- Department of Entomology, Texas Agricultural and Mechanical (A&M) University, College Station, TX, United States
Efforts to eradicate mosquito-borne diseases have increased the demand for genetic control strategies, many of which involve the release of genetically modified (GM) mosquito males into natural populations. The first hurdle for GM males is to compete with their wild-type counterparts for access to females. Here, we introduce an eye color-based mating assay, in which both Lvp wild-type and kynurenine 3-monooxygenase (kmo)-null males compete for access to kmo-null females, and therefore the eye color phenotype (black or white) of the progeny is dependent on the parental mating pair. A series of tests addressed that male mating competitiveness between the two strains can significantly be influenced by adult density, light intensity, and mating duration. Interestingly, the mating competitiveness of males was not correlated with body size, which was negatively influenced by a high larval density. Lastly, this eye color-associated assay was applied to characterize GM mosquitoes in their mating competitiveness, establishing this method as a fast and precise way of benchmarking this fitness parameter for laboratory-raised males.
Introduction
Aedes aegypti is a prevalent vector of arboviral diseases and is responsible for infectious diseases worldwide, with a majority of the population at risk for infection (1). Pathogens transmitted by Aedes aegypti include Zika, yellow fever, dengue, and chikungunya viruses, and infection with these pathogens inflicts a substantial medical and socioeconomic burden upon developing countries. The absence of highly effective, affordable vaccines or antiviral treatments against some of these viruses and the mosquito’s increasing resistance to pesticides has heightened the need for fundamental methods of vector-borne disease regulation, such as genetic control approaches (2, 3).
For the past 20 years, various genetics-based strategies have been developed to control vector mosquito populations to prevent the transmission of mosquito-borne diseases (4–9). In particular, gene drive-based approaches are hypothesized to be highly efficient in spreading synthetic effector genes (5). The development of the Clustered Regularly Interspaced Short Palindromic Repeats (CRISPR)/CRISPR-associated protein 9 (Cas9) system dramatically accelerated the technical development of gene drive approaches in Anopheles and Aedes families for either eradicating the target species or replacing vectors with novel traits that would prevent disease transmission (10–15). Genetic control strategies for mosquitoes are based on the release of laboratory-generated, genetically modified (GM) mosquitoes to reduce the wild species or inactivate disease-transmitting ability (16, 17). In order to implement these technologies effectively, it is a prerequisite to understand the biology of the target mosquito species (e.g., development, mating behaviors, and reproduction) and evaluate if the engineered genetic components and effectors would also enable the GM mosquitoes to survive in their natural populations and successfully pass the intended trait to their offspring.
One of the limiting aspects of genetics-based population control is the mating competitiveness of transgenic or sterile male mosquitoes (18, 19). Aedes aegypti males are developmentally competent for mating around 24 hours post emergence and swarm near blood-meal sources around sunrise and sunset (20, 21). Males locate and orient to females harmonizing to the flight tone emissions of females (22, 23). These brief pre-copulatory interactions (<1 min) typically end in either a successful synchronized mating flight or decisive rejection by the female (24). In terms of mating frequencies, males can inseminate multiple females while females mate only once and store enough sperm for their lifetime breeding (20). Given this male-skewed operational sex ratios (OSRs) in a swarm, males likely go through interference- or scrambling-based competition for the limited number of females (23, 25).
The mating competence of mosquitoes can be measured by the independent pairing of individual males and females (26, 27) or large-scaled crossing in generations (13). Here, we attempted to establish a laboratory assay to evaluate mating success of competing wild-type Ae. aegypti mosquitoes and a kmo-null strain (28) that has white eyes due to lack of pigments. Offspring of these crosses displayed eye color patterns that directly indicated the parental lineage. Thus, the eye color-based assay provides an efficient tool for evaluating the mating competitiveness of GM mosquito males in laboratory populations.
Materials and methods
Mosquito strains and standard rearing conditions
The Aedes aegypti Liverpool (Lvp) wild-type strain was maintained at 27°C and 70% ( ± 10%) relative humidity, with a day/night cycle of 14-hr light and 10-hr dark. Fertilized eggs were hatched in the pan filled with 500 mL of distilled water and 200 mg of powdered fish food (TetraMin Tropical Flakes). At the L1 instar stage, larvae were manually counted to be ~500 per pan and replenished with fresh water and food every two days. In the pupation period, pupae were manually picked up and separated with sex distinction by using a size-based sorter and/or by identifying genitalia under the dissecting microscope. Adult mosquitoes were fed 10% sucrose solution and mated in a 1-to-3 ratio of male-to-female. The mated females were fed on defibrinated sheep blood (Colorado Serum Company) using an artificial membrane feeder and oviposited on a wet-filter paper kept in a cup of ~30 ml of distilled water. The egg papers were dehydrated and sealed in a plastic bag for up to 4 months of storage at room temperature.
In addition to Lvp, this study includes gene knockout or transgenic strains. The kmo-null strain (28) is the TALEN-generated gene knockout strain for the kynurenine 3-monooxygenase (kmo) gene. The nos-I-SceI strain (29) is the Mariner Mos1-driven transgenic mosquito that express the I-SceI nuclease under the activity of germline-specific nanos promoter (30, 31) and has the genetic background of kmo-null (kmo-/-). The strnΔ41/+ strain is the CRISPR/Cas9-driven gene knockout mosquito line for Aedes aegypti stretchin, a pupae-specific muscle gene (32) and has the genetic background of wild-type kmo (kmo+/+).
Standard mating competition assay
Aedes aegypti Lvp (kmo+/+, black eye) males and kmo-null (kmo-/-, white eye) males were utilized as marker strains, which allow us to determine which males had access to kmo-null females for successful mating. For a standard condition, a triplicated mating experiment was set up consisting of 25 kmo-null males, 25 Lvp males, and 50 virgin kmo-null females in a 306.8 in3 enclosure with the 1:1 number ratio of males and females. Adults were chosen at 3 – 5 days post-eclosion for male mating competitiveness tests. For the synchronous mating condition, the two groups of males were firstly settled in the enclosure, and then the virgin females were introduced. The mating period was given for 24 hours in the growth chamber with an indoor light intensity of ~75 lux. The females were blood-fed 3 days after mating and were allowed to oviposit individually in a 24-well tissue culture plate with a 2% low melting point agarose via the EAgaL plate method (33). The oviposited embryos were hatched in the EAgaL plate, and all F1 offspring larvae at L2/3 instar stages were scored for eye colors under the dissecting microscope.
Mating competition assays with modified conditions
In addition to the initial test condition, various environmental factors were applied for potential effects on mosquito mating. First, a distance effect was tested by using a larger cage (1,728 in3). Second, for adult density-dependent mating efficiency, we set up three separate mating enclosures (306.8 in3, 170 oz.) containing either 50 males and 50 females (Density = 0.33/in3, 0.6/oz), 125 males and 125 females (Density = 0.98/in3, 1.8/oz.), or 250 males and 250 females (Density = 1.63/in3, 2.9/oz.). Third, a light intensity of ~515 lux was tested for the dusk/dawn-dependent mating competition for various time courses such as 30 minutes, 5 hours, and 24 hours. After each mating time allowed, all males were taken out from the enclosure, and females were kept in a growth chamber with a light intensity of ~75 lux.
The density-dependent larval-pupal growth test
Lvp (kmo+/+) and kmo-null (kmo-/-) embryos were hatched under vacuum to ensure that all larvae were the same age. The L1 larval instars were manually counted for low density (LD: 100 larvae per group) or high density (HD: 1,000 larvae per group) and placed into their respective pans filled with 500 mL of distilled water and 200 mg of fish food. Larval instars were kept in an incubation chamber with replenishment of water and food every two days. As larval growth is delayed by high density and competition to the limited food source, we hatched embryos of the HD groups 5 days earlier than those of the LD groups. This enabled the acquisition of adult mosquitoes at similar ages from different larval growth conditions. For the determination of larval/pupal growth rates, numbers of live/dead larvae/pupae were counted every day starting from 3 days after hatching (DAH), until they finished pupation. Photos were taken of pupae from each experimental group using a camera attachment (Leica M165 FC). Pupal sizes were determined by measuring the longitudinal length of the thorax by using ImageJ.
Results
The kmo-dependent eye pigmentation is an efficient trait for an assay that determines male’s mating competitiveness
In order to evaluate the mating competency of male mosquitoes under various laboratory conditions, we first developed a mating assay with the use of the Ae. aegypti Lvp wild-type (kmo+/+) strain and a kmo-null (kmo-/-) strain that has white eyes due to no pigmentation (28). In the mating assay (Figure 1A), we allowed both wild-type and kmo-null males to compete for access to kmo-/- females with a 1:1 ratio and determined mating pairs by scoring the eye color phenotype of the offspring produced from individual females. If a WT male mates successfully with a kmo-null female, the female should produce offspring with black eyes due to the heterozygous genotype (kmo+/-). However, mating between males and females of the kmo-null strain, 100% of offspring should display white eyes (kmo-/-). Finally, if a kmo-null female mates with both Lvp and kmo-null males, her offspring would have mixed phenotypes of eye color.
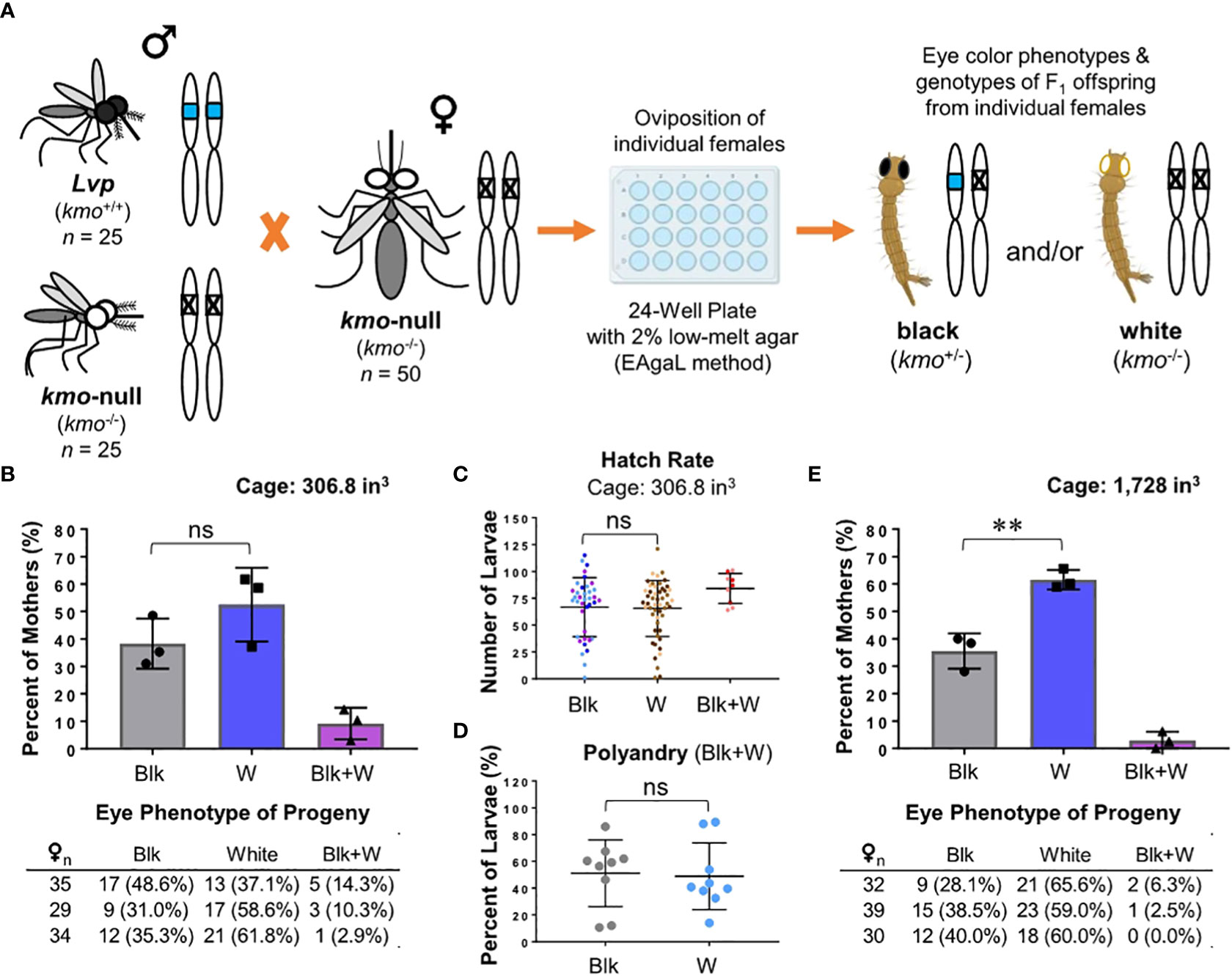
Figure 1 The eye pigmentation-based mating competition assay. (A) Illustration of the workflow for the mating competition assay by utilizing both kmo-null (kmo-/-) and Lvp wild-type (kmo+/+) males for mating with kmo-null (kmo-/-) female mosquitoes. Following blood feeding, females are placed individually and allowed to oviposit in a 24-well plate with a 2% low melting point agarose. For F1 larvae per female, eye color is examined to determine which male successfully mated with each kmo-null female; black-eyes (kmo+/-) from WT male and white-eyes (kmo-/-) from a kmo-null male. (B-D) The standard mating competition assay. Mosquitoes, obtained from the standard rearing conditions (see Materials and Methods), were tested at 3 – 5 days post eclosion for mating competitiveness in the enclosure with dimensions of 306.8 in3 under the indoor light intensity (~75 lux), an adult density of 0.33 mosquitoes/in3 (25 Lvp males + 25 kmo-null males + 50 kmo-null females), and 24-hr mating duration (see Materials and Methods). Phenotypes of F1 offspring were scored to determine parental mating pairs with the kmo-null mother: Blk, black eye (Lvp father); W, white eye (kmo-null father); Blk+W, a combination of both. Experimental data were obtained from triplicated tests. Tukey’s multiple comparisons test (1-way ANOVA): ns (no significance), P>0.05. (B) Phenotypes of F1 offspring were obtained from individual F0 females. Each bar represents the percentage of mothers that produced progeny of parental mating pair-specific eye colors. Auxiliary tables contained number counts of F0 females. (C) Mating pair-dependent female fecundity was determined by the number of eggs laid by individual females. Each dot represents an individual female. The different colors in a cluster represent which replicate the female originated from. (D) For F1 larvae produced by polyandrous females (Blk+W), potential post-mating competitiveness between the two genotypes of sperm was examined based on the proportions of eye color phenotypes. (E) The standard mating competition assay was performed in a larger enclosure (1,728 in3) with a lower adult density (0.058 mosquitoes/in3). Tukey’s multiple comparisons test (1-way ANOVA): **, P<0.01.
As the first step of optimization, we tested the mating competition described above in a 306.8 in3 enclosure for the 24-hr mating period (Figure 1B). Based on eye color phenotypes of F1 larvae, there was no difference in the number of females that mated with either Lvp or kmo-null males. In addition to mating competitiveness, our assay enables us to determine post-mating effects on female reproduction. If the males of a test strain carry a sterile genetic element, the mate pair would cause the female to produce low numbers of F1 offspring larvae. Our result showed that both Lvp and kmo-/- descendant F1 groups had identical larval hatching rates (Figure 1C). Interestingly, we observed the mixed phenotype of F1 offspring from around 10% of females (Figure 1B). The true rate of polyandry is predicted to be higher than that due to stealth events from mating pairs of an identical genotype, much as those (>10%) observed in a previous semi-field test (34). When we scored the mixed phenotypes of F1 larvae produced by polyandrous females, the phenotypic proportions (black eye vs. white eye) were identical (Figure 1D), indicating no potential post-mating competitiveness between the two strains. When this experiment was repeated in a larger enclosure (1,728 in3) (Figure 1E), the kmo-null males appeared to out-compete the Lvp males for mating the females by ~25%, which might contribute to a relatively low rate (~5%) of mixed mating events, suggesting that mosquito mating behaviors may be related to adult density. Taken together, the kmo-null males appeared to be similar to wild-type concerning reproductive fitness in our mating competition test with adult numbers of 50♂+50♀ in a 306.8 in3 enclosure for the 24-hr mating period.
Effects of adult density, light intensity, and mating duration on the eye color-dependent mating competition assay
Within a natural swarm, males go through an extensively interactive, scrambling-based competition for the limited number of females, and a mating pair forms shortly after females enter the swarm (23–25). For this condition, we tested to see if variable adult density may affect the mating competency of the kmo-null males and the rate of polyandrous mating events. We set up 3 separate mating enclosures with various adult mosquito densities (Figure 2): Group 1, ♂ (25 Lvp + 25 kmo-null) + ♀ (50 kmo-null) in 306.8 in3 (0.33/in3); Group 2, ♂ (75 Lvp + 75 kmo-null) + ♀ (150 kmo-null) in 306.8 in3 (0.98/in3); Group 3, ♂ (125 Lvp + 125 kmo-null) + ♀ (250 kmo-null) in 306.8 in3 (1.63/in3). Overall, no difference of mating proportions was observed in diverse density conditions, with Group 2 showing higher mating competitiveness of WT males, and all experimental groups showed a rate of polyandry of ~20 – 30% (Figure 2A). However, there were significant biases in phenotypic proportions of F1 offspring produced by polyandrous females, when adult numbers increased above 300 mosquitoes in 306.8 in3 (0.98/in3) (Figure 2B). This result indicates that a high adult density in a cage-based test may cause a bias of post-mating for different types of sperm.
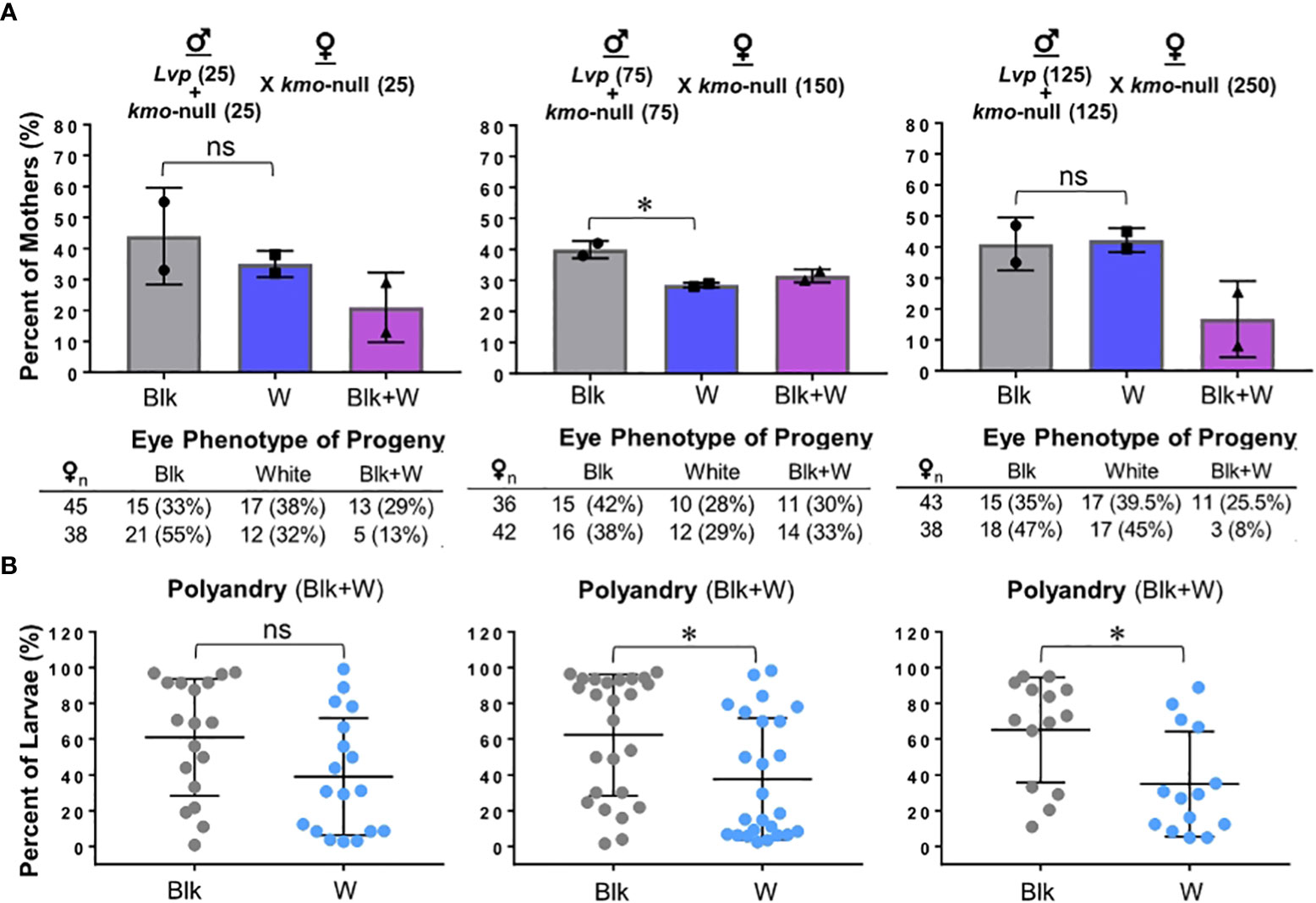
Figure 2 The adult density-dependent mating competition assay. Mosquitoes, obtained from the standard rearing conditions (see Materials and Methods), were tested at 3 – 5 days post eclosion for mating competitiveness in the standard test conditions (see Materials and Methods) with modification of adult numbers in the enclosure with dimensions of 306.8 in3: 100 adults (0.33 mosquitoes/in3); 300 adults (0.98 mosquitoes/in3); 500 adults (1.63 mosquitoes/in3). Phenotypes of F1 offspring were scored to determine parental mating pairs with the kmo-null mother: Blk, black eye (Lvp father); W, white eye (kmo-null father); Blk+W, a combination of both. Experimental data were obtained from duplicated tests. Tukey’s multiple comparisons test (1-way ANOVA): *, P<0.05; ns (no significance), P>0.05. (A) Phenotypes of F1 offspring were obtained from individual F0 females. Each bar represents the percentage of mothers that produced progeny of parental mating pair-specific eye colors. Auxiliary tables contained number counts of F0 females. (B) For F1 larvae produced by polyandrous females (Blk+W), potential post-mating competitiveness between the two genotypes of sperm was examined based on the proportions of eye color phenotypes.
While the formation of swarms and mating pairs can occur throughout the day, peak activity is at dusk and immediately the following dawn, and Ae. aegypti swarming is facultative and occurs in micro-swarms (20). Therefore, we also examined how light intensity and duration would affect the mating behavior of males. Three enclosures were set up by containing 30 Lvp males, 30 kmo-null males, and 60 kmo-null females under the light intensity of dawn/dusk (~515 lux) and were given the opportunity to mate for 30-min, 5-hr, or 24-hr after which all male mosquitoes were removed (Figure 3). We did not observe obvious differences in the proportion of females mating with WT or kmo-null males in all three groups, with the 24-hr group showing higher mating competitiveness of kmo-null males. (Figure 3A). This result suggests that dawn/dusk light intensity may influence the mating behavior because no difference of mating competitiveness was identified in the standard test conditions using the indoor light intensity (75 lux) for the 24-hr mating duration (Figures 1B and 2A). All three groups had a rate of polyandry between ~7 – 14% (Figure 3A). We also scored the rates of oviposition and embryo hatching from individual females in each group (Figure 3B). In the 30-min mating group, only about a half of females were able to lay eggs, and of these most hatched to larvae. In contrast, in 5-hr and 24-hr mating groups, the rates of both oviposition and embryo hatching increased. Moreover, the 30-min mating group had unequal proportions of post-mating in the polyandrous female group (Figure 3C). Overall, this result suggests that a 5-hr window is sufficient to assess competition of male mosquitoes for access to females.
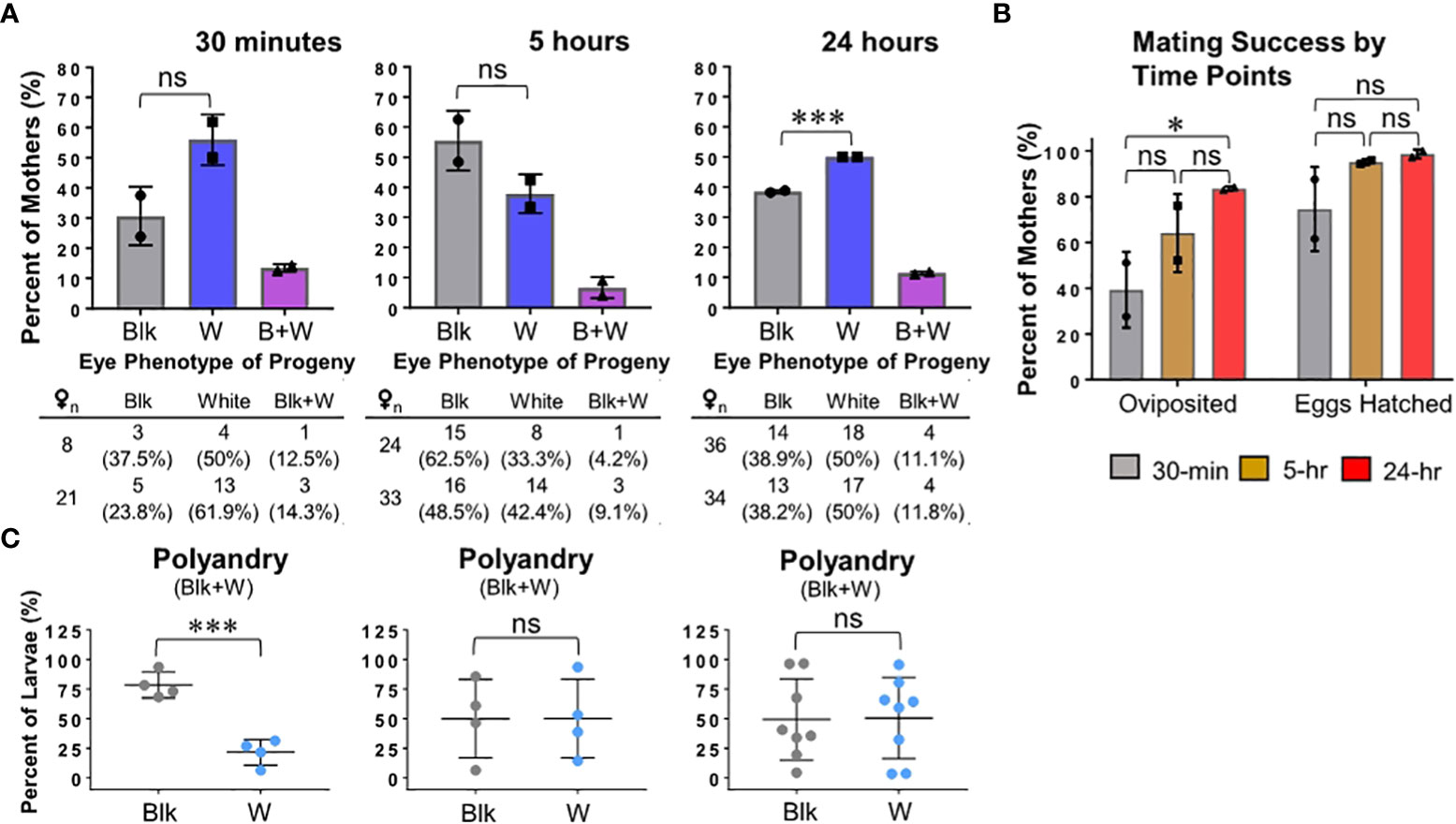
Figure 3 The mosquito mating competition assay under the dawn/dusk light intensity and various durations of mating. Mosquitoes, obtained from the standard rearing conditions (see Materials and Methods), were tested at 3 – 5 days post eclosion for mating competitiveness in the standard test conditions (see Materials and Methods) with modification of light intensity (~515 lux) and mating duration (30-min, 5-hr, or 24-hr). Phenotypes of F1 offspring were scored to determine parental mating pairs with the kmo-null mother: Blk, black eye (Lvp father); W, white eye (kmo-null father); Blk+W, a combination of both. Experimental data were obtained from duplicated tests. Tukey’s multiple comparisons test (1-way ANOVA): ***, P<0.001; ns (no significance), P>0.05. (A) Phenotypes of F1 offspring were obtained from individual F0 females. Each bar represents the percentage of mothers that produced progeny of parental mating pair-specific eye colors. Auxiliary tables contained number counts of F0 females. (B) Mating duration-associated reproduction success was determined by the rates of ovipositing females and egg-hatching. (C) For F1 larvae produced by polyandrous females (Blk+W), potential post-mating competitiveness between the two genotypes of sperm was examined based on the proportions of eye color phenotypes.
High larval density retarded pupal morphogenesis but did not affect the mating competence of adult males
Mosquito body size is correlated with reproductive success: Larger females and males are generally associated with higher fecundity and sperm production capacity, respectively (35, 36). Meanwhile, males of intermediate size are often the most successful in swarming (37), and limited sugar feeding negatively correlates with male mating success (38). Here, we further tested how the quality of the juvenile habitat would affect the body size and the mating competitiveness of the adult males (Figure 4). WT and kmo-null mosquito larvae were raised under two different conditions: low density (LD, 100 larvae in 0.5 L) or high density (HD, 1,000 larvae in 0.5 L). Compared to LD, the body sizes of the HD mosquitoes were smaller, potentially due to higher larval competition for a limited amount of the food source (Figure 4A). Also, the high larval density-associated stress significantly delayed the larva-to-pupa transition with pupation peaks 4 days later than those of the LD groups (Figure 4B). However, when HD males competed with LD males for mating with LD females, there was no significant difference identified, based on mating success with females (Figure 4C) and post-mating proportions per individual polyandrous female (Figure 4D). This result showed that small-sized males, resulted from the high larval density conditions, were fully capable of mating competition with their LD-grown larger counterparts. This indicates that body size itself is not a critical factor for successful mate competition in the laboratory, coinciding with previously obtained results (39). Intriguingly, when both LD males competed for mating with LD females, kmo-null males had higher mating competitiveness than Lvp (Figure 4C). This suggests that low larval density-associated nutritional enrichment might have been related to differential mating competitiveness, as the two strains were identical when they were reared in the standard protocol (500 larvae in 0.5 L of water) (Figures 1B and 2A).
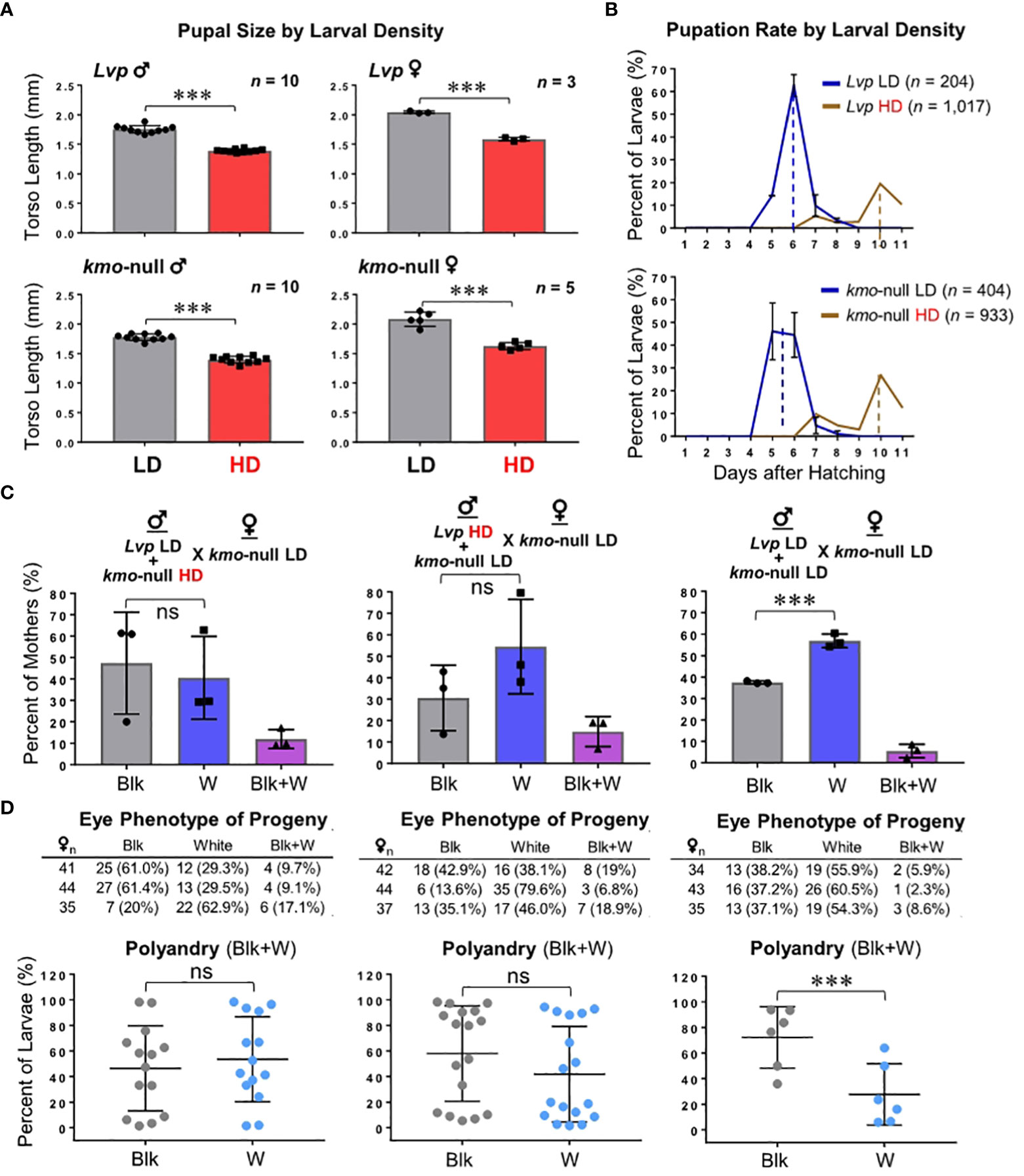
Figure 4 The effects of larval growth conditions on male mating competitiveness. Two groups of adults were obtained from low larval density (LD: 100 larvae in 0.5 L of water) and high larval density (HD: 1,000 larvae in 0.5 L of water), compared to the standard conditions of mosquito rearing in our laboratory (see Materials and Methods). Mosquitoes were tested at 3 – 5 days post-eclosion for mating competitiveness in the standard test conditions (see Materials and Methods). Phenotypes of F1 offspring were scored to determine parental mating pairs with the kmo-null mother: Blk, black eye (Lvp father); W, white eye (kmo-null father); Blk+W, a combination of both. Experimental data were obtained from triplicated tests. Tukey’s multiple comparisons test (1-way ANOVA): ***, P<0.001; ns (no significance), P>0.05. (A) Comparison of pupal sizes of kmo-null (kmo-/-) or Lvp wild-type (kmo+/+) that were grown in the two larval densities. The longitudinal lengths of the pupal thorax were measured by ImageJ. (B) Comparison of the rate of pupation in the two larval densities. The line graphs represent the daily percentage of pupation out of total larvae at hatching. The dotted lines indicate the pupation peaks for each larval density condition. (C) Phenotypes of F1 offspring were obtained from individual F0 females. Each bar represents the percentage of mothers that produced progeny of parental mating pair-specific eye colors. Auxiliary tables contained number counts of F0 females. (D) For F1 larvae produced by polyandrous females (Blk+W), potential post-mating competitiveness between the two genotypes of sperm was examined based on the proportions of eye color phenotypes.
Evaluation of genetically modified mosquitoes for mating competence
Genetic control approaches, such as precision-guided Sterile Insect Technique (pgSIT), Female-specific Release of Insects carrying Dominant Lethal (FsRIDL), and gene drive, rely on the mating biology of sexually reproductive species (17–19). Evaluating and improving the mating success of laboratory-produced GM mosquito strains is critical for the effectiveness of genetic modification strategies that involve mass release into natural populations (19). We used the eye color-based mating assay to evaluate two GM mosquitoes for their ability to mate in limited competition (Figure 5). The nos-I-SceI strain (29) is a transgenic mosquito expressing the I-SceI homing endonuclease under the activity of the germline-specific nanos promoter (30, 31), which can be the genetic component of a self-eliminating gene drive cassette. The strnΔ41/+ strain is a gene knockout mosquito for a haplo-sufficient flight muscle gene named stretchin, and the homozygous mutation can completely disrupt flight ability of adult mosquitoes and female fertility (32), a promising genetic trait to control mosquito population. WT males were included as the competing counterpart for nos-I-SceI that has the kmo-null genetic background, while kmo-/- males were the competitors of strnΔ41/+ strain that has the wild-type kmo. Both GM males displayed normal competence for mating with females (Figure 5A), and the presence of these modifications did not disrupt post mating biases in polyandrous females (Figure 5B) or reproductive fitness such as female fecundity (Figure 5C) and offspring hatching (Figure 5D). Thus, the current mating assay provides initial laboratory-based experimental evidence that nos-I-SceI and stretchin are promising genetic components and a target gene, respectively, for genetic control approaches with a strategy of massive release of GM mosquitoes.
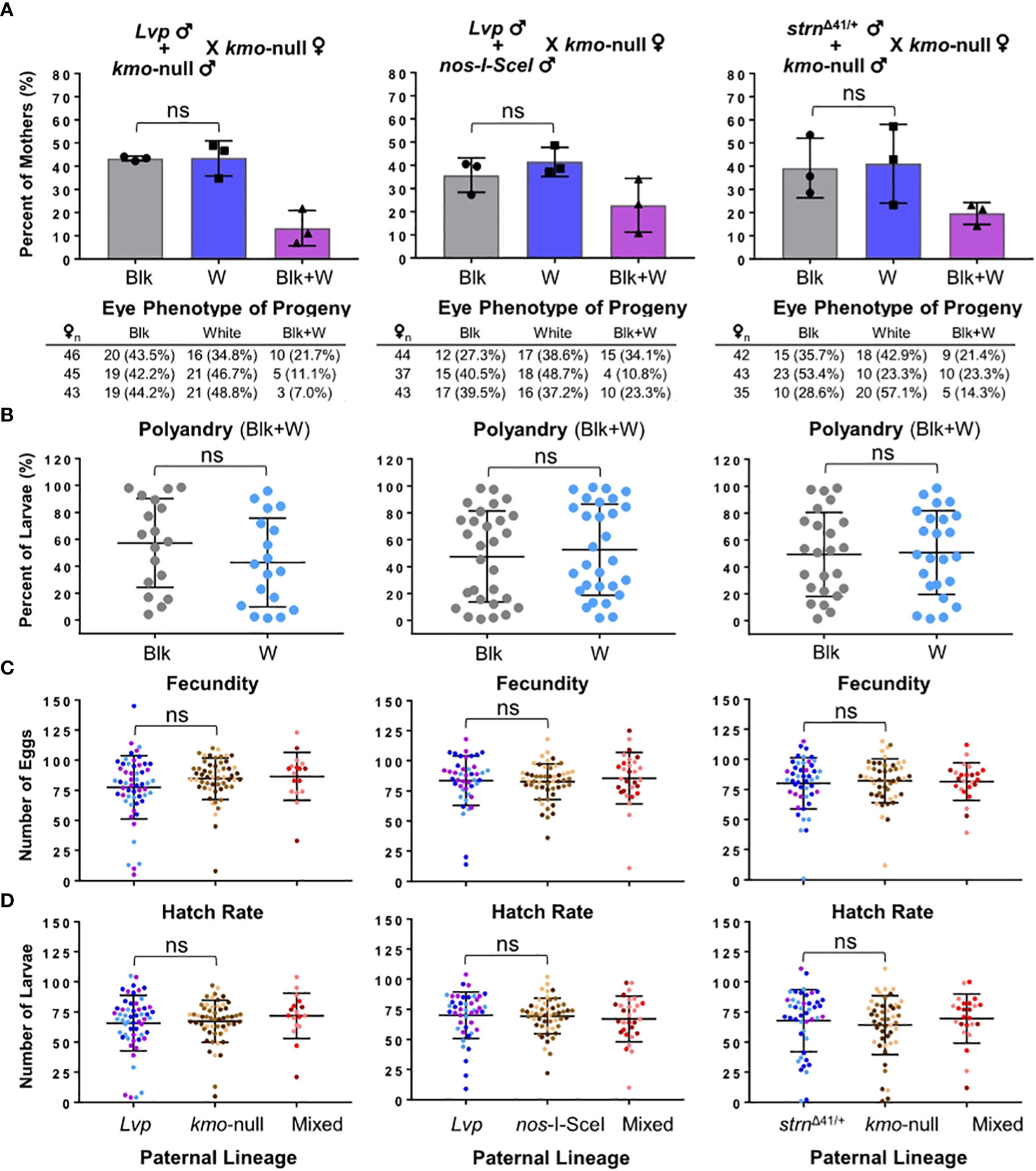
Figure 5 Evaluation of potential effects of a transgene component and a gene knockout on male mating competence. Two GM mosquito strains, nos-I-SceI and strnΔ41/+, were obtained from the standard rearing conditions (see Materials and Methods) and tested at 3 – 5 days post-eclosion for mating competitiveness in the standard test conditions (see Materials and Methods) with the optimized mating duration (5-hr). Phenotypes of F1 offspring were scored to determine parental mating pairs with the kmo-null mother: Blk, black eye (Lvp father); W, white eye (kmo-null father); Blk+W, a combination of both. Experimental data were obtained from triplicated tests. Tukey’s multiple comparisons test (1-way ANOVA): ns (no significance), P>0.05. (A)Phenotypes of F1 offspring were obtained from individual F0 females. Each bar represents the percentage of mothers that produced progeny of parental mating pair-specific eye colors. Auxiliary tables contained number counts of F0 females. (B) For F1 larvae produced by polyandrous females (Blk+W), potential post-mating competitiveness between the two genotypes of sperm was examined based on the proportions of eye color phenotypes. (C, D) Mating pair-dependent female fecundity and fertility were determined by the number of eggs laid by individual females (C) and by the number of larvae that the individual female produced (D), respectively. Each dot represents an individual female. The different colors in a cluster represent which replicate the female originated from.
Discussion
In genetics-based control methods such as Sterile Insect Technique (SIT), Release of Insects carrying a Dominant Lethal (RIDL) and/or gene drive (4–7, 17), the GM organism carries mutations or genetic elements that permit lethality or the genetic trait to rapidly spread to the target species population (5). For the prevention of mosquito-borne diseases, nuclease-based gene drive technologies have been advanced to suppress laboratory populations of disease-transmitting species or render vectors unable to transmit pathogens (4, 40). Recent advances in synthetic biology and gene editing technologies have expedited the development of highly effective gene drive strategies in malaria and dengue mosquitoes (8–15). With respect to unforeseen ecological risks of high invasiveness and self-propagating nature of gene drive (41–45), biosafety technologies have also been developed as numerous systems of local confinement (15), self-exhaustion (46), self-elimination (29), and anti-CRISPR breaks such as CATCHA, e-CHACRs, ERACRs, and the anti-CRISPR AcrIIA4 protein (47–49).
To be field-effective, any genetic elements or modifications in SIT, RIDL or gene drive systems and the nuclease-targeted genomic loci should be appropriately evaluated for their relative fitness (13). Many genetic control programs are based on mosquito releases and sexual reproduction. Therefore, the GM adult males, either generated from the laboratory or emerged from the released eggs, must be successful in competition with their natural counterparts for mates. In this study, we sought to develop an efficient laboratory method to test the mating competitiveness of adult Aedes aegypti mosquitoes. This led to the development of a mating protocol utilizing Ae. aegypti Lvp wild-type and kmo-deficient strains so that one could determine the parental mating pair based upon eye colors (black or white) of the offspring produced by individual females. Based on our results, the mating behavior and sexual reproductive fitness of the white-eyed kmo-null male was almost identical to those of the black-eyed Lvp under the following laboratory conditions: 100 adults with a 1:1 ratio of sexes [♂ Lvp (n = 25) + ♂ kmo-null (n = 25) + ♀ kmo-null (n = 50)]; the enclosure with 306.8 in3; 5 or 12 hours of mating duration; an indoor light intensity of ~75 lux.; a growth chamber of 27°C and 70% ( ± 10%) relative humidity; a day/night cycle of 14-hr light and 10-hr dark; ~500 larvae in a pan of 0.5 L of distilled water. In these test conditions, we were able to examine the sexual reproductive fitness of laboratory-raised males, with respect to larval growth conditions, adult sizes, germline-specific nuclease expression, or heterozygous knockout of a flight muscle gene.
Notably, our results also suggested that there were some test modifications to be further understood for male mating competitiveness between Lvp and kmo-null strains. First, when adult density got lower in a larger size of the enclosure compared to the standard test conditions, the kmo-null males had a higher mating competitiveness. In contrast, the opposite outcome (Lvp > kmo-null) was resulted by a higher density with larger numbers of adults (n = 300). Second, the light intensity of dawn/dusk (~515 lux) increased the mating competitiveness of kmo-null males, when the mating time was given for 24 hours. Third, when both strains were reared in the low larval density conditions (100 larvae per 0.5 L), the kmo-null males had a higher mating competitiveness than Lvp. Although it is premature to interpret how kmo-dependent eye pigmentation or gene function would be related to mating behaviors of mosquito males, various environmental factors are expected to be further tested to develop a more promising tool for screening field-optimized GM strains. Indeed, the current mating competition assay should be evaluated for its capability to rapidly screen for any non-competitive GM strains, and this would be further optimized with potential mutant strains of aberrant mating behaviors.
As compared to an individual mate pairing procedure scoring the chosen males one at a time, the eye color-based assay allows for evaluating the mating competitiveness of males in a swarm-imitating setting in a relatively hands-off fashion. In addition, the individual female oviposition allowed us to measure mating pair-specific oviposition, fecundity, and embryo hatching, which precisely indicate the potential effects of the engineered genetic components on post-mating processes. Interestingly, this method revealed a range of female polyandry based on individual female-produced progeny groups of mixed eye colors. Despite that Ae. aegypti females are thought to be monandrous, multiple paternity can occur in a natural setting (50). In a laboratory setting, ~24% of females showed multiple male crosses right after the first mating, but females become completely refractory to a second mating within 2 hours (51). Similarly, our assay revealed that polyandry was shown to occur in ~5 – 30% of females and presumably even higher given that a mixed paternity by males of an identical eye color cannot be scored. Moreover, potential mating competition in post-mating phases were significantly influenced by increased adult densities per enclosure. Thus, this high rate of multiple paternity should also be considered for diverse cage-based tests of genetic control approaches, and it will be important to identify how it would influence the rates of population modification.
The eye color-based mating competition assay is a very easy, quick, and precise tool for screening gene transmission rates of any transgenic mosquitoes for male releasing programs of mosquito population control, with respect to their mating success against wild-type competitors. This assay is currently limited to Aedes aegypti. However, the conserved role of the kmo gene in eye pigmentation suggests its versatility to other mosquito species. To optimize the assay further for natural populations, it would be worth performing mating competition in a larger cage or a semi-field condition. In addition, the 1:1 number ratio of both sexes can be adjusted in further tests because less females are considered available for mating than males, especially in swarm-like conditions (20, 23, 25). Lastly, this assay can be further validated for mating competitiveness of GM males against natural mosquitoes directly obtained from a target area.
Data availability statement
The original contributions presented in the study are included in the article/supplementary materials. Further inquiries can be directed to the corresponding author.
Author contributions
BC conducted all experiments. BC, ZA, and KC conceived research and experimental design and wrote the manuscript. All authors contributed to the article and approved the submitted version.
Funding
This work was supported by the National Institute of Allergies and Infectious Diseases of the National Institutes of Health (NIH-NIAID) under award numbers (R01AI148787 and R01AI137112), and by the United States Department of Agriculture (USDA) National Institute of Food and Agriculture, Hatch project 1018401. The content is solely the responsibility of the authors and does not necessarily represent the official views of the National Institutes of Health or the USDA.
Acknowledgments
We thank Chanell Dawson and Xiangyu Shi in the Adelman laboratory at Texas A&M University for excellent technical assistance for this study. Some illustrations in Figure 1A were generated using BioRender.com through a license to Texas A&M University.
Conflict of interest
The authors declare that the research was conducted in the absence of any commercial or financial relationships that could be construed as a potential conflict of interest.
Publisher’s note
All claims expressed in this article are solely those of the authors and do not necessarily represent those of their affiliated organizations, or those of the publisher, the editors and the reviewers. Any product that may be evaluated in this article, or claim that may be made by its manufacturer, is not guaranteed or endorsed by the publisher.
References
1. Wilder-Smith A, Gubler DJ, Weaver SC, Monath TP, Heymann DL, Scott TW. Epidemic arboviral diseases: priorities for research and public health. Lancet Infect Dis (2017) 17:e101–6. doi: 10.1016/S1473-3099(16)30518-7
2. Bouzid M, Brainard J, Hooper L, Hunter PR. Public health interventions for aedes control in the time of zikavirus– a meta-review on effectiveness of vector control strategies. PloS Negl Trop Dis (2016) 10:e0005176. doi: 10.1371/journal.pntd.0005176
3. Dahmana H, Mediannikov O. Mosquito-borne diseases emergence/resurgence and how to effectively control it biologically. Pathogens (2020) 9:310. doi: 10.3390/pathogens9040310
4. Alphey L. Can CRISPR-Cas9 gene drives curb malaria? Nat Biotechnol (2016) 34:149–50. doi: 10.1038/nbt.3473
5. Burt A. Heritable strategies for controlling insect vectors of disease. Philos Trans R Soc B Biol Sci (2014) 369:20130432. doi: 10.1098/rstb.2013.0432
6. Sinkins SP, Gould F. Gene drive systems for insect disease vectors. Nat Rev Genet (2006) 7:427–35. doi: 10.1038/nrg1870
7. Burt A. Site-specific selfish genes as tools for the control and genetic engineering of natural populations. Proc R Soc B Biol Sci (2003) 270:921–8. doi: 10.1098/rspb.2002.2319
8. Raban RR, Marshall JM, Akbari OS. Progress towards engineering gene drives for population control. J Exp Biol (2020) 223:jeb208181. doi: 10.1242/jeb.208181
10. Gantz VM, Jasinskiene N, Tatarenkova O, Fazekas A, Macias VM, Bier E, et al. Highly efficient Cas9-mediated gene drive for population modification of the malaria vector mosquito anopheles stephensi. Proc Natl Acad Sci U.S.A. (2015) 112:E6736–43. doi: 10.1073/pnas.1521077112
11. Hammond A, Galizi R, Kyrou K, Simoni A, Siniscalchi C, Katsanos D, et al. A CRISPR-Cas9 gene drive system targeting female reproduction in the malaria mosquito vector anopheles gambiae. Nat Biotechnol (2016) 34:78–83. doi: 10.1038/nbt.3439
12. Kyrou K, Hammond AM, Galizi R, Kranjc N, Burt A, Beaghton AK, et al. A CRISPR–Cas9 gene drive targeting doublesex causes complete population suppression in caged anopheles gambiae mosquitoes. Nat Biotechnol (2018) 36:1062–71. doi: 10.1038/nbt.4245
13. Carballar-Lejarazú R, Ogaugwu C, Tushar T, Kelsey A, Pham TB, Murphy J, et al. Next-generation gene drive for population modification of the malaria vector mosquito, anopheles gambiae. Proc Natl Acad Sci U.S.A. (2020) 117:22805–14. doi: 10.1073/pnas.2010214117
14. Simoni A, Hammond AM, Beaghton AK, Galizi R, Taxiarchi C, Kyrou K, et al. A male-biased sex-distorter gene drive for the human malaria vector anopheles gambiae. Nat Biotechnol (2020) 38:1054–60. doi: 10.1038/s41587-020-0508-1
15. Li M, Yang T, Kandul NP, Bui M, Gamez S, Raban R, et al. Development of a confinable gene drive system in the human disease vector aedes aegypti. Elife (2020) 9:e51701. doi: 10.7554/eLife.51701
16. Alphey L. Genetic control of mosquitoes. Annu Rev Entomol (2014) 59:205–24. doi: 10.1146/annurev-ento-011613-162002
17. Raban R, William ACG, Omar SA. A perspective on the expansion of the genetic technologies to support the control of neglected vector-borne diseases and conservation. Front Trop Dis (2022) 3:999273. doi: 10.3389/fitd.2022.999273
18. South A, Catteruccia F. Sexual selection and the evolution of mating systems in mosquitoes. Adv In Insect Phys (2016) 51:67–92. doi: 10.1016/bs.aiip.2016.04.004
19. Cator LJ, Wyer CAS, Harrington LC. Mosquito sexual selection and reproductive control programs. Trends Parasitol (2021) 37:330–9. doi: 10.1016/j.pt.2020.11.009
20. Oliva CF, Damiens D, Benedict MQ. Male Reproductive biology of aedes mosquitoes. Acta Trop (2014) 132:12–9. doi: 10.1016/j.actatropica.2013.11.021
21. Cator LJ, Harrington LC. The harmonic convergence of fathers predicts the mating success of sons in aedes aegypti. Anim Behav (2011) 82:627–33. doi: 10.1016/j.anbehav.2011.07.013
22. Cator LJ, Arthur BJ, Harrington LC, Hoy RR. Harmonic convergence in the love songs of the dengue vector mosquito. Science (2009) 323:1077–9. doi: 10.1126/science.1166541
23. Andrés M, Su MP, Albert J, Cator LJ. Buzzkill: targeting the mosquito auditory system. Curr Opin Insect Sci (2020) 40:11–7. doi: 10.1016/j.cois.2020.04.003
24. Yuval B. Mating systems of blood-feeding flies. Annu Rev Entomol (2006) 51:413–40. doi: 10.1146/annurev.ento.51.110104.151058
25. Shishika D, Manoukis NC, Butail S, Paley DA. Male Motion coordination in anopheline mating swarms. Sci Rep (2014) 4:6318. doi: 10.1038/srep06318
26. Duvall LB, Basrur NS, Molina H, McMeniman CJ, Vosshall LB. A peptide signaling system that rapidly enforces paternity in the aedes aegypti mosquito. Curr Biol (2017) 27:3734–42. doi: 10.1016/j.cub.2017.10.074
27. Carvalho DO, Chuffi S, Ioshino RS, Marques ICS, Fini R, Costa MK, et al. Mosquito pornoscopy: Observation and interruption of aedes aegypti copulation to determine female polyandric event and mixed progeny. PloS One (2018) 13:e0209146. doi: 10.1371/journal.pone.0209146
28. Aryan A, Anderson MAE, Myles KM, Adelman ZN. TALEN-based gene disruption in the dengue vector aedes aegypti. PloS One (2013) 8:e60082. doi: 10.1371/journal.pone.0060082
29. Chae K, Dawson C, Valentin C, Contreras B, Zapletal J, Myles KM, et al. Engineering a self-eliminating transgene in the yellow fever mosquito, aedes aegypti. PNAS Nexus (2022) 1:pgac037. doi: 10.1093/pnasnexus/pgac037
30. Calvo E, Walter M, Adelman ZN, Jimenez A, Onal S, Marinotti O, et al. Nanos (nos) genes of the vector mosquitoes, anopheles gambiae, anopheles stephensi and aedes aegypti. Insect Biochem Mol Biol (2005) 35:789–98. doi: 10.1016/j.ibmb.2005.02.007
31. Adelman ZN, Jasinskiene N, Onal S, Juhn J, Ashikyan A, Salampessy M, et al. Nanos gene control DNA mediates developmentally regulated transposition in the yellow fever mosquito aedes aegypti. Proc Natl Acad Sci U.S.A. (2007) 104:9970–5. doi: 10.1073/pnas.0701515104
32. Chae K, Valentin C, Dawson C, Jakes E, Myles KM, Adelman ZN. A knockout screen of genes expressed specifically in Ae. aegypti pupae reveals a critical role for stretchin in mosquito flight. Insect Biochem Mol Biol (2021) 132:103565. doi: 10.1016/j.ibmb.2021.103565
33. Tsujimoto H, Adelman ZN. Improved fecundity and fertility assay for aedes aegypti using 24 well tissue culture plates (EAgaL plates). J Vis Exp (2021) 4:171. doi: 10.3791/61232
34. Helinski MEH, Valerio L, Facchinelli L, Scott TW, Ramsey J, Harrington LC. Evidence of polyandry for aedes aegypti in semifield enclosures. Am J Trop Med Hyg (2012) 86:635–41. doi: 10.4269/ajtmh.2012.11-0225
35. Briegel H. Fecundity, metabolism, and body size in anopheles (Diptera: Culicidae), vectors of malaria. J Med Entomol (1990) 27:839–50. doi: 10.1093/jmedent/27.5.839
36. Ponlawat A, Harrington LC. Age and body size influence male sperm capacity of the dengue vector aedes aegypti (diptera: culicidae). J Med Entomol (2007) 44:422–6. doi: 10.1603/0022-2585(2007)44[422:AABSIM]2.0.CO;2
37. Diabate A, Tripet F. Targeting male mosquito mating behaviour for malaria control. Parasites Vectors (2015) 8:347. doi: 10.1186/s13071-015-0961-8
38. Gary RE, Cannon JW, Foster WA. Effect of sugar on male anopheles gambiae mating performance, as modified by temperature, space, and body size. Parasites Vectors (2009) 2:19. doi: 10.1186/1756-3305-2-19
39. Aldersley A, Pongsiri A, Bunmee K, Kijchalao U, Chittham W, Fansiri T, et al. Too “sexy” for the field? paired measures of laboratory and semi-field performance highlight variability in the apparent mating fitness of aedes aegypti transgenic strains. Parasites Vectors (2019) 12:357. doi: 10.1186/s13071-019-3617-2
40. Noble C, Adlam B, Church GM, Esvelt KM, Nowak MA. Current CRISPR gene drive systems are likely to be highly invasive in wild populations. Elife (2018) 7:e33423. doi: 10.7554/eLife.33423
41. Oye KA, Esvelt K, Appleton E, Catteruccia F, Church G, Kuiken T, et al. Regulating gene drives. Science (2014) 345:626–8. doi: 10.1126/science.1254287
42. Akbari OS, Bellen HJ, Bier E, Bullock SL, Burt A, Church GM, et al. Safeguarding gene drive experiments in the laboratory: Multiple stringent confinement strategies should be used whenever possible. Science (2015) 349:927–9. doi: 10.1126/science.aac7932
43. Adelman Z, Akbari O, Bauer J, Bier E, Bloss C, Carter SR, et al. Rules of the road for insect gene drive research and testing. Nat Biotechnol (2017) 35:716–8. doi: 10.1038/nbt.3926
44. Kaebnick GE, Heitman E, Collins JP, Delborne JA, Landis WG, Sawyer K, et al. Precaution and governance of emerging technologies. Science (2016) 354:710–1. doi: 10.1126/science.aah5125
45. James S, Collins FH, Welkhoff PA, Emerson C, J Godfray HC, Gottlieb M, et al. Pathway to deployment of gene drive mosquitoes as a potential biocontrol tool for elimination of malaria in sub-Saharan Africa: Recommendations of a scientific working group. Am J Trop Med Hyg (2018) 98:1–49. doi: 10.4269/ajtmh.18-0083
46. Noble C, Min J, Olejarz J, Buchthal J, Chavez A, Smidler AL, et al. Daisy-chain gene drives for the alteration of local populations. Proc Natl Acad Sci U.S.A. (2019) 116:8275–82. doi: 10.1073/pnas.1716358116
47. Xu XRS, Bulger EA, Gantz VM, Klanseck C, Heimler SR, Auradkar A, et al. Active genetic neutralizing elements for halting or deleting gene drives. Mol Cell (2020) 80:246–62. doi: 10.1016/j.molcel.2020.09.003
48. Wu B, Luo L, Gao XJ. Cas9-triggered chain ablation of cas9 as a gene drive brake. Nat Biotechnol (2016) 34:137–8. doi: 10.1038/nbt.3444
49. Taxiarchi C, Beaghton A, Don NI, Kyrou K, Gribble M, Shittu D, et al. A genetically encoded anti-CRISPR protein constrains gene drive spread and prevents population suppression. Nat Commun (2021) 12:3977. doi: 10.1038/s41467-021-24214-5
50. Richardson JB, Jameson SB, Gloria-Soria A, Wesson DM, Powell J. Evidence of limited polyandry in a natural population of aedes aegypti. Am J Trop Med Hyg (2015) 93:189–93. doi: 10.4269/ajtmh.14-0718
Keywords: Aedes aegypti, mosquito mating, reproductive fitness, vector-borne diseases, genetic control, gene drive, Sterile Insect Techniques (SITs), Release of Insects carrying Dominant Lethal (RIDL)
Citation: Contreras B, Adelman ZN and Chae K (2023) Evaluating the mating competency of genetically modified male mosquitoes in laboratory conditions. Front. Trop. Dis 4:1106671. doi: 10.3389/fitd.2023.1106671
Received: 24 November 2022; Accepted: 30 January 2023;
Published: 13 February 2023.
Edited by:
Adam E. Vorsino, United States Fish and Wildlife Service, United StatesReviewed by:
Robyn Raban, University of California, San Diego, United StatesRobert Tyrrell Jones, University of London, United Kingdom
Copyright © 2023 Contreras, Adelman and Chae. This is an open-access article distributed under the terms of the Creative Commons Attribution License (CC BY). The use, distribution or reproduction in other forums is permitted, provided the original author(s) and the copyright owner(s) are credited and that the original publication in this journal is cited, in accordance with accepted academic practice. No use, distribution or reproduction is permitted which does not comply with these terms.
*Correspondence: Keun Chae, a2V1bi5jaGFlQGFnLnRhbXUuZWR1