- 1Department of Pharmacology, All India Institute of Medical Sciences, Kalyani, West Bengal, India
- 2Safety Physician, iCore Digital Operations and Platforms (DOP), Wipro Limited, Pune, India
Vaccine development tools for fungal infections are undergoing transformation where newer technologies like nanotechnology and bioinformatics are used to create new and improved vaccine candidates. Immunocompromised individuals and those with multiple chronic conditions are especially vulnerable to invasive fungal infections. These patients are at increased risk of developing widespread infections and experiencing poor health outcomes. Current management of fungal infections is associated with diagnostic challenges, side effects, and resistance. Vaccination is an effective strategy to prevent infections and boost immunity. Despite the significant burden of fungal disease, there are currently no licensed fungal vaccines available. This review is focused on various vaccine development strategies, including whole-cell, subunit, and nucleic acid-based vaccines. Various challenges like safety concerns, weak and nonspecific immune response, ideal adjuvants, and the need for improved drug delivery systems are also highlighted in this review. Sustained antigenic response, addressing host immune response variability, and eliciting persistent predictable immune response are crucial for vaccine development. Standardized protocols and robust preclinical studies are essential for the clinical development of potential vaccine candidates. Exploring novel targets using advanced technologies like bioinformatics, nanotechnology, and reverse vaccinology are being rapidly explored.
1 Introduction
In the realm of public health, invasive fungal infections (IFIs) have been identified as one of the major global health concerns, especially in people with impaired immunity due to chemotherapy and organ transplants, or individuals with immunodeficiency such as HIV/AIDS, as well as those with poor hygiene or belonging to financially less privileged social classes. Though imprecise, studies have suggested that IFIs can have an annual incidence of 6.5 million and an annual mortality rate of 3.8 million people globally (1). Thus, there arises an urgent need to prioritize the development of antifungal vaccines, which can empower comprehensive efforts to overcome this formidable community health crisis (2).
Fungi are pervasive in the environment, and many of them are pathogenic in nature. The most common fungi are Candida, Aspergillus, Cryptococcus, and Pneumocystis (3). They can cause a wide variety of infections ranging from a minor infection to severe disseminated diseases like invasive candidiasis, aspergillosis, and cryptococcal meningitis (4).
Increased use of immunosuppressive therapies, misuse of broad-spectrum antibiotics, and the booming population of immunocompromised people are increasing the risk of infection to great extent (5). The limited number of antifungal drugs and the emergence of resistant strains have further complicated the condition (6). Traditional antifungal therapies (azoles, polyenes, and echinocandins) are effective but are often associated with unwanted side effects, drug interactions, and the gradual development of resistance (7). Moreover, delays in diagnosis and the low concentration of the drug at the infection site are other contributing factors that enhance the seriousness of the condition (8).
Antifungal vaccines have a potential for disease prophylaxis and active control of such infections. Vaccines can be helpful in boosting immunity, amplifying the ability to identify a virulent pathogen and generate an effective response in such pre-emptive efforts, which can suppress the risk of infection, cut down the extra burden on healthcare delivery systems, and ultimately ensure patient safety (9).
Multiple platforms have been used for the development of antifungal vaccines. These include whole-cell vaccines, subunit vaccines, and nucleic acid-based vaccines. Whole-cell vaccines, composed of inactivated or attenuated fungal cells, have good prospects in murine models but have issues of safety and reproducibility (10). Subunit vaccines are preferred due to their increased safety and specificity but immune response is often weak (11). Nucleic acid-based vaccines (DNA and RNA vaccines) are an upcoming novel approach offering better protection and improved safety profile.
However, optimization of delivery systems, robust immunogenicity, and the risk of adverse effect are some concerns to be addressed (12).
Diversity among pathogens and variability in host immune responses are some areas that need attention to make broad-spectrum vaccines (13). Owing to the lack of clarity between correlates of protection, ill-understood mechanisms regarding immunogenicity, and complicated regulatory criteria, clinical studies on fungal vaccines have been impacted heavily. To facilitate the transformation of promising vaccine candidates from animal experiments to clinical practice, standardized protocols and guidelines for the development of fungal vaccines, evaluation, and approval are imperative (14).
IFIs are usually defined as systemic infections resulting from yeasts or molds in deep-seated tissues. In contrast to superficial fungal infections, they are usually fatal conditions with high rates of morbidity and mortality (15).
Mycosis in humans can have a wide range, from minor dermal infection, superinfection followed by bacterial infection, and even severe lethal conditions involving multiple organs and systems. We can also classify fungal infections according to sites and severity of the infections, superficial, subcutaneous, and invasive. Invasive is a term that generally denotes introduction of the infection to the circulation and reaching the tissues supplied by the blood. Immune status of the host, site of infection, and virulence capability of the fungi play a vital role in the severity of the disease and the prognosis of the patient (16–18).
The development of mild fungal infection into IFI is influenced by several factors. These include the type of fungus, the host’s immune system, duration and severity of the infection, and the presence of underlying medical conditions. Major risk factors include neutropenia, bone marrow suppression, hematological malignancies, bone marrow transplantation, prolonged (>4 weeks) treatment with corticosteroids, prolonged (>7 days) stays in intensive care, chemotherapy, and HIV infection (16, 17).
The most common invasive infections are associated with Candida sp., Aspergillus sp., Cryptococcus sp., Pneumocystis sp., etc. In addition, Blastomyces, Histoplasma, Paracoccidioides, and Coccidioides are endemic fungal strains that have also been implicated in causing severe systemic infections in immunocompromised patients (18, 19) (Table 1).
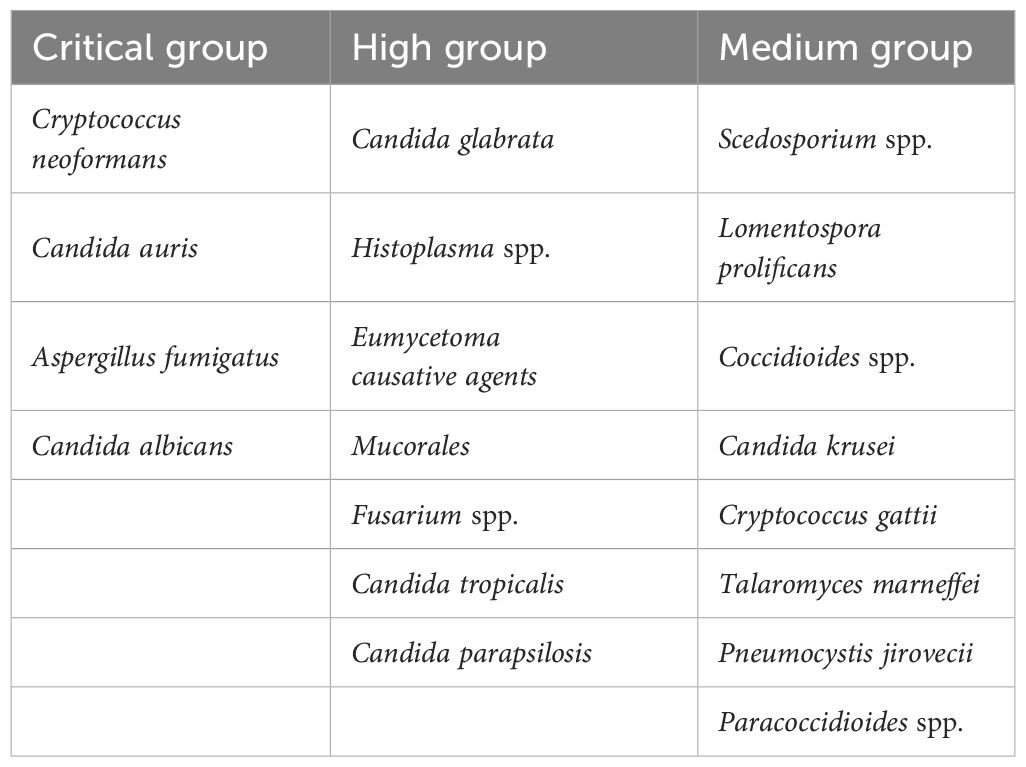
Table 1 WHO fungal pathogen priority list (20).
2 Platforms for fungal vaccine development
Despite the diverse array of platforms for fungal vaccine development, the majority remain in preclinical stages. Few have progressed to clinical phases, and none of the fungal vaccines have been approved by regulatory agencies. The following section of this article will delve into the current landscape of fungal vaccine development platforms (Figure 1).
2.1 Live attenuated fungal vaccines
Live attenuated fungal vaccines operate on the fundamental principle of eliciting an immune response through exposure to a modified or weakened form of the pathogenic fungi. This attenuated strain, while incapable of eliciting overt disease, stimulates robust protective immunity within the host against subsequent exposure to the virulent wild-type pathogen.
A genetically modified Blastomyces dermatitidis strain deficient in WI-1 adhesin has exhibited promising vaccine efficacy against lethal pulmonary infections induced by various strains. The attenuated fungal strain triggers a delayed-type hypersensitivity reaction and a predominantly type-1 cytokine response, with interferon-gamma playing a key role. Additionally, its cell wall/membrane antigens contribute to protective immunity, highlighting the potential of targeting virulence factors for the development of novel antifungal vaccines (21). In a murine model, a genetically engineered Candida albicans strain with a tetracycline-regulatable NRG1 gene, rendered avirulent through controlled morphology, elicited protective immunity against candidiasis. This protection was found to be dependent on T cell-mediated adaptive immune responses, as evidenced by the absence of protection in T cell-deficient mice (22). In a murine model of systemic candidiasis, a live attenuated C. albicans strain deficient in the HOG1 gene (CNC13) demonstrated protective efficacy, predominantly mediated by the elicitation of robust cellular and humoral immune responses. Proteomic analysis identified eight antigenic proteins eliciting protective IgG2a antibodies, including two novel antigens (Imh3p and Acs2p), which can be potential candidates for future fungal vaccine development (23).
Live attenuated fungal vaccines present a compelling approach for conferring durable protection against fungal pathogens by eliciting potent and comprehensive humoral and cell-mediated immunity, mirroring the complexity of natural infection responses. However, concerns regarding potential reversion to virulence in immunocompromised individuals, stringent storage requirements, and limited clinical data pose challenges to their widespread implementation.
2.2 Killed whole-cell vaccine
Killed whole-cell fungal vaccines employ inactivated (killed by heat, chemicals, or radiation) whole fungal cells to elicit a protective immune response against fungal infections. This approach offers a safer alternative to live attenuated vaccines, particularly for immunocompromised individuals, as they can eliminate the risk of reversion to virulence.
Inactivated C. neoformans fbp1Δ cells elicit a robust Th1-mediated immune response, conferring protection against a broad spectrum of fungal pathogens, like Cryptococcus and Aspergillus strains, and partial protection against C. albicans, in both immunocompetent and CD4+ T cell-deficient murine models (24). Inactivated Saccharomyces cerevisiae cells by heat have exhibited prophylactic efficacy against Candida, Aspergillus, Coccidioides, and Rhizopus, in preclinical murine studies, suggesting their potential as a broad-spectrum antifungal vaccine candidate. While it may not be suitable for commercialization because the model is not a purified preparation, it serves as a valuable positive control in evaluating novel molecular vaccine candidates and elucidating key components of effective pan-fungal vaccines (25).
Killed whole-cell fungal vaccines offer a safer alternative for immunocompromised individuals due to their non-replicating nature, potentially providing broad-spectrum and pan-fungal protection. However, their efficacy may be limited by weaker immune responses and potential for adverse reactions, necessitating further research to optimize their formulation and adjuvant selection.
2.3 Recombinant (subunit) vaccine
Recombinant fungal vaccines, composed of purified antigenic components such as proteins or polysaccharides, offer a safe and effective alternative to live attenuated vaccines for immunocompromised individuals. While their efficacy often requires co-administration with potent adjuvants to elicit robust immune responses, these subunit vaccines present a promising approach for prophylactic interventions against fungal pathogens.
In a randomized phase 2, placebo-controlled, double-blind clinical trial, the recombinant subunit vaccine NDV-3, targeting the C. albicans adhesin/invasin protein, exhibited a favorable safety profile and elicited immune responses in women with recurrent vulvovaginal candidiasis (26). In preclinical studies, the innovative vaccine candidate PEV7, a fusion of truncated C. albicans Sap2 protein and influenza virosomes, demonstrated potent induction of both systemic and mucosal antibodies. Intravaginal administration of PEV7 conferred significant, durable protection against candidal vaginitis in a rat model (27).
Recombinant subunit fungal vaccines offer targeted immune responses and enhanced safety, particularly for immunocompromised individuals, but may exhibit reduced immunogenicity and require adjuvant co-administration to elicit robust protection.
2.4 Conjugate vaccine
Conjugate fungal vaccines are composed of a polysaccharide antigen covalently linked to a carrier protein, enhancing immunogenicity and inducing robust, long-lasting antibody responses against the target fungus. This approach circumvents the weak immunogenicity of polysaccharide antigens alone and offers a promising avenue for developing effective prophylactic interventions against invasive fungal diseases.
The conjugation of Cryptococcus neoformans capsular glucuronoxylomannan (GXM) to tetanus toxoid elicits antibody-mediated protection against experimental cryptococcal infection in mice, marking a significant milestone in the development of immunotherapies for systemic fungal diseases (28). The covalent linkage of galactoxylomannan (GalXM) from C. neoformans to either bovine serum albumin or Bacillus anthracis protective antigen, facilitated by the cyanylating agent CDAP, elicited potent and durable antibody responses in murine models (29). A novel vaccine candidate, Lam-CRM, consisting of laminarin (derived from Laminaria digitata) conjugated to diphtheria toxoid CRM197, has exhibited protective effects in preclinical murine models against both disseminated and mucosal candidiasis caused by C. albicans, as well as lethal challenge with Aspergillus fumigatus. The protective mechanism is likely mediated by anti-β-glucan antibodies, which exhibit direct antifungal properties in vitro by binding to and inhibiting fungal hyphal growth (30).
Conjugate fungal vaccines elicit robust, T cell-dependent antibody responses against target fungal polysaccharides, offering broad-spectrum protection. However, challenges in production complexity and potential for carrier-induced epitopic suppression warrant further optimization.
2.5 DNA vaccines
DNA vaccines, comprising plasmids encoding target antigens and potentially co-stimulatory molecules or cytokines, offer a promising approach for eliciting robust immune responses through direct antigen presentation by host antigen-presenting cells (APCs). Despite theoretical advantages, their translation to human use has been hindered by challenges related to safety, immunogenicity, and efficacy, necessitating further research and optimization (31).
In a murine model of paracoccidioidomycosis, a DNA vaccine encoding the immune-protective peptide 10 (P10) elicited significant and durable protection against Paracoccidioides brasiliensis infection, concurrent with an expansion of memory CD4+ T cells and Foxp3+ regulatory T cells, crucial for balancing effective fungal clearance with mitigation of immunopathology (32). Another DNA vaccine encoding tandem variants of the Pneumocystis p55 antigen (p55-TAG) significantly reduced pathogen burden and lung inflammation, and elicited cellular and humoral immune responses in immunosuppressed rats, demonstrating its potential as a prophylactic intervention against Pneumocystis pneumonia (PCP) (33).
DNA fungal vaccines offer targeted antigen expression and cellular immunity while being inherently safer than live attenuated vaccines. However, limited clinical data, delivery challenges, and potential for unintended genomic integration necessitate further investigation.
2.6 Pan-fungal vaccine
Leveraging the conserved nature of fungal cell wall polysaccharides, a pan-fungal vaccine strategy has been proposed involving the conjugation of these polysaccharides to immunogenic peptides. This approach aims to elicit broad-spectrum protective immune responses against diverse fungal pathogens by targeting shared epitopes, potentially addressing the growing threat of invasive fungal diseases.
A highly conserved 13-amino-acid sequence within the fungal chaperone protein calnexin, identified across various ascomycete species, elicits protective CD4+ T-cell responses and demonstrates potential as a broad-spectrum vaccine candidate. Immunization with calnexin encapsulated within glucan particles (GPs) induces robust antigen-specific CD4+ T-cell expansion, conferring protection against virulent strains in preclinical models (34). As discussed, earlier heat-killed yeast (HKY) S. cerevisiae exhibits broad-spectrum prophylactic efficacy against diverse fungal pathogens in murine models, including Aspergillus, Coccidioides, Candida, Cryptococcus, and Rhizopus, highlighting its potential as a pan-fungal vaccine candidate (25). Pan-fungal vaccines, targeting conserved fungal antigens, offer the potential for broad-spectrum protection against diverse pathogens and simplified vaccination strategies, particularly in immunocompromised individuals. However, challenges remain in identifying suitable antigens, eliciting comprehensive immune responses, and navigating regulatory hurdles due to the complexity of demonstrating efficacy against multiple pathogens.
2.7 Nanotechnology in fungal vaccine development
In vaccine development, nanoparticles act as potent carriers, enhancing antigen stability, improving immunogenicity, and potentially serving as immunostimulatory adjuvants. Diverse nanostructures, including polymeric nanoparticles, nanostructured lipid carriers, phospholipid-based vesicles, dendrimers, nano-emulsions, and metallic/magnetic nanoparticles, are currently being explored as vaccine adjuvants.
Liposomal nanoparticles incorporating fatty acyl analogs of muramyldipeptide (MDP) demonstrate promising adjuvant potential for fungal vaccine development, eliciting Th1-biased immune response and stimulating cellular proliferation, crucial for effective antifungal immunity (35).
In preclinical studies, both liposomal and PLGA nanoparticle platforms have demonstrated successful delivery of the DNAhsp65 vaccine, eliciting protective immunity and mitigating pulmonary fungal burden in a murine model of paracoccidioidomycosis. Notably, the intranasal administration of the liposomal formulation presents a potentially more accessible and convenient route for adjuvant vaccine therapy against systemic fungal infections in clinical settings (36).
Nanotechnology-based fungal vaccines offer enhanced antigen stability, targeted delivery, and improved immunogenicity, but their development is hindered by potential biocompatibility concerns, regulatory hurdles, and cost-effectiveness challenges.
3 Current vaccine strategies against various invasive fungal infections
3.1 Invasive candidiasis
It is a severe systemic infection (global prevalence: 100,000 to 400,000 per year) due to Candida sp. (C. albicans 50%–60%) that can disseminate to the circulation and deep organs from mucosal surfaces (37). Risk factors include prolonged hospitalization, broad-spectrum antibiotics, central venous catheters, immunosuppression, recent surgery with high morbidity (38), and 20% to 50% mortality rate (37).
3.1.1 Pathophysiology
Deep-seated infection due to Candida occurs after disrupting host mucosal protection or indwelling medical devices and invasion into the bloodstream (39). As phagocyte-dependent innate immunity and adaptive T-cell responses are critical for infection control, impaired neutrophil and T-cell defects cause enhanced susceptibility (40). The interaction between candidal virulence factors (e.g., hydrolytic enzymes, adhesins, morphological and phenotypic switching, and formation of biofilm) and the host’s immune status determines disease progression and severity (41).
3.1.2 Vaccine development strategies
3.1.2.1 Live attenuated vaccines
Genetically engineered strains of Candida have been developed by researchers to protect animals against systemic infections in preclinical studies. In rodent models, the tet-NRG1 strain of C. albicans, which can be treated by doxycycline, has demonstrated an effective response (22). Immunogenicity is also seen in strains like CNC13, PCA-2, and gpi7 (23, 42–44). As vaccine vehicles, avirulent strains of S. cerevisiae have been tried due to their flexible genetic makeup and other adjuvant-like properties (45).
3.1.2.2 Recombinant subunit vaccines
Owing to the inherent complexities in the production of live vaccines and potential adverse effects, researchers are focusing on recombinant protein vaccines that have specific immunogenicity and better safety profile due to the absence of the pathogen (46).
Key recombinant protein vaccines for candidiasis include the following:
● Als1p and Als3p proteins: Because of their pivotal role in adhesion as well as infection, these protein-derived vaccines have shown protection against various preclinical models (47, 48).
● NDV-3: Recombinant protein like Als3p using alum for the adjuvant generates immunogenicity as strong B- and T-cell responses and provides ample safety against mucosal and disseminated candidiasis (49, 50).
● PEV-7: In rodents, a genetically engineered version of aspartyl proteinase-2 in influenza virosomes offers immunogenicity as well as protection (27).
● Mdh1p: Malate dehydrogenase has also been found to be a promising antigen (51).
Potent and specific adjuvants (e.g., inulin combined with TLR agonists) have been found to be vital in amplifying immune response, causing heightened protection in preclinical animal models.
3.1.2.3 Conjugate vaccines
Polysaccharides can stimulate B cells, and with the help of polysaccharide-bound peptides, they play a key role in the presentation of antigens to T cells (52).
Common polysaccharide epitopes (e.g., β-glucans) demonstrate promising opportunities for developing pan-vaccines, which can be helpful against variable fungal infections. Vaccines against C. neoformans and other Candida sp., using a similar concept and conjugating shared polysaccharides with proteins like TT or diphtheria toxin that can invoke immune response, have been tried in the recent past as well (46, 47).
3.1.2.4 Killed whole-cell vaccines
These approaches are safe and stable due to the low risk of pathogenic transformation, a simpler manufacturing process, and low cost. However, they also come with several problems including incomplete protection against all kinds of candida infection.
● Heat-killed C. albicans: Intranasal immunization of heat-killed Candida and a genetically engineered heat-labile toxin of Escherichia coli (R192G) have been found to be effective in animal models except mucosal infections (53).
● Heat-killed S. cerevisiae: Heat-killed subcutaneous vaccines from S. cerevisiae provide cross-protection against fungi including C. albicans, A. fumigatus, and Coccidioides posadasii. The immune response is due to antibody against common cell wall glycans and activation of Th1 and Th17 cellular responses (25, 54, 55). S. cerevisiae is used due to its simplicity regarding culturability and manipulation, with well-understood physiology and molecular biology. β-1,3-D-glucan and mannan, proteins found in yeast cell walls, tend to generate an immune response and make it a good adjuvant.
Challenges and potential: The challenge remains a comprehensive protection in all forms of candidiasis including mucosal infections. Heat-killed vaccines have a unique cross-protective nature, and they can be of great help in yeast display, recombinant yeast cells, and virus-like particle preparations. These vaccines have better patient compliance and ease of administration via the oral route (54–56).
3.1.2.5 Oral vaccines
They are convenient, cost-effective approaches especially for mass vaccination to protect at-risk populations. Novel antigenic candidates and adjuvant targets are imperative for developing such vaccines. Key developments in this field are as follows:
● Antigen display: Eno1p antigen has been effectively displayed on the surfaces of E. coli and S. cerevisiae cells, indicating them to be promising oral vaccines for the future.
● Mice immunized with Eno1p (oral, intranasal, and subcutaneous): They were shown to enhance anti-Eno1p antibody levels and protection against C. albicans [Eno1p-expressing S. cerevisiae (oral) → 60% and L. casei cells 20% protection] (51, 57).
Challenges with adjuvants: Conventional adjuvants used for injectable routes of administration are mostly ineffective in the environment of enteric systems.
Future directions: Proteomic analysis is a promising technology in drug target identification. Several ongoing research into new antigenic candidates through proteomics aim to further enhance the effectiveness of oral vaccines against candidiasis (58).
3.1.2.6 Bacterial ghost vaccines
Hollow bacterial cells with core components like immunogens or drugs retain the ability of the organism to induce strong immune responses. This approach could pave the way for highly effective anti-Candida vaccines (59). Intraperitoneal administration of Candida ghosts with nanoparticles of silver and gold offers enhanced humoral and cellular immunity, faster wound healing, and controlled inflammation in Sprague–Dawley albino rats. They also stimulated white blood cell proliferation and systemic interferon (IFN)-γ levels and did not trigger hyperallergic responses (60). Bacterial ghosts inherently aggravate the innate immune response of the host and provides better efficacy of the vaccine without disrupting the pathogen’s structural features (59).
3.2 Invasive aspergillosis
Invasive aspergillosis (IA) is a fatal fungal infection caused by Aspergillus sp., primarily A. fumigatus. The global prevalence of this infection is approximately 200,000 cases annually, with a high mortality rate (30% to 90% in high-risk populations) (61).
3.2.1 Pathophysiology
The infectivity of the fungus is due to accidental inhalation of spores followed by germination, hyphae formation, and bloodstream invasion leading to widespread organ dissemination (62). Impaired phagocytic and cell-mediated immunity in special populations (i.e., immunodeficient people) facilitates fungal proliferation (63).
3.2.2 Vaccine development strategies
3.2.2.1 Crude vaccines
Whole or fractionated Aspergillus can protect the rodents, but the efficacy differs with heat-killed and live conidia. Live conidia show aggravated immune response due to tissue growth and filamentous forms. Challenges in this type of vaccine include autoimmune response and reactogenicity (64, 65).
3.2.2.2 Pan-fungal vaccines
They are known to target several fungal species because of the presence of common antigens among different fungi. β-1,3-glucan with diptheria toxoid provides protection against multiple fungal infections (aspergillosis and candidiasis) (66). NXT-2 peptide binds to fungal conidia and hyphae, enhancing immune response and resulting in mortality reduction in preclinical studies (67).
3.2.2.3 Dendritic cell-based vaccines
Dendritic cells (DCs) pulsed with Aspergillus conidia and RNA have shown efficacy in infections after haploidentical transplants in preclinical and small clinical trials (68). Owing to the increased cost and non-specific adaptive immune response, no vaccine has been tried in large clinical trials (66, 68–70). Because of environmental interruption in the form of exposure to fungal conidia, specific and immunogenic antigens need to be identified for vaccine production in order to surmount non-specific antibody responses after immunization (71).
3.3 Invasive cryptococcosis
Invasive cryptococcosis, a serious life-threatening fungal infection, is caused by inhalation of infectious spores or desiccated yeast cells of encapsulated yeast C. neoformans and C. gattii (72). Over 180,000 cases are registered annually (mortality rate ranging from 20%–70%) with a high burden in Sub-Saharan Africa and Southeast Asia (73). High-risk groups include immunocompromised patients, those who underwent organ transplantation, and those with an advanced age (74).
3.3.1 Pathophysiology
Inhalation of infectious particles, followed by dissemination to the central nervous system and other organs through circulation, is the usual route of infection. Cell-mediated immunity, including response of T-helper cells and activated macrophages, has a vital role in infection control. Capsular polysaccharide diminishes phagocytosis and the host immune response, contributing to its infectivity, mainly affecting the respiratory and nervous systems, but can also include skin, bones, and prostate (75).
3.3.2 Vaccine development strategies
The capsule is composed of GXM, a major virulence factor that acts as the cause for both challenges and advantages for vaccine development (76).
3.3.2.1 Whole yeast approaches
It includes designing new strains of Cryptococcus that provide immunity via DC stimulation (77, 78). A study has reported that heat-inactivated acapsular C. gattii stimulates Th17 cells and thus decreases the infection (79).
3.3.2.2 Polysaccharide-based vaccines
GXM was the first experimental vaccine to induce immunogenicity without the involvement of T cells. Conjugation with the GXM-tetanus toxoid has shown improvement in antigenicity, inducing partially protective murine antibodies (28, 80). The GXM-conjugated mimetic peptide helps in the generation of protective antibodies (81).
3.3.2.3 Mutant strains
It includes engineered strains that lack fungal proteins like chitosan and provides immunity via Th-1 cells (82). Other strains like Fbox protein knockout mutants and the morphogenesis regulator protein Znf2 over expressive strains can be of use too (83, 84).
3.3.2.4 Protein antigen vaccines
To create strains with decreased virulence for ideal vaccine candidate, Chitosan, GXM, F-box protein and Steryl glycosides like key factors are used as adjuvant (24, 82, 83, 85–87).
3.3.2.5 Subunit vaccines
Nonhomologous proteins of chitosan deacetylase class are included (88).
3.3.2.6 Innovative formulations
Tetraethyl orthosilicate (TEOS) helps in the encapsulation of Cda2 antigen within GPs and has played a role in providing heat stability and protection against fatal respiratory infections (89, 90).
3.4 Invasive mucormycosis
Multiple fungi (Rhizopus, Mucor, and Lichtheimia) of the order Mucorales cause severe and life-threatening infection, with invasive mucormycosis mostly affecting patients with impaired immune systems (91). Though global incidence has been 0.2 to 1.7 cases per million annually, during the COVID-19 pandemic, there was a massive surge of morbidity and mortality (77). Risk factors include defective phagocytic activity and innate immune system, prolonged neutropenia, diabetes mellitus, use of corticosteroids, organ transplantation, and iron overload.
3.4.1 Pathophysiology
Virulence includes iron entrapment abilities of the host, causing extensive angioinvasion and tissue death. Proteolytic enzymes facilitate tissue invasion and dissemination. The fungi can escape the host’s immune response through suppression of oxidative burst and cytokine production (78).
3.4.2 Vaccine development strategies
3.4.2.1 Proteome studies
▪ Identification of two protein candidates can be effective in various mucormycetes (88).
▪ A vaccine that has multi-epitopes can be the ideal candidate, which can have a prominent immunological and physicochemical nature (79).
3.4.2.2 Immunoinformatics approach
▪ For mucormycosis due to different fungi, newer epitopes with activity against Mucor circinelloides and Rhizopus oryzae provide better population protection and immune response.
▪ Molecular docking analyses demonstrated a stable and effective vaccine in minimal disordered regions (92).
3.5 Invasive histoplasmosis
It is a potentially life-threatening fungal infection due to a dimorphic fungus (93). Immunocompromised individuals like those with HIV/AIDS, organ transplant recipients, and patients treated with immunosuppressives drugs are at higher risk for developing invasive disease (94).
3.5.1 Pathophysiology
Inhalation of Histoplasma spores are subsequently taken up by alveolar macrophages. They are transformed to the yeast phase, after which they replicate, leading to a disseminated infection (95). While primarily being a respiratory infection, it can spread to the liver, spleen, bone marrow, and central nervous system, leading to complications such as pulmonary fibrosis, adrenal insufficiency, and neurological deficits, impacting their quality of life (96, 97).
3.5.2 Vaccine development strategies
▪ Antigens derived from cell wall and membrane extracts recognized by T cells triggered protective immunity against lethal Histoplasma challenge in rodents.
▪ Specific antigen vaccines:
• rHsp60 glycoprotein vaccine activates specific CD4+ or CD8+ T cells and provides protection in animal models. However, because of the similarity with its human counterpart, this vaccine shows a higher risk of autoimmune reaction and restricted its wide use (98, 99).
• A specific IgG1 isotype of monoclonal antibodies against Hsp60 and surface M antigen demonstrated immunogenicity in murine models (100, 101).
▪ Reverse vaccinology: Using knowledge of pharmacogenetics, comprehensive genome sequencing and computational analysis indicated that β-1,3-glucanosyltransferase and proteins similar across strains can be included in future immunotherapy (102).
3.6 Invasive coccidioidomycosis
Dimorphic fungi Coccidioides immitis and C. posadasii cause this disseminated fatal infection that is endemic in North and South America and Mexico. The risk is higher for patients with impaired immunity.
3.6.1 Pathophysiology
Spores of Coccidioides are inhaled, and within alveolar macrophages, they undergo endosporulation, leading to severe complications in bones, joints, and the central nervous system, leading, in turn, to severe manifestations (103). There is less risk of interpersonal transmission, which can only take place in cases of organ transplantation or laboratory accidents (104).
3.6.2 Vaccine development strategies
3.6.2.1 Formalin-killed spherules
▪ A 1967 study found that vaccinated rodents with formalin-killed spherules have shown protection (105).
▪ A double blind study in 3,000 subjects showed limited success due to risk of toxicity and lesser disease incidence (106). However, soluble spherule models have shown to be helpful in murine models (107).
3.6.2.2 Targeted antigen approaches
▪ Pmp1 and Cs-Ag (108) have shown good immunogenicity and identified as promising vaccine candidates.
▪ Subcutaneous injection with antigen Ag2 for prime as well as booster doses causes improved cell-mediated responses. Increased Th1 and Th17 responses indicated potential benefits (109).
3.6.2.3 Cellular vaccination
Intranasal DC transfection with Ag2 DNA generates specific IgG responses, though further studies are needed to understand the immune response better (110, 111).
3.6.2.4 Other strategies
Intervention of genes like CTS2, CTS3, and ARD1 in HLA-DR4 transgenic mice displayed Th1 and Th17 cell expansion and engagement of innate immunity, proving to be a useful tool for future therapeutics (112).
3.7 Invasive paracoccidioidomycosis
Invasive paracoccidioidomycosis is a similar life-threatening condition due to dimorphic fungus Paracoccidioides infection. It is endemic in Latin America (highest prevalence in Brazil, Colombia, Venezuela, and Argentina) (113). Risk factors include immunocompromised state, male gender, smoking habits, and workers with exposure to soil or dust (114).
3.7.1 Pathophysiology
Inhaled spores engulfed by macrophages in the alveoli and converted to the yeast phase and following multiplication cause severe disseminated infection to other organs (115). Person-to-person transmission rarely occurs by accident or through organ transplantation.
3.7.2 Vaccine development strategies
▪ Therapeutic DNA vaccines: gp43 encoded DNA vaccines using plasmids displayed mixed T helper responses that can be amplified and specified using plasmids encoding the P10 minigene. IL-12 added to these vaccines improves the immune response and suppresses the fungal loads significantly in long-term infections (116).
▪ Immunotherapy with peptide P10: Antifungal treatment consisting of chemotherapy and P10 peptide immunization increases IL-12 and IFN-γ production while reducing IL-4 and IL-10, enhancing the efficacy of antifungal treatment (117). P10-based immunotherapy, even without chemotherapy, has shown good response with the addition of adjuvants, nanoparticles, and DC priming (88).
▪ The P10 segment of the gp43 antigen of P. brasiliensis has the ability to generate robust T-helper immune response through IFN-γ, leading to fungal elimination (32, 118).
▪ Fungal whole cells: Yeast cells attenuated by methods like gamma-irradiation evoke strong immune reaction with T-helper cells and prove to be another option for research and trials (88).
▪ Protective antibodies: gp43 targeting monoclonal antibodies showed promise in immunotherapy (119–121).
▪ A newer drug delivery system and the implementation of gene therapy can be a good prospect for the future (88).
3.8 Invasive blastomycosis
Dimorphic fungus B. dermatitidis causes serious fungal infections in certain regions of North America (122, 123). Patients with impaired immunity or occupational or accidental exposure to soil or decaying vegetation are mostly at risk of getting infected (38).
3.8.1 Pathophysiology
Inhaled spores from aerosol is the main infective form that has the ability to cause both pulmonary and extrapulmonary infection that can disseminate and cause severe infections (38).
3.8.2 Vaccine development strategies
▪ Recombinant BAD1: Though this vaccine could induce immunogenicity, it has a modest role in enhancing survival or the significant lowering of fungal burden. When IL-12 was added to improve its efficacy, alteration of the antibody response and aggravation of the delayed-type hypersensitivity reactions are observed (13). Monoclonal antibodies targeting BAD1 did not raise killing power or protection. However, B-cell knockout mice demonstrated better protection. BAD1-null mutant displayed notable survival and attenuated murine fungal load through the generation of robust IFN-γ production and T-cell activation (124).
▪ Vaccination strategy: People with increased risk of exposure like construction workers, agricultural workers, and those engaging in activities like spelunking in endemic areas can benefit from this vaccination. The recommended age for vaccination would be approximately 2–3 years (13).
3.9 Invasive Pneumocystis pneumonia
Invasive PCP is caused by Pneumocystis jirovecii. It causes severe and fatal opportunistic infection primarily in immunodeficient individuals worldwide. The primary risk factor for developing PCP is impaired immunity, especially CD4+ T-cell deficiency (125).
3.9.1 Pathophysiology
It is transmitted mainly through the airborne route. The trophic forms attach and infiltrate the epithelial cells and then undergo asexual reproduction, causing the accumulation of cysts and trophic forms in the alveoli (126). Pathological hallmarks include diffuse alveolar damage and alveolar casts, interstitial inflammation, and fibrosis in severe cases.
3.9.2 Vaccine development strategies
3.9.2.1 Whole organism vaccines
In past decades, inactivated whole organisms have been tested to provide strong protection in animal models (127–129). Intranasal vaccination with non-viable fungus with cholera toxin B induced prominent immunogenicity through IgA and IgG antibody responses (128). Pneumocystis cultivation in laboratories has halted the viability and mass production of this approach (130).
3.9.2.2 Subunit vaccines
▪ Pneumocystis cross-reactive antigen 1 (Pca1): This antigen was known as A12 antigen in the past. Pca1 provides protective response through the suppression of organism burden and lung inflammation. It has also displayed cross-reactivity with several Pneumocystis species (131).
▪ SPD1: It has similarity with P. jirovecii and induces IgG antibody responses and enhances memory B-cell development, ultimately eliminating organism burden in chronic infection (132).
3.9.2.3 Other antigen candidates
Major surface glycoprotein (MSG) and recombinant p55 antigen have been targeted for both therapeutic and prophylactic vaccine development (130, 133, 134).
3.10 Invasive sporotrichosis
It is a form of subacute or chronic subcutaneous mycosis due to dimorphic Sporothrix schenckii and its related species, targeting immunocompromised individuals as well as people with occupational exposure to infected vegetation or soil (135). The disease has a global distribution, especially in tropical and subtropical regions. Apart from immunosuppression or immunodeficiency, occupational or accidental contact (e.g., gardeners, farmers, and florists) and inoculation through injury with contaminated plant materials or soil are common sources of infection (136).
3.10.1 Pathophysiology
Pathogenesis: Infection is primarily acquired through percutaneous inoculation due to traumatic implantation. Inhalation and zoonotic transmission (from infected animals) are less common routes (137).
3.10.2 Vaccine development strategies
▪ Whole organism and glycoprotein-based vaccines: S. schenckii-infected mice showed high titers of anti gp70 antibodies and reduced colony-forming units (CFUs) (138). Monoclonal antibodies targeting gp70 (mAbP6E7) are seen to induce phagocytosis and reduce fungal burdens and are potential agents for prophylaxis and therapeutics (139).
▪ Cell wall protein vaccines: Passive transfer of serum from mice vaccinated with ssCWP (S. schenckii cell wall proteins) and adjuvants showed immunogenicity after S. schenckii challenge (140).
▪ Recombinant phage vaccines: Chen et al. developed a vaccine that used a recombinant phage displaying a gp70 peptide, and it can reduce the fungal burden in S. globosa infections through heightened Th1 cell responses and through a robust humoral response (139).
▪ Antibody-based therapies: Antibodies against phage-KR extracted from vaccinated mice also displayed protection against S. globosa infection through suppressed colonization of the fungi, increased survival rates, and inhibited inflammatory changes in mice (141).
▪ Immunoproteomic approaches: Using immunoproteomic methods, newer targets in the form of antigenic peptides from S. brasiliensis were identified. Peptides like ZR3, ZR4, and ZR8 helped in increasing cytokine levels and CD4+ T-cell proliferation and reduced lesion sizes in sporotrichosis (142).
4 Fungal vaccines in pipeline (2018 onwards)
There are several ongoing studies on exploring new horizons in the field of fungal vaccines. Most of these are in preclinical phases. The latest vaccines for individual fungal infection that have been examined since 2018 are described here (Table 2; Figures 2, 3).
4.1 Invasive candidiasis
4.1.1 Cell-based vaccine
4.1.1.1 EV
Extracellular vesicles (EVs) from C. albicans were used as vaccine against murine candidiasis. They augmented specific serum IgG antibodies production, producing robust humoral immune response. It also decreased fungal load in various organs like liver, kidney, and spleen in a candidal murine model. The vaccine with or without an adjuvant can protect lethally challenged mice entirely, according to a study (143).
4.1.2 Heat-killed vaccine
4.1.2.1 V132
Sublingual heat-killed vaccine trained immunity in peripheral blood mononuclear cells and monocytes, resulting in amplified oxidative phosphorylation and glycolysis. These changes occurred by enhanced pro-inflammatory responses through robust Th1/Th17 responses. Specifically, stimulation with adjuvant (MV140) leads to stronger Th17 responses in immunized mice, eliciting both innate and adaptive immunity (144).
4.1.3 Live attenuated vaccine
● FLO8: The yeast form of the FLO8-deficient C. albicans strain conferred protection against lethal infection through inducing IL-10 induced by the Dectin-2/CARD9 pathway in macrophages and DCs. IL-10 inhibits T-cell apoptosis, preventing thymus atrophy. This facilitates the constant release of T cells from thymus, enhancing strong Th1-based immunity responses that produce protection from polymicrobial infection (145).
● Low-virulence Candida species: Myeloid-derived suppressor cells’ (MDSCs’) defense is facilitated by Gr-11 leukocytes against intra-abdominal infection. Ly6G1 G-MDSC enhanced trained innate immunity (TII), which is produced after inoculation (IP/IV) of the live attenuated strain, which decreases organ-specific fungal load in the murine model (146).
● gpi7 strain: Mutant gpi7 strain produces long-lived plasma cells (LLPCs) that induce Dectin-1-facilitated innate immunity response regulating adaptive humoral immunity, facilitating the production of candidacidal antibodies. Specifically, it activates Th2 cell-mediated immune response by increasing IL-4 and IL-13 levels. This pathway could also initiate RelB induction to stimulate IL-18, thus generating a novel horizon for adaptive humoral immunity as well as innate immunity mediated by Dectin-1 (44).
4.1.4 Subunit vaccine
● rHyr1p-N: Hyphal-regulated protein-1 (Hyr1) helps in the growth of fungal hyphae, which evades phagocytolysis, an important host defense mechanism, thus contributing to virulence, in which recombinant Hyr1 produces immunity mediated by high levels of IgG and IgA antibodies. These antibodies promote effective opsonic activity, cytotoxicity, complement activation, and improved neutrophilic chemotaxis. rHyr1p-N, formulated with Freund’s adjuvant (FA), effectively shields neonatal mice from systemic candidiasis by inducing a vertically transmitted strong immune response (147).
● Hsp90-CTD: The heat shock protein 90 C-terminal domain, which is also known as Hsp90-CTD, mixed with polyethyleneimine (PEI) formed the nano-vaccine. The vaccine induces a rapid and long-lasting humoral and cellular antibody response. It also significantly decreased kidney damage by candidal infection in a murine model (148).
● rSap2: Secretory aspartate protease (Sap) helps in bond formation, hyphal development, biofilm establishment, and immune response facilitating penetration of host cells. The vaccine produces both humoral and cellular immune responses by Sap2-specific antibodies (IgG and IgM) and neutrophil-mediated fungicidal activity and boosts Th1/Th2/Th17 cytokine levels, indicating an immunomodulatory role (149).
● NDV-3A: A phase 2 trial was conducted to check the effectiveness of the NDV-3A vaccine. The preparation was based on recombinant hypha-precise surface antigen (Als3) fortified with alum. It included 188 women with recurrent vulvovaginal candidiasis (RVVC). The study demonstrated safety and effectiveness by producing strong rapid B- and T-cell immune response. Results of the trial facilitated the effectiveness of NDV-3A in treating vulvovaginal candidiasis. Disease recurrence rates were also reduced (150).
● CaS1: It targets fungal enolase (Eno1) and disrupts the binding of CaEno1 to plasminogen. Based on an in vitro experiment, scFv CaS1 (a single-chain variable antibody) has anti-fungal properties comparable to fluconazole. Furthermore, the intramuscular administration of CaS1 in white leghorn chicken leads to a distinguished reduction in fungal load and cytokine levels by producing specific IgG antibodies (151).
4.2 Invasive aspergillosis
4.2.1 Heat-killed vaccine
4.2.1.1 HK-sgl1
The sterylglucosidase-encoding gene (SGL1)-deficient, avirulent A. fumigatus strain (Δsgl1) demonstrated whole host protection in the mouse model. SGs are immunomodulatory glycolipids added with Δsgl1 as adjuvants. Mice with intranasal inoculation with Δsgl1 conidia survived without any visible morbidity signs until day 75 post-test while all control group mice succumbed before day 15 (152).
4.2.2 Subunit vaccine
● AF. KEX1: This vaccine targets recombinant endoprotease Kexin. AF. KEX1-vaccinated murine produced reduced mortality rate and significantly inhibited fungal load in lung compared to non-vaccinated mice. This is inversely associated with the anti-AF. KEX1 IgG antibody levels were determined following subcutaneous injection (153).
● VesiVax® Af3/9: Two liposomal formulations developed as VesiVax® Af3 and VesiVax® were conjugated to form this vaccine. Added with Lipidated Tucaresol, Monophosphoryl Lipid A, and PAM-3-CAG as adjuvant, the vaccine produced protective CD4+ T-cell immunity. It provided protection to the immunized mice (154).
4.3 Invasive cryptococcosis
4.3.1 Cell-based vaccine
4.3.1.1 DC vaccine
The vaccine produces protective pro-inflammatory responses including neutrophil stimulation and granuloma formation in cryptococcosis by promoting long-term memory Th17 cells, which can be demonstrated at least 6 months post-vaccination in the lungs. It also induces IL-17A upon antigen re-challenge (155).
4.3.2 Heat-killed vaccine
● H99γ: An epigenetically engineered C. neoformans strain induced immunity generated in immunocompromised T cell-deficient mice by expressing IFN-γ. This protection is directed by STAT1-mediated classical macrophage (MΦ) activation, which is associated with TII against cryptococcosis (156, 157).
● Znf2: It is a regulatory protein that helps in the transition of dimorphic fungi also interacting with the host. Vaccines with ZNF2 overexpression, both heat-killed (HK) or live attenuated (LA) formulations, reduce virulence and promote protective immunity in mice from lethal fungal strain. These cells with overexpressed ZNF2 help the host to distinguish protective Th cells, induced Th1/Th17 immune response of CD41 T cells in murine lungs at post-immunization day 7 (158).
● HK/LA-cda1Δcda2Δ: The mutant strain lacks two key enzymes (Cda1 and Cda2) that help in converting chitin to chitosan in the cell wall. This modification is vital for the virulence. Without sufficient chitosan, the fungus is more vulnerable to host defenses and is cleared more effectively. The vaccine incorporating the cda1Δcda2Δ mutant strain induces a Th1- and Th2-mediated strong protective adaptive immune response in mice (159).
4.3.3 Live attenuated vaccine
● GXM-SG: The vaccine accumulates sterylglucosides (SGs) and retains capsular polysaccharide GXM. Fungal γδ T cells, by activating TLR2 signaling pathway, induce IFN-γ and IL-17A. The vaccine generates a strong γδ T-cell response indicating a mixed Th1 and Th17 type of immunity, which is crucial for host protection in immunocompromised animals (87, 160).
● Sgl1: Ergosterol 3β-D-glucoside is accumulated by live attenuated Sgl1 deficit C. neoformans(Cn) strain (Δ Sgl1), which converts Cn avirulent and protects immunocompromised mice (161).
● Fbp1: E3 ligase lacking SCF subunit protein (Fbp1) helps in proteolysis and fungal virulence. HK-fbp1 revealed increased Th1 and Th17 responses, demonstrated by augmented IFN-γ and IL-17A titer, which is protective against virulent strain, even in immunocompromised mice. Early-treated animals also exhibited decreased Th2 cytokines (IL-13). This result is significant in the context of the HIV/AIDS-induced immunocompromised state, which is a profound risk factor in humans (24, 162).).
4.3.4 Subunit vaccine
● Cda2-Pep1: The vaccine based on peptides Cda2 produced from the C. neoformans ZNF2-brf1Δ mutant strain helps in stimulating robust CD4+ T-cell responses. Animals vaccinated with Cda2-Pep1 were significantly protected compared to the unvaccinated group. GP adjuvant promotes protective Th1 and Th17 responses (163).
● Glucan particle vaccine: This was loaded with recombinant Cryptococcal proteins. The vaccine employs GPs to deliver recombinant proteins, which stimulate the immune system to recognize and combat Cryptococcus infections. The immune response involves both humoral and cellular type. GPs added with mouse serum albumin (MSA) engaging Dectin-1 and complement receptors promote a strong activation of innate immune responses in a murine model (164).
● EV: The lipid bilayer of EVs is coated with a mannoprotein-embedded fibrillar embellishment, with the capsular polysaccharide forming the outermost covering. BALB/c mice immunized with two different doses (1 and 10 μg) of EV-protein derived from an avirulent Cn mutant strain (cap59Δ) showed a robust IgG antibody production that extend the survival time in a murine model. These results point to the fact that cryptococcal EVs can induce an adaptive immunity (82).
4.4 Invasive mucormycosis
4.4.1 Multivalent peptide vaccine
4.4.1.1 BFV
The vaccine targets the ferrous permease (FTR1) protein, necessary for iron accumulation by fungi, important for their virulence. This epitope (CTL, HTL, and LBL)-based vaccine produces a strong immunogenic response due to CTL, HTL, and LBL response. Added adjuvants RS09 and human β-defensin-3 help induce innate as well as adaptive immunity by activating B cells, Th cells, macrophages, cytotoxic T cells, and natural killer (NK) cells in a mouse model of mucormycoses (84).
4.5 Invasive histoplasmosis
4.5.1 Subunit vaccine
4.5.1.1 Alkaline extract of Histoplasma capsulatum
The alkaline extract, composed of GPs, induces robust Th1/Th17 cell base immunity by producing both IFN-γ and IL-17 in organs such as the lungs. The vaccine also decreased approximately 80% of fungal load and significantly improved survival in an animal model (165).
4.6 Invasive coccidioidomycosis
4.6.1 Cell-based vaccine
4.6.1.1 Ag2/pRA-DC
The vaccine involves the use of DCs transfected with Ag2/PRA plasmid DNA to induce immunity. DC activates T cell-mediated immune response. The vaccinated mice showed no visible signs of morbidity or no noteworthy deviations in lung injury indicators like lactate, albumin, and other lung proteins. The vaccine induced cytokine-secreting cells (IL-17, IL-4, and IFN-γ) in murine organs. It also resulted in augmented serum IgG levels (111).
4.6.2 Live attenuated vaccine
4.6.2.1 Cps1
The Δcps1 vaccine induces adaptive immunity. Subcutaneous immunization with live Δcps1 arthroconidia of beagle dogs alleviated pulmonary coccidioidomycosis by elevated CD4+ T cells releasing IFN-γ in organs such as the lungs and splenocyte of immunized dogs. No animal demonstrated sickness, thus not warranting clinical veterinary consultation. Every dog was immune from clinically relevant coccidioidomycosis (166).
4.6.3 Subunit vaccine
4.6.3.1 GCP-rCpa1
The vaccine consists of a recombinant Coccidioides antigen (rCpa1) packaged with adjuvant Glucan Chitin Particle (GCP). This vaccine produces a strong Th17 response via CARD9-mediated Dectin-1/2 signaling pathway activation (175). GCP-rCpa1 stirred greater macrophage penetration at injection sites. It demonstrated a meaningfully greater decrease of fungal load in the HLA-DR4 transgenic murine model, which also showed increased IL-17 production by Th1/Th17 cells. They also exhibited increased survival rates compared to animals immunized with GCPs alone (167).
4.7 Invasive paracoccidioidomycosis
4.7.1 Subunit vaccine
4.7.1.1 Peptide P10
The vaccine encompasses P. brasiliensis glycoprotein-derived peptide P10, absorbing with cationic liposomes (CAF01). The vaccine induces Th1 response, leading to cellular immunity. P10 and CAF01 significantly decreased fungal load in the lungs of infected mice and was associated with protective granulomatous tissue responses, indicating effective therapeutic potential against Para-coccidioidomycosis (168). This peptide encapsulated with chitosan nanoparticles improved bioavailability and stability and demonstrated immunomodulatory effect enhancement. It caused mixed Th1 and Th2 immune responses. The intranasal inoculation of the P10-chitosan nanoparticle vaccine reduced the fungal burden (CFUs) in the lungs of vaccinated mice. Notably, the conjugated P10-chitosan nanoparticles permitted a maximum of 20-fold vaccine dose reduction compared to previous studies (169).
4.8 Invasive blastomycoses
4.8.1 Subunit vaccine
4.8.1.1 Bl-Eng2
δ-inulin-based adjuvants (Advax) mixed with agonists of Toll-like receptor (TLR) boost endoglucanase2 (Bl-Eng2)-mediated immune response, producing proinflammatory antigen and dectin-2 pathway agonist. Bl-Eng2/Advax3 comprising TLR9 agonist and Bl-Eng2/Advax8 comprising TLR4 agonist are more effective in providing the best immunity against pulmonary Blastomycoses rather than complete FA. Overall, 50% of mice vaccinated with Bl-Eng2/Advax3 had survived up to 24 days, whereas animal vaccinated with only Bl-Eng2 succumbed 14 days post-vaccination. Among the adjuvants, Advax3 was better in producing IFN-γ and IL-17, which releases Bl-Eng2-specific T cells (170).
4.9 Invasive Pneumocystis pneumonia
4.9.1 Subunit vaccine
● Recombinant A121–85: This is derived from the B-cell epitope region of the A12 surface protein of P. carinii. Recombinant A121–85 protein induced both types of immune responses (humoral and cellular) by increasing serum IgG titer, promoting IFN-γ secretion by CD4+ and CD8+ T cells, and elevating the titer of pro-inflammatory cytokines like IL-17, IFN-γ, and IL-12 in the lungs. Vaccination with recombinant A121–85 meaningfully reduced the fungal load in an immunocompromised murine model (171).
● Recombinant KEX1: This vaccine induced robust and durable humoral immunity responses generated by memory B-cell responses against KEX1. Antibody titer could be maintained during tacrolimus- and methylprednisolone-induced immunosuppression in non-human primates (172).
● KEX1/(α -GC): The therapeutic immunization strategy boosts the immune response through adjuvant α-galactosylceramide. α-GC works by inducing invariant natural killer T (iNKT) cells. The KEX1/(α-GC) vaccine showed significant anti-KEX1 IgG titer elevation and produced long-lasting humoral memory responses without the presence of CD4+ T cells, vital for HIV-infected immunocompromised patients with diminished CD4+ T-cell counts (173).
4.10 Invasive sporotrichosis
4.10.1 Live attenuated vaccine
4.10.1.1 Δcps1
The Δcps1 mutant vaccine works by removing the CPS1 gene, which is vital for the survival and virulence. This deletion results in a strain that can stimulate an immune response without causing diseases. The Δcps1 vaccine induces a protective immune response characterized by the augmented release of Th1, Th17, IFN-γ, and IL-17A. This response helps in the activation of neutrophils and macrophages, leading to enhanced fungal clearance and reduced lesion size (142).
4.10.2 Subunit vaccine
4.10.2.1 SsEno/PGA
The vaccine contains recombinant enolase (rSsEno) from S. schenckii, mixed with Montanide™ PetGel A (PGA), a polymeric adjuvant containing dispersed sodium polyacrylate microparticles in water. Increased IFN-γ levels with Th1 cells (IFN-γ/CD4+ T cells) and elevated rSsEno-specific IgG titers produce a robust Th1 immune response. However, it did not induce a significant Th17 response (174).
4.11 Pan-fungal vaccine
● NXT-2: It is a recombinant peptide with vaccine-like features used in vaccinated mice and nonhuman primates against Aspergillus, Candida, and Pneumocystis. It generates antibodies that bind to the fungal surface inducing opsonophagocytic killing. It also induces strong and long-lasting plasma IgG titers leading to inhibited biofilm development, which is a vital virulence factor of Candida. In animal models of aspergillosis, NXT-2 associated with an important decline in mortality and lung fungal load and had a twofold mean survival period. In an HIV-infected primate model of Pneumocystis infection, reduced incidence of opportunistic infection and chronic colonization was reported. Overall, the NXT-2 vaccine shows promising broad-spectrum antifungal efficacy across multiple fungal pathogens in various animal models (67).
● CRM197: The β-glucan-based vaccine CRM197 can harvest protective immunity in mice diseased with C. albicans and A. fumigatus. β-Glucans are also a strong activator of TII (176).
5 Challenges and the way forward
Fungal infections pose a significant threat, particularly to immunocompromised individuals and those with multiple comorbidities as they often experience disseminated disease and have poor clinical outcomes. Despite the high disease burden, there are no licensed fungal vaccine preparations. Appropriate adjuvants and drug delivery systems that generate good immune response are currently limited (83).
Fungal immune response is highly variable, complex, and an unpredictable immunological phenomenon. As intraspecies and interspecies antigenic variation exists among the fungi, targeting multiple epitopes seems to be a pertinent solution. Translation response from animal models to humans in vaccine development is variable. Hence, reactogenicity and safety evaluation of the preclinical models must be robust. Experiments in multiple animal models, ex vivo studies in human cell lines, and the use of advanced bioinformatics can be a promising solution (177).
Pan-fungal vaccines to confer immunity against multiple systemic fungal infections can be a potent solution to the current problem. β-Glucan targets have shown to stimulate trained immunity, i.e., activation of innate immunity leading to epigenetic changes that enhance response to subsequent infections by a wide variety of fungal species. Common T- and B-cell epitope targets could be identified and combined to yield new candidates (178).
Nanotechnology-based drug designing can be an effective technique for a better outcome. Reverse vaccinology principles can be utilized to study the proteome of fungal species in in silico models. Computational strategies can be used to identify novel targets. EV-based fungal platforms (like Cryptococcus) are a promising way forward. Protective antigens of such fungal cell wall can act as antigenic targets for future development (10).
Exploring novel targets will hold the key to successful clinical development of fungal vaccines. Research efforts addressing key scientific and logistical barriers can pave the way for future availability of clinically approved effective fungal vaccines.
Author contributions
DA: Formal Analysis, Methodology, Project administration, Resources, Visualization, Writing – original draft, Writing – review & editing, Conceptualization, Data curation, Investigation, Supervision, Validation, Software. OB: Data curation, Formal analysis, Investigation, Methodology, Project administration, Resources, Software, Supervision, Validation, Visualization, Writing – original draft, Writing – review & editing. SP: Conceptualization, Formal analysis, Investigation, Methodology, Project administration, Resources, Supervision, Validation, Visualization, Writing – original draft, Writing – review & editing, Software. DP: Methodology, Project administration, Resources, Visualization, Writing – original draft, Writing – review & editing, Formal analysis, Supervision, Investigation, Software. BA: Conceptualization, Data curation, Funding acquisition, Investigation, Methodology, Project administration, Resources, Software, Supervision, Validation, Visualization, Writing – original draft, Writing – review & editing.
Funding
The author(s) declare that no financial support was received for the research, authorship, and/or publication of this article.
Conflict of interest
Author SP was employed by the company Wipro Limited.
The remaining authors declare that the research was conducted in the absence of any commercial or financial relationships that could be construed as a potential conflict of interest.
Publisher’s note
All claims expressed in this article are solely those of the authors and do not necessarily represent those of their affiliated organizations, or those of the publisher, the editors and the reviewers. Any product that may be evaluated in this article, or claim that may be made by its manufacturer, is not guaranteed or endorsed by the publisher.
References
1. Denning DW. Global incidence and mortality of severe fungal disease. Lancet Infect Diseases. (2024) 24:e428–38. doi: 10.1016/S1473-3099(23)00692-8
2. Brown GD, Denning DW, Gow NAR, Levitz SM, Netea MG, White TC. Hidden killers: human fungal infections. Sci Transl Med. (2012) 4(165):165–13. doi: 10.1126/scitranslmed.3004404
3. Henriet S, Verweij PE, Holland SM, Warris A. Invasive fungal infections in patients with chronic granulomatous disease. In: Curtis N, Finn A, Pollard AJ, editors. Hot Topics in Infection and Immunity in Children IX. Springer New York, New York, NY (2013). p. 27–55. doi: 10.1007/978-1-4614-4726-9_3
4. Hoenigl M. Invasive fungal disease complicating coronavirus disease 2019: when it rains, it spores. Clin Infect Diseases. (2021) 73:e1645–8. doi: 10.1093/cid/ciaa1342
5. Vallabhaneni S, Chiller TM. Fungal infections and new biologic therapies. Curr Rheumatol Rep. (2016) 18:29. doi: 10.1007/s11926-016-0572-1
6. Perlin DS, Rautemaa-Richardson R, Alastruey-Izquierdo A. The global problem of antifungal resistance: prevalence, mechanisms, and management. Lancet Infect Diseases. (2017) 17:e383–92. doi: 10.1016/S1473-3099(17)30316-X
7. Drgona L, Khachatryan A, Stephens J, Charbonneau C, Kantecki M, Haider S, et al. Clinical and economic burden of invasive fungal diseases in Europe: focus on pre-emptive and empirical treatment of Aspergillus and Candida species. Eur J Clin Microbiol Infect Dis. (2014) 33:7–21. doi: 10.1007/s10096-013-1944-3
8. Shoham S, Marr KA. Invasive fungal infections in solid organ transplant recipients. Future Microbiol. (2012) 7:639–55. doi: 10.2217/fmb.12.28
9. Santos E, Levitz SM. Fungal vaccines and immunotherapeutics. Cold Spring Harbor Perspect Med. (2014) 4:a019711–a019711. doi: 10.1101/cshperspect.a019711
10. Inácio MM, Moreira ALE, Cruz-Leite VRM, Mattos K, Silva LOS, Venturini J, et al. Fungal vaccine development: state of the art and perspectives using immunoinformatics. J Fungi (Basel). (2023) 9:633. doi: 10.3390/jof9060633
11. Burchill MA, Tamburini BA, Pennock ND, White JT, Kurche JS, Kedl RM. T cell vaccinology: exploring the known unknowns. Vaccine. (2013) 31(2):297–305. doi: 10.1016/j.vaccine.2012.10.096
12. Giron LB, Dweep H, Yin X, Wang H, Damra M, Goldman AR, et al. Plasma markers of disrupted gut permeability in severe COVID-19 patients. Front Immunol. (2020) 12:686240. doi: 10.1101/2020.11.13.20231209
13. Feldmesser M. Prospects of vaccines for medically important fungi. Med Mycol. (2005) 43:571–87. doi: 10.1080/13693780500402138
14. Kaur G, Chawla S, Kumar P, Singh R. Advancing vaccine strategies against candida infections: exploring new frontiers. Vaccines. (2023) 11:1658. doi: 10.3390/vaccines11111658
15. Pagano L, Mayor S. Invasive fungal infections in high-risk patients: report from TIMM-8 2017. Future Sci OA. (2018) 4:FSO307. doi: 10.4155/fsoa-2018-0019
16. Ribes JA, Vanover-Sams CL, Baker DJ. Zygomycetes in human disease. Clin Microbiol Rev. (2000) 13:236–301. doi: 10.1128/CMR.13.2.236
17. Baddley JW. Clinical risk factors for invasive aspergillosis. Med Mycol. (2011) 49(Suppl 1):S7–12. doi: 10.3109/13693786.2010.505204
18. Malcolm TR, Chin-Hong PV. Endemic mycoses in immunocompromised hosts. Curr Infect Dis Rep. (2013) 15:536–43. doi: 10.1007/s11908-013-0387-4
19. Fang W, Wu J, Cheng M, Zhu X, Du M, Chen C, et al. Diagnosis of invasive fungal infections: challenges and recent developments. J Biomed Science. (2023) 30:42. doi: 10.1186/s12929-023-00926-2
20. WHO fungal priority pathogens list to guide research, development and public health action. Available online at: https://www.who.int/publications-detail-redirect/9789240060241.
21. Wüthrich M, Filutowicz HI, Klein BS. Mutation of the WI-1 gene yields an attenuated Blastomyces dermatitidis strain that induces host resistance. J Clin Invest. (2000) 106:1381–9. doi: 10.1172/JCI11037
22. Saville SP, Lazzell AL, Chaturvedi AK, Monteagudo C, Lopez-Ribot JL. Efficacy of a Genetically Engineered Candida albicans tet-NRG1 Strain as an Experimental Live Attenuated Vaccine against Hematogenously Disseminated Candidiasis. Clin Vaccine Immunol. (2009) 16:430–2. doi: 10.1128/CVI.00480-08
23. Fernández-Arenas E, Molero G, Nombela C, Diez-Orejas R, Gil C. Low virulent strains of Candida albicans: unravelling the antigens for a future vaccine. Proteomics. (2004) 4:3007–20. doi: 10.1002/pmic.200400929
24. Wang Y, Wang K, Masso-Silva JA, Rivera A, Xue C. A heat-killed cryptococcus mutant strain induces host protection against multiple invasive mycoses in a murine vaccine model. mBio. (2019) 10:e02145–19. doi: 10.1128/mBio.02145-19
25. Martinez M, Clemons KV, Stevens DA. Heat-killed yeast as a pan-fungal vaccine. Methods Mol Biol. (2017) 1625:23–30. doi: 10.3390/vaccines11111625/2017
26. Edwards JE, Schwartz MM, Schmidt CS, Sobel JD, Nyirjesy P, Schodel F, et al. A fungal immunotherapeutic vaccine (NDV-3A) for treatment of recurrent vulvovaginal candidiasis—A phase 2 randomized, double-blind, placebo-controlled trial. Clin Infect Dis. (2018) 66:1928–36. doi: 10.1093/cid/ciy185
27. De Bernardis F, Amacker M, Arancia S, Sandini S, Gremion C, Zurbriggen R, et al. A virosomal vaccine against candidal vaginitis: immunogenicity, efficacy and safety profile in animal models. Vaccine. (2012) 30:4490–8. doi: 10.1016/j.vaccine.2012.04.069
28. Devi S. Preclinical efficacy of a glucuronoxylomannan-tetanus toxoid conjugate vaccine of Cryptococcus neoformans in a murine model. Vaccine. (1996) 14:841–4. doi: 10.1016/0264-410X(95)00256-Z
29. Chow SK, Casadevall A. Evaluation of Cryptococcus neoformans galactoxylomannan-protein conjugate as vaccine candidate against murine cryptococcosis. Vaccine. (2011) 29:1891–8. doi: 10.1016/j.vaccine.2010.12.134
30. Torosantucci A, Bromuro C, Chiani P, De Bernardis F, Berti F, Galli C, et al. A novel glyco-conjugate vaccine against fungal pathogens. J Exp Med. (2005) 202:597–606. doi: 10.1084/jem.20050749
31. Nami S, Mohammadi R, Vakili M, Khezripour K, Mirzaei H, Morovati H. Fungal vaccines, mechanism of actions and immunology: A comprehensive review. Biomedicine Pharmacotherapy. (2019) 109:333–44. doi: 10.1016/j.biopha.2018.10.075
32. De Amorim J, Magalhães A, Muñoz JE, Rittner GMG, Nosanchuk JD, Travassos LR, et al. DNA vaccine encoding peptide P10 against experimental paracoccidioidomycosis induces long-term protection in presence of regulatory T cells. Microbes Infection. (2013) 15:181–91. doi: 10.1016/j.micinf.2012.11.007
33. Fan H, Guo JY, Ma SL, Zhang N, An CL. Synthetic p55 tandem DNA vaccine against Pneumocystis carinii in rats. Microbiol Immunol. (2016) 60:397–406. doi: 10.1111/1348-0421.12386
34. Wüthrich M, Brandhorst TT, Sullivan TD, Filutowicz H, Sterkel A, Stewart D, et al. Calnexin induces expansion of antigen-specific CD4+ T cells that confer immunity to fungal ascomycetes via conserved epitopes. Cell Host Microbe. (2015) 17:452–65. doi: 10.1016/j.chom.2015.02.009
35. Effenberg R, Turánek Knötigová P, Zyka D, Čelechovská H, Mašek J, Bartheldyová E, et al. Nonpyrogenic Molecular Adjuvants Based on norAbu-Muramyldipeptide and norAbu-Glucosaminyl Muramyldipeptide: Synthesis, Molecular Mechanisms of Action, and Biological Activities in Vitro and in Vivo. J Med Chem. (2017) 60:7745–63. doi: 10.1021/acs.jmedchem.7b00593
36. Ribeiro AM, Souza ACO, Amaral AC, Vasconcelos NM, Jeronimo MS, Carneiro FP, et al. Nanobiotechnological approaches to delivery of DNA vaccine against fungal infection. J BioMed Nanotechnol. (2013) 9:221–30. doi: 10.1166/jbn.2013.1491
37. Pappas PG, Lionakis MS, Arendrup MC, Ostrosky-Zeichner L, Kullberg BJ. Invasive candidiasis. Nat Rev Dis Primers. (2018) 4:18026. doi: 10.1038/nrdp.2018.26
38. Chapman SW, Dismukes WE, Proia LA, Bradsher RW, Pappas PG, Threlkeld MG, et al. Clinical practice guidelines for the management of blastomycosis: 2008 update by the infectious diseases society of America. Clin Infect Diseases. (2008) 46:1801–12. doi: 10.1086/588300
39. Clancy CJ, Nguyen MH. Diagnosing invasive candidiasis. J Clin Microbiol. (2018) 56:e01909–17. doi: 10.1128/JCM.01909-17
40. Musilova M, Tranter M, Bennett SA, Wadham J, Anesio AM. Stable microbial community composition on the Greenland Ice Sheet. Front Microbiol. (2015) 6:193. doi: 10.3389/fmicb.2015.00193
41. Netea MG, Joosten LAB, van der Meer JWM, Kullberg BJ, van de Veerdonk FL. Immune defence against Candida fungal infections. Nat Rev Immunol. (2015) 15:630–42. doi: 10.1038/nri3897
42. Tso GHW, Reales-Calderon JA, Pavelka N. The elusive anti-candida vaccine: lessons from the past and opportunities for the future. Front Immunol. (2018) 9:897. doi: 10.3389/fimmu.2018.00897
43. Bistoni F, Vecchiarelli A, Cenci E, Puccetti P, Marconi P, Cassone A. Evidence for macrophage-mediated protection against lethal Candida albicans infection. Infect Immun. (1986) 51:668–74. doi: 10.1128/iai.51.2.668-674.1986
44. Shen H, Yu Y, Chen SM, Sun JJ, Fang W, Guo SY, et al. Dectin-1 facilitates IL-18 production for the generation of protective antibodies against candida albicans. Front Microbiol. (2020) 11:1648. doi: 10.3389/fmicb.2020.01648
45. Austriaco N. Yeast oral vaccines against infectious diseases. Front Microbiol. (2023) 14:1150412. doi: 10.3389/fmicb.2023.1150412
46. Nami S, Aghebati-Maleki A, Morovati H, Aghebati-Maleki L. Current antifungal drugs and immunotherapeutic approaches as promising strategies to treatment of fungal diseases. BioMed Pharmacother. (2019) 110:857–68. doi: 10.1016/j.biopha.2018.12.009
47. Wang Xj, Sui X, Yan L, Wang Y, Cao Yb, Jiang Yy. Vaccines in the treatment of invasive candidiasis. Virulence. (2015) 6:309–15. doi: 10.4161/21505594.2014.983015
48. Ibrahim AS, Spellberg BJ, Avanesian V, Fu Y, Edwards JE. The anti- candida vaccine based on the recombinant N-terminal domain of als1p is broadly active against disseminated candidiasis. Infect Immun. (2006) 74:3039–41. doi: 10.1128/IAI.74.5.3039-3041.2006
49. Schmidt CS, White CJ, Ibrahim AS, Filler SG, Fu Y, Yeaman MR, et al. NDV-3, a recombinant alum-adjuvanted vaccine for Candida and Staphylococcus aureus, is safe and immunogenic in healthy adults. Vaccine. (2012) 30:7594–600. doi: 10.1016/j.vaccine.2012.10.038
50. Alqarihi A, Singh S, Edwards JE, Ibrahim AS, Uppuluri P. NDV-3A vaccination prevents C. albicans colonization of jugular vein catheters in mice. Sci Rep. (2019) 9:6194. doi: 10.1038/s41598-019-42517-y
51. Shibasaki S. Oral Immunization Against Candidiasis Using Lactobacillus casei Displaying Enolase 1 from Candida albicans. Sci Pharm. (2014) 82:697–708. doi: 10.3797/(ISSN)0036-8709
52. Sahu SR, Bose S, Singh M, Kumari P, Dutta A, Utkalaja BG, et al. Vaccines against candidiasis: Status, challenges and emerging opportunity. Front Cell Infect Microbiol. (2022) 12:1002406. doi: 10.3389/fcimb.2022.1002406
53. Cárdenas-Freytag L, Cheng E, Mayeux P, Domer JE, Clements JD. Effectiveness of a Vaccine Composed of Heat-Killed Candida albicans and a Novel Mucosal Adjuvant, LT(R192G), against Systemic Candidiasis. Kozel TR editor. Infect Immun. (1999) 67:826–33. doi: 10.1128/IAI.67.2.826-833.1999
54. Liu M, Clemons KV, Johansen ME, Martinez M, Chen V, Stevens DA. Saccharomyces as a vaccine against systemic candidiasis. Immunol Investigations. (2012) 41:847–55. doi: 10.3109/08820139.2012.692418
55. Stevens DA, Clemons KV, Liu M. Developing a vaccine against aspergillosis. Med Mycol. (2011) 49(Suppl 1):S170–176. doi: 10.3109/13693786.2010.497775
56. Kumar R, Kumar P. Yeast-based vaccines: New perspective in vaccine development and application. FEMS Yeast Res. (2019) 19(2):foz007. doi: 10.1093/femsyr/foz007/5298404
57. Shibasaki S, Aoki W, Nomura T, Miyoshi A, Tafuku S, Sewaki T, et al. An oral vaccine against candidiasis generated by a yeast molecular display system. Pathog Disease. (2013) 69:262–8. doi: 10.1111/fim.2013.69.issue-3
58. Aoki W, Ueda T, Tatsukami Y, Kitahara N, Morisaka H, Kuroda K, et al. Time-course proteomic profile of Candida albicans during adaptation to a fetal serum. Pathog Disease. (2013) 67:67–75. doi: 10.1111/2049-632X.12003
59. Chen X, Wang J, Wang S, Jin J, Li J, Gao S, et al. Real-world assessment of the effectiveness of posaconazole for the prophylaxis and treatment of invasive fungal infections in hematological patients: A retrospective observational study. Med (Baltimore). (2021) 100:e26772. doi: 10.1097/MD.0000000000026772
60. Abdelnaby MA, Shoueir KR, Ghazy AA, Abdelhamid SM, El Kemary MA, Mahmoud HE, et al. Synthesis and evaluation of metallic nanoparticles-based vaccines against Candida albicans infections. J Drug Delivery Sci Technology. (2022) 68:102862. doi: 10.1016/j.jddst.2021.102862
61. Bongomin F, Gago S, Oladele R, Denning D. Global and multi-national prevalence of fungal diseases—Estimate precision. JoF. (2017) 3:57. doi: 10.3390/jof3040057
62. Gago S, Denning DW, Bowyer P. Pathophysiological aspects of Aspergillus colonization in disease. Med Mycology. (2019) 57:S219–27. doi: 10.1093/mmy/myy076
64. Rivera A, Van Epps HL, Hohl TM, Rizzuto G, Pamer EG. Distinct CD4 + -T-cell responses to live and heat-inactivated aspergillus fumigatus conidia. Infect Immun. (2005) 73:7170–9. doi: 10.1128/IAI.73.11.7170-7179.2005
65. Clemons KV, Martinez M, Chen V, Liu M, Yoon HJ, Stevens DA. Protection against experimental aspergillosis by heat-killed yeast is not antibody dependent. Med Mycology. (2014) 52:422–6. doi: 10.1093/mmy/myt015
66. Pattison HT, Millar BC, Moore JE. Fungal vaccines. Br J BioMed Sci. (2021) 78:167–76. doi: 10.1080/09674845.2021.1907953
67. Rayens E, Rayens MK, Norris KA. Demographic and socioeconomic factors associated with fungal infection risk, United States, 2019. Emerg Infect Dis. (2022) 28:1955–69. doi: 10.3201/eid2810.220391
68. Perruccio K, Tosti A, Burchielli E, Topini F, Ruggeri L, Carotti A, et al. Transferring functional immune responses to pathogens after haploidentical hematopoietic transplantation. Blood. (2005) 106:4397–406. doi: 10.1182/blood-2005-05-1775
69. Bozza S, Perruccio K, Montagnoli C, Gaziano R, Bellocchio S, Burchielli E, et al. A dendritic cell vaccine against invasive aspergillosis in allogeneic hematopoietic transplantation. Blood. (2003) 102:3807–14. doi: 10.1182/blood-2003-03-0748
70. Wang YY, Pan YB, Wan ZY, Li JJ, Bao J, Zhang JS, et al. Anti-inflammatory Polyketides from an Endophytic Fungus Chaetomium sp. UJN-EF006 of Vaccinium bracteatum. Chem Biodivers. (2024) 21:e202400002. doi: 10.1002/cbdv.202400002
71. Levitz SM. Aspergillus vaccines: Hardly worth studying or worthy of hard study? Med Myco. (2017) 55:103–8. doi: 10.1093/mmy/myw081
72. Kwon-Chung KJ, Fraser JA, Doering TL, Wang ZA, Janbon G, Idnurm A, et al. Cryptococcus neoformans and Cryptococcus gattii, the Etiologic Agents of Cryptococcosis. Cold Spring Harbor Perspect Med. (2014) 4:a019760–a019760. doi: 10.1101/cshperspect.a019760
73. Rajasingham R, Smith RM, Park BJ, Jarvis JN, Govender NP, Chiller TM, et al. Global burden of disease of HIV-associated cryptococcal meningitis: an updated analysis. Lancet Infect Dis. (2017) 17:873–81. doi: 10.1016/S1473-3099(17)30243-8
74. Lin YY, Shiau S, Fang CT. Risk factors for invasive cryptococcus neoformans diseases: A case-control study. PloS One. (2015) 10:e0119090. doi: 10.1371/journal.pone.0119090
75. Voelz K, May RC. Cryptococcal interactions with the host immune system. Eukaryot Cell. (2010) 9:835–46. doi: 10.1128/EC.00039-10
76. Devi SJ, Schneerson R, Egan W, Ulrich TJ, Bryla D, Robbins JB, et al. Cryptococcus neoformans serotype A glucuronoxylomannan-protein conjugate vaccines: synthesis, characterization, and immunogenicity. Infect Immun. (1991) 59:3700–7. doi: 10.1128/iai.59.10.3700-3707.1991
77. Chakrabarti A, Dhaliwal M. Epidemiology of mucormycosis in India. Curr Fungal Infect Rep. (2013) 7:287–92. doi: 10.1007/s12281-013-0152-z
78. Nicolás FE, Murcia L, Navarro E, Navarro-Mendoza MI, Pérez-Arques C, Garre V. Mucorales species and macrophages. JoF. (2020) 6:94. doi: 10.3390/jof6020094
79. Soltan MA, Eldeen MA, Elbassiouny N, Kamel HL, Abdelraheem KM, El-Gayyed HA, et al. In Silico Designing of a Multitope Vaccine against Rhizopus microsporus with Potential Activity against Other Mucormycosis Causing Fungi. Cells. (2021) 10:3014. doi: 10.3390/cells10113014
80. Casadevall A, Pirofski L. Insights into Mechanisms of Antibody-Mediated Immunity from Studies with Cryptococcus neoformans. CMM. (2005) 5:421–33. doi: 10.2174/1566524054022567
81. Datta K, Lees A, Pirofski La. Therapeutic efficacy of a conjugate vaccine containing a peptide mimotope of cryptococcal capsular polysaccharide glucuronoxylomannan. Clin Vaccine Immunol. (2008) 15:1176–87. doi: 10.1128/CVI.00130-08
82. Rizzo J, Wong SSW, Gazi AD, Moyrand F, Chaze T, Commere PH, et al. Cryptococcus extracellular vesicles properties and their use as vaccine platforms. J Extracellular Vesicles. (2021) 10:e12129. doi: 10.1002/jev2.12129
83. Oliveira LVN, Wang R, Specht CA, Levitz SM. Vaccines for human fungal diseases: close but still a long way to go. NPJ Vaccines. (2021) 6:1–8. doi: 10.1038/s41541-021-00294-8
84. Araf Y, Moin AT, Timofeev VI, Faruqui NA, Saiara SA, Ahmed N, et al. Immunoinformatic design of a multivalent peptide vaccine against mucormycosis: targeting FTR1 protein of major causative fungi. Front Immunol. (2022) 13:863234. doi: 10.3389/fimmu.2022.863234
85. Upadhya R, Lam WC, Maybruck B, Specht CA, Levitz SM, Lodge JK. Induction of protective immunity to cryptococcal infection in mice by a heat-killed, chitosan-deficient strain of cryptococcus neoformans. mBio. (2016) 7:e00547–16. doi: 10.1128/mBio.00547-16
86. Ueno K, Yanagihara N, Shimizu K, Miyazaki Y. Vaccines and protective immune memory against cryptococcosis. Biol Pharm Bull. (2020) 43:230–9. doi: 10.1248/bpb.b19-00841
87. Colombo AC, Rella A, Normile T, Joffe LS, Tavares PM, de S Araújo GR, et al. Cryptococcus neoformans glucuronoxylomannan and sterylglucoside are required for host protection in an animal vaccination model. mBio. (2019) 10:e02909–18. doi: 10.1128/mBio.02909-18
88. Chechi JL, da Costa FAC, Figueiredo JM, de Souza CM, Valdez AF, Zamith-Miranda D, et al. Vaccine development for pathogenic fungi: current status and future directions. Expert Rev Vaccines. (2023) 22:1136–53. doi: 10.1080/14760584.2023.2279570
89. Soto ER, Specht CA, Rus F, Lee CK, Abraham A, Levitz SM, et al. An efficient (nano) silica - In glucan particles protein encapsulation approach for improved thermal stability. J Control Release. (2023) 357:175–84. doi: 10.1016/j.jconrel.2023.03.027
90. Soto ER, Specht CA, Lee CK, Levitz SM, Ostroff GR. One step purification—Vaccine delivery system. Pharmaceutics. (2023) 15:1390. doi: 10.3390/pharmaceutics15051390
91. Cornely OA, Hoenigl M, Lass-Flörl C, Chen SCA, Kontoyiannis DP, Morrissey CO, et al. Defining breakthrough invasive fungal infection-Position paper of the mycoses study group education and research consortium and the European Confederation of Medical Mycology. Mycoses. (2019) 62:716–29. doi: 10.1111/myc.12960
92. Naveed M, Ali U, Karobari MI, Ahmed N, Mohamed RN, Abullais SS, et al. A vaccine construction against COVID-19-associated mucormycosis contrived with immunoinformatics-based scavenging of potential mucoralean epitopes. Vaccines (Basel). (2022) 10(5):664. doi: 10.3390/vaccines10050664
93. Wheat LJ, Azar MM, Bahr NC, Spec A, Relich RF, Hage C. Histoplasmosis. Infect Dis Clinics North America. (2016) 30:207–27. doi: 10.1016/j.idc.2015.10.009
94. Kauffman CA. Histoplasmosis: a clinical and laboratory update. Clin Microbiol Rev. (2007) 20:115–32. doi: 10.1128/CMR.00027-06
95. Wheat LJ, Freifeld AG, Kleiman MB, Baddley JW, McKinsey DS, Loyd JE, et al. Clinical practice guidelines for the management of patients with histoplasmosis: 2007 update by the infectious diseases society of America. Clin Infect Dis. (2007) 45:807–25. doi: 10.1086/521259
96. Gerson SL. Prolonged granulocytopenia: the major risk factor for invasive pulmonary aspergillosis in patients with acute leukemia. Ann Intern Med. (1984) 100:345. doi: 10.7326/0003-4819-100-3-345
97. Wheat L. State-of-the-art review of pulmonary fungal infections. Semin Respir Infections. (2002) 17:158–81. doi: 10.1053/srin.2002.33685
98. Roth MT, Zamith-Miranda D, Nosanchuk JD. Immunization strategies for the control of histoplasmosis. Curr Trop Med Rep. (2019) 6:35–41. doi: 10.1007/s40475-019-00172-3
99. Deepe GS. Preventative and therapeutic vaccines for fungal infections: from concept to implementation. Expert Rev Vaccines. (2004) 3:701–9. doi: 10.1586/14760584.3.6.701
100. Guimarães AJ, Frases S, Gomez FJ, Zancopé-Oliveira RM, Nosanchuk JD. Monoclonal antibodies to heat shock protein 60 alter the pathogenesis of histoplasma capsulatum. Infect Immun. (2009) 77:1357–67. doi: 10.1128/IAI.01443-08
101. Nosanchuk JD, Zancopé-Oliveira RM, Hamilton AJ, Guimarães AJ. Antibody therapy for histoplasmosis. Front Microbio. (2012) 3. doi: 10.3389/fmicb.2012.00021
102. Almeida PCS, Roque BS, Felice AG, Jaiswal AK, Tiwari S, Azevedo V, et al. Comparative genomics of histoplasma capsulatum and prediction of new vaccines and drug targets. JoF. (2023) 9:193. doi: 10.3390/jof9020193
103. Rosenstein NE, Emery KW, Werner SB, Kao A, Johnson R, Rogers D, et al. Risk factors for severe pulmonary and disseminated coccidioidomycosis: kern county, California, 1995-1996. Clin Infect Dis. (2001) 32:708–14. doi: 10.1086/319203
104. Kusne S, Taranto S, Covington S, Kaul DR, Blumberg EA, Wolfe C, et al. Coccidioidomycosis transmission through organ transplantation: A report of the OPTN ad hoc disease transmission advisory committee. Am J Transplant. (2016) 16:3562–7. doi: 10.1111/ajt.13950
105. Kong YC, Levine HB. Experimentally induced immunity in the mycoses. Bacteriol Rev. (1967) 31:35–53. doi: 10.1128/br.31.1.35-53.1967
106. Pappagianis D. Evaluation of the protective efficacy of the killed coccidioides immitis spherule vaccine in humans. Am Rev Respir Dis. (1993) 148:656–60. doi: 10.1164/ajrccm/148.3.656
107. Zimmermann CR, Johnson SM, Martens GW, White AG, Zimmer BL, Pappagianis D. Protection against lethal murine coccidioidomycosis by a soluble vaccine from spherules. Infect Immun. (1998) 66:2342–5. doi: 10.1128/IAI.66.5.2342-2345.1998
108. Pan S, Cole GT. Molecular and biochemical characterization of a Coccidioides immitis-specific antigen. Infect Immun. (1995) 63:3994–4002. doi: 10.1128/iai.63.10.3994-4002.1995
109. Hayden CA, Hung CY, Zhang H, Negron A, Esquerra R, Ostroff G, et al. Maize-produced ag2 as a subunit vaccine for valley fever. J Infect Dis. (2019) 220:615–23. doi: 10.1093/infdis/jiz196
110. Castro-Lopez N, Hung CY. Immune response to coccidioidomycosis and the development of a vaccine. Microorganisms. (2017) 5(1):13. doi: 10.3390/microorganisms5010013
111. Awasthi S, Vilekar P, Conkleton A, Rahman N. Dendritic cell-based immunization induces Coccidioides Ag2/PRA-specific immune response. Vaccine. (2019) 37:1685–91. doi: 10.1016/j.vaccine.2019.01.034
112. Boniche-Alfaro C, Kischkel B, Thomaz L, Carvalho-Gomes MM, Lopes-Bezerra LM, Nosanchuk JD, et al. Antibody- based immunotherapy combined with antimycotic drug TMP- SMX to treat infection with paracoccidioides brasiliensis. Front Immunol. (2021) 12:725882. doi: 10.3389/fimmu.2021.725882
113. Martinez R. EPIDEMIOLOGY OF PARACOCCIDIOIDOMYCOSIS. Rev Inst Med Trop S Paulo. (2015) 57:11–20. doi: 10.1590/S0036-46652015000700004
114. Jenks JD, White PL, Kidd SE, Goshia T, Fraley SI, Hoenigl M, et al. An update on current and novel molecular diagnostics for the diagnosis of invasive fungal infections. Expert Rev Mol Diagn. (2023) 23:1135–52. doi: 10.1080/14737159.2023.2267977
115. Benard G. Pathogenesis and classification of paracocidioidomycosis: new insights from old good stuff. Open Forum Infect Dis. (2021) 8:ofaa624. doi: 10.1093/ofid/ofaa624
116. Rittner GMG, Muñoz JE, Marques AF, Nosanchuk JD, Taborda CP, Travassos LR. Therapeutic DNA vaccine encoding peptide P10 against experimental paracoccidioidomycosis. PloS Negl Trop Dis. (2012) 6:e1519. doi: 10.1371/journal.pntd.0001519
117. Magalhães A, Ferreira KS, Almeida SR, Nosanchuk JD, Travassos LR, Taborda CP. Prophylactic and therapeutic vaccination using dendritic cells primed with peptide 10 derived from the 43-kilodalton glycoprotein of paracoccidioides brasiliensis. Clin Vaccine Immunol. (2012) 19:23–9. doi: 10.1128/CVI.05414-11
118. Braga CJM, Rittner GMG, Muñoz Henao JE, Teixeira AF, Massis LM, Sbrogio-Almeida ME, et al. Paracoccidioides brasiliensis Vaccine Formulations Based on the gp43-Derived P10 Sequence and the Salmonella enterica FliC Flagellin. Infect Immun. (2009) 77:1700–7. doi: 10.1128/IAI.01470-08
119. Boniche C, Rossi SA, Kischkel B, Barbalho FV, Moura ÁND, Nosanchuk JD, et al. Immunotherapy against systemic fungal infections based on monoclonal antibodies. J Fungi (Basel). (2020) 6:31. doi: 10.3390/jof6010031
120. De Mattos Grosso D, De Almeida SR, Mariano M, Lopes JD. Characterization of gp70 and Anti-gp70 Monoclonal Antibodies in Paracoccidioides brasiliensis Pathogenesis. Infect Immun. (2003) 71:6534–42. doi: 10.1128/IAI.71.11.6534-6542.2003
121. Buissa-Filho R, Puccia R, Marques AF, Pinto FA, Muñoz JE, Nosanchuk JD, et al. The Monoclonal Antibody against the Major Diagnostic Antigen of Paracoccidioides brasiliensis Mediates Immune Protection in Infected BALB/c Mice Challenged Intratracheally with the Fungus. Infect Immun. (2008) 76:3321–8. doi: 10.1128/IAI.00349-08
122. Bariola J, Vyas K. Pulmonary blastomycosis. Semin Respir Crit Care Med. (2011) 32:745–53. doi: 10.1055/s-0031-1295722
123. Castillo CG, Kauffman CA, Miceli MH. Blastomycosis. Infect Dis Clinics North America. (2016) 30:247–64. doi: 10.1016/j.idc.2015.10.002
124. Wüthrich M, Klein BS. Investigation of anti–WI‐1 adhesin antibody‐Mediated protection in experimental pulmonary blastomycosis. J Infect Dis. (2000) 181:1720–8. doi: 10.1086/315473
125. Morris A, Norris KA. Colonization by pneumocystis jirovecii and its role in disease. Clin Microbiol Rev. (2012) 25:297–317. doi: 10.1128/CMR.00013-12
126. Miceli A, Krishnamurthy K. Blastomycosis. In: StatPearls. StatPearls Publishing, Treasure Island (FL (2024). Available at: http://www.ncbi.nlm.nih.gov/books/NBK441987/.
127. Harmsen AG, Chen W, Gigliotti F. Active immunity to Pneumocystis carinii reinfection in T-cell-depleted mice. Infect Immun. (1995) 63:2391–5. doi: 10.1128/iai.63.7.2391-2395.1995
128. Pascale JM, Shaw MM, Durant PJ, Amador AA, Bartlett MS, Smith JW, et al. Intranasal Immunization Confers Protection against Murine Pneumocystis carinii Lung Infection. Infect Immun. (1999) 67:805–9. doi: 10.1128/IAI.67.2.805-809.1999
129. Garvy BA, Wiley JA, Gigliotti F, Harmsen AG. Protection against Pneumocystis carinii pneumonia by antibodies generated from either T helper 1 or T helper 2 responses. Infect Immun. (1997) 65:5052–6. doi: 10.1128/iai.65.12.5052-5056.1997
130. Gigliotti F, Wiley JA, Harmsen AG. Immunization with Pneumocystis carinii gpA Is Immunogenic but Not Protective in a Mouse Model of P. carinii Pneumonia. Infect Immun. (1998) 66:3179–82. doi: 10.1128/IAI.66.7.3179-3182.1998
131. Wells J, Gigliotti F, Simpson-Haidaris PJ, Haidaris CG. Epitope Mapping of a Protective Monoclonal Antibody against Pneumocystis carinii with Shared Reactivity to Streptococcus pneumoniae Surface Antigen PspA. Infect Immun. (2004) 72:1548–56. doi: 10.1128/IAI.72.3.1548-1556.2004
132. Gingerich AD, Norris KA, Mousa JJ. Pneumocystis pneumonia: immunity, vaccines, and treatments. Pathogens. (2021) 10:236. doi: 10.3390/pathogens10020236
133. Smulian AG, Sullivan DW. Theus SA. Immunization with recombinant p55 antigen provides partial protection against infection: characterization of epitope recognition associated with immunization. Microbes Infection. (2000) 2:127–36. doi: 10.1016/S1286-4579(00)00275-6
134. Theus SA, Sullivan DW, Walzer PD, Smulian AG. Cellular responses to a 55-kilodalton recombinant Pneumocystis carinii antigen. Infect Immun. (1994) 62:3479–84. doi: 10.1128/iai.62.8.3479-3484.1994
135. Chakrabarti A, Bonifaz A, Gutierrez-Galhardo MC, Mochizuki T, Li S. Global epidemiology of sporotrichosis. Med Mycology. (2015) 53:3–14. doi: 10.1093/mmy/myu062
136. Chakrabarti A, Slavin MA. Endemic fungal infections in the Asia-Pacific region. Med Mycol. (2011) 49:337–44. doi: 10.3109/13693786.2010.551426
137. Barros MBDL, De Almeida Paes R, Schubach AO. Sporothrix schenckii and sporotrichosis. Clin Microbiol Rev. (2011) 24:633–54. doi: 10.1128/CMR.00007-11
138. Nascimento RC, Almeida SR. Humoral immune response against soluble and fractionate antigens in experimental sporotrichosis. FEMS Immunol Med Microbiol. (2005) 43:241–7. doi: 10.1016/j.femsim.2004.08.004
139. De Almeida SR. Advances in vaccine development against sporotrichosis. Curr Trop Med Rep. (2019) 6:126–31. doi: 10.1007/s40475-019-00183-0
140. Portuondo DL, Batista-Duharte A, Ferreira LS, Martínez DT, Polesi MC, Duarte RA, et al. A cell wall protein-based vaccine candidate induce protective immune response against Sporothrix schenckii infection. Immunobiology. (2016) 221:300–9. doi: 10.1016/j.imbio.2015.10.005
141. Chen F, Jiang R, Wang Y, Zhu M, Zhang X, Dong S, et al. Recombinant Phage Elicits Protective Immune Response against Systemic S. globosa Infection in Mouse Model. Sci Rep. (2017) 7:42024. doi: 10.1038/srep42024
142. de Almeida JRF, Jannuzzi GP, Kaihami GH, Breda LCD, Ferreira KS, de Almeida SR. An immunoproteomic approach revealing peptides from Sporothrix brasiliensis that induce a cellular immune response in subcutaneous sporotrichosis. Sci Rep. (2018) 8:4192. doi: 10.1038/s41598-018-22709-8
143. Vargas G, Honorato L, Guimarães AJ, Rodrigues ML, Reis FCG, Vale AM, et al. Protective effect of fungal extracellular vesicles against murine candidiasis. Cell Microbiol. (2020) 22:e13238. doi: 10.1111/cmi.13238
144. Martin-Cruz L, Sevilla-Ortega C, Benito-Villalvilla C, Diez‐Rivero CM, Sanchez-Ramón S, Subiza JL, et al. A combination of polybacterial MV140 and candida albicans V132 as a potential novel trained immunity-based vaccine for genitourinary tract infections. Front Immunol. (2021) 11:612269. doi: 10.3389/fimmu.2020.612269
145. Lv QZ, Li DD, Han H, Yang YH, Duan JL, Ma HH, et al. Priming with FLO8-deficient Candida albicans induces Th1-biased protective immunity against lethal polymicrobial sepsis. Cell Mol Immunol. (2021) 18:2010–23. doi: 10.1038/s41423-020-00576-6
146. Lilly EA, Bender BE, Esher Righi S, Fidel PLJ, Noverr MC. Trained Innate Immunity Induced by Vaccination with Low-Virulence Candida Species Mediates Protection against Several Forms of Fungal Sepsis via Ly6G(+) Gr-1(+) Leukocytes. mBio. (2021) 12:e0254821. doi: 10.1128/mBio.02548-21
147. Singh S, Nabeela S, Barbarino A, Ibrahim AS, Uppuluri P. Antibodies targeting Candida albicans Als3 and Hyr1 antigens protect neonatal mice from candidiasis. Front Immunol. (2022) 13:925821. doi: 10.3389/fimmu.2022.925821
148. Jin Z, Dong YT, Liu S, Liu J, Qiu XR, Zhang Y, et al. Potential of polyethyleneimine as an adjuvant to prepare long-term and potent antifungal nanovaccine. Front Immunol. (2022) 13:843684. doi: 10.3389/fimmu.2022.843684
149. Shukla M, Rohatgi S. Vaccination with Secreted Aspartyl Proteinase 2 Protein from Candida parapsilosis Can Enhance Survival of Mice during C. tropicalis-Mediated Systemic Candidiasis. Infect Immun. (2020) 88(10):e00312-20. doi: 10.1128/IAI.00312-20
150. Edwards JEJ, Schwartz MM, Schmidt CS, Sobel JD, Nyirjesy P, Schodel F, et al. A fungal immunotherapeutic vaccine (NDV-3A) for treatment of recurrent vulvovaginal candidiasis-A phase 2 randomized, double-blind, placebo-controlled trial. Clin Infect Dis. (2018) 66:1928–36. doi: 10.1093/cid/ciy185
151. Leu SJ, Lee YC, Lee CH, Liao PY, Chiang CW, Yang CM, et al. Generation and Characterization of Single Chain Variable Fragment against Alpha-Enolase of Candida albicans. Int J Mol Sci. (2020) 21:2903. doi: 10.3390/ijms21082903
152. Fernandes CM, Normile TG, Fabri JHTM, Brauer VS, de S Araújo GR, Frases S, et al. Vaccination with live or heat-killed aspergillus fumigatus ΔsglA conidia fully protects immunocompromised mice from invasive aspergillosis. mBio. (2022) 13:e0232822. doi: 10.1128/mbio.02328-22
153. Rayens E, Rabacal W, Kang SE, Celia BN, Momany M, Norris KA. Vaccine-induced protection in two murine models of invasive pulmonary aspergillosis. Front Immunol. (2021) 12:670578. doi: 10.3389/fimmu.2021.670578
154. Slarve M, Holznecht N, Reza H, Gilkes A, Slarve I, Olson J, et al. Recombinant Aspergillus fumigatus antigens Asp f 3 and Asp f 9 in liposomal vaccine protect mice against invasive pulmonary aspergillosis. Vaccine. (2022) 40:4160–8. doi: 10.1016/j.vaccine.2022.05.057
155. Ueno K, Yanagihara N, Otani Y, Shimizu K, Kinjo Y, Miyazaki Y. Neutrophil-mediated antifungal activity against highly virulent Cryptococcus gattii strain R265. Med Mycol. (2019) 57:1046–54. doi: 10.1093/mmy/myy153
156. Hole CR, Wager CML, Castro-Lopez N, Campuzano A, Cai H, Wozniak KL, et al. Induction of memory-like dendritic cell responses in vivo. Nat Commun. (2019) 10:2955. doi: 10.1038/s41467-019-10486-5
157. Leopold Wager CM, Hole CR, Campuzano A, Castro-Lopez N, Cai H, Caballero Van Dyke MC, et al. IFN-γ immune priming of macrophages in vivo induces prolonged STAT1 binding and protection against Cryptococcus neoformans. PloS Pathog. (2018) 14:e1007358.
158. Lin J, Pham T, Hipsher K, Glueck N, Fan Y, Lin X. Immunoprotection against cryptococcosis offered by znf2 depends on capsule and the hyphal morphology. mBio. (2022) 13:e0278521. doi: 10.1128/mbio.02785-21
159. Upadhya R, Lam WC, Hole CR, Parchment D, Lee CK, Specht CA, et al. Cryptococcus neoformans Cda1 and Cda2 coordinate deacetylation of chitin during infection to control fungal virulence. Cell Surf. (2021) 7:100066. doi: 10.1016/j.tcsw.2021.100066
160. Normile TG, Chu TH, Sheridan BS, Del Poeta M. Vaccine protection by Cryptococcus neoformans Δsgl1 is mediated by γδ T cells via TLR2 signaling. Mucosal Immunol. (2022) 15:1416–30. doi: 10.1038/s41385-022-00570-3
161. Pereira de Sa N, Taouil A, Kim J, Clement T, Hoffmann RM, Burke JE, et al. Structure and inhibition of Cryptococcus neoformans sterylglucosidase to develop antifungal agents. Nat Commun. (2021) 12:5885. doi: 10.1038/s41467-021-26163-5
162. Wang Y, Wang K, Rivera A, Xue C. Development of a heat-killed fbp1 mutant strain as a therapeutic agent to treat invasive cryptococcus infection. Microbiol Spectr. (2023) 11:e0495522. doi: 10.1128/spectrum.04955-22
163. Specht CA, Homan EJ, Lee CK, Mou Z, Gomez CL, Hester MM, et al. Protection of mice against experimental cryptococcosis by synthesized peptides delivered in glucan particles. mBio. (2021) 13:e0336721. doi: 10.1101/2021.11.12.468465
164. Hester MM, Lee CK, Abraham A, Khoshkenar P, Ostroff GR, Levitz SM, et al. Protection of mice against experimental cryptococcosis using glucan particle-based vaccines containing novel recombinant antigens. Vaccine. (2020) 38:620–6. doi: 10.1016/j.vaccine.2019.10.051
165. Deepe GS, Buesing WR, Ostroff GR, Abraham A, Specht CA, Huang H, et al. Vaccination with an alkaline extract of Histoplasma capsulatum packaged in glucan particles confers protective immunity in mice. Vaccine. (2018) 36:3359–67. doi: 10.1016/j.vaccine.2018.04.047
166. Shubitz LF, Robb EJ, Powell DA, Bowen RA, Bosco-Lauth A, Hartwig A, et al. Δcps1 vaccine protects dogs against experimentally induced coccidioidomycosis. Vaccine. (2021) 39:6894–901. doi: 10.1016/j.vaccine.2021.10.029
167. Hung CY, Zhang H, Castro-Lopez N, Ostroff GR, Khoshlenar P, Abraham A, et al. Glucan-Chitin Particles Enhance Th17 Response and Improve Protective Efficacy of a Multivalent Antigen (rCpa1) against Pulmonary Coccidioides posadasii Infection. Infect Immun. (2018) 86(11):e00070-18. doi: 10.1128/IAI.00070-18
168. Araújo MVde, Santos Júnior SRD, Nosanchuk JD, Taborda CP. Therapeutic vaccination with cationic liposomes formulated with dioctadecyldimethylammonium and trehalose dibehenate (CAF01) and peptide P10 is protective in mice infected with paracoccidioides brasiliensis. J Fungi (Basel). (2020) 6(4):347. doi: 10.3390/jof6040347
169. Rodrigues Dos Santos Junior S, Kelley Lopes da Silva F, Santos Dias L, Oliveira Souza AC, Valdemir de Araujo M, Buffoni Roque da Silva L, et al. Intranasal vaccine using P10 peptide complexed within chitosan polymeric nanoparticles as experimental therapy for paracoccidioidomycosis in murine model. J Fungi (Basel). (2020) 6(3):160. doi: 10.3390/jof6030160
170. Wüthrich M, Dobson HE, Ledesma Taira C, Okaa UJ, Dos Santos Dias L, Isidoro-Ayza M, et al. Combination adjuvants enhance recombinant protein vaccine protection against fungal infection. mBio. (2021) 12:e0201821. doi: 10.1128/mBio.02018-21
171. Tong T, Wang Z, Xu Y, Shen J. Immunization with Pneumocystis carinii A121–85 antigen activates immune function against P. carinii. BMC Immunol. (2021) 22:40. doi: 10.1186/s12865-021-00436-6
172. Cobos Jiménez V, Rabacal W, Rayens E, Norris KA. Immunization with Pneumocystis recombinant KEX1 induces robust and durable humoral responses in immunocompromised non-human primates. Hum Vaccin Immunother. (2019) 15:2075–80. doi: 10.1080/21645515.2019.1631135
173. Rabacal W, Schweitzer F, Kling HM, Buzzelli L, Rayens E, Norris KA. A therapeutic vaccine strategy to prevent Pneumocystis pneumonia in an immunocompromised host in a non-human primate model of HIV and Pneumocystis co-infection. Front Immunol. (2022) 13:1036658. doi: 10.3389/fimmu.2022.1036658
174. Téllez-Martínez D, Batista-Duharte A, Portuondo DL, Carlos IZ. Prophylactic and therapeutic vaccines against sporotrichosis. Feasibility and prospects. Microbes Infection. (2019) 21:432–40. doi: 10.1016/j.micinf.2019.05.003
175. Campuzano A, Zhang H, Ostroff GR, dos Santos Dias L, Wüthrich M, Klein BS, et al. CARD9-Associated Dectin-1 and Dectin-2 Are Required for Protective Immunity of a Multivalent Vaccine against Coccidioides posadasii Infection. J Immunol. (2020) 204:3296–306. doi: 10.4049/jimmunol.1900793
176. Loh JT, Lam KP. Fungal infections: Immune defense, immunotherapies and vaccines. Adv Drug Delivery Rev. (2023) 196:114775. doi: 10.1016/j.addr.2023.114775
177. Lionakis MS, Drummond RA, Hohl TM. Immune responses to human fungal pathogens and therapeutic prospects. Nat Rev Immunol. (2023) 23:433–52. doi: 10.1038/s41577-022-00826-w
Keywords: invasive fungal infections, vaccine development, nanotechnology, bioinformatics, Candida vaccine, cryptococcal vaccine, pan fungal vaccine
Citation: Alapan D, Bisweswar O, Prasenjit S, Prasanjit D and Arkapal B (2024) Recent advances in the clinical development of antifungal vaccines: a narrative review. Front. Trop. Dis 5:1446477. doi: 10.3389/fitd.2024.1446477
Received: 09 June 2024; Accepted: 12 July 2024;
Published: 08 August 2024.
Edited by:
Elantamilan D., All India Institute of Medical Sciences, IndiaReviewed by:
Srinivasa Reddy Bonam, University of Texas Medical Branch at Galveston, United StatesJoão Alves De Araújo Filho, Universidade Federal de Goiás, Brazil
Copyright © 2024 Alapan, Bisweswar, Prasenjit, Prasanjit and Arkapal. This is an open-access article distributed under the terms of the Creative Commons Attribution License (CC BY). The use, distribution or reproduction in other forums is permitted, provided the original author(s) and the copyright owner(s) are credited and that the original publication in this journal is cited, in accordance with accepted academic practice. No use, distribution or reproduction is permitted which does not comply with these terms.
*Correspondence: Bandyopadhyay Arkapal, YXJrYXBhbC5waGFybWFAYWlpbXNrYWx5YW5pLmVkdS5pbg==
†These authors have contributed equally to this work
‡ORCID: Das Alapan, orcid.org/0000-0002-7727-3977
Ojha Bisweswar, orcid.org/0000-0003-3021-239X
Sarkar Prasenjit, orcid.org/0000-0001-9672-4772
Das Prasenjit, orcid.org/0009-0006-8807-0629
Bandyopadhyay Arkapal, orcid.org/0001-9755-0233