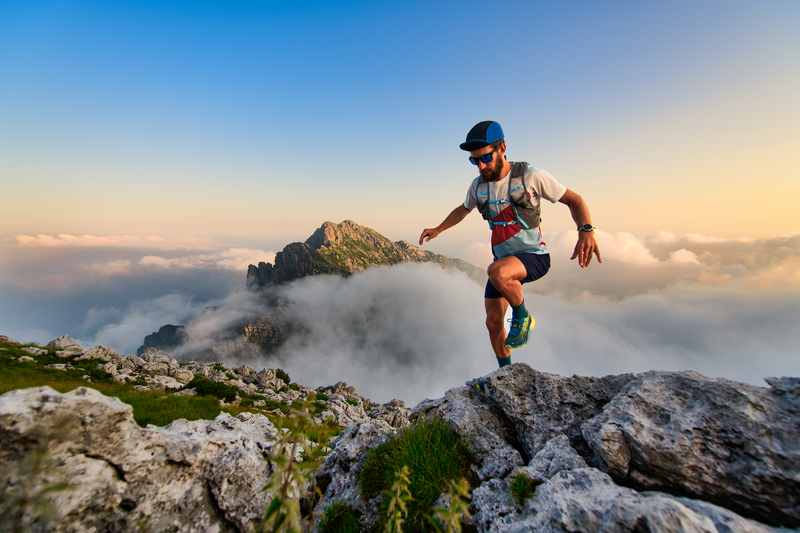
95% of researchers rate our articles as excellent or good
Learn more about the work of our research integrity team to safeguard the quality of each article we publish.
Find out more
MINI REVIEW article
Front. Tuberc. , 15 November 2023
Sec. Immunological Basis of Tuberculosis
Volume 1 - 2023 | https://doi.org/10.3389/ftubr.2023.1303505
Vaccination is crucial for the control of tuberculosis (TB), and safe, more effective, and accessible vaccines against Mycobacterium tuberculosis (Mtb) infection are critically needed to achieve TB control milestones envisioned in the End TB Strategy. TB vaccine research and development faces numerous challenges including, but not limited to, insufficient knowledge of the most informative antigens to prioritize as potential vaccine candidates, lack of defined correlates of protection, and incomplete knowledge of anatomical and cellular locations of the Mtb-infected cell in vivo, among others. To take stock of the progress, challenges, and opportunities in TB vaccine R&D, the Stop TB Partnership Working Group on New TB Vaccines (WGNV), in partnership with the National Institute of Allergy and Infectious Diseases (NIAID) cohosted a two-day virtual workshop on 13–14 June 2023 with experts from all over the world. In this report, we summarize key themes and discussions from the meeting, highlighting progress and gaps in the TB vaccine research.
Under the theme “Recognition and control of Mycobacterium tuberculosis (Mtb)-infected cells: from basics to the clinic,” the objectives were to: (i) Elucidate the mechanisms by which the immune system recognizes Mtb-infected cell, (ii) Explore when, where, how, and the degree to which recognition can lead to control of the intracellular microbe, (iii) Discuss the translational implications of current developments in the field, and (iv) Foster discussions to address gaps in our understanding of the immune response to Mtb, to enable identification of correlates of protection and development of novel interventions like new TB vaccines.
The first session explored mechanisms by which the host immune system recognizes the Mtb-infected cell. Paul Ogongo (University of California San Francisco) discussed the evidence for disease-specific antigens. Ogongo shared a report by Musvosvi et al. (1), which identified antigenic peptides targeted by T-cell receptor similarity groups associated with control or progression, demonstrating that distinct Mtb antigens are associated with infection outcomes. In a second report by Meier et al. (2) cytokine responses to distinct Mtb antigens differentiated TB progressors from non-progressors 2 years prior to TB progression. Finally, Ogongo described his unpublished work in the Ernst Lab, illustrating immunodominant Mtb antigens that have conserved T-cell epitopes induced a predominant Th1 response, while rare Mtb antigens with variable T-cell epitopes were skewed toward Th17 responses. Overall, Ogongo highlighted that distinct Mtb antigens are associated with infection outcomes, induce functional responses that precede progression to TB disease, and can determine human T-cell differentiation.
Next, Sam Behar (University of Massachusetts Chan Medical School), highlighted evidence suggesting that poor T-cell recognition of infected cells is a barrier to protective immunity. T-cell recognition of Mtb-infected cells may depend on the number of bacteria or the amount of antigen present (3), and further evidence indicates murine Mtb-specific CD8 T-cells only recognize infected macrophages with high bacterial burden (4). Thus, high intracellular numbers of Mtb may induce macrophage death, release of bacterial antigens or exosome production, leading to acquisition of antigens and cellular debris by uninfected bystander cells that can cross-present antigens. It is therefore important to understand how antigen presentation by bystander antigen presenting cells (APC) and the consequent T-cell activation affect disease pathogenesis.
Cecilia Lindestam Arlehamn (La Jolla Institute for Immunology), finally highlighted differentially recognized T-cell epitopes in the spectrum of Mtb infection. Lindestam Arlehamn et al. (5) identified ~400 CD4 T-cell epitopes in IGRA+ individuals, with vast heterogeneity of epitope-specific responses to Mtb. Recently, Panda et al. (6) provided evidence for differences in T-cell reactivity against Mtb antigens between TB active and IGRA+ individuals. Using longitudinal cohorts and sequential sampling, future investigations should determine when T-cells begin to respond to these differentially recognized peptide antigens and potentially identify signatures of progression and correlates of protection. The anatomical location of differentially recognized antigens, species specificity, and differences in the antigenic repertoire within an individual are unknown. Furthermore, HLA diversity as a source of heterogeneity should be investigated to identify HLA alleles that are associated with TB disease or contained Mtb infection.
During the panel discussion, it was appreciated that TB vaccines are largely based on secreted protein antigens. It was suggested that secreted Mtb antigens are likely processed via class II and class I pathways, although only some of these antigens are preferentially taken up by bystander cells. Since certain antigens are more likely to be presented or secreted by an infected cell, multiple antigens in TB vaccines are necessary to confer protective immunity. The tissue microenvironments were also discussed as important for shaping immune responses with regard to antigen presentation and recognition. The granuloma has different microenvironments with cells in different immune states that influence patterns of antigen expression, which ultimately need to be considered and further explored in vivo. Since the field lacks a comprehensive understanding of where Mtb persists in humans, consideration of alternate concepts of where Mtb persists (anatomic and cellular locations) has important implications for vaccine design and delivery. Consequently, mechanisms of extracellular bacteria killing via cytotoxic effectors need consideration. Together, the complexities of tissue microenvironments, Mtb strain diversity, and Mtb location remain bottlenecks to experimentally evaluate antigen recognition in vivo.
The second session focused on immune control of intracellular infection. Mihai Netea (Radboud University Medical Center) presented on BCG-induced trained immunity that elicits an antigen-agnostic response through mechanisms involving immunological pathways, metabolic rewiring, and epigenetic processes in responding cells. BCG-induced control of Mtb infection is associated with enhanced myeloid- and innate-associated responses (7). Regarding trained immunity, Moorlag et al. (8) showed that administration of β-glucan prior to Mtb infection induced higher functional responses by monocytes in vitro, reduced bacterial burden in vitro and in vivo, and conferred longer survival in infected mice. During the panel discussion, it was noted that the superior efficacy of intravenous BCG (9–11) may be due to the effect of the vaccine on bone marrow progenitor cells (central trained immunity). Importantly, trained immunity involves innate cells beyond myeloid subsets, including non-conventional T-cells, NK cells, and other innate lymphoid cells (ILCs). Trained immunity is improved when there is interaction with the cells of the adaptive immune system which secrete IFN-γ to amplify trained immunity. The panel noted that natural mucosal immunity demonstrates “tolerance-associated” features. For example, lung NK cells generally lack evidence of activation as a protective mechanism to prevent inflammation-induced pathology [reviewed in Cong and Wei (12)]. Therefore, TB vaccine research should consider harnessing the combined induction of classical adaptive immunity and trained immunity in novel vaccination strategies to elicit responses distinct from mechanisms favoring tolerance in the tissues.
The second speaker, David Lewinsohn (Oregon Health and Science University), presented data and efforts aimed at addressing a fundamental: Where is Mtb located in the human host? Lewinsohn proposed that studies should consider vaccine-induced responses in the airways, since favorable airway immunity may provide early control and facilitate the development of adaptive immunity (13). Non-classical, MR1-restricted, Mtb-reactive CD8+ T-cells (mucosal-associated invariant T-cells - MAIT) were enriched in the upper airways and efficiently enhanced IFN-γ production in response to Mtb-infected epithelial cells (14), suggesting that although epithelial cells are generally poorly infected by Mtb, they may provide a niche for Mtb persistence. Lewinsohn also presented recent evidence demonstrating that T-cell functionality, rather than phenotype, is associated with Mtb restriction in the non-human primates (NHP) granuloma (15), and polycytotoxic T-cells can control Mtb (16–18). Thus, new vaccines and the analysis of vaccine responses should consider the cytolytic capacity of vaccine-induced responder cells and the cytolytic molecules that restrict Mtb growth.
Finally, Henry Mwandumba (Malawi Liverpool Wellcome Programme), focused on how innate immune cells in the human lung shape responses to Mtb infection and explored reprogramming of the lung myeloid compartment to prevent the establishment of infection. Boosting early innate responses in the lung may be necessary to limit Mtb growth and clear initial infection (19). Upon Mtb exposure, different myeloid cell populations in the lung are infected by Mtb (20, 21), some of which are effective at controlling infection, while others provide a niche for Mtb persistence. However, permissive cells contributing toward the establishment of infection are not well characterized and there is limited data on bacterial permissiveness as an immune function. There is a need for identifying correlates of bacterial permissiveness, in addition to immune correlates of protection and/or risk. Mwandumba then discussed macrophage ontogeny as the primary underpinning to macrophage-associated control of Mtb infection in the lung, as demonstrated by Huang and colleagues (21). Specifically, the ability to control Mtb infection was determined by the metabolic profile of macrophages: glycolysis predominated in the restrictive recruited interstitial macrophages, while fatty acid oxidation was the dominant metabolic pathway of permissive alveolar macrophages in the murine lung. Thus, the evidence suggests that epigenetic programming and metabolic rewiring of tissue-resident macrophage lineages by new TB vaccines could be a novel strategy to impact Mtb infection, control, and persistence in humans.
Relevent to TB immunity in the lung, it was recently (22) demonstrated that tissue-specific activation of T-cells influences their effector functions. Specifically, IFN-γ in the mediastinal lymph node skewed T-cells to be Th1-like effector regulatory T-cells driving their close interaction with type 1 dendritic cells (DCs) and subsequent suppression of cytotoxic T-cell responses. It is worth considering whether a similar mechanism applies during TB. Studying the lung airways provides an opportunity to understand early events occurring upon exposure to the bacteria. To address the limitation of obtaining human lung tissue samples, it is possible to leverage computational approaches to infer the interactions in the alveolar space to the lung parenchyma.
The third session fostered discussions on translating basic findings into clinical settings. Stephen Carpenter (Case Western Reserve University) highlighted the possible causes and mechanisms of delayed memory T-cell responses to infected cells in the lung. T-cells are detected in the lungs ~2 weeks following aerosol infection (23–25). However, many of the antigen-specific CD4 T-cells in the lungs do not produce IFN-γ following infection (26). Carpenter proposed that there may be a lack of T-cell recognition of infected alveolar macrophages, which accounts for nearly all Mtb-infected cells during this initial period (27, 28). Mtb-infected macrophages can evade T-cell recognition using Type I and Type II evasion. General inhibition of T-cell recognition (Type I evasion) occurs independent of TCR/antigen specificity and results from inhibitory cytokines or impaired pro-inflammatory cytokine and co-stimulatory receptor expression by Mtb-infected macrophages (29, 30). On the other hand, Type II evasion of T-cell recognition is TCR-dependent and/or antigen-specific (31). During Type II evasion, TCR/antigen-specific recognition can be hindered by Mtb changing its patterns of antigen expression, antigen export from infected to non-infected cells (32), or altered antigen repertoires presented by dendritic cells (DCs) during T-cell priming, which may be different from peptides presented by infected macrophages in the lung. Indeed, some studies have identified TCRs that were unable to recognize infected macrophages and were limited in their ability to control bacterial infection in mice [reviewed in Yang et al. (33)] and in human vaccination studies (34). Finally, Carpenter presented data from his lab showing that the addition of Mtb antigens to Mtb-infected macrophages can increase the number of activated human CD4 T-cells in some individuals. Using TCR sequencing, certain clonally-expanded TCRs were identified as unique to the addition of exogenous antigens, providing further evidence of the antigen-specific mechanisms that may govern Type II evasion of T-cell recognition in humans.
Next, Munyaradzi Musvosvi (South African Tuberculosis Vaccine Initiative, University of Cape Town), focused on the use of single-cell TCR sequencing of Mtb-specific T-cells to identify priority antigens for TB vaccine development. Bertholet et al. (34) demonstrated that distinct Mtb antigens varied in their ability to confer a protective response against Mtb. Antigen availability can determine the quality of T-cell response (35). For example, antigens expressed at high levels throughout the course of Mtb infection (e.g., ESAT-6) drive terminal differentiation of T-cells, while antigens poorly expressed during the chronic phase of infection (e.g., Ag85B) result in less differentiated T-cells (35). Recently, Musvosvi clustered Mtb–specific T-cells into TCR similarity groups based on shared amino acid motifs within CDR3β chains and identified groups enriched in either progressors or controllers, in two longitudinal cohorts (1) (also highlighted by Ogongo in his talk). Further studies using a reporter system to perform Mtb genome-wide protein screening (36) identified the cognate epitopes to some of the similarity groups enriched in controllers and progressors, suggesting the existence of “good” and “bad” antigens (37, 38). Musvosvi, therefore, presented a platform for identifying antigens to prioritize for TB vaccine R&D.
Chetan Seshadri (University of Washington) closed the session by focusing on protection from the perspective of the human infection model. Seshadri highlighted the concept of “resistors,” who did not develop evidence of infection or active disease, despite close contact with active TB cases (39), but exhibit immune responses to Mtb antigens that were IFN-γ-independent (40). Seshadri then discussed that the “non-conventional” immune response to Mtb is mediated, partly, by donor-unrestricted T-cells (DURTs) (41), including MR1-restricted T-cells, which recognize non-peptide antigens (42). Several studies suggest that DURTs may provide help to other immune cells, rather than acting as primary effectors (43–45). Seshadri proposed that antigens recognized by DURTs should be validated and included in pre-clinical and clinical development. For vaccination strategies, it is worth considering whether DURTs can be engineered to induce an effector response and adaptive immunity and whether those responses can be Mtb-specific. Seshadri suggested that developing a controlled human Mtb infection model could be considered for evaluating in situ T-cell clonotypic expansions to discover new antigens for TB vaccines using approaches developed by Munyaradzi and colleagues.
IFN-γ-independent responses to Mtb antigens was discussed at length during open forum. While quantifying IFN-γ remains informative and crucial, other cytokine responses to various Mtb antigens also provide valuable readouts. Since there is overlap between antigens and antigen specificity, a combination of immune response readouts and inclusion of multiple antigens should be considered in future vaccine studies. The existence of Mtb strain-specific transcriptional changes in infected macrophages has been reported (46), and Mtb strains are differentially recognized by toll-like receptors (TLRs) with an impact on the immune response (47). Furthermore, human macrophage responses to clinical isolates from Mtb complex can discriminate between ancient and modern lineages (48). Thus, it is possible that Mtb strain-specific immune responses could inform whether prior exposure favors subsequent immune responses that determine the clinical outcome. The panel discussion also considered the types of antigens used in immunological assays and how, for example, Mtb lysates and peptide pools differentially influence the type of cells that respond in recall assays. Therefore, it is imperative to identify signatures that encompass a holistic response and include more immune cells involved in immunity to TB beyond conventional T-cells. Overall, there is a need to invest in systems that integrate various mechanisms for detecting novel antigens and exploring existing antigens, while evaluating the harmonized and interlinked role of immune cells in containing Mtb infection.
The last session focused on issues regarding the development of clinical correlates that reflect recognition of the infected cell. Bryan Bryson (Massachusetts Institute of Technology) presented FucoID as a technology for identifying antigen-specific T-cells and cell-cell interactions and discussed the potential of this technique as an unbiased approach. With FucoID technology, the fucosyltransferase (FT)-labeled (49) Mtb-infected cell acts as a “living tetramer,” which in turn, labels interacting T-cells. Paired with technologies such as CITEseq, FucoID enables phenotypic characterization of Mtb-specific T-cells. Potentially all cells interacting with labeled, Mtb-infected cells could be identified and used to determine the proportion of vaccine-induced responses. Such tools could also be employed for understanding early immune interactions occurring in the lung airways following Mtb exposure.
Simone Joosten (Leiden University Medical Center) presented on growth inhibition assays as a correlate of protection against TB. The Mycobacterial Growth Inhibition Assay (MGIA) provides a measure of growth control by immune cells and gives insights into mechanisms associated with protective immune responses against Mtb infection (7). New data from Joosten's group (in revision), found that MGIA identified groups of individuals who could or could not control growth of BCG pre- or post-vaccination. Moreover, mechanisms of control may be different between natural and vaccine-induced protection. Since functional measurements of effector responses are important to evaluate protective immunity, improvement of the MGIA platform will provide novel insights into host defense mechanisms associated with control.
The last speaker of this session was Jacqueline Achkar (Albert Einstein College of Medicine) who spoke on protective antigen specificity of antibodies and B cells in TB. Several studies have provided clear evidence for antibody-mediated immunity against TB, including FcγR-mediated effects but knowledge on protective B cell antigens remains limited (50–56). Recent mucosal and intravenous BCG vaccine studies with NHPs showed associations of airway IgA, IgG, and IgM with protection against TB disease (9, 57, 58), highlighting the potential protective role of antibodies and B cells in the lung during initial Mtb exposure and infection [reviewed in Boom et al. (59)]. Ishida and colleagues (Achkar lab, in press) have identified IgA responses to epitopes of the Mtb surface glycan arabinomannan prior to Mtb infection that were associated with the outcome of controlled (LTBI) vs. TB disease outcome in cynomolgus macaques, suggesting that pre-existing antibodies can protect against TB. However, despite the evidence for a protective role of antibodies and B cells in TB, major knowledge gaps, such as the following, remain: (i) The range of protective Mtb antigens and their epitopes, including their expression on infected cells; (ii) Differences between mucosal and systemic antibodies and their mechanisms of protection; (iii) The role of antibody isotypes at different stages of Mtb infection; and (iv) The role of B cells, independent of antibodies, and their interaction with other immune cells. During the panel discussion, the role of antibodies was discussed in terms of their contributions toward the formation of immune complexes that trigger complement activation, which has been identified as a correlate of risk in TB progressors and TB patients. However, it remains to be determined which specific antibody:antigen complexes contribute toward complement activation and their associations with disease outcome. Finally, exposure to non-tuberculous mycobacteria (NTM) and Mtb strains with different virulence and expressed antigenic repertoires were discussed as potential contributing factors toward the heterogeneous effects of B cell and antibody responses.
A comprehensive understanding of immune recognition and control of the Mtb-infected cell is required to accelerate vaccine development against TB. There is no consensus on the definition of a functional profile that infers a protective immune response to Mtb in humans. Furthermore, whether a functional profile is determined by the host or driven by the Mtb antigens themselves, or both is unresolved. These issues are relevant for vaccine design, where immunogenic antigens are intended to induce protective (long-lasting) immunity. However, in the absence of a functional readout reflecting a protective profile in humans, it is challenging to infer which antigens are ideal for inclusion in novel vaccine candidates. We propose that investing more research into developing correlates of protection would be greatly beneficial and impactful. We also propose that vaccine trials include an array of readouts, in addition to efficacy and T-cell immunogenicity endpoints. For example, bacterial killing to evaluate bacilli clearance by effector cells and quantifying functional responses beyond conventional cytokines and T-cells. The use of spatial technologies holds potential for identifying vaccines with protective efficacy for development of clinical correlates reflecting recognition of the infected cell. A major theme of the workshop was to explore T-cell subsets beyond CD4 T-cell recognition. In this regard, other T-cell subsets, such as CD8 T-cells and DURTs, require more investigation into their antigen recognition capabilities to discover novel antigens for inclusion as potential vaccines.
Human TB research relies on studying immune responses in circulation by flow-based assays. While valuable, these studies are limited by their inability to mirror the immune responses at the site of disease. Furthermore, the knowledge of the epitopes displayed by Mtb-infected cell is limited, thus, there is a need for better measurements of these responses. Sampling broncho-alveolar lavage (BAL) fluid enables studying TB immunology in the airways and alveolar space but interactions in the airways do not fully represent the lung parenchyma. Although sampling human tissues is not possible in a clinical setting, postmortem tissue sampling offers alternatives. Multi-omics approaches in humans and NHPs hold great potential to develop tools that accurately infer interactions and responses to bridge the gap between the periphery, airways, and parenchyma in humans. Finally, since well-characterized human cohorts are fundamental to vaccine research, studies that include overlap between different stages of infection and disease are important to capture the spectrum of TB disease, and such studies should integrate clinical data to capture the variability between cohorts and participants. Additional studies should consider development of controlled human infection models to provide insights into variability observed in Mtb infection outcomes. Thus, the key bottlenecks in advancing TB vaccine research are the absence of immune correlates of protection for validation of vaccine candidates in development and the lack of knowledge of where Mtb is located the human host. However, opportunities exist to accelerate efforts to develop better TB vaccines through computational approaches that enable projection of observations in animal models into humans and the use of multi-species approach to identify correlates of protection.
CY: Writing – original draft, Writing – review & editing. MNM: Writing – original draft, Writing – review & editing. PO: Writing – original draft, Writing – review & editing.
The author(s) declare that no financial support was received for the research, authorship, and/or publication of this article.
We would like to thank the National Institute of Allergy and Infectious Diseases (NIAID) and the Stop TB Partnership Working Group on New TB Vaccines (WGNV) for hosting the workshop. We thank the session co-chairs: CY, David Lewinsohn, Sara Suliman, Nelita du Plessis, MNM, Nicole Frahm, Virginie Rozot, and Willem Hanekom for moderating the sessions and guiding the open forum discussions. We thank the speakers for their contributions and for reviewing the sections of their presentations, and the participants for contributing to the discussions. Special gratitude to the planning committee for their contribution to organizing and developing the workshop program and for their review of this report: David Lewinsohn [Stop TB Partnership Working Group on New TB Vaccines (WGNV) and Oregon Health and Science University], Katrin Eichelberg (NIAID-NIH), and Jennifer Woolley (WGNV).
The authors declare that the research was conducted in the absence of any commercial or financial relationships that could be construed as a potential conflict of interest.
All claims expressed in this article are solely those of the authors and do not necessarily represent those of their affiliated organizations, or those of the publisher, the editors and the reviewers. Any product that may be evaluated in this article, or claim that may be made by its manufacturer, is not guaranteed or endorsed by the publisher.
The content is solely the responsibility of the authors and does not represent the views of the NIH or the HHWF.
1. Musvosvi M, Huang H, Wang C, Xia Q, Rozot V, Krishnan A, et al. T cell receptor repertoires associated with control and disease progression following Mycobacterium tuberculosis infection. Nat Med. (2023) 29:258–69. doi: 10.1038/s41591-022-02110-9
2. Meier NR, Battegay M, Ottenhoff TH, Furrer H, Nemeth J, Ritz N, et al. HIV-infected patients developing tuberculosis disease show early changes in the immune response to novel Mycobacterium tuberculosis antigens. Front Immunol. (2021) 12:620622. doi: 10.3389/fimmu.2021.620622
3. Lu YJ, Barreira-Silva P, Boyce S, Powers J, Cavallo K, Behar SM, et al. CD4 T cell help prevents CD8 T cell exhaustion and promotes control of Mycobacterium tuberculosis infection. Cell Rep. (2021) 36:109696. doi: 10.1016/j.celrep.2021.109696
4. Mott D, Yang J, Baer C, Papavinasasundaram K, Sassetti CM, Behar SM, et al. High bacillary burden and the ESX-1 Type VII secretion system promote MHC class I Presentation by Mycobacterium tuberculosis–infected macrophages to CD8 T cells. J Immunol. (2023) 210:1531–42. doi: 10.4049/jimmunol.2300001
5. Lindestam Arlehamn CS, Gerasimova A, Mele F, Henderson R, Swann J, Greenbaum JA, et al. Memory T cells in latent Mycobacterium tuberculosis infection are directed against three antigenic islands and largely contained in a CXCR3+ CCR6+ Th1 subset. PLoS Pathog. (2013) 9:e1003130. doi: 10.1371/journal.ppat.1003130
6. Panda S, Morgan J, Cheng C, Saito M, Gilman RH, Ciobanu N, et al. Identification of differentially recognized T cell epitopes in the spectrum of Mtb infection. bioRxiv [Preprint]. (2023). doi: 10.1101/2023.04.12.536550
7. Joosten SA, van Meijgaarden KE, Arend SM, Prins C, Oftung F, Korsvold GE, et al. Mycobacterial growth inhibition is associated with trained innate immunity. The J Clin Inv. (2018) 128:1837–51. doi: 10.1172/JCI97508
8. Moorlag SJ, Khan N, Novakovic B, Kaufmann E, Jansen T, van Crevel R, et al. β-Glucan induces protective trained immunity against Mycobacterium tuberculosis infection: a key role for IL-1. Cell Rep. (2020) 31:107634. doi: 10.1016/j.celrep.2020.107634
9. Darrah PA, Zeppa JJ, Maiello P, Hackney JA, Wadsworth MH, Hughes TK, et al. Prevention of tuberculosis in macaques after intravenous BCG immunization. Nature. (2020) 577:95–102. doi: 10.1038/s41586-019-1817-8
10. Darrah PA, Zeppa JJ, Wang C, Irvine EB, Bucsan AN, Rodgers MA, et al. Airway T cells are a correlate of IV Bacille Calmette-Guerin-mediated protection against tuberculosis in rhesus macaques. Cell Host Microbe. (2023) 12:6. doi: 10.1016/j.chom.2023.05.006
11. Liu YE, Darrah PA, Zeppa JJ, Kamath M, Laboune F, Douek DC, et al. Blood transcriptional correlates of BCG-induced protection against tuberculosis in rhesus macaques. Cell Rep Med. (2023) 4:101096. doi: 10.1016/j.xcrm.2023.101096
12. Cong J, Wei H. Natural killer cells in the lungs. Front Immunol. (2019) 10:1416. doi: 10.3389/fimmu.2019.01416
13. Nyendak M, Swarbrick GM, Duncan A, Cansler M, Huff EW, Hokey D, et al. Adenovirally-induced polyfunctional T cells do not necessarily recognize the infected target: lessons from a phase I trial of the AERAS-402 vaccine. Sci Rep. (2016) 6:36355. doi: 10.1038/srep36355
14. Harriff MJ, Cansler ME, Toren KG, Canfield ET, Kwak S, Gold MC, et al. Human lung epithelial cells contain Mycobacterium tuberculosis in a late endosomal vacuole and are efficiently recognized by CD8+ T cells. PLoS One. (2014) 9:e97515. doi: 10.1371/journal.pone.0097515
15. Gideon HP, Hughes TK, Tzouanas CN, Wadsworth MH, Tu AA, Gierahn TM, et al. Multimodal profiling of lung granulomas in macaques reveals cellular correlates of tuberculosis control. Immunity. (2022) 55:827–46. doi: 10.1016/j.immuni.2022.04.004
16. Spencer CT, Abate G, Sakala IG, Xia M, Truscott SM, Eickhoff CS, et al. Granzyme A produced by γ9δ2 T cells induces human macrophages to inhibit growth of an intracellular pathogen. PLoS Pathog. (2013) 9:e1003119. doi: 10.1371/journal.ppat.1003119
17. van Meijgaarden KE, Haks MC, Caccamo N, Dieli F, Ottenhoff TH, Joosten SA, et al. Human CD8+ T-cells recognizing peptides from Mycobacterium tuberculosis (Mtb) presented by HLA-E have an unorthodox Th2-like, multifunctional, Mtb inhibitory phenotype and represent a novel human T-cell subset. PLoS Pathog. (2015) 11:e1004671. doi: 10.1371/journal.ppat.1004671
18. Busch M, Herzmann C, Kallert S, Zimmermann A, Höfer C, Mayer D, et al. Lipoarabinomannan-responsive polycytotoxic T cells are associated with protection in human tuberculosis. Am J Resp Crit Care Med. (2016) 194:345–55. doi: 10.1164/rccm.201509-1746OC
19. North RJ, Jung YJ. Immunity to tuberculosis. Annu Rev Immunol. (2004) 22:599–623. doi: 10.1146/annurev.immunol.22.012703.104635
20. Srivastava S, Ernst JD, Desvignes L. Beyond macrophages: the diversity of mononuclear cells in tuberculosis. Immunol Rev. (2014) 262:179–92. doi: 10.1111/imr.12217
21. Huang L, Nazarova EV, Tan S, Liu Y, Russell DG. Growth of Mycobacterium tuberculosis in vivo segregates with host macrophage metabolism and ontogeny. J Exp Med. (2018) 215:1135–52. doi: 10.1084/jem.20172020
22. Zagorulya M, Yim L, Morgan DM, Edwards A, Torres-Mejia E, Momin N, et al. Tissue-specific abundance of interferon-gamma drives regulatory T cells to restrain DC1-mediated priming of cytotoxic T cells against lung cancer. Immunity. (2023) 56:386–405. doi: 10.1016/j.immuni.2023.01.010
23. Chackerian AA, Alt JM, Perera TV, Dascher CC, Behar SM. Dissemination of Mycobacterium tuberculosis is influenced by host factors and precedes the initiation of T-cell immunity. Inf Immun. (2002) 70:4501–9. doi: 10.1128/IAI.70.8.4501-4509.2002
24. Wolf AJ, Desvignes L, Linas B, Banaiee N, Tamura T, Takatsu K, et al. Initiation of the adaptive immune response to Mycobacterium tuberculosis depends on antigen production in the local lymph node, not the lungs. The J Exp Med. (2008) 205:105–15. doi: 10.1084/jem.20071367
25. Urdahl KB, Shafiani S, Ernst JD. Initiation and regulation of T-cell responses in tuberculosis. Mucosal Immunol. (2011) 4:288–93. doi: 10.1038/mi.2011.10
26. Bold TD, Banaei N, Wolf AJ, Ernst JD. Suboptimal activation of antigen-specific CD4+ effector cells enables persistence of M. tuberculosis in vivo. PLoS Pathog. (2011) 7:e1002063. doi: 10.1371/journal.ppat.1002063
27. Cohen SB, Gern BH, Delahaye JL, Adams KN, Plumlee CR, Winkler JK, et al. Alveolar macrophages provide an early Mycobacterium tuberculosis niche and initiate dissemination. Cell Host Microbe. (2018) 24:439–46. doi: 10.1016/j.chom.2018.08.001
28. Rothchild AC, Olson GS, Nemeth J, Amon LM, Mai D, Gold ES, et al. Alveolar macrophages generate a noncanonical NRF2-driven transcriptional response to Mycobacterium tuberculosis in vivo. Sci Immunol. (2019) 4:eaaw. doi: 10.1126/sciimmunol.aaw6693
29. Gern BH, Adams KN, Plumlee CR, Stoltzfus CR, Shehata L, Moguche AO, et al. TGFβ restricts expansion, survival, and function of T cells within the tuberculous granuloma. Cell Host Microbe. (2021) 29:594–606. doi: 10.1016/j.chom.2021.02.005
30. Gail DP, Suzart VG, Du W, Sandhu AK, Jarvela J, Nantongo M, et al. Mycobacterium tuberculosis impairs human memory CD4+ T cell recognition of M2 but not M1-like macrophages. Iscience. (2023) 26:107706. doi: 10.1016/j.isci.2023.107706
31. Yang H, Wallace Z, Dorrell L. Therapeutic targeting of HIV reservoirs: how to give T cells a new direction. Front Immunol. (2018) 9:2861. doi: 10.3389/fimmu.2018.02861
32. Srivastava S, Grace PS, Ernst JD. Antigen export reduces antigen presentation and limits T cell control of M. tuberculosis Cell Host Microbe. (2016) 19:44–54. doi: 10.1016/j.chom.2015.12.003
33. Yang JD, Mott D, Sutiwisesak R, Lu YJ, Raso F, Stowell B, et al. Mycobacterium tuberculosis-specific CD4+ and CD8+ T cells differ in their capacity to recognize infected macrophages. PLoS Pathog. (2018) 14:e1007060. doi: 10.1371/journal.ppat.1007060
34. Bertholet S, Ireton GC, Kahn M, Guderian J, Mohamath R, Stride N, et al. Identification of human T cell antigens for the development of vaccines against Mycobacterium tuberculosis. The J Immunol. (2008) 181:7948–57. doi: 10.4049/jimmunol.181.11.7948
35. Moguche AO, Musvosvi M, Penn-Nicholson A, Plumlee CR, Mearns H, Geldenhuys H, et al. Antigen availability shapes T cell differentiation and function during tuberculosis. Cell Host Microbe. (2017) 21:695–706. doi: 10.1016/j.chom.2017.05.012
36. Huang H, Wang C, Rubelt F, Scriba TJ, Davis MM. Analyzing the Mycobacterium tuberculosis immune response by T-cell receptor clustering with GLIPH2 and genome-wide antigen screening. Nat Biotechnol. (2020) 38:1194–202. doi: 10.1038/s41587-020-0505-4
37. Ogongo P, Ernst JD. Finding antigens for TB vaccines: the good, the bad and the useless. Nat Med. (2023) 29:35–6. doi: 10.1038/s41591-022-02123-4
38. Pradhan A, Vorkas CK. T cells target TB. Cell Host Microbe. (2023) 31:329–30. doi: 10.1016/j.chom.2023.02.003
39. Stein CM, Nsereko M, Malone LL, Okware B, Kisingo H, Nalukwago S, et al. Long-term stability of resistance to latent Mycobacterium tuberculosis infection in highly exposed tuberculosis household contacts in Kampala, Uganda. Clin Inf Dis. (2019) 68:1705–12. doi: 10.1093/cid/ciy751
40. Lu LL, Smith MT, Yu KK, Luedemann C, Suscovich TJ, Grace PS, et al. IFN-γ-independent immune markers of Mycobacterium tuberculosis exposure. Nat Med. (2019) 25:977–87. doi: 10.1038/s41591-019-0441-3
41. Van Rhijn I, Moody D. Donor unrestricted T cells: a shared human T cell response. The J Immunol. (2015) 195:1927–32. doi: 10.4049/jimmunol.1500943
42. Cross DL, Layton ED, Yu KK, Smith MT, Aguilar MS, Li S, et al. MR1.-restricted T cell clonotypes are associated with ‘resistance' to M. tuberculosis infection. bioRxiv. (2022). doi: 10.1101/2022.10.12.511825
43. Zaidi I, Diallo H, Conteh S, Robbins Y, Kolasny J, Orr-Gonzalez S, et al. γδ T cells are required for the induction of sterile immunity during irradiated sporozoite vaccinations. The J Immunol. (2017) 199:3781–8. doi: 10.4049/jimmunol.1700314
44. Gaya M, Barral P, Burbage M, Aggarwal S, Montaner B, Navia AW, et al. Initiation of antiviral B cell immunity relies on innate signals from spatially positioned NKT cells. Cell. (2018) 172:517–33. doi: 10.1016/j.cell.2017.11.036
45. Jensen O, Trivedi S, Meier JD, Fairfax KC, Hale JS, Leung DT, et al. A subset of follicular helper-like MAIT cells can provide B cell help and support antibody production in the mucosa. Sci Immunol. (2022)7: eabe. doi: 10.1126/sciimmunol.abe8931
46. Koo MS, Subbian S, Kaplan G. Strain specific transcriptional response in Mycobacterium tuberculosis infected macrophages. Cell Commun Signal. (2012) 10:1–15. doi: 10.1186/1478-811X-10-2
47. Carmona J, Cruz A, Moreira-Teixeira L, Sousa C, Sousa J, Osorio NS, et al. Mycobacterium tuberculosis strains are differentially recognized by TLRs with an impact on the immune response. PLoS ONE. (2013) 8:e67277. doi: 10.1371/journal.pone.0067277
48. Portevin D, Gagneux S, Comas I, Young D. Human macrophage responses to clinical isolates from the Mycobacterium tuberculosis complex discriminate between ancient and modern lineages. PLoS Pathog. (2011) 7:e1001307. doi: 10.1371/journal.ppat.1001307
49. Liu Z, Li JP, Chen M, Wu M, Shi Y, Li W, et al. Detecting tumor antigen-specific T cells via interaction-dependent fucosyl-biotinylation. Cell. (2020) 183:1117–33. doi: 10.1016/j.cell.2020.09.048
50. Chen T, Blanc C, Eder AZ, Prados-Rosales R, Souza ACO, Kim RS, et al. Association of human antibodies to arabinomannan with enhanced mycobacterial opsonophagocytosis and intracellular growth reduction. The J Inf Dis. (2016) 214:300–10. doi: 10.1093/infdis/jiw141
51. Chen T, Blanc C, Liu Y, Ishida E, Singer S, Xu J, et al. Capsular glycan recognition provides antibody-mediated immunity against tuberculosis. The J Clin Invest. (2020) 130:1808–22. doi: 10.1172/JCI128459
52. Lu LL, Chung AW, Rosebrock TR, Ghebremichael M, Yu WH, Grace PS, et al. A functional role for antibodies in tuberculosis. Cell. (2016) 167:433–43. doi: 10.1016/j.cell.2016.08.072
53. Li H, Wang XX, Wang B, Fu L, Liu G, Lu Y, et al. Latently and uninfected healthcare workers exposed to TB make protective antibodies against Mycobacterium tuberculosis. Proc Nat Acad Sci. (2017) 114:5023–8. doi: 10.1073/pnas.1611776114
54. Prados-Rosales R, Carreño L, Cheng T, Blanc C, Weinrick B, Malek A, et al. Enhanced control of Mycobacterium tuberculosis extrapulmonary dissemination in mice by an arabinomannan-protein conjugate vaccine. PLoS Pathog. (2017) 13:e1006250. doi: 10.1371/journal.ppat.1006250
55. Watson A, Li H, Ma B, Weiss R, Bendayan D, Abramovitz L, et al. Human antibodies targeting a Mycobacterium transporter protein mediate protection against tuberculosis. Nat Commun. (2021) 12:602. doi: 10.1038/s41467-021-20930-0
56. Liu Y, Chen T, Zhu Y, Furey A, Lowary TL, Chan J, et al. Features and protective efficacy of human monoclonal antibodies targeting Mycobacterium tuberculosis arabinomannan. JCI Insight. (2023) 12:e167960. doi: 10.1172/jci.insight.167960
57. Dijkman K, Sombroek CC, Vervenne RA, Hofman SO, Boot C, Remarque EJ, et al. Prevention of tuberculosis infection and disease by local BCG in repeatedly exposed rhesus macaques. Nature Med. (2019) 25:255–62. doi: 10.1038/s41591-018-0319-9
58. Irvine EB, O'Neil A, Darrah PA, Shin S, Choudhary A, Li W, et al. Robust IgM responses following intravenous vaccination with Bacille Calmette–Guérin associate with prevention of Mycobacterium tuberculosis infection in macaques. Nat Immunol. (2021) 22:1515–23. doi: 10.1038/s41590-021-01066-1
Keywords: Mycobacterium tuberculosis, recognition, control, infected cell, immunity, vaccines, antigens
Citation: Young C, Mkhonza MN and Ogongo P (2023) Recognition and control of Mycobacterium tuberculosis-infected cells: from basics to the clinic: a NIAID/WGNV workshop report 2023. Front. Tuberc. 1:1303505. doi: 10.3389/ftubr.2023.1303505
Received: 28 September 2023; Accepted: 25 October 2023;
Published: 15 November 2023.
Edited by:
Vishwanath Venketaraman, Western University of Health Sciences, United StatesReviewed by:
Alissa Rothchild, University of Massachusetts Amherst, United StatesCopyright © 2023 Young, Mkhonza and Ogongo. This is an open-access article distributed under the terms of the Creative Commons Attribution License (CC BY). The use, distribution or reproduction in other forums is permitted, provided the original author(s) and the copyright owner(s) are credited and that the original publication in this journal is cited, in accordance with accepted academic practice. No use, distribution or reproduction is permitted which does not comply with these terms.
*Correspondence: Paul Ogongo, cGF1bC5vZ29uZ29AdWNzZi5lZHU=
†These authors have contributed equally to this work
‡ORCID: Mbali N. Mkhonza orcid.org/0000-0001-8380-7368
Disclaimer: All claims expressed in this article are solely those of the authors and do not necessarily represent those of their affiliated organizations, or those of the publisher, the editors and the reviewers. Any product that may be evaluated in this article or claim that may be made by its manufacturer is not guaranteed or endorsed by the publisher.
Research integrity at Frontiers
Learn more about the work of our research integrity team to safeguard the quality of each article we publish.