- 1Instituto de Biología Experimental (IBE), Facultad de Ciencias, Instituto de Medicina Tropical (IMT), Facultad de Medicina, Universidad Central de Venezuela (UCV), Caracas, Venezuela
- 2Unidad de Química Medicinal, Facultad de Farmacia, Universidad Central de Venezuela (UCV), Caracas, Venezuela
- 3Laboratorio de Fisiología Molecular, Facultad de Medicina, Universidad Central de Venezuela (UCV), Caracas, Venezuela
Leishmaniasis is a complex tropical disease caused by the protozoan parasite Leishmania spp. Classical chemotherapy includes pentavalent antimonial; however, pentamidine, amphotericin B, and miltefosine have been used. Chemo-resistance remains a risk for successful treatment; thus, target identification and development of selective drugs remain a priority in controlling this disease. Evidence indicates that 6-phosphogluconate dehydrogenase (6PGDH), β-tubulin protein, and ATP-dependent transporters (ABCs-T) are potential targets to be addressed. The pentose phosphate pathway key enzyme 6PGDH is essential for protecting kinetoplastid parasites from oxidative stress and differs from the mammalian host enzyme (<35% AA sequence identity). An optimized 3D model has been used to select high -affinity compounds toward the enzyme through virtual screening and subsequent evaluation in vivo. In kinetoplasts, tubulins are highly conserved proteins essential for microtubule formation. However, compared to other eukaryotic cells, there is a differential susceptibility of kinetoplastid proteins to antimicrotubular agents, e.g., colchicine resistance. A comparison of experimental models between bovine and Leishmania β-tubulin protein allowed us to identify structural modification products of various amino acid substitutions, which hinder the access of colchicine to the binding pocket of the Leishmania protein. Similar changes are found in the β-tubulin sequence of other kinetoplastids such as Trypanosoma cruzi, T. brucei, and T. evansi. The evaluation of the β-tubulin protein as a therapeutic target and the compounds that selectively interact with it was carried out using in silico approaches. The activities of ABC-Transporters are related to the main causes of drug resistance, and the collected evidence suggests that for the ABC-Transporter blocker glibenclamide, there is a: (1) differential susceptibility of Leishmania spp. vs. macrophages; (2) greater susceptibility of axenic amastigotes vs. promastigotes; and (3) glibenclamide-glucantime synergistic drug interaction in macrophage-infected cells. Herein, we discuss the potential value of designing ABC-Transporter blockers for combination therapy in the treatment of leishmaniasis. The examples mentioned above highlight the importance of the search for new therapeutic targets and pharmacophores for the design of alternative treatments for the disease.
1 Introduction
As for many if not all tropical diseases, the concept of having robust chemotherapy constitutes a fundamental portion of strategies for disease control (1). To achieve this challenge, the main questions that arise include: which target should we choose to exploit its capabilities that might end up in designing and producing a compound that once transformed into a medicament would be efficient in treating the disease? What is the patient population to which we would deliver that medicine? Finally, would treatment failure (TF) and drug resistance (DR) be major risk factors that would challenge the success of the medicament and would end up in (re) emergence and spreading of the disease, in our case leishmaniasis, worldwide? Thus, a fundamental challenge to be solved is the protection of the few existing drugs against DR, and the same is true for those being designed.
Leishmaniasis, the disease we are addressing through this review, is a multifaceted neglected tropical disease of high impact caused by species of the protozoan parasite Leishmania spp. There is no effective vaccine against leishmaniasis (2); the treatment of leishmaniasis is based on chemotherapy through the administration of pentavalent antimonials, such as Glucantime® and Pentostan™, despite the fact that their mechanism of action is still practically unknown; likewise, their toxicity and low efficacy have been demonstrated. Alternative drugs include amphotericin B, pentamidine, aminoglycoside paramomycin, and miltefosine (MIL), and the latter is the first oral treatment used for visceral leishmaniasis (VL). Unfortunately, MIL has a huge limitation for its use in fertile women due to its demonstrated teratogenicity. Although potential therapeutic treatment options relate to drug combinations, their effectiveness has only been demonstrated in experimental animals (3–10).
Antimonials (SSG) have been used for many decades but have been stopped in the Indian subcontinent due to the massive emergence of DR against it (11). Indeed, chemo-resistance against pentavalent antimonial signifies a very serious challenge in the control of VL (12, 13). MIL has become a first-line drug in the Indian subcontinent, replacing SSG. However, 10 years of use have been enough to decrease its efficacy significantly; fortunately, it is not yet completely associated with MIL-DR. Knowledge of SSG-DR and mechanisms of experimental MIL-DR that have been already analyzed may help to better understand the mechanism behind it although this will take us to the initial point with massive SSG-DR. In both cases, DR can be countered by combination therapy.
Thus, the study of DR must be part of drug discovery pipelines and must be included in drug discovery projects to learn about the efficiency of novel compounds on sets of strains including recent clinical isolates with DR and to further optimize potential compounds and should be monitored in clinical trials. It is important to mention that ongoing clinical trials guided by the Drug for Neglected Diseases Initiative (DNDi) have incorporated assays for all types of leishmaniasis including those potentially related to DR emergence (14, 15).
An additional point to be addressed is the wide spread of the disease in tropical areas and how the global warming is spreading it even more to non-tropical areas of the world. A deprived Public Health approach to these issues contributes to the ecologically inadequate disposition of used medicines “invading nature” and resulting in the slow but constant enhancement of a large reservoir of resistance genes both in the human microflora and in the environment. The inevitable consequence is the potential donation and transfer of resistance genes to human pathogens by horizontal gene transfer (16). The effect that horizontal gene transfer has on gene acquisition in Leishmania is not well defined; however, the potential relevance this mechanism might have for the emergence of DR for chemotherapy against this parasite cannot be discarded.
Therefore, the identification of the mechanisms directly involved in the evolution and emergence of DR in Leishmania, as well as the size and spread of natural chemo-resistant species, seems to be a must. This also goes for the description of the changes that occur at the physiological level in Leishmania as a consequence of, or concomitantly with, the development of DR. Such changes contribute to the overall characteristics of the chemo-resistant phenotype determined by such modifications, and their comprehension could be a determinant for the design of new chemotherapeutic strategies against leishmaniasis (17).
For a cell to be alive, in our case Leishmania, it has to survive and reproduce. In the case of parasites, the cells must additionally develop strategies to undermine treatments (16, 17). These strategies include mechanisms to extrude drugs from the cell, the use of microenvironment places to hide, and genetic switches that permit the selection of clones that survive through natural selection under drug pressure (16, 17). Infection with a resistant strain constitutes primary resistance, and drug pressure may produce acquired resistance in a host infected with a susceptible strain. But primary resistance is not a mere replication of acquired resistance. Altered virulence or resistance-inherent instability are also factors that may differentiate primary and acquired resistance (10, 18). Thus, the efficacy of drugs used for the treatment of diseases, including leishmaniasis, may differ as a result of the presence of a resistant strain or a consequence of the poor immune status of the patient; it might also be due to pharmacokinetic properties affecting clearance of the drug and natural variances in drug sensitivities of Leishmania species (19).
This means that the search for new targets and alternatives for treating the disease remains a priority. One alternative that has been considered to address this important health problem might be the use of State-of-the-Art technologies previously used in the development and rational design of drugs, i.e., the so-called, “omic” tools and Artificial Intelligence among others (20–22). The final aim is to improve the development of compounds that can end up being useful drugs for enhancing the diversity of medicaments used for the treatment of leishmaniasis.
Last but not least, the strong potential of three-dimensional (3D) modeling and molecular dynamics (MD) studies for the structural prediction of proteins has been of great importance in the identification of novel therapeutic targets. These tools have allowed for the establishment of comparative studies between crystal structures of various proteins and those described in different organisms whose X-ray structures are unknown. This review focuses on discussing the evaluation of diverse molecules, like the metabolic enzyme 6-phosphogluconate dehydrogenase (6PGDH; EC 1.1.1.44) and the structural protein β-tubulin from different kinetoplastids, as potential drug targets. Likewise, we elaborate on the potential value of membrane transporters like the ABC-Transporters (ABC-T) and their blockers as useful tools to be used in CT for the treatment of leishmaniasis.
2 Potential drug targets in kinetoplastids
Different studies indicate the crucial importance of metabolism throughout the life cycle of the parasite. Thus, many drugs have been evaluated against potential targets belonging to pathways such as the glycolytic and/or pentose phosphate pathways (8, 22–26). For example, the enzyme 6PGDH of the oxidative branch of the pentose phosphate pathway plays a central role since by its action, kinetoplastids gain reducing power in the form of NADP+ and generate ribose-5-phosphate (ribose-5P), which is fundamental for subsequent nucleotide biosynthesis. NADPH produced by 6PGD is crucial for the balance of oxygen-reactive species and for maintaining the redox potential necessary for protection against oxidative stress (25, 26). Several studies have been addressed at the molecular, structural, and biochemical level for 6PGDH in parasitic trypanosomatids; the findings suggest significant differences among Leishmania (Leishmania) mexicana, Trypanosoma brucei, and T. cruzi enzymes, also when comparing it with the human homologous enzyme (27–30).
2.1 The 6PGDH of different kinetoplastids
Of particular interest has been the enzyme 6PGDH where different studies have been addressed at the molecular, structural, and biochemical levels in trypanosomatid. The findings suggest significant differences among Leishmania (Leishmania) mexicana (Lm6PGDH), Trypanosoma brucei (Tb6PGDH), and T. cruzi (Tc6PGDH) enzymes, when comparing it with the human homologous enzyme (Hs6PGDH); such differences are derived from modifications in particular residues that affect the structural conformation of the active site cavity (27–30). Based on its central role in the metabolism of kinetoplastids, we have attempted to characterize the Lm6PGDH as a selective drug target in these organisms by the use of three-dimensional (3D) homology modeling and MD studies for the structural prediction of proteins.
2.1.1 Molecular modelling of the Lm6PGDH and Tc6PGDH
Although the enzyme Tb6PGDH has been characterized and its crystal structure has been reported (31, 32), the enzymes Lm6PGDH and Tc6PGDH seem to be unstable, and the conditions to obtain their crystal structure have not been possible to define; however, such structure, in complex with NADP(H), has recently been obtained for L. donovani (Ld6PGD) enzyme (33).
The genes for Lm6PGDH and Tc6PGDH enzymes have been identified and sequenced, and the 3D structure of the corresponding proteins was generated through the SWISS- MODEL modeling server (34), using the crystalline structure of the T. brucei enzyme as a template (Protein Databank: 1PGJ A and B chains), and further refinement was done with MD simulation using NAMD (35). A high degree of identity in the amino acid sequence was found (71.19% for Lm6PGDH and 78.29% for Tc6PGDH). The structural observations for the enzyme 6PGDH were established from the 3D homology models and the data reflected good agreement between the structures of these kinetoplastid enzymes; however, significant differences have been found when compared to the crystalline structure of the human enzyme (27). The active form of the enzyme was a homo-dimmer and three main domains were identified in each subunit: I, the coenzyme domain; II, the helical domain; and III, the tail. The C-terminal tail comprised the C-terminal residues of a subunit; it penetrated domain II of the adjacent subunit, thus joining the two monomers (Figure 1). The three-dimensional crystal structure of the L. donovani (Ld6PGD) in complex with NADP(H) has been recently solved, revealing a previously unknown conformation of NADPH (33).
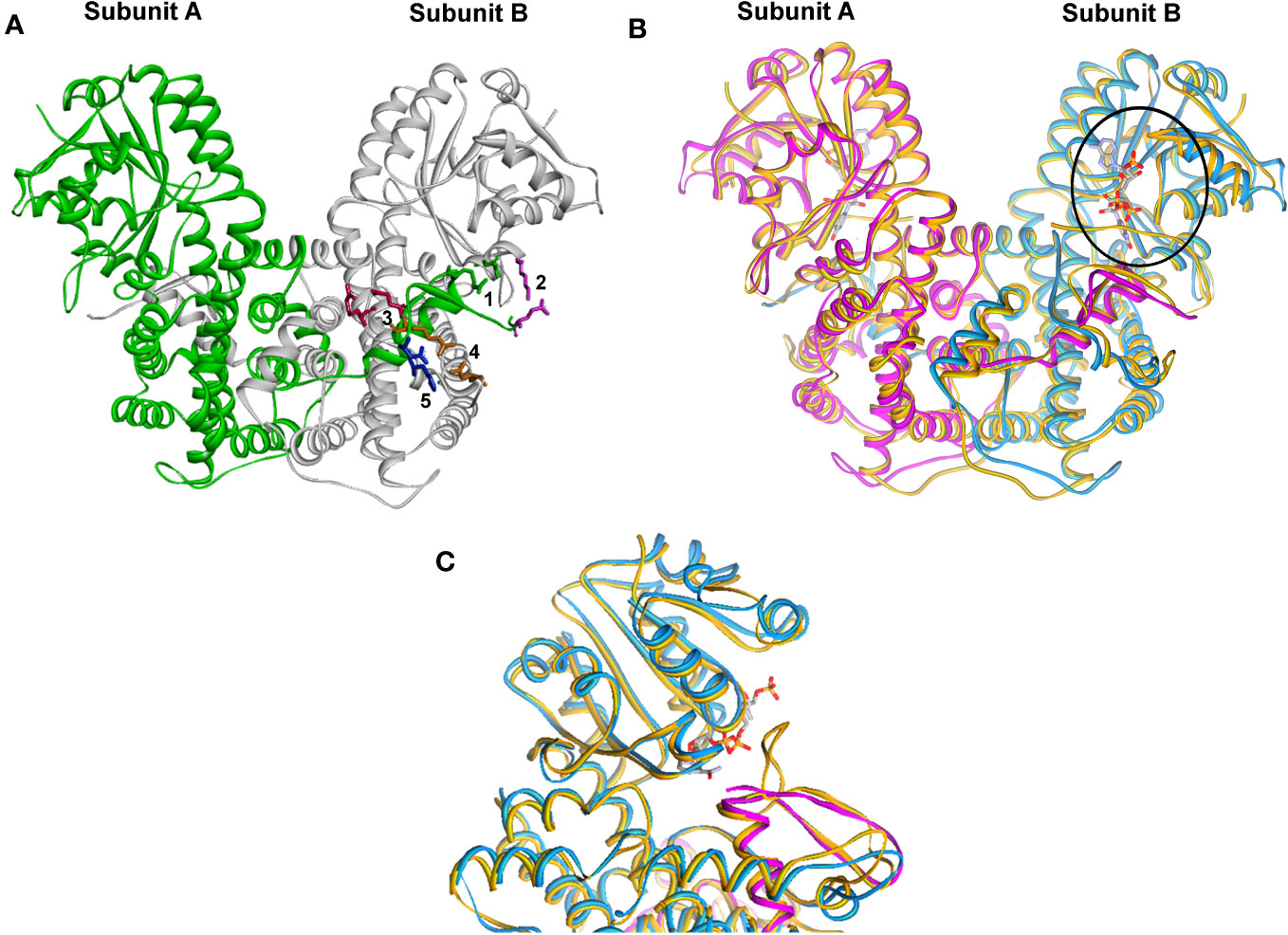
Figure 1 Structural molecular modeling of the Lm6PGDH and Tc6PGDH. (A) Crystal structure of the Tb6PGDH dimer (green loop). The details show the salt bridges in colors: green is 1, purple is 2, fuchsia is 3, orange is 4, and blue is 5. (B) Overlapping of the dimers of Lm6PGDH (one subunit as purple loop and the other blue) and Hs6-PGDH (mustard loop). The circle shows the NADPH in the coenzyme-binding domain. (C) Close up of the active site showing the differences in the coenzyme-binding site between Hs6-PGDH and Lm6-PGDH; in Kinetoplastids, it is GxGxxG and in mammals GxAxxG.
2.1.2 Kinetic Stability of the 6PGDH enzyme: Tb6PGDH vs. Lm6PGDH and Tc6PGDH
The structure of Tb6PGDH (PDB: 1PGJ) reveals that ionic interactions link both subunits of the homodimer; additionally, at the C-terminal tail, five residues form salt bridges with other amino acid subunits (Figure 2). Two of these salt bridges lie in the coenzyme domain (bridge 1: 459Arg-134Glu and bridge 2: 477Glu-139Lys) and the other three are situated in the helical domain (bridge 3: 463Glu-259Arg, bridge 4: 464Arg-246Asp, and bridge 5: 466Asp-255His).
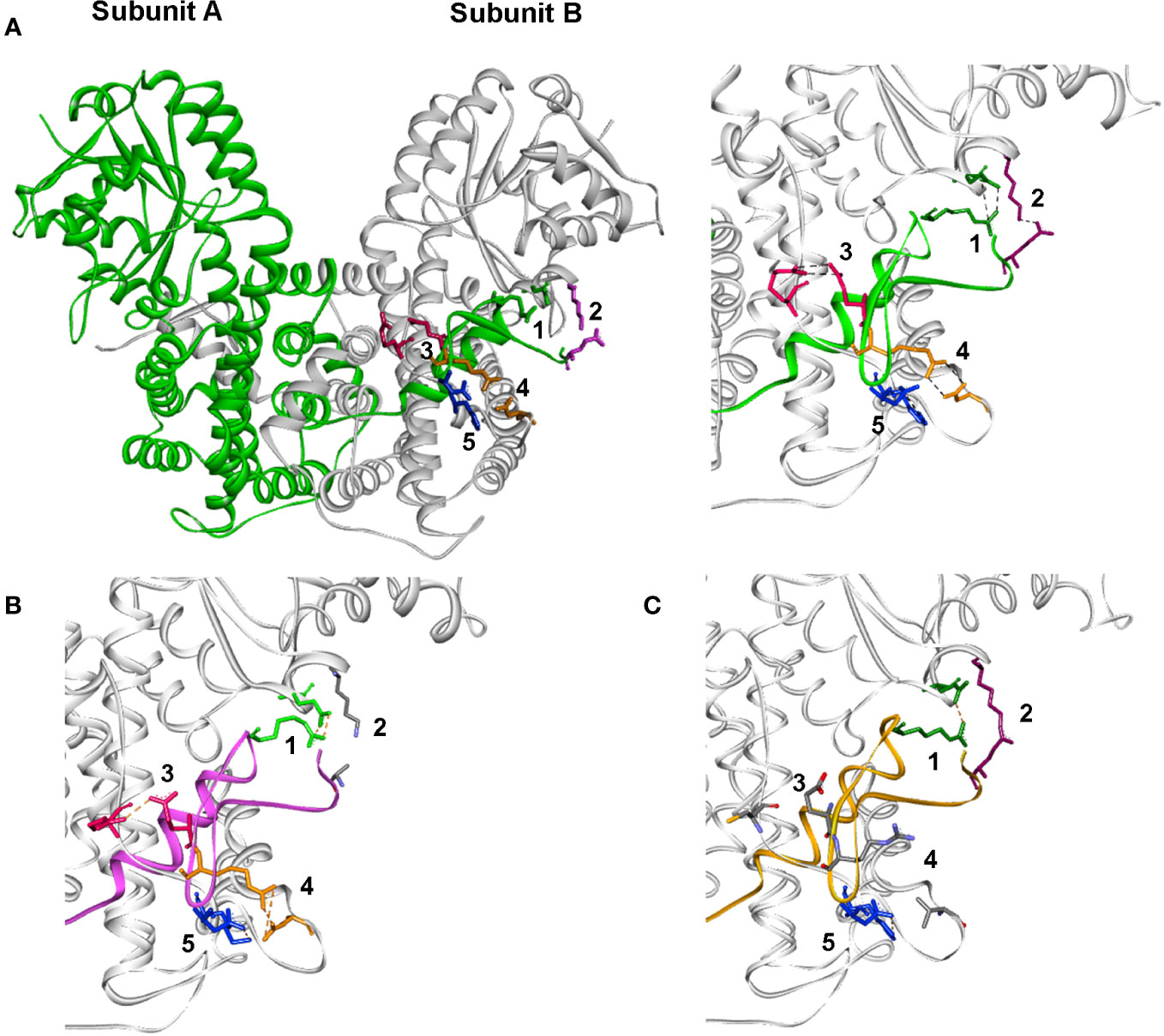
Figure 2 The 6PGDH dimer in different Kinetoplastids. Detail in the active site for (A) T. brucei; (B) L. (L.) mexicana, and (C) T. cruzi (ionic pairs between sub-units: 1 green, 2 lilac, 3 orange, 4 fuchsia, and 5 blue). *Missing pairs are colored by atom type.
A comparison of the 3D structures of the enzymes Tb6PGDH, Lm6PGDH, and Tc6PGDH show the conservation of salt bridge 1, which is characteristic of trypanosomatid enzymes (32). In Lm6PGDH, only four potential salt bridge sites are present, corresponding to salt bridges 1, 3, 4, and 5 (27). In this sequence, the change of a charged 477Glu residue to a neutral residue at the same position (477) eliminates the electrostatic interaction that defines bridge 2. In Tc6PGDH, only three interactions corresponding to salt bridges 1, 2, and 5 are present. In this sequence, the effective changes from 259Arg to 259Cys and from 246Asp to 246Val modify the conformation of salt bridges, leading to the loss of salt bridges 3 and 4 described in the coenzyme domain of the T. brucei enzyme. Specific genomic alterations that decrease the number of salt bridges disturb the quaternary structure of the ionic interactions of both subunits of the homodimer and determine the kinetic instability of the native enzymes in L. (L.) mexicana and T. cruzi. Site-directed mutagenesis experiments in T. cruzi to restore the corresponding bridges have demonstrated that it is possible to recover the stability of the enzyme (29, 30). In addition, preliminary studies of in silico site-directed mutagenesis with the 6PGDH structural models of T. cruzi and L. (L.). mexicana suggest that the instability observed in native enzymes could be due to weak associations between both enzyme subunits (27).
To understand the role that salt bridge interactions play in the enzyme’s structure and dynamic behavior, we have carried out preliminary studies performing comparative MD simulations for the native and mutated 6PGDH enzymes of T. brucei, T. cruzi, and L. (L.) mexicana. Preliminary results have determined differences in behavior between the native enzymes of these kinetoplastid organisms, suggesting that 6PGDH should be a potential target for the development of new therapeutic drugs against these parasites (Figure 2).
2.1.3 Virtual screening of chemical libraries
Virtual screening of databases has played a leading role in drug discovery. This CADD (Computer-Aided Drug Design) tool provides an efficient and cost-effective alternative to experimental high-throughput screening, enabling rapid identification of potentially active compounds. The essential enzymatic functions of 6PGDH for parasite survival and viability make it a specific and selective target; additionally, this enzyme differs sufficiently from that of the mammalian host (<35% AA sequence identity), a fact that is fundamental to prevent any cross-reactivity or interaction that could be adverse. An optimized 3D model for this enzyme has been obtained and used for a high-throughput docking study (35, 36). We evaluated the binding affinity, binding modes, critical interactions, and pharmaceutical properties of the lead compounds, which will be tested in vivo in the future.
2.2 Tubulin genes
Tubulins constitute fundamental proteins of eukaryotic cell microtubules. The genes that code for these proteins can be found as single or multiple genes (multigenic families) with a high degree of sequence identity throughout evolution (37, 38); the same features have been found in kinetoplastids (39, 43–46).
2.2.1 The beta-tubulin genes
In particular, the coding region of β-tubulin genes has shown sufficient variability to differentiate the other Leishmania from other kinetoplastids. On the other hand, the flanking region has been used as a molecular marker in the identification and discrimination between the subgenera of Leishmania, L. (Leishmania) and L. (Viannia), respectively; such distinction demonstrates a high sensitivity, specificity, and reliability in the identification of the parasite (39, 43–46).
2.2.2 Colchicine resistance in kinetoplastids
Previous work has shown that drugs such as colchicine and most benzimidazoles have little or no effect on Leishmania cell cultures (47–52), suggesting that there are differences in susceptibility to some of these drugs between mammalian β-tubulin and Leishmania spp. These differences prompted us to carry out a comparative structural study of the colchicine-binding site between Leishmania and other organisms such as bovine and porcine β tubulin.
2.2.3 Molecular modeling, molecular dynamic and docking
The 3D molecular structure of β-tubulin from L. (V.) guyanensis was established for the first time (53), with the support of the experimental 3D structures of porcine (1TUB) and bovine (1Z2B) β-tubulin, using the SWISS-MODEL modeling server and the DeepView/Swiss-PdbViewer 3.7 (54) to obtain an initial model and NAMD refinement (35). The comparative analysis showed an important structural change in the colchicine-binding pocket of L. (V.) guyanensis, which consists of an elongation of an α-helix structure and in turn, a torsion of it, which causes a displacement of structure toward the inside of the pocket, which would result in a steric impediment that makes it difficult for the colchicine to have access (Figure 3). On the other hand, in comparison with bovine, the colchicine pocket of L. (V.) guyanensis showed a set of amino acid substitutions leading to physicochemical changes in the region, generating unfavorable conditions for the binding of a hydrophobic molecule such as colchicine. The comparative topological theoretical 3D structure of the colchicine-binding pocket obtained from L. (L.) mexicana and L. (L.) major (Figure 3), species belonging to the subgenus Leishmania, coincided with what was observed in L. (V.) guyanensis, thus supporting the studies reported in the literature on the resistance of parasites of the genus Leishmania to the drug colchicine (51, 52). These differences open the possibility of evaluating this region as a potential pharmacological target. In this sense, we are working on the identification of potential compounds that selectively interact with this region using a combination of in silico approaches such as DM and high-throughput docking. It has been of great interest to find the same structural changes in T. cruzi, T. Evansi, and T. brucei. Thus, we concluded that resistance to colchicine in kinetoplastid organisms is a product of structural changes in the β-tubulin colchicine-binding pocket (53). In silico studies are in progress to define this region as a selective drug target.
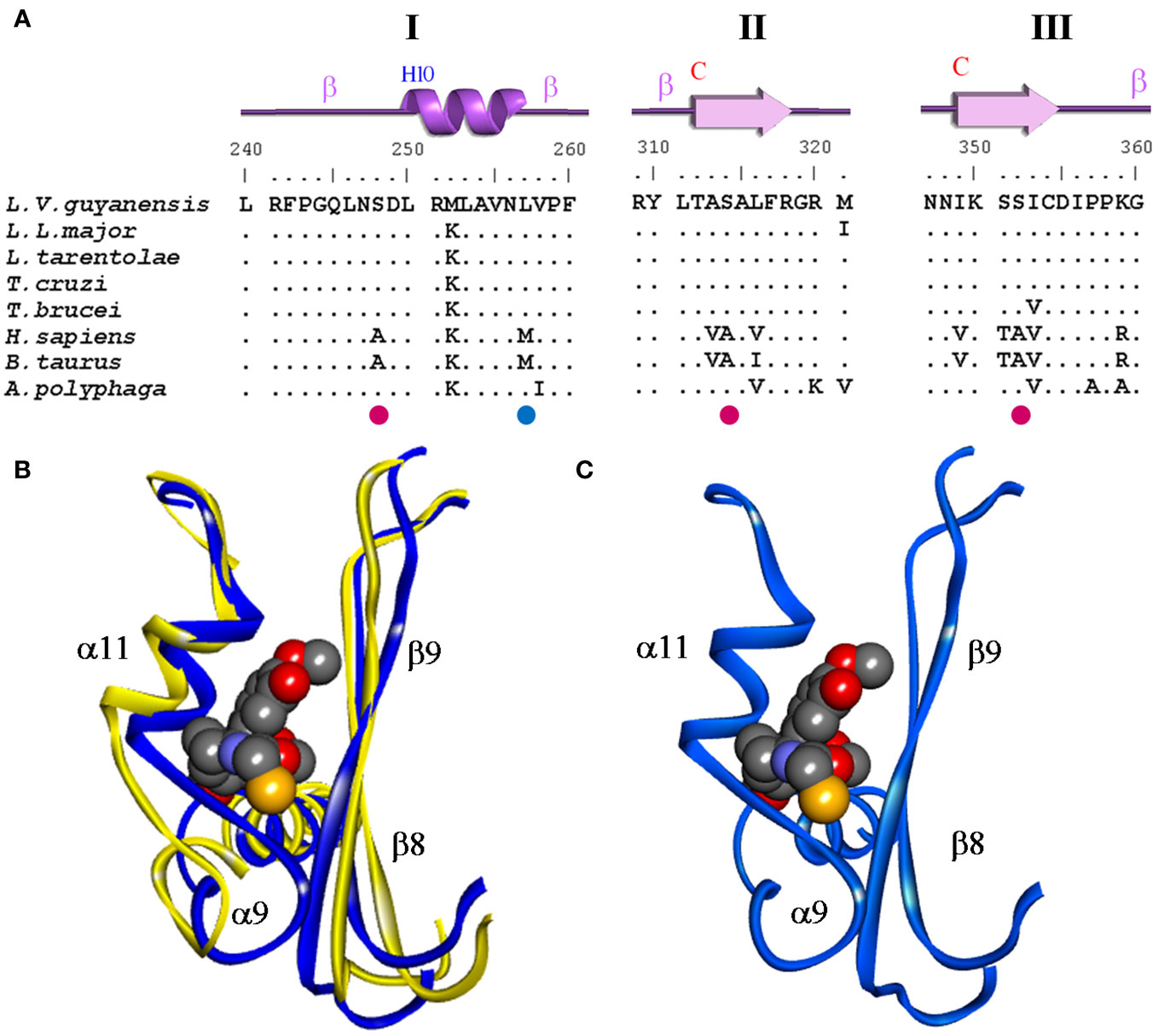
Figure 3 Comparison of the colchicine-binding pocket of L. (V.) guyanensis compared to other organisms. (A) Alignment of amino acid residues sequence of the β-tubulin colchicine- binding domains I, II, and III of different organisms. Eleven amino acid substitutions (AAS) can be observed. Identical amino acid residues are indicated by points and the four non- synonymous substitutions are highlighted colors. (B) Superimposition of both 3D structures is presented. Conformation of the colchicine-binding domain of the β−tubulin protein of bovine (yellow) and Leishmania (blue). The ligand colchicine (CN2) bound to the domain and secondary structure elements associated with this region are presented and defined as follows: helix α9-10 and β9-10 sheet. (C) 3D structure of the colchicine-binding domain of the β-tubulin protein of Leishmania (blue). The region comprises the amino acids from 242 to 262 of the β-tubulin protein where the steric impediment is generated. Modified from Ref. 50.
2.3 ABC-transporter and ABC-T blockers
In previous paragraphs, we mentioned that many of the anti-parasite drugs used nowadays target soluble enzymes, generate unspecific oxidative stress, or act by an uncertain mechanism of action within the intracellular milieu of the parasite. On the other hand, in the last decade, libraries of drug-like compounds resulting from the scrutiny of large-scale phenotypic screenings have been used for free by researchers, thus improving the identification of novel potential targets. Several of the compound hits have been found to inhibit membrane proteins, i.e., parasite ions and metabolite channels and transporters (22, 55).
The biophysical and biochemical properties of transmembrane transporters and channels constitute a usual base for their classification, i.e., the mechanism of transport and substrate selectivity as well as their location and function. Due to the ubiquity of these proteins, the acquired knowledge may be helpful to understand their function at many sites, which is relevant for the herein infectious disease studied, leishmaniasis. For example, channels and transporters (i) present in the infected host, (ii) in the parasite plasma membrane, (iii) in parasite organelle membranes, (iv) or (toxic compounds) drug efflux transporters. What is clear is that the methodological approaches commonly used to analyze them in mammalian cells have proven to be difficult to apply in protozoans in general and in these parasites in particular (55).
In fact, as has been described by Jimenez and Mesones, 2022 (56), transport mechanisms in the plasma membrane of Leishmania have been studied with a variety of methods. Thus, active transport and ion exchange mechanisms have been characterized, which is knowledge crucial for the understanding of the physiology of these parasites. They also might be of therapeutic relevance as initially, these parasites were unsuitable for the direct use of most electrophysiological techniques; the use of indirect methods, such as ion channel incorporation into planar bilayers could give a hint of their presence and function.
We accomplished initial data regarding this goal by using a method that allowed us to incorporate membrane vesicles in planar bilayers promoting their fusion by a method that uses nystatin as a fusing agent (57). We initially identified current spikes, representing the transient activity of multiple sterol-dependent nystatin channels (Ny-L). Afterward, we used Leishmania plasma membrane nystatin suspensions (Ny-P) that fused readily to the planar bilayers. In this case, although most of the obtained current spikes were similar to those seen using Ny-L, some current spikes were followed by smaller “square-shaped” current transitions, similar to those described for ion channels from other preparations. As this gating activity was only seen after the addition of Ny-P, the presence of ion channels in the Leishmania plasma membrane could then be inferred. The most frequent ones were two distinct anionic-type channels; records of multiple channel incorporation were also seen, suggesting that channels with different unitary conductance were present in the preparation (58). This approach demonstrated to be a powerful and convincing tool for the study of the biophysics of the plasma membrane of Leishmania parasites. However, to further our analysis we decided to apply cellular biology and biochemical methods in order to better understand the associated holistic physiology and better identify potential targets for the design of adequate therapeutic strategies.
It is widely recognized that the physiology behind ion channels and transport functions may be a promising avenue for developing drugs and strategies against leishmaniasis that may slow down the development of drug resistance (59); although as is usual for any other mechanism shared by eukaryotes, modulation of functions has the potential drawback that chemo-resistance to the considered compound might emerge. The conclusion that has to be raised then is that the risk/benefit profile of the use of inhibitors and drugs should be evaluated on a case-by-case basis.
Our goal was to study the physiology behind DR. In fact, as has been initially demonstrated for L. (L.) tarentolae and has recently been described in association with Cutaneous Leishmaniasis (CL) (60, 61), the overexpression of membrane-bound ATP-binding cassette (ABC) proteins such as the P-glycoprotein (P-gp) may be directly linked to DR in Leishmania (61). Thus, we initiated a series of studies to better understand this phenomenon in Leishmania, as well as the physiology behind the function of ion transport systems. ABC transporters modulate intracellular trafficking of chemotherapeutic agents and their efflux through the plasma membrane. P-gp has been found in chemo-sensitive and chemo-resistant cells of many origins, suggesting its physiological and pathological significance. So, it remains an interesting question to be addressed whether and how P-gp can be targeted to improve therapy against leishmaniasis (61).
Then, fostering our study on the physiology of Leishmania parasites, especially those producing CL, we analyzed the presence of transporters at different levels by the use, among others, of Glibenclamide (GLIB), a sulfonylurea that inhibits ABC proteins with dissimilar functions, such as the K+-ATP channel-associated sulfonylurea receptor, the cystic fibrosis transport regulator, the ABC1 transporter of immune cells, the P-glycoprotein (P-gp), the Arabidopsis multidrug resistance-related protein AtMRP5, and the multidrug resistance-associated protein (MRP) (ABCC1) of cancer cells (62).
A detailed description of our findings is summarized in Supplementary Table 1; herein, we will mention major findings to convince the readers about the pertinence of designing ABC transporter blockers for combination therapy in the treatment of leishmaniasis:
i. Differential susceptibility of Leishmania spp. vs. macrophages: These results were the initial evidence that suggested Leishmania’s susceptibility to blockers associated with ABC transporters. It is interesting to mention that blocking such systems might impair the survival of promastigotes and the persistence of parasites in host cells is also decreased by GLIB, i.e., infection of macrophages belonging either to cell lines or peritoneal-exudate macrophages (PEC) was not significantly decreased by the drug concentrations around the promastigotes’ EC50; however, survival of intracellular parasites decreased significantly in the presence of the drug without affecting macrophage viability (63, 64).
ii. Greater susceptibility of axenic amastigotes vs. promastigotes: Cultures of axenic amastigotes of L. (L.) mexicana were more susceptible to GLIB than promastigotes and genetically stable lines of drug-resistant promastigotes were affected by their growth under conditions of transformation to axenic amastigotes; their transformation did not appear to be affected by the drug. Greater sensitivity of axenic parasites to the drug may be due to membrane remodeling, with better exposure to the drug targets (65).
iii. Synergistic glibenclamide-glucantime drug interaction in macrophage-infected cells: The efficacy of glucantime in decreasing the infection rate of Leishmania-infected macrophages is strongly improved when used in combination with GLIB. Our results suggest strongly that GLIB targets in L. major is an intracellular compartment associated with a multivesicular system. This compartment is simultaneously labeled by the acidic marker LysoTracker-red and it may represent the organelle where antimonials are sequestered (62).
All in all, these results demonstrate the potential value of combination therapy by combining a classical drug like glucantime with an ABC transporter blocker against leishmaniasis (62). Interestingly, in in vivo experiments performed both in mice and humans, GLIB affects the size of the skin lesion and positively modulates the expression of interferon-γ as well as decreases the expression of tumor necrosis factor α, IL-4, and IL-10 (66, 67).
These data allowed us to further our studies, and initiate the molecular description of the mechanisms involved in the above-mentioned effects. Thus, we experimentally selected and began to characterize GLIB-resistant Leishmania strains (Figure 4).
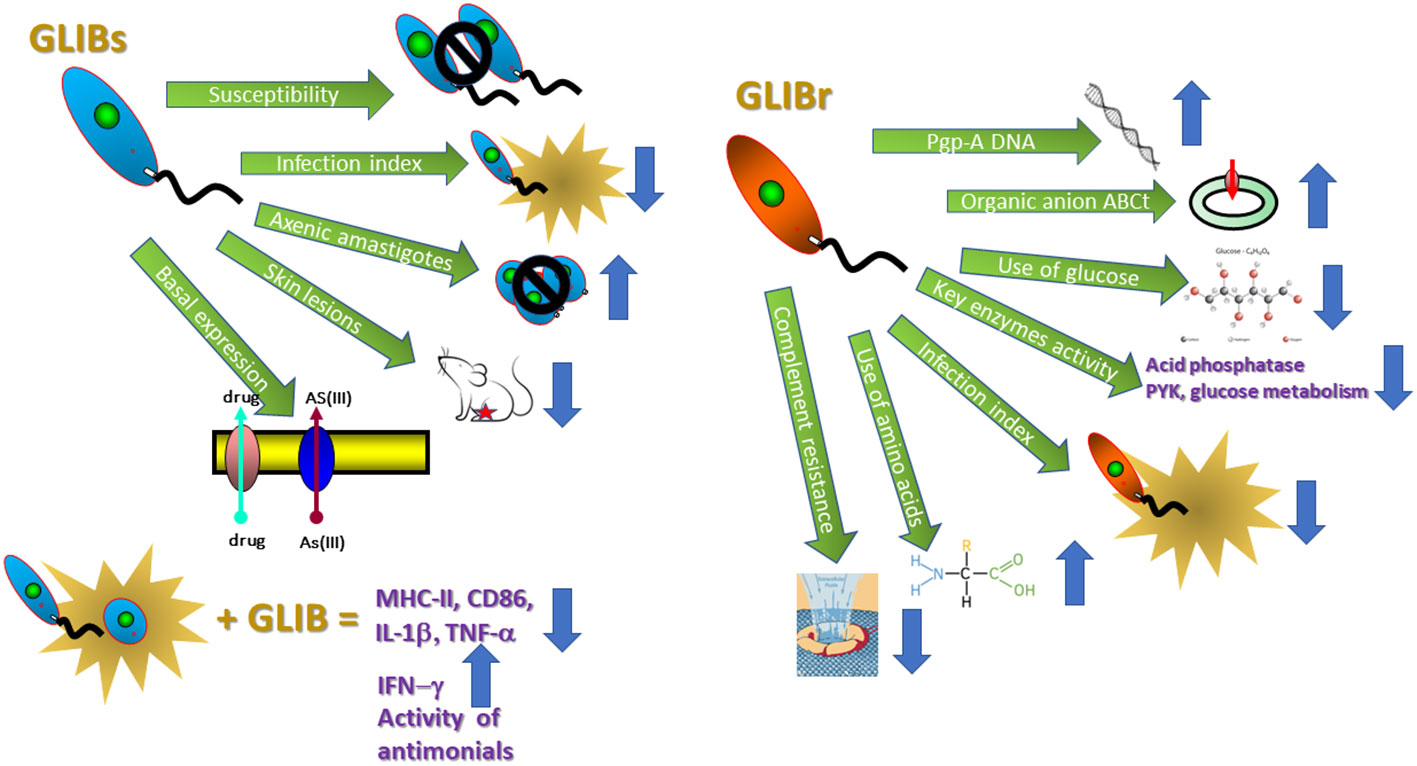
Figure 4 Mechanisms involved in GLIB-resistant Leishmania strains. The figure displays the mean effects produced by GLIB in GLIBs: glibenclamide sensitive and GLIBr: glibenclamide resistant Leishmania parasites. AS(III), arsenite; MHC-II, major histocompatibility complex II; CD86, Cluster of Differentiation 86; IL-1β, interleukin 1 beta; TNF-α, tumoral necrosis factor alpha; IFN-γ, interferon gamma; PYK, pyruvate kinase. Please refer to Supplementary Material Table 1 for specific effects.
Our results suggest that: (i) the expression of ABC transporters in these parasites is constitutive and there is an increased expression of organic anion ABC transporters in GLIB- resistant Leishmania; (ii) there are metabolic adaptations in Leishmania that determine DR and seem to differ from those described in mammalian drug-resistant cells. As a physiological consequence of the challenge imposed by drug pressure, these metabolic adaptations promote a stage-dependent modulation of energy substrate uptake and use; (iii) GLIB-resistant parasites are characterized by less ability to differentiate into infective metacyclic; they are less resistant to the lytic effect of the alternative complement pathway and are less infective toward either macrophage cell lines or BALB/c-derived macrophages; (iv) as a way to maintain the chemo-resistant phenotype, the parasitic cells make use of changes that are traduced in modified metabolic preferences, expression of surface proteins, and differentiation and infectivity capacity (68–72).
Notably, infected BALB/c mice macrophages treated with GLIB express lower levels of MHC-II and CD86 and levels of IL-1β (60). In in vivo assays in mice infected with L. major that may develop VL, the resistant host (C57Black/6 mice) progresses toward dominant Th-1 immune response to parasite antigens, whereas the susceptible mice advance toward a typical Th-2 response and surrender to infection (66, 67).
From a pharmacological point of view, it would be interesting to test if the results obtained in vitro using reference strains could be validated with parasites isolated from patients’ lesions conserving their intact phenotype. Also, a fundamental question to be addressed would be the analysis of these markers and their correlation with therapeutic failure in Leishmania isolated from infected patients.
The take-home message seems to be that both a decrease in parasite-fitness virulence and the intervention of an effective immune response are fundamental to promoting the cure of leishmaniasis. Both effects can be obtained with GLIB in in vitro and in vivo experiments. This suggests that GLIB may either be considered a good pharmacophore for developing new compounds (ABC transporter blockers) or it may be used together with leishmanicidal drugs in combination therapy.
2.3.1 ABC transporters
What are ATP-binding cassette (ABC) transporters? These molecules encompass a primeval superfamily of proteins conserved through evolution spanning from bacteria to humans. They can be defined as proteins integrated into the membrane that actively transport substrates of diverse chemical origins across the lipid bilayer of cellular membranes fundamental for many processes. One outstanding characteristic is that the outward (export and secretion) or inward (import) movement of molecules through ABC transporters is coupled to ATP hydrolysis (73, 74).
Drugs and antibiotics, as well as the uptake of essential nutrients, constitute ABC transporter substrates in bacteria. Prokaryotes incorporation of small solutes like histidine, maltose, peptides, or ribose is mainly mediated through ABC transporters. In fungi and parasites, they mediate virtually all the export translocation of substrates from the cytosol out of the cell or into intracellular organelles (73, 74).
A fundamental function of ABC transporters throughout all kingdoms is associated with resistance to antimicrobial drugs. In mammals, ABC transporters mediate chemoresistance, especially through the overexpression of the multidrug-resistance (MDR) proteins (ABCB1), MRP (ABCC1), and the breast cancer resistance proteins (BCRP, ABCG2; mitoxantrone-resistance proteins). However, in diseases including cystic fibrosis, secondary diabetes and children’s hyperinsulinemic hypoglycemia, or the X-linked peroxisome disorder known as adrenoleukodystrophy, the malfunction of ABC transporters is commonly recognized.
Finally, antigen-processing proteins, TAP1, and TAP2 belong to ABC transporters used for antigenic peptides’ transport across the endoplasmic reticulum. Their role pertains to antigen processing or is associated with drug delivery and interaction in epithelial cells (73, 74).
2.3.2 Structure–activity relationships for ABC transporter blockers
ABC transporters, placed either at the plasma membrane or at the intracellular organelle membranes, can be described through all kingdoms in nature. Their very well-preserved role in translocating molecules through different cell barriers is a characteristic that strongly suggests the vital role they might have for cell and organism survival. Multiple structural variations of these transporters work as import or export transporters and recognize a multitude of compounds including dyes, toxic ions, and clinically relevant antibiotics and anticancer drugs (73, 74). The transmembrane domains constitute structural structures characterizing them. They allow the protein to be anchored to the membrane and to shape the needed pore for substrate transport to occur (73, 74). The ATP-binding domains are responsible for the release of ATP energy. The description of high-resolution structural data for multiple ABC transporters has occurred over the last two decades (74); this fact has contributed to transforming the previous three-dimensional static view of the transporter to a dynamic and useful one (75).
The ensemble of structures revealed in the architecture of ABC transporters shapes their mechanistic view; however, this structure machine-like interpretation is not trivial and should be carried out with carefulness until the architecture of many transporters, including substrates or without them, is analyzed (70). One recent example is the plasma membrane ABC transporter (PfMRP1; PF3D7_0112200) from Plasmodium falciparum. Pfmrp-1 has been identified as a solute importer. Of note, this function has not been previously observed for ABC transporters in this organism. Interestingly, parasites that express a disrupted Pfmrp-1 become resistant to folate analogs, methotrexate, and aminopterin. This effect is mediated through a reduced accumulation of the compound in the parasite cytoplasm (76).
Furthermore, at the atomic level, as has been thoroughly described by Ramos et al. (2018) (77), areas that still need to be elucidated are: (i) specific conformational changes in transmembrane domains that induce the unidirectional substrate transport against the concentration gradient; (ii) molecular events (substrate and ATP binding, ATP hydrolysis, and product release) that provide the “power stroke” and trigger each of these conformational changes in TMD; (iii) the way these conformational changes couple with ATP binding and hydrolysis (77).This information is fundamental for a rational design of drugs to block the activity of ABC transporters.
Additionally, the chemical structures of the ABC transporters’ substrates are very wide including inorganic ions, organic compounds, bile acids, glutathione and glucuronide conjugates, and lipids. Additionally, small peptides can be translocated through ABC transporters. This wide variety of substrates constitutes a huge challenge to perform and understand structure–activity relationships (SARs) underlying transport mechanisms clogging the design of specific inhibitors to block their function (73, 74).
Finally, the extensive distribution from bacteria to humans and the multiple mechanisms related to ABC transporters highlight the need to better understand their physiological functions, factors affecting their expression, and which principles are common to them.
2.3.3 Development of ABC transporter blockers
A common mechanism for DR relies on the export of drugs by transporters. This means that agents specifically designed to either competitively or non-competitively block ABC transporters related to DR should be able to impair drug extrusion from cells and thus may be able to help restore the cytotoxic action of drugs. However, since they may act on multiple ABC transporters, enhanced levels of toxicity at different non-target sites can be observed. Thus, the description and/or design of ABC-transporter blockers has a clinical meaning beyond doubt. Although scientific advance in this area has not been fast enough, obtained information until now is extremely helpful for the comprehension of SAR, the way binding sites for drugs and modulators are built up within the protein, and the functional connection between the generation of energy and translocation of drugs (73, 74).
ABC transporter blockers can be classified according to their generation in three types as described in Supplementary Table 2. Those belonging to first-generation inhibitors are pharmaceutical compounds already in use for alternative treatments but able to block P-gp. Within them, we can mention calcium channel inhibitors like verapamil; antiestrogens like tamoxifen and toremifene; anti-arrhythmic and neuroleptics like quinidin; and immunosuppressants like cyclosporin A, reserpine, and yohimbine. Their clinical efficacy is limited by their toxicity and has never been shown to inhibit P-gp in patients (73, 74, 78–82).
Second-generation P-gp modulators include R-verapamil, GF120918, MS-209, PSC-833, VX-710, or VX-853, compounds that are derived from first-generation P-gp blockers. Although these compounds are clinically active and inhibit P-gp in patients, the significant pharmacokinetic interaction produced delays the excretion of the primary agent, which is an issue that ends up in increased toxicity, and consequently reduces the dose of the primary agent (73, 74, 78–82).
Third-generation modulators are highly selective inhibitors of P-gp and are designed to interact with specific ABC transporters with high affinity and efficacy at a nanomolar range. Natural inhibitors include flavonoids, compounds that are many fold more active than verapamil. They are extracted from plants, express low toxicity, and increase cellular accumulation of substances in MDR P-gp-overexpressing cells. This mainly accounted for the selective inhibition of ABC transporters. The mechanism of action of flavonoids relates to their binding to the nucleotide-binding domain of ABC transporters. This attachment occurs in a site different from that occupied by ATP; of note, their inhibitory activity correlates with their hydrophobicity (73, 74, 78–82).
Most of the P-gp inhibitors that have been tested against MRP- 1 or BCRP had no inhibitory effect, the main reason being that P-gp recognizes hydrophobic compounds, while MRP-1 recognizes hydrophilic substrates. Additionally, the data against BCRP is hard to understand since this protein transports structurally and functionally organic substrates, including hydrophobic compounds; weak bases; organic anions; and glucuronide-, sulfate-, glutamylate-, and glutathione-conjugates (73, 74).
A multitarget dataset of 1,167 ABC transporter inhibitors has been recently evaluated for 604 molecular substructures in a statistical binary pattern distribution scheme (83). As clearly stated by the authors, Stephan et al. (2022) (83), this binary pattern multitarget dataset (ABC_BPMDS) seems to be a useful tool for the design of (i) poly-pharmacological agents, (ii) highly potent and selective ABC transporter-targeting agents, and (iii) agents that avoid clearance by the focused ABC transporters [e.g., at the blood-brain barrier (BBB)].
All the facts described suggest how difficult it is to design ABC transporter blockers. The main reasons are clearly stated by Ponte-Sucre et al. (2009) (74) and herein summarized: (i) they express a strongly conserved primary sequence although few orthologous pairs of transporters are shared between phyla; (ii) they have an extended substrate flexibility, suggesting redundancy for the same substrates, a fact that makes gene losses tolerable; (iii) they present multiple binding sites, a fact that hinders the development of inhibitors and impairs an appropriate SAR comprehension for P-gp and MRP (73, 74). Last but not least, the movement speed of substrates across membranes through P-gp and ABC transporters constitute a key point and deciding factor in determining whether a compound is a P-gp substrate (slowly permeating) or inhibitor (rapidly permeating), even more than its membrane affinity. The functional consequences are clear: if a P-gp modulator/blocker cycles repeatedly and fast, it prevents the export of the accompanying drug, thus increasing its intracellular concentration; in this case, the modulator acts as a competitive inhibitor. One additional point to be mentioned is that the functional dynamics of the membrane might set the way drugs enter the cell, for example, more rapidly than P-gp can export them; in this case, a reduced DR might be obtained (73, 74).
2.3.4 Applications of ABC transporter blockers in Leishmania
The function of ABC transporters expressed in wild-type cells is mainly related to the maintenance of low levels of cell xenobiotics, control of drug–drug interaction, and facilitation of drug disposal. Their function is related to the pumping back of molecules into spaces and compartments where they were originally located. This means that they in a way establish a limit on the success of membrane transport systems. We have mentioned that leishmaniasis is a disease whose cure seems to be related to decreased parasite fitness, evaluated as virulence, and the intervention of an effective immune response. The appropriate behavior that GLIB as an ABC transporter blocker has both in vitro and in vivo makes it a representative good pharmacophore for developing compounds to be used in combination therapy with antileishmanial drugs with effective results.
This means that as ABC transporters are involved in so many cellular mechanisms and the fact that there is a rising concern related to the emergence of DR in leishmaniasis associated with the overexpression of ABC transporters in Leishmania parasites, the identification, design, feasibility, and value of ABC transporter blockers may represent a valuable tool that may contribute to increase the efficiency of first-line drugs as well as trace the location of ABC transporters in vivo. This makes ABC transporter blockers fundamental for the comprehension and management of the clinical scenario (73, 74).
Thus, new anti-parasitic drugs should be designed to be “poor” ABC transporter substrates. This has been tried for microorganisms in general although the success of this approach has been partial. Based on the presented results of GLIB use in Leishmania, we can suggest some hints to be tested for the design of ABC transporter blockers that might be crucial to advance to better anti-Leishmania pharmacotherapy (73, 74).
i. The development and design of drugs minimally used as substrates by the ABC transporters. As this may be very challenging, the strategy of developing blockers that specifically target ABC transporters seems to be one promising avenue to overcome the partial success of antimicrobial and antiparasitic chemotherapy.
ii. The identification of compounds that by inhibiting the efflux pump may increase the intracellular concentration and effect of the first-line administered drug. For example, the approach of using an ABC transporter blocker to enhance the systemic levels of orally administered drugs may increase the availability of drugs whose transport back to the lumen of the gastrointestinal tract depends on ABC transporters. This issue would result in diminishing drug toxicity and side effects.
iii. The combination of ABC transporter substrates and blockers may be a strategy that could help in the analysis of DR in patients and identify the in vivo location of ABC transporters. The measurement of the corresponding mRNA and protein concentrations is the usual method to identify the cellular localization and levels of ABC transporters. However, the use of 11C-labeled ABC transporter substrates and inhibitors might be helpful to envisage the dynamic function of these proteins in vivo and to visualize the function (and changes) of P-gp-mediated transport through positron emission techniques.
iv. As mentioned by Higgins, 2007 (40), the time has arrived to understand that we cannot fight against DR but we have to find alternatives to avoid and avert it. ABC transporter blockers may end up being useful tools to prevent the advent of DR. In fact, for example, we can explore if the ABC transporter blockers may avoid the selection of resistant strains with increased expression of ABC transporters like MDR or MRP.
Three final ideas are important to stress. Therapeutic failure and drug resistance are similar but not the same, for example, the different epidemiological context of New World leishmaniasis makes therapeutic failure more complex and unpredictable than therapeutic failure from Old World leishmaniasis. Furthermore, clinical cure, grounded on symptoms and parasitological cure, is doubtful if we are in the presence of immunosuppressed patients in which after “cure”, parasite re-emergence can develop. This is especially so for VL “successfully” cured patients who can then enter a (quiescent) state that may be compared to asymptomatic patients (41). Asymptomatic patients conform to a group of patients that are challenging for basic and public health researchers. Why do some patients remain without symptoms although are infected and some develop the disease? Do they conform to a group with a quantitative spreading potential? Do they carry enough parasites available to infect sand flies? Are they protagonists in spreading DR parasites? Why are they silent? Do they have a role in these individuals? What is the metabolic status of parasites present in asymptomatic individuals? Are they quiescent-like cells? Can they enter in reactivation afterward? What is their sensitivity to immune response? What is their drug susceptibility?
We can hypothesize that continued therapy after detecting DR may represent the potential maintenance of a “less healthy” variant and as a way to, maybe, decrease the parasitic load without a drug-mediated inhibition. However, new levels of virulence might develop in parasite communities as a response to chemotherapy. Intentional selection of drug-resistant variants, potentially with reduced fitness, may be proposed as a way to improve the therapy. But since evolution can go in either direction, a less capable drug-resistant parasite could evolve into a competent isolate, i.e., upon elimination of drug pressure can be again transmitted to new human recipients. This means that we need to design a validated protocol to measure cell fitness (42) (in the presence and absence of drugs). Last but not least, and as previously mentioned, the evolution of resistant strains might result in compensatory mutations that could eventually return cell potency to that found in a wild strain. This means that the phenotype of species subjected to drug pressure must be continuously evaluated and validated, for example, through characteristics such as metabolite usage and enzymatic pathway fluxes in parallel with resistance selection and infectivity (63).
Finally, intracellular drug concentration constitutes a critical parameter determining cellular DR. This intracellular drug concentration is established by the steadiness between drug entrance through the membrane and drug export by the competent transporters present in the cell. Compounds with similar structures act at the same site and by the same mechanism, but different spots and mechanisms are normally used for inhibition and/or binding at P-gp, MRP-1, or ABC transporters. This means that a general SAR of substrate or inhibitors of ABC transporters represents a challenge (73).
3 Final remarks
Until now, the main treatment against leishmaniasis has been chemotherapy; DR remains one of the main challenges faced; thus, the identification of new therapeutic targets and drugs is essential for the development of alternative therapeutic treatments for the control of diseases. The inclusion of genomic studies, computational approaches, AI, and bioinformatics-based structure-based drug design methods have been essential to evaluate different proteins of metabolic pathways, which are important for parasites’ survival, and identify drug targets against trypanosomatid parasites. Through the present review, we have analyzed: (a) the pentose phosphate pathway key enzyme 6PGDH, which is essential for protecting kinetoplastid parasites from oxidative stress. The results demonstrate that 6PGDH differs from the mammalian host enzyme; (b) the β−tubulin which is a cell microtubule protein that is fundamental for the eukaryotic cells’ structural conformation and illustrates that there are modifications represented by amino acid substitutions in the colchicine-binding pocket in Leishmania, compared to the bovine and human proteins that prevent the access of colchicine to the β-tubulin protein. These facts offer advantages to drug design due to selectivity issues that can be exploited. Finally, due to the fact that ABC transporters modulate intracellular trafficking of chemotherapeutic agents, and their efflux through the plasma membrane, and these molecules have been found to be over-expressed in chemo-resistant Leishmania, we ask ourselves the potential value of designing ABC-T blockers for combination therapy in the treatment of leishmaniasis. These examples highlight how the search and description of new therapeutic targets and pharmacophores are fundamental in the alternative and rationally designed treatments of the disease.
Author contributions
This manuscript has been written, read, and approved by all the authors. There is an agreement between the authors on the order in which they should appear. All authors contributed to the article and approved the submitted version.
Funding
The authors are grateful to the Universidad Central de Venezuela Council for Research for grants received through the development of this research.
Acknowledgments
The authors are grateful for the support conferred by Institute of Experimental Biology (IBE) from Faculty of Sciences, and Institute of Tropical Medicine (IMT), Faculty of Medicine, Universidad Central de Venezuela (UCV) to AM-L; the Pharmaceutical Institute of Research from the Faculty of Pharmacy, Universidad Central de Venezuela to MLS; and to the Siebold-Collegium Institute for Advanced Studies from the University of Würzburg and the Alexander von Humboldt Foundation, Germany to AP-S. Likewise, we have had the support of the Ministry of Popular Power for Science, Technology and Innovation through the Leishmaniasis and Trypanosomiasis Network.
Conflict of interest
The authors declare that the research was conducted in the absence of any commercial or financial relationships that could be construed as a potential conflict of interest.
The author AM-L declared that they were an editorial board member of Frontiers, at the time of submission. This had no impact on the peer review process and the final decision.
Publisher’s note
All claims expressed in this article are solely those of the authors and do not necessarily represent those of their affiliated organizations, or those of the publisher, the editors and the reviewers. Any product that may be evaluated in this article, or claim that may be made by its manufacturer, is not guaranteed or endorsed by the publisher.
Supplementary material
The Supplementary Material for this article can be found online at: https://www.frontiersin.org/articles/10.3389/fitd.2023.1241044/full#supplementary-material
References
1. Hefnawy A, Berg M, Dujardin JC, De Muylder G. Exploiting knowledge on Leishmania drug resistance to support the quest for new drugs. Trends Parasitol (2017) 33(3):162–74. doi: 10.1016/j.pt.2016.11.003
2. Mutiso JM, Macharia JC, Kiio MN, Ichagichu JM, Rikoi H, Gicheru MM. Development of Leishmania vaccines: predicting the future from past and present experience. J BioMed Res (2013) 27(2):85–102. doi: 10.7555/JBR.27.20120064
3. Croft SL, Olliaro P. Leishmaniasis chemotherapy-challenges and opportunities. Clin Microbiol Infect (2011) 17(10):1478–83. doi: 10.1111/j.1469-0691.2011.03630.x
4. den Boer M, Argaw D, Jannin J, Alvar J. Leishmaniasis impact and treatment access. Clin Microbiol Infect (2011) 17(10):1471–7. doi: 10.1111/j.1469-0691.2011.03635.x
5. Serrano-Martin X, Payares G, De Lucca M, Martínez JC, Mendoza-León A, Benaim G. Amiodarone and miltefosine act synergistically against Leishmania mexicana and can induce parasitological cure in a murine model of cutaneous leishmaniasis. Antimicrob Agents Chemother (2009) 53:5108–13. doi: 10.1128/AAC.00505-09
6. Tiuman TS, Santos AO, Ueda-Nakamura T, Filho BP, Nakamura CV. Recent advances in leishmaniasis treatment. Int J Infect Dis (2011) 15(8):e525–32. doi: 10.1016/j.ijid.2011.03.021
7. Daga MK, Rohatgi I, Mishra R. Leishmaniasis. Indian J Crit Care Med (2021) 25(Suppl 2):S166–S170. doi: 10.5005/jp-journals-10071-23844
8. Loureiro I, Faria J, Santarem N, Smith TK, Tavares J, Cordeiro-da-Silva A. Potential drug targets in the pentose phosphate pathway of Trypanosomatids. Curr Med Chem (2018) 25(39):5239–65. doi: 10.2174/0929867325666171206094752
9. Nagle AS, Khare S, Kumar AB, Supek F, Buchynskyy A, Mathison CJN, et al. Recent developments in drug discovery for Leishmaniasis and human African Trypanosomiasis. Chem Rev (2014) 114:11305–47. doi: 10.1021/cr500365f
10. Ansari MY, Dikhit MR, GCh S, Ali V, Das P. Recent advancement and treatment of leishmaniasis based on pharmaco informatics approach: Current and future outlook. Gene Rep (2017) 9:86–97. doi: 10.1016/j.genrep.2017.09.003
11. Croft SL, Seifert K, Yardley V. Current scenario of drug development for leishmaniasis. Indian J Med Res (2006) 123(3):399–410.
12. Hendrickx S, Guerin PJ, Caljon G, Croft SL, Maes L. Evaluating drug resistance in visceral leishmaniasis: the challenges. Parasitology (2018) 45(4):453–63. doi: 10.1017/S0031182016002031
13. Ponte-Sucre A, Gamarro F, Dujardin JC, Barrett MP, López-Vélez R, García-Hernández R, et al. Drug resistance and treatment failure in leishmaniasis: A 21st century challenge. PloS Negl Trop Dis (2017) 11(12):e0006052. doi: 10.1371/journal.pntd.0006052
14. Alcântara LM, Ferreira TCS, Gadelha FR, Miguel DC. Challenges in drug discover targeting TriTryp diseases with an emphasis on leishmaniasis. Int J Parasitol Drugs Drug Resist (2018) 8(3):430–9. doi: 10.1016/j.ijpddr.2018.09.006
15. DNDi. Annual report 20 years. Available at: https://dndi.org/about/annual-reports/.
16. Ponte-Sucre A, Díaz E, Padrón Nieves M. The concept of fitness and drug resistance in Leishmania. In: Ponte-Sucre A, Diaz E, Padrón Nieves M, editors. Drug resistance in Leishmania parasites. Consequences, molecular mechanisms, and possible treatments. Wien: Springer Verlag (2013). p. 431–449.
17. Ponte-Sucre A. Physiological consequences of drug resistance in Leishmania and their relevance for chemotherapy. Kinetoplastid Biol Dis (2003) 2(1):14. doi: 10.1186/1475-9292-2-14
18. Ouellette M, Drummelsmith J, Papadopoulou B. Leishmaniasis: drugs in the clinic, resistance and new developments. Drug Resist Update (2004) 7(4-5):257–66. doi: 10.1016/j.drup.2004.07.002
19. Ghobakhloo N, Motazedian MH, Pourmohammadi B, Yousefi Z. Evaluation of correlation between the in vitro susceptibility of field isolates of Leishmania major and clinical outcomes of meglumine antimoniate therapy in Fars Province, Iran. J Arthropod Borne Dis (2017) 11(1):132–8.
20. Van den Kerkhof M, Sterckx YG, Leprohon P, Maes L, Caljon G. Experimental strategies to explore drug action and resistance in kinetoplastid parasites. Microorganisms (2020) 8(6):950. doi: 10.3390/microorganisms8060950
21. Soni M, Pratap JV. Development of novel anti-leishmanials: The case for structure-based approaches. Pathogens (2022) 11:950. doi: 10.3390/pathogens11080950
22. Singh R, Kashif M, Srivastava P, Manna PP. Recent Advances in chemotherapeutics for leishmaniasis: Importance of the cellular biochemistry of the parasite and its molecular interaction with the host. Pathogens (2023) 12:706. doi: 10.3390/pathogens12050706
23. Raj S, Sasidharan S, Balaji SN, Saudagar P. An overview of biochemically characterized drug targets in metabolic pathways of Leishmania parasite. Parasitol Res (2020) 119:2025–37. doi: 10.1007/s00436-020-06736-x
24. Kovářová J, Barrett MP. The pentose phosphate pathway in parasitic trypanosomatids. Trends Parasitol (2016) 32(8):622–34. doi: 10.1016/j.pt.2016.04.010
25. Maugeri DA, Cazzulo JJ. The pentose phosphate pathway in. Trypanosoma cruzi FEMS Microbiol Lett (2004) 234(1):117–23. doi: 10.1016/j.femsle.2004.03.018
26. Opperdoes FR, Coombs GH. Metabolism of Leishmania: proven and predicted. Trends Parasitol (2007) 23(4):149–58. doi: 10.1016/j.pt.2007.02.004
27. González D, Pérez JL, Serrano ML, Igoillo-Esteve M, Cazzulo JJ, Barrett MP, et al. The 6-phosphogluconate dehydrogenase of Leishmania (Leishmania) mexicana: Gene characterization and protein structure prediction. J Mol Microbiol Biotechnol (2011) 19(4):213–23. doi: 10.1159/000320697
28. Barrett MP, Le Page RW. A 6-phosphogluconate dehydrogenase gene from Trypanosoma brucei. Mol Biochem Parasitol (1993) 57(1):89–99. doi: 10.1016/0166-6851(93)90247-u
29. Esteve MI, Cazzulo JJ. The 6-phosphogluconate dehydrogenase from Trypanosoma cruzi: the absence of two inter-subunit salt bridges as a reason for enzyme instability. Mol Biochem Parasitol (2004) 133(2):197–207. doi: 10.1016/j.molbiopara.2003.10.007
30. Igoillo-Esteve M, Maugeri D, Stern AL, Beluardi P, Cazzulo JJ. The pentose phosphate pathway in Trypanosoma cruzi: a potential target for the chemotherapy of Chagas disease. Acad Bras Cienc (2007) 79(4):649–63. doi: 10.1590/s0001-37652007000400007
31. Hanau S, Rinaldi E, Dallocchio F, Gilbert IH, Dardonville C, Adams MJ, et al. 6-phosphogluconate dehydrogenase: a target for drugs in African trypanosomes. Curr Med Chem (2004) 11(19):2639–50. doi: 10.2174/0929867043364441
32. Phillips C, Dohnalek J, Gover S, Barrett MP, Adams MJ. A 2.8 Å resolution structure of 6-phosphogluconate dehydrogenase from the protozoan parasite Trypanosoma brucei: comparison with the sheep enzyme accounts for differences in activity with coenzyme and substrate analogues. J Mol Biol (1998) 282(3):667–81. doi: 10.1006/jmbi.1998.2059
33. Berneburg I, Stumpf M, Velten A-S, Rahlfs S, Przyborski J, Becker K, et al. Structure of Leishmania donovani 6-phosphogluconate dehydrogenase and inhibition by phosphine gold(I) complexes: A potential approach to leishmaniasis treatment. Int J Mol Sci (2023) 24:8615. doi: 10.3390/ijms24108615
34. Schwede T, Kopp J, Guex N, Peitsch MC. SWISS-MODEL: An automated protein homology-modeling server. Nucleic Acids Res (2003) 31(13):3381–5. doi: 10.1093/nar/gkg520
35. Phillips JC, Braun R, Wang W, Gumbart J, Tajkhorshid E, Villa E, et al. Scalable molecular dynamics with NAMD. J Comput Chem (2005) 26(16):1781–802. doi: 10.1002/jcc.20289
36. Ghosh S, Nie A, An J, Huang Z, Bryant SH. Structure-based virtual screening of chemical libraries for drug discovery. Curr Opin Chem Biol (2006) 10(3):194–202. doi: 10.1016/j.cbpa.2006.04.002
37. Cleveland DW, Sullivan KF. Molecular biology and genetics of tubulin. Annu Rev Biochem (1985) 54:331–65. doi: 10.1146/annurev.bi.54.070185.001555
38. Mendoza-León A, Barker DC. Variation of a multigene family in New World Leishmania: the β-tubulin gene region. In: Tapia FJ, Cáceres- Dittmar G, Sánchez MA, editors. Molecular and Immune Mechanisms in the Pathogenesis of Cutaneous Leishmaniasis. Austin, Tex USA: R.G. Landes Company Biomedical Publishers (1996). p. 107–28.
39. Mendoza-León A, Luis L, Martinez C. The β-tubulin gene region as a molecular marker to distinguish Leishmania parasites. In: Aquino de Muro M, Rapley R, editors. Methods in Molecular Biology. Gene Probes Principles and Protocols, vol. 179 . Totowa, NJ, USA: Humana Press (2002). p. 61–83. doi: 10.13140/RG.2.1.3850.3846
40. Jackson AP, Vaughan S, Gull K. Evolution of tubulin gene arrays in Trypanosomatid parasites: genomic restructuring in Leishmania. BMC Genomics (2006) 7:261. doi: 10.1186/1471-2164-7-261
41. Mendoza-León A, Havercroft JC, Barker DC. The RFLP analysis of the beta-tubulin gene region in New World Leishmania. Parasitology (1995) 111(01):1–9. doi: 10.1017/s0031182000064544
42. Eresh S, de Bruijn MH, Mendoza-León A, Barker DC. Leishmania (Viannia) lainsoni occupies a unique niche within the subgenus Viannia. Trans R Soc Trop Med Hyg (1995) 89(2):231–6. doi: 10.1016/0035-9203(95)90509-x
43. Luis L, Ramírez A, Aguilar CM, Eresh S, Barker DC, Mendoza-León A. The genomic fingerprinting of the coding region of the beta-tubulin gene in Leishmania identification. Acta Trop (1998) 69(3):193–204. doi: 10.1016/s0001-706x(97)00128-9
44. Katiyar SK, Gordon VR, McLaughlin GL, Edlind TD. Antiprotozoal activities of benzimidazoles and correlations with beta-tubulin sequence. Antimicrob Agents Chemother (1994) 38(9):2086–90. doi: 10.1128/AAC.38.9.2086
45. Lacey E. Mode of action of benzimidazoles. Parasitol Today (1990) 6(4):112–5. doi: 10.1016/0169-4758(90)90227-u
46. Moulay L, Rebert-Gero M, Brown S, Gendron MC, Tournier F. Sinefungin and taxol effects on cell cycle and cytoskeleton of Leishmania donovani promastigotes. Exp Cell Res (1996) 226:283–91. doi: 10.1006/excr.1996.0229
47. Uppuluri S, Knipling L, Sackett DL, Wolff J. Localization of the colchicine-binding site of tubulin. Proc Natl Acad Sci USA (1993) 90(24):11598–602. doi: 10.1073/pnas.90.24.11598
48. Werbovetz KA, Brendle JJ, Sackett DL. Purification, characterization, and drug susceptibility of tubulin from Leishmania. Mol Biochem Parasitol (1999) 98(1):53–65. doi: 10.1016/s0166-6851(98)00146-7
49. Li W, Sun H, Xu S, Zhu Z, Xu J. Tubulin inhibitors targeting the colchicine binding site: a perspective of privileged structures. Future Medicinal Chem (2017) 9(15):1765–94. doi: 10.4155/fmc-2017-0100
50. Luis L, Serrano ML, Hidalgo M, Mendoza-León A. Comparative analyses of the β- tubulin gene and molecular modeling reveal molecular insight into the colchicine resistance in kinetoplastids organisms. BioMed Res Int (2013) 2013:843748. doi: 10.1155/2013/843748
51. Guex N, Peitsch MC. SWISS-MODEL and the Swiss-PdbViewer: an environment for comparative protein modeling. Electrophoresis (1997) 18(15):2714–23. doi: 10.1002/elps.1150181505
52. Meier A, Erler H, Beitz E. Targeting channels and transporters in protozoan parasite infections. Front Chem (2018) 6:88. doi: 10.3389/fchem.2018.00088
53. Jimenez V, Mesones S. Down the membrane hole: Ion channels in protozoan parasites. PloS Pathog (2022) 18(12):e1011004. doi: 10.1371/journal.ppat.1011004
54. Woodbury DJ. Nystatin/ergosterol method for reconstituting ion channels into planar lipid bilayers. Methods Enzymol (1999) 294:319–39. doi: 10.1016/s0076-6879(99)94020-x
55. DiFranco M, Villaroel A, Ponte-Sucre A, Quiñonez M, Drujan D, Dagger F. Incorporation of ion channels from the plasma membrane of L. mexicana into planar bilayers. Acta Cient Venez (1995) 45:206–7.
56. Picci G, Marchesan S, Caltagirone C. Ion channels and transporters as therapeutic agents: from biomolecules to supramolecular medicinal chemistry. Biomedicines (2022) 10(4):885. doi: 10.3390/biomedicines10040885
57. Boozhmehrani MJ, Eslami G, Khamesipour A, Jafari AA, Vakili M, Hosseini SS, et al. The role of ATP-binding cassette transporter genes expression in treatment failure cutaneous leishmaniasis. AMB Express (2022) 12(1):78. doi: 10.1186/s13568-022-01419-5
58. Légaré D, Papadopoulou B, Roy G, Mukhopadhyay R, Haimeur A, Dey S, et al. Efflux systems and increased trypanothione levels in arsenite-resistant Leishmania. Exp Parasitol (1997) 87(3):275–82. doi: 10.1006/expr.1997.4222
59. Padrón-Nieves M, Díaz E, Machuca C, Romero A, Ponte-Sucre A. Glibenclamide modulates glucantime activity and disposition in Leishmania major. Exp Parasitol (2009) 121(4):331–7. doi: 10.1016/j.exppara.2008.12.008
60. Ponte-Sucre A, Figarella K, Moll H. Experimental leishmaniasis: the glibenclamide-triggered decrease in parasite growth correlates with changes in macrophage features. Immunopharmacol Immunotoxicol (2001) 23(3):477–86. doi: 10.1081/iph-100107345
61. Silva N, Camacho N, Figarella K, Ponte-Sucre A. Cell differentiation and infectivity of Leishmania mexicana are inhibited in a strain resistant to an ABC-transporter blocker. Parasitology (2004) 128(Pt 6):629–34. doi: 10.1017/s0031182004005098
62. Orué A, Pérez JL, Fuentes J, Odremán I, Serrano-Martín X, Mendoza-León A. Leishmania sp.: efecto de la glibenclamida, un bloqueador de canales de K+ATP, sobre el ciclo de vida in vitro. Salus (2007) 11(1):32–6.
63. Serrano-Martín X, Payares G, Mendoza-León A. Glibenclamide, a blocker of K+(ATP) channels, shows antileishmanial activity in experimental murine cutaneous leishmaniasis. Antimicrob Agents Chemother (2006) 50(12):4214–6. doi: 10.1128/AAC.00617-06
64. El-Din SS. Immunomodulatory role of ATP inhibitor: glibenclamide and its impact on the pathogenesis of murine Leishmania major infection. Parasitologists United J (2015) 8:68. doi: 10.4103/1687-7942.163415
65. Ponte-Sucre A, Campos Y, Vasquez J, Moll H, Mendoza-León A. Sensitivity of Leishmania spp. to glibenclamide and 4-aminopyridine: a tool for the study of drug resistance development. Mem Inst Oswaldo Cruz (1997) 92:601–6. doi: 10.1590/s0074-02761997000500007
66. Ponte-Sucre A, Mendoza-León A, Moll H. Experimental leishmaniasis: synergistic effect of ion channel blockers and interferon-gamma on the clearance of Leishmania major by macrophages. Parasitol Res (2001) 87(1):27–31. doi: 10.1007/s004360000290
67. Machuca C, Rodríguez A, Herrera M, Silva S, Ponte-Sucre A. Leishmania amazonensis: metabolic adaptations induced by resistance to an ABC transporter blocker. Exp Parasitol (2006) 114(1):1–9. doi: 10.1016/j.exppara.2006.02.008
68. García N, Figarella K, Mendoza-León A, Ponte-Sucre A. Changes in the infectivity, pyruvate kinase activity, acid phosphatase activity and P-glycoprotein expression in glibenclamide-resistant Leishmania mexicana. Parasitol Res (2000) 86(11):899–904. doi: 10.1007/s004360000257
69. Uzcategui NL, Figarella K, Camacho N, Ponte-Sucre A. Substrate preferences and glucose uptake in glibenclamide-resistant Leishmania parasites. Comp Biochem Physiol C Toxicol Pharmacol (2005) 140(3-4):395–402. doi: 10.1016/j.cca.2005.04.002
70. Ponte-Sucre A. Availability and applications of ATP-binding cassette (ABC) transporter blockers. Appl Microbiol Biotechnol (2007) 76(2):279–86. doi: 10.1007/s00253-007-1017-6
71. Ponte-Sucre A, Padrón Nieves M, Díaz E. ABC transporter blockers and reversal of drug resistance in microorganisms. In: Ponte-Sucre A, editor. ABC transporters in microorganisms: Research, Innovation and Value as Targets against Drug Resistance. Norwich, UK: Horizon Scientific Press (2009). p. 177–95.
72. Lewinson O, Orelle C, Seeger MA. Structures of ABC transporters: handle with care. FEBS Lett (2020) 594(23):3799–814. doi: 10.1002/1873-3468.13966
73. Silva M, Calçada C, Osório N, Baptista V, Thathy V, Teixeira M, et al. The Plasmodium falciparum protein PfMRP1 functions as an influx ABC transporter. Res Square (2022). doi: 10.21203/rs.3.rs-1210682/v1
74. Ramos MJ, Fernandes PA. Structure, dynamics, and energetics of ATP hydrolysis by ABC transporters. ACS Cent Sci (2018) 4(10):1300–2. doi: 10.1021/acscentsci.8b00631
75. ABC Transporters and Cancer Drug Resistance. Available at: https://www.sigmaaldrich.com/VE/es/technical-documents/technical-article/research-and-disease-areas/cancer-research/abc-transporters-and-cancer-drug-resistance.
76. Perez-Tomas R. Multidrug resistance: retrospect and prospects in anti-cancer drug treatment. CMC (2006) 13(16):1859–76. doi: 10.2174/092986706777585077
77. McDevitt CA, Callaghan R. How can we best use structural information on P-glycoprotein to design inhibitors? Pharmacol Ther (2007) 113(2):429–41. doi: 10.1016/j.pharmthera.2006.10.003
78. Teicher BA. Cancer drug resistance. In: Cancer Drug Discovery and Development. Totowa, NJ, EE.UU: Humana Press (2006). doi: 10.1007/978-1-59745-035-5
79. Leonard GD, Polgar O, Bates SE. ABC transporters and inhibitors: new targets, new agents. Curr Op Investigational Drugs (2002) 3(11):1652–9.
80. Stefan SM, Jansson PJ, Pahnke J, Namasivayam V. A curated binary pattern multitarget dataset of focused ATP-binding cassette transporter inhibitors. Sci Data (2022) 9(1):446. doi: 10.1038/s41597-022-01506-z
81. Higgins CF. Multiple molecular mechanisms for multidrug resistance transporters. Nature (2007) 446:749–57. doi: 10.1038/nature05630
82. Vanaerschot M, Dumetz F, Roy S, Ponte-Sucre A, Arevalo J, Dujardin JC. Treatment failure in leishmaniasis: drug-resistance or another (epi-) phenotype? Expert Rev Anti Infect Ther (2014) 12(8):937–46. doi: 10.1586/14787210.2014.916614
Keywords: leishmaniasis, target, drug, treatment, tubulin, modeling, glibenclamide
Citation: Mendoza-León A, Serrano G. ML and Ponte-Sucre A (2023) Challenges in drug discovery and description targeting Leishmania spp.: enzymes, structural proteins, and transporters. Front. Trop. Dis 4:1241044. doi: 10.3389/fitd.2023.1241044
Received: 16 June 2023; Accepted: 06 October 2023;
Published: 30 November 2023.
Edited by:
Gabriela Santos-Gomes, New University of Lisbon, PortugalReviewed by:
Claudia Jassica Moreno, New University of Lisbon, PortugalCecilia Parodi, Universidad Nacional de Salta , Argentina
Copyright © 2023 Mendoza-León, Serrano G. and Ponte-Sucre. This is an open-access article distributed under the terms of the Creative Commons Attribution License (CC BY). The use, distribution or reproduction in other forums is permitted, provided the original author(s) and the copyright owner(s) are credited and that the original publication in this journal is cited, in accordance with accepted academic practice. No use, distribution or reproduction is permitted which does not comply with these terms.
*Correspondence: Alexis Mendoza-León, YW1lbmRvemE1MEBnbWFpbC5jb20=; María Luisa Serrano G., bWFyaWEuc2VycmFub0B1Y3YudmU=; Alicia Ponte-Sucre, YWlwb250ZUBnbWFpbC5jb20=