- 1Department of Clinical Sciences, College of Veterinary Medicine and Biomedical Sciences, Center for Immune and Regenerative Medicine, Colorado State University, Ft. Collins, CO, United States
- 2Department of Clinical Sciences, College of Veterinary Medicine and Biomedical Sciences, Colorado State University, Ft. Collins, CO, United States
- 3Department of Biomedical Sciences, College of Veterinary Medicine and Biomedical Sciences, Colorado State University, Ft. Collins, CO, United States
Advances in stem cell technology, including the use of induced pluripotent stem cells (iPSC) to produce neurons and glial cells, offer new hope for patients with neurological disease and injuries. Pet dogs with spinal cord injuries provide an important spontaneous animal model for evaluating new approaches to stem cell therapy. Therefore, studies were conducted to identify optimal conditions for generating neural progenitor cells (NPC) from canine induced pluripotent stem cells (iPSC) for preliminary evaluation in animals with spinal cord injury. We found that canine NPC could be induced to differentiate into mature neural cells, including glia and neurons. In addition, canine NPC did not form teratomas when injected in NOD/SCID mice. In a pilot study, two dogs with chronic spinal cord injury underwent fluoroscopically guided intrathecal injections of canine NPC. In follow-up MRI evaluations, tumor formation was not observed at the injection sites. However, none of the animals experienced meaningful clinical or electrophysiological improvement following NPC injections. These studies provide evidence that canine iPSC can be used to generate NPC for evaluation in cellular therapy of chronic spinal cord injury in the dog spontaneous injury model. Further refinements in the cell implantation procedure are likely required to enhance stem cell treatment efficacy.
Introduction
Stem cell therapy offers new hope for patients suffering from spinal cord injury (SCI) (1, 2). A number of small clinical trials have investigated the potential for stem cell transplantation in SCI, including mesenchymal stem cells and neural stem cells derived from embryonic stem cells and directly from spinal tissue (3–7). In general, human clinical trials of stem cell therapy in SCI have demonstrated modest improvements in spinal cord function following cellular therapy, but recovery is varied and the optimal cell type for transplantation is yet to be determined (8). Induced pluripotent stem cells (iPSC) represent one potential new source of cells for therapy of SCI (9). Human iPSCs have been shown to differentiate into functional neurons and glial cells after engraftment into injured rat and mouse spinal cords; and grow long axons that extend through the injury sites and form connections with existing neurons (9–13). However, there are also significant potential risks to the use of iPSC derived stem cells for cellular therapy, including in particular the risk of the tumor formation, particularly teratomas (14); combined with the difficulties of generating stable, clinical grade iPS derived neural cell lines (15). Thus, there is considerable interest in determining whether iPSC-derived cells are effective for SCI, and whether they can be administered without the risk of tumor formation.
Large animal testing in models other than rodent induced SCI are essential to fully assess the safety and clinical efficacy of stem cell therapy (16, 17). Pet dogs with spontaneous SCI, typically due to intervertebral disk rupture or automobile trauma, offer a valuable and realistic animal model for spinal cord injury research. Numerous research studies with implanted mesenchymal stem cells (MSC) have been performed in research dogs with induced SCI (18–21). In addition, several clinical trials have reported the results of MSC injections in spontaneous, chronic SCI in dogs, with some evidence of increased locomotion, and no adverse effects from the injections (22–25). To date, we are unaware of the evaluation of iPSC-derived stem cells in natural or induced SCI in dogs.
Therefore, in this report we evaluated whether canine iPSC could be induced to differentiate into NPCs and to form mature neural cells in vitro. In addition, the safety and tumorogenicity of canine NPCs was evaluated in mouse models. Finally, studies were also done to assess the safety and potential efficacy of NPCs following intra-spinal injection in dogs with naturally-occurring spinal cord injuries.
Materials and Methods
Generation of Canine Neural Progenitor Cells (NPCs or NPC)
A canine iPS cell line was generated as previously described (26) from skin biopsy fibroblast derived from a 6-year old male standard poodle To differentiate iPS cells into Neurospheres (neural progenitor cells), colonies were digested to single cell suspension and re-suspended in media containing DMEM/F12 (27) (Life Technologies Corp. Grand Island NY), Antibiotic-Antimycotic, B27 (Thermo Fisher Scientific Waltham, MA), 20 ng/mL Recombinant Human FGF-basic (Fibroblast Growth Factor-basic, bFGF), 20 ng/mL Recombinant Human EGF (Epidermal Growth Factor) (PeproTech, Rock Hill, NJ), and 2 μg/mL Heparin (Sigma-Aldrich, St. Louis MO). Dissociated cells were plated in 100 mm ultra-low attachment culture dishes (Corning Inc. Corning, NY) at a density of 4 × 105 cells/mL, on day 10 neurospheres were collected and used for injection or for further differentiation.
Generation of Mature Neurons and Astrocytes
NPCs were collected and plated on round cover slips coated with 20 μg/mL laminin and poly-ornithine (Sigma-Aldrich, St. Louis MO) in a 24 well cell culture plate (Corning Inc. Corning, NY). Mature neurons were grown in neuronal growth media containing DMEM/F12, B27, N2, Glutamax, antibiotic-antimycotic solution, and NEAA (Thermo Fisher Scientific Waltham, MA). Astrocytes were grown with the addition of 1% fetal bovine serum (VWR Life Science, Radnor, PA). Mature neurons and astrocytes were detached using Accutase (Stemcell Technologies Inc. Vancouver, Canada) after 14 days and processed for PCR or stained using ICC as described below.
Generation of Oligodendrocytes
NPCs were plated onto laminin/ poly-ornithine coated cover slips in oligodendrocyte differentiation base media, containing DMEM/F12, 50% Glucose, Glutamax, Sodium Pyruvate. N2, penicllin-streptomcin, and 20 ng/mL bFGF was added to oligo base media from day 0–4, 20 ng/mL bFGF and EGF was added from day 5–8, bFGF, EGF and also 10 ng/mL PDGF-AA (Platelet-Derived Growth Factor-AA) (PeproTech, Rock Hill, NJ) was added from day 9–12. After day 12, 30 ng/mL of T3 (3,3,5 triiodo-thryonine) (Sigma-Aldrich, St. Louis, MO) was added to oligodendrocyte differentiation base until the appearance of oligodendrocytes (~6–8 days later), which were collected for PCR or stained using ICC.
Immunocytochemical (ICC) Evaluation of Differentiated Neurons and Astrocytes
ICC was performed according to previously published protocol (28). Primary antibodies used in these studies include: anti-vimentin (clone V9; Merck Millipore, Billerica, MA), anti-TUJ1 (beta III tubulin; clone TUJ1 Covance Inc. San Diego, CA), and anti-MAP2α (clone AP20; Merck Millipore, Billerica, MA). Corresponding rabbit and mouse irrelevant isotype antibodies were used at concentrations matching the primary antibodies (eBioscience Inc, San Diego CA). Secondary antibodies used were donkey anti mouse IgG or donkey anti rabbit IgG (Jackson ImmunoResearch Laboratories, Inc. West Grove, PA) conjugated to Cy3. Visualization of florescence staining was performed on Olympus IX83 spinning disk confocal microscope. Images were imported as Tiff files to Photoshop CC 2015, and adjusted with high definition resolution (HDR) toning. For each stain, HDR toning was applied to corresponding isotype control stains as well.
Immunocytochemical Evaluation of NPC Cultures
NPC immunostaining was performed according to previously published protocols (26, 29). Primary antibodies used were anti-Nestin (clone 10C2; Merck Millipore, Billerica, MA), anti-TUJ1 (beta III tubulin; clone TUJ1 Covance Inc. San Diego, CA), anti-Vimentin (clone V9; Merck Millipore, Billerica, MA), anti-Oct3/4 (clone H134, Santa Cruz Biotechnology, Inc. Dallas, TX), anti-Nanog (Clone H-155 Santa Cruz Biotechnology Inc. Dallas, TX).
Gene Expression Profiles of NPC and Differentiated Lineage Cells
Total mRNA was isolated from each cell type using the RNeasy kit (Qiagen, Germantown, MD). cDNA was synthesized using a QuantiTect Reverse Transcription Kit (Qiagen, Germantown, MD) according to the manufacture's protocol. Primers for RT-PCR were designed using Primer-BLAST (NCBI) (Table 1). RT-PCR reaction was performed using iQ SYBR Green Supermix (Bio Rad Hercules, CA). Amplification was performed by the MX3000P (Agilent Technologies, Inc. Santa Clara, CA). Data including amplification plots and dissociation curves was then analyzed using the Mx3000p version 2.0 software. Fold change was calculated using the ddCt method, Ct values were normalized to canine fibroblasts derived from the same donor dog as the iPSC, using the housekeeping gene GAPDH.
Preparation of NPC for Injection
NPC were collected from cell culture dishes by gravitational settling. Spheroids were then dissociated into single cell suspension using Accumax cell detachment solution (Stemcell Technologies Vancouver, BC). Cells were washed twice using sterile PBS, and resuspend at a concentration of 2 × 107 /mL in sterile PBS.
Enrollment and NPC Administration to Dogs With Spinal Cord Injury
This study was conducted in accordance with appropriate IACUC and Clinical Review Board protocols, permissions, or exemptions (CRB protocol number VCS #2015-016).
Three pet dogs with naturally-occurring SCI were evaluated at the Colorado State University Veterinary Teaching Hospital for management of chronic (>4 weeks duration) severe thoracolumbar spinal cord injury. These animals met study eligibility requirements and were enrolled in the stem cell trial after owners signed informed consent. Enrollment criteria included the following: focal or multifocal spinal cord injury caudal to T3 (based on neurological exam and MRI), absence of perceptible nociception, and lack of voluntary ambulation using the pelvic limbs for at least 1-month post injury. One dog was excluded from the study due to Unable to undergo anesthesia for the subsequent MRI and electrodiagnostic testing due to grave comorbidities and was euthanized for unrelated reasons.
Stem cell injections were done in the T3-S1 spinal cord segments, with the exact locations determined by MRI images of the spinal cord lesions. Fluoroscopy was used to guide a 22-gauge spinal needle through the interarcuate ligament to the ventral spinal canal. The NPC were injected into 3 sites in each dog: the center of the spinal lesion, the spinal cord one vertebral space cranial to the lesion, and into the spinal cord one vertebral space caudal to the primary lesion site. Each injection site received 2 × 106 NPC., in a total injection volume per site of 100 ul. Each dog was maintained on daily cyclosporine therapy at a 5 mg/kg BID for 24 weeks to help prevent rejection of engrafted NPC.
MRI Imaging
Two dogs enrolled in the study had total of three T3-S3 MRI studies performed. A pre-treatment MRI was performed immediately prior to NPC injection. The initial MRI study included the following sequences: T1- and T2-weighted sagittal images, T1- and T2-weighted transverse images, and DTI images.
Electrodiagnostic Studies
Evoked spinal cord and somato-sensensory potential studies were performed in each dog, under general anesthesia (30). For the evoked spinal cord potentials, anodal stimulating electrodes were placed at the level of the greater trochanter of the femur and just proximal to the tarsus. Corresponding cathodal electrodes were placed 5–7 mm proximal to the anodes. Electrical pulses were of 0.05 ms in duration and delivered at a rate of 4 pulses/second. Evoked potentials were recorded at each interarcuate space between the 7th lumbar vertebra and two spaces cranial to the site of the lesion. An active recording electrode with a 2 mm bared tip was placed percutaneously into each interarcuate ligament. A reference electrode with a 3 mm bared tip was placed in the epaxial muscles 1–2 cm lateral to the active electrode. A ground electrode was placed subcutaneously within 5 cm of the recording site. The stimulations were repeated in exactly the same fashion from both pelvic limbs. Simultaneous with the other recordings, somatosensory evoked potentials were recorded percutaneously from the skull overlying the somatosensory cortex contralateral to the stimulated nerve. The active recording electrode was placed against the bone overlying the somatosensory cortex with the reference electrode placed subcutaneously at the intercanthal space.
Results
NPC Induction From Canine iPSC
Cellular aggregation was first observed 24 h after dissociation of iPSC and placement in neurosphere medium, After 10 days, dissociated iPSC cells formed spheroids consisting of >50 cells (Figure 1D). These NPCs expressed the neural stem cell marker nestin (Figure 1A) (31), the intermediate filament protein vimentin (Figure 1B), and the neuron-specific class III β-tubulin (TUJ1) protein (Figure 1C), as revealed by immunocytology. There was low expression of pluripotency marker Oct3/4 (POU5F1) (Figure 1E) and no expression of stem cell factor Nanog (Figure 1F). NPC were also negative for astrocyte marker GFAP (Glial fibrillary acidic protein) and post mitotic neuronal marker MAP2a (Microtubule-Associated Protein 2).
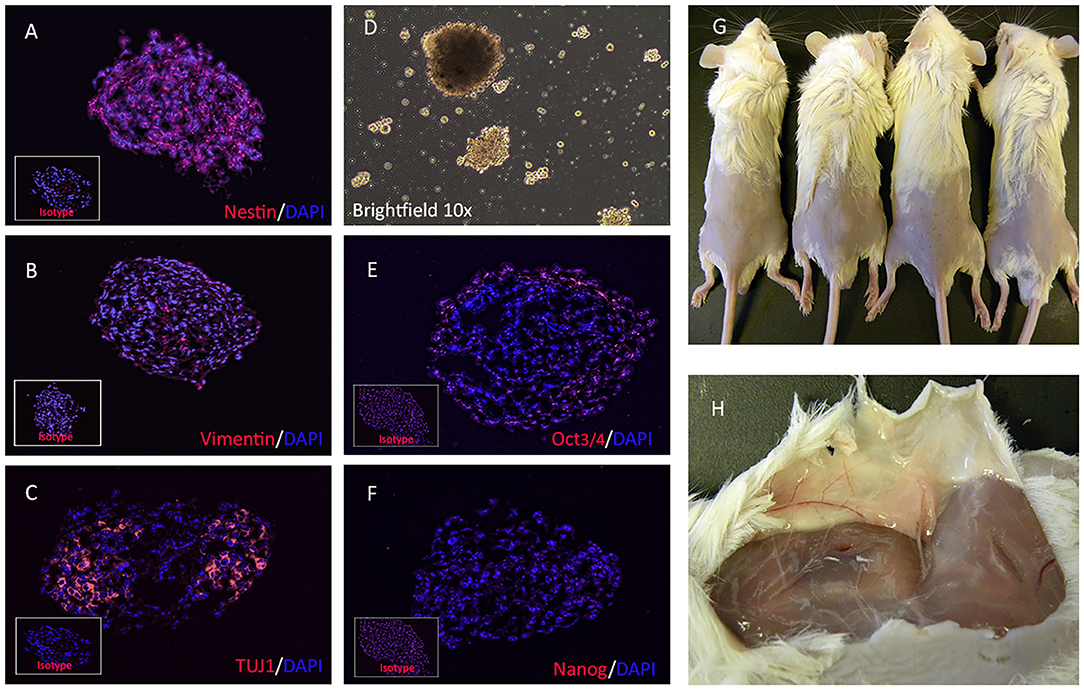
Figure 1. Immunofluorescence characterization and teratogenicity of NPC. NPCs immunostained for detection of intracellular neural stem cell specific antigens, including nestin (A), vimentin (B), and beta-tubulin (C), and pluripotency factors Oct3/4 (E) and Nanog (F). Bright field image showing cluster of neural stem cells formed in vitro (D). Inset boxes depict staining with corresponding isotype antibodies. Dissociated NPCs were embedded in Matrigel and injected s.c in NOD/SCID mice. Teratomas failed to form in any of the 4 mice injected with NPCs (G) and tumor tissues was also not detected after subcutaneous dissection (H).
Evaluation of NPC Cells for Teratoma Formation in Immune Deficient Mice
To address this possibility of teratoma formation from canine NPC cells, NOD/SCID Mice (n = 4 per group) were injected s,c with 2 × 106 NPC embedded in Matrigel. Mice were observed for 90 days for s.c. tumor formation. Tumor formation was not observed at euthanasia 90 days post-injection (Figure 1G), and tumor tissue could not be detected in the dissected subcutaneous tissues in any of the 4 mice (Figure 1H).
In vitro Differentiation of NPC to Neuronal and Glial Subtypes
To verify that NPC cultures contained multipotent progenitor cells, NPC were plated in differentiation media (32) (Figure 2E). The NPC (Figure 2A) differentiated into astrocytes (Figure 2B), mature neurons (Figure 2C), and oligodendrocytes (Figure 2D). The NPC derived neurons expressed MAP2a (Microtubule-Associated Protein 2), a neuron specific protein found in post-mitotic neurons (Figure 3A) (33). In addition, differentiated neurons also expressed TUJ1 (neuron-specific class III β-tubulin), a marker found in immature neurons (Figure 3C), and type III intermediate filament protein Vimentin, which is found in growing axonal neuritis (34) (Figure 3B). NPC cultures could also be induced to differentiate into astrocytes with the addition of 1% FBS, as revealed by expression of the astrocyte marker GFAP (Figure 3D) (35). Astrocyte cultures were negative for neuronal markers MAP2a and TUJ1 (data not shown). Differentiated cells were also evaluated for expression of stem cell, neuronal, astroglial, or oligodendroglial genes, using qRT-PCR. NPC cultures were found to upregulate expression of mRNA for SOX2 (SRY-box 2), stem cell factor receptor kit, and the transcription factor NKX6.1 (NK6 Homeobox 1) (Figure 3E) (27). Neuronally-differentiated cells upregulated expression of the nuclear protein RNA binding protein, and neuronal differentiation antigen 6 (NeuroD6) (36), while astrocytic cultures exhibited upregulated expression of mRNA for nerve growth factor and Ras Association Domain Family Member 10 (RASSF10) (Figure 3G) (37). Oligodendrocyte cultures had upregulated expression of oligodendrocyte lineage transcription factor 2 (OLIG2), oligodendrocyte myelin glycoprotein (OMG), and myelin basic protein (MBP) (Figure 3H) (38).
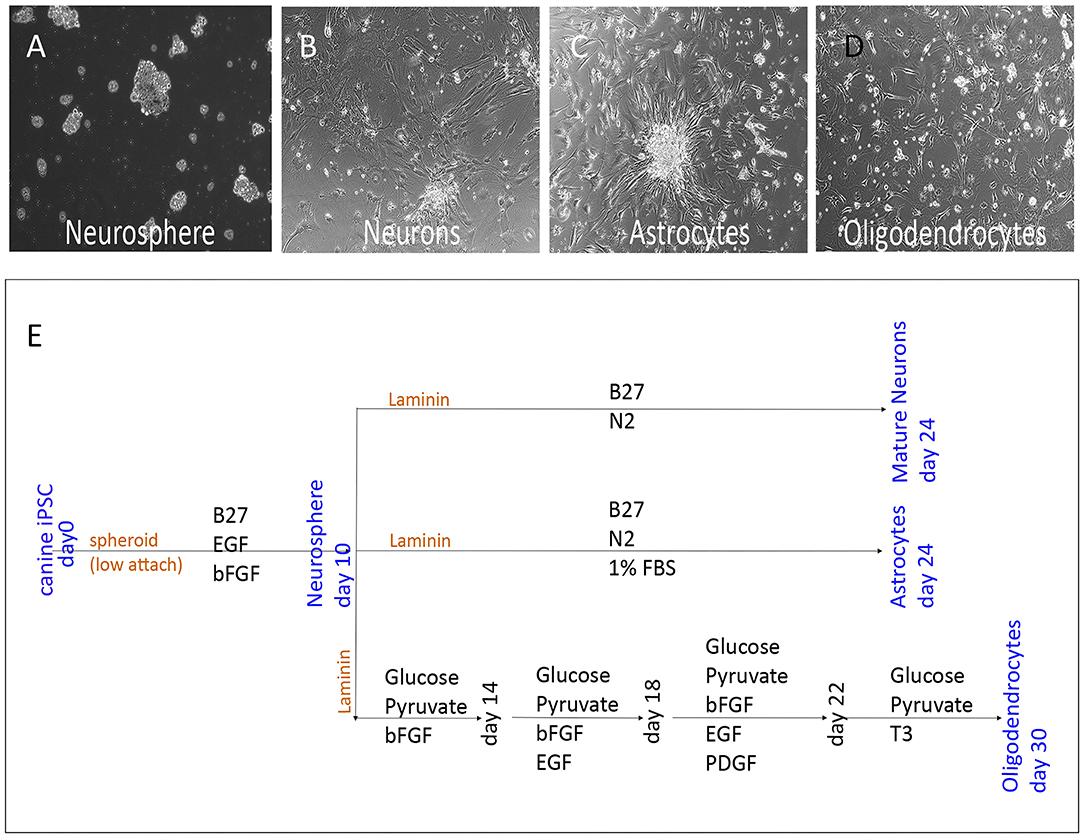
Figure 2. Lineage specific differentiation scheme of NPC. Bright field images (10x) of NPC cultures (A) showing spheroidal clusters in suspension, as well as differentiated neurons (C) with elongated thin projections adhered to culture dish. Astrocytes (B) with star shaped glial morphology, and oligodendrocytes (D) with branching microglia. Schematic of the cell culture differentiation protocol, including growth factors and substrates used as well as differentiation time (E).
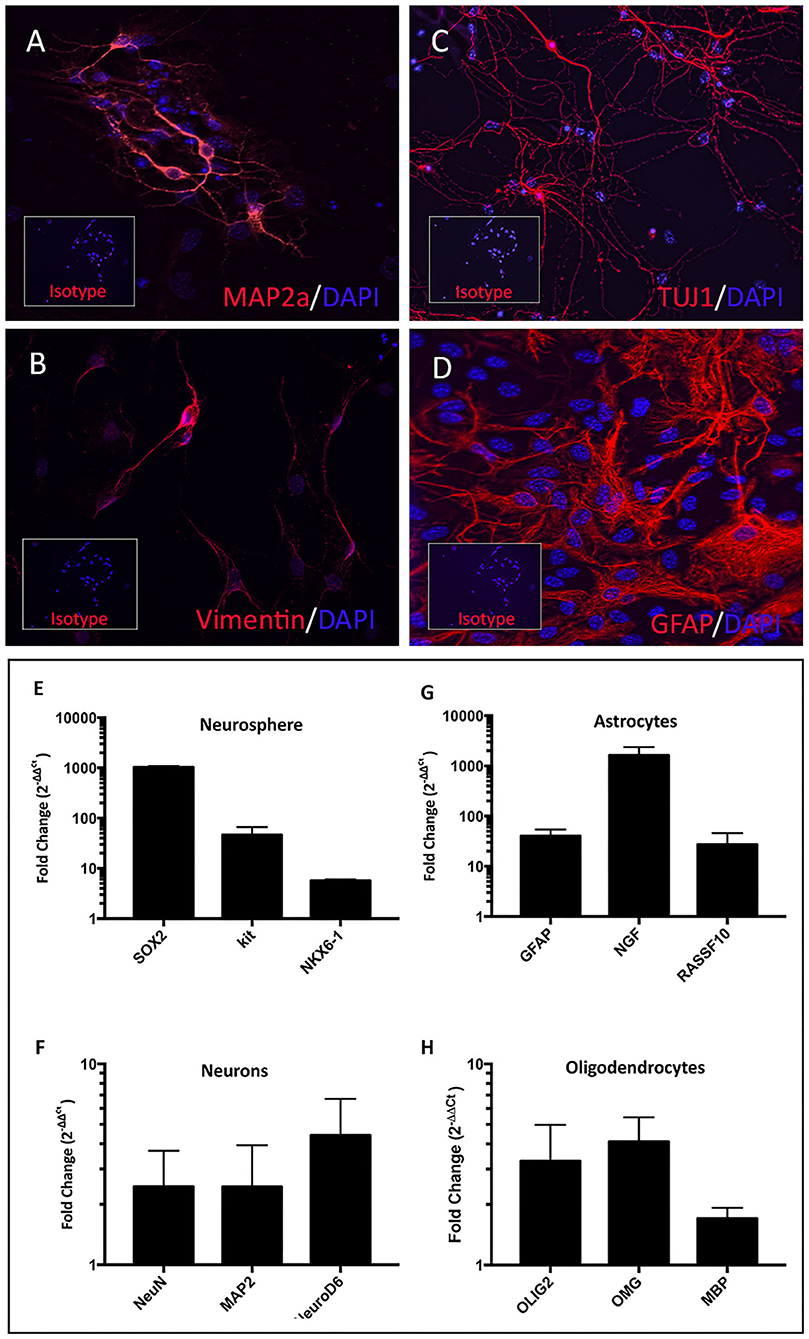
Figure 3. Characterization of terminally differentiated neural cells. Differentiated neurons expressed lineage specific markers MAP2a (A), vimentin (B), and TUJ1 (C). Astrocytes expressed GFAP (D). Inset boxes depict staining with appropriate isotype control antibodies. NPC cultures exhibited upregulated SOX2, Kit, and NXK6.1 compared to skin fibroblasts derived from the same animal (E). Fold changes shown in log scale on y-axis. Astrocyte cultures upregulated mRNA for GFAP, NGF, and RASSF10 (G). Mature neuron cultures upregulated NeuN, MAP2, and NeuroD6 (F), while oligodendrocyte cultures upregulated OLIG2, OMG, and MBP (H).
Evaluation of Spinal Cord Injection Sites Following Intraspinal Injection of NPC
Two dogs were included in the initial pilot study of NPC injection for SCI. NPCs were injected into 3 sites as described in materials and methods (Figures 4E–G). The spinal cord injuries were due to spontaneous, traumatic thoracolumbar disk rupture. Dog 1 had a spinal cord lesion at T11-T12, dog 2 had a spinal cord injury at T13-L1 (Figures 4A,C). Both dogs had undergone heminlaminectomies to decompress spinal cord compression at 2 months prior to study entry. Both dogs were evaluated clinically and by MRI examination following NPC administration at 3 and 6 months, with dog 2 also undergoing a third MRI examination at 12 months after NPC injection. At the time repeat MRI studies were done, neurological and electrophysiological evaluation was also performed. Neither of the animals experienced clinical or electrophysiological improvement following NPC injections; evoked potentials in control regions were consistent with normal reference ranges (30), and there were no evoked potentials when testing the injured regions both pre and up to 6 months post NPC injections. No notable changes were seen in the soft tissues or bony tissues of the spinal cord at either of the 3 injection sites (Figures 4B,D).
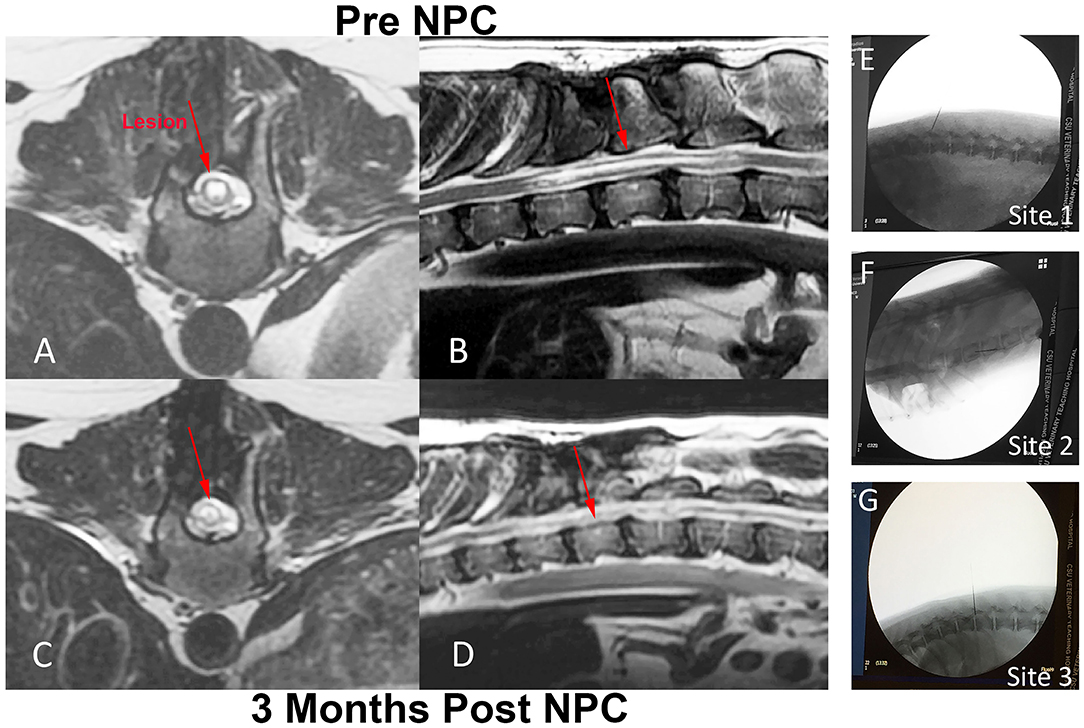
Figure 4. Intra-spinal NPC injection procedure and MRI evaluation of injection sites in a dog with chronic SCI. Fluoroscopic images of injection sites including original injury site (E), as well as an injection location cranial (F) and caudal (G) to the original injury site. T2-weighted MR images were obtained from dog 2, immediately prior to NPC injection (A,B) and 3 months after the intra-spinal injection procedure (C,D). Prior to NPC injection, there was a T2 hyper-intense lesion extending from T11 to T13 present within the spinal cord on both the transverse and sagittal images (red arrows A,B). Following the NPC injections, the lesion at 3 months did not change in size or extent compared to the pre-injection images, nor was there evidence of tumor growth at the injection site (red arrows C,D).
Following the NPC injections, the lesion at 3 months did not change in size or extent compared to the pre-injection images (red arrows Figures 4C,D). Glial scaring remains unchanged in intensity and extent before and after injections. In addition, there was no evidence of tumor formation at any injection site from the MRI evaluations.
In all treated dogs, no adverse effects that could be attributed to NPC injection were observed at any time point after NPC injection during the 6–12 month follow-up period. Clinical neurological evaluation including assessment of spontaneous ambulation and responses to cutaneous stimuli did not reveal any notable improvement in neurological function at any of the time points.
Discussion
The implementation of iPSC-derived neural stem cells in human SCI trials requires first rigorous proof of their safety and efficacy using appropriate animal models. Dogs with naturally-occurring SCI offer a particularly compelling model of chronic SCI to model neural stem cell therapies for humans (16, 17, 39). This study is the first to our knowledge to show the differentiation of canine iPSC to NPC. The results of these characterizations were consistent with results in other species (15, 40), and also canine stem cell studies using embryonic or adipose stem cells (32, 41). We also demonstrate the safety of administering iPSC-derived NPC in dogs with follow-up for 6–12 months, particularly with respect to tumor formation at the injection site.
The other endpoint of the study was to evaluate the impact of NPC administration on recovery of neurological function in dogs with chronic SCI. Though this was a small pilot study involving only 2 animals, these early results suggest that direct intraspinal injection of cells alone is unlikely to provide significant functional improvement. There are several potential explanations for the failure to observe clinical benefit. For one, direct cell injection without surgical debridement of the spinal injury site does not remove debris and scar tissue from the original injury, which likely significantly impairs any regeneration from the injected NPC. Indeed, others have reported in non-human primate models that surgical access to the injection site is essential to provide a receptive bed for stem cell administration (42). It is also reported that stem cells must be administered in a matrix such as collagen or fibrin matrix (43) to retain the cells in the lesion site and to promote survival and axonal connection. In addition, many experimental stem cell treatments for SCI have been evaluated in animals within weeks after a spinal cord lesion was administered, at a time when significant gliosis and scarring had not occurred (8). In contrast, all 3 animals in the present study had SCI of at least 60 days duration, at time frame where spontaneous recovery would be considered a remote possibility. These important differences between acute and chronic SCI also play an important role in regulating the different responses to stem cell injections and survival (44).
Considering our findings from this pilot study, it will be interesting in the future to continue to evaluate stem cell therapies in the dog chronic SCI model, which provides a much more realistic animal model for evaluation of new cellular therapies for SCI treatment in humans than current rodent models. The availability of canine iPSC, and the technologies to generate large numbers of NPC should also facilitate these new studies.
Conclusion
In this study, we have shown for that canine iPSC are able to differentiate into neuronal and glial lineage cells following the induction of NPC. Differentiated cells were verified by ICC and PCR. A total of two dogs were treated with iPS derived NPC for chronic spinal cord injury up to 4 months post injury. We concluded that to increase the chance of improvement in a chronic injury such as these, additional steps must be taken prior to the intra-spinal injection of cells. These steps may include surgical debridement of the original injury site, embedding of the NPC in a matrix, and also possibly an earlier administration of NPC within weeks of injury.
Data Availability Statement
The raw data supporting the conclusions of this article will be made available by the authors, without undue reservation upon reasonable request.
Ethics Statement
All procedures involving live animals were approved by the Institutional Animal Care and Use Committee at Colorado State University. Written informed consent was obtained from the owners for the participation of their animals in this study.
Author Contributions
LC and SD wrote manuscript with support from SM. SM and RP performed neurological surgery. LW assisted with Fluoroscopy and MRI imaging. CA performed in vitro experiments. LC performed in vitro experiments and data analysis. All authors contributed to the article and approved the submitted version.
Funding
This work was supported by funding from the Shipley Foundation.
Conflict of Interest
The authors declare that the research was conducted in the absence of any commercial or financial relationships that could be construed as a potential conflict of interest.
Acknowledgments
The authors wish to acknowledge support provided by other members of the Dow lab, as well as Kaitlyn McNamara, MS, DVM for repetition of the differentiation assays for validation of techniques used.
References
1. Fan X, Wang J-Z, Lin X-M, Zhang L. Stem cell transplantation for spinal cord injury: a meta-analysis of treatment effectiveness and safety. Neur Regener Res. (2017) 12:815–25. doi: 10.4103/1673-5374.206653
2. Zhu Y, Uezono N, Yasui T, Nakashima K. Neural stem cell therapy aiming at better functional recovery after spinal cord injury. Dev Dyn. (2017) 247:75–84. doi: 10.1002/dvdy.24558
3. Shin JC Kim KN Yoo J Kim IS Yun S Lee H . Clinical trial of human fetal brain-derived neural stem/progenitor cell transplantation in patients with traumatic cervical spinal cord injury. Neural Plast. (2015) 2015:630932. doi: 10.1155/2015/630932
4. Anderson AJ, Piltti KM, Hooshmand MJ, Nishi RA, Cummings BJ. Preclinical efficacy failure of human CNS-Derived stem cells for use in the pathway study of cervical spinal cord injury. Stem Cell Rep. (2017) 8:249–63. doi: 10.1016/j.stemcr.2016.12.018
5. Larocca TF, Macedo CT, Souza BSF, Andrade-Souza YM, Villarreal CF, Matos AC, et al. Image-guided percutaneous intralesional administration of mesenchymal stromal cells in subjects with chronic complete spinal cord injury: a pilot study. Cytotherapy. (2017) 19:1189–96. doi: 10.1016/j.jcyt.2017.06.006
6. Manley NC, Priest CA, Denham J, Wirth ED, Lebkowski JS. Human embryonic stem cell-derived oligodendrocyte progenitor cells: preclinical efficacy and safety in cervical spinal cord injury. Stem Cells Transl Med. (2017) 6:1917–29. doi: 10.1002/sctm.17-0065
7. Zhao Y, Tang F, Xiao Z, Han G, Wang N, Yin N, et al. Clinical study of neuroregen scaffold combined with human mesenchymal stem cells for the repair of chronic complete spinal cord injury. Cell Transplant. (2017) 26:891–900. doi: 10.3727/096368917X695038
8. Yousefifard M, Rahimi-Movaghar V, Nasirinezhad F, Baikpour M, Safari S, Saadat S, et al. Neural stem/progenitor cell transplantation for spinal cord injury treatment; a systematic review and meta-analysis. Neuroscience. (2016) 322:377–97. doi: 10.1016/j.neuroscience.2016.02.034
9. Goulão M, Lepore AC. iPS cell transplantation for traumatic spinal cord injury. Curr Stem Cell Res Ther. (2016) 11:321–8. doi: 10.2174/1574888X10666150723150059
10. Kawabata S, Takano M, Numasawa-Kuroiwa Y, Itakura G, Kobayashi Y, Nishiyama Y, et al. Grafted human iPS cell-derived oligodendrocyte precursor cells contribute to robust remyelination of demyelinated axons after spinal cord injury. Stem Cell Rep. (2017) 6:1–8. doi: 10.1016/j.stemcr.2015.11.013
11. Niclis JC, Turner C, Durnall J, McDougal S, Kauhausen JA, Leaw B, et al. Long-distance axonal growth and protracted functional maturation of neurons derived from human induced pluripotent stem cells after intracerebral transplantation. Stem Cells Transl Med. (2017) 6:1547–56. doi: 10.1002/sctm.16-0198
12. Forbes LH, Andrews MR. Grafted human iPSC-derived neural progenitor cells express integrins and extend long-distance axons within the developing corticospinal tract. Front Cell Neurosci. (2019) 13:26. doi: 10.3389/fncel.2019.00026
13. Nagoshi N, Tsuji O, Nakamura M, Okano H. Cell therapy for spinal cord injury using induced pluripotent stem cells. Regener Ther. (2019) 11:75–80. doi: 10.1016/j.reth.2019.05.006
14. Kimbrel EA, Lanza R. Hope for regenerative treatments: toward safe transplantation of human pluripotent stem-cell-based therapies. Regen Med. (2015) 10:99–102. doi: 10.2217/rme.14.89
15. Rosati J, Ferrari D, Altieri F, Tardivo S, Ricciolini C, Fusilli C, et al. Establishment of stable iPS-derived human neural stem cell lines suitable for cell therapies. Cell Death Dis. (2018) 9:937. doi: 10.1038/s41419-018-0990-2
16. Hoffman AM, Dow SW. Concise review: stem cell trials using companion animal disease models. Stem Cells. (2016) 34:1709–29. doi: 10.1002/stem.2377
17. Gabel BC, Curtis EI, Marsala M, Ciacci JD. A review of stem cell therapy for spinal cord injury: large animal models and the frontier in humans. World Neurosurg. (2017) 98(Suppl. C):438–43. doi: 10.1016/j.wneu.2016.11.053
18. Lim JH, Byeon YE, Ryu HH, Jeong YH, Lee YW, Kim WH, et al. Transplantation of canine umbilical cord blood-derived mesenchymal stem cells in experimentally induced spinal cord injured dogs. J Vet Sci. (2007) 8:275–82. doi: 10.4142/jvs.2007.8.3.275
19. Jung DI, Ha J, Kang BT, Kim JW, Quan FS, Lee JH, et al. A comparison of autologous and allogenic bone marrow-derived mesenchymal stem cell transplantation in canine spinal cord injury. J Neurol Sci. (2009) 285:67–77. doi: 10.1016/j.jns.2009.05.027
20. Ryu HH, Lim JH, Byeon YE, Park JR, Seo MS, Lee YW, et al. Functional recovery and neural differentiation after transplantation of allogenic adipose-derived stem cells in a canine model of acute spinal cord injury. J Vet Sci. (2009) 10:273–84. doi: 10.4142/jvs.2009.10.4.273
21. McMahill BG, Spriet M, Sisó S, Manzer MD, Mitchell G, McGee J, et al. Feasibility study of canine epidermal neural crest stem cell transplantation in the spinal cords of dogs. Stem Cells Transl Med. (2015) 4:1173–86. doi: 10.5966/sctm.2015-0018
22. Park SS, Lee YJ, Lee SH, Lee D, Choi K, Kim WH, et al. Functional recovery after spinal cord injury in dogs treated with a combination of Matrigel and neural-induced adipose-derived mesenchymal Stem cells. Cytotherapy. (2012) 14:584–97. doi: 10.3109/14653249.2012.658913
23. Ryu HH, Kang BJ, Park SS, Kim Y, Sung GJ, Woo HM, et al. Comparison of mesenchymal stem cells derived from fat, bone marrow, Wharton's jelly, and umbilical cord blood for treating spinal cord injuries in dogs. J Vet Med Sci. (2013) 72:1617–30. doi: 10.1292/jvms.12-0065
24. Sarmento CAP, Rodrigues MN, Bocabello RZ, Mess AM, Miglino MA. Pilot study: bone marrow stem cells as a treatment for dogs with chronic spinal cord injury. Regener Med Res. (2014) 2:9. doi: 10.1186/2050-490X-2-9
25. Escalhão CCM, Ramos IP, Hochman-Mendez C, Brunswick THK, Souza SAL, Gutfilen B, et al. Safety of allogeneic canine adipose tissue-derived mesenchymal stem cell intraspinal transplantation in dogs with chronic spinal cord injury. Stem Cells Int. (2017) 2017:3053759. doi: 10.1155/2017/3053759
26. Chow L, Johnson V, Regan D, Wheat W, Webb S, Koch P, et al. Safety and immune regulatory properties of canine induced pluripotent stem cell-derived mesenchymal stem cells. Stem Cell Res. (2017) 25(Suppl. C):221–32. doi: 10.1016/j.scr.2017.11.010
27. Ebert AD, Shelley BC, Hurley AM, Onorati M, Castiglioni V, Patitucci TN, et al. EZ spheres: a stable and expandable culture system for the generation of pre-rosette multipotent stem cells from human ESCs and iPSCs. Stem Cell Res. (2013) 10:417–27. doi: 10.1016/j.scr.2013.01.009
28. Chow L, Johnson V, Coy JW, Regan DP, Dow SW. Mechanisms of immune suppression utilized by canine adipose and bone marrow-derived mesenchymal stem cells. Stem Cells Dev. (2016) 26:374–89. doi: 10.1089/scd.2016.0207
29. Gomes IC, Acquarone M, Maciel Rde M, Erlich RB, Rehen SK. Analysis of pluripotent stem cells by using cryosections of embryoid bodies. J Vis Exp. (2010) 46:2344. doi: 10.3791/2344
30. Cuddon PA. Electrophysiology in neuromuscular disease. Vet Clin North Am Small Anim Pract. (2002) 32:31–62. doi: 10.1016/S0195-5616(03)00079-2
31. Kim EY, Lee K-B, Yu J, Lee JH, Kim KJ, Han K-W, et al. Neuronal cell differentiation of mesenchymal stem cells originating from canine amniotic fluid. Hum Cell. (2014) 27:51–8. doi: 10.1007/s13577-013-0080-9
32. Wilcox JT, Lai JKY, Semple E, Brisson BA, Gartley C, Armstrong JN, et al. Synaptically-competent neurons derived from canine embryonic stem cells by lineage selection with EGF and Noggin. PLoS ONE. (2011) 6:e19768. doi: 10.1371/journal.pone.0019768
33. Soltani MH, Pichardo R, Song Z, Sangha N, Camacho F, Satyamoorthy K, et al. Microtubule-associated protein 2, a marker of neuronal differentiation, induces mitotic defects, inhibits growth of melanoma cells, and predicts metastatic potential of cutaneous melanoma. Am J Pathol. (2005) 166:1841–50. doi: 10.1016/S0002-9440(10)62493-5
34. Yabe JT, Chan WK, Wang FS, Pimenta A, Ortiz DD, Shea TB. Regulation of the transition from vimentin to neurofilaments during neuronal differentiation. Cell Motil Cytoskeleton. (2003) 56:193–205. doi: 10.1002/cm.10137
35. Hol EM, Pekny M. Glial fibrillary acidic protein (GFAP) and the astrocyte intermediate filament system in diseases of the central nervous system. Curr Opin Cell Biol. (2015) 32:121–30. doi: 10.1016/j.ceb.2015.02.004
36. Gascon S, Masserdotti G, Russo GL, Gotz M. Direct neuronal reprogramming: achievements, hurdles, and new roads to success. Cell Stem Cell. (2017) 21:18–34. doi: 10.1016/j.stem.2017.06.011
37. Chandrasekaran A, Avci HX, Leist M, Kobolák J, Dinnyés A. Astrocyte differentiation of human pluripotent stem cells: new tools for neurological disorder research. Front Cell Neurosci. (2016) 10:215. doi: 10.3389/fncel.2016.00215
38. Piao J, Major T, Auyeung G, Policarpio E, Menon J, Droms L, et al. Human embryonic stem cell-derived oligodendrocyte progenitors remyelinate the brain and rescue behavioral deficits following radiation. Cell Stem Cell. (2015) 16:198–210. doi: 10.1016/j.stem.2015.01.004
39. McMahill BG, Borjesson DL, Sieber-Blum M, Nolta JA, Sturges BK. Stem cells in canine spinal cord injury–promise for regenerative therapy in a large animal model of human disease. Stem Cell Rev. (2015) 11:180–93. doi: 10.1007/s12015-014-9553-9
40. Liu Y, Zheng Y, Li S, Xue H, Schmitt K, Hergenroeder GW, et al. Human neural progenitors derived from integration-free iPSCs for SCI therapy. Stem Cell Res. (2017) 19:55–64. doi: 10.1016/j.scr.2017.01.004
41. Blecker D, Elashry MI, Heimann M, Wenisch S, Arnhold S. New insights into the neural differentiation potential of canine adipose tissue-derived mesenchymal stem cells. Anat Histol Embryol. (2017) 46:304–15. doi: 10.1111/ahe.12270
42. Iwai H, Shimada H, Nishimura S, Kobayashi Y, Itakura G, Hori K, et al. Allogeneic neural stem/progenitor cells derived from embryonic stem cells promote functional recovery after transplantation into injured spinal cord of nonhuman primates. Stem Cells Transl Med. (2015) 4:708–19. doi: 10.5966/sctm.2014-0215
43. Lu P, Woodruff G, Wang Y, Graham L, Hunt M, Wu D, et al. Long-distance axonal growth from human induced pluripotent stem cells after spinal cord injury. Neuron. (2014) 83:789–96. doi: 10.1016/j.neuron.2014.07.014
Keywords: neural progenitor, induced pluripotent stem cell, spinal cord injury, spinal cord injection, teratoma
Citation: Chow L, McGrath S, de Arruda Saldanha C, Whalen LR, Packer R and Dow S (2020) Generation of Neural Progenitor Cells From Canine Induced Pluripotent Stem Cells and Preliminary Safety Test in Dogs With Spontaneous Spinal Cord Injuries. Front. Vet. Sci. 7:575938. doi: 10.3389/fvets.2020.575938
Received: 24 June 2020; Accepted: 19 August 2020;
Published: 05 November 2020.
Edited by:
Alonso Guedes, University of Minnesota Twin Cities, United StatesReviewed by:
Laura Calza, University of Bologna, ItalyAlberto Granato, Catholic University of the Sacred Heart, Italy
Copyright © 2020 Chow, McGrath, de Arruda Saldanha, Whalen, Packer and Dow. This is an open-access article distributed under the terms of the Creative Commons Attribution License (CC BY). The use, distribution or reproduction in other forums is permitted, provided the original author(s) and the copyright owner(s) are credited and that the original publication in this journal is cited, in accordance with accepted academic practice. No use, distribution or reproduction is permitted which does not comply with these terms.
*Correspondence: Lyndah Chow, bHluZGFoLmNob3dAY29sb3N0YXRlLmVkdQ==