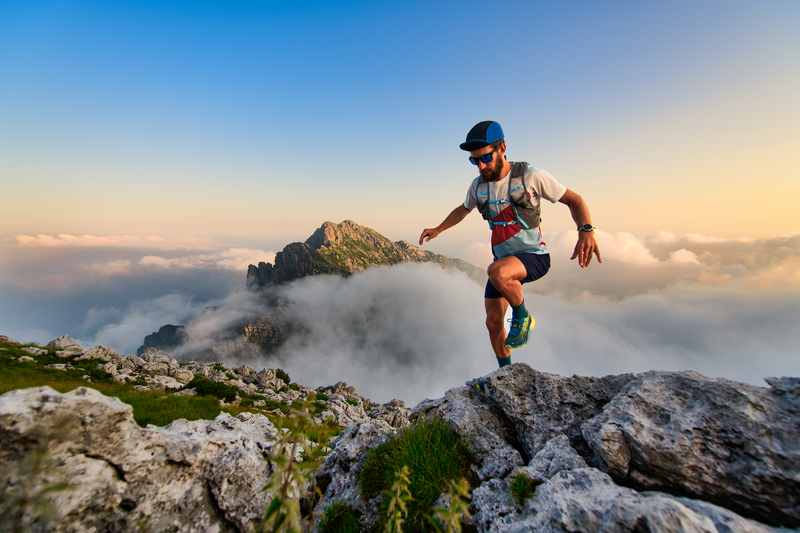
95% of researchers rate our articles as excellent or good
Learn more about the work of our research integrity team to safeguard the quality of each article we publish.
Find out more
REVIEW article
Front. Vet. Sci. , 25 August 2017
Sec. Veterinary Infectious Diseases
Volume 4 - 2017 | https://doi.org/10.3389/fvets.2017.00138
This article is part of the Research Topic Mechanisms of Persistence, Survival, and Transmission of Bacterial Foodborne Pathogens in Production Animals View all 12 articles
Salmonella are important pathogens worldwide and a predominant number of human infections are zoonotic in nature. The ability of strains to form biofilms, which is a multicellular behavior characterized by the aggregation of cells, is predicted to be a conserved strategy for increased persistence and survival. It may also contribute to the increasing number of infections caused by ingestion of contaminated fruits and vegetables. There is a correlation between biofilm formation and the ability of strains to colonize and replicate within the intestines of multiple host species. These strains predominantly cause localized gastroenteritis infections in humans. In contrast, there are salmonellae that cause systemic, disseminated infections in a select few host species; these “invasive” strains have a narrowed host range, and most are unable to form biofilms. This includes host-restricted Salmonella serovar Typhi, which are only able to infect humans, and atypical gastroenteritis strains associated with the opportunistic infection of immunocompromised patients. From the perspective of transmission, biofilm formation is advantageous for ensuring pathogen survival in the environment. However, from an infection point of view, biofilm formation may be an anti-virulence trait. We do not know if the capacity to form biofilms prevents a strain from accessing the systemic compartments within the host or if loss of the biofilm phenotype reflects a change in a strain’s interaction with the host. In this review, we examine the connections between biofilm formation, Salmonella disease states, degrees of host adaptation, and how this might relate to different transmission patterns. A better understanding of the dynamic lifecycle of Salmonella will allow us to reduce the burden of livestock and human infections caused by these important pathogens.
The current system of Salmonella nomenclature is based on layers of genetic, biochemical, and serological classification. New Salmonella strains can be categorized into species and subspecies according to DNA relatedness at the genomic level, originally shown through DNA–DNA hybridization, and the presence or absence of 11 biochemical traits (1, 2). Two species have been defined, Salmonella bongori and Salmonella enterica, of which S. enterica is further split into six subspecies that are designated by a Roman numeral and name (I, enterica; II, salamae; IIIa, arizonae; IIIb, diarizonae; IV, houtenae; and VI, indica) (Figure 1) (3). Salmonella are further subdivided into serovars using the classical Kauffman and White classification system (4). Serovars are representative of a unique combination of flagellar antigens (H1 and H2) and (lipopolysaccharide) oligosaccharide (O) or capsular polysaccharide (K) antigens (5). Using this classical system, more than 2,600 serovars have been identified, with their given name reflecting their combination of antigens, or in the case of serovars in subspecies enterica, a name representing their associated disease, host specificity, geographic origin, or relationship to other identified serovars (5, 6). Whole genome sequencing (WGS) is increasingly being used for classification, such as the sequencing of every Salmonella isolate by Public Health England (7) or the new typing scheme developed for S. enterica serovar Typhi (8). Strain typing via WGS has so far demonstrated promising results, both by supporting the current structure of Salmonella serovar nomenclature and by providing improved resolution of the phylogenetic relationship between Salmonella isolates (7). In regards to infection, subspecies I, or S. enterica subspecies enterica is the most well-represented among serovars and disease, accounting for approximately 60% of all serovars identified and greater than 95% of Salmonella isolates obtained from humans and domestic mammals (9, 10). In contrast, Salmonella isolates belonging to the remaining species and subspecies are normally obtained from cold-blooded hosts, and are only occasionally able to cause infections in humans (11).
Figure 1. Salmonella taxonomy and general classifications. The genus Salmonella is classified into species, subspecies, and serovars based on the White–Kauffman–Le Minor scheme. Serovars are often grouped into non-typhoidal or typhoidal categories; however, this referencing approach is not a part of the official Salmonella classification scheme. For a expanded version of the taxonomical distribution of Salmonella, readers are referred to Ref. (9).
Pathogenic Salmonella strains cause three main types of infections in humans. Gastroenteritis (150 million annual cases) is caused by many of the serovars in subspecies enterica, with serovars Typhimurium and Enteritidis being the most common (12, 13). In immunocompetent individuals, gastroenteritis infections involve the short-term colonization of the pathogen within the gastrointestinal (GI) tract, resulting in a localized inflammatory immune response accompanied by profuse diarrhea (14). The Salmonella serovars causing gastroenteritis are collectively referred to as non-typhoidal Salmonella (NTS). Enteric or typhoid fever (26.9 million annual cases) is caused by S. enterica serovars Typhi and Paratyphi, which are known as typhoidal Salmonella (15). This distinct disease involves invasion of the extra-intestinal compartment, often without the induction of inflammation or diarrhea (16). While such infections can occur asymptomatically, clinical manifestations of typhoidal Salmonella infections may include a persistent and gradual fever that elevates in a stepwise manner, as well as other symptoms, such as headache, chills, nausea, coughing, malaise, or a rapid pulse (16). The yearly mortality attributed to typhoidal Salmonella is estimated at ~145,000 deaths, which is more than double the number of deaths associated with gastroenteritis (15). After spreading systemically in their host, typhoidal Salmonella have the potential to persist for several weeks to years as a result of the pathogen’s intracellular association with monocytes and macrophages and potential long-term colonization of the gall bladder (17). The third human disease is caused by a group of NTS strains that cause systemic infections and have an increased association with bloodstream infections in sub-Saharan Africa (18). Like typhoidal Salmonella infections, invasive non-typhoidal Salmonella (iNTS) disease frequently lacks diarrheal symptoms, with febrile illness being the dominant clinical presentation in 95% of cases (19). This disease has a huge burden of mortality in the hardest hit areas, with an estimated 681,000 deaths per year. Due to non-specific symptomology and multidrug resistance of iNTS strains, there are often poor clinical outcomes despite correct diagnosis (18). A review of several clinical studies has revealed a significant association between invasive infections with NTS and immunocompromised populations, particularly children with malnutrition or severe malaria and adults with advanced infections of human immunodeficiency virus (HIV) (18–20). Failure of the immune system to maintain the intestinal epithelial barrier or to control intracellular Salmonella infections in these individuals provides a unique opportunity for NTS serovars to persist in the host (19).
Salmonella species continue to have a significant impact on global health. In a recent study analyzing the impact of 22 of the world’s most important foodborne pathogens, non-typhoidal, and typhoidal Salmonella were listed #1 and #2 in terms of disability adjusted life years (DALY) (15). The DALY metric is a measure of the overall disease burden, or the number of years lost due to ill-health, disability or early death. However, it does not take into account the economic impact of disease. In the United States of America alone, the impact of Salmonella infections related to hospital time, treatment costs, and lost work productivity have been estimated in the billions of dollars each year (21, 22). In addition to these estimates, Salmonella also presents as a significant economic challenge for producer groups and governments that are tasked with screening for and eliminating these pathogens within livestock species. Taken together, the different measures of impact demonstrate why it is so important to understand the complete Salmonella lifecycle, including how cells survive, persist and are transmitted between hosts.
The majority of bacterial life in nature is thought to exist in biofilms, a mode of growth where cells aggregate and become embedded in a self-produced extracellular matrix, usually in contact with a physical surface. Up to 40% of human and livestock diseases are thought to be biofilm-related and have enormous medical and economic impacts (23, 24). In addition, most of these biofilms are polymicrobial in nature (25). The exact reasons why bacteria aggregate together are not fully understood. There are examples of emergent behaviors associated with aggregation of larger numbers of cells, such as the enhanced breakdown of chitin by Vibrio cholerae (26). There is also the possibility that polymer production within the biofilm is the result of cells competing with each other for access to oxygen or nutrients (27). The presence of extracellular polymers themselves can create a unique microenvironment for cells within a biofilm, by inducing potential oxygen gradients (28, 29), or signaling nutrient limitation (30). An example of this was demonstrated for Pseudomonas aeruginosa, where the presence of DNA in the extracellular matrix imposed a cation restriction on the cells inside the biofilm, which in turn led to increased antibiotic resistance in the biofilm cells (31). Thus, the characteristic properties of a biofilm may be due in part to the physical barriers provided by the matrix polymers and also to the microenvironments induced by growth at high cell densities within the matrix.
For Salmonella, the best studied biofilm phenotype has been termed the rdar morphotype, named for the red, dry, and rough appearance of colonies grown on agar plates containing Congo red dye (32, 33). Congo red accumulates within the rdar colony due to the presence of the proteinaceous curli fimbriae, which are functional amyloid structures that are resistant to detergents, pH, and proteases (32, 34), and cellulose, the β1-4-linked glucose polymer, which is another resistant polymer (35). These two components function as the extracellular matrix scaffold, with curli providing short-range interactions between cells and cellulose providing long-range interactions over the distance of the entire colony (36). Together their production leads to a rough and dry colony appearance (37) (Figure 2A). There are other polymers known to be present within the rdar extracellular matrix, such as the O-antigen capsule (38) and polysaccharides yet to be fully characterized (39), as well as proteins, such as flagella, that contribute to the architecture of the resulting colony (40). In standing liquid cultures, biofilms with a matrix comprised of curli and cellulose have been described as pellicles, which refer to the film of cell growth that appears at the air–liquid interface (33, 41, 42) (Figure 2B). BapA, a large Salmonella protein containing numerous repeated sequences, has been shown to contribute to the strength and integrity of these pellicles (43). There are also biofilms formed at the air–liquid interface in severely nutrient-limited liquid media that are composed of cellulose, but not curli (42, 44). We recently developed an in vitro flask model for studying Salmonella biofilm development, where the cells in the culture differentiate into two distinct populations: multicellular aggregates and planktonic cells. The multicellular aggregates produce the same polymers as standard biofilms (45) and accumulate in the bottom of the flask, whereas the planktonic cells remain suspended in the growth media (Figure 2C). The proportion of each cell type within replicate flask populations is relatively stable (Figure 2D).
Figure 2. Examples of Salmonella biofilm formation. (A) Colonies grown for 48 h at 28°C on solid 1% tryptone media form the characteristic surface patterns of the red, dry, and rough (rdar) morphotype. The colony appears red when the media is supplemented with the dye Congo red. (B) Pellicle formation at the air–liquid interface of a 1% tryptone liquid culture [adapted from Ref. (46)]. (C) Salmonella form multicellular aggregates and planktonic cells within the bulk liquid phase of a flask culture. (D) The number of colony forming units (CFU) present in aggregate or planktonic cell subpopulations from (C) was calculated using conversion factors determined from serial dilution plating after homogenization (1.92 × 109 CFU per 1.0 OD600 for planktonic cells; 1.73 × 108 CFU/mg for aggregates). The green bars and blue bars represent the proportion of planktonic cells and aggregates comprising the total number of cells in the population; points on the right side of the graph represent total CFU values for each cell type from nine replicate flask cultures. The percentage values represent the average proportion of each cell type.
Each of the biofilms described above, except for cellulose-dominated biofilms formed on glass, are related in regulatory mechanisms and are activated in a similar way (Figure 3A). Regulation feeds through CsgD, a transcriptional regulatory protein that activates the biosynthesis of the majority of biofilm polymers described above (46, 47). The favored growth conditions for biofilm formation are media of low osmolarity, at temperatures below 30°C, and in the presence of gluconeogenic substrates, such as amino acids (33). Nutrient limitation is known to activate polymer production, but there are many inputs into the csgD promoter, which is part of one of the most complex regulatory networks in Salmonella [see Ref. (47) or (48) for a comprehensive review]. Important biofilm-activating factors include microaerophilic oxygen levels (49), iron limitation (33) and the presence of bis-(3′–5′)-cyclic dimeric guanosine monophosphate, or cyclic-di-GMP (50). Cyclic-di-GMP is a bacteria-specific secondary messenger molecule that is known to activate biofilm formation when produced at high levels, almost universally in all bacterial species where it has been examined [see Ref. (51) for a review]. In Salmonella, there are 22 enzymes that can potentially regulate c-di-GMP levels, but only a subset affect csgD expression directly (44, 52). Finally, the presence of glucose has been shown to repress biofilm formation and csgD transcription (53, 54). This indicates that biofilm formation is a choice heavily influenced by the growth potential in the environment surrounding the cells.
Figure 3. Salmonella phenotypes that result from the activity of CsgD and c-di-GMP. (A) Synthesis of biofilm-associated polymers is regulated at the genetic level by CsgD and the secondary messenger molecule c-di-GMP. Multiple environmental conditions act as inducing signals for csgD expression, CsgD synthesis, and c-di-GMP production. These environmental conditions (represented here as a lightning bolt) are transduced into intracellular signals via outer membrane proteins, two-component signal transduction systems, regulatory proteins, and enzymes associated with c-di-GMP production. A select set of diguanylate cyclases (STM1283, STM2123, STM2672, and STM1987) can contribute to the c-di-GMP pool that induces BcsE and BcsA activity, resulting in cellulose biosynthesis. CsgD is the master transcriptional regulator associated with Salmonella biofilm formation. In its unphosphorylated active state, CsgD promotes the expression of adrA, a potent diguanylate cyclase associated with promoting cellulose biosynthesis. CsgD is additionally responsible for activating the transcription of genes and operons associated with the biosynthesis of curli fimbriae, O-antigen capsule, and BapA protein. Genes and operons are shown as open arrows, proteins as ovals, and c-di-GMP molecules as dark blue circles. Positive regulation is denoted as green arrows, while regulatory inhibition is shown as flat-headed red arrows. Activation via c-di-GMP molecules is shown as blue arrows. (B) Multiple environmental signals can induce the biosynthesis of biofilm polymers. However, some conditions can activate c-di-GMP production and cellulose biosynthesis independently from other biofilm polymers. Under biofilm-inducing conditions, only a subset of Salmonella cells in the total population will synthesize high levels of CsgD. This subpopulation enters a CsgD-ON state, which results in significant c-di-GMP production and biosynthesis of biofilm matrix polymers. Cells within the biofilm are able to survive and persist in harsh environmental conditions. In contrast, some Salmonella cells in the population do not have sufficient synthesis of CsgD, resulting in a CsgD-OFF state and subsequently low intracellular concentrations of c-di-GMP. These cells remain in a planktonic state, are highly motile, and synthesize the type-three secretion system (T3SS)-1, resulting in a virulent cell subpopulation. Due to the CsgD-independent activity of some diguanylate cyclases, Salmonella cells can have high intracellular levels of c-di-GMP while in a CsgD-OFF state. As such, these cells may synthesize cellulose in the absence of other major biofilm matrix polymers. The relatively low expression/activity of virulence-associated factors in cells aggregated together within cellulose or other biofilm polymers is due at least in part to the state of c-di-GMP pools within the cells.
S. enterica serovar Typhi has been shown to form unique biofilms on the surface of gallstones (55, 56). These biofilm-coated gallstones have been observed in association with chronic human Typhi carriers (57) and the presence of bile is a key inducing factor (56). Curli fimbriae are not involved in the formation of these biofilms, although the O-antigen capsule and flagella have been implicated (58, 59). These biofilms are distinct from the rdar morphotype and are specific to serovar Typhi; they are likely to have a unique function in the Salmonella lifecycle and as such, are not discussed extensively in this review.
In the early days of genome-wide comparisons in Salmonella, researchers had identified multiple different fimbrial types, each with scattered distribution between the different S. enterica serovars (60). For the current picture of fimbrial distribution, see Ref. (61, 62). Due to pioneering work with Type I and P fimbriae in E. coli (63, 64), it was predicted that the presence of different fimbrial types would allow Salmonella cells to attach to the intestinal epithelium of specific host species (65). One example was the association of SEF14 fimbriae with poultry-associated S. enterica serovars (60, 66). Curli fimbriae were unique in that they were conserved in the Salmonella genus (60); the csgA gene coding for the major curli subunit (formerly agfA) was developed as an early Salmonella diagnostic, detectable in 603 of 604 tested strains (67). Curli fimbriae and the corresponding biosynthetic operons were also detected in E. coli (60, 68, 69), which had a last common ancestor with Salmonella approximately 100 million years ago (70). Hammar et al. identified the presence of two polycistronic csg operons responsible for curli biosynthesis in E. coli (71), which were later identified in Salmonella (72) and subsequently shown to be interchangeable cross-species (69). These findings brought up interesting questions about curli fimbriae and why they would be conserved in Salmonella strains that were capable of colonizing so many different host species. It indicated that curli fimbriae were involved in a common aspect of the Salmonella lifecycle that was shared by many diverse strains.
One clue about curli function came as a result of the unusual protocol for curli purification. After the majority of S. enterica serovar Enteritidis cellular material was solubilized or had been removed by enzymatic digestion, purified curli were isolated from the top of a preparative SDS-PAGE, as the material that did not enter the gel (32). Purified curli fibers remained intact after boiling in the presence of SDS, exposure to sodium hydroxide, or digestion with proteinase K, treatments that would depolymerize or degrade most if not all other fimbrial types (32). Resuspension in >70% formic acid was the only treatment found to depolymerize the curli fibers into their structural subunits (32, 73); it should be noted that no improved alternative to this procedure has been published in the last 25 years. We know now that curli fimbriae are a functional amyloid with extensive cross-β structure (74, 75), hence their extreme stability. It was apparent early on that curli fimbriae could provide physical stability and possible resistance for aggregated Salmonella cells.
Another indication of the potential function of curli fibers came from the optimal growth conditions for their production in vitro. Curli fimbriae were originally discovered within a strain of E. coli isolated from cattle manure (76). The fimbriae originally discovered in S. enterica serovar Enteritidis by Collinson et al. were thought to be distinct from curli fimbriae in E. coli due to differences in amino acid residues and due to constitutive expression in serovar Enteritidis at 37°C and 28°C (32). However, the amino acid differences were based on the false identification of the curli subunit by Olsén et al. (76), who had in fact identified Crl, a biofilm transcriptional regulatory protein (named Crl for curli). The constitutive production of curli in serovar Enteritidis was found to be due to a single nucleotide polymorphism (SNP) in the promoter region that changed the transcriptional regulation (33). Most strains of Salmonella, it has since been found, only produce curli fimbriae at temperatures below 32°C (76). In addition, curli production requires low osmolarity and appears to be triggered by nutrient limitation (32, 33, 77). One could envision that similar conditions might naturally exist in non-host environments. Hence, researchers started to think more about curli production and Salmonella aggregation in the context of the environment.
Anriany et al. (78) first analyzed the survival properties of rdar colonies formed by Salmonella serovar Typhimurium (78), due to the apparent similarities to the “rugose” colony morphology in V. cholerae, which had previously been shown to enhance Vibrio survival (79). Cells in rdar colonies had enhanced survival upon exposure to hydrogen peroxide and to acidic pH, as compared to non-rdar colonies. Cellulose was discovered as the second major component of the Salmonella biofilm extracellular matrix (42, 80); it is also extremely resistant and forms extensively hydrogen-bonded sheets (35). Solano et al. (42) treated rdar colonies with sodium hypochlorite, which is used as a common waterline disinfectant, and showed that the presence of cellulose provided protection to the cells. Other researchers extended these results to include pellicle biofilms formed at the air–liquid interface in liquid cultures (81). Scher et al. also tested heat and acidity but the pellicle cells were not significantly more resistant than stationary-phase cells. We performed a series of survival experiments, taking advantage of the unique properties of rdar colonies, specifically that they can be lifted off the agar surface in one piece (33). We started placing intact colonies on plastic surfaces and allowing them to dry out before periodically inoculating pieces of these colonies into fresh liquid media. Within 1–2 months, we realized that not only were cells staying viable in this dried out state, but also there was no apparent lag-time when placed into fresh media. Therefore, we performed an experiment comparing survival of rdar colonies to colonies formed by Salmonella mutant strains without curli, without cellulose, or without the entire extracellular matrix (ΔcsgD) (37). After 3 months, the rdar colonies displayed 3–10 times enhanced survival compared to the biofilm mutants. After 9 months, the difference was as high as 30 times increased survival and exposure of the dried colonies to sodium hypochlorite yielded an even bigger survival difference. At the time that these desiccation experiments were performed, collaborators had discovered a new polysaccharide capsule in Salmonella that was part of the biofilm extracellular matrix (38). Gibson et al. performed desiccation experiments using a lyophilizer, and proved that the O-antigen capsule was the major factor providing desiccation resistance to cells. This reinforced the role of polysaccharides in the biofilm matrix to maximize water retention, nutrient trapping, and provide buffering (82).
As a final test of the potential importance of the biofilm extracellular matrix in the survival of Salmonella cells, we examined the viability of dried out rdar morphotype colonies after 2.5 years (83). The recovery of these cells was problematic in that they did not grow well on a variety of selective media commonly used for Salmonella isolation, such as SS or XLD agar. On non-selective media, the recovery rate after 30 months was ~5% of the starting number of cells; however, when evaluating viability using a live-dead cell stain, over 50% of cells appeared to be alive (84). The discrepancy in measured cell number between plating and live-dead staining suggested that cells might be in a type of viable, non-culturable (VBNC) state (85, 86). The existence of a VBNC state would add to the difficulty in eradicating Salmonella in agricultural or food-processing settings. Perhaps most importantly, cells in 2.5-year old rdar colonies retained an ability to cause infections in the mouse model of infection (83). There are many differing theories about how Salmonella can persist in industrial and/or agricultural settings, including survival in the local rodent or insect populations (87–89). The survival results for rdar colonies indicate that S. enterica strains could survive on their own in dryness or without exogenous nutrients for a long period of time.
In agricultural and industrial settings, biofilm formation has long been considered a factor to explain the extreme persistence of Salmonella. Outbreaks of human gastroenteritis have been linked to the consumption of a wide variety of foods or food products, not just fresh foods that may come into contact with contaminated water sources but also processed foods that go through extremes of dryness (84). Often researchers have analyzed the isolates/strains that are associated with outbreaks or with agricultural/industrial persistence to check their biofilm-forming ability, which has tended to be overwhelmingly positive (90–92). However, as yet, there has been no positive confirmation that the rdar morphotype is “the” critical factor. We performed a project examining Salmonella colonization of egg-conveyer belts used in modern poultry barns, because one farm had their flock re-infected by the same strain of S. enterica serovar Enteritidis over a 3-year period and the conveyor belt was identified as the source of contamination. Isolates taken from each year all formed rdar colonies, the rdar colonies were resistant to treatment with common disinfectants used in the industry, and all isolates formed robust biofilms on pieces of egg belt (93). However, the presence of rdar biofilm had no measurable effect on survival when contaminated pieces of egg belt were treated with disinfectant. We concluded that it is difficult to recreate real-world situations in an in vitro setting.
Several studies have provided convincing evidence to implicate biofilm formation as an important factor in the interaction between Salmonella and plants [reviewed in Ref. (94)]. Curli fimbriae, cellulose, and O-antigen capsule are involved in different stages of plant colonization and Salmonella persistence within or on plant tissue (95–97). In their laboratory study, Lapidot and Yaron reported the observation of multicellular aggregates beneath the surface of parsley leaves grown in soil irrigated with contaminated water (98). Perhaps, the closest real-world evidence of enhanced biofilm formation having a biological impact was with the recent E. coli outbreak in Germany in 2011. The O104:H4 strain that was associated with contaminated fenugreek seeds caused the highest rates of hemolytic–uremic syndrome ever recorded and displayed clinical evidence of increased biofilm formation (99). Analysis of this strain showed that it had acquired a unique gene that led to enhanced biofilm formation (100). It is possible that biofilms were involved in the initial attachment to the fenugreek seeds, as previously demonstrated for alfalfa seeds (101), which would have facilitated both the dissemination and persistence of the outbreak strain.
Collinson et al. first proposed that aggregation could provide Salmonella with a mechanism for surviving the harsh conditions of the host intestinal tract to ensure that a “viable and sufficient” inoculum could reach the epithelial layer (32). This seemed a valid hypothesis because although the dogma was that a high infectious dose was required for Salmonella infections, there were multiple epidemiological trace-backs to outbreaks that seemingly had a low inoculum (102). One could envision small aggregates being taken up by a host and the presence of resistant extracellular matrix polymers shielding cells during passage through the stomach. This would lower the infectious dose required to cause infections. The biofilm flask cultures described (Figure 1), provided an opportunity to test this hypothesis. Cultures of wild-type S. enterica serovar Typhimurium were competed in mouse infections with an isogenic, curli-negative mutant that was unable to aggregate. To our surprise, the non-aggregated mutant strain consistently outcompeted the wild-type strain (45). In our more recent studies, planktonic and aggregate cell types were isolated from the biofilm flask cultures, and competed during co-infections of mice. Again, the non-aggregated cells consistently outcompeted the aggregates (103). There is other recently published evidence that the presence of extracellular matrix factors reduce Salmonella virulence in the mouse model of infection (104). The results from these studies have established that the formation of rdar biofilms is not a virulence adaptation.
The results above created a conundrum because curli fimbriae have been well established as potent inducers of the innate immune system. Curli represent a pathogen-associated molecular pattern (PAMP) that causes activation of toll-like receptors 1 and 2 (105, 106), as well as intracellular NOD-like receptors (107). There is also evidence that curli can bind to multiple host proteins, such as contact-phase proteins or extracellular matrix proteins fibronectin and laminin [reviewed in Ref. (34)]. Although this research would seem to indicate that curli are produced in vivo, studies have yet to show that CsgD and curli are produced during infection. Deletion of csgBA (formerly agfBA), encoding the main curli subunit proteins, caused no noticeable impairment of Salmonella virulence (108). We monitored the expression of a curli reporter fusion in vivo using a whole animal imager, but we did not detect expression in any of the 13 mice that were screened (45). We hypothesized that the host immune system does interact with Salmonella rdar biofilms during an infection, perhaps immediately after ingestion of Salmonella biofilm cells.
Cellulose, the other main structural component of rdar biofilms, may have an important role in host–pathogen interactions during Salmonella infection. In contrast to curli biosynthesis, which is regulated at the transcriptional level by CsgD (69, 72), the bcs operons encoding the cellulose biosynthesis enzymes are transcriptionally independent of CsgD and the rdar morphotype (80). Activation of cellulose production is largely dependent on allosteric activation of the BcsA subunit by c-di-GMP (51). CsgD is responsible for inducing the expression of adrA, the first diguanylate cyclase enzyme to be associated with cellulose biosynthesis in Salmonella (36, 50). The transcriptional uncoupling of cellulose biosynthesis from CsgD regulation thus provides an opportunity for cellulose production to occur independently of the biofilm phenotype. As stated above, the Salmonella genome contains multiple diguanylate cyclase genes. The evidence for a CsgD-independent pathway for cellulose production was first provided by Ref. (42) and has since been demonstrated in E. coli (109). In a pair of subsequent studies, Lasa and colleagues performed systematic deletion and re-integration of the genes for each c-di-GMP synthase enzyme, resulting in the identification of four c-di-GMP synthesizing enzymes that can each independently induce Salmonella biofilm formation at 37°C (44, 110) (Figure 3A).
Within the host, there are potentially multiple cues that can induce c-di-GMP-based activation of cellulose biosynthesis. Co-incubation of Salmonella with Sal4, a protective IgA antibody secreted into the intestinal lumen, has been shown to increase c-di-GMP levels and cellulose production through activation of YeaJ (STM1283) (111), one of the enzymes identified by Lasa and colleagues. Cellulose has also proven important for attachment of E. coli and Salmonella to the surface of intestinal cells, although the exact c-di-GMP synthase enzymes responsible for this process are still unknown (112, 113). Further, Salmonella have been shown to produce measurable amounts of cyclic-di-GMP and cellulose while inside macrophages in response to low intracellular concentrations of magnesium and to changes in intracellular ATP levels (114). These authors discovered the production of cellulose in vivo by studying a mgtC mutant strain. mgtC is a key virulence gene that is expressed inside macrophages; deletion of mgtC caused attenuation of virulence and an increase in cellulose production. However, the mgtC mutant strain was no longer attenuated if the accumulation of cellulose was prevented, suggesting that cellulose was impeding virulence. Most surprisingly, these authors found that a Salmonella cellulose synthase mutant was hypervirulent, and killed mice faster than the wild-type strain (114). Ahmad et al. also recently published evidence that cellulose impedes Salmonella virulence; synthesis of BcsZ was necessary to reduce cellulose production in vivo and to maintain virulence (115). Mills et al. (116) identified l-arginine as another potential signal that is present inside the macrophage and is able to induce c-di-GMP biosynthesis and cellulose production. Finally, previous work out of the Romling lab demonstrated that cellulose production inhibits host cell invasion (117, 118). Altogether, the evidence indicates that Salmonella cellulose production plays a key role in host–pathogen interactions and that the interactions may be much more complex than initial experiments demonstrating that cellulose production was not essential for Salmonella virulence (42).
Since the discovery of curli fimbriae and description of the rdar morphotype, researchers have examined the conservation of this phenotype within the Salmonella genus. These data are summarized in Table 1. In general, the rdar morphotype is conserved in a majority of non-typhoidal S. enterica serovars, such as Enteritidis and Typhimurium, in strains that normally would cause gastroenteritis. We proved that csgD (curli) promoter function was conserved in a diverse collection of strains, representing S. bongori and all six S. enterica subspecies (119). However, many of the strains had lost biofilm formation due to trans changes in the rdar regulatory network likely caused by domestication of the strains (46, 120). This provided a cautionary note that these kinds of extracellular phenotypes can be easily lost during laboratory passage (120).
Römling and colleagues first identified a connection between increased host adaptation in Salmonella strains and an inability to form biofilms (121). One particular variant of serovar Typhimurium (i.e., var. Copenhagen) that causes a systemic disease in pigeons (129) had lost the ability to form the rdar morphotype. Their analysis was expanded to include other host-adapted serovars, such as Cholerasuis (pigs), Gallinarum (chickens), and host-restricted serovar Typhi (humans), which were almost entirely rdar negative (Table 1). We reported that two Typhi strains were rdar positive (37); however, this was a mistake caused by the presence of a contaminating rdar-positive isolate within the stock cultures. We subsequently tested >200 Typhi isolates and all were rdar negative (45). We also identified S. enterica subspecies arizonae (IIIa) strains as being rdar negative due to inactivating SNPs in the csgD (curli) promoters (46). Subspecies arizonae isolates rarely cause human infections, but are frequently isolated from the gut of reptiles and snakes, and therefore, may be part of the commensal microflora (130). In S. Typhimurium var. Copenhagen isolates, the prevalent mutation was a G to T transversion in the −35 region in the csgD promoter, which may partially explain the loss of the biofilm phenotype (121). For S. Typhi, the intergenic region between the divergent curli operons has conserved sequence, but multiple mutations exist within the curli (csg) and cellulose (bcs) biosynthesis operons, where preliminary stop codons within csgD and bcsC genes may eliminate the possibility for synthesis of curli fimbriae and cellulose altogether (121, 131). We speculated that sequence mutations or cis changes in the csg genes/promoters are indicative of a change in the lifestyle of Salmonella isolates (46). Each of the serovars above that are lacking rdar biofilm formation have evidence of a restricted host range.
The most recent screening efforts have focused on iNTS strains from sub-Saharan Africa. With a few exceptions, it appears that biofilm formation is impaired in these strains (Table 1). Ramachandran et al. (125) performed colony desiccation and sodium hypochlorite experiments with serovar Typhimurium ST313 isolates and demonstrated that they were also impaired for survival. This preliminary evidence suggests that iNTS isolates may have undergone an evolutionary change in lifestyle when compared to their gastroenteritis-causing NTS counterparts.
Central to Salmonella pathogenesis is its ability to modify host cell biology via two type-three secretion systems (T3SS), T3SS-1 and T3SS-2 (132). These specialized organelles span the bacterial inner and outer membranes and allow for the delivery of effector proteins into the cytoplasm of eukaryotic cells (133, 134). Type-three secretion systems are found exclusively in Gram-negative bacteria (135, 136). For Salmonella, genes for the T3SS-1 or T3SS-2 apparatus, regulatory components, and nearly all associated effector proteins are found within horizontally acquired DNA regions known as Salmonella Pathogenicity Islands (SPIs) (134). The SPI-1 region contains genes associated with T3SS-1, is found in all serovars of S. enterica and S. bongori, and is important for the invasion of intestinal epithelial cells (137, 138). In contrast, the full-length SPI-2 region harboring genes for T3SS-2 is present exclusively in S. enterica, and is associated with the intracellular survival of Salmonella within eukaryotic cells (137).
Non-typhoidal Salmonella infections in immunocompetent individuals can be generalized into three important steps: invasion, inflammation, and intestinal replication. While decades of literature have been dedicated to understanding invasion and inflammation, recent research has uncovered important aspects of Salmonella replication and transmission in the inflamed intestine. In this section, we evaluate what is known about these important steps in gastroenteritis associated with NTS infections and consider how biofilm biology and Salmonella transmission may relate to our current understanding of Salmonella pathogenesis.
Following Salmonella entry into the host via contaminated food or water, cells travel through the digestive system and localize to the distal ileum and colon of the GI tract (139). Salmonella cells rely on flagellar motility and chemotaxis systems to traverse the intestinal mucus layer and identify sites on the host cell that are permissive for invasion (140). Fimbriae and other protein adhesins on the bacterial cell surface initiate association of the pathogen to the targeted epithelial cell; the needle complex of the T3SS-1 is critical for stabilizing this interaction. It is currently hypothesized that expression and synthesis of the T3SS-1 is induced by several important cues provided by the local host environment, including low-oxygen tension, high osmolarity, near-neutral pH, and acetate production levels from the resident microflora (141). The secretion apparatus is established in a step-wise manner, requiring formation of the basal body within the bacterial cell membranes to facilitate secretion of the needle complex (142). Proteins attached to the end of the T3SS-1 needle, collectively referred to as the translocon, are then inserted into the host cell membrane, creating a pore that allows for the injection of Salmonella effector proteins into the host cell (143). Establishment of a secretion-competent T3SS-1 is a mandatory prerequisite for the process of host cell invasion [reviewed in Ref. (144)]. Pathogen cells that traverse the epithelial cell layer encounter tissue mononuclear cells (i.e., macrophages and dendritic cells) within the lamina propria, resulting in the uptake of the pathogen into a phagosome (139). Salmonella depend on the T3SS-2 and associated effectors to manipulate the phagosome environment and promote pathogen survival and replication within the Salmonella-containing vacuole (SCV). The effectors are responsible for re-modeling the SCV environment and are hypothesized to provide a potential source of nutrients during pathogen replication (144).
Detection of PAMPs and components injected by the pathogen during its uptake into host cells elicits the production of a proinflammatory immune response (139). This response inhibits the spread of NTS past the lamina propria in three ways: (1) by activating infected macrophages and inducing killing of intracellular Salmonella, (2) through recruitment of neutrophils to the infection site for extracellular killing, and (3) by stimulating epithelial cells to release antimicrobial peptides into the intestinal lumen to control the replication of NTS cells (139). While host inflammation effectively controls the NTS cells that have invaded the epithelial cell layer, it acts as a potent stimulator of growth of the NTS population in the intestinal lumen (139). Recent studies have shown two mechanisms by which NTS are able to exploit the host inflammatory response. Epithelial cells release the antimicrobial agent lipocalin-2, a molecule that binds to enterochelin, an iron chelation molecule used by Gram-negative bacteria in the gut to acquire iron from the host (145). In addition to enterochelin, NTS are able to produce a second iron chelation molecule, salmochelin, which cannot be bound by lipocalin-2 (145). As a result, NTS cells in the intestinal lumen continue to replicate while the local microbiota are starved for iron. The low-oxygen conditions of the intestinal lumen promote the establishment of an anaerobic microbiota that use fermentation to derive energy from available amino acids and complex polysaccharide (139). Hydrogen sulfide is produced as a byproduct of this fermentation, which is immediately converted to thiosulfate by the epithelial cell layer of the colon (146). During inflammation, neutrophils infiltrate the intestinal lumen and release reactive oxygen species molecules as part of the mechanism for the extracellular killing of bacterial pathogens (146). The association of reactive oxygen species with thiosulfate molecules results in tetrathionate, which can be used by NTS as an electron acceptor during anaerobic respiration. In addition to activating a metabolic response that promotes growth of the pathogen, anaerobic respiration further allows NTS to utilize carbon sources that would otherwise metabolize poorly during aerobic fermentation (147). Altogether, NTS can use these mechanisms to promote their own growth at the expense of the existing host microbiota.
Two features that distinguish enteric or typhoid fever from gastroenteritis are the relative absence of inflammation and an innate immune response, and the replication of typhoidal Salmonella in the systemic compartment of the host. These changes in pathogenesis are linked to genomic differences between typhoidal and NTS. Approximately 200 functional genes in NTS have been inactivated or functionally disrupted in S. Typhi and S. Paratyphi A (148). Many of the mutations in S. Typhi affect processes used by NTS to induce intestinal inflammation, including motility and chemotaxis, adherence to and invasion of host cells, and loss of virulence factors associated with intracellular replication (148). The loss of these functions suggest that S. Typhi may gain access to the systemic compartment through a mechanism distinct from active invasion of intestinal epithelial cells. Although this mechanism remains elusive, it is hypothesized that microfold (M) cells that sample the intestinal lumen actively take in S. Typhi cells and transfer them to macrophages and dendritic cells within the gut-associated lymphoid tissue of the Peyer’s patches, located in the small intestine (16). Typhoidal Salmonella are unique/distinct from non-typhoidal serovars in that they are able to persist in this intracellular niche without activating the immune response and infiltration of neutrophils that would otherwise restrict typhoidal infections (149). Within these immune cells, typhoidal Salmonella are shuttled to other sites in the body associated with the mononuclear phagocyte system (previously known as the reticuloendothelial system), taking residence in such places as the liver, spleen, mesenteric lymph nodes, bone marrow, as well as the gall bladder (17, 20). Typhoidal Salmonella are thought to transfer back into the duodenum via the biliary tract, resulting in intermittent shedding of typhoidal cells in the feces (17).
The lack of inflammation associated with typhoidal Salmonella infections suggests important differences in the pathogen’s surface antigens or its interactions with host cells. For example, the potential downregulation in serovar Typhi flagellar expression results in decreased inflammation (150). Other gene mutations identified in S. Typhi include regulatory elements affecting the O-antigen structure, which may limit exposure of this important PAMP to immune cells (151). Further, the typhoidal Salmonella genome also possesses 300 to 400 unique genes that are absent in NTS. Of these additional accessory genes, the Vi capsule plays an important role in reducing the host inflammatory response to the presence of S. Typhi cells. Production of the Vi capsule limits complement deposition on the surface of S. Typhi cells, masks surface antigens that would normally activate the host immune response, and provides resistance to phagocytic killing (16, 17, 152). Further, the Vi capsule has also been demonstrated to induce production of the cytokine interleukin 10, an important anti-inflammatory molecule (153). Vi-negative mutants of S. Typhi were unable to cause enteric fever in human infection trials (154). However, the Vi capsule cannot solely account for differences between typhoidal and NTS infections, as this capsule is not expressed by other typhoidal serovars (i.e., S. Paratyphi A) (149). It is likely that serovar-specific combinations of gene acquisition and gene loss are responsible for the ability of typhoidal Salmonella strains to evade the host immune response. There are several other reasons for the host-restriction of serovar Typhi strains, as recently discovered by Spanò et al. (155). Further study of these factors will provide insight for understanding how Salmonella serovars and strains progress from host-generalists to host-adapted and finally to becoming host restricted.
Despite their differences in host range, non-typhoidal and typhoidal Salmonella serovars maintain a genetic relatedness at the species level (149). Therefore, Salmonella pathogens present an opportunity to study the biological factors that are important for transmission (156).
Nearly all NTS serovars associated with human disease demonstrate the ability to colonize multiple host species and induce gastroenteritis. In North America, NTS infections are often associated with the ingestion of contaminated food or water, but can also be transmitted directly from zoonotic sources, such as domestic or food animals, through the fecal–oral route (84). Outbreaks of NTS have been associated with a wide range of food products, including animal-based (meat, poultry, eggs), plant-based (tomatoes, sprouts, melons, lettuce, mangoes, raw almonds) and processed foods (powdered infant formula, dry seasonings, cereals, peanut butter) (84). However, a number of NTS outbreaks in developed countries emphasize the importance of environmental reservoirs as an intermediary step for transmission of this pathogen. Several reported cases exemplify the ability of NTS to persist in the non-host environment. Surface runoff contaminated with animal feces was suspected as the source of two separate cases in 2008 of drinking water contamination affecting communities in the United States (157, 158). Similarly, a study analyzing 288 cases of drinking water-related outbreaks in Canada between the years 1971 and 2001 noted water treatment practices and nearby wildlife as the most frequently reported sources of contamination (159). Several reports of NTS outbreaks associated with fresh produce have traced contamination to irrigation water or animal manure used to fertilize fields (160–162). Of particular interest are two separate outbreaks of NTS infections in the United States in 2002 and 2005, both of which were linked to a rare strain of S. Newport in tomatoes (162). In both outbreaks, investigators were able to trace back the unique strain to a Virginian farm, where the strain had been isolated from a contaminated pond used to irrigate the fields (162). The identification of this same rare strain in pond water samples taken years apart indicates the added importance of Salmonella persistence in environmental reservoirs. A similar case of non-typhoidal S. Typhimurium persistence was observed for a Danish pig farm associated with recurring infections in its herd (163). Samples collected by Baloda and colleagues over a 2-year period revealed the presence of the same Salmonella clone in the piggery, the feed provided to the animals, and in the pig manure used to fertilize agricultural soil (163). Farmland soil treated with manure yielded viable S. Typhimurium cells for 14 days following spread, providing further evidence for the survival of NTS within the non-host environment. The authors hypothesized that the persistence of Salmonella in this setting could result in a cycle of re-infection of the pig herd from environmental reservoirs, potentially explaining the long-term presence of NTS at the farm. While it may be logical to infer a role for biofilm formation in such cases of Salmonella persistence, it will be important for future research endeavors to include efforts to characterize the physiological state of Salmonella cells in situ.
For typhoidal Salmonella, chronic persistence within their current host increases the opportunities for subsequent transmission events. Between 5 and 10% of patients recovering from enteric fever experience a relapse in infection with the same typhoidal Salmonella strain, resulting in milder symptoms than before and fecal shedding of the pathogen for 3 weeks to 3 months following the initial infection (17). While the mechanism behind this short-term persistence in the host is unclear, it is hypothesized that typhoidal Salmonella can remain dormant within immature immune cells in the bone marrow (16). Between 2 and 4% of people living in areas endemic for enteric fever are associated with a chronic carrier state that involves asymptomatic carriage of typhoidal Salmonella for more than a year (16). Epidemiological studies hoping to identify factors associated with the chronic carrier state are difficult due to the asymptomatic nature of infections in these hosts (17). However, recent evidence points to persistence of typhoidal Salmonella within the gall bladder. It is currently hypothesized that typhoidal Salmonella cells first localize to the liver and replicate in the resident macrophage (Kupffer) cells before traveling to the gall bladder via the biliary tract (17). Bile, a digestive secretion with detergent and antimicrobial properties, contributes to the sterility of the gall bladder. In a landmark study assessing the incidence of gall bladder disease in patients associated with acute or chronic infections with typhoidal Salmonella, the authors detected gallstones in nearly 90% of chronically infected patients (164). Microscopic analysis of this interaction suggested that typhoidal Salmonella cells use fimbrial protein structures on their surface to attach to gallstones, while growth in the presence of bile stimulates the production of protective extracellular polysaccharides (59). It is currently hypothesized that short-term human carriers are mainly responsible for the transmission of enteric fever in endemic areas, while long-term chronic carriers are responsible for resurgence of infections in endemic regions despite attempts to control such infections (17). Blaser and Kischner proposed that Typhi carriers would have been necessary in hunter gatherer societies to allow the disease to spread to others even after everyone in the local family group had been infected and had acquired immunity (165). Typhoidal Salmonella serovars are transmitted primarily from person to person through food and water contaminated with human feces; as such, infections are more frequent in low-income countries that lack available safe water resources and have poor sanitation standards (166). Cases of typhoidal infections in high-income countries are usually the result of patients traveling to endemic areas, but can also be spread by individuals that are chronically infected with S. Typhi (166).
Like typhoidal serovars, strains of iNTS induce a fever-like illness and persist within the systemic compartment of infected individuals (19). As of today, humans are the only identified reservoir for invasive strains of NTS (167, 168). Thus, the speculation is that these strains are transmitted similarly to Typhi, with the human carrier being the main source of new infections. iNTS infections occur predominately in children between 6 and 18 months of age and in adults between 25 and 40 years old (166). For children, the predominant host risk factors are HIV infection, malnutrition, and malaria, while advanced HIV infection is the main risk factor in adults (19). Further, cases of iNTS disease in children and adults are strongly correlated with the rainy season in sub-Saharan Africa, which could be the result of waterborne transmission, malaria, or malnutrition during this season (20, 166).
Our current understanding of Salmonella biofilm formation has been primarily shaped by its characterization in the laboratory. Most early comparisons between biofilm-positive and biofilm-negative isolates were done at a population level. While this yielded valuable information, it was hard to envision how these biofilm phenotypes would manifest in nature. For example, Serra et al. showed that there was massive cell heterogeneity within rdar colonies (40), therefore, ascribing functions to the rdar colony as a whole may not be biologically accurate. Furthermore, this density of cells (i.e., 1010–1011 cells per colony) would not be sticking together in the environment in one piece, except under very special circumstances, such as a wastewater treatment plant (169), but even in those conditions there are many different species involved so the dynamics would be different. This led us (and others) to the question: “How does the rdar morphotype appear in nature?” To simulate the environment, we attempted to induce S. enterica serovar Typhimurium aggregation at low cell densities in liquid cultures (45). Under these conditions, the population of cells in liquid culture differentiated into two forms: multicellular aggregates and planktonic cells. We showed that the aggregates produced curli and cellulose polymers and had many of the properties of a rdar biofilm (45). We know now that the biofilm cells and single cells arise due to bistable production of CsgD (i.e., biofilm cells are CsgD-ON; single cells are CsgD-OFF) (170) (Figure 3B).
In a large-scale RNA-seq experiment, we compared the gene expression profile of Salmonella multicellular aggregates and planktonic cells (103). Although they are formed under the same growth conditions, these clonal cell types had differential expression of over 1,856 genes, which represents approximately 35% of all genes in the serovar Typhimurium genome. Previous work had identified genes corresponding to carbon central metabolism and the general stress response being expressed during biofilm formation (54). Transcriptome analysis expanded this by identifying increased expression of genes important for the metabolism of amino acids, lipids, and nucleotides. We demonstrated that biofilm cells were more resistant to desiccation and antibiotics than the planktonic cells. The transcriptome of planktonic cells was vastly different from multicellular aggregates, with significant expression of multiple virulence traits, including the T3SS-1. In the literature, it was thought that expression of the T3SS-1 was exclusively induced by in vivo conditions inside the host, which is an important first step in Salmonella pathogenesis (133). As such, expression of T3SS-1 in biofilm-inducing conditions was highly unexpected and required rigorous functional validation. We confirmed that the proteins for the secretion apparatus and its effectors were synthesized under these atypical environmental conditions. The increased abundance of T3SS-1 provided a virulence advantage for planktonic cells compared to multicellular aggregates both for invasion of a human intestinal cell line in vitro and during competitive infections in mice. Determining how the T3SS-1 is induced in the planktonic cell subpopulation remains an important question for us. The relative absence of SPI-1 expression in multicellular aggregates may also provide an important clue for the molecular link between the persistence and virulence phenotypes. While it is tempting to speculate that there is a direct link between CsgD and SPI-1 expression, as implied by other studies (171), such a relationship has not yet been established. It is plausible that the presence of the extracellular matrix may provide an important feedback signal that ultimately inhibits the expression of virulence factors such as the T3SS-1. Regulation between SPI-1 and biofilm formation may also be indirect in nature. Desai and colleagues recently demonstrated that SsrB, a transcriptional regulator encoded within SPI-2, can switch between promoting expression of the T3SS-2 within the acidic macrophage vacuole and relieving H-NS silencing of csgD expression (172). Establishing the genetic link between persistence and virulence is an important direction for the future of Salmonella biofilm research.
As described in Section “Correlation between Biofilm Formation and Host Specificity,” biofilm formation is highly conserved in Salmonella strains associated with gastroenteritis, but is lost in Salmonella strains that are responsible for invasive disease or are adapted to life in a particular host. Romling and colleagues were the first to discuss this correlation in the context of Salmonella biology (121). They suggested that loss of biofilm formation in invasive strains/serovars/species was a pathoadaptive trait, presumably to improve the fitness of the pathogen so that it is able to survive better in host tissues (173). Part of the reasoning behind this was the knowledge that Shigella and enteroinvasive E. coli, two pathogens which breach the intestinal epithelium (174), have lost the rdar morphotype due to multiple insertions and deletions in the curli biosynthesis operons, indicating strong selection pressure against this phenotype (175). In contrast, E. coli strains that were commensal inhabitants of the GI tract appeared to retain an ability to express the rdar morphotype (112). We reported the same trend in E. coli as in Salmonella; among 284 E. coli isolates from diverse host species, we observed that host-generalist isolates were 84% rdar-positive, whereas isolates that had the largest genetic differences and were the most likely to be host-adapted were less than 50% rdar-positive (176). The 115 human isolates that we screened were only 36% rdar-positive versus 169 isolates from different animal hosts that ranged from 59 to 93% rdar-positive. We reasoned that human commensal E. coli were more host-adapted and would have less reliance on transmission via the environment as compared to transient strains that cause extra-intestinal infections and are only in the host for a short time. However, reduced rdar prevalence in our study was also correlated with an increase in the presence of virulence genes, meaning that the dynamics of E. coli colonization are complex (176, 177). The possibility of a pathoadaptive trait conserved between species indicates that similar selection pressures are acting on Salmonella and E. coli. Römling et al. focused on the interactions with the intestinal epithelium, arguing that when a pathogen crosses this barrier the dominant selection pressure comes from the host immune system (121). While the immune system undoubtedly plays an important role, there are also strong pressures on pathogens to maintain their transmission cycles (156). Therefore, loss or impairment of the rdar morphotype could also reflect a change in strain transmission patterns (125). At present, we do not know which aspect of the Salmonella lifestyle has the greatest evolutionary influence on the relationship between host adaptation and ability to produce rdar biofilms.
Bistable CsgD expression and analysis of the aggregate and planktonic cell subpopulations has shifted our perception of Salmonella biofilm formation and the process of pathogen transmission. What once was considered a population-level phenotype is now understood as a regulatory phenomenon mediated at the single cell level (170). What is the purpose of this phenotype switching in the life cycle of Salmonella? Is there an advantage for Salmonella to express virulence factors in a non-host setting? Compared to the host niche, where the conditions of host–pathogen interactions are relatively defined, life in non-host environments and the process of transmission are unpredictable. In bacteria, bistable genetic networks are often associated with the formation of two distinguishable phenotypes within a clonal population (178), which is thought to allow genotypes to persist in fluctuating environments (179). Based on this theory and our characterization of the planktonic and aggregated cell subpopulations, we hypothesize that “phenotype switching” improves the overall chances for Salmonella transmission. In a scenario where Salmonella immediately encounters its next host, planktonic cells would be able to instigate a new infection. In contrast, if a host were not encountered, Salmonella biofilm cells would be prepared to survive in non-host environments for a long time until an opportunity for infection arises. This unpredictable step in the Salmonella life cycle places equal selection pressure on virulence and persistence phenotypes.
Since non-host environments have unpredictable conditions, it would be a poor evolutionary choice for Salmonella cells to adapt once they arrive there. Rather, Salmonella likely requires anticipatory genetic regulation where cells pre-emptively express the phenotype that is necessary for the next step in their life cycle (180). If bistable CsgD expression is necessary for cells to prepare for transmission, conditions within the GI tract may provide important cues to induce this differentiation. In the GI niche, Salmonella cells are exposed to host temperatures, low pH, high osmolarity, bile acids, antimicrobial peptides, iron limitation, and in some cases, nutrient limitation (141, 181). Some of these conditions (i.e., temperature and osmolarity) may favor the expression of virulence factors such as the T3SS-1 (141), while repressing Salmonella biofilm formation (33, 49). Conversely, exposure to stresses such as bile, antimicrobial peptides, iron limitation, or poor nutrient availability would promote csgD expression and the biofilm phenotype. Regulation of csgD expression and synthesis is incredibly complex, and few studies have attempted to understand the hierarchy of this regulation (33, 49, 77, 182). For example, iron limitation has been shown to over-ride the normal temperature shut-off of biofilm formation at 37°C (33). It is possible that microenvironments within the intestinal niche provide strong activating signals required to generate the CsgD-ON state. In the lumen of the small intestine, high bile concentrations have been shown to increase the intracellular concentration of cyclic-di-GMP in the enteropathogen V. cholerae [reviewed in Ref. (181)] and to influence biofilm formation (183). For Salmonella, high concentrations of c-di-GMP activate csgD expression and promote the biofilm phenotype (51). Expression of the T3SS-1 is known to be location-dependent; Salmonella cells positioned at the surface of the intestinal epithelial layer were 100% positive for T3SS-1 expression, while the majority of cells in the lumen were negative for this virulence trait (184). We predict that biofilm expression may also be induced by signals produced toward the end of host–pathogen interactions. These signals may include intestinal inflammation and the associated rapid replication of Salmonella within the lumen (139, 146, 147, 185). The reactive oxygen species produced by neutrophils undoubtedly provides an important cue for activation of the bacterial cell stress response. Metabolic cues, such as the pathways involved with anaerobic respiration during this stage [i.e., ethanolamine and 1,2-propanediol (186)] or the nutrient limitation caused by rapid Salmonella replication following inflammation, may also add a temporal element to the anticipatory regulation of Salmonella biofilm formation. Consistent with this, our transcriptomic analysis revealed that genes for the ethanolamine and 1,2-propanediol metabolic pathways have increased expression within the CsgD-ON multicellular aggregates (103).
Our ability to elucidate the in situ regulation of Salmonella biofilm formation is limited by the reality that Salmonella biofilms have yet to be observed in nature. However, the enteric bacteria V. cholerae has been shown to exist both as planktonic cells and in multicellular aggregates in human stool samples (187, 188). We previously investigated csgD expression and the biofilm phenotype in Salmonella during murine infection by using luciferase reporters fused to biofilm-related gene promoters. csgD expression was activated within the mouse intestine during the course of infection, but the expression of curli biosynthesis genes (csgBAC) was only observed within fecal pellets that had passed out of the infected mice (45). From this experiment, we concluded that synthesis of the extracellular matrix only occurs after passage from the host. However, we have since learned that there is a limitation in the ability to detect bacterial luciferase during the course of infection (189). Heavy bacterial loads were required for consistent luciferase detection, despite expression from a strong constitutive promoter. This means that if activation of extracellular matrix production was limited to only a small subpopulation of cells as part of CsgD bistability, signaling from the csgBAC promoter would not be detectable using bioluminescence as a marker. Work in our laboratory is currently underway to determine if curli fimbriae and multicellular aggregates can be observed during murine infections by using single cell detection techniques (i.e., tissue sectioning and confocal microscopy).
The lifecycle of Salmonella involves exposure to both the host and natural (extracorporeal) environments (190). Evidence from our own and other research groups suggests that the rdar biofilm morphotype controlled by CsgD is crucial to the transmission success of Salmonella, allowing cells to survive the natural environment that is encountered between host infections (45, 77, 83, 103). Similarly, it may be possible that Salmonella produces a cellulose-based biofilm during host infection to mitigate stresses that arise from host immune responses or the harsh intracellular setting of a macrophage (111, 114). While the c-di-GMP signaling network is important for the production of either type of biofilm, most of the enzymes responsible for synthesizing c-di-GMP are exclusively expressed and activated in either a CsgD-dependent or -independent manner (44, 110, 191). Further research is necessary to not only identify the host sites and signals that activate the production of cellulose biofilm during infection, but to determine the reasons why cellulose biofilm production is important in host–pathogen interactions. It is possible that the c-di-GMP-specific pathway for cellulose biofilm expression has evolved to reduce the severity of its virulence and increase the possibility for Salmonella to successfully transmit to a future host.
In this review, we have touched upon new aspects of Salmonella biology that may have important implications for understanding transmission patterns. NTS strains that briefly colonize the host and cause gastroenteritis must contend with survival in both host and non-host environments. On the one hand, they are adapted to colonize the intestines of many different host species and to replicate to high numbers within the inflamed intestinal environment. Perhaps through biofilm formation and the bistable expression of CsgD, the formation of specialized subpopulations of cells (i.e., multicellular aggregates and planktonic cells) represents an evolutionary trade-off for mitigating the unpredictable nature of transmission via the fecal–oral route. In contrast, invasive strains of non-typhoidal and typhoidal Salmonella have evolved to avoid the immune system in order to persist chronically within the systemic niche of the host. This feature of host adaptation relieves the selection pressure placed on Salmonella to persist within an environmental reservoir and correlates with loss of the biofilm phenotype. It could also reflect that loss of biofilm phenotypes relieves selection pressure from the host immune system, which would contribute to their ability to persist in vivo (Figure 4).
Figure 4. Host interactions, lifecycles, and biofilm-forming ability of Salmonella strains. Host-generalist Salmonella strains have a varied lifecycle, in which several host species and environments are encountered, and zoonotic transfer to humans may occur. Transfer may also occur through ingestion of contaminated vegetables (i.e., tomatoes, sprouts) or processed foods. Infections are localized to the intestine and the selection pressures are on intestinal replication and transmission. In contrast, host-adapted and host-restricted Salmonella strains have an evolutionary narrowed lifecycle, in which transmission is primarily between individual hosts. Selection pressures are on immune avoidance with the objective of long-term persistence within the host. The loss of biofilm formation in host-adapted and host-restricted strains is thought to reflect a shift in selection pressures caused by a change in lifecycle.
Recent advances in DNA sequencing technology have expanded the availability of pathogen genomic sequences, which has led to unprecedented characterization of both classical and emerging Salmonella strains. iNTS strains are an example of how genome sequencing can be complemented by our understanding of Salmonella pathogenesis to develop a genetic signature for host adaptation. Similarly, future research efforts are needed to isolate the core genetic processes that govern biofilm formation. We predict that a biofilm gene signature or gene expression profile could be used to predict the transmission properties of Salmonella strains associated with future outbreaks. Understanding common reservoirs and transmission routes is crucial for developing effective public health efforts to reduce the worldwide disease burden of Salmonella pathogens.
KM and MP prepared the figures, KM and AW wrote the paper, and MP and WK edited the paper. All authors read and approved the final manuscript.
The authors declare that the research was conducted in the absence of any commercial or financial relationships that could be construed as a potential conflict of interest.
This research was supported by a grant from the Natural Sciences and Engineering Research Council (NSERC) (#2017-05737) to AW and through the Jarislowsky Chair in Biotechnology. KM was supported by a Canada Graduate Scholarship from NSERC, and MP was supported by a NSERC-CREATE scholarship through the Integrated Training Program in Infectious Diseases, Food Safety and Public Policy at the University of Saskatchewan.
1. Crosa JH, Brenner DJ, Ewing WH, Falkow S. Molecular relationships among the salmonelleae. J Bacteriol (1973) 115:307–15.
2. Nataro JP, Bopp CA, Fields PI, Kaper JB, Strockbine NA. Escherichia, Shigella, and Salmonella. 10th ed. In: Versalovic J, Carroll KC, Funke G, Jorgensen JH, Landry ML, Warnock DW, editors. Manual of Clinical Microbiology. Washington, DC: American Society of Microbiology (2011). p. 603–26.
3. Tindall BJ. Nomenclature and taxonomy of the genus Salmonella. Int J Syst Evol Microbiol (2005) 55:521–4. doi:10.1099/ijs.0.63580-0
4. Le Minor L, Popoff MY. Designation of Salmonella enterica sp. nov., nom. rev., as the type and only species of the genus Salmonella: request for an opinion. Int J Syst Evol Microbiol (1987) 37:465–8. doi:10.1099/00207713-37-4-465
5. Grimont P, Weill FX. Antigenic Formulae of the Salmonella Serovars. Paris: WHO Collaborating Centre for Reference and Research on Salmonella (2007).
6. Dieckmann R, Malorny B. Rapid screening of epidemiologically important Salmonella enterica subsp. enterica serovars by whole-cell matrix-assisted laser desorption ionization-time of flight mass spectrometry. Appl Environ Microbiol (2011) 77:4136–46. doi:10.1128/AEM.02418-10
7. Ashton PM, Nair S, Peters TM, Bale JA, Powell DG, Painset A, et al. Identification of Salmonella for public health surveillance using whole genome sequencing. PeerJ (2016) 4:e1752. doi:10.7717/peerj.1752
8. Wong VK, Baker S, Connor TR, Pickard D, Page AJ, Dave J, et al. An extended genotyping framework for Salmonella enterica serovar typhi, the cause of human typhoid. Nat Commun (2016) 7:12827. doi:10.1038/ncomms12827
9. Achtman M, Wain J, Weill F-X, Nair S, Zhou Z, Sangal V, et al. Multilocus sequence typing as a replacement for serotyping in Salmonella enterica. PLoS Pathog (2012) 8:e1002776. doi:10.1371/journal.ppat.1002776
10. Centers for Disease Control and Prevention (CDC). National Salmonella Surveillance Overview. Atlanta, GA: US Department of Health and Human Services, CDC (2011).
11. Brenner FW, Villar RG, Angulo FJ, Tauxe R, Swaminathan B. Salmonella nomenclature. J Clin Microbiol (2000) 38:2465–7.
12. Majowicz SE, Musto J, Scallan E, Angulo FJ, Kirk M, O’Brien SJ, et al. The global burden of nontyphoidal Salmonella gastroenteritis. Clin Infect Dis (2010) 50:882–9. doi:10.1086/650733
13. Tennant SM, MacLennan CA, Simon R, Martin LB, Khan MI. Nontyphoidal Salmonella disease: current status of vaccine research and development. Vaccine (2016) 34:2907–10. doi:10.1016/j.vaccine.2016.03.072
14. Wallis TS, Galyov EE. Molecular basis of Salmonella-induced enteritis. Mol Microbiol (2000) 36:997–1005. doi:10.1046/j.1365-2958.2000.01892.x
15. Kirk MD, Pires SM, Black RE, Caipo M, Crump JA, Devleesschauwer B, et al. World Health Organization estimates of the global and regional disease burden of 22 foodborne bacterial, protozoal, and viral diseases, 2010: a data synthesis. PLoS Med (2015) 12:e1001921. doi:10.1371/journal.pmed.1001921
16. Dougan G, Baker S. Salmonella enterica serovar typhi and the pathogenesis of typhoid fever. Annu Rev Microbiol (2014) 68:317–36. doi:10.1146/annurev-micro-091313-103739
17. Gunn JS, Marshall JM, Baker S, Dongol S, Charles RC, Ryan ET. Salmonella chronic carriage: epidemiology, diagnosis, and gallbladder persistence. Trends Microbiol (2014) 22:648–55. doi:10.1016/j.tim.2014.06.007
18. Reddy EA, Shaw AV, Crump JA. Community-acquired bloodstream infections in Africa: a systematic review and meta-analysis. Lancet Infect Dis (2010) 10:417–32. doi:10.1016/S1473-3099(10)70072-4
19. Feasey NA, Dougan G, Kingsley RA, Heyderman RS, Gordon MA. Invasive non-typhoidal Salmonella disease: an emerging and neglected tropical disease in Africa. Lancet (2012) 379:2489–99. doi:10.1016/S0140-6736(11)61752-2
20. Gordon MA. Salmonella infections in immunocompromised adults. J Infect (2008) 56:413–22. doi:10.1016/j.jinf.2008.03.012
21. Hoffmann S, Batz MB, Morris JG Jr. Annual cost of illness and quality-adjusted life year losses in the United States due to 14 foodborne pathogens. J Food Prot (2012) 75(7):1292–302. doi:10.4315/0362-028X.JFP-11-417
22. USDA. Cost Estimates of Foodborne Illnesses. (2014). Available from: https://www.ers.usda.gov/data-products/cost-estimates-of-foodborne-illnesses/
23. Fux CA, Costerton JW, Stewart PS, Stoodley P. Survival strategies of infectious biofilms. Trends Microbiol (2005) 13:34–40. doi:10.1016/j.tim.2004.11.010
24. Jacques M, Aragon V, Tremblay YDN. Biofilm formation in bacterial pathogens of veterinary importance. Anim Health Res Rev (2010) 11:97–121. doi:10.1017/S1466252310000149
25. Wolcott R, Costerton JW, Raoult D, Cutler SJ. The polymicrobial nature of biofilm infection. Clin Microbiol Infect (2013) 19:107–12. doi:10.1111/j.1469-0691.2012.04001.x
26. Drescher K, Nadell CD, Stone HA, Wingreen NS, Bassler BL. Solutions to the public goods dilemma in bacterial biofilms. Curr Biol (2014) 24:50–5. doi:10.1016/j.cub.2013.10.030
27. Xavier JB, Foster KR. Cooperation and conflict in microbial biofilms. Proc Natl Acad Sci U S A (2007) 104:876–81. doi:10.1073/pnas.0607651104
28. Létoffé S, Chalabaev S, Dugay J, Stressmann F, Audrain B, Portais J-C, et al. Biofilm microenvironment induces a widespread adaptive amino-acid fermentation pathway conferring strong fitness advantage in Escherichia coli. PLoS Genet (2017) 13:e1006800. doi:10.1371/journal.pgen.1006800
29. Wessel AK, Arshad TA, Fitzpatrick M, Connell JL, Bonnecaze RT, Shear JB, et al. Oxygen limitation within a bacterial aggregate. MBio (2014) 5:e00992. doi:10.1128/mBio.00992-14
30. Melaugh G, Hutchison J, Kragh KN, Irie Y, Roberts A, Bjarnsholt T, et al. Shaping the growth behaviour of biofilms initiated from bacterial aggregates. PLoS One (2016) 11:e149683. doi:10.1371/journal.pone.0149683
31. Mulcahy H, Charron-Mazenod L, Lewenza S. Extracellular DNA chelates cations and induces antibiotic resistance in Pseudomonas aeruginosa biofilms. PLoS Pathog (2008) 4:e1000213. doi:10.1371/journal.ppat.1000213
32. Collinson SK, Emödy L, Müller KH, Trust TJ, Kay WW. Purification and characterization of thin, aggregative fimbriae from Salmonella enteritidis. J Bacteriol (1991) 173:4773–81. doi:10.1128/jb.173.15.4773-4781.1991
33. Römling U, Sierralta WD, Eriksson K, Normark S. Multicellular and aggregative behaviour of Salmonella typhimurium strains is controlled by mutations in the agfD promoter. Mol Microbiol (1998) 28:249–64. doi:10.1046/j.1365-2958.1998.00791.x
34. Barnhart MM, Chapman MR. Curli biogenesis and function. Annu Rev Microbiol (2006) 60:131–47. doi:10.1146/annurev.micro.60.080805.142106
35. Römling U, Galperin MY. Bacterial cellulose biosynthesis: diversity of operons, subunits, products, and functions. Trends Microbiol (2015) 23:545–57. doi:10.1016/j.tim.2015.05.005
36. Römling U, Rohde M, Olsén A, Normark S, Reinköster J. AgfD, the checkpoint of multicellular and aggregative behaviour in Salmonella typhimurium regulates at least two independent pathways. Mol Microbiol (2000) 36:10–23. doi:10.1046/j.1365-2958.2000.01822.x
37. White AP, Gibson DL, Kim W, Kay WW, Surette MG. Thin aggregative fimbriae and cellulose enhance long-term survival and persistence of Salmonella. J Bacteriol (2006) 188:3219–27. doi:10.1128/JB.188.9.3219-3227.2006
38. Gibson DL, White AP, Snyder SD, Martin S, Heiss C, Azadi P, et al. Salmonella produces an O-antigen capsule regulated by AgfD and important for environmental persistence. J Bacteriol (2006) 188:7722–30. doi:10.1128/JB.00809-06
39. de Rezende CE, Anriany Y, Carr LE, Joseph SW, Weiner RM. Capsular polysaccharide surrounds smooth and rugose types of Salmonella enterica serovar typhimurium DT104. Appl Environ Microbiol (2005) 71:7345–51. doi:10.1128/AEM.71.11.7345-7351.2005
40. Serra DO, Richter AM, Klauck G, Mika F, Hengge R. Microanatomy at cellular resolution and spatial order of physiological differentiation in a bacterial biofilm. MBio (2013) 4:e103–13. doi:10.1128/mBio.00103-13
41. Collinson SK, Doig PC, Doran JL, Clouthier S, Trust TJ, Kay WW. Thin, aggregative fimbriae mediate binding of Salmonella enteritidis to fibronectin. J Bacteriol (1993) 175:12–8. doi:10.1128/jb.175.1.12-18.1993
42. Solano C, García B, Valle J, Berasain C, Ghigo J-M, Gamazo C, et al. Genetic analysis of Salmonella enteritidis biofilm formation: critical role of cellulose. Mol Microbiol (2002) 43:793–808. doi:10.1046/j.1365-2958.2002.02802.x
43. Latasa C, Roux A, Toledo-Arana A, Ghigo J-M, Gamazo C, Penadés JR, et al. BapA, a large secreted protein required for biofilm formation and host colonization of Salmonella enterica serovar enteritidis. Mol Microbiol (2005) 58:1322–39. doi:10.1111/j.1365-2958.2005.04907.x
44. Solano C, García B, Latasa C, Toledo-Arana A, Zorraquino V, Valle J, et al. Genetic reductionist approach for dissecting individual roles of GGDEF proteins within the c-di-GMP signaling network in Salmonella. Proc Natl Acad Sci U S A (2009) 106:7997–8002. doi:10.1073/pnas.0812573106
45. White AP, Gibson DL, Grassl GA, Kay WW, Finlay BB, Vallance BA, et al. Aggregation via the red, dry, and rough morphotype is not a virulence adaptation in Salmonella enterica serovar typhimurium. Infect Immun (2008) 76:1048–58. doi:10.1128/IAI.01383-07
46. White AP, Surette MG. Comparative genetics of the rdar morphotype in Salmonella. J Bacteriol (2006) 188:8395–406. doi:10.1128/JB.00798-06
47. Steenackers H, Hermans K, Vanderleyden J. Salmonella biofilms: an overview on occurrence, structure, regulation and eradication. Food Res Intern (2012) 45:502–31. doi:10.1016/j.foodres.2011.01.038
48. Simm R, Ahmad I, Rhen M, Le Guyon S, Römling U. Regulation of biofilm formation in Salmonella enterica serovar typhimurium. Future Microbiol (2014) 9:1261–82. doi:10.2217/fmb.14.88
49. Gerstel U, Park C, Römling U. Complex regulation of csgD promoter activity by global regulatory proteins. Mol Microbiol (2003) 49:639–54. doi:10.1046/j.1365-2958.2003.03594.x
50. Simm R, Morr M, Kader A, Nimtz M, Römling U. GGDEF and EAL domains inversely regulate cyclic di-GMP levels and transition from sessility to motility. Mol Microbiol (2004) 53:1123–34. doi:10.1111/j.1365-2958.2004.04206.x
51. Romling U, Galperin MY, Gomelsky M. Cyclic di-GMP: the first 25 years of a universal bacterial second messenger. Microbiol Mol Biol Rev (2013) 77:1–52. doi:10.1128/MMBR.00043-12
52. Ahmad I, Cimdins A, Beske T, Römling U. Detailed analysis of c-di-GMP mediated regulation of csgD expression in Salmonella typhimurium. BMC Microbiol (2017) 17:27. doi:10.1186/s12866-017-0934-5
53. Hufnagel DA, Evans ML, Greene SE, Pinkner JS, Hultgren SJ, Chapman MR. The catabolite repressor protein-cyclic AMP complex regulates csgD and biofilm formation in uropathogenic Escherichia coli. J Bacteriol (2016) 198:3329–34. doi:10.1128/JB.00652-16
54. White AP, Weljie AM, Apel D, Zhang P, Shaykhutdinov R, Vogel HJ, et al. A global metabolic shift is linked to Salmonella multicellular development. PLoS One (2010) 5:e11814. doi:10.1371/journal.pone.0011814
55. Crawford RW, Rosales-Reyes R, Ramírez-Aguilar Mde LL, Chapa-Azuela O, Alpuche-Aranda C, Gunn JS. Gallstones play a significant role in Salmonella spp. gallbladder colonization and carriage. Proc Natl Acad Sci U S A (2010) 107:4353–8. doi:10.1073/pnas.1000862107
56. Prouty AM, Schwesinger WH, Gunn JS. Biofilm formation and interaction with the surfaces of gallstones by Salmonella spp. Infect Immun (2002) 70:2640–9. doi:10.1128/IAI.70.5.2640-2649.2002
57. Gonzalez-Escobedo G, Marshall JM, Gunn JS. Chronic and acute infection of the gall bladder by Salmonella typhi: understanding the carrier state. Nat Rev Microbiol (2011) 9:9–14. doi:10.1038/nrmicro2490
58. Crawford RW, Gibson DL, Kay WW, Gunn JS. Identification of a bile-induced exopolysaccharide required for Salmonella biofilm formation on gallstone surfaces. Infect Immun (2008) 76:5341–9. doi:10.1128/IAI.00786-08
59. Marshall JM, Flechtner AD, La Perle KM, Gunn JS. Visualization of extracellular matrix components within sectioned Salmonella biofilms on the surface of human gallstones. PLoS One (2014) 9:e89243. doi:10.1371/journal.pone.0089243
60. Bäumler AJ, Gilde AJ, Tsolis RM, van der Velden AW, Ahmer BM, Heffron F. Contribution of horizontal gene transfer and deletion events to development of distinctive patterns of fimbrial operons during evolution of Salmonella serotypes. J Bacteriol (1997) 179:317–22. doi:10.1128/jb.179.2.317-322.1997
61. Nuccio SP, Thomson NR, Fookes M, Baumler AJ. Fimbrial signature arrangements in Salmonella. In: Porwollik S, editor. Salmonella: From Genome to Function. Norfolk, UK: Caister Academic Press (2011). p. 149–61.
62. Yue M, Rankin SC, Blanchet RT, Nulton JD, Edwards RA, Schifferli DM. Diversification of the Salmonella fimbriae: a model of macro- and microevolution. PLoS One (2012) 7:e38596. doi:10.1371/journal.pone.0038596
63. Hull RA, Gill RE, Hsu P, Minshew BH, Falkow S. Construction and expression of recombinant plasmids encoding type 1 or D-mannose-resistant pili from a urinary tract infection Escherichia coli isolate. Infect Immun (1981) 33:933–8.
64. Lund B, Marklund BI, Strömberg N, Lindberg F, Karlsson KA, Normark S. Uropathogenic Escherichia coli can express serologically identical pili of different receptor binding specificities. Mol Microbiol (1988) 2:255–63. doi:10.1111/j.1365-2958.1988.tb00027.x
65. Kline KA, FAlker S, Dahlberg S, Normark S, Henriques-Normark B. Bacterial adhesins in host-microbe interactions. Cell Host Microbe (2009) 5:580–92. doi:10.1016/j.chom.2009.05.011
66. Rajashekara G, Munir S, Alexeyev MF, Halvorson DA, Wells CL, Nagaraja KV. Pathogenic role of SEF14, SEF17, and SEF21 fimbriae in Salmonella enterica serovar enteritidis infection of chickens. Appl Environ Microbiol (2000) 66:1759–63. doi:10.1128/AEM.66.4.1759-1763.2000
67. Doran JL, Collinson SK, Burian J, Sarlós G, Todd EC, Munro CK, et al. DNA-based diagnostic tests for Salmonella species targeting agfA, the structural gene for thin, aggregative fimbriae. J Clin Microbiol (1993) 31:2263–73.
68. Collinson SK, Emödy L, Trust TJ, Kay WW. Thin aggregative fimbriae from diarrheagenic Escherichia coli. J Bacteriol (1992) 174:4490–5. doi:10.1128/jb.174.13.4490-4495.1992
69. Romling U, Bian Z, Hammar M, Sierralta WD, Normark S. Curli fibers are highly conserved between Salmonella typhimurium and Escherichia coli with respect to operon structure and regulation. J Bacteriol (1998) 180:722–31.
70. Lawrence JG, Ochman H. Molecular archaeology of the Escherichia coli genome. Proc Natl Acad Sci U S A (1998) 95:9413–7. doi:10.1073/pnas.95.16.9413
71. Hammar M, Arnqvist A, Bian Z, Olsén A, Normark S. Expression of two csg operons is required for production of fibronectin- and congo red-binding curli polymers in Escherichia coli K-12. Mol Microbiol (1995) 18:661–70. doi:10.1111/j.1365-2958.1995.mmi_18040661.x
72. Collinson SK, Clouthier SC, Doran JL, Banser PA, Kay WW. Salmonella enteritidis agfBAC operon encoding thin, aggregative fimbriae. J Bacteriol (1996) 178:662–7. doi:10.1128/jb.178.3.662-667.1996
73. Collinson SK, Parker JM, Hodges RS, Kay WW. Structural predictions of AgfA, the insoluble fimbrial subunit of Salmonella thin aggregative fimbriae. J Mol Biol (1999) 290:741–56. doi:10.1006/jmbi.1999.2882
74. Chapman MR, Robinson LS, Pinkner JS, Roth R, Heuser J, Hammar M, et al. Role of Escherichia coli curli operons in directing amyloid fiber formation. Science (2002) 295:851–5. doi:10.1126/science.1067484
75. Shewmaker F, McGlinchey RP, Thurber KR, McPhie P, Dyda F, Tycko R, et al. The functional curli amyloid is not based on in-register parallel β-sheet structure. J Biol Chem (2009) 284:25065–76. doi:10.1074/jbc.M109.007054
76. Olsén A, Jonsson A, Normark S. Fibronectin binding mediated by a novel class of surface organelles on Escherichia coli. Nature (1989) 338:652–5. doi:10.1038/338652a0
77. Gerstel U, Römling U. Oxygen tension and nutrient starvation are major signals that regulate agfD promoter activity and expression of the multicellular morphotype in Salmonella typhimurium. Environ Microbiol (2001) 3:638–48. doi:10.1046/j.1462-2920.2001.00235.x
78. Anriany YA, Weiner RM, Johnson JA, De Rezende CE, Joseph SW. Salmonella enterica serovar typhimurium DT104 displays a rugose phenotype. Appl Environ Microbiol (2001) 67:4048–56. doi:10.1128/AEM.67.9.4048-4056.2001
79. Wai SN, Mizunoe Y, Takade A, Kawabata SI, Yoshida SI. Vibrio cholerae O1 strain TSI-4 produces the exopolysaccharide materials that determine colony morphology, stress resistance, and biofilm formation. Appl Environ Microbiol (1998) 64:3648–55.
80. Zogaj X, Nimtz M, Rohde M, Bokranz W, Römling U. The multicellular morphotypes of Salmonella typhimurium and Escherichia coli produce cellulose as the second component of the extracellular matrix. Mol Microbiol (2001) 39:1452–63. doi:10.1046/j.1365-2958.2001.02337.x
81. Scher K, Romling U, Yaron S. Effect of heat, acidification, and chlorination on Salmonella enterica serovar typhimurium cells in a biofilm formed at the air-liquid interface. Appl Environ Microbiol (2005) 71:1163–8. doi:10.1128/AEM.71.3.1163-1168.2005
82. Sutherland IW. The biofilm matrix – an immobilized but dynamic microbial environment. Trends Microbiol (2001) 9:222–7. doi:10.1016/S0966-842X(01)02012-1
83. Apel D, White AP, Grassl GA, Finlay BB, Surette MG. Long-term survival of Salmonella enterica serovar typhimurium reveals an infectious state that is underrepresented on laboratory media containing bile salts. Appl Environ Microbiol (2009) 75:4923–5. doi:10.1128/AEM.00363-09
84. Waldner L, MacKenzie K, Köster W, White A. From exit to entry: long-term survival and transmission of Salmonella. Pathogens (2012) 1:128–55. doi:10.3390/pathogens1020128
85. Gupte AR, de Rezende CLE, Joseph SW. Induction and resuscitation of viable but nonculturable Salmonella enterica serovar typhimurium DT104. Appl Environ Microbiol (2003) 69:6669–75. doi:10.1128/AEM.69.11.6669-6675.2003
86. Lesne J, Berthet S, Binard S, Rouxel A, Humbert F. Changes in culturability and virulence of Salmonella typhimurium during long-term starvation under desiccating conditions. Int J Food Microbiol (2000) 60:195–203. doi:10.1016/S0168-1605(00)00311-1
87. Davies RH, Wray C. Mice as carriers of Salmonella enteritidis on persistently infected poultry units. Vet Rec (1995) 137:337–41. doi:10.1136/vr.137.14.337
88. Lapuz R, Tani H, Sasai K, Shirota K, Katoh H, Baba E. The role of roof rats (Rattus rattus) in the spread of Salmonella enteritidis and S. infantis contamination in layer farms in eastern Japan. Epidemiol Infect (2007) 136:1267–9. doi:10.1017/S095026880700948X
89. Wales AD, Carrique-Mas JJ, Rankin M, Bell B, Thind BB, Davies RH. Review of the carriage of zoonotic bacteria by arthropods, with special reference to Salmonella in mites, flies and litter beetles. Zoonoses Public Health (2009) 57(5):299–314. doi:10.1111/j.1863-2378.2008.01222.x
90. De Oliveira DCV, Fernandes Júnior A, Kaneno R, Silva MG, Araújo Júnior JP, Silva NCC, et al. Ability of Salmonella spp. to produce biofilm is dependent on temperature and surface material. Foodborne Pathog Dis (2014) 11:478–83. doi:10.1089/fpd.2013.1710
91. Laviniki V, Lopes GV, Pellegrini DP. Biofilm formation by Salmonella enterica strains isolated from feed mills. Proceedings Safe Pork 2015, Epidemiology and Control of Hazards in Pork Production Chain. Porto, Portugal (2015). p. 209–12. Available from: http://lib.dr.iastate.edu/safepork/2015/allpapers/53/
92. Vestby LK, Møretrø T, Ballance S, Langsrud S, Nesse LL. Survival potential of wild type cellulose deficient Salmonella from the feed industry. BMC Vet Res (2009) 5:43. doi:10.1186/1746-6148-5-43
93. Stocki SL, Annett CB, Sibley CD, McLaws M, Checkley SL, Singh N, et al. Persistence of Salmonella on egg conveyor belts is dependent on the belt type but not on the rdar morphotype. Poult Sci (2007) 86:2375–83. doi:10.3382/ps.2007-00121
94. Yaron S, Römling U. Biofilm formation by enteric pathogens and its role in plant colonization and persistence. Microb Biotechnol (2014) 7:496–516. doi:10.1111/1751-7915.12186
95. Cowles KN, Willis DK, Engel TN, Jones JB, Barak JD. Diguanylate cyclases AdrA and STM1987 regulate Salmonella enterica exopolysaccharide production during plant colonization in an environment-dependent manner. Appl Environ Microbiol (2016) 82:1237–48. doi:10.1128/AEM.03475-15
96. Barak JD, Jahn CE, Gibson DL, Charkowski AO. The role of cellulose and O-antigen capsule in the colonization of plants by Salmonella enterica. Mol Plant Microbe Interact (2007) 20:1083–91. doi:10.1094/MPMI-20-9-1083
97. Barak JD, Gorski L, Naraghi-Arani P, Charkowski AO. Salmonella enterica virulence genes are required for bacterial attachment to plant tissue. Appl Environ Microbiol (2005) 71:5685–91. doi:10.1128/AEM.71.10.5685-5691.2005
98. Lapidot A, Yaron S. Transfer of Salmonella enterica serovar typhimurium from contaminated irrigation water to parsley is dependent on curli and cellulose, the biofilm matrix components. J Food Prot (2009) 72:618–23. doi:10.4315/0362-028X-72.3.618
99. Safadi RA, Abu-Ali GS, Sloup RE, Rudrik JT, Waters CM, Eaton KA, et al. Correlation between in vivo biofilm formation and virulence gene expression in Escherichia coli O104:H4. PLoS One (2012) 7:e41628. doi:10.1371/journal.pone.0041628
100. Richter AM, Povolotsky TL, Wieler LH, Hengge R. Cyclic-di-GMP signalling and biofilm-related properties of the Shiga toxin-producing 2011 German outbreak Escherichia coli O104:H4. EMBO Mol Med (2014) 6:1622–37. doi:10.15252/emmm.201404309
101. Breuer T, Benkel DH, Shapiro RL, Hall WN, Winnett MM, Linn MJ, et al. A multistate outbreak of Escherichia coli O157:H7 infections linked to alfalfa sprouts grown from contaminated seeds. Emerg Infect Dis (2001) 7:977–82. doi:10.3201/eid0706.010609
102. Blaser MJ, Newman LS. A review of human salmonellosis: I. Infective dose. Rev Infect Dis (1982) 4:1096–106. doi:10.1093/clinids/4.6.1096
103. MacKenzie KD, Wang Y, Shivak DJ, Wong CS, Hoffman LJL, Lam S, et al. Bistable expression of CsgD in Salmonella enterica serovar typhimurium connects virulence to persistence. Infect Immun (2015) 83:2312–26. doi:10.1128/IAI.00137-15
104. Adcox HE, Vasicek EM, Dwivedi V, Hoang KV, Turner J, Gunn JS. Salmonella extracellular matrix components influence biofilm formation and gallbladder colonization. Infect Immun (2016) 84:3243–51. doi:10.1128/IAI.00532-16
105. Tükel Ç, Raffatellu M, Humphries AD, Wilson RP, Andrews-Polymenis HL, Gull T, et al. CsgA is a pathogen-associated molecular pattern of Salmonella enterica serotype typhimurium that is recognized by toll-like receptor 2. Mol Microbiol (2005) 58:289–304. doi:10.1111/j.1365-2958.2005.04825.x
106. Tükel Ç, Nishimori JH, Wilson RP, Winter MG, Keestra AM, Van Putten JPM, et al. Toll-like receptors 1 and 2 cooperatively mediate immune responses to curli, a common amyloid from enterobacterial biofilms. Cell Microbiol (2010) 12:1495–505. doi:10.1111/j.1462-5822.2010.01485.x
107. Rapsinski GJ, Wynosky-Dolfi MA, Oppong GO, Tursi SA, Wilson RP, Brodsky IE, et al. Toll-like receptor 2 and NLRP3 cooperate to recognize a functional bacterial amyloid, curli. Infect Immun (2015) 83:693–701. doi:10.1128/IAI.02370-14
108. Weening EH, Barker JD, Laarakker MC, Humphries AD, Tsolis RM, Bäumler AJ. The Salmonella enterica serotype typhimurium lpf, bcf, stb, stc, std, and sth fimbrial operons are required for intestinal persistence in mice. Infect Immun (2005) 73:3358–66. doi:10.1128/IAI.73.6.3358-3366.2005
109. Da Re S, Ghigo JM. A CsgD-independent pathway for cellulose production and biofilm formation in Escherichia coli. J Bacteriol (2006) 188:3073–87. doi:10.1128/JB.188.8.3073-3087.2006
110. García B, Latasa C, Solano C, Portillo FG-D, Gamazo C, Lasa I. Role of the GGDEF protein family in Salmonella cellulose biosynthesis and biofilm formation. Mol Microbiol (2004) 54:264–77. doi:10.1111/j.1365-2958.2004.04269.x
111. Amarasinghe JJ, D’Hondt RE, Waters CM, Mantis NJ. Exposure of Salmonella enterica serovar typhimurium to a protective monoclonal IgA triggers exopolysaccharide production via a diguanylate cyclase-dependent pathway. Infect Immun (2013) 81:653–64. doi:10.1128/IAI.00813-12
112. Bokranz W, Wang X, Tschäpe H, Römling U. Expression of cellulose and curli fimbriae by Escherichia coli isolated from the gastrointestinal tract. J Med Microbiol (2005) 54:1171–82. doi:10.1099/jmm.0.46064-0
113. Monteiro C, Saxena I, Wang X, Kader A, Bokranz W, Simm R, et al. Characterization of cellulose production in Escherichia coli nissle 1917 and its biological consequences. Environ Microbiol (2009) 11:1105–16. doi:10.1111/j.1462-2920.2008.01840.x
114. Pontes MH, Lee E-J, Choi J, Groisman EA. Salmonella promotes virulence by repressing cellulose production. Proc Natl Acad Sci U S A (2015) 112:5183–8. doi:10.1073/pnas.1500989112
115. Ahmad I, Rouf SF, Sun L, Cimdins A, Shafeeq S, Guyon S, et al. BcsZ inhibits biofilm phenotypes and promotes virulence by blocking cellulose production in Salmonella enterica serovar typhimurium. Microb Cell Fact (2016) 15(1):177. doi:10.1186/s12934-016-0576-6
116. Mills E, Petersen E, Kulasekara BR, Miller SI. A direct screen for c-di-GMP modulators reveals a Salmonella typhimurium periplasmic l-arginine-sensing pathway. Sci Signal (2015) 8:ra57. doi:10.1126/scisignal.aaa1796
117. Ahmad I, Lamprokostopoulou A, Le Guyon S, Streck E, Barthel M, Peters V, et al. Complex c-di-GMP signaling networks mediate transition between virulence properties and biofilm formation in Salmonella enterica serovar typhimurium. PLoS One (2011) 6:e28351. doi:10.1371/journal.pone.0028351
118. Lamprokostopoulou A, Monteiro C, Rhen M, Römling U. Cyclic di-GMP signalling controls virulence properties of Salmonella enterica serovar typhimurium at the mucosal lining. Environ Microbiol (2010) 12:40–53. doi:10.1111/j.1462-2920.2009.02032.x
119. Boyd EF, Wang FS, Whittam TS, Selander RK. Molecular genetic relationships of the salmonellae. Appl Environ Microbiol (1996) 62:804–8.
120. Davidson CJ, White AP, Surette MG. Evolutionary loss of the rdar morphotype in Salmonella as a result of high mutation rates during laboratory passage. ISME J (2008) 2:293–307. doi:10.1038/ismej.2008.4
121. Römling U, Bokranz W, Rabsch W, Zogaj X, Nimtz M, Tschäpe H. Occurrence and regulation of the multicellular morphotype in Salmonella serovars important in human disease. Int J Med Microbiol (2003) 293:273–85. doi:10.1078/1438-4221-00268
122. Solomon EB, Niemira BA, Sapers GM, Annous BA. Biofilm formation, cellulose production, and curli biosynthesis by Salmonella originating from produce, animal, and clinical sources. J Food Prot (2005) 68:906–12. doi:10.4315/0362-028X-68.5.906
123. Boyd EF, Wang FS, Beltran P, Plock SA, Nelson K, Selander RK. Salmonella reference collection B (SARB): strains of 37 serovars of subspecies I. J Gen Microbiol (1993) 139(Pt 6):1125–32. doi:10.1099/00221287-139-6-1125
124. Malcova M, Hradecka H, Karpiskova R, Rychlik I. Biofilm formation in field strains of Salmonella enterica serovar typhimurium: identification of a new colony morphology type and the role of SGI1 in biofilm formation. Vet Microbiol (2008) 129:360–6. doi:10.1016/j.vetmic.2007.12.006
125. Ramachandran G, Aheto K, Shirtliff ME, Tennant SM. Poor biofilm-forming ability and long-term survival of invasive Salmonella typhimurium ST313. Pathog Dis (2016) 74:ftw49. doi:10.1093/femspd/ftw049
126. Singletary LA, Karlinsey JE, Libby SJ, Mooney JP, Lokken KL, Tsolis RM, et al. Loss of multicellular behavior in epidemic African nontyphoidal Salmonella enterica serovar typhimurium ST313 strain D23580. MBio (2016) 7:e2265. doi:10.1128/mBio.02265-15
127. Okoro CK, Kingsley RA, Connor TR, Harris SR, Parry CM, Al-Mashhadani MN, et al. Intracontinental spread of human invasive Salmonella typhimurium pathovariants in sub-Saharan Africa. Nat Genet (2012) 44:1215–21. doi:10.1038/ng.2423
128. Ashton PM, Owen SV, Kaindama L, Rowe WPM, Lane C, Larkin L, et al. Salmonella enterica Serovar Typhimurium ST313 Responsible for Gastroenteritis in the UK Are Genetically Distinct from Isolates Causing Bloodstream Infections in Africa. bioRxiv (2017). 1–25.
129. Rabsch W, Andrews HL, Kingsley RA, Prager R, Tschäpe H, Adams LG, et al. Salmonella enterica serotype typhimurium and its host-adapted variants. Infect Immun (2002) 70:2249–55. doi:10.1128/IAI.70.5.2249-2255.2002
130. Mahajan RK, Khan SA, Chandel DS, Kumar N, Hans C, Chaudhry R. Fatal case of Salmonella enterica subsp. arizonae gastroenteritis in an infant with microcephaly. J Clin Microbiol (2003) 41:5830–2. doi:10.1128/JCM.41.12.5830-5832.2003
131. Parkhill J, Dougan G, James KD, Thomson NR, Pickard D, Wain J, et al. Complete genome sequence of a multiple drug resistant Salmonella enterica serovar typhi CT18. Nature (2001) 413:848–52. doi:10.1038/35101607
132. Agbor TA, McCormick BA. Salmonella effectors: important players modulating host cell function during infection. Cell Microbiol (2011) 13:1858–69. doi:10.1111/j.1462-5822.2011.01701.x
133. Moest TP, Méresse S. Salmonella T3SSs: successful mission of the secret(ion) agents. Curr Opin Microbiol (2013) 16:38–44. doi:10.1016/j.mib.2012.11.006
134. Wisner A, Desin T, White AP, Potter A, Köster W. The Salmonella pathogenicity island-1 and -2 encoded type III secretion systems. In: Kumar Y, editor. Salmonella – A Diversified Superbug. Rijeka: InTech (2012). p. 469–94.
135. Costa TRD, Felisberto-Rodrigues C, Meir A, Prevost MS, Redzej A, Trokter M, et al. Secretion systems in Gram-negative bacteria: structural and mechanistic insights. Nat Rev Microbiol (2015) 13:343–59. doi:10.1038/nrmicro3456
136. Hu Y, Huang H, Cheng X, Shu X, White AP, Stavrinides J, et al. A global survey of bacterial type III secretion systems and their effectors. Environ Microbiol (2017) 8:e1002983. doi:10.1111/1462-2920.13755
137. Hensel M. Evolution of pathogenicity islands of Salmonella enterica. Int J Med Microbiol (2004) 294:95–102. doi:10.1016/j.ijmm.2004.06.025
138. Ochman H, Groisman EA. The evolution of invasion by enteric bacteria. Can J Microbiol (1995) 41:555–61. doi:10.1139/m95-074
139. Thiennimitr P, Winter SE, Bäumler AJ. Salmonella, the host and its microbiota. Curr Opin Microbiol (2012) 15:108–14. doi:10.1016/j.mib.2011.10.002
140. Misselwitz B, Barrett N, Kreibich S, Vonaesch P, Andritschke D, Rout S, et al. Near surface swimming of Salmonella typhimurium explains target-site selection and cooperative invasion. PLoS Pathog (2012) 8:e1002810. doi:10.1371/journal.ppat.1002810
141. Altier C. Genetic and environmental control of Salmonella invasion. J Microbiol (2005) 43:85–92.
142. Izoré T, Job V, Dessen A. Biogenesis, regulation, and targeting of the type III secretion system. Structure (2011) 19:603–12. doi:10.1016/j.str.2011.03.015
143. Lara-Tejero M, Galan JE. Salmonella enterica serovar typhimurium pathogenicity island 1-encoded type III secretion system translocases mediate intimate attachment to nonphagocytic cells. Infect Immun (2009) 77:2635–42. doi:10.1128/IAI.00077-09
144. van der Heijden J, Finlay BB. Type III effector-mediated processes in Salmonella infection. Future Microbiol (2012) 7:685–703. doi:10.2217/fmb.12.49
145. Raffatellu M, George MD, Akiyama Y, Hornsby MJ, Nuccio S-P, Paixao TA, et al. Lipocalin-2 resistance confers an advantage to Salmonella enterica serotype typhimurium for growth and survival in the inflamed intestine. Cell Host Microbe (2009) 5:476–86. doi:10.1016/j.chom.2009.03.011
146. Winter SE, Thiennimitr P, Winter MG, Butler BP, Huseby DL, Crawford RW, et al. Gut inflammation provides a respiratory electron acceptor for Salmonella. Nature (2010) 467:426–9. doi:10.1038/nature09415
147. Thiennimitr P, Winter SE, Winter MG, Xavier MN, Tolstikov V, Huseby DL, et al. Intestinal inflammation allows Salmonella to use ethanolamine to compete with the microbiota. Proc Natl Acad Sci U S A (2011) 108:17480–5. doi:10.1073/pnas.1107857108
148. McClelland M, Sanderson KE, Clifton SW, Latreille P, Porwollik S, Sabo A, et al. Comparison of genome degradation in paratyphi A and typhi, human-restricted serovars of Salmonella enterica that cause typhoid. Nat Genet (2004) 36:1268–74. doi:10.1038/ng1470
149. Gal-Mor O, Boyle EC, Grassl GA. Same species, different diseases: how and why typhoidal and non-typhoidal Salmonella enterica serovars differ. Front Microbiol (2014) 5:391. doi:10.3389/fmicb.2014.00391/abstract
150. Winter SE, Raffatellu M, Wilson RP, Rüssmann H, Bäumler AJ. The Salmonella enterica serotype typhi regulator TviA reduces interleukin-8 production in intestinal epithelial cells by repressing flagellin secretion. Cell Microbiol (2008) 10:247–61. doi:10.1111/j.1462-5822.2007.01037.x
151. Crawford RW, Wangdi T, Spees AM, Xavier MN, Tsolis RM, Bäumler AJ. Loss of very-long O-antigen chains optimizes capsule-mediated immune evasion by Salmonella enterica serovar typhi. MBio (2013) 4:e232–213. doi:10.1128/mBio.00232-13
152. Wangdi T, Lee C-Y, Spees AM, Yu C, Kingsbury DD, Winter SE, et al. The Vi capsular polysaccharide enables Salmonella enterica serovar typhi to evade microbe-guided neutrophil chemotaxis. PLoS Pathog (2014) 10:e1004306. doi:10.1371/journal.ppat.1004306
153. Wilson RP, Raffatellu M, Chessa D, Winter SE, Tükel Ç, Bäumler AJ. The Vi-capsule prevents toll-like receptor 4 recognition of Salmonella. Cell Microbiol (2008) 10:876–90. doi:10.1111/j.1462-5822.2007.01090.x
154. Zhang X-L, Jeza VT, Pan Q. Salmonella typhi: from a human pathogen to a vaccine vector. Cell Mol Immunol (2008) 5:91–7. doi:10.1038/cmi.2008.11
155. Spanò S, Liu X, Galan JE. Proteolytic targeting of Rab29 by an effector protein distinguishes the intracellular compartments of human-adapted and broad-host Salmonella. Proc Natl Acad Sci U S A (2011) 108:18418–23. doi:10.1073/pnas.1111959108
156. Baumler AJ, Fang FC. Host specificity of bacterial pathogens. Cold Spring Harb Perspect Med (2013) 3:a010041. doi:10.1101/cshperspect.a010041
157. Berg R. The Alamosa Salmonella outbreak: a gumshoe investigation. J Environ Health (2008) 71:54–8.
158. Kozlica J, Claudet AL, Solomon D, Dunn JR, Carpenter LR. Waterborne outbreak of Salmonella I 4,[5],12:i-. Foodborne Pathog Dis (2010) 7:1431–3. doi:10.1089/fpd.2010.0556
159. Schuster CJ, Ellis AG, Robertson WJ, Charron DF, Aramini JJ, Marshall BJ, et al. Infectious disease outbreaks related to drinking water in Canada, 1974–2001. Can J Public Health (2005) 96:254–8. doi:10.2307/41995866
160. Centers for Disease Control and Prevention. Multistate outbreaks of Salmonella serotype Poona infections associated with eating cantaloupe from Mexico – United States and Canada, 2000–2002. MMWR Morb Mortal Wkly Rep (2002) 51:1044–7.
161. Centers for Disease Control and Prevention. Outbreak of Salmonella serotype Saintpaul infections associated with multiple raw produce items – United States, 2008. MMWR Morb Mortal Wkly Rep (2008) 57:929–34.
162. Greene SK, Daly ER, Talbot EA, Demma LJ, Holzbauer S, Patel NJ, et al. Recurrent multistate outbreak of Salmonella Newport associated with tomatoes from contaminated fields, 2005. Epidemiol Infect (2007) 136:1046–9. doi:10.1017/S095026880700859X
163. Baloda SB, Christensen L, Trajcevska S. Persistence of a Salmonella enterica serovar typhimurium DT12 clone in a piggery and in agricultural soil amended with Salmonella-contaminated slurry. Appl Environ Microbiol (2001) 67:2859–62. doi:10.1128/AEM.67.6.2859-2862.2001
164. Schiøler H, Christiansen ED, Høybye G, Rasmussen SN, Greibe J, The Danish Salca-Group. Biliary calculi in chronic Salmonella carriers and healthy controls: a controlled study. Scand J Infect Dis (2015) 15:17–9. doi:10.3109/inf.1983.15.issue-1.04
165. Blaser MJ, Kirschner D. The equilibria that allow bacterial persistence in human hosts. Nature (2007) 449:843–9. doi:10.1038/nature06198
166. Crump JA, Sjölund-Karlsson M, Gordon MA, Parry CM. Epidemiology, clinical presentation, laboratory diagnosis, antimicrobial resistance, and antimicrobial management of invasive Salmonella infections. Clin Microbiol Rev (2015) 28:901–37. doi:10.1128/CMR.00002-15
167. Kariuki S, Revathi G, Kariuki N, Kiiru J, Mwituria J, Muyodi J, et al. Invasive multidrug-resistant non-typhoidal Salmonella infections in Africa: zoonotic or anthroponotic transmission? J Med Microbiol (2006) 55:585–91. doi:10.1099/jmm.0.46375-0
168. Kariuki S, Revathi G, Gakuya F, Yamo V, Muyodi J, Hart CA. Lack of clonal relationship between non-typhi Salmonella strain types from humans and those isolated from animals living in close contact. FEMS Immunol Med Microbiol (2002) 33:165–71. doi:10.1111/j.1574-695X.2002.tb00587.x
169. Larsen P, Nielsen JL, Otzen D, Nielsen PH. Amyloid-like adhesins produced by floc-forming and filamentous bacteria in activated sludge. Appl Environ Microbiol (2008) 74:1517–26. doi:10.1128/AEM.02274-07
170. Grantcharova N, Peters V, Monteiro C, Zakikhany K, Römling U. Bistable expression of CsgD in biofilm development of Salmonella enterica serovar typhimurium. J Bacteriol (2010) 192:456–66. doi:10.1128/JB.01826-08
171. Ahmad I, Wigren E, Le Guyon S, Vekkeli S, Blanka A, Mouali el Y, et al. The EAL-like protein STM1697 regulates virulence phenotypes, motility and biofilm formation in Salmonella typhimurium. Mol Microbiol (2013) 90:1216–32. doi:10.1111/mmi.12428
172. Desai SK, Winardhi RS, Periasamy S, Dykas MM, Jie Y, Kenney LJ. The horizontally-acquired response regulator SsrB drives a Salmonella lifestyle switch by relieving biofilm silencing. Elife (2016) 5:2467. doi:10.7554/eLife.10747
173. Sokurenko EV, Hasty DL, Dykhuizen DE. Pathoadaptive mutations: gene loss and variation in bacterial pathogens. Trends Microbiol (1999) 7:191–5. doi:10.1016/S0966-842X(99)01493-6
174. Kaper JB, Nataro JP, Mobley HLT. Pathogenic Escherichia coli. Nat Rev Microbiol (2004) 2:123–40. doi:10.1038/nrmicro818
175. Sakellaris H, Hannink NK, Rajakumar K, Bulach D, Hunt M, Sasakawa C, et al. Curli loci of Shigella spp. Infect Immun (2000) 68:3780–3. doi:10.1128/IAI.68.6.3780-3783.2000
176. White AP, Sibley KA, Sibley CD, Wasmuth JD, Schaefer R, Surette MG, et al. Intergenic sequence comparison of Escherichia coli isolates reveals lifestyle adaptations but not host specificity. Appl Environ Microbiol (2011) 77:7620–32. doi:10.1128/AEM.05909-11
177. Escobar Páramo P, Le Menac’h A, Le Gall T, Amorin C, Gouriou S, Picard B, et al. Identification of forces shaping the commensal Escherichia coli genetic structure by comparing animal and human isolates. Environ Microbiol (2006) 8:1975–84. doi:10.1111/j.1462-2920.2006.01077.x
178. Veening J-W, Smits WK, Kuipers OP. Bistability, epigenetics, and bet-hedging in bacteria. Annu Rev Microbiol (2008) 62:193–210. doi:10.1146/annurev.micro.62.081307.163002
179. Ackermann M. A functional perspective on phenotypic heterogeneity in microorganisms. Nat Rev Microbiol (2015) 13:497–508. doi:10.1038/nrmicro3491
180. Mitchell A, Romano GH, Groisman B, Yona A, Dekel E, Kupiec M, et al. Adaptive prediction of environmental changes by microorganisms. Nature (2009) 460:220–4. doi:10.1038/nature08112
181. Silva AJ, Benitez JA. Vibrio cholerae biofilms and cholera pathogenesis. PLoS Negl Trop Dis (2016) 10:e4330. doi:10.1371/journal.pntd.0004330
182. Kader A, Simm R, Gerstel U, Morr M, Römling U. Hierarchical involvement of various GGDEF domain proteins in rdar morphotype development of Salmonella enterica serovar typhimurium. Mol Microbiol (2006) 60:602–16. doi:10.1111/j.1365-2958.2006.05123.x
183. Sengupta C, Mukherjee O, Chowdhury R. Adherence to intestinal cells promotes biofilm formation in Vibrio cholerae. J Infect Dis (2016) 214:1571–8. doi:10.1093/infdis/jiw435
184. Diard M, Garcia V, Maier L, Remus-Emsermann MNP, Regoes RR, Ackermann M, et al. Stabilization of cooperative virulence by the expression of an avirulent phenotype. Nature (2013) 494:353–6. doi:10.1038/nature11913
185. Nuccio SP, Bäumler AJ. Comparative analysis of Salmonella genomes identifies a metabolic network for escalating growth in the inflamed gut. MBio (2014) 5:e929–914. doi:10.1128/mBio.00929-14
186. Faber F, Thiennimitr P, Spiga L, Byndloss MX, Litvak Y, Lawhon S, et al. Respiration of microbiota-derived 1,2-propanediol drives Salmonella expansion during colitis. PLoS Pathog (2017) 13:e1006129. doi:10.1371/journal.ppat.1006129
187. Faruque SM, Biswas K, Udden SMN, Ahmad QS, Sack DA, Nair GB, et al. Transmissibility of cholera: in vivo-formed biofilms and their relationship to infectivity and persistence in the environment. Proc Natl Acad Sci U S A (2006) 103:6350–5. doi:10.1073/pnas.0601277103
188. Nelson EJ, Chowdhury A, Harris JB, Begum YA, Chowdhury F, Khan AI, et al. Complexity of rice-water stool from patients with Vibrio cholerae plays a role in the transmission of infectious diarrhea. Proc Natl Acad Sci U S A (2007) 104:19091–6. doi:10.1073/pnas.0706352104
189. Shivak DJ. Exploring the Dynamics of Salmonella Transmission in a Murine Model of Infection. Master’s thesis, University of Saskatchewan, Saskatoon, Saskatchewan, Canada (2015). Available from: http://hdl.handle.net/10388/ETD-2015-08-2196.
190. Winfield MD, Groisman EA. Role of nonhost environments in the lifestyles of Salmonella and Escherichia coli. Appl Environ Microbiol (2003) 69:3687–94. doi:10.1128/AEM.69.7.3687-3694.2003
Keywords: Salmonella, biofilms, curli, cellulose, gastroenteritis, host adaptation
Citation: MacKenzie KD, Palmer MB, Köster WL and White AP (2017) Examining the Link between Biofilm Formation and the Ability of Pathogenic Salmonella Strains to Colonize Multiple Host Species. Front. Vet. Sci. 4:138. doi: 10.3389/fvets.2017.00138
Received: 10 June 2017; Accepted: 09 August 2017;
Published: 25 August 2017
Edited by:
Michael Kogut, Agricultural Research Service (USDA), United StatesReviewed by:
Ryan Arsenault, University of Delaware, United StatesCopyright: © 2017 MacKenzie, Palmer, Köster and White. This is an open-access article distributed under the terms of the Creative Commons Attribution License (CC BY). The use, distribution or reproduction in other forums is permitted, provided the original author(s) or licensor are credited and that the original publication in this journal is cited, in accordance with accepted academic practice. No use, distribution or reproduction is permitted which does not comply with these terms.
*Correspondence: Aaron P. White, YWFyb24ud2hpdGVAdXNhc2suY2E=
Disclaimer: All claims expressed in this article are solely those of the authors and do not necessarily represent those of their affiliated organizations, or those of the publisher, the editors and the reviewers. Any product that may be evaluated in this article or claim that may be made by its manufacturer is not guaranteed or endorsed by the publisher.
Research integrity at Frontiers
Learn more about the work of our research integrity team to safeguard the quality of each article we publish.