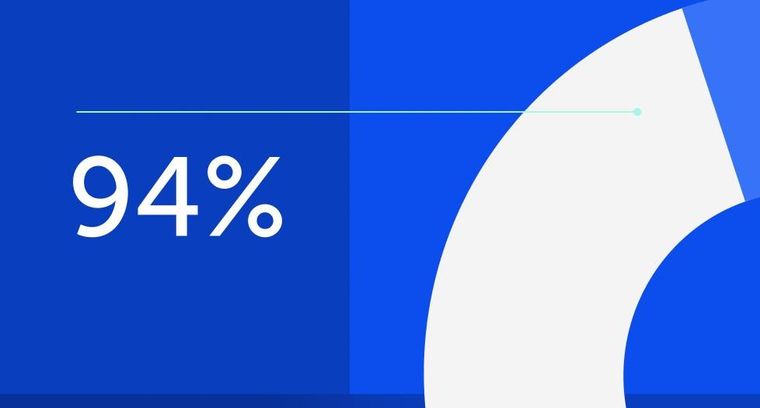
94% of researchers rate our articles as excellent or good
Learn more about the work of our research integrity team to safeguard the quality of each article we publish.
Find out more
REVIEW article
Front. Trop. Dis., 01 May 2024
Sec. Vector Biology
Volume 5 - 2024 | https://doi.org/10.3389/fitd.2024.1308585
This article is part of the Research TopicLatest Advances in the Biological Control of Vectors of Human Tropical DiseasesView all 6 articles
Infections caused by vector-borne pathogens impose a significant burden of morbidity and mortality in a global scale. In their quest for blood, hematophagous arthropods penetrate the host skin and may transmit pathogens by the bite. These pathogens are deposited along with saliva and a complex mixture of vector derived factors. Hematophagous arthopod vectors have evolved a complex array of adaptations to modulate the host immune response at the bite site with the primary goal to improve blood feeding, which have been exploited throughout evolution by these pathogens to enhance infection establishment in the host. While this paradigm has been firmly established in mouse models, comparable data from human studies are scarce. Here we review how the host skin immune response to vector bites in animal models is hijacked by microbes to promote their pathogenesis. We mainly explored four distinct vector-pathogen pairs of global health importance: sand flies and Leishmania parasites, Ixodes scapularis ticks and Borrelia burgdorferi, Aedes aegypti mosquitoes and arboviruses, and Anopheles gambiae mosquitos and Plasmodium parasites. Finally, we outline how critical it is for the field of vector biology to shift from rodent models to clinical studies focused on the interface of vector-pathogen-host immune system to push further the frontiers of knowledge of the field.
Infectious pathogens transmitted by arthropod vectors cause over 700,000 deaths every year worldwide, with 80% of the world’s population at risk of contracting a vector-borne disease, making prevention of vector-transmitted infections an important global health priority (1). While taking a blood meal, arthropods may deposit in host skin an infectious inoculum comprised of both pathogen and vector derived factors. Differences in the methods of blood feeding by vector arthropods are widely known. Briefly, some vectors, such as mosquitoes for example, are solenophages, which means feeding by using their mandibles to pierce and probe small blood vessels, then bloodmeal is obtained directly from the blood vessels. Others, such as ticks and sandflies, are telmophages, and use their mouthparts to cut and tear skin pieces and blood vessels, and as a result suck blood from the pool of blood that leaks around the bite site (2, 3). Components present in the infectious inoculum include, inter alia, salivary proteins, microbiota, nucleosides, microRNAs, and exosomes (4–6). The combination of tissue trauma and vector derived factors also alters the local immune response in the skin in such a manner that facilitates establishment and dissemination of infection, thereby exacerbating disease. Vector bite-mediated enhancement of infection is a remarkably well-conserved paradigm across a diverse range of vector and pathogen species in animal models, yet there is comparatively little data from human studies. Here, we review cutaneous immunity to vector bites in four vector-pathogen pairs, focusing on how each pathogen exploits the skin immune response to vector bites and vector derived factors in rodent models. We conclude each section by comparing and contrasting results from these preclinical models to human studies, highlighting the paucity of clinical data on this topic.Importantly, there is a rich evidence based on in vitro and biochemical studies which detail the immunomodulatory mechanisms by which vector saliva and other vector derived components exert their effects, as well as studies in animal models of how vector saliva or salivary proteins affect other host physiological aspects not related to the immune response. These topics have been well-covered in several recent review articles elsewhere (7–10).
The scope of our review focused on two major types of primary research studies: (1) in vivo preclinical animal models to investigate the effects of vector bite, vector derived factors including arthropod saliva, microbiome, and exosomes on both the skin immune response and pathogen establishment, dissemination, or disease severity, and (2) clinical studies of human skin immune responses to either controlled challenge with uninfected vector bites or to naturally acquired vector bites. Whenever applicable we contrasted preclinical data with clinical studies. We focused the search mainly on - but not limited to - four different vector-pathogen pairs: (1) sand flies and Leishmania, (2) ticks and Borrelia, (3) Aedes and arboviruses, and (4) Anopheles and Plasmodium. PubMed and Google Scholar were used as the primary databases for the literature search. The initial Boolean search was: (skin OR cutaneous) AND (immune OR immunity) AND (saliva OR salivary) AND (“sand fly” OR “sand flies” OR Ixodes OR Aedes OR Anopheles). Preprints were excluded. Primary research articles retrieved in the initial search were manually reviewed and filtered according to the scope described above. The “Cited by” function in Google Scholar and “Citations” function in the Web of Science (Clarivate) database were used in a secondary search of articles that referenced results from the primary search, in order to look for additional articles meeting the scope criteria for the review. Illustration content of drafts and initial sketches of figures were created by the authors based on literature search on skin anatomy, skin immunological environment and vector biology. All illustrations were executed in a flat graphical style with superficial elements like shadows, effects, reflections, and other extraneous details kept to a minimum to simplify the image and increase comprehension of the material. Adobe illustrator was used for layout, labeling and drawing of digital assets.
The skin is the largest organ of the body, making up 12-15% of the body’s total weight, and representing a physical, chemical and immunological barrier that protects the interior body from external insults, while also interacting with the environment. A comprehensive description of skin architecture has been reviewed elsewhere (11, 12). Briefly, mammalian skin consists of two distinct compartments: the epidermis and the dermis. The epidermis comprises four dense cell layers: the stratum corneum (SC), stratum granulosum (SG), stratum spinosum (SS), and stratum basale (SB). These may vary in thickness, depending on the body part, but consist of 5-10 cell layers in humans and only 2-3 in mice. Moreover, while mouse skin is densely comprised of hair follicles, human skin possesses large interfollicular areas and sweat glands, which are almost entirely absent in mice (13, 14). Underneath the epidermis is the dermis, where cell density is much sparser and filled with extracellular matrix proteins (laminins, collagens, proteoglycans, fibrillins, matricellular proteins, Latent TGF-β binding proteins and elastin) that give the skin its physical structure (15).
Comparative allometric scaling of rodents and humans has shown that they display a similar ratio of skin thickness relative to body mass, though skin thickness of humans in the abdomen reaches 2401 µm, while mice display 10 times less thickness in the skin flank (16). Moreover, mechanical properties also differ between these species, for example, viscoelasticity, which is directly related to tissue relaxation and deformation, is significantly higher in larger animals due to their greater epidermal depth. Consequently, tissue viscoelasticity properties affect the penetration force required by vectors to successfully insert their mouthparts into human skin versus mice. In fact, the structure of the mosquito proboscis counteracts host skin deformation and displacement through its vibratory motion, harpoon-shape and notches in the maxillae to anchor mouthparts in the host skin and facilitate insertion of the proboscis (17, 18). While mouse epidermis is comparatively thin (~25 µm), human epidermis on average reaches 100 µm in depth. Nevertheless, vectors possess enough length on their mouthparts (sand flies: 230-360 µm; mosquitos: 1900-2450 µm; ticks: ~500 µm) to penetrate or lacerate through the reticular layer of the dermis in both humans and mice, directly exposing the dermal immune system to vector derived factors and tissue damage (14).
Many skin resident cells are sentinels of the immune system that act as critical first line responders to breaches in the skin or infection. In the epidermis, keratinocytes are the major cell type and express receptors that recognize pathogens and danger signals, including surface and endosomal Toll-like receptors, nucleotide-binding domain leucine-rich repeat-containing (NLR) family proteins, and mannose-binding receptors (MRs) (19, 20). The combination of antimicrobial peptides (AMPs) secreted by keratinocytes, hair follicles, and the fluids produced by sebaceous and sweat glands fine-tune unique skin microbial niches within distinct sites in the body, which ultimately mediate distinctions between the microbiome of humans and mice (21). The skin microbiome composition plays an important role in driving the attractiveness of mosquitos to odors related to the generation of volatile organic compounds emitted by the skin’s commensal bacteria. The manipulation of the composition of the skin microbiota through application of topical probiotics has emerged as a novel technique for vector control (22). Langerhans cells (LCs) form a network distributed along the basal and suprabasal layers of the epidermis and hair follicles. Though morphologically similar to dendritic cells (DCs), their developmental origin indicates LCs are a specialized subset of epidermis-resident macrophages that exhibit a mixture of functions similar to both DCs and macrophages (23, 24). Under homeostasis, LCs are immature antigen presenting cells (APCs) that gain migratory capacity upon a maturation process mediated by recognition of infection or inflammation; LCs also provide signals for homing of intraepithelial T cells (23, 25). Mice possess another cell subtype, absent in humans, dendritic epidermal T cells (DETCs) expressing a limited set of γδ T cell receptors, which respond to self-antigens expressed by damaged, stressed, or transformed keratinocytes. This cells comprise the majority of T cells in the epidermis of mice at steady state (14, 26).
Under steady state conditions, fibroblasts are the dominant cell type in the dermis and produce the collagen network and other components that constitute the extracellular matrix. Dendritic cells, macrophages, mast cells and lymphocytes are the primary dermal professional immune cells heterogeneously spread throughout the dermis (27). In addition to professional immune cells, sensory neurons, blood and lymphatic vessels are also found in the dermis (28). Similar to DCs, dermal macrophages have a diverse range of subsets residing in the skin that remain sessile under homeostasis and are thought to play a role in tissue homeostasis and in the activation of effector or resident T cells, despite being poor inducers of naïve T cells. Nevertheless, DCs are intrinsically better antigen presenting cells with migratory capacity and high expression o costimulatory receptors required to activate naïve T cells (20, 29). M2 macrophages associated with wound healing and resolving inflammation are thought to be predominant in the skin at steady state (30–32). Mast cells, scattered throughout the dermis, possess between 50 to 200 different granules stored in their cytoplasm and harbor inflammatory mediators such as histamine, heparin and multiple cytokines (33). Cross linking of multiple FcϵRI receptors leads to mast cell degranulation of pre-stored inflammatory mediators, but also to active secretion of inflammatory cytokines such as IL-13, TNF-α and IL-6 through calcium mobilization and nuclear translocation of NF-kB (33). Upon infection or tissue injury, neutrophils are the first leukocytes to be recruited to the skin, and to amplify inflammatory reactions initiated by resident myeloid cells followed by infiltration of inflammatory monocytes. These inflammatory monocytes can differentiate into either dendritic cells or inflammatory macrophages depending on inflammatory cues they receive in the periphery. Although acute inflammatory reactions follow a similar pattern in the skin of both mice and humans, mice have a pronounced reduction in the proportion of neutrophils in the bloodstream (10-25%) compared to humans (50-70%) and lack expression of neutrophil defensins (14, 20).
Human skin contains more than 20 million resident T cells with a remarkably diverse TCR repertoire, which in total represents over 2.8 fold more than the absolute number of T cells found in the bloodstream (34). Interestingly, most T cells found in the skin immune compartment are T helper 1 (Th1) effector memory cells. The proportion of lymphocytes in the murine bloodstream is much larger than in humans, comprising 75-90% of circulating leukocytes, while in humans this number is limited to 30-50% (14). Upon priming by an antigen presenting cell, T cells undergo clonal expansion, and based on the cytokine profile present in that microenvironment, CD4+ T cells will acquire distinct helper phenotypes, classically identified as Th1, Th2, Th17 and regulatory T cells (Tregs). However, the phenotype of differentiated T helper cells can be pliable, as demonstrated by recent findings that skin injury due to sand fly challenge releases alarmins that increase production of type 2 cytokines and co-expression of both GATA-3 and ROR-γt on S. epidermidis-specific IL-17A+ CD8 T cells (35).
Leishmania sp. are protozoan parasites transmitted by the bite of phlebotomine sand flies. The resultant disease, leishmaniasis, manifests in three main clinical forms: cutaneous (CL), mucocutaneous (MCL), and visceral leishmaniasis (VL). In their infectious metacyclic form, Leishmania parasites are regurgitated by the sand fly into the host skin according to the “blocked fly hypothesis”, but alternatively parasites may also be passively deposited in the skin after repeated probing (36, 37). These parasites are quickly internalized, primarily by neutrophils which are the first wave of infiltrating cells to respond to parasite inoculation (38). Though parasite expansion in vivo does not require neutrophils, these cells have been reported to shield parasites and to maintain intracellular promastigotes viable and infective (39–41). A fraction of infected neutrophils act as “Trojan horses” and can directly transfer parasite cargo to the mononuclear phagocytic system (41). Moreover, efferocytosis of L. major-infected apoptotic neutrophils impairs dendritic cell maturation (39). Similarly, Leishmania amazonensis-infected macrophages in the presence of resting apoptotic neutrophils upregulate production of TGF-β and PGE-2, favoring parasite replication (42). Multiple species, including both promastigote and amastigote stages, promote the release of neutrophil extracellular traps (NETs), web-like structures composed of chromatin decorated with microbicidal proteins that are extruded to the extracellular space by activated neutrophils. NETs can attach to the negatively charged surface of most microbes, likely due to electrostatic interactions with cationic NET components (43). Still, most Leishmania species possess mechanisms to evade the microbicidal effect of NETs (44–46). NETs contain several immunomodulatory molecules, including proteins and microRNAs, that are tethered to the DNA backbone and regulate the responses of neighboring immune cells (47). The biological significance of NETs in vivo have been explored in the context of autoimmunity and inflammatory disordes (48), but the biological significance of these phenomena in leishmaniasis awaits further investigation. Of note, plasticity in the transcriptional program of neutrophils shaped by molecular cues in the microenvironment directly impacts the effector response profile of these cells and their maturation status, which may potentially affect disease outcome, given the prominent role neutrophils play in susceptibility to Leishmania infection. A more detailed review on neutrophil functional plasticity in the context of leishmaniasis can be found here (49).
Interestingly, a strain of Leishmania major (Seidman), isolated from a patient with non-healing cutaneous lesions, preferentially infects dermal resident macrophages and neutrophils during the early stages of the inflammatory reaction (41, 50). This subpopulation of resident dermal macrophages, characterized by high expression of mannose receptor (MRhigh dermal macrophages), is self-sufficient and does not require replenishment by monocytes, unlike most skin resident macrophages. Furthermore, MRhigh dermal macrophages display an M2-like phenotype, which is mediated by cooperation with IL-4/13 secreting eosinophils, despite strong type I immunity with high levels of IFN-γ induced by L. major Seidman infection (50, 51). Transfer of L. major parasites to a permissive subpopulation of Ly6C+CCR2+ inflammatory monocytes contributes to parasite expansion and downmodulates monocyte maturation to evade immune activation and intracellular parasite killing (40). Interestingly, the recruitment of this permissive population of monocytes seems to be driven by an early IFN-γ production at the site of infection, which challenges the classical notion that IFN-γ exclusively benefits the host by boosting macrophage killing of intracellular Leishmania parasites (52).
As hosts and parasites co-evolve, parasites acquire virulence factors and other adaptations to counterbalance advantageous adaptations acquired by the host and vice-versa. Many of these adaptations target the host immune system. The Leishmania lipophosphoglycan (LPG) coat protects parasites from complement-mediated lysis and at the same time mediates opsonization, favoring establishment of infection. Moreover, LPG prevents or delays phagosome fusion with late endocytic organelles and lysosomes to allow parasites to differentiate into amastigotes and replicate inside the parasitophorus vacuole (53). The main intracellular antimicrobial pathways used by macrophages to promote killing of Leishmania are the oxidative burst and lysosomal enzymatic activation. In turn, Leishmania parasites have evolved to thwart this mechanism with LPG and the surface metalloprotease GP63, which impairs recruitment of NADPH oxidase to the phagosome. Inflammasome activation also contributes to macrophage resistance to infection by promoting protective inflammatory cell death, interrupting parasite replication and enhancing the inflammatory reaction. Although these evasion mechanisms are not as well characterized in the context of inflammasome activation, certain species (e.g. L. major and L. mexicana) prevent IL-1β production through GP63, while L. amazonensis and L. donovani have been shown to affect expression of inflammasome components in macrophages (54). Furthermore, amastigotes actively expose phosphatidylserine on their surface. This evasion mechanism is termed “apoptotic mimicry,” as recognition of this surface moiety by macrophages leads to production of TGF-β and IL-10, thereby dampening macrophage-mediated microbicidal responses (55).
Following the pioneering discovery of T helper 1 and T helper 2 subpopulations (56), the first evidence that gave biological significance for these cell subsets came in the context of Leishmania major infection (57), which demonstrated that a balance between these two differentiation patterns had divergent effects on disease outcome in the mouse model. In contrast to the mouse model, the severity of human leishmaniasis does not correlate clearly with Th1/Th2 polarization. If we take American CL as an example where mixed Th1/Th2 responses are usually observed, the magnitude of T cell activation shows stronger correlation with disease severity than the type of T helper polarization. Similarly, poor activation of T cells may also impact disease pathology. In this context, while MCL can be placed at one extreme with strong T cell activation, diffuse cutaneous leishmaniasis is positioned on the opposite side with the weakest T cell responses, and localized CL is placed in the center (58). Even nowadays for mouse models, the once assumed “Th1-Th2 paradigm” does not fully describe the dynamics of adaptive immunity to leishmaniasis with the discovery of additional T helper subpopulations (53). Th17 cells, for example, have been described in chronic lesions of patients, while the roles of these cells are still uncertain and seems to be Leishmania species-specific (59). Moreover, regulatory T cells have been associated with latency and disease relapse (53).
Cytotoxic CD8 T cells play a prominent role in the control of viral and intracellular pathogens. The hallmark of this T cell subset is the production of IFN-γ, TNF-α and the killing of target cells mediated by the exocytosis of lytic granules containing perforin, granzymes A/B and granulysin (60). Novais et al. (61) demonstrated that CD8 T cells promote pathology in mice infected with L. braziliensis through a mechanism dependent on perforin and IL-1β secretion largely attributed to enhanced neutrophilic infiltration. Similarly in humans, CD8 T cells correlate with CL immunopathology and promote inflammasome activation in infected macrophage in vitro through a mechanism dependent on perforin and enhanced potassium efflux (62, 63). IL-10-producing CD8 T cells have also been implicated in human immunopathology caused by L. guyanensis infection and in patients suffering from post-kala-azar dermal leishmaniasis (PKDL). However, the biological significance of regulatory CD8 T cells in the immune response to Leishmania sp. remains unknown (60).
In 1988, sand fly saliva was shown to enhance Leishmania virulence and infection establishment (64). Mice inoculated with Leishmania parasites and sand fly salivary gland homogenate (SGH) developed skin lesions five to ten times larger and containing 5000-fold more parasites than mice injected with parasites alone (64, 65). It was later shown that co-inoculation of SGH and parasites in the skin of rodents resulted in robust upregulation of the Th2 cytokines IL-4 and IL-5 in the epidermis, compared to L. major alone (66). Similarly, addition of SGH reshaped dermal cells to produce lower levels of Th1 cytokines, such as IFN-γ and IL-12, and higher levels of Th2 cytokines in response to L. major infection (67). Early upregulation of IL-4 by keratinocytes in the epidermis within the first hours following infection is essential to promote Th1 differentiation (68). Moreover, saliva from the New World sandfly Lutzomyia longipalpis drives Th17 polarization, which promotes neutrophil infiltration at the inoculation site (69). In the skin air pouch model, SGH from Lu. longipalpis and Lu. intermedia promoted the influx of neutrophils and macrophages, with the latter depending on CCL2/MCP-1 (70). Sand fly proteins of the yellow related salivary protein family act directly as neutrophil chemoattractants in vivo – rPduM10 and rPduM35 from Ph. dubosqi, and rLJM17 and rLJM11 from Lu. longipalpis (71). While these proteins act through G protein coupled receptors and depend on calcium signaling, their structure does not resemble any known chemokines. Importantly, these yellow proteins increased both Leishmania major parasite load in the skin and pathology, while antibody blockade of these proteins ameliorates these effects.
Further studies demonstrated that some recombinant sand fly salivary proteins enhance Leishmania infection in mouse models. The vasodilator, maxadilan, was the first salivary polypeptide shown to increase both skin lesion size and parasite burden when co-inoculated with L. major (72). In human monocytes treated with L. major, maxadilan increased IL-6 while inhibiting TNF-α secretion (73). Lundep, a sand fly salivary protein with endonuclease activity, also enhanced L. major infection (74). Lundep cleaves neutrophil extracellular traps (NETs), thereby facilitating survival of Leishmania during the early steps of the inflammatory reaction. Co-inoculation of Lundep with L. major increased skin lesion size and local parasite load, and this enhancement was abrogated when the endonuclease active site of Lundep was mutated. Nevertheless, considering the prolonged influx of neutrophils, and the unknown amount of Lundep present in the infective inoculum, the effect of Lundep in vivo is likely transient if any, especially in the face of continuous NET production by infiltrating waves of neutrophils.
In the sand fly midgut, Leishmania parasites secrete proteophosphoglycans that create a gel-like structure that obstructs the anterior midgut and promotes the regurgitation of parasites during a blood meal (75). Parasite-secreted promastigote secretory gel (PSG) exacerbates CL lesions and increases parasite load (75, 76), and in L. infantum infection, PSG increases parasite visceralization to the spleen (77). Moreover, PSG in combination with parasite-secreted chitinases promotes stomadeal valve dysfunction in the sand fly midgut, leading to persistent vector feeding attempts and facilitating transmission to multiple hosts (78). PSG is notable for its effects on macrophages, including recruitment of macrophages to the bite site, and enhancing the intracellular growth of L. mexicana in macrophages in an arginase-dependent manner (79). PSG induces dermal expression of insulin growth factor-1 (IGF-1) and its receptor, which are critical regulators of wound healing and alternative macrophage activation, thereby promoting Leishmania parasite survival (80).
Exosomes are extracellular vesicles of endossomal origin with a size range of 30-150nm secreted by virtually all eukaryotic cells with a prominent role in intercellular communication. Leishmania-secreted exosomes can be identified by Western blot and electron microscopy of the inoculum egested by Leishmania-infected sand flies after artificial membrane feeding (81). When co-inoculated, exosomes exacerbated CL lesions, increased parasite loads at bite sites (82), and increased draining lymph node expression of IL-2, IL-4, IFN-γ, IL-17a, IL-23, and IL-10 mRNAs (81). One potential mechanism of action of parasite secreted exosomes includes carriage of virulence factors. Indeed, expression of GP63 in purified exosomes from L. amazonensis is attributed to enhanced pathology observed by co-inoculation of parasites and purified exosomes in the footpad, since this phenotype was not recapitulated by exosomes purified from promastigotes carrying an epissomal antisence inhibitior fragment targetting GP63 (83). Interestingly, Leishmania RNA virus 1 (LRV1) exploits the exosome secretion pathway to exit and infect other L. guyanensis parasites. LRV-infected species have been linked to enhanced parasite virulence and more severe clinical manifestations of Leishmaniasis. Accordingly, co-inoculation of parasites with LRV1-containing exosomes enhanced pathology on mice as well (84).
Two-photon intravital microscopy of mouse skin demonstrated that sand fly bites trigger an immediate burst of neutrophil infiltration into the bite site that was not observed after needle injection (38). This observation was expanded upon by another study that implicated the sand fly gut microbiota as the driver of bite-specific infiltration by neutrophils (4). Sand flies have a rich gut microbiota that is essential for Leishmania development to maturity (85–87). During an infected bite, sand flies egest their midgut microbiota into the skin, a critical event which triggers activation of the NLRP3 inflammasome and secretion of IL-1β by neutrophils. This creates a positive feedback loop that sustains recruitment of these cells to the bite site (4). Providing antibiotics to Leishmania-infected sand flies or blocking the effect of IL-1β in mice with the IL-1 receptor antagonist anakinra abrogated neutrophil infiltration to the skin and prevented visceralization of L. donovani to the spleen. These findings identify the vector gut microbiota as a key player in the initiation of acute inflammation that is essential for parasite survival and establishment of infection after transmission by bite. Tissue damage also plays an important role in neutrophil infiltration at bite sites (38, 41). The contribution of the host microbiome per se driving neutrophil chemotaxis once the skin has been breached and microbes have access to the tissue also needs to be addressed. Especially, since certain signals are more potent inducers of neutrophil chemotaxis than others – a property named hierarchical chemotaxis (88). Hence, further studies are neede to address the relative contribution of each component found in bite sites for neutrophil infiltration, including vector salivary proteins.
Sand fly bites and SGH activate cellular stress responses that are co-opted by Leishmania to facilitate intracellular survival. Lu. longipalpis bites or intradermal SGH injection induce expression of nuclear factor-erythroid-2-related factor 2 (Nrf2), a master regulator of cytoprotective responses to oxidative stress. Among the plethora of genes activated by Nrf2, one of its most well-studied downstream effectors, heme oxygenase-1 (HO-1), drives parasite survival by reducing pro-inflammatory cytokine production and suppressing oxidative killing of amastigotes (89). Strikingly, HO-1 production is a universal host skin response to the bite of bloodfeeding arthropods. Furthermore, following L. major natural transmission, we observed that transient HO-1 inhibition during the first few days following infection was sufficient to impair host tolerance during the chronic stage of the disease (90). Both resident and monocyte-derived macrophages at the sand fly bite site ingest red blood cells, which produce HO-1 and contribute to recycling of iron through a specialized subset of CD91+CD163+ skin macrophages (90). Considering that bloodfeeding arthropods have evolved sophisticated pharmacologic tools to manipulate the host immune response to favor feeding and are exploited by parasites to establish disease, why did evolutionary pressure imposed by arthropods “allowed” such high levels of HO-1 at the site of the bite? Indeed, saliva from species as divergent as Aedes aegypti and Lutzomyia longipalpis induced HO-1 production at the site of the bite (90). Hence, in this context, there are a few possible explanations, none of them mutually exclusive: (i) HO-1 induction may be innocuous and neither favor feeding, nor parasite establishment, (ii) a mechanism that benefits both the sand fly and parasite by quenching inflammation-induced coagulation, (iii) a mechanism that protects host cells from the heme-mediated oxidative burst but also protects sand fly midgut cells and Leishmania parasites, making the bloodmeal “easy to digest”, or (iv) a way for the host to better tolerate disease, preserving longer the host for repeated feeding and from the pathogen point of view as an effective reservoir. Hence, HO-1 may have multiple roles in host-vector-parasite dynamics, pointing to the importance of additional studies of HO-1 in the skin response to arthropod bites. These results highlight the potential of HO-1 as a target for host directed therapy to limit tissue damage driven by parasite infection. Finally, the immunological consequences of red blood cells release in the host skin dermis have been largely overlooked and warrant further investigation, especially since this is a conserved feature of the bite site of all bloodfeeding arthropods.
One the most groundbreaking observations in the field of vector biology demonstrated that pre-exposure of rodents to SGH or sand fly bites protects against a secondary Leishmania challenge due to the generation of antibodies against salivary proteins, which reduce parasite virulence and prevent an early type 2 cytokine storm produced by epidermal cells in response to SGH (66, 91). In mice, pre-exposure to uninfected Ph. duboscqi bites confers protection against L. major, which is associated with the induction of a delayed type hypersensitivity (DTH) response and increased IFN-γ expression by both NK and CD4+ T cells (92). These studies paved the way for several subsequent vaccination trials targeting specific sand fly salivary proteins. Indeed, immunization with sand fly salivary proteins – even in the absence of Leishmania antigens – affords partial protection against cutaneous and visceralizing Leishmania species in a variety of animal models including mice, hamsters (93), and non-human primates (94). Immunization with LJM11, a salivary protein from Lu. longipalpis, conferred protection against L. major-infected Lu. longipalpis sand fly bites in mice (95). This protective effect was preserved in B cell-deficient mice but was abrogated by depletion of CD4+ T cells. Immunization with a DNA plasmid coding for LJM19, another salivary protein from Lu. Longipalpis, was shown to afford protection from visceral leishmaniasis in hamsters challenged with L. infantum combined with SGH, and was associated with an increased ratio of IFN-γ/TGF-β mRNA in the spleen, prolonged survival, and low parasite loads (93). Collectively, immunization with distinct salivary proteins from sand flies elicits an immunological response consistent with that associated with protective immunity to Leishmania infection. Further studies are needed to address the prospect of using salivary proteins as components of a human leishmaniasis vaccine co-formulated with parasite-derived antigens. Alternatively, immunodominant salivary proteins have the potential to be a useful surveillance tool for the measurement of human-vector contact to monitor the impact of vector control interventions (96).
A handful of studies have investigated the skin immune response to experimental sand fly feeding on human volunteers, with a focus on delayed type hypersensitivity (DTH) responses. A DTH response is an inflammatory manifestation of cell-mediated immunity mediated by antigen-specific T cells. As memory T cells recognize cognate peptides expressed on APCs, these T cells will become activated and produce chemokines and cytokines that will enhance local infiltration of the skin by other leukocytes and promote vascular permeability, leading to edema. In people with documented previous exposure to Ph. papatasi, sand fly bites provoked an early DTH reaction at the bite site that started 6 to 8 hours after the bite and persisted up to 72 hrs (97). Vinhas et al. investigated the immune response to Lu. longipalpis bites in human volunteers who tested negative for both antibodies against SGH and Leishmania antigens prior to sand fly exposure. Two hours after sand fly bites exposure, subjects had red hemorrhagic marks at the bite site indicative of an immediate response; within 24 hours these spots turned to pink indurated papules indicative of a delayed responsed to the bite. 45-60 days post 3 sequential exposures to sand fly bites is sufficient to generate specific antibodies against salivary proteins and prime T cells. Strinkinly, a recall response can be elicited up to 1 year post-exposure (98).
To better define the mechanisms of human skin immunity to sand fly bites in an endemic population, Oliveira et al. characterized the DTH response of people living in a CL endemic area of Mali to experimental bites of colony-reared Ph. duboscqi sand flies, the primary vector species for L. major in the region (99). Skin biopsies of the bite site showed marked chronic inflammation with CD4+ and CD8+ T cells and macrophages, along with high IFN-γ production, consistent with a Th1-polarized DTH response. In contrast to cellular immunity, high antibody titers to LinB13, a salivary protein from Lu. intermedia, is associated to disease severity in disseminated leishmaniasis and chemotherapy failure in these patients. Thus, antibody titers against salivary proteins may also be applicable in the context of disease prognosis (100).
Overall, extensive progress has been made in understanding the mechanisms of the host response to sand fly bites in animal models. Despite progress in this field, there is a limited number of studies analyzing dermatological and immunological responses to sand fly bites in humans. Additional human studies are required to ascertain if mechanistic observations from mice studies can be translated to the clinic, which will provide much needed mechanistic insights on potential therapeutic interventions for leishmaniasis. Of note, important advances have been made to standardize a protocol to conduct clinical trials employing controlled human infection with sand fly-delivered L. major parasites as a measure of vaccine efficacy (101, 102). We have summarized the main pathways activated in the host skin during Leishmania transmission in Figure 1.
Figure 1 In addition to parasites, sand flies egest an infective inoculum containing a complex assortment of biologically active molecules that modulate host hemostatic and immunological systems with the primary intent to allow blood feeding. However, parasites have taken advantage of these scenario to enhance their capacity to establish infection. The sand fly proboscis will lacerate the skin and reach the dermis, where components such as salivary proteins, promastigote secretory gel (PSG), exosomes, and gut microbiota are inoculated in the dermis in a pool of blood. Parasites are quickly internalized by an influx of neutrophils, which preserve viable promastigotes inside of them. Promastigotes largely differentiate to amastigotes and replicate inside mononuclear phagocytes, primarily macrophages. Deposition of salivary components enhance recruitment of an inflammatory infiltrate consisting of macrophages, neutrophils, and inflammatory monocytes. The tissue stress induced by sand fly saliva will also promote Nrf2 nuclear translocation and HO-1 production. HO-1 suppresses the production of reactive oxygen species (ROS) and TNF-α, which may favor parasite replication inside macrophages. HO-1 will also enhance host tolerance to infection as it quenches leukocyte infiltration and production of CXCL1, CCL2 and IL-1β. Saliva can directly increase secretion of prostaglandin E2 (PGE2), which increases parasite burden. Boost in IL-17 production by vector saliva enhance neutrophil infiltration, while apoptotic neutrophils impair macrophages activation. Furthermore, elevation in the levels of PGE2 and IL-10 lead to auto-downregulation of MHC class II and CD86 on dendritic cells, which diminish antigen presentation capacity at bite sites. In addition, the gut microbiota deposited along with salivary molecules induces MMP9, mast cell degranulation and IL-1β production by neutrophils. IL-1β production by neutrophils create an autocrine loop that prolong neutrophil infiltration and allow L. donovani dissemination to the spleen. In turn, IL-1β and PSGs recruit a surplus of neutrophils to the bite site. All these interactions help to upregulate cytokines such as IL-3, IL-5, TNF-α, IFN-γ, MCP-1 in the epidermis and down regulate cytokines in the dermis such as IFN-γ and IL-12, which help to establish the infection pathogenesis. Finally, immunizations with salivary proteins from sand flies (Apyrase, LJM11, LJM19 and PpSP15) elicit an immunological response consistent with protective immunity to Leishmania infection.
Unlike most other blood-feeding arthropods, ticks are “pool-feeders” that sustain prolonged attachment/feeding to the host skin through unique strategies acquired throughout evolution to counteract host hemostatic and immunological responses (103). Upon recognition of damage caused by a tick bite in the dermis, the host will mount a response to maintain blood hemostasis, heal the wound and neutralize pathogens that may enter through breached skin (104). To circumvent host skin immunity, ticks rely mostly on the powerful and dynamic arsenal of hundreds of proteins found in their saliva (103) (Figure 2). Tick saliva composition changes every ~24 hours, alternating secretion of different homologous proteins that retain the same function, but whose differences in amino acid sequence allow evasion to antigen specific immunity (104–107). The biological impact of salivary proteins on host skin physiology is vast, preventing pain, itching, vasoconstriction, wound healing, platelet aggregation and blood coagulation, in addition to modulating both the adaptive and innate compartments of the immune system (104, 107). Recent findings demonstrate robust and sustained induction of heme oxygenase-1 at the site of Ornithodorus turicata (soft ticks) bites in mice (90). Indeed, a comprehensive proteomic analysis of differentially expressed proteins found in rabbit skin following bites of all life stages of H. longicornis shows a sustained upregulation of HMOX1 at 24-72 hours post-bite reaching up to a 100-fold increase (108). Consistent with other vectors, biological properties of tick saliva allow indirect promotion of pathogen transmission from the tick to its mammalian host (109). Various tick-borne pathogens, including viruses, eukaryotic parasites and bacteria are delivered to the host during feeding (110). The most prevalent bacterial tick-borne disease in humans is Lyme borreliosis (LB), caused mainly by Borrelia burgdorferi (111).
Figure 2 During attachment process, ticks bite will rapidly elicit neutrophils (CXCL2/3/4, IL-1β and IL-5) and monocyte (CCL2/4/5 and others) chemoattractants. Resident T-cells in the dermis and dendritic cells, keratinocytes and Langerhans cells in the epidermis are activated, while effector Th2 cells are also mobilized to the expanding feeding lesion. Included among the large array of salivary proteins are: SALP-20, that block complement and effectively disables immune challenge; lipocalins, which block histamine secreted by mast cells, which minimizes pain and/or itch receptors and helps the tick avoid recognition by the parasitized host; metalloproteases (MTP), including angiotensin-converting enzymes, cystatins and saliva kininase inhibit T cell activation and proliferation; SALP-25 and ISL 929/13373 that blocks neutrophils production of superoxide; SALP-15 and Sialostatin that block T-cell activation; Serpin, which block platelet secreting thrombin and prevents blood clotting. Later in the feeding cycle, tick histamine release factor (tHRF) is secreted, which induces release of histamine and dilation of the vasculature in the bite lesion, facilitating rapid blood uptake by the feeding tick. Prostaglandin E-2 (PGE-2) is produced in response to tick bite, suppressing an inflammatory response from macrophages, and also blocking IL-12 and TNF-α secretion by dendritic cells. The combined activity of these tick salivary proteins and other vector derived factors act to minimize inflammation, create a favorable microenvironment that facilitates dissemination of tick-borne pathogens, including Borrelia burgdorferi spirochetes that are inoculated into the bite lesion within 3 days of tick attachment and feeding.
IgE-mediated reactions against α-Gal–containing foods caused by tick bites have become an emerging allergic disease globally. Ixodes sp. saliva carries galactose-α-1,3-galactose (α-Gal)-containing proteins, which are absent in primates but transmitted to the host by tick bites leading to the induction of α-Gal–specific IgE, which will promote severe allergic reactions to foods like meat and dairy. Moreover, subcutaneous sensitization with tick salivary gland protein extracts alone is sufficient to induce IgE antibodies in response to α-Gal, supporting the role of skin inoculation of tick saliva as a mechanism that precipitates α-Gal syndrome (AGS). Ticks from the genera, Ixodes, Amblyomma, Haemaphysalis and Rhipicephalus have all been associated with the induction of this syndrome (112–115).
Once the tick salivary glands have been colonized, Borrelia spirochetes are subsequently deposited into the hemorrhagic feeding pool, typically 2 to 3 days post Ixodes spp. attachment (116). Co-inoculation of spirochetes with tick saliva promotes persistent skin infection and increases bacterial dissemination to distant tissues relative to intradermal inoculation of bacteria itself (117–120). Tick saliva has been shown in vitro to inhibit the complement system and it has been hypothesized that this activity suppresses the host effector function of granulocytes, macrophages, NK cells and B cells, and downmodulates Th1 differentiation in favor of a Th2 phenotype, which has been associated with Borrelia persistence (103, 121–123). Immunization against tick salivary protein Salp25D prevents Borrelia spirochete establishment in the vector by blocking detoxification of reactice oxygen species acquired from the blood meal (124). Of note, Borrelia spirochetes themselves have virulence factors that aid in dissemination and host immunomodulation (OspC, adhesions, decorin-binding protein, bbk13, etc.) (107, 125). Saliva from Rhipicephalus microplus suppresses T cell activation and Th1 polarization in cattle through upregulation of PD-L1 expression on CD14+ and CD11c+ cells. PD-L1 expression on these cells, in turn, inhibits activation of T cells by binding and signaling through the co-inhibitory T cell receptor, PD-1. The effect of R. microplus-saliva has been attributed to PGE2 induction, which may favour PD-L1 expression at skin bite sites in cattle (126). In this context, another potent anti-inflammatory salivary protein found in tick saliva is the Ixodes persulcatus salivary protein (IpSAP), which binds and blocks signaling through lymphotoxin β receptor (LTβR) involved in NF-kB activation and cell death processes mediated by TNF receptor associated factors (TRAF). This interaction leads to decreased skin inflammation in mice and elevated Borrelia bacterial loads and Lyme arthritis severity. The homolog of IpSAP in I. scapularis (IsSAP) exerts similar effects, and was also found to dampen serum levels of CCL5 and G-CSF, illustrating a potential mechanism by which IpSAP inhibits leukocyte recruitment to the skin (127). Interestingly, recent findings have demonstrated that egestion of Dae2, an antimicrobial toxin found in tick saliva, can lyse S. epidermidis and other gram-positive bacteria found in the skin microbiome to protect the tick from acquiring opportunist infections from the host skin while feeding. At the same time, Dae2 has only limited impact on Borrelia infection, which promotes tick tolerance to this infection by limiting its excessive proliferation in the vector (128). Of note, tick saliva carries a heterogenous population of extracellular vesicles with a size ranging from 173-200nm, which distinctly regulate infection of A. phagocytophilum and F. tularensis in the mammalian host during feeding. While extracellular vesicles from Ixodes scapularis favored infection of the mild rickettsial agent A. phagocytophilum to the mammalian host, extracellular vesicles from D. andersoni enhanced host tolerance to the lethal sepsis caused by F. tularensis in mice (129. Furthermore, exosomes isolated from the saliva and salivary glands of Ixodes scapularis impair human keratinocyte migration in culture, while enhancing IL-8 production and decreasing CXCL12 production from keratinocytes (130). Underscoring the central role played by this primitive cellular communication mechanism in distinct facets of interspecies cellular interactions with prominent implications for pathogen transmission and regulation of host skin immunity.
Most arthropods vectors incorporate diverse microbial symbionts, many of which are beneficial or even essential to the physiology and development of the vector. Among the most important are endosymbionts that play a mutualistic role in the vector’s biological and nutritional processes. Examples include Coxiella species in ticks, e.g., Amblyomma americanum, which are widespread throughout the tick’s tissues and are essential for the tick’s development and survival (131). Another example is Rickettsia buchneri, an endosymbiont prevalent in the Lyme disease tick, Ixodes scapularis (132, 133). However, certain of these endosymbionts may be transmitted to host skin during tick blood feeding. A Coxiella sp., Candidatus Coxiella massiliensis, was identified causing infection in human skin associated with development of an eschar (134). In contrast to the endosymbionts, mosquitoes, biting flies, and ticks often have enteric transient bacteria acquired from their external environment in their midguts. A recent study (135) showed that a common core of bacterial families, e.g., Enterobacteriaceae, Sphingomonadaceae, Pseudomonadaceae, etc., have genes that code for B vitamins and other essential nutrients, as well as essential amino acids and proteins that protect against oxidative stress and affect vector fitness. Yet, the role of the microbiome in the regulation of host skin immunity and how this consortium may affect transmission of tick-borne pathogens require further investigation.
Experiments in “non-permissive” hosts such as guinea pigs and rabbits show that certain host animals develop an acquired tick immunity upon repeated exposure to tick bites, resulting in early tick detachment and impairment of tick engorgement. These resistance effects are associated with massive infiltration of the skin by basophils and eosinophils, and interestingly, this acquired tick resistance (ATR) can be transferred to a tick-naïve host by T cell adoptive transfer (136, 137).
Extensive recent work has shed light into the mechanism behind ATR. Mouse models of ATR with Haemaphysalis longicornis larvae have demonstrated that antibody-mediated depletion of basophils abrogates ATR in mice. Interestingly, basophils are not the major population found at the site of tick bites in mice, but rather neutrophils and mononuclear phagocytes (138). Recruitment of basophils following 2nd tick infestation requires antigen specific IL-3 producing skin memory CD4+ T cells. Intravital imaging shows basophils swarming around tick bites and degranulation of these cells is mediated by the IgE Fc receptor, FcϵRI (139). Finally, secretion of histamine by basophils is critical to promote ATR (140). Of note, evidence acquired from ATR models in mice needs to be evaluated with caution since the incidence of tick detachment and impairment of tick engorgement is marginal in the mouse model. This contrasts with the classical models of guinea pigs and rabbits, where over 90% of ticks detach and/or cannot complete a blood meal, and infiltration of basophils is more pronounced (141, 142).
Alterations in skin architecture may also provide insights to better understand the sequential steps that lead to ATR. Skin lesions in the permissive host Peromyscus leucopus (white-footed mouse) retain preserved dermal architecture despite cellular infiltration upon repeated infestation, while bites on the non-permissive host Cavia porcellus (guinea pig) demonstrate hyperkeratotic changes to the epidermal layer and a breakdown of dermal architecture leading to a leukocyte-filled cavitary lesion, which appears to promote tick dislodgement, pointing for a possible role of keratinocytes hyperproliferation in ATR (143). A better mechanistic understanding of the factors and signals that drive ATR is critical to guide vector control strategies to limit tick feeding in the humans.
In Lyme disease-endemic areas, individuals with previous exposure to uninfected tick bites have been protected from Lyme disease, likely due to a hypersensitivity response which may lead to prompt removal of feeding ticks prior to spirochete transmission (144). In humans, mRNA levels of macrophage chemoattractant (CCL2/3/4) and neutrophil chemoattractant (CXCL1/8) are upregulated alongside IL-1β and IL-5 within the first 24 hours of Ixodes ricinus tick bites, yet this response becomes less apparent after 24 hours of attachment. Moreover, levels of lymphocyte cell markers and chemoattractants remain unaltered (145). While significant work has been done to characterize the role of salivary proteins in animal models such as guinea pigs, mice, and rabbits, more work is needed to characterize the role of tick saliva in humans (107). Although tick bites are characterized in animal models by severe damage to the skin with histopathological signs of necrosis in the dermis and epidermis, the early inflammatory response observed in the skin of bitten volunteers is relatively mild with increased levels of lymphocytes, mononuclear phagocytes and the variable presence of neutrophils. Strobl et al. performed a clinical study to explore features of skin immune responses to recent naturally acquired tick bites (≤9 days). Biopsies of the tick bite sites showed increased infiltration of neutrophils, B cells, and T cells, while the numbers of dermal DCs and Langerhans cells decreased. T cells at the bite site showed a significant bias towards CD8+ over CD4+ T cells, a reduction in Th2 cells, and ILC1, ILC2, and ILC3 subsets. In contrast, the tick bite induced significant increases in the numbers of resident memory T cells (CD69+ and CD103+ subsets) and γδ T cells. Using an ex vivo skin explant model, the authors demonstrated that tick SGE creates a permissive skin environment for Borrelia infection by suppressing local recruitment of neutrophils, macrophages, and T cells (146). Furthermore, the presence of cement – a mucoid substance produced by tick salivary glands – seems to be inversely correlated with the intensity of the host skin inflammatory reaction, indicating that cement components have an immunosuppresive effect on human skin (147). Basophil infiltration is also one of the hallmarks of tick bites in human skin (141). Recent advances in the understanding of α-Gal syndrome have demonstrated that T cells from AGS patients display specificity to peptides derived from proteins present in tick saliva regardless of the presence of α-Gal, which skews T cell differentiation towards a Th2 phenotype. Yet, a fraction of B cells proliferates in response to α-Gal epitopes and requires T cell support for activation via CD40L and IL-4 (148).
Mosquito-borne arboviruses have experienced a striking re-emergence in the last few decades, causing substantial public health alarm (149). Most notably, the number of cases of dengue fever has doubled in the last 20 years (150), and we recently experienced a Zika virus epidemic in the Americas (1). Epidemiological assessments estimate that mosquito-borne arboviruses pose a threat to half of the global population (151, 152). In this context, Aedes aegypti is the primary vector of several mosquito-borne viruses such as dengue (DENV), Zika (ZKV), chikungunya (CHIKV), yellow fever (YFV), Rift Valley fever (RVFV), Cache Valley (CVV) and West Nile (WNV) viruses (153). During feeding, Ae. aegypti penetrates the skin with its proboscis, then takes a blood meal over the course of a few minutes (154). After inserting its proboscis, the mosquito salivates into the wound, depositing both saliva as well as arboviruses into the skin (153, 155). In the context of disease transmission, Ae. aegypti saliva enhances arboviral infection and transmission for several viruses.
The enhancing role of Aedes saliva in pathogen transmission and disease pathogenesis after mosquito bites has been investigated in animal models for many different arboviroses, including CVV (156), RVF (157), DENV (158, 159), SFV (160) and CHIKV (161). In the presence of saliva, all these viruses exhibited more extensive systemic dissemination, increased viral titers, leading to higher mortality in mice. In general, the mosquito bite delays the onset, but increases the peak and duration of viremia in mouse models. Early studies with mice demonstrated that pre-exposure to mosquito bites enhances viremia when CVV is inoculated at the bite site (156), and that mosquitoes likely inject the virus into the skin tissue but not directly into the vasculature, as removal of bitten tails one to six hours after the bite reduced pathology and prolonged mouse survival (156, 162). Infection with DENV in pre-exposed mice is known to enhance disease morbidity and mortality due to the generation of non-neutralizing antibodies that enhance viral uptake by Fcγ receptor-bearing cells. Using an animal model of antibody dependent enhancement in dengue, investigators have demonstrated that excision of the bitten skin 4 hours after co-inoculation of saliva with DENV did not prevent disease in Ifnar–/– susceptible mice (163), indicating that the high dose of saliva co-injected in this model was sufficient to accelerated viral dissemination within this time frame. Nevertheless, tissue structural properties may have favored virus dissemination in the mouse ear compared to the tail skin model. Schimid et al., also observed enhancement of vascular endothelial permeability, migration of dendritic cells to draining lymph nodes and increased myeloid cell infiltration at the site of infection. The effect of saliva on viral dissemination and disease progression was further validated in humanized mice engrafted with human hematopoietic cells (164) and non-human primates (165, 166). Natural transmission via vector also modulates viral tissue dissemination in rhesus macaques, since subcutaneous needle infection with 104 PFU of ZIKV in the cranial dorsum yielded no detectable viral RNA in the cerebrum, ovary, uterus, or eyelid conjunctivae as aopposed to natural transmission (165).
Aedes bites trigger an inflammatory reaction characterized by interactions at the site of the bite involving resident skin cells, infiltrating cells, danger signals, and various vector derived factors including vector salivary gland content, which is the most well studied (Figure 3) (163, 167). The mosquito bite itself upregulates the local expression of neutrophil (CXCL1, CXCL2, CXCL3, and CXCL5) and monocyte (CCL2, CCL4, CCL5, CCL7, and CCL12)-attracting chemokines (160, 163, 167). Additionally, after mosquito bites, an increase in mast cells, eosinophils, and CD4+ T cells numbers was observed in SGE-sensitized mice when compared to non-sensitized mice (168). In the context of infection, co-inoculation of DENV and Aedes saliva in mice enhanced neutrophil and monocyte recruitment and migration of antigen presenting cells (APCs) to the draining lymph nodes, enhancing viral dissemination (163). Indeed, IL-1β-driven influx of neutrophils and monocytes is critical for retention of virus in the skin and viral systemic dissemination, leading to enhanced pathology (160). Furthermore, Ae. aegypti saliva possesses a serine protease cofactor CLIPA3 that digests extracellular matix proteins to facilitate viral attachment, dissemination, and cell migration (169).
Figure 3 During a blood meal, an infected Aedes aegypti mosquito introduces its proboscis into the skin, eggesting saliva and viral particles into the dermis, leading to endothelial permeability, leakage and lysis of RBCs triggering an orchestrated immune response at bite site. At the epidermis, arboviruses replicate in their primary target cells, keratinocytes and Langerhans cells. Neutrophils and monocytes are rapidly recruited from the bloodstream to the dermis by neutrophil (CXCL1, CXCL2, CXCL3, and CXCL5) and monocyte (CCL2, CCL4, CCL5, CCL7, and CCL12)-chemoattractants. Additionally, monocytes are recruited by neutrophils in an IL-1β dependent loop. Neutrophil-driven inflammation, leads to further recruitment of monocytes, antigen presenting cells (APCs) and other myeloid cells. Infected cells disseminate the virus to the skin draining lymph nodes. Salivary proteins cause a local oedema causing viral retention at the bite site. The recruited cells in combination with upregulation of IL-4, IL-10, IL-12 and IFN-β, and downregulation of IL-2, IFN-γ,TNF-α and CXCL10, due to mosquito saliva, enhance viral dissemination and disease pathogenesis. NeSt1, a salivary protein from Aedes aegypt, has been shown to directly activate neutrophils to secrete IL-1β and CXCL2, while AgBR1, also isolated from mosquito saliva, can elicit neutrophil infiltration and induce secretion of cytokines. In case of sensitized skin to mosquito saliva (insert box) myeloid cells are fastly recruited, while IgE and IgG antibodies recognize several salivary proteins as allergens.
Aside from chemokines, mosquito bites regulate the expression of several genes involved in the immune response to infection. Conserved features of this mosquito bite response include a shift in the skin inflammatory reaction towards a type 2 response with upregulation of IL-4, IL-5, IL-10, and downmodulation of IL-2 (160, 161, 166, 167, 170). Concomitantly, downregulation of TLR3 and TLR7 supports the role of the vector in the establishment of viral infection by suppressing pattern recognition receptors and antiviral responses (159, 161, 170). Delayed expression of type I interferons and IFN-γ has been described following co-inoculation of viruses at mosquito bite sites, which correlates with enhanced viral load and dissemination to draining lymph nodes (160).
Several specific salivary proteins from Ae. aegypti have been shown to modulate the innate immune response to enhance arboviral infection and replication in animal models. For instance, neutralization of the Aedes salivary proteins neutrophil stimulating factor 1 (NeSt1) (171) and AgBR1 (172) by passive immunization led to decreased infiltration of neutrophils and monocytes at the bite site, as well as reduced expression of IL-1β and CXCL2, and IL-1β, IL-6, CXCL1, and CCL2, respectively. This downregulation of the inflammatory response ultimately led to partial protection against mosquito transmitted ZIKV infection and ameliorated disease pathology. Moreover, a dual passive immunization approach with both AgBR1 and NeSt1 anti-sera resulted in a robust protection with significantly reduced viremia and enhanced survival against mosquito transmitted ZIKV infection (173). The salivary protein Aedes aegypti Neutrophil Recruitment Protein (AaNRP) enhances viral load of ZIKV and DENV 2 both in the skin and systemically (174). Mechanistically, authors demonstrated that AaNRP binds directly to TLR1 and TLR4, and promotes the production of the chemokines CXCL1, CXCL2, and CXCL3 from skin resident macrophages in a MyD88-dependent manner. In turn, these chemokines recruit neutrophils, monocytes, macrophages, and dendritic cells to the skin, thereby increasing the local reservoir of arbovirus-susceptible cells and facilitating systemic dissemination. Additionally, LTRIN, a 15-kDa Aedes salivary protein, promotes dimerization and activation of LTβR and subsequent inhibition of NF-kB activation, IL-1α, IL-6 and TNF-α secretion in mouse bone marrow derived macrophages (BMDMs), mouse skin fibroblasts (MSFs), human umbilical vein endothelial cells (HUVECs) and human THP-1 monocytic cells (175), which dampens antiviral response. Sun et al. identified a salivary protein, Aedes aegypti venom allergen-1 (AaVA-1), involved in the activation of autophagy, which favors transmission of flaviviruses in the host (176). In animals bitten by ZIKV-infected Aedes aegypti, AaVA-1 silencing resulted in a decrease in viremia and mortality. Intense hemorrhage was observed in the skin of Stat1-/- susceptible mice inoculated with saliva from control mosquitos in comparison to saliva from DENV infected AaSG34 mosquitoes (177). Sialokinin is a salivary protein that acts as vasodilator and contributes to successful feeding of mosquitoes (178). Targeted mutation in sialokinin gene, leading to loss of function, reduced capacity of saliva to enhance blood perfusion at the site of the bite, and compromised endothelial permeability and migration of neutrophils. In contrast, some proteins in Ae. aegypti can inhibit viral infection; for example, inoculation of Aedes D7 recombinant protein has been shown to inhibit DENV infection in vitro and in vivo through an unknown mechanism that might envolve enhancing host cell resistance or direct binding to virion envelope proteins (169).
Early studies described the human skin reaction to mosquito bites, including allergic reactions, persistent inflammation, and histopathological features at the bite site in response to Ae. aegypti bites (179–183). Histopathological analysis of human skin sections obtained from subjects with a mosquito skin reaction showed an inflammatory response characterized by increased vascular permeability and influx of neutrophils and eosinophils peaking at 6 hrs, followed by lymphocytes and macrophages 24 to 48 hrs after the bite (181, 182). Notably, most human studies showed different levels of sensitization to Ae. aegypti bites, characterized by an immediate hypersensitivity reaction followed by a delayed type hypersensitivity (DTH) response. Immediately after the first Aedes exposure, subjects developed wheal shaped erythema, followed by a late papular reaction by 24 hours post-bite. Repeated Aedes exposure resulted in progressive desensitization (179, 180, 182, 183). Recently, a study conducted transcriptional profiling and immunophenotyping of bitten skin of healthy subjects from Cambodia, where Aedes aegypti is prevalent. Volunteers had pre-exposure to mosquitoes confirmed by anti-NS1 antibodies, but only a small fraction of participantes reported previous dengue infection, which might indicate that likely most individuals were asymptomatic to infection. Many features of the host skin immunity against mosquitoes bites previously observed in rodents were validated in this cohort such as early granulocytes infiltration and skewed response towards type 2 immunity. Salivary gland extract suppressed IFN-γ and IL-2 production in PBMCs in a recall stimulation assay with PMA/ionomycin, however whether this a unspecific effect of saliva on T cell activation or a result of the expansion of regulatory antigen-specific cells remains to be clarified (184).
Rockwell and Johnson first proposed the involvement of mosquito saliva in the skin allergic reactions of humans to mosquito bites (182). Mice sensitized to mosquito bites had similar responses compared to humans who developed a severe allergic local skin reaction to Ae. aegypti bites (185, 186). Both mice and humans had high blood levels of IgE and IgG against whole Ae. aegypti saliva with antibody titers positively correlating with the observed skin allergic reactions. These studies led to the identification of several mosquito salivary allergens from Aedes, Culex and Anopheles, reviewed elsewhere (187, 188).
In summary, animal studies thus far have elucidated the effect of Aedes salivary proteins and their importance in arboviral pathogenesis, while the role of other important vector derived factors such as the microbiota remain largely unexplored. Non-human primates (165, 166) and humanized mouse models are seeing increasing use in infected Aedes challenge studies to more closely model human immune responses (164, 189). Nevertheless, major gaps still exist regarding mosquito-host immune interactions at the bite site. Notably, the handful of human studies available had a limited number of subjects and lack the in-depth immunological characterization available with current technologies.
Malaria is a vector-borne disease caused by Plasmodium parasites transmitted via the bite of a female Anopheles mosquito, which deposits the infective sporozoite form of Plasmodium into the host skin. Malaria is one of the deadliest infections worldwide, which accounted for approximately 247 million cases and 619,000 deaths in 2021 (190). Here, we review current knowledge of how Anopheles bites and their saliva influence Plasmodium infection and pathogenesis in mouse models and humans.
There has been substantial debate as to whether Anopheles bites and saliva affect the establishment and dissemination of Plasmodium infection. Early studies to address this question arrived at conflicting conclusions and were confounded by (1) the inability to measure or inoculate equivalent numbers of sporozoites by syringe needle versus Anopheles bite, (2) testing only in animals immunized with malaria vaccine candidates (191), and (3) non-physiologic routes of inoculation, including intraperitoneal infection with infected RBCs (192) or intravenous co-inoculation of Anopheles SGE with sporozoites (193). In mice infected intradermally with sporozoites, Kebaier et al. found that co-inoculation with SGE had no effect on the percentage of mice that developed parasitemia or the length of the prepatent period, which characterizes the phase between transmission and the detection of parasites in the blood and other internal organs.
A study by Schneider et al. helped shed light on this debate by employing a murine model of cerebral malaria using An. stephensi and Plasmodium berghei (194). Sporozoites were injected intradermally either alone or at the site of An. stephensi bites, and mosquito-bitten mice showed higher parasitemia, and markedly decreased survival (35% vs 80% for unbitten), though interestingly there was no difference in parasite load in the skin, draining lymph nodes, or liver. Presence of saliva at the site of infection enhanced granulocyte and eosinophil infiltration, but reduced recruitment of DCs compared to mice injected with sporozoites alone. Mice bitten by An. stephensi showed higher mRNA expression of the Th2 cytokines IL-4 and IL-10 in the skin and draining lymph node, as well as higher expression of the immune checkpoint proteins CTLA-4 and IL-18 binding protein, the latter of which antagonizes IFN-γ production. In total, this cerebral malaria model demonstrates that Anopheles bites exacerbate malarial disease without necessarily affecting organ parasite load, and this effect is potentially mediated by polarization towards a Th2 response and inhibitory signals via immune checkpoint pathways (194).
The question of whether pre-exposure to Anopheles bites protects against malaria has been similarly controversial. Both Donovan et al. and Fonseca et al. showed lower levels of parasitemia in pre-exposed mice after infected mosquito challenge (192, 195), however Kebaier et al. found mosquito pre-exposure had no effect on the overall percentage of mice that developed detectable parasitemia by 14 days post-challenge (193). Fonseca et al. and Kebaier et al. also report conflicting results on whether pre-exposure to Anopheles bites affects the duration of the prepatent period. The contrasting findings may be attributable to differences in pre-exposure protocols, Plasmodium species used, and the chosen endpoint for parasitemia.
With regard to liver infection, mice pre-exposed to uninfected Anopheles stephensi bites and challenged with Plasmodium yoelii-infected An. stephensi show lower liver parasite burdens compared to bite-naïve mice, and this protection requires IFN-γ (195). Pre-exposed mice showed increased IFN-γ and decreased IL-4 mRNA expression in the skin, although pre-exposure has no effect on skin parasite burden following infected mosquito challenge (193, 195). Interestingly, pre-exposure to Anopheles bites results in lower liver parasite burdens even when P. yoelii is given intravenously. Accordingly, both the liver and spleen of pre-exposed mice show increased mRNA expression of IFN-γ and IL-12p40, and lower expression of IL-4. Similarly, using An. stephensi infected with Plasmodium chabaudi, Fonseca et al. showed pre-exposed mice had higher numbers of splenic CD4+ T cells compared to bite-naïve mice, and that these CD4+ T cells express higher levels of both IFN-γ and IL-4 (192). Overall, these studies suggest that pre-exposure to Anopheles bites potentially generates a humoral response that will neutralize salivary componenets that favor transmission but can also induce a cellular Th1 responses that cross protects against Plasmodium infection not only in the skin, but also systemically in visceral organs.
Many other aspects of the host immune response can be modulated by anopheline saliva. Anopheles saliva induces the degranulation of human mast cell lines in culture, and activation of mast cells in the mouse skin. In the murine model, An. stephensi bites lead to neutrophil recruitment to the skin, hyperplasia of skin-draining lymph nodes, and a pronounced influx of CD3+, B220+, CD11b+, and CD11c+ leukocytes (196). Intradermal injection of Anopheles SGE into mice induces transcriptional upregulation of CXCL11, IFN-γ, CXCL10, granzyme B, CXCL9, IL-2Rβ, TGF-β, IL-2, and CD69 (197).
Several proteins present in Anopheles saliva exhibit immunoregulatory activity. Saliva from An. stephensi contains a high molecular weight glycoprotein which functions as a neutrophil chemotactic factor (NCF) at the bite site (198), though the identity of this protein remains to be determined. Despite of this, agaphelin, an anopheline salivary protein whose expression is upregulated upon Plasmodium falciparum infection, suppresses several neutrophil functions, including production of neutrophil extracellular traps, elastase/cathepsin-mediated platelet aggregation, and neutrophil-mediated coagulation (199). Furthermore, Sporozoite-associated mosquito saliva protein 1 (SAMSP1) inhibits neutrophil chemotaxis in vivo and in vitro (200). Therefore, overall evidence supports that Anopheles saliva composition suppress neutrophil infiltration and response. Non-protein salivary factors likely also modulate host immunity; for instance, Anopheles coluzzii saliva is enriched for several microRNAs (miRNAs) which show high sequence similarity to human miRNAs and are predicted to regulate key intracellular immune signaling and chemokine pathways (5). Regarding immunization studies, an A. gambiae salivary protein, AgTRIO, contributes to protection to challenge with either P. falciparum-infected A. gambiae or A. stephensi mosquitoes when humanized mice are passively immunized with antisera against AgTRIO. Active immunization with AgTRIO also resulted in reduced parasitemia in mice exposed to P. berghei-infected A. gambiae mosquitoes. Regarding possible mechanisms of this protection, two-photon microscopy revealed that treatment with AgTRIO antisera led to decreased sporozoite velocity and movement in murine skin (201). Furthermore, AgTRIO mRNA vaccine conjugated to lipid nanoparticles and a monoclonal IgG2a antibody, 13F-1, has also confered considerable protection to mice exposed to Plasmodium berghei-infected mosquitoes, pointing that this target could potentially aid vaccines developed against parasite antigens. Another interesting aspect of AgTRIO is that although the bite per se does not induce measurable antibody titers against AgTRIO nor confers protection to disease, tthe humoral responses are can be boosted by Anopheles bites following active immunization, which indicates a more sustained effect of this vaccine prototype in endemic areas (202, 203). More salivary proteins from Anopheles have also shown protection to infected-mosquito bites reducing liver burden when administrated as both active and passive immunizations (SAMSP1 and AgSAP) and could be potentially suitable candidates to a multi-component vaccine (200, 204). Interentingly, salivary proteins in the anopheline saliva may also compromise sporozoites infetivity. A salivary protein homolog of The Gamma interferon inducible lysosomal thiol reductase (GILT), name Mosquito GILT, or mosGILT, has been shown to bind Plasmodium sporozoites and impair parasite motility at the host skin, which is critical for establishment of infection in the mammalian host. Despite of this, biological significance of this mechanism is controversial in the context of whole saliva as most proteins favor infection establishment hence its main role may be related to containment of parasites inside the salivary glands or to avoid overinfection of the host and preserve a source of bloodmeals longer (205). The key immunoregulatory effects triggered by Anopheles saliva at bite sites, as outlined in this review, are summarized in Figure 4.
Figure 4 Anopheles saliva contains many bioactive molecules that perform a range of functions once deposited into the host at the bite site. The recombinant protein mosGILT has been shown to slow sporozoite movement in the host, while the recombinant protein AgTRIO is recognized by host IgG antibodies and contributes to protection to challenge with either P. falciparum-infected A. gambiae or A. stephensi mosquitos. The circumsporozoite (CSP) surface protein is recognized by antibodies, creating an immune complex that blocks the mosquito proboscis, which can disrupt feeding. Neutrophils (recruited by NCF), regulatory T cells (Tregs), helper T cells, dendritic cells, mast cells, and neutrophils are recruited to the bite site. IFN-γ is upregulated, while IL-4 is downregulated in response to mosquito saliva. Mast cells become activated in response to Anopheles bites, releasing histamine.
Studies have revealed that the microbiome of mosquitoes varies across different tissues, including the cuticle surface, midgut, salivary glands, and reproductive tract, with potential direct and indirect impacts on pathogen transmission (206). Direct impacts involve vectorial competence, affecting the ability of mosquitoes to acquire, maintain, and transmit pathogens. Indirect impacts concern vectorial capacity, dependent on ecological niche dynamics. For instance, mosquito microbiota can influence vector competence for DENV by modulating midgut enzymes (207). Attention to the indirect effects of microbiota on vectorial capacity is growing, exemplified by Serratia’s role, a mosquito gut endosymbiont, in curtailing hematophagy and delivering antimalarial effectors (208; 209). Moreover, Wolbachia infection has been successful in reducing vector competence and disease transmission, notably in Aedes mosquitoes (210, 211). Variations in microbiome composition, such as dominance of Pseudomonas sp. in malaria-free regions, suggest a potential microbiome-gut-brain-axis with implications for vector competence (212, 213). Despite progress, knowledge gaps persist, underscoring the need for further research into the mosquito microbiome’s impacts on transmission dynamics (214).
There is scant data on human skin immune responses to the bites of Anopheles mosquitos. A limited case series describes the variability of rashes elicited by the bites of Anopheles quadrimaculatus, ranging from immediate wheal responses, to delayed papules, to no reactions at all (182). In one pediatric patient with severe mosquito allergy, the An. quadrimaculatus bite site was infiltrated with neutrophils, lymphocytes, plasma cells, histiocytes, and eosinophils, extending to the subcutis. Since this early study, there has been minimal to absent further clinical investigation of cutaneous immunity to Anopheles bites in humans. Controlled human malaria infection (CHMI) has emerged as an important tool to evaluate the efficacy of vaccine formulations. Similar results, compared to field trials have been observed for vaccines such as R32ToxA and RTS,S (215). Furthermore, clinical studies focusing on the immunological understanding of the initial Anopheles-Plasmodium-skin interaction events could be readily incorporated into existing CHMI protocols. Of note, during the elaboration of this review WHO has approved and recommended two vaccines for malaria: R21/Matrix-M and RTS,S/AS01. Both vaccines have proven to be safe in trials conducted in the African continent with young infants and required booster shots to maximize effectiveness. However, R21/Matrix-M has shown higher efficacy (>75%) despite a smaller study cohort compared to RTS,S/AS01 (30%), which performed slightly better in children aged 5-17 months (216, 217). RTS,S/AS01 is a recombinant protein vaccine that targets the circumsporozoite protein of P. falciparum, expressed by the parasite during the pre-erythrocytic stage. In its construction, hepatitis B surface antigen (HBsAg) has been fused to the C-terminus and central repeats of the CSP (RTS). RTS construct is then co-expressed on yeasts with unmodified recombinant HBsAg (S) to generate 22nm lipid-protein RTS,S particles, which display 1:4 ratio of the protein RTS and S, respectively. Such excessive amount of S protein could be related to RTS,S limited efficacy. On the other hand, R21 is considered a next generation RTS,S-like vaccine as it lacks the excess of HBsAg found in the former and is delivered as a single CSP-HBsAg fusion protein (218). Nevertheless, both R21 and RTS,S require boosts and the extent of the protective effect of these vaccines remain unknown. Clinical trials have attested the safety and immunogenicity of a peptide-based vaccines derived from A. gambiae saliva based on in silico selection of predicted T-cell epitopes. AGS-v PLUS is a vaccine containing five synthetic mosquito salivary peptides, while AGV-v contains four peptides. Immunization of mice with AGS-v has conferred more than 50% protection in a experimental model of malaria infection delivered by infected bites of A. gambiae with P. yoelii nigeriensis (219, 220). Therefore, a multi-component vaccine may be useful to finally achieve eradication of Malaria.
As seen across these four distinct vector-pathogen pairs, vector biologists have gleaned remarkable insights into how skin immune responses to vector bites facilitate pathogen dissemination, a unifying paradigm built on over three decades of research. Looking towards the coming decade, the development of multiple novel experimental methodologies paves the way for promising new lines of investigation into the mechanisms behind vector bite responses in the host skin, particularly in humans. Undoubtedly, the mouse has taken us far – but vector biologists must now make a concerted effort to study human responses to vector bites in clinical studies to assure research translational power (221). Continued progress will rely on multidisciplinary collaborations between clinicians, immunologists, vector biologists and others whose complementary expertise and creativity will drive our efforts to generate the knowledge required to eradicate vector-borne diseases.
JL: Investigation, Supervision, Validation, Writing – original draft, Writing – review & editing. RK: Data curation, Resources, Validation, Visualization, Writing – review & editing. JD: Investigation, Validation, Writing – original draft, Writing – review & editing. MD: Investigation, Writing – original draft. GP: Investigation, Writing – original draft. MS: Investigation, Writing – original draft. EL: Investigation, Writing – original draft. JO: Investigation, Validation, Writing – original draft, Writing – review & editing. DS: Investigation, Supervision, Validation, Writing – original draft, Writing – review & editing. TD-V: Conceptualization, Formal Analysis, Investigation, Project administration, Supervision, Validation, Writing – original draft, Writing – review & editing.
The author(s) declare financial support was received for the research, authorship, and/or publication of this article. This work was supported by the NIAID Intramural Research Program, the NIAID Transition Program in Clinical Research, and the NIH Bench-to-Bedside Program 2016 from the NIH Clinical Center, Dimensions Sciences, and Conselho Nacional de Desenvolvimento Cientıfíco e Tecnológico (CNPq).
We are grateful to all of those we have had the privilege to work with during the initial drafts of this project.
The authors declare that the research was conducted in the absence of any commercial or financial relationships that could be construed as a potential conflict of interest.
All claims expressed in this article are solely those of the authors and do not necessarily represent those of their affiliated organizations, or those of the publisher, the editors and the reviewers. Any product that may be evaluated in this article, or claim that may be made by its manufacturer, is not guaranteed or endorsed by the publisher.
2. Kazimírová M, Sulanová M, Trimnellt AR, Kozánek M, Vidlicka L, Labuda M, et al. Anticoagulant activities in salivary glands of tabanid flies. Med Vet Entomol. (2002) 16:301–9. doi: 10.1046/j.1365-2915.2002.00379.x
3. Bernard Q, Jaulhac B, Boulanger N. Skin and arthropods: an effective interaction used by pathogens in vector-borne diseases. Eur J Dermatol. (2015) 25 Suppl 1:18–22. doi: 10.1684/ejd.2015.2550
4. Dey R, Joshi AB, Oliveira F, Pereira L, Guimaraes-Costa AB, Serafim TD, et al. Gut Microbes Egested during Bites of Infected Sand Flies Augment Severity of Leishmaniasis via Inflammasome-Derived IL-1beta. Cell Host Microbe. (2018) 23:134–143 e136. doi: 10.1016/j.chom.2017.12.002
5. Arca B, Colantoni A, Fiorillo C, Severini F, Benes V, Di Luca M, et al. MicroRNAs from saliva of anopheline mosquitoes mimic human endogenous miRNAs and may contribute to vector-host-pathogen interactions. Sci Rep. (2019) 9:2955. doi: 10.1038/s41598-019-39880-1
6. Sultana H, Neelakanta G. Arthropod exosomes as bubbles with message(s) to transmit vector-borne diseases. Curr Opin Insect Sci. (2020) 40:39–47. doi: 10.1016/j.cois.2020.05.017
7. Schneider CA, Calvo E, Peterson KE. Arboviruses: how saliva impacts the journey from vector to host. Int J Mol Sci. (2021) 22:9173. doi: 10.3390/ijms22179173
8. Ali A, Zeb I, Alouffi A, Zahid H, Almutairi MM, Ayed Alshammari F, et al. Host immune responses to salivary components - A critical facet of tick-host interactions. Front Cell Infect Microbiol. (2022) 12:809052. doi: 10.3389/fcimb.2022.809052
9. Leal-Galvan B, Arocho Rosario C, Oliva Chávez A. Extracellular vesicles and immunomodulation in mosquitoes and ticks. Encyclopedia. (2022) 2:873–81. doi: 10.3390/encyclopedia2020057
10. Arora G, Chuang YM, Sinnis P, Dimopoulos G, Fikrig E. Malaria: influence of Anopheles mosquito saliva on Plasmodium infection. Trends Immunol. (2023) 44:256–65. doi: 10.1016/j.it.2023.02.005
11. Som PM, Laitman JT, Mak K. Embryology and anatomy of the skin, its appendages, and physiologic changes in the head and neck. Neurographics. (2017) 7:390–415. doi: 10.3174/ng.9170210
12. Nafisi S, Maibach H. Skin penetration of nanoparticles. In: Shegokar R, Souto E, editors. Emerging Nanotechnologies in Immunology, 1st ed. Elsevier (2018).
13. Pasparakis M, Haase I, Nestle FO. Mechanisms regulating skin immunity and inflammation. Nat Rev Immunol. (2014) 14:289–301. doi: 10.1038/nri3646
14. Zomer HD, Trentin AG. Skin wound healing in humans and mice: Challenges in translational research. J Dermatol Sci. (2018) 90:3–12. doi: 10.1016/j.jdermsci.2017.12.009
15. Nystrom A, Bruckner-Tuderman L. Matrix molecules and skin biology. Semin Cell Dev Biol. (2019) 89:136–46. doi: 10.1016/j.semcdb.2018.07.025
16. Wei JCJ, Edwards GA, Martin DJ, Huang H, Crichton ML, Kendall M. Allometric scaling of skin thickness, elasticity, viscoelasticity to mass for micro-medical device translation: from mice, rats, rabbits, pigs to humans. Sci Rep. (2017) 7:15885. doi: 10.1038/s41598-017-15830-7
17. Aoyagi S, Takaoki Y, Takayanagi H, Huang CH, Tanaka T, Suzuki M, et al. (2012). “Equivalent negative stiffness mechanism using three bundled needles inspired by mosquito for achieving easy insertion”, in: 2012 IEEE/RSJ International Conference on Intelligent Robots and Systems, . doi: 10.1109/IROS.2012.6386088
18. Li ADR, Putra KB, Chen L, Montgomery JS, Shih A. Mosquito proboscis-inspired needle insertion to reduce tissue deformation and organ displacement. Sci Rep. (2020) 10:12248. doi: 10.1038/s41598-020-68596-w
19. Szolnoky G, Bata-Csorgo Z, Kenderessy AS, Kiss M, Pivarcsi A, Novak Z, et al. A mannose-binding receptor is expressed on human keratinocytes and mediates killing of Candida albicans. J Invest Dermatol. (2001) 117:205–13. doi: 10.1046/j.1523-1747.2001.14071.x
20. Nestle FO, Di Meglio P, Qin JZ, Nickoloff BJ. Skin immune sentinels in health and disease. Nat Rev Immunol. (2009) 9:679–91. doi: 10.1038/nri2622
21. Byrd AL, Belkaid Y, Segre JA. The human skin microbiome. Nat Rev Microbiol. (2018) 16:143–55. doi: 10.1038/nrmicro.2017.157
22. Lucas-Barbosa D, DeGennaro M, Mathis A, Verhulst NO. Skin bacterial volatiles: propelling the future of vector control. Trends Parasitol. (2022) 38(1):15–22. doi: 10.1016/j.pt.2021.08.010
23. Clayton K, Vallejo AF, Davies J, Sirvent S, Polak ME. Langerhans cells-programmed by the epidermis. Front Immunol. (2017) 8:1676. doi: 10.3389/fimmu.2017.01676
24. Doebel T, Voisin B, Nagao K. Langerhans cells - the macrophage in dendritic cell clothing. Trends Immunol. (2017) 38(11):817–28. doi: 10.1016/j.it.2017.06.008
25. Ali N, Rosenblum MD. Regulatory T cells in skin. Immunology. (2017) 152:372–81. doi: 10.1111/imm.12791
26. Jiang X, Campbell JJ, Kupper TS. Embryonic trafficking of gammadelta T cells to skin is dependent on E/P selectin ligands and CCR4. Proc Natl Acad Sci U.S.A. (2010) 107:7443–8. doi: 10.1073/pnas.0912943107
27. Nguyen AV, Soulika AM. The dynamics of the skin’s immune system. Int J Mol Sci. (2019) 20. doi: 10.3390/ijms20081811
28. Kabashima K, Honda T, Ginhoux F, Egawa G. The immunological anatomy of the skin. Nat Rev Immunol. (2019) 19:19–30. doi: 10.1038/s41577-018-0084-5
29. Malissen B, Tamoutounour S, Henri S. The origins and functions of dendritic cells and macrophages in the skin. Nat Rev Immunol. (2014) 14:417–28. doi: 10.1038/nri3683
30. Tamoutounour S, Guilliams M, Montanana Sanchis F, Liu H, Terhorst D, Malosse C, et al. Origins and functional specialization of macrophages and of conventional and monocyte-derived dendritic cells in mouse skin. Immunity. (2013) 39:925–38. doi: 10.1016/j.immuni.2013.10.004
31. Epelman S, Lavine KJ, Randolph GJ. Origin and functions of tissue macrophages. Immunity. (2014) 41:21–35. doi: 10.1016/j.immuni.2014.06.013
32. Yanez DA, Lacher RK, Vidyarthi A, Colegio OR. The role of macrophages in skin homeostasis. Pflugers Arch. (2017) 469:455–63. doi: 10.1007/s00424-017-1953-7
33. Krystel-Whittemore M, Dileepan KN, Wood JG. Mast cell: a multi-functional master cell. Front Immunol. (2015) 6:620. doi: 10.3389/fimmu.2015.00620
34. Clark RA, Chong BF, Mirchandani N, Yamanaka K, Murphy GF, Dowgiert RK, et al. A novel method for the isolation of skin resident T cells from normal and diseased human skin. J Invest Dermatol. (2006) 126:1059–70. doi: 10.1038/sj.jid.5700199
35. Harrison OJ, Linehan JL, Shih HY, Bouladoux N, Han SJ, Smelkinson M, et al. Commensal-specific T cell plasticity promotes rapid tissue adaptation to injury. Science. (2019) 363. doi: 10.1126/science.aat6280
36. Kamhawi S. Phlebotomine sand flies and Leishmania parasites: friends or foes? Trends Parasitol. (2006) 22(9):439–45. doi: 10.1016/j.pt.2006.06.012
37. Courtenay O, Peters NC, Rogers ME, Bern C. Combining epidemiology with basic biology of sand flies, parasites, and hosts to inform leishmaniasis transmission dynamics and control. PloS Pathog. (2017) 13(10):e1006571. doi: 10.1371/journal.ppat.1006571
38. Peters NC, Egen JG, Secundino N, Debrabant A, Kimblin N, Kamhawi S, et al. In vivo imaging reveals an essential role for neutrophils in leishmaniasis transmitted by sand flies. Science. (2008) 321:970–4. doi: 10.1126/science.1159194
39. Ribeiro-Gomes FL, Peters NC, Debrabant A, Sacks DL. Efficient capture of infected neutrophils by dendritic cells in the skin inhibits the early anti-leishmania response. PloS Pathog. (2012) 8:e1002536. doi: 10.1371/journal.ppat.1002536
40. Romano A, Carneiro MBH, Doria NA, Roma EH, Ribeiro-Gomes FL, Inbar E, et al. Divergent roles for Ly6C+CCR2+CX3CR1+ inflammatory monocytes during primary or secondary infection of the skin with the intra-phagosomal pathogen Leishmania major. PloS Pathog. (2017) 13:e1006479. doi: 10.1371/journal.ppat.1006479
41. Chaves MM, Lee SH, Kamenyeva O, Ghosh K, Peters NC, Sacks D. The role of dermis resident macrophages and their interaction with neutrophils in the early establishment of Leishmania major infection transmitted by sand fly bite. PloS Pathog. (2020) 16:e1008674. doi: 10.1371/journal.ppat.1008674
42. Afonso L, Borges VM, Cruz H, Ribeiro-Gomes FL, DosReis GA, Dutra AN, et al. Interactions with apoptotic but not with necrotic neutrophils increase parasite burden in human macrophages infected with Leishmania amazonensis. J Leukoc Biol. (2008) 84(2):389–96. doi: 10.1189/jlb.0108018
44. Gabriel C, McMaster WR, Girard D, Descoteaux A. Leishmania donovani promastigotes evade the antimicrobial activity of neutrophil extracellular traps. J Immunol. (2010) 185(7):4319–27. doi: 10.4049/jimmunol.1000893
45. Carlsen ED, Hay C, Henard CA, Popov V, Garg NJ, Soong L. Leishmania amazonensis amastigotes trigger neutrophil activation but resist neutrophil microbicidal mechanisms. Infect Immun. (2013) 81(11):3966–74. doi: 10.1128/IAI.00770-13
46. Hurrell BP, Schuster S, Grün E, Coutaz M, Williams RA, Held W, et al. Rapid sequestration of Leishmania mexicana by neutrophils contributes to the development of chronic lesion. PloS Pathog. (2015) 11(5):e1004929. doi: 10.1371/journal.ppat.1004929
47. Linhares-Lacerda L, Temerozo JR, Ribeiro-Alves M, Azevedo EP, Mojoli A, Nascimento MTC, et al. Neutrophil extracellular trap-enriched supernatants carry microRNAs able to modulate TNF-α production by macrophages. Sci Rep. (2020) 10(1):2715. doi: 10.1038/s41598-020-59486-2
48. Wigerblad G, Kaplan MJ. Neutrophil extracellular traps in systemic autoimmune and autoinflammatory diseases. Nat Rev Immunol. (2023) 23:274–88. doi: 10.1038/s41577-022-00787-0
49. DeSouza-Vieira T. Metamorphosis of neutrophil transcriptional programme during Leishmania infection. Parasite Immunol. (2022) 44(6):e12922. doi: 10.1111/pim.12922
50. Lee SH, Charmoy M, Romano A, Paun A, Chaves MM, Cope FO, et al. Mannose receptor high, M2 dermal macrophages mediate nonhealing Leishmania major infection in a Th1 immune environment. J Exp Med. (2018) 215:357–75. doi: 10.1084/jem.20171389
51. Lee SH, Chaves MM, Kamenyeva O, Gazzinelli-Guimaraes PH, Kang B, Pessenda G, et al. M2-like, dermal macrophages are maintained via IL-4/CCL24-mediated cooperative interaction with eosinophils in cutaneous leishmaniasis. Sci Immunol. (2020) 5. doi: 10.1126/sciimmunol.aaz4415
52. Carneiro MB, Lopes ME, Hohman LS, Romano A, David BA, Kratofil R, et al. Th1-Th2 Cross-regulation controls early Leishmania infection in the skin by modulating the size of the permissive monocytic host cell reservoir. Cell Host Microbe. (2020) 27(5):752–68.e7. doi: 10.1016/j.chom.2020.03.011
53. Rossi M, Fasel N. The criminal association of Leishmania parasites and viruses. Curr Opin Microbiol. (2018) 46:65–72. doi: 10.1016/j.mib.2018.07.005
54. Zamboni DS, Sacks DL. Inflammasomes and Leishmania: in good times or bad, in sickness or in health. Curr Opin Microbiol. (2019) 52:70–6. doi: 10.1016/j.mib.2019.05.005
55. de Freitas Balanco JM, Moreira ME, Bonomo A, Bozza PT, Amarante-Mendes G, Pirmez C, et al. Apoptotic mimicry by an obligate intracellular parasite downregulates macrophage microbicidal activity. Curr Biol. (2001) 11(23):1870–3. doi: 10.1016/s0960-9822(01)00563-2
56. Mosmann TR, Cherwinski H, Bond MW, Giedlin MA, Coffman RL. Two types of murine helper T cell clone. I. Definition according to profiles of lymphokine activities and secreted proteins. J Immunol. (1986) 136(7):2348–57. doi: 10.4049/jimmunol.136.7.2348
57. Scott P, Natovitz P, Coffman RL, Pearce E, Sher A. Immunoregulation of cutaneous leishmaniasis. T cell lines that transfer protective immunity or exacerbation belong to different T helper subsets and respond to distinct parasite antigens. J Exp Med. (1988) 168(5):1675–84. doi: 10.1084/jem.168.5.1675
58. Silveira FT, Lainson R, De Castro Gomes CM, Laurenti MD, Corbett CE. Immunopathogenic competences of Leishmania (V.) braziliensis and L. (L.) amazonensis in American cutaneous leishmaniasis. Parasite Immunol. (2009) 31(8):423–31. doi: 10.1111/j.1365-3024.2009.01116.x
59. Gonzalez K, Calzada JE, Corbett CEP, Saldaña A, Laurenti MD. Involvement of the inflammasome and Th17 cells in skin lesions of human cutaneous Leishmaniasis caused by Leishmania (Viannia) panamensis. Mediators Inflamm. (2020) 2020:9278931. doi: 10.1155/2020/9278931
60. Stäger S, Rafati S. CD8(+) T cells in leishmania infections: friends or foes? Front Immunol. (2012) 3:5. doi: 10.3389/fimmu.2012.00005
61. Novais FO, Carvalho AM, Clark ML, Carvalho LP, Beiting DP, Brodsky IE, et al. CD8+ T cell cytotoxicity mediates pathology in the skin by inflammasome activation and IL-1β production. PloS Pathog. (2017) 13(2):e1006196. doi: 10.1371/journal.ppat.1006196
62. Cardoso TM, Lima JB, Bonyek-Silva Í, Nunes S, Feijó D, Almeida H, et al. Inflammasome activation by CD8+ T cells from patients with cutaneous Leishmaniasis caused by Leishmania braziliensis in the immunopathogenesis of the disease. J Invest Dermatol. (2021) 141(1):209–13.e2. doi: 10.1016/j.jid.2020.05.106
63. Cardoso TM, Machado Á, Costa DL, Carvalho LP, Queiroz A, et al. Protective and pathological functions of CD8+ T cells in Leishmania braziliensis infection. Infect Immun. (2015) 83(3):898–06. doi: 10.1128/IAI.02404-14
64. Titus RG, Ribeiro JM. Salivary gland lysates from the sand fly Lutzomyia longipalpis enhance Leishmania infectivity. Science. (1988) 239:1306–8. doi: 10.1126/science.3344436
65. Theodos CM, Titus RG. Salivary gland material from the sand fly Lutzomyia longipalpis has an inhibitory effect on macrophage function in vitro. Parasite Immunol. (1993) 15:481–7. doi: 10.1111/j.1365-3024.1993.tb00634.x
66. Belkaid Y, Kamhawi S, Modi G, Valenzuela J, Noben-Trauth N, Rowton E, et al. Development of a natural model of cutaneous leishmaniasis: powerful effects of vector saliva and saliva preexposure on the long-term outcome of Leishmania major infection in the mouse ear dermis. J Exp Med. (1998) 188:1941–53. doi: 10.1084/jem.188.10.1941
67. Mbow ML, Bleyenberg JA, Hall LR, Titus RG. Phlebotomus papatasi sand fly salivary gland lysate down-regulates a Th1, but up-regulates a Th2, response in mice infected with Leishmania major. J Immunol. (1998) 161:5571–7. doi: 10.4049/jimmunol.161.10.5571
68. Ehrchen JM, Roebrock K, Foell D, Nippe N, von Stebut E, Weiss JM, et al. Keratinocytes determine Th1 immunity during early experimental leishmaniasis. PloS Pathog. (2010) 6(4):e1000871. doi: 10.1371/journal.ppat.1000871
69. Teixeira CR, Santos CDS, Prates DB, Dos Santos RT, Araujo-Santos T, De Souza-Neto SM, et al. Lutzomyia longipalpis Saliva Drives Interleukin-17-Induced Neutrophil Recruitment Favoring Leishmania infantum Infection. Front Microbiol. (2018) 9:881. doi: 10.3389/fmicb.2018.00881
70. Teixeira CR, Teixeira MJ, Gomes RB, Santos CS, Andrade BB, Raffaele-Netto I, et al. Saliva from Lutzomyia longipalpis induces CC chemokine ligand 2/monocyte chemoattractant protein-1 expression and macrophage recruitment. J Immunol. (2005) 175:8346–53. doi: 10.4049/jimmunol.175.12.8346
71. Guimaraes-Costa AB, Shannon JP, Waclawiak I, Oliveira J, Meneses C, de Castro W, et al. A sand fly salivary protein acts as a neutrophil chemoattractant. Nat Commun. (2021) 12:3213. doi: 10.1038/s41467-021-23002-5
72. Morris RV, Shoemaker CB, David JR, Lanzaro GC, Titus RG. Sandfly maxadilan exacerbates infection with Leishmania major and vaccinating against it protects against L. major infection. J Immunol. (2001) 167:5226–30. doi: 10.4049/jimmunol.167.9.5226
73. Rogers KA, Titus RG. Immunomodulatory effects of Maxadilan and Phlebotomus papatasi sand fly salivary gland lysates on human primary in vitro immune responses. Parasite Immunol. (2003) 25:127–34. doi: 10.1046/j.1365-3024.2003.00623.x
74. Chagas AC, Oliveira F, Debrabant A, Valenzuela JG, Ribeiro JM, Calvo E. Lundep, a sand fly salivary endonuclease increases Leishmania parasite survival in neutrophils and inhibits XIIa contact activation in human plasma. PloS Pathog. (2014) 10:e1003923. doi: 10.1371/journal.ppat.1003923
75. Giraud E, Svobodova M, Muller I, Volf P, Rogers ME. Promastigote secretory gel from natural and unnatural sand fly vectors exacerbate Leishmania major and Leishmania tropica cutaneous leishmaniasis in mice. Parasitology. (2019) 146:1796–802. doi: 10.1017/S0031182019001069
76. Rogers ME, Ilg T, Nikolaev AV, Ferguson MA, Bates PA. Transmission of cutaneous leishmaniasis by sand flies is enhanced by regurgitation of fPPG. Nature. (2004) 430:463–7. doi: 10.1038/nature02675
77. Rogers ME, Corware K, Muller I, Bates PA. Leishmania infantum proteophosphoglycans regurgitated by the bite of its natural sand fly vector, Lutzomyia longipalpis, promote parasite establishment in mouse skin and skin-distant tissues. Microbes Infect. (2010) 12:875–9. doi: 10.1016/j.micinf.2010.05.014
78. Bates PA. Transmission of Leishmania metacyclic promastigotes by phlebotomine sand flies. Int J Parasitol. (2007) 37(10):1097–106. doi: 10.1016/j.ijpara.2007.04.003
79. Rogers M, Kropf P, Choi BS, Dillon R, Podinovskaia M, Bates P, et al. Proteophosophoglycans regurgitated by Leishmania-infected sand flies target the L-arginine metabolism of host macrophages to promote parasite survival. PloS Pathog. (2009) 5:e1000555. doi: 10.1371/journal.ppat.1000555
80. Giraud E, Lestinova T, Derrick T, Martin O, Dillon RJ, Volf P, et al. Leishmania proteophosphoglycans regurgitated from infected sand flies accelerate dermal wound repair and exacerbate leishmaniasis via insulin-like growth factor 1-dependent signalling. PloS Pathog. (2018) 14:e1006794. doi: 10.1371/journal.ppat.1006794
81. Atayde VD, Aslan H, Townsend S, Hassani K, Kamhawi S, Olivier M. Exosome secretion by the parasitic Protozoan leishmania within the sand fly midgut. Cell Rep. (2015) 13:957–67. doi: 10.1016/j.celrep.2015.09.058
82. Barbosa FMC, Dupin TV, Toledo MDS, Reis N, Ribeiro K, Cronemberger-Andrade A, et al. Extracellular vesicles released by Leishmania (Leishmania) amazonensis promote disease progression and induce the production of different cytokines in macrophages and B-1 cells. Front Microbiol. (2018) 9:3056. doi: 10.3389/fmicb.2018.03056
83. da Silva Lira Filho A, Fajardo EF, Chang KP, Clément P, Olivier M. Leishmania exosomes/extracellular vesicles containing GP63 are essential for enhance cutaneous leishmaniasis development upon co-inoculation of Leishmania amazonensis and its exosomes. Front Cell Infect Microbiol. (2022) 11:709258. doi: 10.3389/fcimb.2021.709258
84. Atayde VD, Da Silva Lira Filho A, Chaparro V, Zimmermann A, Martel C, Jaramillo M, et al. Exploitation of the Leishmania exosomal pathway by Leishmania RNA virus 1. Nat Microbiol. (2019) 4:714–23. doi: 10.1038/s41564-018-0352-y
85. Kelly PH, Bahr SM, Serafim TD, Ajami NJ, Petrosino JF, Meneses C, et al. The Gut Microbiome of the Vector Lutzomyia longipalpis Is Essential for Survival of Leishmania infantum. mBio. (2017) 8. doi: 10.1128/mBio.01121-16
86. Louradour I, Monteiro CC, Inbar E, Ghosh K, Merkhofer R, Lawyer P, et al. The midgut microbiota plays an essential role in sand fly vector competence for Leishmania major. Cell Microbiol. (2017) 19. doi: 10.1111/cmi.12755
87. Omondi ZN, Demir S, Arserim SK. Entomological survey of the sand fly fauna of Kayseri Province: focus on visceral and cutaneous leishmaniasis in Central Anatolia, Turkey. Turkiye Parazitol Derg. (2020) 44:158–63. doi: 10.4274/tpd
88. Metzemaekers M, Gouwy M, Proost P. Neutrophil chemoattractant receptors in health and disease: double-edged swords. Cell Mol Immunol. (2020) 17:433–50. doi: 10.1038/s41423-020-0412-0
89. Luz NF, Desouza-Vieira T, De Castro W, Vivarini AC, Pereira L, Franca RR, et al. Lutzomyia longipalpis Saliva Induces Heme Oxygenase-1 Expression at Bite Sites. Front Immunol. (2018) 9:2779. doi: 10.3389/fimmu.2018.02779
90. Desouza-Vieira T, Iniguez E, Serafim TD, De Castro W, Karmakar S, Disotuar MM, et al. Heme oxygenase-1 induction by blood-feeding arthropods controls skin inflammation and promotes disease tolerance. Cell Rep. (2020) 33:108317. doi: 10.1016/j.celrep.2020.108317
91. Kamhawi S, Belkaid Y, Modi G, Rowton E, Sacks D. Protection against cutaneous leishmaniasis resulting from bites of uninfected sand flies. Science. (2000) 290:1351–4. doi: 10.1126/science.290.5495.1351
92. Teixeira C, Gomes R, Oliveira F, Meneses C, Gilmore DC, Elnaiem DE, et al. Characterization of the early inflammatory infiltrate at the feeding site of infected sand flies in mice protected from vector-transmitted Leishmania major by exposure to uninfected bites. PloS Negl Trop Dis. (2014) 8:e2781. doi: 10.1371/journal.pntd.0002781
93. Gomes R, Teixeira C, Teixeira MJ, Oliveira F, Menezes MJ, Silva C, et al. Immunity to a salivary protein of a sand fly vector protects against the fatal outcome of visceral leishmaniasis in a hamster model. Proc Natl Acad Sci U.S.A. (2008) 105:7845–50. doi: 10.1073/pnas.0712153105
94. Oliveira F, Rowton E, Aslan H, Gomes R, Castrovinci PA, Alvarenga PH, et al. A sand fly salivary protein vaccine shows efficacy against vector-transmitted cutaneous leishmaniasis in nonhuman primates. Sci Transl Med. (2015) 7:290ra290. doi: 10.1126/scitranslmed.aaa3043
95. Gomes R, Oliveira F, Teixeira C, Meneses C, Gilmore DC, Elnaiem DE, et al. Immunity to sand fly salivary protein LJM11 modulates host response to vector-transmitted leishmania conferring ulcer-free protection. J Invest Dermatol. (2012) 132:2735–43. doi: 10.1038/jid.2012.205
96. Iniguez E, Saha S, Petrellis G, Menenses C, Herbert S, Gonzalez-Rangel Y, et al. A composite recombinant salivary proteins biomarker for Phlebotomus argentipes provides a surveillance tool postelimination of visceral Leishmaniasis in India. J Infect Dis. (2022) 226(10):1842–51. doi: 10.1093/infdis/jiac354
97. Belkaid Y, Valenzuela JG, Kamhawi S, Rowton E, Sacks DL, Ribeiro JM. Delayed-type hypersensitivity to Phlebotomus papatasi sand fly bite: An adaptive response induced by the fly? Proc Natl Acad Sci U.S.A. (2000) 97:6704–9. doi: 10.1073/pnas.97.12.6704
98. Vinhas V, Andrade BB, Paes F, Bomura A, Clarencio J, Miranda JC, et al. Human anti-saliva immune response following experimental exposure to the visceral leishmaniasis vector, Lutzomyia longipalpis. Eur J Immunol. (2007) 37:3111–21. doi: 10.1002/eji.200737431
99. Oliveira F, Traore B, Gomes R, Faye O, Gilmore DC, Keita S, et al. Delayed-type hypersensitivity to sand fly saliva in humans from a leishmaniasis-endemic area of Mali is Th1-mediated and persists to midlife. J Invest Dermatol. (2013) 133:452–9. doi: 10.1038/jid.2012.315
100. Carvalho AM, Viana SM, Andrade BB, Oliveira F, Valenzuela JG, Carvalho EM, et al. Immune response to LinB13, a lutzomyia intermedia salivary protein correlates with disease severity in tegumentary leishmaniasis. Clin Infect Dis. (2022) 75:1754–62. doi: 10.1093/cid/ciac258
101. Ashwin H, Sadlova J, Vojtkova B, et al. Characterization of a new Leishmania major strain for use in a controlled human infection model. Nat Commun. (2021) 12:215. doi: 10.1038/s41467-020-20569-3
102. Parkash V, Ashwin H, Sadlova J, Vojtkova B, Jones G, Martin N, et al. A clinical study to optimise a sand fly biting protocol for use in a controlled human infection model of cutaneous leishmaniasis (the FLYBITE study). Wellcome Open Res. (2021) 6:168. doi: 10.12688/wellcomeopenres
103. Wikel S. Ticks and tick-borne pathogens at the cutaneous interface: host defenses, tick countermeasures, and a suitable environment for pathogen establishment. Front Microbiol. (2013) 4:337. doi: 10.3389/fmicb.2013.00337
104. Simo L, Kazimirova M, Richardson J, Bonnet SI. The essential role of tick salivary glands and saliva in tick feeding and pathogen transmission. Front Cell Infect Microbiol. (2017) 7:281. doi: 10.3389/fcimb.2017.00281
105. Kemp DH, Stone BF, Binnington KC. Tick attachment and feeding: role of the mouthparts, feeding apparatus, salivary gland secretions, and the host response. In: Obenchain FD, Galun R, editors. Physiology of Ticks. (1982). (Amsterdam, The Netherlands: Elsevier) p. 119–68.
106. : Elsevier Sonenshine DE, Roe RM. Biology of Ticks. New York, NY: Oxford University Press (2014).
107. Bernard Q, Grillon A, Lenormand C, Ehret-Sabatier L, Boulanger N. Skin interface, a key player for borrelia multiplication and persistence in lyme borreliosis. Trends Parasitol. (2020) 36:304–14. doi: 10.1016/j.pt.2019.12.017
108. Zhang X, Zhang B, Masoudi A, Wang X, Xue X, Li M, et al. Comprehensive analysis of protein expression levels and phosphorylation levels in host skin in response to tick (Haemaphysalis longicornis) bite. J Proteomics. (2020) 226:103898. doi: 10.1016/j.jprot.2020.103898
109. Nuttall PA, Labuda M. Saliva-assisted transmission of tick-borne pathogens. In: Bowman AS, Nuttall PA, editors. Ticks: Biology, Disease and Control. Cambridge University Press, Cambridge, UK (2008). p. 205–19.
110. De La Fuente J, Estrada-Pena A, Venzal JM, Kocan KM, Sonenshine DE. Overview: Ticks as vectors of pathogens that cause disease in humans and animals. Front Biosci. (2008) 13:6938–46. doi: 10.2741/3200
111. Seinost G, Dykhuizen DE, Dattwyler RJ, Golde WT, Dunn JJ, Wang IN, et al. Four clones of Borrelia burgdorferi sensu stricto cause invasive infection in humans. Infect Immun. (1999) 67:3518–24. doi: 10.1128/IAI.67.7.3518-3524.1999
112. Cabezas-Cruz A, Hodžić A, Román-Carrasco P, Mateos-Hernández L, Duscher GG, Sinha DK, et al. Environmental and molecular drivers of the α-Gal syndrome. Front Immunol. (2019) 10:1210. doi: 10.3389/fimmu.2019.01210
113. Apostolovic D, Mihailovic J, Commins SP, Wijnveld M, Kazimirova M, Starkhammar M, et al. Allergenomics of the tick Ixodes ricinus reveals important α-Gal-carrying IgE-binding proteins in red meat allergy. Allergy. (2020) 75(1):217–20. doi: 10.1111/all.13978
114. Hashizume H, Fujiyama T, Umayahara T, Kageyama R, Walls AF, Satoh T. Repeated Amblyomma testudinarium tick bites are associated with increased galactose-α-1,3- galactose carbohydrate IgE antibody levels: A retrospective cohort study in a single institution. J Am Acad Dermatol. (2018) 78(6):1135–41.e3. doi: 10.1016/j.jaad.2017.12.028
115. Mitchell CL, Lin FC, Vaughn M, Apperson CS, Meshnick SR, Commins SP. Association between lone star tick bites and increased alpha-gal sensitization: evidence from a prospective cohort of outdoor workers. Parasit Vectors. (2020) 13(1):470. doi: 10.1186/s13071-020-04343-4
116. Des Vignes F, Piesman J, Heffernan R, Schulze TL, Stafford KC 3rd, Fish D. Effect of tick removal on transmission of Borrelia burgdorferi and Ehrlichia phagocytophila by Ixodes scapularis nymphs. J Infect Dis. (2001) 183:773–8. doi: 10.1086/318818
117. Zeidner NS, Schneider BS, Nuncio MS, Gern L, Piesman J. Coinoculation of Borrelia spp. with tick salivary gland lysate enhances spirochete load in mice and is tick species-specific. J Parasitol. (2002) 88:1276–8. doi: 10.1645/0022-3395(2002)088[1276:COBSWT]2.0.CO;2
118. Machackova M, Obornik M, Kopecky J. Effect of salivary gland extract from Ixodes ricinus ticks on the proliferation of Borrelia burgdorferi sensu stricto in vivo. Folia Parasitol (Praha). (2006) 53:153–8. doi: 10.14411/fp.2006.020
119. Horka H, Cerna-Kyckova K, Skallova A, Kopecky J. Tick saliva affects both proliferation and distribution of Borrelia burgdorferi spirochetes in mouse organs and increases transmission of spirochetes to ticks. Int J Med Microbiol. (2009) 299:373–80. doi: 10.1016/j.ijmm.2008.10.009
120. Rudolf I, Sikutova S, Kopecky J, Hubalek Z. Salivary gland extract from engorged Ixodes ricinus (Acari: Ixodidae) stimulates in vitro growth of Borrelia burgdorferi sensu lato. J Basic Microbiol. (2010) 50:294–8. doi: 10.1002/jobm.200900237
121. Skallova A, Iezzi G, Ampenberger F, Kopf M, Kopecky J. Tick saliva inhibits dendritic cell migration, maturation, and function while promoting development of Th2 responses. J Immunol. (2008) 180:6186–92. doi: 10.4049/jimmunol.180.9.6186
122. Zeidner NS, Schneider BS, Rutherford JS, Dolan MC. Suppression of Th2 cytokines reduces tick-transmitted Borrelia burgdorferi load in mice. J Parasitol. (2008) 94:767–9. doi: 10.1645/GE-1416.1
123. Vesely DL, Fish D, Shlomchik MJ, Kaplan DH, Bockenstedt LK. Langerhans cell deficiency impairs Ixodes scapularis suppression of Th1 responses in mice. Infect Immun. (2009) 77:1881–7. doi: 10.1128/IAI.00030-09
124. Narasimhan S, Sukumaran B, Bozdogan U, Thomas V, Liang X, Deponte K, et al. A tick antioxidant facilitates the Lyme disease agent’s successful migration from the mammalian host to the arthropod vector. Cell Host Microbe. (2007) 2:7–18. doi: 10.1016/j.chom.2007.06.001
125. Kern A, Schnell G, Bernard Q, Boeuf A, Jaulhac B, Collin E, et al. Heterogeneity of Borrelia burgdorferi sensu stricto population and its involvement in borrelia pathogenicity: study on murine model with specific emphasis on the skin interface. PloS One. (2015) 10:e0133195. doi: 10.1371/journal.pone.0133195
126. Sajiki Y, Konnai S, Ikenaka Y, Gulay KCM, Kobayashi A, Parizi LF, et al. Tick saliva-induced programmed death-1 and PD-ligand 1 and its related host immunosuppression. Sci Rep. (2021) 11:1063. doi: 10.1038/s41598-020-80251-y
127. Jin L, Jiang BG, Yin Y, Guo J, Jiang JF, Qi X, et al. Interference with LTβR signaling by tick saliva facilitates transmission of Lyme disease spirochetes. Proc Natl Acad Sci U S A. (2022) 119:e2208274119. doi: 10.1073/pnas.2208274119
128. Hayes BM, Radkov AD, Yarza F, Flores S, Kim J, Zhao Z, et al. Ticks resist skin commensals with immune factor of bacterial origin. Cell. (2020) 183:1562–1571 e1512. doi: 10.1016/j.cell.2020.10.042
129. Oliva Chávez AS, Wang X, Marnin L, Archer NK, Hammond HL, McClure Carroll EE, et al. Tick extracellular vesicles enable arthropod feeding and promote distinct outcomes of bacterial infection. Nat Commun. (2021) 12:3696. doi: 10.1038/s41467-021-23900-8
130. Zhou W, Tahir F, Wang JC, Woodson M, Sherman MB, Karim S, et al. Discovery of exosomes from tick saliva and salivary glands reveals therapeutic roles for CXCL12 and IL-8 in wound healing at the tick-human skin interface. Front Cell Dev Biol. (2020) 8:554. doi: 10.3389/fcell.2020.00554
131. Klyachko O, Stein BD, Grindle N, Clay K, Fuqua C. Localization and visualization of a coxiella-type symbiont within the lone star tick, Amblyomma americanum. Appl Environ Microbiol. (2007) 73:6584–94. doi: 10.1128/AEM.00537-07
132. Cull B, Burkhardt NY, Wang X-R, Thorpe CJ, Oliver JD, Kurtti TJ, et al. The Ixodes scapularis Symbiont Rickettsia buchneri Inhibits Growth of Pathogenic Rickettsiaceae in Tick Cells: Implications for Vector Competence. Front Vet Sci. (2021) 8:748427. doi: 10.3389/fvets.2021.748427
133. Oliver JD, Price LD, Burkhardt NY, Heu CC, Khoo BS, Thorpe CJ, et al. Growth Dynamics and Antibiotic Elimination of Symbiotic Rickettsia buchneri in the Tick Ixodes scapularis (Acari: Ixodidae). Appl Environ Microbiol. (2021) 87. doi: 10.1128/AEM.01672-20
134. Angelakis E, Mediannikov O, Jos S-L, Berenger J-M, Parola P, Raoult D. Candidatus Coxiella massiliensis infection. Emerg Infect Dis. (2016) 22:285–8. doi: 10.3201/eid2202.150106
135. Sonenshine DE, Stewart PE. Microbiomes of blood-feeding arthropods: genes coding for essential nutrients and relation to vector fitness and pathogenic infections. A Rev Microorg. (2021) 9. doi: 10.3390/microorganisms9122433
136. Wikel SK, Allen JR. Acquired resistance to ticks. iii. Cobra venom factor and the resistance response. Immunology. (1977) 32:457–65.
137. Brossard M, Fivaz V. Ixodes ricinus L.: mast cells, basophils and eosinophils in the sequence of cellular events in the skin of infested or re-infested rabbits. Parasitology. (1982) 85:583–92. doi: 10.1017/S0031182000056365
138. Wada T, Ishiwata K, Koseki H, Ishikura T, Ugajin T, Ohnuma N, et al. Selective ablation of basophils in mice reveals their nonredundant role in acquired immunity against ticks. J Clin Invest. (2010) 120:2867–75. doi: 10.1172/JCI42680
139. Ohta T, Yoshikawa S, Tabakawa Y, Yamaji K, Ishiwata K, Shitara H, et al. Skin CD4(+) memory T cells play an essential role in acquired anti-tick immunity through interleukin-3-mediated basophil recruitment to tick-feeding sites. Front Immunol. (2017) 8:1348. doi: 10.3389/fimmu.2017.01348
140. Tabakawa Y, Ohta T, Yoshikawa S, Robinson EJ, Yamaji K, Ishiwata K, et al. Histamine released from skin-infiltrating basophils but not mast cells is crucial for acquired tick resistance in mice. Front Immunol. (2018) 9:1540. doi: 10.3389/fimmu.2018.01540
141. Karasuyama H, Tabakawa Y, Ohta T, Wada T, Yoshikawa S. Crucial role for basophils in acquired protective immunity to tick infestation. Front Physiol. (2018) 9:1769. doi: 10.3389/fphys.2018.01769
142. Lynn GE, Diktas H, Deponte K, Fikrig E. Naturally Acquired Resistance to Ixodes scapularis Elicits Partial Immunity against Other Tick Vectors in a Laboratory Host. Am J Trop Med Hyg. (2021) 104:175–83. doi: 10.4269/ajtmh.20-0776
143. Anderson JM, Moore IN, Nagata BM, Ribeiro JMC, Valenzuela JG, Sonenshine DE. Ticks, Ixodes scapularis, Feed Repeatedly on White-Footed Mice despite Strong Inflammatory Response: An Expanding Paradigm for Understanding Tick-Host Interactions. Front Immunol. (2017) 8:1784. doi: 10.3389/fimmu.2017.01784
144. Burke G, Wikel SK, Spielman A, Telford SR, Mckay K, Krause PJ, et al. Hypersensitivity to ticks and Lyme disease risk. Emerg Infect Dis. (2005) 11:36–41. doi: 10.3201/eid1101.040303
145. Glatz M, Means T, Haas J, Steere AC, Mullegger RR. Characterization of the early local immune response to Ixodes ricinus tick bites in human skin. Exp Dermatol. (2017) 26:263–9. doi: 10.1111/exd.13207
146. Strobl J, Mündler V, Müller S, Gindl A, Berent S, Schötta AM, et al. Tick feeding modulates the human skin immune landscape to facilitate tick-borne pathogen transmission. J Clin Invest. (2022) 132:e161188. doi: 10.1172/JCI161188
147. Mihara M. A histopathologic study of the human skin in the early stage after a tick bite: a special reference to cutaneous tissue reaction to the cement substance of tick saliva. Yonago Acta Med. (2017) 60:186–99. doi: 10.33160/yam.2017.09.009
148. Apostolovic D, Grundström J, Kiewiet MBG, Perusko M, Hamsten C, Starkhammar M, et al. Th2-skewed T cells correlate with B cell response to α-Gal and tick antigens in α-Gal syndrome. J Clin Invest. (2023) 133(6):e158357. doi: 10.1172/JCI158357
149. Huang YS, Higgs S, Vanlandingham DL. Emergence and re-emergence of mosquito-borne arboviruses. Curr Opin Virol. (2019) 34:104–9. doi: 10.1016/j.coviro.2019.01.001
150. Stanaway JD, Shepard DS, Undurraga EA, Halasa YA, Coffeng LE, Brady OJ, et al. The global burden of dengue: an analysis from the Global Burden of Disease Study 2013. Lancet Infect Dis. (2016) 16:712–23. doi: 10.1016/S1473-3099(16)00026-8
151. Weaver SC, Reisen WK. Present and future arboviral threats. Antiviral Res. (2010) 85:328–45. doi: 10.1016/j.antiviral.2009.10.008
152. Kamal M, Kenawy MA, Rady MH, Khaled AS, Samy AM. Mapping the global potential distributions of two arboviral vectors Aedes aEgypti and Ae. albopictus under changing climate. PloS One. (2018) 13:e0210122. doi: 10.1371/journal.pone.0210122
153. Powell JR. Mosquito-borne human viral diseases: why Aedes aEgypti? Am J Trop Med Hyg. (2018) 98:1563–5. doi: 10.4269/ajtmh.17-0866
154. Ribeiro JM. Role of saliva in blood-feeding by arthropods. Annu Rev Entomol. (1987) 32:463–78. doi: 10.1146/annurev.en.32.010187.002335
155. Ribeiro JM, Francischetti IM. Role of arthropod saliva in blood feeding: sialome and post-sialome perspectives. Annu Rev Entomol. (2003) 48:73–88. doi: 10.1146/annurev.ento.48.060402.102812
156. Edwards JF, Higgs S, Beaty BJ. Mosquito feeding-induced enhancement of Cache Valley Virus (Bunyaviridae) infection in mice. J Med Entomol. (1998) 35:261–5. doi: 10.1093/jmedent/35.3.261
157. Le Coupanec A, Babin D, Fiette L, Jouvion G, Ave P, Misse D, et al. Aedes mosquito saliva modulates Rift Valley fever virus pathogenicity. PloS Negl Trop Dis. (2013) 7:e2237. doi: 10.1371/journal.pntd.0002237
158. Christofferson RC, Mccracken MK, Johnson AM, Chisenhall DM, Mores CN. Development of a transmission model for dengue virus. Virol J. (2013) 10:127. doi: 10.1186/1743-422X-10-127
159. Mccracken MK, Christofferson RC, Chisenhall DM, Mores CN. Analysis of early dengue virus infection in mice as modulated by Aedes aEgypti probing. J Virol. (2014) 88:1881–9. doi: 10.1128/JVI.01218-13
160. Pingen M, Bryden SR, Pondeville E, Schnettler E, Kohl A, Merits A, et al. Host inflammatory response to mosquito bites enhances the severity of arbovirus infection. Immunity. (2016) 44:1455–69. doi: 10.1016/j.immuni.2016.06.002
161. Agarwal A, Joshi G, Nagar DP, Sharma AK, Sukumaran D, Pant SC, et al. Mosquito saliva induced cutaneous events augment Chikungunya virus replication and disease progression. Infect Genet Evol. (2016) 40:126–35. doi: 10.1016/j.meegid.2016.02.033
162. Turell MJ, Tammariello RF, Spielman A. Nonvascular delivery of St. Louis encephalitis and Venezuelan equine encephalitis viruses by infected mosquitoes (Diptera: Culicidae) feeding on a vertebrate host. J Med Entomol. (1995) 32:563–8. doi: 10.1093/jmedent/32.4.563
163. Schmid MA, Glasner DR, Shah S, Michlmayr D, Kramer LD, Harris E. Mosquito saliva increases endothelial permeability in the skin, immune cell migration, and dengue pathogenesis during antibody-dependent enhancement. PloS Pathog. (2016) 12:e1005676. doi: 10.1371/journal.ppat.1005676
164. Cox J, Mota J, Sukupolvi-Petty S, Diamond MS, Rico-Hesse R. Mosquito bite delivery of dengue virus enhances immunogenicity and pathogenesis in humanized mice. J Virol. (2012) 86:7637–49. doi: 10.1128/JVI.00534-12
165. Dudley DM, Newman CM, Lalli J, Stewart LM, Koenig MR, Weiler AM, et al. Infection via mosquito bite alters Zika virus tissue tropism and replication kinetics in rhesus macaques. Nat Commun. (2017) 8:2096. doi: 10.1038/s41467-017-02222-8
166. Mccracken MK, Gromowski GD, Garver LS, Goupil BA, Walker KD, Friberg H, et al. Route of inoculation and mosquito vector exposure modulate dengue virus replication kinetics and immune responses in rhesus macaques. PloS Negl Trop Dis. (2020) 14:e0008191. doi: 10.1371/journal.pntd.0008191
167. Schneider BS, Soong L, Zeidner NS, Higgs S. Aedes aEgypti salivary gland extracts modulate anti-viral and TH1/TH2 cytokine responses to sindbis virus infection. Viral Immunol. (2004) 17:565–73. doi: 10.1089/vim.2004.17.565
168. Henrique MO, Neto LS, Assis JB, Barros MS, Capurro ML, Lepique AP, et al. Evaluation of inflammatory skin infiltrate following Aedes aEgypti bites in sensitized and non-sensitized mice reveals saliva-dependent and immune-dependent phenotypes. Immunology. (2019) 158:47–59. doi: 10.1111/imm.13096
169. Conway MJ, Watson AM, Colpitts TM, Dragovic SM, Li Z, Wang P, et al. Mosquito saliva serine protease enhances dissemination of dengue virus into the mammalian host. J Virol. (2014) 88:164–75. doi: 10.1128/JVI.02235-13
170. Thangamani S, Higgs S, Ziegler S, Vanlandingham D, Tesh R, Wikel S. Host immune response to mosquito-transmitted chikungunya virus differs from that elicited by needle inoculated virus. PloS One. (2010) 5:e12137. doi: 10.1371/journal.pone.0012137
171. Hastings AK, Uraki R, Gaitsch H, Dhaliwal K, Stanley S, Sproch H, et al. Aedes aEgypti NeSt1 protein enhances zika virus pathogenesis by activating neutrophils. J Virol. (2019) 93. doi: 10.1128/JVI.00395-19
172. Uraki R, Hastings AK, Marin-Lopez A, Sumida T, Takahashi T, Grover JR, et al. Aedes aEgypti AgBR1 antibodies modulate early Zika virus infection of mice. Nat Microbiol. (2019) 4:948–55. doi: 10.1038/s41564-019-0385-x
173. Marin-Lopez A, Wang Y, Jiang J, Ledizet M, Fikrig E. AgBR1 and NeSt1 antisera protect mice from Aedes aEgypti-borne Zika infection. Vaccine. (2021) 39:1675–9. doi: 10.1016/j.vaccine.2021.01.072
174. Wang Z, Nie K, Liang Y, Niu J, Yu X, Zhang O, et al. A mosquito salivary protein-driven influx of myeloid cells facilitates flavivirus transmission. EMBO J. (2024). doi: 10.1038/s44318-024-00056-x
175. Jin L, Guo X, Shen C, Hao X, Sun P, Li P, et al. Salivary factor LTRIN from Aedes aEgypti facilitates the transmission of Zika virus by interfering with the lymphotoxin-beta receptor. Nat Immunol. (2018) 19:342–53. doi: 10.1038/s41590-018-0063-9
176. Sun P, Nie K, Zhu Y, Liu Y, Wu P, Liu Z, et al. A mosquito salivary protein promotes flavivirus transmission by activation of autophagy. Nat Commun. (2020) 11:260. doi: 10.1038/s41467-019-14115-z
177. Sri-In C, Weng SC, Chen WY, Wu-Hsieh BA, Tu WC, Shiao SH. A salivary protein of Aedes aEgypti promotes dengue-2 virus replication and transmission. Insect Biochem Mol Biol. (2019) 111:103181. doi: 10.1016/j.ibmb.2019.103181
178. Martin-Martin I, Valenzuela Leon PC, Amo L, Shrivastava G, Iniguez E, Aryan A, et al. Aedes aegypti sialokinin facilitates mosquito blood feeding and modulates host immunity and vascular biology. Cell Rep. (2022) 39(2):110648. doi: 10.1016/j.celrep.2022.110648
180. Heilesen B. Studies on mosquito bites. Acta Allergol. (1949) 2:245–67. doi: 10.1111/j.1398-9995.1949.tb03310.x
181. Goldman L, Rockwell E, Richfield DF 3rd. Histopathological studies on cutaneous reactions to the bites of various arthropods. Am J Trop Med Hyg. (1952) 1:514–25. doi: 10.4269/ajtmh.1952.1.514
182. Rockwell EM, Johnson P. The insect bite reaction. II. Evaluation of the allergic reaction. J Invest Dermatol. (1952) 19:137–55. doi: 10.1038/jid.1952.78
183. Killby VA, Silverman PH. Hypersensitive reactions in man to specific mosquito bites. Am J Trop Med Hyg. (1967) 16:374–80. doi: 10.4269/ajtmh.1967.16.374
184. Guerrero D, Vo HTM, Lon C, Bohl JA, Nhik S, Chea S, et al. Evaluation of cutaneous immune response in a controlled human in vivo model of mosquito bites. Nat Commun. (2022) 13:7036. doi: 10.1038/s41467-022-34534-9
185. Peng Z, Simons FE. Comparison of proteins, IgE, and IgG binding antigens, and skin reactivity in commercial and laboratory-made mosquito extracts. Ann Allergy Asthma Immunol. (1996) 77:371–6. doi: 10.1016/S1081-1206(10)63335-2
186. Chen YL, Simons FE, Peng Z. A mouse model of mosquito allergy for study of antigen-specific IgE and IgG subclass responses, lymphocyte proliferation, and IL-4 and IFN-gamma production. Int Arch Allergy Immunol. (1998) 116:269–77. doi: 10.1159/000023955
187. Peng Z, Simons FE. Advances in mosquito allergy. Curr Opin Allergy Clin Immunol. (2007) 7:350–4. doi: 10.1097/ACI.0b013e328259c313
188. Cantillo JF, Fernandez-Caldas E, Puerta L. Immunological aspects of the immune response induced by mosquito allergens. Int Arch Allergy Immunol. (2014) 165:271–82. doi: 10.1159/000371349
189. Vogt MB, Lahon A, Arya RP, Kneubehl AR, Spencer Clinton JL, Paust S, et al. Mosquito saliva alone has profound effects on the human immune system. PloS Negl Trop Dis. (2018) 12:e0006439. doi: 10.1371/journal.pntd.0006439
190. World Health Organization. World Malaria Report 2023. (2023). Available at: https://www.who.int/teams/global-malaria-programme/reports/world-malaria-report-2023.
191. Vaughan JA, Scheller LF, Wirtz RA, Azad AF. Infectivity of Plasmodium berghei sporozoites delivered by intravenous inoculation versus mosquito bite: implications for sporozoite vaccine trials. Infect Immun. (1999) 67:4285–9. doi: 10.1128/IAI.67.8.4285-4289.1999
192. Fonseca L, Seixas E, Butcher G, Langhorne J. Cytokine responses of CD4+ T cells during a Plasmodium chabaudi chabaudi (ER) blood-stage infection in mice initiated by the natural route of infection. Malar J. (2007) 6:77. doi: 10.1186/1475-2875-6-77
193. Kebaier C, Voza T, Vanderberg J. Neither mosquito saliva nor immunity to saliva has a detectable effect on the infectivity of Plasmodium sporozoites injected into mice. Infect Immun. (2010) 78:545–51. doi: 10.1128/IAI.00807-09
194. Schneider BS, Mathieu C, Peronet R, Mecheri S. Anopheles stephensi saliva enhances progression of cerebral malaria in a murine model. Vector Borne Zoonotic Dis. (2011) 11:423–32. doi: 10.1089/vbz.2010.0120
195. Donovan MJ, Messmore AS, Scrafford DA, Sacks DL, Kamhawi S, Mcdowell MA. Uninfected mosquito bites confer protection against infection with malaria parasites. Infect Immun. (2007) 75:2523–30. doi: 10.1128/IAI.01928-06
196. Demeure CE, Brahimi K, Hacini F, Marchand F, Peronet R, Huerre M, et al. Anopheles mosquito bites activate cutaneous mast cells leading to a local inflammatory response and lymph node hyperplasia. J Immunol. (2005) 174:3932–40. doi: 10.4049/jimmunol.174.7.3932
197. Mac-Daniel L, Buckwalter MR, Berthet M, Virk Y, Yui K, Albert ML, et al. Local immune response to injection of Plasmodium sporozoites into the skin. J Immunol. (2014) 193:1246–57. doi: 10.4049/jimmunol.1302669
198. Owhashi M, Harada M, Suguri S, Ohmae H, Ishii A. The role of saliva of Anopheles stephensi in inflammatory response: identification of a high molecular weight neutrophil chemotactic factor. Parasitol Res. (2001) 87:376–82. doi: 10.1007/s004360000355
199. Waisberg M, Molina-Cruz A, Mizurini DM, Gera N, Sousa BC, Ma D, et al. Plasmodium falciparum infection induces expression of a mosquito salivary protein (Agaphelin) that targets neutrophil function and inhibits thrombosis without impairing hemostasis. PloS Pathog. (2014) 10:e1004338. doi: 10.1371/journal.ppat.1004338
200. Chuang YM, Agunbiade TA, Tang XD, Freudzon M, Almeras L, Fikrig E. The effects of A mosquito salivary protein on sporozoite traversal of host cells. J Infect Dis. (2021) 224:544–53. doi: 10.1093/infdis/jiaa759
201. Dragovic SM, Agunbiade TA, Freudzon M, Yang J, Hastings AK, Schleicher TR, et al. Immunization with AgTRIO, a protein in anopheles saliva, contributes to protection against plasmodium infection in mice. Cell Host Microbe. (2018) 23:523–535 e525. doi: 10.1016/j.chom.2018.03.008
202. Chuang YM, Tang XD, Fikrig E. A mosquito AgTRIO monoclonal antibody reduces early plasmodium infection of mice. Infect Immun. (2022) 90:e0035921. doi: 10.1128/IAI.00359-21
203. Chuang YM, Alameh MG, Abouneameh S, Raduwan H, Ledizet M, Weissman D, et al. A mosquito AgTRIO mRNA vaccine contributes to immunity against malaria. NPJ Vaccines. (2023) 8:88. doi: 10.1038/s41541-023-00679-x
204. Arora G, Sajid A, Chuang YM, Dong Y, Gupta A, Gambardella K, et al. Immunomodulation by mosquito salivary protein AgSAP contributes to early host infection by plasmodium. mBio. (2021) 12:e0309121. doi: 10.1128/mBio.03091-21
205. Schleicher TR, Yang J, Freudzon M, Rembisz A, Craft S, Hamilton M, et al. A mosquito salivary gland protein partially inhibits Plasmodium sporozoite cell traversal and transmission. Nat Commun. (2018) 9:2908. doi: 10.1038/s41467-018-05374-3
206. Shi H, Yu X, Cheng G. Impact of the microbiome on mosquito-borne diseases. Protein Cell. (2023) 14:743–61. doi: 10.1093/procel/pwad021
207. Angleró-Rodríguez YI, Talyuli OAC, Blumberg BJ, Kang S, Demby C, Shields A, et al. An Aedes aEgypti-associated fungus increases susceptibility to dengue virus by modulating gut trypsin activity. Elife. (2017) 6. doi: 10.7554/eLife.28844
208. Kozlova EV, Hegde S, Roundy CM, Golovko G, Saldaña MA, Hart CE, et al. Microbial interactions in the mosquito gut determine Serratia colonization and blood-feeding propensity. ISME J. (2021) 15:93–108. doi: 10.1038/s41396-020-00763-3
209. Gao H, Jiang Y, Wang L, Wang G, Hu W, Dong L, et al. Outer membrane vesicles from a mosquito commensal mediate targeted killing of Plasmodium parasites via the phosphatidylcholine scavenging pathway. Nat Commun. (2023) 14(1):5157. doi: 10.1038/s41467-023-40887-6
210. Gomes FM, Barillas-Mury C. Infection of anopheline mosquitoes with Wolbachia: Implications for malaria control. PloS Pathog. (2018) 14:e1007333. doi: 10.1371/journal.ppat.1007333
211. Hoffmann AA, Ahmad NW, Keong WM, Ling CY, Ahmad NA, Golding N, et al. Introduction of Aedes aEgypti mosquitoes carrying wAlbB Wolbachia sharply decreases dengue incidence in disease hotspots. iScience. (2024) 27:108942. doi: 10.1016/j.isci.2024.108942
212. Das De T, Sharma P, Tevatiya S, Chauhan C, Kumari S, Yadav P, et al. Bidirectional microbiome-gut-brain-axis communication influences metabolic switch-associated responses in the mosquito Anopheles culicifacies. Cells. (2022) 11. doi: 10.3390/cells11111798
213. Lee JH, Kim HW, Mustafa B, Lee H, Kwon HW. The relationships between microbiome diversity and epidemiology in domestic species of malaria-mediated mosquitoes of Korea. Sci Rep. (2023) 13. doi: 10.1038/s41598-023-35641-3
214. Cansado-Utrilla C, Zhao SY, McCall PJ, Coon KL, Hughes GL. The microbiome and mosquito vectorial capacity: rich potential for discovery and translation. Microbiome. (2021) 9. doi: 10.1186/s40168-021-01073-2
215. Friedman-Klabanoff DJ, Laurens MB, Berry AA, Travassos MA, Adams M, Strauss KA, et al. The controlled human malaria infection experience at the University of Maryland. Am J Trop Med Hyg. (2019) 100:556–65. doi: 10.4269/ajtmh.18-0476
216. RTS,S Clinical Trials Partnership. Efficacy and safety of RTS,S/AS01 malaria vaccine with or without a booster dose in infants and children in Africa: final results of a phase 3, individually randomised, controlled trial. Lancet. (2015) 386:31–45. doi: 10.1016/S0140-6736(15)60721-8
217. Datoo MS, Natama HM, Somé A, Bellamy D, Traoré O, Rouamba T, et al. Efficacy and immunogenicity of R21/Matrix-M vaccine against clinical malaria after 2 years’ follow-up in children in Burkina Faso: a phase 1/2b randomised controlled trial. Lancet Infect Dis. (2022) 22:1728–36. doi: 10.1016/S1473-3099(22)00442-X
218. Collins KA, Snaith R, Cottingham MG, Gilbert SC, Hill AVS. Enhancing protective immunity to malaria with a highly immunogenic virus-like particle vaccine. Sci Rep. (2017) 7:46621. doi: 10.1038/srep46621
219. Manning JE, Oliveira F, Coutinho-Abreu IV, Herbert S, Meneses C, Kamhawi S, et al. Safety and immunogenicity of a mosquito saliva peptide-based vaccine: a randomised, placebo-controlled, double-blind, phase 1 trial. Lancet. (2020) 395:1998–2007. doi: 10.1016/S0140-6736(20)31048-5
220. Friedman-Klabanoff DJ, Birkhold M, Short MT, Wilson TR, Meneses CR, Lacsina JR, et al. Safety and immunogenicity of AGS-v PLUS, a mosquito saliva peptide vaccine against arboviral diseases: A randomized, double-blind, placebo-controlled Phase 1 trial. EBioMedicine. (2022) 86:104375. doi: 10.1016/j.ebiom.2022.104375
Keywords: skin immune defense, sand flies, mosquitoes, ticks, vector saliva, vector-borne disease, pathogen transmission
Citation: Lacsina JR, Kissinger R, Doehl JSP, Disotuar MM, Petrellis G, Short M, Lowe E, Oristian J, Sonenshine D and DeSouza-Vieira T (2024) Host skin immunity to arthropod vector bites: from mice to humans. Front. Trop. Dis 5:1308585. doi: 10.3389/fitd.2024.1308585
Received: 06 October 2023; Accepted: 08 April 2024;
Published: 01 May 2024.
Edited by:
Maria Fernanda Yasnot, University of Córdoba, ColombiaReviewed by:
Michail Kotsyfakis, Foundation for Research and Technology Hellas (FORTH), GreeceCopyright © 2024 Lacsina, Kissinger, Doehl, Disotuar, Petrellis, Short, Lowe, Oristian, Sonenshine and DeSouza-Vieira. This is an open-access article distributed under the terms of the Creative Commons Attribution License (CC BY). The use, distribution or reproduction in other forums is permitted, provided the original author(s) and the copyright owner(s) are credited and that the original publication in this journal is cited, in accordance with accepted academic practice. No use, distribution or reproduction is permitted which does not comply with these terms.
*Correspondence: Thiago DeSouza-Vieira, dGRlc291emEtdmllaXJhQGJpb2YudWZyai5icg==; dmllaXJhLnRzc3ZAZ21haWwuY29t
†These authors have contributed equally to this work
Disclaimer: All claims expressed in this article are solely those of the authors and do not necessarily represent those of their affiliated organizations, or those of the publisher, the editors and the reviewers. Any product that may be evaluated in this article or claim that may be made by its manufacturer is not guaranteed or endorsed by the publisher.
Research integrity at Frontiers
Learn more about the work of our research integrity team to safeguard the quality of each article we publish.