- 1Max Planck Institute for Evolutionary Anthropology, Leipzig, Germany
- 2Endocrinology Laboratory, German Primate Center, Leibniz Institute for Primate Research, Göttingen, Germany
- 3Epidemiology of Highly Pathogenic Microorganisms, Robert Koch Institute, Berlin, Germany
- 4Taï Chimpanzee Project, Centre Suisse de Recherches Scientifiques en Côte d'Ivoire, Abidjan, Côte d'Ivoire
Abstract: In mammals, the excretion of cortisol can provide energy toward restoring homeostasis and is a major component of the stress response. However, chronically elevated cortisol levels also have suppressive effects on immune function. As mounting an immune response is energetically costly, sick individuals may conserve energy by exhibiting certain sickness behaviors, such as declining activity levels. Due to the complex interplay between immune function and sickness behaviors, endocrinological correlates have received growing attention in the medical community, but so far, this subject was investigated rarely. Furthermore, given the complexities of studying illnesses and immunity in natural settings, correlates of sickness behaviors have yet to be studied in non-human primates in the wild.
Methods: We measured urinary cortisol levels using liquid chromatography–mass spectrometry in a group of wild habituated chimpanzees in Taï National Park, Côte d'Ivoire, before, during, and after a respiratory disease outbreak (main causative pathogen: human respiratory syncytial virus A, with coinfections of Streptococcus pneumoniae). Changes in cortisol levels were then related to urinary neopterin levels, a biomarker of immune system activation.
Results: Urinary cortisol levels were found to be more than 10-fold higher during the outbreak in comparison with levels before and after the outbreak period. Increasing cortisol levels were also associated with increasing neopterin levels. Interestingly, rather atypical patterns in a diurnal decline of cortisol levels were found during infection periods, such that levels remained raised throughout the day.
Conclusion: In conclusion, cortisol increase was related to cellular immune response. Our results suggest that cortisol is a mediator of infectious disease pathogenicity through its impact on the immune system and that wild chimpanzees may be facing energetic stress when sick. By monitoring immune challenges in wild-living animals, our study demonstrates that immune defenses have costs and that these costs are context-specific.
Introduction
To optimize individual fitness, organisms adopt strategies to improve their ability to survive and reproduce in a fluctuating environment. Due to the necessity of differentially allocating limited resources (e.g., energy), this results in trade-offs between maintenance, growth, and reproduction—leading to species-specific life-history patterns (1).
The activation of the hypothalamic–pituitary–adrenal (HPA) axis in response to stressor results in the systemic elevation of glucocorticoids, which, in mammals, is primarily cortisol (2, 3). An increase of cortisol levels in the bloodstream leads to the rapid mobilization of glucose and contributes toward the restoration of homeostasis by enhancing gluconeogenesis. Therefore, an increase in cortisol provides energy in the face of environmental challenges perceived as stressors (4, 5).
The HPA axis also plays a part in regulating the immune system (2, 3, 6), with stressors associated with immune challenges called immune- or inflammatory stressors. Regulating immune function is essential for the survival of an organism during stressful periods, as well as to modulate immune responses to inflammatory diseases (2). During the acute phase response, immune cells are stimulated through, for example, endotoxins stemming from pathogens, such as bacteria. These immune cells release cytokines that stimulate the HPA axis to release cortisol (7). The increase in blood cortisol levels, in turn, modulates the inflammatory response to pathogens (8). Overall, glucocorticoids have suppressive effects on the maturation, differentiation, and proliferation of immune cells, including innate, T cell, and B cell function (3). Innate immune cells are white blood cells mediating innate immunity, for example, basophils, neutrophils, mast cells, and macrophages. Glucocorticoid receptors are found throughout the immune system and in circulating immune cells, such as macrophages (2, 3, 9). The binding of glucocorticoids to these receptors leads to changes in gene expression and the dysregulation of immune functioning, with severe cortisol-associated immune dysregulation, possibly incurring significant health complications (6).
Immune responses are regulated by antigen-presenting cells such as macrophages/monocytes or dendritic cells, which are components of the innate immunity. Helper T cells are regarded as being the most prolific cytokine producer. They can be subdivided into type 1 helper T cells (Th1) and type 2 helper T cells (Th2). Interferon-gamma is the main Th1 cytokine and produces the pro-inflammatory responses responsible for killing intracellular parasites and for perpetuating autoimmune responses. The Th2 type cytokines include, for example, interleukins 4, 5, 13, and 10, which have more of an anti-inflammatory response (10). As cortisol is involved in inhibiting the interferon-gamma response, an increase in plasma cortisol could induce a decrease in Th1 products, which are produced by type 1 T cells to stimulate macrophages (11). Because activated macrophages/monocytes produce neopterin (12), the measurement of neopterin can be used to monitor cell-mediated immune responses caused, for example, by viral and bacterial infections.
Animals with extended stress responses (chronic stressors) have diminished responses to vaccination and slower wound healing, as well as exacerbated viral and bacterial pathogenesis and altered autoimmune diseases (6). Furthermore, elevated stress levels alter rates of contacts among hosts, pathogens, and vectors on host metabolism and activity levels. For example, an in vivo study of viral infection in birds found that, although all individuals became infected after exposure to the virus, only birds with elevated corticosterone levels had viral loads at, or above, the infectious threshold. Moreover, in birds with increased corticosterone levels, the mortality rate was higher than that in controls (13). It was, therefore, hypothesized that elevated glucocorticoid levels put individuals at greater risk from severe illness by affecting their immune system response (6).
The field of ecological immunology (ecoimmunology) aims to understand factors leading to changes in immune system function and, moreover, how these changes affect disease susceptibility in the field and captive settings. A central assumption in ecoimmunology is that mounting an immune response has direct and indirect costs (14). Direct costs include increased metabolic rate and amino acid assimilation, as well as the production of immune proteins involved in the acute phase response. Additional costs of a disease can also occur during infection, such as the development of fever—a key and extremely energy-intensive process and feature of the sickness response (15). The amount of energy required to increase body temperature during the febrile process is considerably high; for example, in humans, to raise the body temperature by 1°C, the metabolic rate needs to be increased by 10–15% (15, 16). Indirect costs of immune system activation include trade-offs with other life-history traits, for example, reduction in growth and reproductive success (14, 17–19). Therefore, to counter against costs incurred during illnesses or periods of short-term energy deficits, an organism may try to conserve energy by downregulating its metabolic rate (5). Sick individuals may also attempt to mitigate costs by increasing their energy resource intake (20) or by adopting sickness behaviors to limit their energy expenditure (21). Behavioral modifications to sickness can be characterized by an overall reduction in physical activity, such as decreased levels of locomotion, sexual behavior, exploration, aggression, food and water intake, and social interest, as well as an increase in sleep duration and shivering to increase heat production (21, 22). Hart (23) proposed that adopting behavioral symptoms of sickness, in combination with the fever response, represents a highly organized strategy to fight infection.
In captive animals, continuous food availability may allow individuals to increase their energy intake, which can cover the costs of immune system activation when sick. It can, therefore, be assumed that energy homeostasis within captive individuals would not be dramatically affected by illness. In contrast, available energy is typically limited in natural environments and, thus, must be allocated among competing physiological processes (14). Moreover, comparing immune system components as an indicator of immune challenges in wild and captive living animals, some blood markers were found to be elevated in wild living birds and dolphins (24, 25). Also, wild chimpanzees experience challenges to their immune system more frequently than captive chimpanzees (26), with severe outbreaks of the respiratory disease reported in the majority of field sites where wild chimpanzees are regularly observed (27–34). Biological similarities and the close genetic relatedness between humans and apes predispose apes to cross-species barrier transmission, and anthroponotic transmission risk is exacerbated due to, for example, tourism (35). Human viruses can cause lethal outbreaks in chimpanzees, even when being nonlethal and mild in humans, indicating a lack of resistance to those viruses in chimpanzees (30, 31, 35). During disease outbreaks, chimpanzees exhibited energy-conserving sickness behaviors, they were found to be less active (29, 35), more lethargic, traveled only short distances, often built day nests, rose late in the morning, and retired early at night (36).
In chimpanzees, physiological changes in, for example, neopterin levels (a marker of cell-mediated immune responses) showed a significant increase concerning sickness behavior, such as extreme fatigue, lethargy, and inappetence (29). However, the extent to which hormones, for example, steroid hormones, may modulate sickness behaviors has only recently been investigated. Furthermore, hormonal correlates of sickness behaviors have yet to be studied in non-human primates within a natural context (e.g., natural infection without treatment, without food provisioning, and exposure to potentially detrimental environmental factors, such as weather and temperature changes) due to the complexities of studying illnesses and immunity outside a controlled clinical setting (37). To study animals under natural conditions is important, given that costs of immunity may be difficult to avoid in a natural environment (14, 18); disease outbreaks in wild living animals can provide an opportunity to test how energy availability and allocation are affected by illness.
Capitalizing on a respiratory disease outbreak in a group of wild habituated chimpanzees in the Taï National Park, Côte d'Ivoire, we investigated, as one of the first studies, how energy availability and allocation are affected by illness by comparing urinary cortisol levels before, during, and after the respiratory disease outbreak. We then tested several predictions related to urinary cortisol changes during the study period as follows. We predicted that cortisol levels increased during the respiratory outbreak to mobilize glucose reserves. Furthermore, we predicted a positive correlation of urinary cortisol and neopterin (a measure of the innate cell-immune response), as mounting an immune response is costly, and sick animals will need to make energy more accessible. Our alternative prediction was that urinary cortisol levels were negatively correlated with urinary neopterin levels, as increasing cortisol levels shift the immune response from the Th1 response to the Th2 response, and neopterin is released from cells that are mainly involved in the Th1 response.
Materials and Methods
Urine samples were collected from a group of wild chimpanzees before (February 1st to November 12th, 2009), during (November 29th to December 19th, 2009), and after (December 22nd, 2009 to November 16th, 2010) a respiratory outbreak in Taï National Park, Côte d'Ivoire. The respiratory outbreak occurred between November and December 2009 in the south community of chimpanzees who are habituated to human presence and regularly followed since 1994 as part of the Taï Chimpanzee Project (38). Before the outbreak, the south community contained 37 individuals with 18 males (6 adults, 3 adolescents, 3 juveniles, and 6 infants) and 19 females (10 adults, 2 adolescents, and 7 infants) (age–sex class defined from Boesch and Boesch-Achermann) (39). The outbreak lasted from November 26th, when some individuals were observed to exhibit the first signs of respiratory illness (including coughing, sneezing, nasal discharge, and dyspnea) (40), until December 20th, when all surviving individuals ceased to show any signs. During the outbreak, 86% of chimpanzees showed respiratory signs of illness. Diagnostics performed on lung samples from those who died during the outbreak led to the identification of human respiratory syncytial virus A as the main causative pathogen, with coinfections of Streptococcus pneumoniae found in some individuals (41). Individuals with particularly severe disease symptoms were treated with a long-acting antibiotic shot (Extencilline, Sanofi-Aventis, France) through remote injection, with 9 of the 12 treated individuals surviving (41). Additional detailed information on the progression of the disease and the pathogen has been published previously (33, 41). Transmission of the human respiratory syncytial virus from humans to apes has often been presumed, but only a few cases have been proven and reported (35, 42, 43). Because there have been several fatal outbreaks of respiratory disease linked to human respiratory viruses in the Taï chimpanzees, extensive health and hygiene measures, quarantine procedures, and behavioral rules, such as wearing face masks, have been applied (44). Moreover, a long-term health monitoring program, as well as a permanent veterinarian on-side, was implemented since the year 2000 (45).
Sample Analysis
This study included a total of 186 urine samples: 83 samples from 27 individuals (average = 2.6 samples/individual) before the respiratory outbreak, 56 samples from 19 individuals (average = 3.1 samples/individual) during the outbreak, and 47 samples from 20 individuals (average = 2.4 samples/individual) after the outbreak. In eight individuals, urine samples were only collected while symptomatic (e.g., coughing and nasal discharge), whereas five chimpanzees died during the outbreak before a urine sample could be collected, and three chimpanzees remained asymptomatic throughout the outbreak. Urine samples were collected on plastic sheets or leaves and then transferred with a disposable plastic pipette into vials. After collection, urine samples were frozen in liquid nitrogen upon arrival in the camp and finally transported frozen to the Max Planck Institute for Evolutionary Anthropology (MPI-EVA) in Leipzig, Germany. At the MPI-EVA in Leipzig, samples were stored at −80°C before being analyzed.
Urinary cortisol was measured with liquid chromatography–mass spectrometry. For each sample, 10 μl urine was extracted following Hauser (46) with modifications (47). In summary, urine sample extraction included hydrolysis, followed by solid-phase extraction. Afterward, two liquid–liquid extractions were performed, followed by solvolysis and an additional liquid–liquid extraction.
In all samples, urinary neopterin had been previously measured for a study on immune system activation during the same respiratory outbreak (33). To correct for variations in urine dilution, both urinary cortisol and neopterin measures were corrected for specific gravity (SG) using the formula as presented in Miller et al. (48). The SG population average for the wild chimpanzees was 1.017. Urinary cortisol results are expressed in urinary cortisol (nanogram per milliliter) corrected for SG (corr. SG).
Ethical approval was not required for this study because urine samples were collected non-invasively without animal disturbance or harming the chimpanzees.
Statistical Analyses
To explore factors explaining variation in urinary cortisol levels (nanogram per milliliter corr. SG) in healthy and sick chimpanzees, we ran a linear mixed model (LMM) (49) with a Gaussian error structure and identity link function. The model was fitted in R v.3.4.3 (50) using the R-package lme4, function “lmer” (51). The full model included log-transformed urinary cortisol levels (nanogram per milliliter corr. SG) as the response variable. To test whether urinary neopterin levels (log-transformed) were positively associated with cortisol levels during each of the three periods (before, during, and after the outbreak), we included a sample period (before, during, and after) as interaction with urinary neopterin levels (log-transformed). We further included, as predictor variables, sex and age at the time of sampling (z-transformed) to a mean of zero and a standard deviation of one to achieve comparable estimates (52). To control for externally caused changes in pathogen load, we added the following control variables: sample collection time (z-transformed), survival of the animal (yes or no), and whether the individual had been treated with antibiotics (yes or no). To limit type I error rates to a nominal level of 5% (53, 54), we included individual and sample collection dates as random effects, with random slopes for sample collection date and urinary neopterin levels, respectively. To investigate the significance of each fixed effect, we compared the full model with a null model that excluded the predictor variables while retaining control predictors, as well as the random effects and the random slopes, using a likelihood ratio test (R-function “ANOVA”) (55). A post hoc comparison was then performed for each period (before, during, and after) using the function “glht” from the R-package “multcomp” (56, 57).
We assessed the LMM for the required normal distribution and homogeneity of residuals via visual inspection of the q-q plot of the residuals and by plotting residuals against fitted values. The tests revealed no deviation from the assumption. Collinearity was evaluated by determining variance inflation factors (VIF) (58) using the function “vif” from the R-package “car” (59), which revealed that collinearity was not an issue (maximum VIF: 1.2). Model stability was examined by excluding levels of the random effects one at a time from the model and comparing the resulting model estimates for these data with those of the full data set. The results revealed no indication of any influential levels of random effects to exist. Significance for all tests was set to P = 0.05.
As urinary cortisol levels are known to decline throughout the day (60), we included sample collection time as a control variable into the LMM. Surprisingly, in contrast to previous chimpanzee studies (60–63), sample collection time was not a significant effect in our model (P = 0.091). To investigate whether this was due to altered diurnal urinary cortisol patterns in sick individuals, we ran a second LMM in which we included the interaction of health status (sick or healthy) with sample collection time as the only test predictor and excluded sample periods from the model, as this would overlap with health status. All other variables, such as urinary neopterin levels, sex, and age at sampling time, survival of the animal (yes or no), and whether the individual had been treated with antibiotics (yes or no), were included as control terms into the second LMM. Random effects were the same as described for the first LMM. We tested all model assumptions, as described earlier. No model assumptions were violated, and collinearity was also not an issue (maximum VIF: 1.5).
Results
Cortisol Levels Change During a Respiratory Outbreak Period
The average urinary cortisol level was 12.3 (ng/ml corr. SG) before the outbreak, which increased to an average of 140.5 (ng/ml corr. SG) during the outbreak, and declined to an average of 9.7 (ng/ml corr. SG) after chimpanzees did not anymore exhibit respiratory signs of illness (Table 1). Therefore, urinary cortisol levels increased more than 10-fold in chimpanzees with signs of illness in comparison with those in chimpanzees without (Figure 1). Two asymptomatic chimpanzees showed no changes in urinary cortisol levels during the outbreak (Supplementary Figure 1).
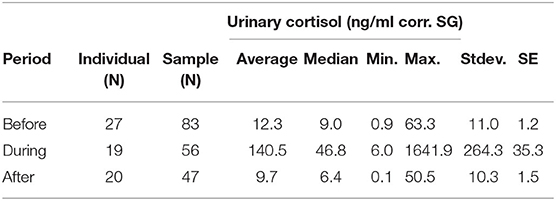
Table 1. Urinary cortisol levels (ng/ml corr. SG) in wild chimpanzees during each sampling period before, during, and after the respiratory outbreak between November—December 2009.
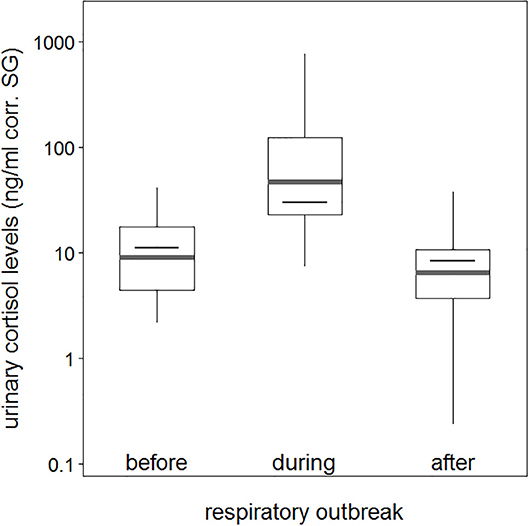
Figure 1. Urinary cortisol levels about sample periods (before, during, and after a respiratory outbreak). Indicated are the median (gray bar) and the fitted model and its 95% confidence intervals (black bar and error bars). Boxes indicate quartiles (25 and 75%), and vertical lines represent quantiles (2.5 and 97.5%). The y-axis is log-transformed.
Comparison of the full model to the null model was significant (χ2 = 114.0, df = 5, P < 0.001); however, the interaction between urinary neopterin levels with sample period was not a significant predictor for all three periods (before, during, and after the outbreak), indicating that urinary neopterin and urinary cortisol levels underwent similar changes (Estimate = −0.141, SE = 0.229, P = 0.841). Therefore, we ran a reduced model without the interaction term, with both urinary neopterin and sample period as independent predictor variables. The reduced model showed that both urinary neopterin and sample period (before, during, and after) were significant predictors of urinary cortisol levels (Table 2). Urinary cortisol and neopterin levels were positively associated, with increasing neopterin levels corresponding to increasing cortisol levels. No effect was found for sex or age at the time of sampling (Table 2, Supplementary Figure 2). Interestingly, the control variable sample collection time was also not a significant predictor of urinary cortisol levels, although urinary cortisol levels showed a trend in decline as the day progressed, with lower levels found later in the day (Table 2).
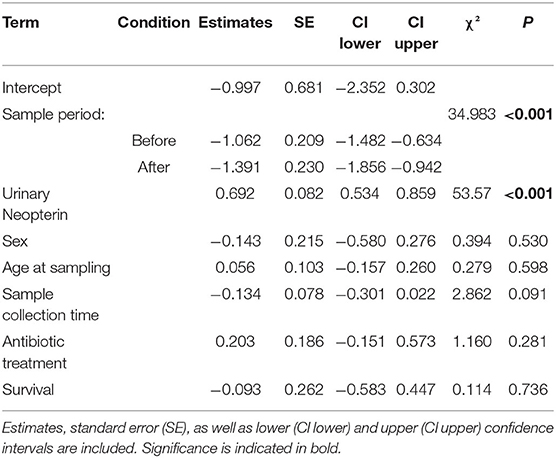
Table 2. LMM results testing the influence of urinary neopterin, sampling period (before, during, and after the outbreak), age, and sex in sick and healthy wild chimpanzees during a respiratory outbreak on urinary cortisol levels.
A post hoc comparison showed that urinary cortisol levels were significantly higher in sick chimpanzees than cortisol levels measured before and after the respiratory outbreak (before vs. during: Estimate = −1.062, SE = 0.209, P < 0.001; after vs. during: Estimate = −1.391, SE = 0.230, P < 0.001). Urinary cortisol levels before and after the respiratory outbreak were not significantly different (before vs. after: Estimate = 0.329, SE = 0.329, P = 0.108).
Diurnal Variation of Cortisol Levels
The comparison of the second full model to the null model was also significant (full-null model comparison: χ2 = 40.9, df = 3, P < 0.001), with the interaction between health status and sample collection time as the only effect tested. During asymptomatic periods before and after the outbreak, urinary cortisol levels were found to decline with sample collection time. However, when chimpanzees showed signs of respiratory disease, this pattern was no longer visible (Figure 2).
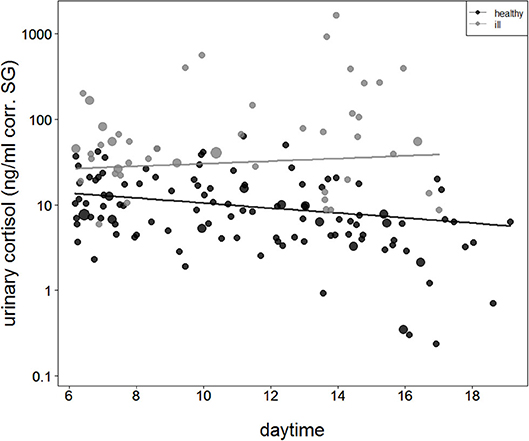
Figure 2. Urinary cortisol levels concerning sample collection time during asymptomatic periods (healthy = filled black circles and black straight line) and during the respiratory outbreak (ill = filled gray circles and straight gray line). The y-axis is log-transformed.
Discussion
In wild habituated chimpanzees, urinary cortisol levels increased when individuals exhibited signs of respiratory disease. These increases in cortisol levels were found to be related to the innate immune response as urinary neopterin levels increased positively with cortisol levels. Finally, although urinary cortisol levels showed the expected diurnal decline when chimpanzees were asymptomatic, interestingly, the diurnal decline was no longer significant when chimpanzees experienced respiratory signs of illness.
In our study, urinary cortisol showed a 10-fold increase during illness. Concerning other changes in urinary cortisol levels in chimpanzees, this is five times more than the nearly 2-fold increase, comparing periods of unstable vs. with stable male dominance relationship periods (62) or control days with hunting days or days with intergroup encounters (64). The comparably substantial increase in cortisol levels found in this study indicates that sick chimpanzees drastically increased glucose availability in their blood.
During the outbreak, increased cortisol levels in the wild chimpanzees may also indicate that they may have experienced fever, as it has been previously shown in goats, guinea pigs, and humans that fever always accompanied an increase in cortisol levels (7, 65, 66). Additionally, in humans, fever is energetically costly, as raising the body temperature requires increasing the basal metabolic rate (67). As the immune response requires energy for optimal functioning (68), it is possible that the wild chimpanzees were not able to compensate energy expenditure by increasing their energy intake while ill. As a consequence, cortisol secretion increased during this period, as glucocorticoids are essential for mobilizing stored energy resources (69). Additionally, certain sickness behaviors may further decrease the available energy budget, such as lower rates of foraging and, therefore, energy intake. Behavioral strategies may exist to conserve energy expenditure, for example, it has been previously shown in primates that when parasite infections were high, resting rates increase, whereas more costly behaviors (e.g., grooming and copulation) decrease (70, 71). In chimpanzees, energy may be restricted during periods of illness. For example, in Kanyawara chimpanzees during a respiratory outbreak, urinary c-peptide levels, a marker of energy balance, were found to decline despite favorable feeding conditions (72). Furthermore, during respiratory outbreaks in both Taï and Gombe chimpanzee populations, daily food intake decreased, as well as overall activity and travel distances (35, 36, 40, 73). It is, therefore, hypothesized that these sickness behaviors might help to conserve energy during an illness.
Wild animals may mount a greater cortisol response than captive animals. At the same time, wild animals are not typically provisioned with medications and food and water and still need to travel, climb, and forage for food, even when ill. In captive animals, however, an increase in energy intake through regular provisioning may allow them to cover the costs of mounting an immune response when ill. In captivity, sick animals also have shorter, or no, travel distances to forage for food, as it is usually provided in closer proximity. Therefore, during an illness, it can be assumed that energy homeostasis within a captive individual would be less affected than within wild animals during an illness. Cortisol levels were found to be stable in modern western humans during an experimental immune challenge. During this immune challenge, subjects were found to increase their caloric intake, which may have offset the energy costs incurred from mounting an immune response (37).
However, energetic stress may not be the exclusive explanation for elevated urinary cortisol levels during illness. Another factor could also be social stress as a consequence of isolation, as chimpanzees are highly social animals. During illness, a chimpanzee becomes isolated, often traveling at a slower pace than the group or spending extended periods of nesting (39, 71, 74). May those behavioral changes also help to conserve energy; however, self-distancing may also increase their psychological stress level; for example, this reduces the possibility of social buffering (75) and also increases predation risk (76).
Changes in the diurnal cortisol pattern support the prediction that wild chimpanzees mobilize glucose reserves when ill and, therefore, have increased urinary cortisol levels. As observed in healthy chimpanzees during our study, urinary cortisol levels were found to decline during the day—a characteristic pattern found in healthy chimpanzees (60–63), gorillas (77, 78), macaques (79), and humans (77, 80). The diurnal decline in urinary cortisol levels during days without symptoms is similar to the general pattern of diurnal decline observed in chimpanzees at this site (63). However, in chimpanzees displaying signs of a respiratory infection, urinary cortisol levels remained elevated throughout the day. A loss or disturbance of diurnal decline in cortisol levels has also been reported in humans with various disorders, including chronic fatigue syndrome, anxiety disorders, fibromyalgia, rheumatoid arthritis, depression, bipolar disorders, and respiratory diseases (81, 82). Moreover, the slope of diurnal cortisol levels throughout the day was also found to be flatter (83).
By monitoring immune challenges in wild-living animals, our study demonstrates that immune defenses have costs. Given the costs of living in a natural environment with fluctuating resources and in the face of immune challenges, understanding energy allocation between growth, maintenance, and health, as well as the interplay between immune function, sickness behaviors, and endocrinological correlates, is garnering increasing research interest. Here, we show that cortisol may play a role in mediating the innate immune response, with sick individuals exhibiting higher levels of both urinary cortisol and neopterin during an outbreak. Additionally, sick individuals did not display the typical healthy pattern of diurnal decline in cortisol levels—but rather, raised levels throughout the day. Although energetic stress seems to play a role in increasing urinary cortisol during periods of illness, other factors, such as psychological or social stress, should not be discounted. Further examination of sickness behaviors with other endocrinological, immunological, or energetic biomarkers is required to understand better the adoption of various life-history patterns and how organisms may modulate their physiological processes to increase their survival and fitness.
Data Availability Statement
The raw data supporting the conclusions of this article will be made available by the authors, without undue reservation.
Ethics Statement
Ethical approval was not required for this study in line with institutional guidelines and local legislation, because urine samples were collected non-invasively without animals' disturbance or harming the chimpanzees.
Author Contributions
VB, DW, RW, and TD: conception and design. CC, RW, and TD: sample acquisition. VB and AP: statistical analysis. All authors were involved in the interpretation of the data. VB and TD: drafting of the manuscript. All authors revised, reviewed and approved the final version of the manuscript.
Funding
Funding was provided by the Max Planck Society as well as the Deutsche Forschungsgemeinschaft and conducted as part of the research group Sociality and Health in Primates (FOR2136, DE 1135/2–1, LE 1813/10–1, and WI 2637/3-1). CC received funding from the European Research Council under the European Union's Horizon 2020 research and innovation programme (grant agreement no. 679787). The Max Planck Society provides core funding for the Taï Chimpanzee Project since 1997.
Conflict of Interest
The authors declare that the research was conducted in the absence of any commercial or financial relationships that could be construed as a potential conflict of interest.
Acknowledgments
We thank the Ministry of Environment and Forests, Ministry of Higher Education and Scientific Research, and the Office Ivoirien des Parcs et Réserves for permission to conduct the study as well as the staff of the Centre Suisse de Recherches Scientifiques in Côte d'Ivoire and the Taï Chimpanzee Project, field assistants, and veterinarians for their support in the field. Thank you to Vera Schmeling for lab assistance, Frauke Olthoff for technical support, and Livia Victoria Patrono for comments on an earlier version of the manuscript.
Supplementary Material
The Supplementary Material for this article can be found online at: https://www.frontiersin.org/articles/10.3389/fvets.2020.00485/full#supplementary-material
References
1. Hau M. Regulation of male traits by testosterone: implications for the evolution of vertebrate life histories. BioEssays. (2007) 29:133–44. doi: 10.1002/bies.20524
2. Elenkov IJ, Chrousos GP. Stress hormones, Th1/Th2 patterns, pro/anti-inflammatory cytokines and susceptibility to disease. Trends Endocrinol Metab. (1999) 10:359–68. doi: 10.1016/S1043-2760(99)00188-5
3. Webster JI, Tonelli L, Sternberg EM. Neuroendocrine regulation of immunity. Annu Rev Immunol. (2002) 20:125–63. doi: 10.1146/annurev.immunol.20.082401.104914
4. Beehner JC, Bergman TJ. The next step for stress research in primates: to identify relationships between glucocorticoid secretion and fitness. Horm Behav. (2017) 91:68–83. doi: 10.1016/j.yhbeh.2017.03.003
5. Markham AC, Gesquiere LR. Costs and benefits of group living in primates: an energetic perspective. Philos Trans R Soc B Biol Sci. (2017) 372:20160239. doi: 10.1098/rstb.2016.0239
6. Padgett DA, Glaser R. How stress influences the immune response. Trends Immunol. (2003) 24:444–8. doi: 10.1016/S1471-4906(03)00173-X
7. Mphahlele NR, Fuller A, Roth J, Kamerman PR. Body temperature, behavior, and plasma cortisol changes induced by chronic infusion of Staphylococcus aureus in goats. Am J Physiol-Regul Integr Comp Physiol. (2004) 287:R863–9. doi: 10.1152/ajpregu.00064.2004
8. Beishuizen A, Thijs LG. Review: endotoxin and the hypothalamo-pituitary-adrenal (HPA) axis. J Endotoxin Res. (2003) 9:3–24. doi: 10.1177/09680519030090010101
9. Klein SL. Hormonal and immunological mechanisms mediating sex differences in parasite infection. Parasite Immunol. (2004) 26:247–64. doi: 10.1111/j.0141-9838.2004.00710.x
10. Berger A. Science commentary: Th1 and Th2 responses: what are they? BMJ. (2000) 321:424. doi: 10.1136/bmj.321.7258.424
11. Pinto RA, Arrendono SM, Bono MR, Gaggero AA, Díaz PV. T helper 1/t helper 2 cytokine imbalance in respiratory syncytial virus infection is associated with increased endogenous plasma cortisol. Pediatrics. (2006) 117:e878–86. doi: 10.1542/peds.2005-2119
12. Murr C, Widner B, Wirleitner B, Fuchs D. Neopterin as a marker for immune system activation. Curr Drug Metab. (2002) 3:175–87. doi: 10.2174/1389200024605082
13. Gervasi SS, Burgan SC, Hofmeister E, Unnasch TR, Martin LB. Stress hormones predict a host superspreader phenotype in the west nile virus system. Proc R Soc B Biol Sci. (2017) 284:20171090. doi: 10.1098/rspb.2017.1090
14. Brace AJ, Lajeunesse MJ, Ardia DR, Hawley DM, Adelman JS, Buchanan KL, et al. Costs of immune responses are related to host body size and lifespan. J Exp Zool Part Ecol Integr Physiol. (2017) 327:254–61. doi: 10.1002/jez.2084
15. Watkins LR, Maier SF. The pain of being sick: implications of immune-to-brain communication for understanding pain. Annu Rev Psychol. (2000) 51:29–57. doi: 10.1146/annurev.psych.51.1.29
16. Dantzer R. Cytokine-induced sickness behaviour: a neuroimmune response to activation of innate immunity. Eur J Pharmacol. (2004) 500:399–411. doi: 10.1016/j.ejphar.2004.07.040
17. Ashley NT, Wingfield JC. Sickness behavior in vertebrates: allostasis, life-history modulation, hormonal regulation. In: Demas GE, Nelson RJ, editors. Ecoimmunology. (Oxford, NY: Oxford University Press) (2012). p. 45–91.
18. Brace AJ, Sheikali S, Martin LB. Highway to the danger zone: exposure-dependent costs of immunity in a vertebrate Ectotherm. Funct Ecol. (2015) 29:924–30. doi: 10.1111/1365-2435.12402
19. Lochmiller RL, Deerenberg C. Trade-offs in evolutionary immunology: just what is the cost of immunity? Oikos. (2000) 88:87–98. doi: 10.1034/j.1600-0706.2000.880110.x
20. Ruiz M, French SS, Demas GE, Martins EP. Food supplementation and testosterone interact to influence reproductive behavior and immune function in Sceloporus graciosus. Horm Behav. (2010) 57:134–9. doi: 10.1016/j.yhbeh.2009.09.019
21. Lopes PC, Springthorpe D, Bentley GE. Increased activity correlates with reduced ability to mount immune defenses to endotoxin in zebra finches. J Exp Zool Part Ecol Genet Physiol. (2014) 321:422–31. doi: 10.1002/jez.1873
22. Lopes PC. Why are behavioral and immune traits linked? Horm Behav. (2017) 88:52–9. doi: 10.1016/j.yhbeh.2016.09.008
23. Hart BL. Biological basis of the behavior of sick animals. Neurosci Biobehav Rev. (1988) 12:123–37. doi: 10.1016/S0149-7634(88)80004-6
24. Buehler DM, Piersma T, Irene Tieleman B. Captive and free-living red knots Calidris canutus exhibit differences in non-induced immunity that suggest different immune strategies in different environments. J Avian Biol. (2008) 39:560–6. doi: 10.1111/j.0908-8857.2008.04408.x
25. Fair PA, Schaefer AM, Houser DS, Bossart GD, Romano TA, Champagne CD, et al. The environment as a driver of immune and endocrine responses in dolphins (Tursiops truncatus). PLoS ONE. (2017) 12:e0176202. doi: 10.1371/journal.pone.0176202
26. Behringer V, Stevens JMG, Wittig RM, Crockford C, Zuberbühler K, Leendertz FH, et al. Elevated neopterin levels in wild, healthy chimpanzees indicate constant investment in unspecific immune system. BMC Zool. (2019) 4:2. doi: 10.1186/s40850-019-0041-1
27. Chi F, Leider M, Leendertz F, Bergmann C, Boesch C, Schenk S, et al. New Streptococcus pneumoniae clones in deceased wild chimpanzees. J Bacteriol. (2007) 189:6085–8. doi: 10.1128/JB.00468-07
28. Kirchhoff CA. From birth to bones: skeletal evidence for health, disease, and injury in the gombe chimpanzees (Ph.D. dissertation). University of Minnesota, Minneapolis, MN, United States (2010).
29. Löhrich T, Behringer V, Wittig RM, Deschner T, Leendertz FH. The use of neopterin as a noninvasive marker in monitoring diseases in wild chimpanzees. EcoHealth. (2018) 15:792–803. doi: 10.1007/s10393-018-1357-y
30. Negrey JD, Reddy RB, Scully EJ, Phillips-Garcia S, Owens LA, Langergraber KE, et al. Simultaneous outbreaks of respiratory disease in wild chimpanzees caused by distinct viruses of human origin. Emerg Microbes Infect. (2019) 8:139–49. doi: 10.1080/22221751.2018.1563456
31. Patrono LV, Samuni L, Corman VM, Nourifar L, Röthemeier C, Wittig RM, et al. Human coronavirus OC43 outbreak in wild chimpanzees, Côte d'Ivoire, 2016. Emerg Microbes Infect. (2018) 7:118. doi: 10.1038/s41426-018-0121-2
32. Rushmore J, Caillaud D, Matamba L, Stumpf RM, Borgatti SP, Altizer S. Social network analysis of wild chimpanzees provides insights for predicting infectious disease risk. J Anim Ecol. (2013) 82:976–86. doi: 10.1111/1365-2656.12088
33. Wu DF, Behringer V, Wittig RM, Leendertz FH, Deschner T. Urinary neopterin levels increase and predict survival during a respiratory outbreak in wild chimpanzees (Taï National Park, Côte d'Ivoire). Sci Rep. (2018) 8:13346. doi: 10.1038/s41598-018-31563-7
34. Wolf TM, Singer RS, Lonsdorf EV, Maclehose R, Gillespie TR, Lipende I, et al. Syndromic surveillance of respiratory disease in free-living chimpanzees. EcoHealth. (2019) 16:275–86. doi: 10.1007/s10393-019-01400-y
35. Köndgen S, Kühl H, N'Goran PK, Walsh PD, Schenk S, Ernst N, et al. Pandemic human viruses cause decline of endangered great apes. Curr Biol. (2008) 18:260–4. doi: 10.1016/j.cub.2008.01.012
36. Goodall J. The Chimpanzees of Gombe: Patterns of Behavior. Cambridge, MA: Belknap Press of Harvard University Press (1986).
37. Shattuck EC, Muehlenbein MP. Mood, behavior, testosterone, cortisol, and interleukin-6 in adults during immune activation: a pilot study to assess sickness behaviors in humans: sickness behaviors in humans. Am J Hum Biol. (2015) 27:133–5. doi: 10.1002/ajhb.22608
38. Wittig RM. Taï chimpanzees. In: Vonk J, Shackelford T, editors. Encyclopedia of Animal Cognition Behavior. Cham: Springer International Publishing (2018). p. 1–7. doi: 10.1007/978-3-319-47829-6_1564-1
39. Boesch C, Boesch-Achermann H. The Chimpanzees of the Taï Forest: Behavioural Ecology and Evolution. Oxford; New York, NY: Oxford University Press (2000).
40. Schenk S. Respiratorische Erkrankungen bei wildlebenden Schimpansen im Taï-Nationalpark. Berlin: University of Berlin (2007).
41. Köndgen S, Calvignac-Spencer S, Grützmacher K, Keil V, Mätz-Rensing K, Nowak K, et al. Evidence for human Streptococcus pneumoniae in wild and captive chimpanzees: a potential threat to wild populations. Sci Rep. (2017) 7:14581. doi: 10.1038/s41598-017-14769-z
42. Szentiks CA, Köndgen S, Silinski S, Speck S, Leendertz FH. Lethal pneumonia in a captive juvenile chimpanzee (Pan troglodytes) due to human-transmitted human respiratory syncytial virus (HRSV) and infection with Streptococcus pneumoniae. J Med Primatol. (2009) 38:236–40. doi: 10.1111/j.1600-0684.2009.00346.x
43. Clarke CJ, Watt NJ, Meredith A, McIntyre N, Burns SM. Respiratory syncytial virus-associated bronchopneumonia in a young chimpanzee. J Comp Pathol. (1994) 110:207–12. doi: 10.1016/S0021-9975(08)80191-0
44. Gilardi KV, Gillespie TR, Leendertz FH, Macfie EJ, Travis DA, Whittier CA, et al. Best Practice guidelines for Health Monitoring and Disease Control in Great Ape Populations. IUCN International Union for Conservation of Nature (2015). doi: 10.2305/IUCN.CH.2015.SSC-OP.56.en
45. Leendertz FH, Pauli G, Maetz-Rensing K, Boardman W, Nunn C, Ellerbrok H, et al. Pathogens as drivers of population declines: the importance of systematic monitoring in great apes and other threatened mammals. Biol Conserv. (2006) 131:325–37. doi: 10.1016/j.biocon.2006.05.002
46. Hauser B, Deschner T, Boesch C. Development of a liquid chromatography–tandem mass spectrometry method for the determination of 23 endogenous steroids in small quantities of primate urine. J Chromatogr B. (2008) 862:100–12. doi: 10.1016/j.jchromb.2007.11.009
47. Wessling EG, Kühl HS, Mundry R, Deschner T, Pruetz JD. The costs of living at the edge: seasonal stress in wild savanna-dwelling chimpanzees. J Hum Evol. (2018) 121:1–11. doi: 10.1016/j.jhevol.2018.03.001
48. Miller RC, Brindle E, Holman DJ, Shofer J, Klein NA, Soules MR, et al. Comparison of specific gravity and creatinine for normalizing urinary reproductive hormone concentrations. Clin Chem. (2004) 50:924–32. doi: 10.1373/clinchem.2004.032292
49. Baayen RH. Analyzing Linguistic Data. Cambridge, UK; New York, NY: Cambridge University Press (2008) doi: 10.1017/CBO9780511801686
50. R Development Core Team. R: A Language and Environment for Statistical Computing. R Foundation for Statistical Computing. Vienna (2008). Available online at: http://www.R-project.org
51. Bates D, Mächler M, Bolker B, Walker S. Fitting linear mixed-effects models using lme4. ArXiv Prepr ArXiv14065823. (2014) doi: 10.18637/jss.v067.i01
52. Schielzeth H. Simple means to improve the interpretability of regression coefficients. Methods Ecol Evol. (2010) 1:103–13. doi: 10.1111/j.2041-210X.2010.00012.x
53. Barr DJ, Levy R, Scheepers C, Tily HJ. Random effects structure for confirmatory hypothesis testing: keep it maximal. J Mem Lang. (2013) 68:255–78. doi: 10.1016/j.jml.2012.11.001
54. Schielzeth H, Forstmeier W. Conclusions beyond support: overconfident estimates in mixed models. Behav Ecol. (2009) 20:416–20. doi: 10.1093/beheco/arn145
55. Dobson AJ, Barnett AG. An Introduction to Generalized Linear Models. 3rd ed. Boca Raton, FL: CRC Press (2008).
56. Bretz F, Hothorn T, Westfall PH. Multiple Comparisons Using R. Boca Raton, FL: Chapman & Hall/CRC Press (2011).
57. Hothorn T, Bretz F, Westfall P. Simultaneous inference in general parametric models. Biom J. (2008) 50:346–63. doi: 10.1002/bimj.200810425
59. Fox J. An R Companion to Applied Regression. 2nd ed. Thousand Oaks, CA: SAGE Publications (2011).
60. Muller MN, Lipson SF. Diurnal patterns of urinary steroid excretion in wild chimpanzees. Am J Primatol. (2003) 60:161–6. doi: 10.1002/ajp.10103
61. Anestis SF, Bribiescas RG. Rapid changes in chimpanzee (Pan troglodytes) urinary cortisol excretion. Horm Behav. (2004) 45:209–13. doi: 10.1016/j.yhbeh.2003.09.015
62. Preis A, Samuni L, Deschner T, Crockford C, Wittig RM. Urinary cortisol, aggression, dominance and competition in wild, west African male chimpanzees. Front Ecol Evol. (2019) 7:107. doi: 10.3389/fevo.2019.00107
63. Sonnweber R, Araya-Ajoy YG, Behringer V, Deschner T, Tkaczynski P, Fedurek P, et al. Circadian rhythms of urinary cortisol levels vary between individuals in wild male chimpanzees: a reaction norm approach. Front Ecol Evol. (2018) 6:85. doi: 10.3389/fevo.2018.00085
64. Samuni L, Preis A, Deschner T, Wittig RM, Crockford C. Cortisol and oxytocin show independent activity during chimpanzee intergroup conflict. Psychoneuroendocrinology. (2019) 104:165–73. doi: 10.1016/j.psyneuen.2019.02.007
65. Roth J, Hübschle T, Pehl U, Ross G, Gerstberger R. Influence of systemic treatment with cyclooxygenase inhibitors on lipopolysaccharide-induced fever and circulating levels of cytokines and cortisol in guinea-pigs. Pflüg Arch - Eur J Physiol. (2002) 443:411–7. doi: 10.1007/s004240100718
66. Gatti R, Antonelli G, Prearo M, Spinella P, Cappellin E, De Palo EF. Cortisol assays and diagnostic laboratory procedures in human biological fluids. Clin Biochem. (2009) 42:1205–17. doi: 10.1016/j.clinbiochem.2009.04.011
67. Kluger MJ. Fever: role of pyrogens and cryogens. Physiol Rev. (1991) 71:93–127. doi: 10.1152/physrev.1991.71.1.93
68. Canale CI, Henry P-Y. Energetic costs of the immune response and torpor use in a primate: heterotherms and immune response. Funct Ecol. (2011) 25:557–65. doi: 10.1111/j.1365-2435.2010.01815.x
69. Silverman MN, Mukhopadhyay P, Belyavskaya E, Tonelli LH, Revenis BD, Doran JH, et al. Glucocorticoid receptor dimerization is required for proper recovery of LPS-induced inflammation, sickness behavior and metabolism in mice. Mol Psychiatry. (2013) 18:1006–17. doi: 10.1038/mp.2012.131
70. Ghai RR, Fugère V, Chapman CA, Goldberg TL, Davies TJ. Sickness behaviour associated with non-lethal infections in wild primates. Proc R Soc B Biol Sci. (2015) 282:20151436. doi: 10.1098/rspb.2015.1436
71. Patrono LV, Pléh K, Samuni L, Ulrich M, Röthemeier C, Sachse A, et al. Monkeypox virus emergence in wild chimpanzees reveals distinct clinical outcomes and viral diversity. Nat Microbiol. (2020) 955–65. doi: 10.1038/s41564-020-0706-0
72. Emery Thompson M, Muller MN, Wrangham RW, Lwanga JS, Potts KB. Urinary C-peptide tracks seasonal and individual variation in energy balance in wild chimpanzees. Horm Behav. (2009) 55:299–305. doi: 10.1016/j.yhbeh.2008.11.005
73. Wallis J, Lee DR. Primate conservation: the prevention of disease transmission. Int J Primatol. (1999) 20:803–26. doi: 10.1023/A:1020879700286
74. Reynolds V. The Chimpanzees of the Budongo Forest. Oxford, NY: Oxford University Press (2005). doi: 10.1093/acprof:oso/9780198515463.001.0001
75. Wittig RM, Crockford C, Weltring A, Langergraber KE, Deschner T, Zuberbühler K. Social support reduces stress hormone levels in wild chimpanzees across stressful events and everyday affiliations. Nat Commun. (2016) 7:13361. doi: 10.1038/ncomms13361
76. Boesch C. The effects of leopard predation on grouping patterns in forest chimpanzees. Behaviour. (1991) 117:220–41. doi: 10.1163/156853991X00544
77. Czekala NM, Lance VA, Sutherland-Smith M. Diurnal urinary corticoid excretion in the human and gorilla. Am J Primatol. (1994) 34:29–34. doi: 10.1002/ajp.1350340107
78. Robbins MM, Czekala NM. A preliminary investigation of urinary testosterone and cortisol levels in wild male mountain gorillas. Am J Primatol. (1997) 43:51–64. doi: 10.1002/(SICI)1098-2345(1997)43:1<51::AID-AJP4>3.0.CO;2-X
79. van Schaik CP, van Noordwijk MA, van Bragt T, Blankenstein MA. A pilot study of the social correlates of levels of urinary cortisol, prolactin, and testosterone in wild long-tailed macaques (Macaca fascicularis). Primates. (1991) 32:345–56. doi: 10.1007/BF02382675
80. Whitten PL, Brockman DK, Stavisky RC. Recent advances in noninvasive techniques to monitor hormone-behavior interactions. Yearb Phys Anthropol. (1998) 41:1–23. doi: 10.1002/(SICI)1096-8644(1998)107:27+<1::AID-AJPA2>3.0.CO;2-H
81. Havermans R, Nicolson NA, Berkhof J, deVries MW. Patterns of salivary cortisol secretion and responses to daily events in patients with remitted bipolar disorder. Psychoneuroendocrinology. (2011) 36:258–65. doi: 10.1016/j.psyneuen.2010.07.016
82. Smyth JM, Ockenfels MC, Gorin AA, Catley D, Porter LS, Kirschbaum C, et al. Individual differences in the diurnal cycle of cortisol. Psychoneuroendocrinology. (1997) 22:89–105. doi: 10.1016/S0306-4530(96)00039-X
Keywords: disease monitoring, non-invasive, pan troglodytes, ecoimmunology, costly immune responses
Citation: Behringer V, Preis A, Wu DF, Crockford C, Leendertz FH, Wittig RM and Deschner T (2020) Urinary Cortisol Increases During a Respiratory Outbreak in Wild Chimpanzees. Front. Vet. Sci. 7:485. doi: 10.3389/fvets.2020.00485
Received: 03 April 2020; Accepted: 29 June 2020;
Published: 21 August 2020.
Edited by:
Jeremy N. Marchant-Forde, Livestock Behavior Research Unit (USDA-ARS), United StatesReviewed by:
Liza Rose Moscovice, Leibniz Institute for Farm Animal Biology (FBN), GermanyMoira Harris, Harper Adams University, United Kingdom
Copyright © 2020 Behringer, Preis, Wu, Crockford, Leendertz, Wittig and Deschner. This is an open-access article distributed under the terms of the Creative Commons Attribution License (CC BY). The use, distribution or reproduction in other forums is permitted, provided the original author(s) and the copyright owner(s) are credited and that the original publication in this journal is cited, in accordance with accepted academic practice. No use, distribution or reproduction is permitted which does not comply with these terms.
*Correspondence: Verena Behringer, VmVyZW5hX2JlaHJpbmdlciYjeDAwMDQwO2V2YS5tcGcuZGU=