer M, et al. The third international consensus definitions for sepsis and septic shock (Sepsis-3). JAMA. (2016) 315:801–10. doi: 10.1001/jama.2016.0287
27. Rhodes A, Evans LE, Alhazzani W, Levy M, Antonelli M, Ferrer R, et al. Surviving sepsis campaign: international guidelines for management of sepsis and septic shock: 2016. Intensive Care Med. (2017) 43:304–77. doi: 10.1007/s00134-017-4683-6
28. DeBacker D, Creteur J, Preiser JC, Dubois MJ, Vincent JL. Microvascular blood flow is altered in patients with sepsis. Am J Respir Crit Care Med. (2002) 166:98–104. doi: 10.1164/rccm.200109-016OC
29. Edul VS, Enrico C, Laviolle B, Vazques AR, Ince C, Dubin A. Quantitative assessment of the microcirculation in healthy volunteers and in patients with septic shock. Crit Care Med. (2012) 40:1443–8. doi: 10.1097/CCM.0b013e31823dae59
30. Massey MJ, Hou PC, Filbin M, Wang H, Ngo L, Huang D, et al. Microcirculatory perfusion disturbances in septic shock: results from the ProCESS trial. Crit Care. (2018) 22:308. doi: 10.1186/s13054-018-2240-5
31. Shih CC, Liu CM, Chao A, Lee CT, Hsu YC, Yeh YC. Matched comparison of microcirculation between healthy volunteers and patients with sepsis. Asian J Anesthesiol. (2018) 56:14–22. doi: 10.6859/aja.201803_56(1).0002
32. Trzeciak S, Dellinger RP, Parrillo JE, Guglielmi M, Bajaj J, Abate NL, et al. Early microcirculatory perfusion derangements in patients with severe sepsis and septic shock: relationship to hemodynamics, oxygen transport, and survival. Ann Emerg Med. (2007) 49:88–98.e2. doi: 10.1016/j.annemergmed.2006.08.021
33. Hernandez G, Boerma EC, Dubin A, Bruhn A, Koopmans M, Edul VK, et al. Severe abnormalities in microvascular perfused vessel density are associated to organ dysfunctions and mortality and can be predicted by hyperlactatemia and norepinephrine requirements in septic shock patients. J Crit Care. (2013) 28:538.e9–14. doi: 10.1016/j.jcrc.2012.11.022
34. DeBacker D, Ortiz JA, Salgado D. Coupling microcirculation to systemic hemodynamics. Curr Opin Crit Care. (2010) 16:250–4. doi: 10.1097/MCC.0b013e3283383621
35. DeBacker D, OrbegozoCortes D, Donadello K, Vincent JL. Pathophysiology of microcirculatory dysfunction and the pathogenesis of septic shock. Virulence. (2014) 5:73–9. doi: 10.4161/viru.26482
36. Verdant CL, DeBacker D, Bruhn A, Clausi C, Su F, Wang Z, et al. Evaluation of sublingual and gut mucosal microcirculation in sepsis: a quantitative analysis. Crit Care Med. (2009) 37:2875–81. doi: 10.1097/CCM.0b013e3181b029c1
37. Boerma EC, vander Voort PHJ, Spronk PE, Ince C. Relationship between sublingual and intestinal microcirculatory perfusion in patients with abdominal sepsis*. Critical Care Medicine. (2007) 35:1055–60. doi: 10.1097/01.CCM.0000259527.89927.F9
38. Lima A, vanRooij T, Ergin B, Sorelli M, Ince Y, Specht PAC, et al. Dynamic contrast-enhanced ultrasound identifies microcirculatory alterations in sepsis-induced acute kidney injury. Crit Care Med. (2018) 46:1284–92. doi: 10.1097/CCM.0000000000003209
39. Silverstein DC, Pruett-Saratan A, Drobatz KJ. Measurements of microvascular perfusion in healthy anesthetized dogs using orthogonal polarization spectral imaging. J Vet Emerg Crit Care. (2009) 19:579–87. doi: 10.1111/j.1476-4431.2009.00488.x
40. Peruski AM, Cooper ES. Assessment of microcirculatory changes by use of sidestream dark field microscopy during hemorrhagic shock in dogs. Am J Vet Res. (2011) 72:438–45. doi: 10.2460/ajvr.72.4.438
41. An X, Zhang H, Sun Y, Ma X. The microcirculatory failure could not weaken the increase of systematic oxygen extraction rate in septic shock: an observational study in canine models. Clin Hemorheol Microcirc. (2016) 63:267–79. doi: 10.3233/CH-152022
42. Chelazzi C, Villa G, Mancinelli P, DeGaudio AR, Adembri C. Glycocalyx and sepsis-induced alterations in vascular permeability. Crit Care. (2015) 19:26. doi: 10.1186/s13054-015-0741-z
43. Fleck A, Hawker F, Wallace PI, Ledingham IM, Calman KC. Increased vascular permeability: a major cause of hypoalbuminaemia in disease and injury. Lancet. (1985) 325:781–4. doi: 10.1016/S0140-6736(85)91447-3
44. Uchimido R, Schmidt EP, Shapiro NI. The glycocalyx: a novel diagnostic and therapeutic target in sepsis. Crit Care. (2019) 23:16. doi: 10.1186/s13054-018-2292-6
45. Inagawa R, Okada H, Takemura G, Suzuki K, Takada C, Yano H, et al. Ultrastructural alteration of pulmonary capillary endothelial glycocalyx during endotoxemia. Chest. (2018) 154:317–25. doi: 10.1016/j.chest.2018.03.003
46. Rovas A, Seidel LM, Vink H, Pohlkötter T, Pavenstädt H, Ertmer C, et al. Association of sublingual microcirculation parameters and endothelial glycocalyx dimensions in resuscitated sepsis. Critical Care. (2019) 23:260. doi: 10.1186/s13054-019-2542-2
47. Investigators A, Group ACT, Peake SL, Delaney A, Bailey M, Bellomo R, et al. Goal-directed resuscitation for patients with early septic shock. N Engl J Med. (2014) 371:1496–506. doi: 10.1056/NEJMoa1404380
48. Investigators P, Rowan KM, Angus DC, Bailey M, Barnato AE, Bellomo R, et al. Early, goal-directed therapy for septic shock — a patient-level meta-analysis. N Engl J Med. (2017) 376:2223–34. doi: 10.1056/NEJMoa1701380
49. Pro CI, Yealy DM, Kellum JA, Weissfeld LA, Willochell M, Derek AC, et al. A randomized trial of protocol-based care for early septic shock. N Engl J Med. (2014) 370:1683–93. doi: 10.1056/NEJMoa1401602
50. Ince C, Mik EG. Microcirculatory and mitochondrial hypoxia in sepsis, shock, and resuscitation. J Appl Physiol. (2016) 120:226–35. doi: 10.1152/japplphysiol.00298.2015
51. DeBacker D, Durand A. Monitoring the microcirculation in critically ill patients. Best Pract Res Clin Anaesthesiol. (2014) 28:441–51. doi: 10.1016/j.bpa.2014.09.005
52. Ranzani OT, Monteiro MB, Ferreira EM, Santos SR, Machado FR, Noritomi DT. Reclassifying the spectrum of septic patients using lactate: severe sepsis, cryptic shock, vasoplegic shock and dysoxic shock. Rev Bras Ter Intensiva. (2013) 25:270–8. doi: 10.5935/0103-507X.20130047
53. DeBacker D, Donadello K, Sakr Y, Ospina-Tascon G, Salgado D, Scolletta S, et al. Microcirculatory alterations in patients with severe sepsis: impact of time of assessment and relationship with outcome. Crit Care Med. (2013) 41:791–9. doi: 10.1097/CCM.0b013e3182742e8b
54. Kara A, Akin S, Ince C. Monitoring microcirculation in critical illness. Curr Opin Crit Care. (2016) 22:444–52. doi: 10.1097/MCC.0000000000000335
55. Ocak I, Kara A, Ince C. Monitoring microcirculation. Best Pract Res Clin Anaesthesiol. (2016) 30:407–18. doi: 10.1016/j.bpa.2016.10.008
56. Ince C, Boerma EC, Cecconi M, DeBacker D, Shapiro N, Duranteau J, et al. Second consensus on the assessment of sublingual microcirculation in critically ill patients: results from a task force of the European society of intensive care medicine. Intensive Care Med. (2018) 44:281–99. doi: 10.1007/s00134-018-5070-7
57. Ohansson P, Stensballe J, Ostrowski S. Shock induced endotheliopathy (SHINE) in acute critical illness - a unifying pathophysiologic mechanism. Crit Care. (2017) 21:25. doi: 10.1186/s13054-017-1605-5
58. Janczyk P, Hansen S, Bahramsoltani M, Plendl J. The glycocalyx of human, bovine and murine microvascular endothelial cells cultured in vitro. J Electron Microsc (Tokyo). (2010) 59:291–8. doi: 10.1093/jmicro/dfq007
59. Pries AR, Secomb TW, Gaehtgens P. The endothelial surface layer. Pflugers Arch. (2000) 440:653–66. doi: 10.1007/s004240000307
60. Ebong EE, Macaluso FP, Spray D, Tarbell JM. Imaging the endothelial glycocalyx in vitro by rapid freezing/freeze substitution transmission electron microscopy. Arterioscler Thromb Vasc Biol. (2011) 31:1908–15. doi: 10.1161/ATVBAHA.111.225268
61. Gretz JE, Duling BR. Measurement uncertainties associated with the use of bright-field and fluorescence microscopy in the microcirculation. Microvasc Res. (1995) 49:134–40. doi: 10.1006/mvre.1995.1011
62. Kataoka H, Ushiyama A, Kawakami H, Akimoto Y, Matsubara S, Iijima T. Fluorescent imaging of endothelial glycocalyx layer with wheat germ agglutinin using intravital microscopy. Microsc Res Tech. (2016) 79:31–7. doi: 10.1002/jemt.22602
63. Smith ML, Long DS, Damiano ER, Ley K. Near-wall micro-PIV reveals a hydrodynamically relevant endothelial surface layer in venules in vivo. Biophys J. (2003) 85:637–45. doi: 10.1016/S0006-3495(03)74507-X
64. Megens RT, Reitsma S, Schiffers PH, Hilgers RHP, DeMey JGR, Slaaf DW, et al. Two-photon microscopy of vital murine elastic and muscular arteries. Combined structural and functional imaging with subcellular resolution. J Vasc Res. (2007) 44:87–98. doi: 10.1159/000098259
65. Londono L, Bowen CM, Buckley GJ. Evaluation of the endothelial glycocalyx in healthy anestheized dogs using rapid, patient-side GlycoCheck analysis software. J Vet Emerg Crit Care. (2018) 28:S7.
66. Millar KK, Yozova Y, Londono L, Munday J, Thomson N, Sano H. Evaluation of the endothelial glycocalyx in healthy anesthetized cats using rapid, patient-side glycocheck analysis software. J Vet Emerg Crit Care. (2019) 29:S11.
67. Mullen KM, Regier PJ, Londono L, Millar K, Groover J. Evaluation of jejunal microvasculature of healthy anaesthetized dogs with sidestream dark field video microscopy. Am J Vet Res. (2020) 81:888–93. doi: 10.2460/ajvr.81.11.888
68. Yozova ID, Londono L, Sano H, Thomson N, Munday J, Cave N. Assessment of the endothelial glycocalyx after a fluid bolus in healthy anesthetized cats using rapid, patient-side glycocheck analysis software. J Vet Emerg Crit Care. (2020) 30:S27.
69. Lee DH, Dane MJ, vanden Berg BM, Boels MG, vanTeeffelen JW, deMutsert R, et al. Deeper penetration of erythrocytes into the endothelial glycocalyx is associated with impaired microvascular perfusion. PLoS ONE. (2014) 9:e96477. doi: 10.1371/journal.pone.0096477
70. Dutton RP. Management of traumatic haemorrhage–the US perspective. Anaesthesia. (2015) 70(Suppl 1):108–11.e38. doi: 10.1111/anae.12894
71. Torres LN, Chung KK, Salgado CL, Dubick MA, TorresFilho IP. Low-volume resuscitation with normal saline is associated with microvascular endothelial dysfunction after hemorrhage in rats, compared to colloids and balanced crystalloids. Crit Care. (2017) 21:160. doi: 10.1186/s13054-017-1745-7
72. Ati S, Potter DR, Baimukanova G, Farrel DH, Holcomb JB, Schreiber MA. Modulating the endotheliopathy of trauma: factor concentrate versus fresh frozen plasma. J Trauma Acute Care Surg. (2016) 80:576–85. doi: 10.1097/TA.0000000000000961
73. Milford EM, Reade MC. Resuscitation fluid choices to preserve the endothelial glycocalyx. Crit Care. (2019) 23:77. doi: 10.1186/s13054-019-2369-x
74. Chappell D, Bruegger D, Potzel J, Jacob M. Hypervolemia increases release of atrial natriuretic peptide and shedding of the endothelial glycocalyx. Crit Care. (2014) 18:538. doi: 10.1186/s13054-014-0538-5
75. Tuma M, Canestrini Z, Alwahab Z, Marshall J. Trauma and endothelial glycocalyx: the microcirculatory helmet? Shock. (2016) 46:352–7. doi: 10.1097/SHK.0000000000000635
76. Naumann DN, Beaven A, Dretzke J, Hutchings S, Midwinter MJ. Searching for the optimal fluid to restore microcirculatory flowdynamcis after hemorrhagic shock: a systematic review of preclinical studies. Shock. (2016) 46:609–22. doi: 10.1097/SHK.0000000000000687
77. Amemann P, Hessler M, Kampmeier T, Seidel L, Malek Y, VanAken H, et al. Resuscitation with hydroxyethyl starch maintais hemodynamic coherence in ovine hemorrhagic shock. Anesthesiology. (2020) 132:131–9. doi: 10.1097/ALN.0000000000002998
78. Hutchings SD, Naumann DN, Hopkins P, Mellis C, Riozzi P, Sartini S, et al. Microcirculatory impairment is associated with multiple organ dysfunction following traumatic hemorrhagic shock: the MICROSHOCK study. Crit Care Med. (2018) 46:889–96. doi: 10.1097/CCM.0000000000003275
79. Weinberg JA, MacLennan PA, Vandromme-Cusick MJ, Angotti JM, Magnotti LJ, Kerby JD, et al. Microvascular response to red blood cell transfusion in trauma patients. Shock. (2012) 37:276–81. doi: 10.1097/SHK.0b013e318241b739
80. Tanaka S, Escudier E, Hamada S, Harrois A, Leblanc PE, Vicaut E, et al. Effect of RBC transfusion on sublingual microcirculation in hemorrhagic shock patients: a pilot study. Crit Care Med. (2017) 45:154–60. doi: 10.1097/CCM.0000000000002064
81. Damiani E, Adrario E, Luchetti MM, Scorcella C, Carsetti A, Mininno N, et al. Plasma free hemoglobin and microcirculatory response to fresh or old blood transfusions in sepsis. PLoS ONE. (2015) 10:e0122655. doi: 10.1371/journal.pone.0122655
82. Gruen DS, Brown JB, Guyette FX, Vodovotz Y, Johansson PI, Stensballe J, et al. Prehospital plasma is associated with distinct biomarker expression following injury. JCI. (2020) 5:e135350. doi: 10.1172/jci.insight.135350
83. Ospina-Tascon G, Neves AP, Occhipinti G, Donadello K. Effects of fluids on microvascular perfusion in patients with severe sepsis. Intensive Care Med. (2010) 36:949–55. doi: 10.1007/s00134-010-1843-3
84. Maitland K, Kiguli S, Opoka RO, Engoru C, Olupot-Plupot P, Akech SO, et al. Mortality after fluid bolus in African children with severe infection. N Engl J Med. (2011) 364:2483–95. doi: 10.1056/NEJMoa1101549
85. Andrews B, Semler MW, Muchemwa L, Kelly P, Lakhi S, Heimburger DC, et al. Effect of an early resuscitation protocol on in-hospital mortality among adults with sepsis and hypotension: a randomized clinical trial. JAMA. (2017) 318:1233–40. doi: 10.1001/jama.2017.10913
86. Edul VS, Ince C, Navarro N, Previgliano L, Risso-Vazquez A, Rubatto PN, et al. Dissociation between sublingual and gut microcirculation in the response to a fluid challenge in postoperative patients with abdominal sepsis. Ann Intensive Care. (2014) 4:39. doi: 10.1186/s13613-014-0039-3
87. Hippensteel JA, Uchimido R, Tyler PD, Burke RC, Han X, Zhang F, et al. Intravenous fluid resuscitation is associated with septic endothelial glycocalyx degradation. Crit Care. (2019) 23:259. doi: 10.1186/s13054-019-2534-2
88. Silversides JA, Major E, Ferguson AJ, Mann EE, McAuley DF, Marshall JC, et al. Conservative fluid management or deresuscitation for patients with sepsis or acute respiratory distress syndrome following the resuscitation phase of critical illness: a systematic review and meta-analysis. Intensive Care Med. (2017) 43:155–70. doi: 10.1007/s00134-016-4573-3
89. Marik PE, Linde-Zwirble WT, Bittner EA, Sahatjian J, Hansell D. Fluid administration in severe sepsis and septic shock, patterns and outcomes: an analysis of a large national database. Intensive Care Med. (2017) 43:625–32. doi: 10.1007/s00134-016-4675-y
90. Acheampong A, Vincent JL. A positive fluid balance is an independent prognostic factor in patients with sepsis. Crit Care. (2015) 19:251. doi: 10.1186/s13054-015-0970-1
91. Boyd JH, Forbes J, Nakada TA, Walley KR, Russell JA. Fluid resuscitation in septic shock: a positive fluid balance and elevated central venous pressure are associated with increased mortality. Crit Care Med. (2011) 39:259–65. doi: 10.1097/CCM.0b013e3181feeb15
92. Sadaka F, Juarez M, Naydenov S, O'Brien J. Fluid resuscitation in septic shock: the effect of increasing fluid balance on mortality. J Intensive Care Med. (2014) 29:213–7. doi: 10.1177/0885066613478899
93. Vincent JL, Sakr Y, Sprung CL, Ranieri VM, Reihart K, Gerlach H, et al. Sepsis in European intensive care units: results of the SOAP study. Crit Care Med. (2006) 34:344–53. doi: 10.1097/01.CCM.0000194725.48928.3A
94. Byrne L, Obonyo NG, Diab SD, Dunster KR, Passmore MR, Boon AC, et al. Unintended consequences: fluid resuscitation worsens shock in an ovine model of endotoxemia. Am J Respir Crit Care Med. (2018) 198:1043–54. doi: 10.1164/rccm.201801-0064OC
95. Kang H, Duran CL, Abbey CA, Kaunas RR, Bayless KJ. Fluid shear stress promotes proprotein convertase-dependent activation of MT1-MMP. Biochem Biophys Res Commun. (2015) 460:596–602. doi: 10.1016/j.bbrc.2015.03.075
96. Platt MO, Ankeny RF, Jo H. Laminar shear stress inhibits cathepsin L activity in endothelial cells. Arterioscler Thromb Vasc Biol. (2006) 26:1784–90. doi: 10.1161/01.ATV.0000227470.72109.2b
97. vanHaren FM, Sleigh J, Cursons R, LaPine M, Pickkers P, vander Hoeven JG. The effects of hypertonic fluid administration on the gene expression of inflammatory mediators in circulating leucocytes in patients with septic shock: a preliminary study. Ann Intensive Care. (2011) 1:44. doi: 10.1186/2110-5820-1-44
98. Rhee P, Wang D, Ruff P, Austin B, DeBraux S, Wolcott K, et al. Human neutrophil activation and increased adhesion by various resuscitation fluids. Crit Care Med. (2000) 28:74–8. doi: 10.1097/00003246-200001000-00012
99. Suzuki K, Okada H, Takemura G, Takada C, Kuroda A, Yano H, et al. Neutrophil elastase damages the pulmonary endothelial glycocalyx in lipopolysaccharide-induced experimental endotoxemia. Am J Pathol. (2019) 189:1526–35. doi: 10.1016/j.ajpath.2019.05.002
100. Jacob M, Saller T, Chappell D, Rehm M, Welsch U, Becker BF. Physiological levels of A-, B- and C-type natriuretic peptide shed the endothelial glycocalyx and enhance vascular permeability. Basic Res Cardiol. (2013) 108:347. doi: 10.1007/s00395-013-0347-z
101. Bruegger D, Jacob M, Rehm M, Loetsch M, Welsch U, Conzen P, et al. Atrial natriuretic peptide induces shedding of endothelial glycocalyx in coronary vascular bed of guinea pig hearts. Am J Physiol Heart Circ Physiol. (2005) 289:H1993–9. doi: 10.1152/ajpheart.00218.2005
102. Semler MW, Self WH, Wanderer JP, Ehrenfeld JM, Wang L, Byrne DW, et al. Balanced crystalloids versus saline in critically ill adults. N Engl J Med. (2018) 378:829–39. doi: 10.1056/NEJMoa1711584
103. Finfer S, McEvoy S, Bellomo R, McArthur C, Myburgh J, Norton R. . Impact of albumin compared to saline on organ function and mortality of patients with severe sepsis. Intensive Care Med. (2011) 37:86–96. doi: 10.1007/s00134-010-2039-6
104. Müller RB, Ostrowski SR, Haase N, Wetterslev J, Perner A, Johansson PI. Markers of endothelial damage and coagulation impairment in patients with severe sepsis resuscitated with hydroxyethyl starch 130/0.42 vs Ringer acetate. J Crit Care. (2016) 32:16–20. doi: 10.1016/j.jcrc.2015.11.025
105. Aldecoa C, Llau JV, Nuvials X, Artigas A. Role of albumin in the preservation of endothelial glycocalyx integrity and the microcirculation: a review. Ann Intensive Care. (2020) 10:85. doi: 10.1186/s13613-020-00697-1
106. Bansch P, Statkevicius S, Bentzer P. Plasma volume expansion with 5% albumin compared to Ringer's acetate during normal and increased microvascular permeability in the rat. Anesthesiology. (2014) 121:817–24. doi: 10.1097/ALN.0000000000000363
107. Adamson RH, Clark JF, Radeva M, Kheirolomoom A, Ferrara KW, Curry FE. Albumin modulates S1P delivery from red blood cells in perfused microvessels: mechanism of the protein effect. Am J Physiol Heart Circ Physiol. (2014) 306:H1011–7. doi: 10.1152/ajpheart.00829.2013
108. Zeng Y, Adamson RH, Curry FRE, Tarbell JM. Sphingosine-1-phosphate protects endothelial glycocalyx by inhibiting syndecan-1 shedding. Am J Physiol Heart Circ Physiol. (2014) 306:H363–72. doi: 10.1152/ajpheart.00687.2013
Keywords: microcirculation, macrocirculation, shock, sepsis, hemorrhage, glycocalyx
Citation: Cooper ES and Silverstein DC (2021) Fluid Therapy and the Microcirculation in Health and Critical Illness. Front. Vet. Sci. 8:625708. doi: 10.3389/fvets.2021.625708
Received: 03 November 2020; Accepted: 06 April 2021;
Published: 13 May 2021.
Edited by:
Pablo Martín-Vasallo, University of La Laguna, SpainReviewed by:
Angela Briganti, University of Pisa, ItalyCopyright © 2021 Cooper and Silverstein. This is an open-access article distributed under the terms of the Creative Commons Attribution License (CC BY). The use, distribution or reproduction in other forums is permitted, provided the original author(s) and the copyright owner(s) are credited and that the original publication in this journal is cited, in accordance with accepted academic practice. No use, distribution or reproduction is permitted which does not comply with these terms.
*Correspondence: Edward S. Cooper, Y29vcGVyLjE2OTdAb3N1LmVkdQ==
Disclaimer: All claims expressed in this article are solely those of the authors and do not necessarily represent those of their affiliated organizations, or those of the publisher, the editors and the reviewers. Any product that may be evaluated in this article or claim that may be made by its manufacturer is not guaranteed or endorsed by the publisher.
Research integrity at Frontiers
Learn more about the work of our research integrity team to safeguard the quality of each article we publish.