- 1Institut Pasteur, Université de Paris Cité, CNRS UMR 2000, Génétique Fonctionnelle des Maladies Infectieuses, Paris, France
- 2Institut Pasteur de la Guyane, Medical Entomology Unit, Cayenne, French Guiana, France
- 3Institut Pasteur de la Guyane, Microbiota of Insect Vectors Group, Cayenne, French Guiana, France
- 4Institut Pasteur, Université de Paris Cité, Department of Parasites and Insect Vectors, Paris, France
Anopheles darlingi is a major vector of both Plasmodium falciparum and Plasmodium vivax in South and Central America including French Guiana. However, the vector competence and physiology of this mosquito species have been scarcely studied due to difficulties in rearing it in the laboratory. Here, we report the successful establishment of a robust colony, from a mosquito collection in French Guiana. We describe our mosquito colonization procedure with relevant information on environmental conditions, mating ability, larval development, and survival, recorded over the first six critical generations. Experimental infection showed that our An. darlingi colony has a moderate permissiveness to in vitro produced gametocytes of the P. falciparum NF54 strain originating from Africa. This colony, which has reached its 21st generation, will allow further characterization of An. darlingi life-history traits and of Plasmodium–mosquito interactions with South American malaria parasites.
Introduction
The establishment of mosquito colonies has been instrumental for a better understanding of many of their biological features including development, insecticide resistance, and pathogen transmission. It is also important to test novel insecticides and novel technologies for population suppression or replacement such as the sterile insect technique, the RIDL approach (Release of Insects carrying Dominant Lethals), the use of symbiont bacteria such as Asaia, or the production of transgenic mosquitoes.
Among mosquito species known as vectors of human pathogens, Anopheles species involved in malaria parasite transmission are the more numerous and diverse worldwide compared to mosquito species involved in arbovirus transmission (1). In addition, Anopheles species have specific reproductive characteristics, notably their eurygamous behavior, i.e., their propensity to mate in swarms and the female refractoriness to multiple mating. Those characteristics have been challenging for the successful establishment of colonies of many Anopheles species. To overcome these limitations, one strategy is the forced mating technique, which relies on the manual adjustment of male and female copulatory structures (2–4). Although tricky and highly demanding in human resources, this technique was successful in maintaining some species as laboratory colonies for many years. The methodology has notably been used to colonize and maintain Anopheles dirus and is still used to create and maintain novel Anopheles colonies (5). Interestingly, some species such as Anopheles albitarsis were reported to change to stenogamous free-mating behavior after several generations of colony maintenance by forced copulation (6).
Anopheles darlingi [also called Nyssorhynchus darlingi (7)] is a major vector of both Plasmodium falciparum and Plasmodium vivax in South and Central America including French Guiana (8, 9). As most anophelines, An. darlingi has a eurygamous behavior that precludes its readiness to mate in captivity. In contrast to many Anopheles species belonging to the Cellia and Anopheles subgenera, it is not clear whether Anopheles belonging to the Nyssorhynchus subgenus exhibit a swarming behavior for mating (10). Indeed, Lounibos et al. reported that young F1 virgin An. darlingi females would mate within 2 to 36 h after being released in a village where no mosquito swarm was evidenced (11). In 61-cm-high cages, Villarreal-Treviño et al. observed pseudo-swarms of An. darlingi occurring 5–40 cm above the cage floor (12). This may be due to space constraints yet corroborates field observations that males fly at low altitude. As An. darlingi is a major malaria vector in South America and particularly in the Amazon basin, several laboratories tried to colonize this mosquito species for vector competence studies and additionally for human Plasmodium sporozoite production for vaccine development studies. The oldest published records of the establishment of a free-mating An. darlingi colony go back to 1947 with An. darlingi from the Co‐operative Republic of Guyana (at that time British Guiana), Colombia, and Brazil (13–15). Since then, two reports of Brazilian (Araraquara, Sao Paulo State) An. darlingi colonies have been published in 1970 and 1988 (16, 17) and followed almost 40 years later with the description of three novel free-mating An. darlingi colonies, two from Peru and one from the Rondonia State (Amazon basin—Brazil) (12, 18, 19).
Here, we describe the establishment of a free-mating colony of An. darlingi from a different South American country, French Guiana. By mixing our expertise in Anopheles mosquitoes with published results and improvements along the most recent established Anopheles pseudopunctipennis and An. darlingi free-mating colonies (12, 18, 20, 21), this novel An. darlingi colony was produced in less than 8 months and has currently reached its 21st generation.
Materials and methods
Producing the F1 generation from wild Anopheles darlingi
Wild An. darlingi were captured in Val’ranch (Tonate Macouria village; 05.01616°N 052.52845W), a location where this species is abundant (22), using two Mosquito Magnet® MM300 Executive traps arranged 50 m apart. The capture was performed over a single night with two catching sessions from 6 p.m. to 8 p.m., and from 8 p.m. to 7 a.m. in September 2020. Female mosquitoes were transferred from the traps into 30-cm3 plastic cages (Bugdorm®) covered with damp towels to maintain high humidity. They were transported to the laboratory (Institut Pasteur de la Guyane, Cayenne, French Guiana) in a foam box to buffer ambient temperature. There, they were fed on anesthetized mice. On the fourth day after feeding, one wing was removed from gravid females to induce oviposition, and the females were transferred to individual Eppendorf tubes containing wet filter paper as an additional forced-egg laying method (23). Once the eggs were laid, the females were removed before air freight transfer of tubes with egg clutches to Institut Pasteur (Paris, France). Initial An. darlingi identification relies on adult morphology characteristics and is further confirmed by COI DNA barcoding on adults from the 21st generation, using the CO1 universal primers designed for invertebrates by Folmer et al. (24) (Supplementary Data).
Insectary parameters
Following the publications on An. pseudopunctipennis (20) and An. darlingi (12, 18), the light periodicity was set up at 12:12 with dawn and dusk spanning each 1 h. To stimulate copulation, blue light flashes (LED stroboscopic light) were provided for 45 min just after full darkness at a rate of 140 flashes per minute, each flash lasting ∼0.2 s. The temperature in French Guiana cycles daily between 22 and 32°C when An. darlingi can be found (Supplementary Figure 1, https://donneespubliques.meteofrance.fr/?fond=produit&id_produit=90&id_rubrique=32-; access date 23 August 2019). Considering these natural temperature conditions and expecting an adapted behavior keeping mosquitoes away from high temperatures in the field, the insectary temperature was set at 26°C during the daytime and down to 23°C during nighttime. A constant relative humidity was kept at approximately 75%, which is close to the average humidity observed in the field during An. darlingi peaks (81%, Supplementary Figure 1). A summary of those experimental conditions is presented in Figure 1.
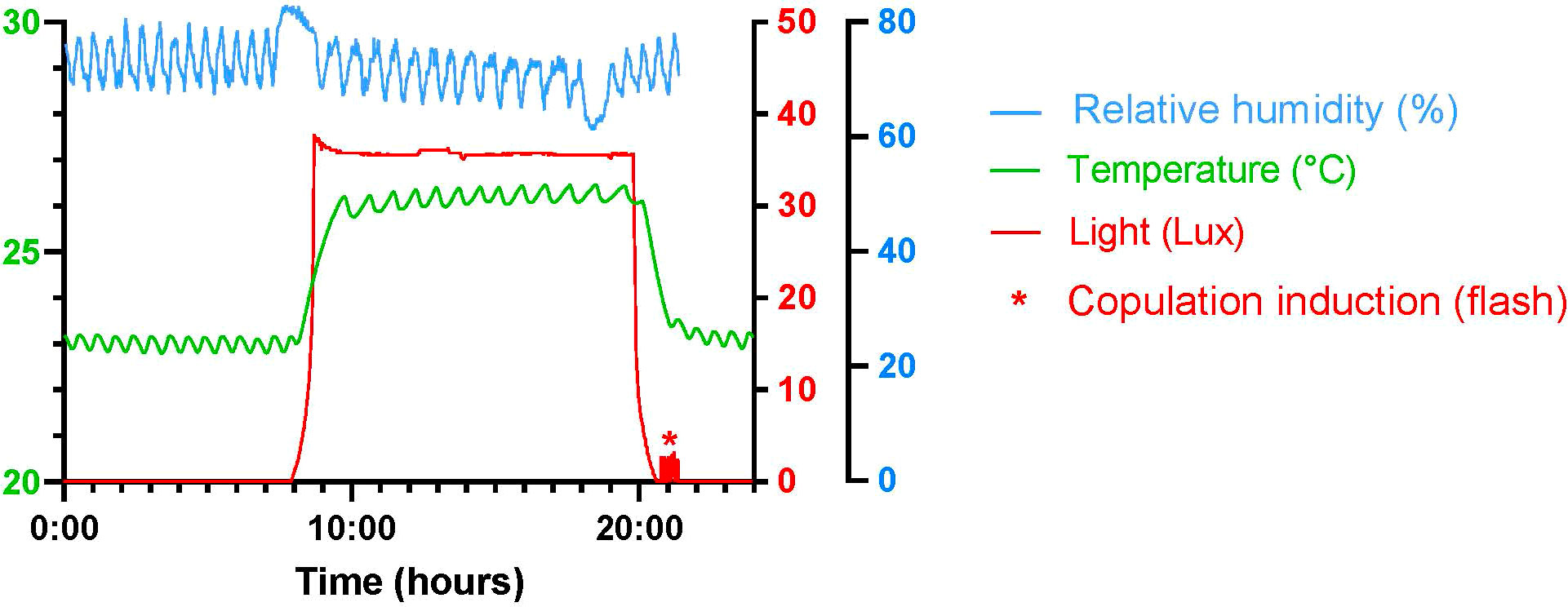
Figure 1 Insectary parameters. The graph represents temperature (green), relative humidity (blue), and light intensity (red) recorded via a Hobo 1019 recorder (MX1104). Temperature and humidity parameters are provided by a dedicated automated system. Light (LED) is controlled by an electronic device. Blue light flashes are provided by a LED stroboscopic light, just after dusk (red asterisk).
Mosquito rearing
Larvae were reared in white square plastic pans filled to 2 cm height with demineralized water supplemented with a mixture of salts (200× solution: NaCl 0.2%; CaSO4-2H2O 0.3%; NaHCO3 1%) and covered with a mosquito net. L1 to L2 larvae were fed with Tetra®TetraMin Baby and in later stages with cat food. When the first pupae appeared, a small container filled with a sterile 10% sucrose solution in mineral water (Volvic®) and holding a dental cotton roll (ROEKO Parotisroll Coltene—10 cm height) was placed in the center of the rearing tray to feed emerging adults. Adults were collected with an in-house made electric aspirator and transferred to mating cages (47.5 × 47.5 × 47.5 cm; BugDorm-4F4545—https://shop.bugdorm.com). Because of the size of the cage, two sucrose solution containers were placed at different heights in the cage. They were replaced every other day.
At each generation, mosquitoes were given 5 to 7 days for free mating (with light-flash induction, as described above) before being fed on anesthetized animals, either rabbit or mice (see details in Results).
Females were allowed to lay eggs in a black plastic tray filled to 1.5 cm height with a 2:1 mix of demineralized water and filter-sterilized water (22-µm filters) from the larval containers as a possible attractive cue to stimulate females to deposit their eggs. Eggs were collected by filtration through an in-house made strainer equipped with Nitex (125-µm fine mesh—Dutscher #987452) and then transferred for hatching in a rearing pan.
Experimental infections with Plasmodium berghei and Plasmodium falciparum
Five-day-old An. darlingi females were infected with P. berghei (GFP ANKA@HSP70 clone) or P. falciparum NF54, concurrently with same-age An. stephensi females (Sda500 strain). The mosquito infections were performed by the local Anopheles infection platform (CEPIA, Institut Pasteur). Before being exposed to an infected blood meal, females were starved from sugar overnight with access to water only in the form of a damp cotton ball, which was removed 4 h before blood feeding. Mosquito infection with P. berghei was performed by placing two anesthetized infected mice on top of the cage for 15 min. Only fully fed females were maintained for 10 days at 21°C for oocyst development. Mosquito infection with P. falciparum was performed by standard membrane feeding assay (SMFA) using glass feeders containing in vitro produced gametocytes as described previously (25). Fully fed females were transferred to small cages and maintained for 7 days (oocyst detection) at 26°C.
Oocysts were detected on dissected midguts stained with 0.1% mercuro-bromo fluorescein (Fluka) in 1× phosphate buffered saline.
Animals—Ethics statement
This study was conducted in strict accordance with the recommendations from the Guide for the Care and Use of Laboratory Animals of the European Union (European Directive 2010/63/UE) and the French Government. The protocol for Institut Pasteur-Paris was approved by the “Comité d’éthique en expérimentation animale de l’Institut Pasteur” CETEA 89 (Permit number: 2013–0132), by the French Ministry of Scientific Research (Permit number: 02174.02). For Institut Pasteur de La Guyane, the protocol was approved by the French Ministry of Scientific Research (Permit number APAFIS 2016081011367627_v5). Mosquito field collection in French Guiana was performed according to the Nagoya Protocol (Number 388019—19 March 2019).
Results
Establishing the first six generations of free-mating An. darlingi
Among 120 caught wild An. darlingi females, 54 took a blood meal on mice, and 34 produced eggs. From these 34 wild An. darlingi females, we obtained ~2,000 F1 eggs that led to 705 adults with a 1:1 sex ratio. Seven days after the first F1 adults emerged, mosquitoes were fed on anesthetized animals for nine consecutive days followed by four additional feedings spaced roughly every 3 days (Figure 2). To avoid mosquitoes drowning in the water of the egg-laying container, this was provided to females after the fifth blood meal only. The container was inspected every day for egg laying and the first eggs were laid after the sixth blood meal. Only 20 eggs were collected on that day. Although eggs were collected almost every day since the sixth blood meal, the size of each egg clutch rarely exceeded 50 eggs. Nevertheless, combining the frequency of blood feedings and egg collection over nearly 3 weeks allowed us to collect ~1,050 F2 eggs that produced 505 adults.
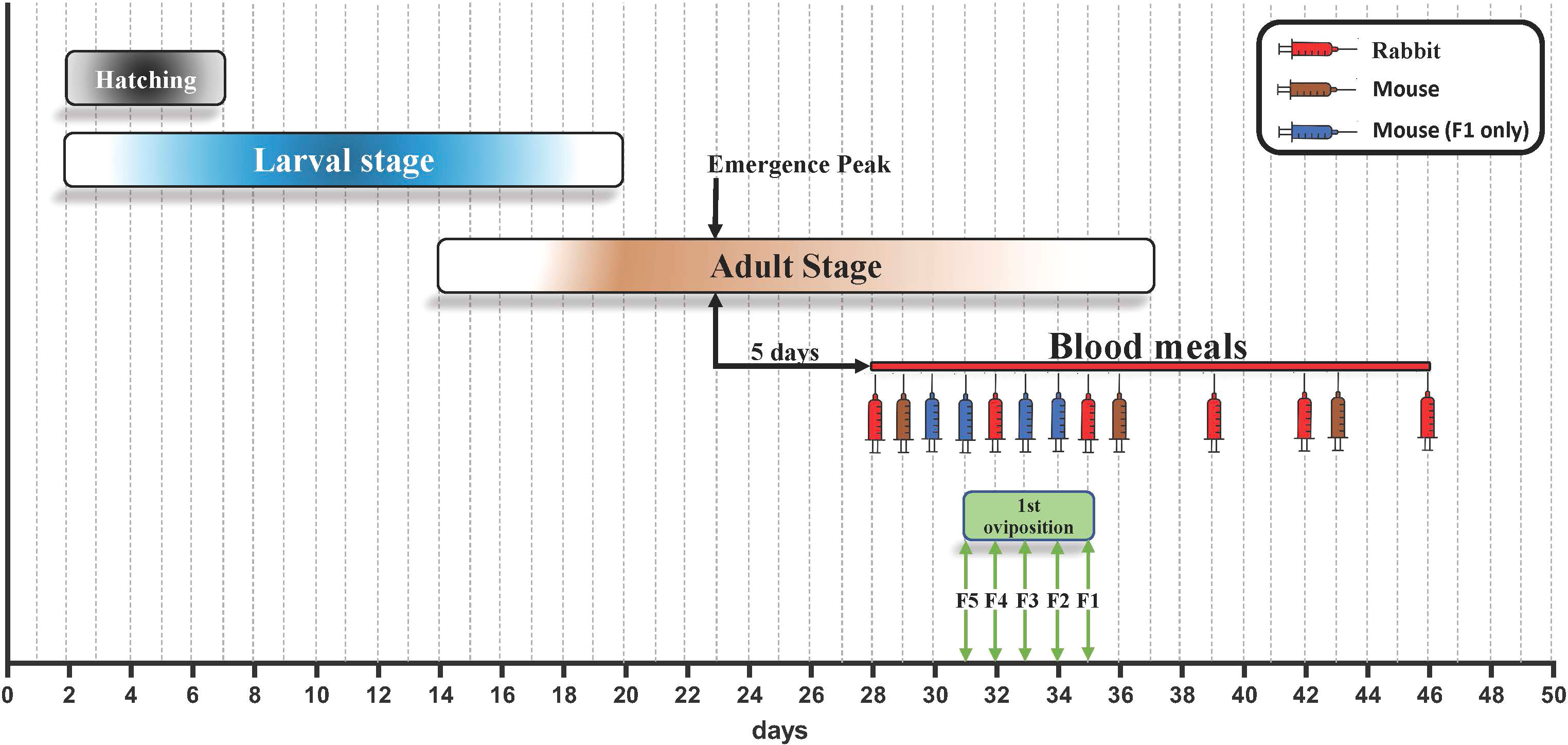
Figure 2 Schematic representation of the production of the first six generations of An. darlingi. The duration of egg hatching, larval stage, and adult stage are color coded. The red line is a schematic representation of the blood feeding strategy that was used over the first six generations by alternate feeding on rabbit (red) and mice (brown). Some additional blood feedings on mice were only provided to the first generation (blue). Between F2 and F6, the number of feedings varied based on the availability of the animals and the egg production and are detailed in Figure 3. At each generation, the time required for the females to lay the first egg batch (green) diminished across generations.
The same procedure and blood feeding scheme (Figure 2) allowed us to reach an F6 population of nearly 3,300 adults without any trouble (Figure 3).
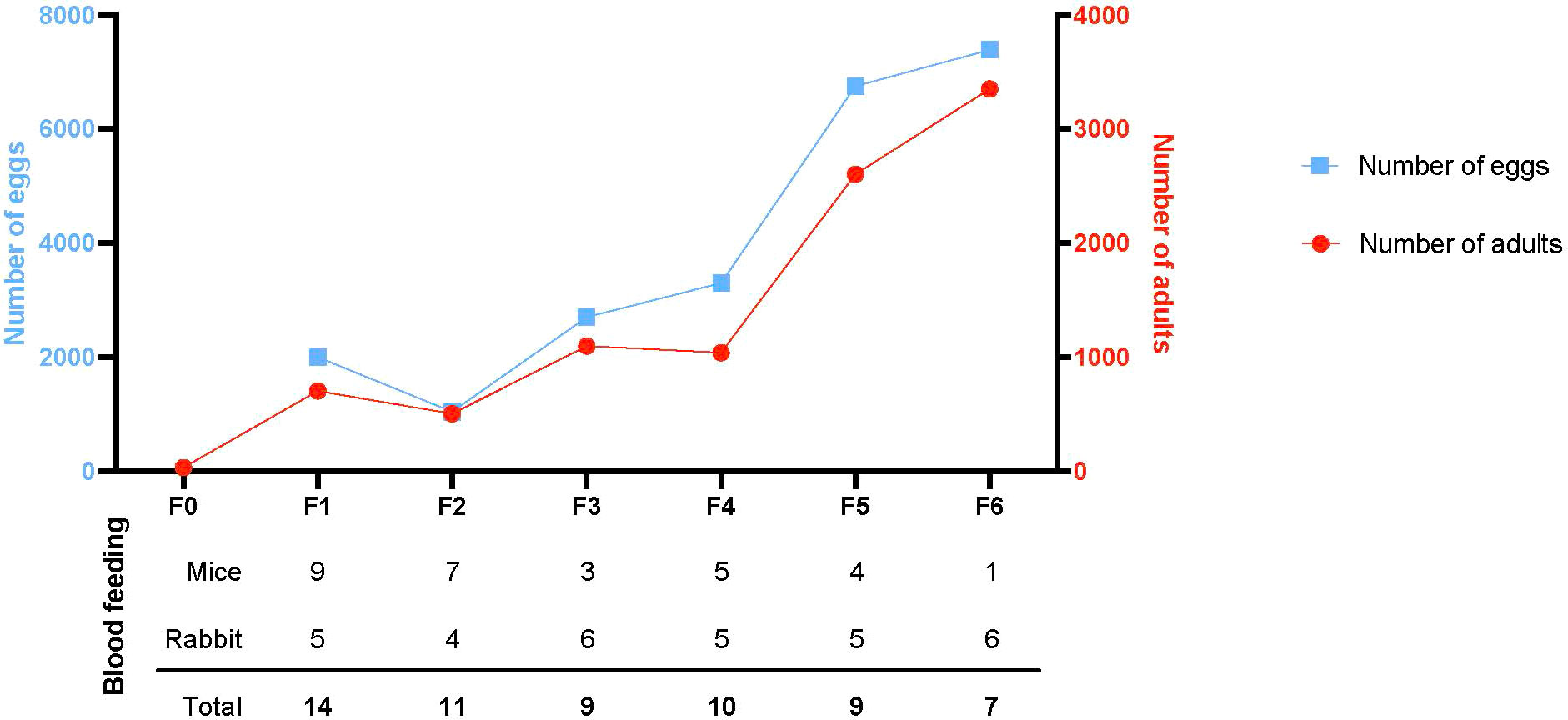
Figure 3 Yield in eggs and adults from F1 to F6. The graph represents the number of eggs obtained at each generation (blue line with squares and left scale) and the subsequent number of adults (red curve with circles and right scale). The number of blood feedings provided at each generation is indicated below.
Bionomic features
Egg production and development
The wild-caught females produced a mean of 59 eggs per blood-fed female (Table 1). This number dropped between an estimated 3 to 13 eggs per female during the establishment of the colony despite providing over nine blood meals to the females (Table 1 and Supplementary Data S1). This low number likely represents the low number of fertilized females and not the intrinsic fecundity of fertilized females. This could be confirmed by additional investigation as spermatheca dissection and single female egg collection.
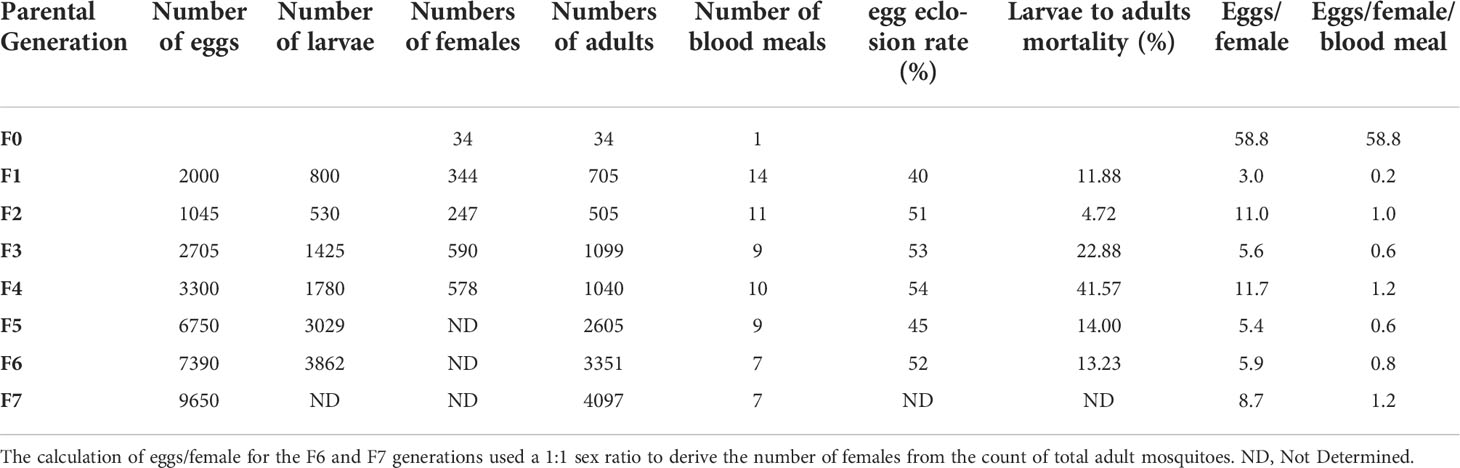
Table 1 This table summarizes the raw data obtained across 7 free mating generations of An. darlingi, starting with 34 wild caught gravid females producing 2000 F1 eggs that led to the production of 705 F1 adults.
Eggs laid on the same day after a blood meal hatched over 2 to 7 days leading to larval stages overlapping from L1 to L3. The hatching rate was relatively stable and rarely exceeded 50% (Table 1 and Figure 4), suggesting that unfertilized blood-fed females can lay unfertilized eggs or that the number of spermatozoids transferred to the female during free mating is not sufficient to fertilize all the oocytes developing in a single blood-fed female.
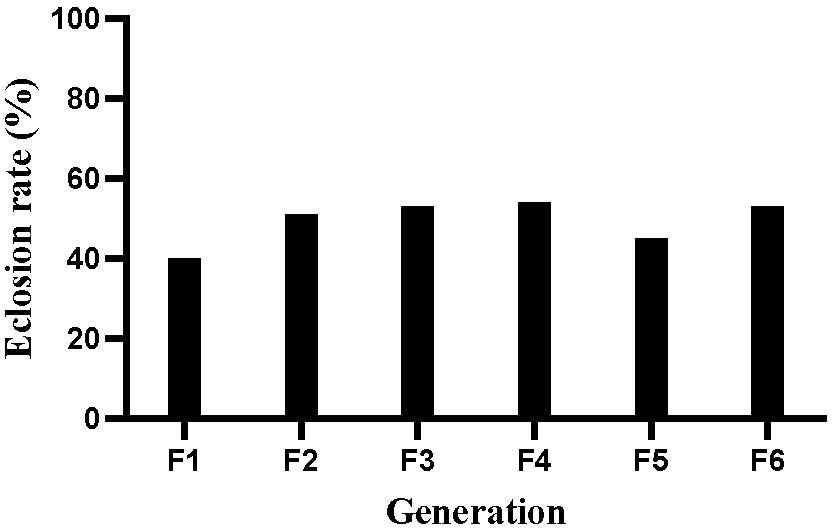
Figure 4 Egg eclosion rate at each generation. The number of L1 larvae hatching from each egg batch was determined by daily counting. L1 to L2 larvae hatching over the weekend were recorded on Mondays.
Larval and pupal development
The time between egg laying and first adult emergence was between 14 and 16 days under our insectary settings. The mortality rate of aquatic stages of An. darlingi was estimated as the ratio between the total number of adults and the total number of young larvae (L1 to L2) at each generation. As shown in Figure 5, the aquatic stage mortality rate was generally lower than 25%, except in F4 where it reached 42%. The proportion of females remained stable between 48% and 56% from F1 to F4, indicating that the sex ratio was not distorted from the expected 1:1 ratio (see Table 1).
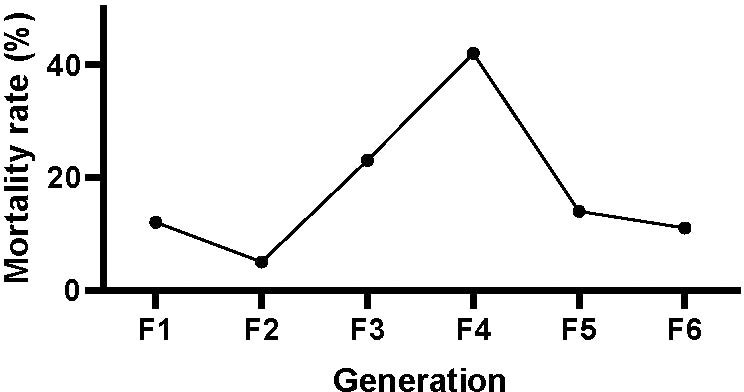
Figure 5 Larval survival success to adulthood across the first six generations of An. darlingi. The survival success is visualized from the mortality rate of larvae that did not reach adulthood, estimated from F1 to F6.
Monitoring the effect of the blue light flashes and shift in night temperature on free mating
To test whether the blue light flashes and the shift in night temperature were mandatory to maintain the colony as free mating after the first five generations, part of the F5 adults, thereafter called F5’, were moved to a similar insectary without any light flashes and with constant temperature (26°C) producing the F6’, while the remaining F5 adults were maintained with blue light flashes and low night temperature when producing the F6 generation. The F7 and F7’ generations were obtained from F6 and F6’, respectively. Conversely, the next two generations from the F7’ parents were submitted to light flashes and maintained at a constant temperature (26°C). As presented in Table 2, we did not detect any significant differences in the egg production between F and F’ at each generation, whether or not normalizing data to the number of blood feedings each generation received (Supplementary Data S2; Wilcoxon test on eggs/female—p = 1; on egg/female/blood feed—p = 1). This suggests that past the fifth generation, neither light flashes nor a shift in night temperature is required for maintaining this free-mating An. darlingi colony.
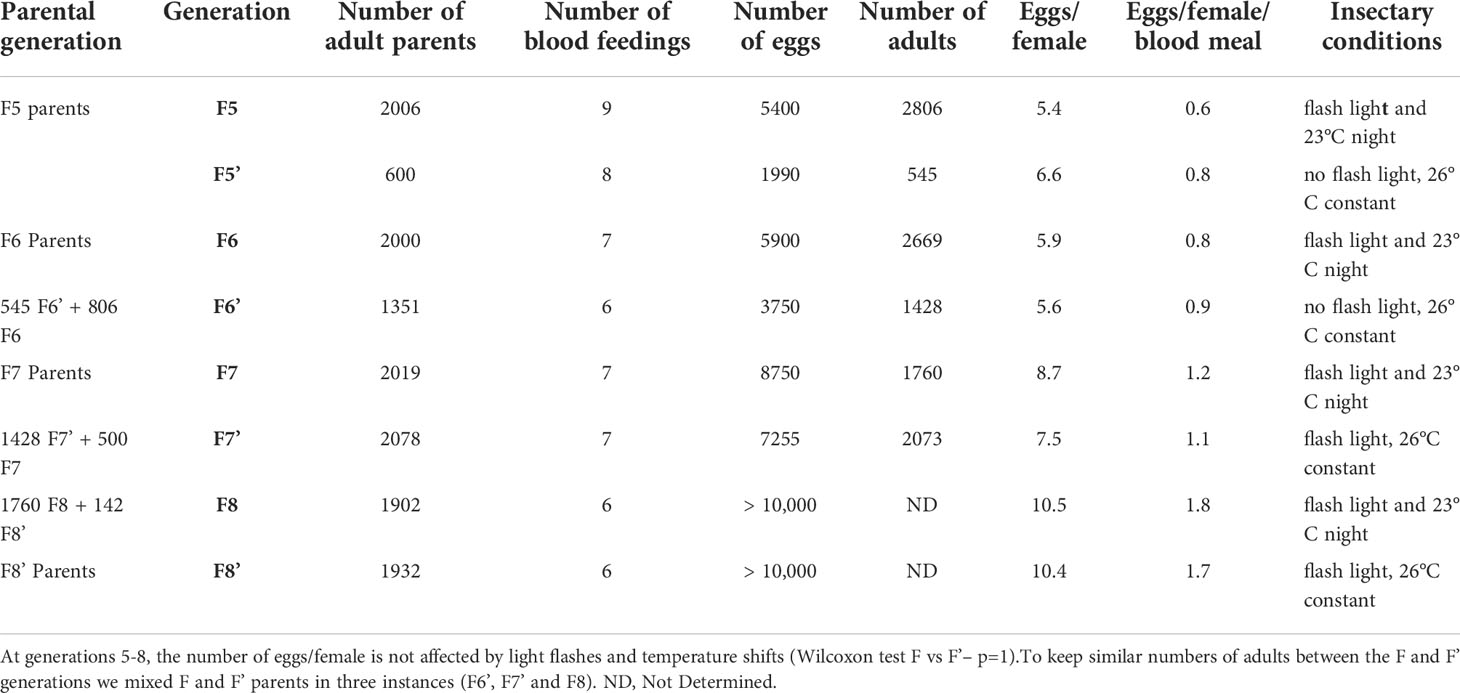
Table 2 Effect of the blue light flashes and shift in night temperature on free mating of An. darlingi.
Some F13 eggs were sent to Institut Pasteur de la Guyane (Cayenne, French Guiana) to rear a duplicate colony. In both laboratories, generation F21 is currently ongoing.
An. darlingi permissiveness to P. berghei and P. falciparum infection
We then tested whether this An. darlingi colony could be a valuable tool for vector competence studies. To this aim, An. darlingi and An. stephensi females were first infected in parallel with P. berghei. None of the 22 blood-fed An. darlingi females developed any oocyst, whereas 75% of the blood-fed An. stephensi females (n = 16) were infected with a mean oocyst load of 85.5 oocysts per infected female (range 27–100; Figure 6A). This is the first report revealing the possible refractoriness of An. darlingi to a rodent malaria parasite.
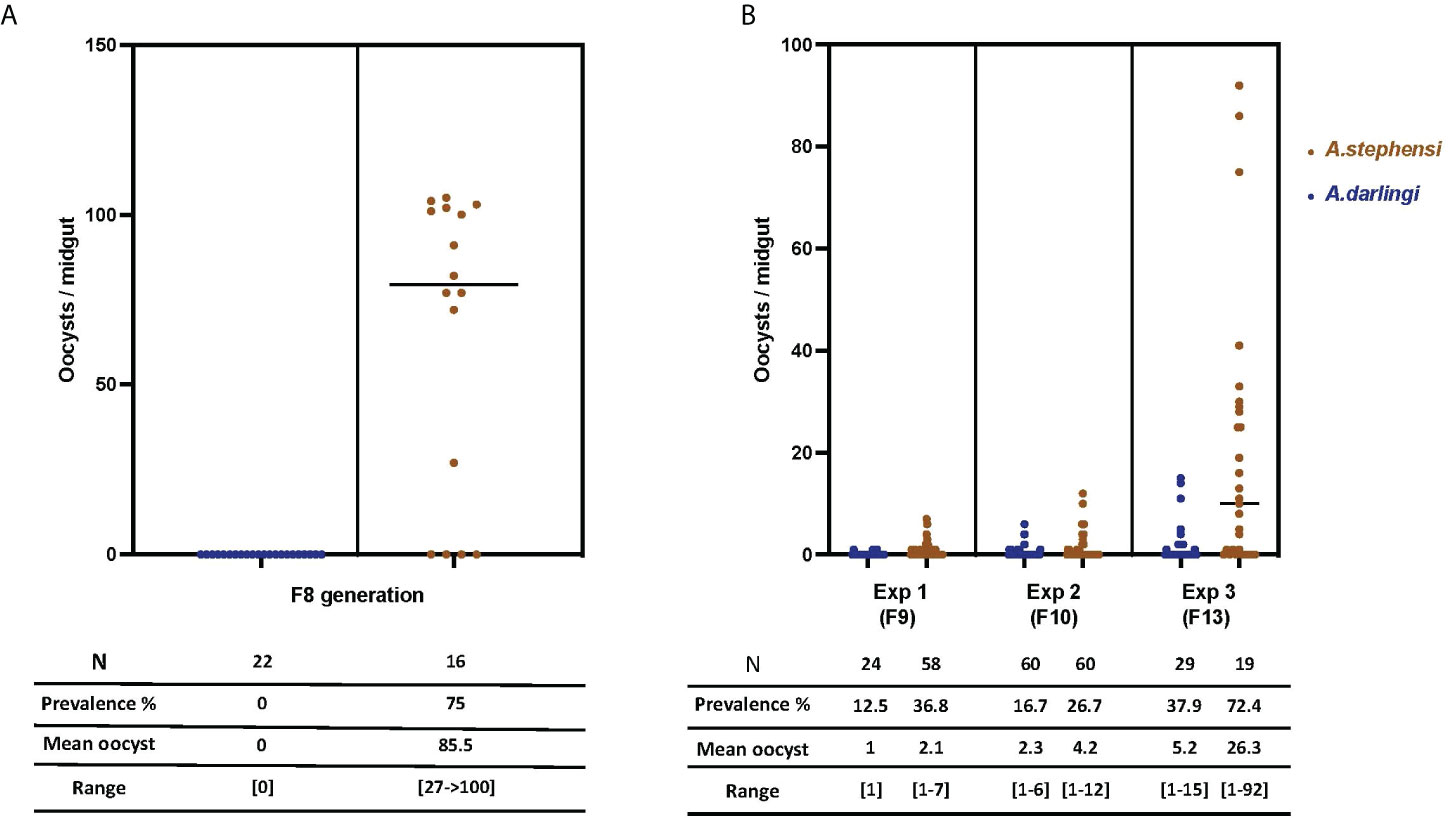
Figure 6 Infection of An. darlingi with P. berghei and P. falciparum. (A) Five-day-old An. darlingi females from the F8 generation, and An. stephensi (Sda 500) were starved overnight from sugar and fed on the same mice harboring P. berghei gametocytes. (B) Five-day old An. darlingi females and An. stephensi (Sda 500) were starved overnight from sugar and fed on P. falciparum NF54 gametocytes. Three experimental infections were performed using F9, F10, and F13 An. darlingi females, corresponding to Experiments 1, 2, and 3, respectively. The horizontal bar on the graph represents the median of developing oocysts per mosquito midgut. Detailed data on prevalence and infection intensity are indicated below. Mean oocyst counts and data range derive from infected midguts only.
Next, An. darlingi and An. stephensi females were infected with the same batch of in vitro produced P. falciparum gametocytes. Across three independent infection experiments, An. darlingi was found susceptible to P. falciparum NF54 (Figure 6B). However, both infection prevalence and oocyst load were lower than the ones of An. stephensi.
Discussion
Here, we report on the successful establishment of a free-mating An. darlingi colony starting from wild-caught females in French Guiana. An. darlingi has a wide geographic distribution from Central America to many countries in South America including the diverse regions of the Amazon basin where this species was identified as a dominant malaria vector [in (1)]. Based on various genetic analyses An. darlingi is composed of clearly isolated populations or clades (8, 26). The present colony likely represents a novel genetic resource of An. darlingi that will offer additional values for a better characterization of the genetic diversity and vectorial competence of this major South American malaria vector.
The colony was established from the progeny of 34 wild-caught females. This limited number of founders provided a starting pool of roughly 2,000 eggs. This number of founders is quite low compared to the numbers used for the most recently established An. darlingi colonies, which were initiated from 135 up to 878 wild-caught females (12, 18, 19).
To establish this colony, we developed a pragmatic approach by pooling information from our preliminary experiments and from published records on An. darlingi colonization since 1947. These data indicated that female insemination was a key parameter and that forced mating did not allow fertilization, even though this technique was easy to perform with An. darlingi ( (12, 18, 20), data not shown). We combined two main strategies. The first one capitalized on recent data obtained with An. pseudopunctipennis (20, 21) and An. darlingi (12) that suggest that mosquito copulation can be induced by providing light flashes just after dusk and decreasing night temperature, as happens in wild mosquito populations after dusk. The second strategy was to provide repeated blood meals to female mosquitoes up to 14 per generation over the first six generations. Providing light flashes after dusk as a copulation inducer was used for the recently established An. darlingi colonies from Peru and Brazil (12, 18, 19) while decreased night temperature was mentioned in only two reports (12, 19). Our data reported in Table 2 show that neither light flashes nor night temperature shift is important past the sixth generation. While we did not assess their role in the first six generations, we observed sexual activity as soon as light flashes started for the F1. In 1947 Bates observed “sexually excited males and mating” after exposure to lights of different colors (14). He reported that mating occurred in the presence of a dim blue light when a limited number of mosquitoes (328 adults) were kept in a small cage, while mosquitoes did not mate without artificial light. In our insectary conditions, even if copulation was induced after dusk using light flashes, copulation was also observed in the daytime under dim light past the 10th generation. Similarly, Villarreal-Treviño et al. reported a very strong impact of the light–temperature combination to favor insemination at the 2nd generation while light stimulation was not required anymore after the 10th generation (12).
Because of limited space, we chose middle-size adult cages (47.5 × 47.5 × 47.5 cm) despite data suggesting that larger cages (60 × 60 × 60 cm) or even bigger (1.2 m2 × 3 m high) do better (12–15). As recommended for Anopheles funestus, another difficult mosquito species to rear, and tested for An. darlingi (12), we used dark containers as egg-laying devices.
Following published data, mosquitoes were given their first blood meal on day 6 or day 7 post-emergence to ensure that the copulation stimuli were efficient enough to produce a reasonable number of fertilized females at each generation. Mosquitoes were then fed almost every day on anesthetized rabbits or mice. Feeding mosquitoes on more than one type of animal blood was reported to favor female fecundity and we previously used this strategy to establish an An. arabiensis colony from Madagascar (Puchot & Bourgouin, unpublished).
As presented in Tables 1 and 2 and Supplementary Figures S2 and S3, female fecundity (eggs/female) seems rather low at each generation. These numbers are in the same range as the ones obtained by Villarreal-Trevino et al. (12), using the same calculation method taking into account the entire female population rather than individual counts per fertilized female (18). It is noticeable that the egg-to-female ratio increased in the eighth generation while decreasing the number of blood meals provided. The number of blood meals has been reduced to six or seven from the 9th generation, and to three from the 13th generation. Meanwhile, we stopped counting the eggs as we had enough to proceed to the next generation.
Regarding the development time from egg to adult, we obtained very similar results to published data in the range of 14 to 16 days (12, 13). Noticeable was the asynchronous larval development from the same egg batch. Whether this was due to genetically different embryonic or larval development time or to the quantity and the type of the food provided to the larvae will need further investigation.
Importantly, we demonstrated that our free-mating An. darlingi colony is susceptible to in vitro produced gametocytes from P. falciparum NF54 originating from Africa. By comparison with concomitant An. stephensi control infections, the prevalence of infection was, however, slightly lower than the one obtained with F1 An. darlingi from Belize infected with the same P. falciparum strain (27). This difference may reflect an overall different genetic background between Belize and French Guiana An. darlingi populations or the selection of genotypes along the establishment of the colony from a limited number of founders. Interestingly, among the other species of the Nyssorhynchus subgenus, Anopheles albimanus is moderately refractory to P. falciparum NF54, whereas it is more permissive to P. falciparum 7G8, originating from Brazil (28). By contrast Anopheles aquasalis is refractory to both P. falciparum strains unless its immune system was affected by silencing the mosquito complement factor LRIM1 (Leucin-Rich-repeat-containing IMmune gene 1) or antibiotic treatment to remove their microbiota (29). When challenged with P. berghei, our colony showed full refractoriness, as observed for An. aquasalis (29), suggesting that this parasite species is not a suitable model to study An. darlingi-Plasmodium interactions. Further investigation would be necessary to determine whether P. berghei is developing more slowly in An. darlingi, as previously shown in An. albimanus (30), and whether Plasmodium yoelii is a suitable rodent malaria model in An. darlingi, as demonstrated in An. albimanus (29).
In conclusion, this novel An. darlingi colony established from French Guiana mosquitoes offers an invaluable tool for a better understanding of An. darlingi genetic diversity, physiology, and vector competence towards South America malaria parasites including P. falciparum and P. vivax.
Data availability statement
The raw data supporting the conclusions of this article will be made available by the authors, without undue reservation.
Author contributions
Conceived the project and funding: CB, JD, and MG. Designed the experiments: NP and CB. Performed the experiments: NP, M-TL, and RC. Analyzed the data: NP and CB. Drafted the paper: CB and NP. Reviewed and edited the manuscript: NP, JD, MG, and CB. All authors contributed to the article and approved the submitted version.
Funding
This work was supported by funds from the LabEx IBEID (The Laboratory of Excellence (LabEx) Integrative Biology of Emerging Infectious Diseases—grant no. ANR-10-LABX-62-IBEID) to CB and MG, FEDER-Contrôle to JD and RC, ANR JCJC MosMi to MG (grant no. ANR-18-CE15-0007), and a Calmette-Yersin Fellowship from Pasteur Network to RC. The funders had no role in study design, data collection and analysis, decision to publish, or preparation of the manuscript.
Acknowledgments
We thank members of the CEPIA platform for infecting our mosquitoes (An. darlingi and An. stephensi) with P. berghei and P. falciparum. Thanks to current and previous members of the Vectopole, especially Pascal Gaborit for technical help and to Anavaj Sakuntabhai for his constant support.
Conflict of interest
The authors declare that the research was conducted in the absence of any commercial or financial relationships that could be construed as a potential conflict of interest.
Publisher’s note
All claims expressed in this article are solely those of the authors and do not necessarily represent those of their affiliated organizations, or those of the publisher, the editors and the reviewers. Any product that may be evaluated in this article, or claim that may be made by its manufacturer, is not guaranteed or endorsed by the publisher.
Supplementary material
The Supplementary Material for this article can be found online at: https://www.frontiersin.org/articles/10.3389/fitd.2022.949300/full#supplementary-material
References
1. Sinka M, Bangs M, Manguin S, Rubio-Palis Y, Chareonviriyaphap T, Coetzee M, et al. A global map of dominant malaria vectors. Parasit Vectors (2012) 5(1):69. doi: 10.1186/1756-3305-5-69
2. Baker RH, Drench WL, Kitzmiller JB. Induced copulation in Anopheles mosquitoes. Mosq News (1962) 22(1):16–7.
3. Ow yang CK, St Maria FL, Wharton RH. Maintenance of a laboratory colony of Anopheles maclalatus (Theobald) by artificial mating. Mosq News (1963) 23:34–5.
4. Baker RH. Mating problems as related to the establishment and maintenance of laboratory colonies of mosquitos. Bull World Health Organ (1964) 31:467–8.
5. Amir A, Sum JS, Lau YL, Vythilingam I, Fong MY. Colonization of Anopheles cracens: a malaria vector of emerging importance. Parasit Vectors (2013) 6(1):81. doi: 10.1186/1756-3305-6-81
6. Horosko S, Lima JBP, Brandolini MB. Establishment of a free-mating colony of Anopheles albitarsis from Brazil. J Am Mosq Control Assoc (1997) 13(1):95–6.
7. Marinotti O. What is in a name? Anopheles darlingi versus Nyssorhynchus darlingi. Trends Parasitol (2021). 3710:856–8. doi: 10.1016/j.pt.2021.07.015
8. Conn JE, Ribolla PE. Chapter 5 - Ecology of Anopheles darlingi, the primary malaria vector in the americas and current nongenetic methods of vector control. In: Adelman ZN, editor. Genetic control of malaria and dengue. Boston: Academic Press (2016). p. 81–102.
9. Sinka ME, Rubio-Palis Y, Manguin S, Patil AP, Temperley WH, Gething PW, et al. The dominant Anopheles vectors of human malaria in the americas: occurrence data, distribution maps and bionomic precis. Parasit Vectors (2010) 3:72. doi: 10.1186/1756-3305-3-72
10. Yuval B. Mating systems of blood-feeding flies. Annu Rev Entomol (2006) 51(1):413–40. doi: 10.1146/annurev.ento.51.110104.151058
11. Lounibos LP, Lima DC, LourenÁo-de-Oliveira R. Prompt mating of released Anopheles darlingi in western Amazonian Brazil. J Am Mosq Control Assoc (1998) 14 2:210–3.
12. Villarreal-Trevino C, Vasquez GM, Lopez-Sifuentes VM, Escobedo-Vargas K, Huayanay-Repetto A, Linton YM, et al. Establishment of a free-mating, long-standing and highly productive laboratory colony of Anopheles darlingi from the Peruvian amazon. Malar J (2015) 14:227. doi: 10.1186/s12936-015-0733-0
15. Americano Freire S, Faria G. Rearing and some data on the biology of A. darlingi. Rev Bras Biol (1947) 7(1):57–66.
16. Corrêa RR, Ferreira E, Ramalho GR, Zaia L. Informe sobre uma colônia de anopheles darlingi. In: XVIII congresso brasileiro de higiene. São Paulo, Brasil: Sociedade Brasileira de Higiene, Rio de Janeiro (1970).
17. Buralli GM, Bergo ES. [Maintenance of Anopheles darlingi root, 1926 colony, in the laboratory]. Rev Inst Med Trop Sao Paulo (1988) 30(3):157–64. doi: 10.1590/S0036-46651988000300006
18. Moreno M, Tong C, Guzman M, Chuquiyauri R, Llanos-Cuentas A, Rodriguez H, et al. Infection of laboratory-colonized Anopheles darlingi mosquitoes by Plasmodium vivax. Am J Trop Med Hyg (2014) 90(4):612–6. doi: 10.4269/ajtmh.13-0708
19. Araujo MDS, Andrade AOSantos N, Pereira DB, Costa GDS, Paulo PFM, et al. Brazil's first free-mating laboratory colony of Nyssorhynchus darlingi. Rev Soc Bras Med Trop (2019) 52:e20190159. doi: 10.1590/0037-8682-0159-2019
20. Lardeux F, Quispe V, Tejerina R, Rodriguez R, Torrez L, Bouchite B, et al. Laboratory colonization of Anopheles pseudopunctipennis (Diptera: Culicidae) without forced mating. C R Biol (2007) 330(8):571–5. doi: 10.1016/j.crvi.2007.04.002
21. Villarreal C, Arredondo-Jiménez JI, Rodriguez MH, Ulloa A. Colonization of Anopheles pseudopunctipennis from Mexico. J Am Mosq Control Assoc (1998) 14(4):369–72.
22. Vezenegho SB, Carinci R, Gaborit P, Issaly J, Dusfour IBriolant S, et al. Anopheles darlingi (Diptera: Culicidae) dynamics in relation to meteorological data in a cattle farm located in the coastal region of French Guiana: Advantage of mosquito magnet trap. Environ Entomol (2015) 44(3):454–62. doi: 10.1093/ee/nvv053
23. Nepomichene TN, Andrianaivolambo L, Boyer S, Bourgouin C. Efficient method for establishing F1 progeny from wild populations of Anopheles mosquitoes. Malaria J (2017) 16(1):21. doi: 10.1186/s12936-017-1681-7
24. Folmer O, Black M, Hoeh W, Lutz R, Vrijenhoek R. DNA Primers for amplification of mitochondrial cytochrome c oxidase subunit I from diverse metazoan invertebrates. Mol Mar Biol Biotechnol (1994) 3(5):294–9.
25. Mitri C, Thiery I, Bourgouin C, Paul RE. Density-dependent impact of the human malaria parasite Plasmodium falciparum gametocyte sex ratio on mosquito infection rates. Proc Biol Sci (2009) 276(1673):3721–6. doi: 10.1098/rspb.2009.0962
26. Prado CC, Alvarado-Cabrera LA, Camargo-Ayala PA, Garzón-Ospina D, Camargo M, Soto-De León SC, et al. Behavior and abundance of anopheles darlingi in communities living in the Colombian Amazon riverside. PloS One (2019) 14(3):e0213335. doi: 10.1371/journal.pone.0213335
27. Grieco JP, Achee NL, Roberts DR, Andre RG. Comparative susceptibility of three species of Anopheles from Belize, central America, to Plasmodium falciparum (NF-54). J Am Mosq Control Assoc (2005) 21(3):279–90. doi: 10.2987/8756-971X(2005)21[279:CSOTSO]2.0.CO;2
28. Molina-Cruz A, Canepa GE, Kamath N, Pavlovic NV, Mu J, Ramphul UN, et al. Plasmodium evasion of mosquito immunity and global malaria transmission: The lock-and-key theory. Proc Natl Acad Sci U.S.A. (2015) 112(49):15178–83. doi: 10.1073/pnas.1520426112
29. Orfano AS, Duarte APM, Molina-Cruz A, Pimenta PF, Barillas-Mury C. Plasmodium yoelii nigeriensis (N67) is a robust animal model to study malaria transmission by south American anopheline mosquitoes. PloS One (2016) 11(12):e0167178. doi: 10.1371/journal.pone.0167178
Keywords: Anopheles darlingi, colony, rearing, Plasmodium, susceptibility, French Guiana
Citation: Puchot N, Lecoq M-T, Carinci R, Duchemin JB, Gendrin M and Bourgouin C (2022) Establishment of a colony of Anopheles darlingi from French Guiana for vector competence studies on malaria transmission. Front. Trop. Dis 3:949300. doi: 10.3389/fitd.2022.949300
Received: 20 May 2022; Accepted: 18 July 2022;
Published: 29 August 2022.
Edited by:
Joao Pinto, New University of Lisbon, PortugalReviewed by:
Thomas Walker, University of London, United KingdomAsh Pathak, University of Georgia, United States
Paulo Ribolla, São Paulo State University, Brazil
Copyright © 2022 Puchot, Lecoq, Carinci, Duchemin, Gendrin and Bourgouin. This is an open-access article distributed under the terms of the Creative Commons Attribution License (CC BY). The use, distribution or reproduction in other forums is permitted, provided the original author(s) and the copyright owner(s) are credited and that the original publication in this journal is cited, in accordance with accepted academic practice. No use, distribution or reproduction is permitted which does not comply with these terms.
*Correspondence: Catherine Bourgouin, Y2Fib3VyZ0BwYXN0ZXVyLmZy