- 1Instituto de Medicina Experimental-Consejo Nacional de Investigaciones Científicas y Técnicas (CONICET), Academia Nacional de Medicina, Buenos Aires, Argentina
- 2Section of Molecular Immunology and Gastroenterology, I. Department of Medicine, University Medical Center Hamburg-Eppendorf, Hamburg, Germany
Traditionally, pathogen-associated molecular patterns (PAMPs) were described as structural molecular motifs shared by different classes of microorganisms. However, it was later discovered that the innate immune system is also capable of distinguishing metabolically active microbes through the detection of a special class of viability-associated PAMPs (vita-PAMPs). Indeed, recognition of vita-PAMPs triggers an extra warning sign not provoked by dead bacteria. Bacterial RNA is classified as a vita-PAMP since it stops being synthesized once the microbes are eliminated. Most of the studies in the literature have focused on the pro-inflammatory capacity of bacterial RNA on macrophages, neutrophils, endothelial cells, among others. However, we, and other authors, have shown that microbial RNA also has down-modulatory properties. More specifically, bacterial RNA can reduce the surface expression of MHC class I and MHC class II on monocytes/macrophages and help evade CD8+ and CD4+ T cell-mediated immune surveillance. This phenomenon has been described for several different bacteria and parasites, suggesting that microbial RNA plays a significant immunoregulatory role in the context of many infectious processes. Thus, beyond the pro-inflammatory capacity of microbial RNA, it seems to be a crucial component in the intricate collection of immune evasion strategies. This review focuses on the different facets of the immune modulating capacity of microbial RNA.
Introduction
Live pathogens trigger more robust immune responses compared to dead pathogens (1, 2). A clear proof of this fact is that vaccines built based on attenuated strains, such as the vaccine against smallpox or poliomyelitis, induce responses of longer duration and are more effective in eradicating diseases compared to formulations using killed pathogens or purified antigens together with adjuvants (1, 3, 4). The difference in the extent of the immune response was initially attributed to the replicating nature of live microbes and their ability to deploy virulence factors. However, live vaccines are attenuated or avirulent, indicating additional mechanisms are at play. Thus, it became evident that the immune system had to have the machinery to detect microbial viability.
Recognition of living microbes was shown to rely on the detection of molecules associated with the metabolic activity and replicative capacity of microorganisms, which are rapidly eliminated when they lose their viability (2). In contrast, traditional PAMPs, such as LPS, lipoproteins and DNA, are structural components that persist even after the loss of metabolic activity. Hence, this particular kind of PAMPs associated with viability were called vita-PAMPs (2).
The best-characterized vita-PAMP is bacterial RNA. First described in 2011, recognition of bacterial RNA in the cytosol of innate immune cells leads to enhanced TRIF-dependent IFN-β secretion and NLRP3-mediated IL-1β production and pyroptosis (2, 5). Detection of prokaryotic RNA is a direct indication that bacteria are metabolically active, that is to say, transcribing genes and translating them into proteins (6).
Beyond RNA, there is only one other established vita-PAMP, namely cyclic-di-adenosine monophosphate (c-di-AMP) (7). This molecule directly binds and activates STING (Stimulator of IFN genes) (8), a direct innate immune sensor of endogenous cyclic di-nucleotide cGMP–cAMP (cGAMP) produced in response to the presence of cytosolic DNA (9). Other than that, bacterial signal peptides and quorum-sensing molecules were also proposed as vita-PAMPs (10), but there is no proof that they are only present in viable bacteria.
In this review we will detail the mechanisms by which microbial RNA can act as a unique warning sign for the immune system. Beyond that, we will describe how this molecule can also be exploited in immune evasion mechanisms by the pathogens themselves.
Recognition of Microbial Rna by Receptors of The Immune System
Among the receptors involved in the recognition of microbial RNA, the Toll-like receptors (TLRs) located in endosomes/phagolysosomes are the most studied. However, not all detect the same kind of RNA molecules. Thus, TLR3 recognizes double-stranded RNA, TLR7 recognizes double-stranded RNA (dsRNA) and single-stranded RNA (ssRNA), and TLR8 is capable of detecting ssRNA and RNA degradation products (11, 12). Another TLR located in endosomes/phagolysosomes and capable of recognizing RNA is TLR13. This TLR is, at least as far as we know, only present in mice and activated by an unmethylated motif present in 23S rRNA (the large ribosomal subunit of bacterial RNA) (13, 14). Besides, TLR receptors can differ in the adapter molecule responsible for signaling downstream of their activation: TLR7, 8 and 13 signal via MyD88, while TLR3 uses TRIF as adapter molecule. The signaling cascade triggered by the activation of these receptors leads to the induction of IFN-regulated factors 3 and 7 (IRF 3 and 7) and the consequent production of type I IFN. In addition, it can promote the activation of the NLRP3 inflammasome and production of classical cytokines of the nuclear factor κB (NF-κB) pathway, such as pro-IL-1β, IL-6 and TNF, depending on the cell type.
RNA can also be recognized by other families of receptors expressed in the cytosol. RIG I-like receptors (RLRs), which include retinoic acid-inducible gene I (RIG-I), melanoma differentiation-associated gene 5 (MDA5) and LGP2, are among such kind of cytosolic RNA receptors (5). Activation of RIG-I and MDA5 by ssRNA or dsRNA, respectively, signal through the mitochondrial antiviral protein (MAVS) to induce type I IFN production and activate the NLRP3 inflammasome, resulting in the production of IL-1β (15–17). Additionally, LGP2 may act as a regulator of RLR activity (5).
Going more deeply into recognition of microbial RNA, activation of endosomal TLR3 and TLRs7-8 has been associated with viral dsRNA and ssRNA respectively (18). However, beyond synthetic small molecules, human monocytes also sense bacterial RNA through TLR8. Examples of this phenomenon is the recognition of Group B streptococcus or Borrelia burgdorferi RNAs (19, 20). Also, human, and murine plasmacytoid dendritic cells recognize bacterial RNA through TLR7 (21). With respect to cytosolic RLRs, MDA5 is associated with sensing of antiviral responses triggered by long viral dsRNA whereas RIG-I senses short dsRNA or ssRNA. But RIG-I also senses RNA from a bacterium such as Listeria monocytogenes (17, 22).
Pro-Inflammatory Capacity of Microbial Rna
Microbial RNA Promotes Monocytes/Macrophages and Dendritic Cells Activation
Alexopoulou et al., in 2001, demonstrated that dsRNA is recognized by TLR3 (23). Moreover, the stimulation of this TLR by dsRNA induces NF-κB activation and type I IFNs production (23). However, the immunostimulatory capacity of ssRNA and the receptors involved in its recognition were unknown. In 2004, Heil et al. reported that GU rich ssRNA, that is oligonucleotides rich in guanosine and uridine, derived from human immunodeficiency virus-1 (HIV-1) induces the secretion of IFN-α and proinflammatory/regulatory cytokines by dendritic cells and macrophages (24). Moreover, the authors showed that murine TLR7 and human TLR8 are the receptors involved in the species-specific recognition of GU rich ssRNA, suggesting for the first time that ssRNA is the physiological ligand for these TLRs (24).
As mentioned, in 2011 Sander et al. described for the first time that bacterial RNA is a vita-PAMP (2). In this study, the authors showed that viable Escherichia coli (non replicative and without virulence factors) induces higher levels of IFN-β than heat-killed E. coli. Furthermore, only viable E. coli induces IL-1β secretion in bone marrow-derived macrophages (BMDM), peritoneal macrophages, and both splenic and bone marrow-derived dendritic cells (BMDC) (2). In addition, viable but not heat-killed E. coli induces NLRP3 and adapter protein ASC-dependent pro-caspase-1 cleavage, and pyroptosis in BMDM. Besides they showed that TRIF (the adapter protein of TLR3) controls “viability-induced” responses, in vitro and in vivo. The authors also demonstrated that the component associated with bacterial viability is the RNA, more specifically uncapped non-polyadenylated prokaryotic mRNA. Finally, the authors showed that viable bacteria RNA could access the NLRP3 inflammasome through leakage to the cytosol during the phagocytosis (2). For its part, Gratz et al. demonstrated that nucleic acids derived from the gram-positive human pathogen Streptococcus pyogenes induce the production of type I IFN by BMDM and BMDC (25). Moreover, type I IFN signaling is required to protect mice against lethal subcutaneous cellulitis caused by S. pyogenes infection (25). In this study, the authors showed that the nucleic acid involved in the production of IFN-β was different for these cellular types. In BMDM the production was dependent on the endosomal delivery of streptococcal DNA, while in BMDC it was induced by streptococcal RNA. In addition, the IFN-β signaling pathway employed for BMDM and BMDC was also different. The production of IFN-β was dependent on IRF3, STING, TBK1 and partially MyD88 for macrophages, and completely dependent on IRF5 and MyD88 for dendritic cells. Surprisingly, TLR3 and TLR7 were not required for the IFN-β production induced by S. pyogenes (25). Previously, Mancuso et al. reported that RNA from Group B streptococcus (GBS) is capable of inducing IFN-β production in BMDC through TLR7, MyD88 and IRF1 (26). Therefore, although GBS and S. pyogenes use MyD88, GBS is TLR7-dependent while S. pyogenes is TLR7-independent (26). On the other hand, Deshmukh et al. reported that the recognition of GBS and other Gram-positive bacteria by macrophages and monocytes relies on bacterial ssRNA (27). ssRNA interacts with a signalling complex, which comprises the TLR adaptors MyD88 and UNC93B (an endoplasmic reticulum protein essential for the delivery of TLRs to the endosome), but not the established MyD88-dependent ssRNA sensors (27). In line with these results, Eigenbrod et al. reported that bacterial RNA from both Gram-negative and Gram-positive bacteria induces caspase-1 activation and IL-1β secretion by murine dendritic cells and BMDM (28). Induction of pro-IL-1β, as well as the priming for caspase-1 activation by bacterial RNA was dependent on UNC93B whereas TLR3, 7, and 9 were dispensable. Additionally, caspase-1 activation and IL-1β production by transfected bacterial RNA were absent in MyD88-deficient cells but independent of TRIF (28). Several years later, in 2014, Signorino et at.. explored the role of murine TLR13 in the context of the infection with the model pathogen GBS. In this study, the authors concluded that although TLR13 participates in the recognition of GBS, the lack of function of this receptor can be compensated by other endosomal TLRs (29). In the study of Cervantes et al., the authors demonstrated that the RNA of Borrelia burgdorferi, the Lyme disease spirochete, is a TLR8 ligand in human monocytes and that transcription of IFN-β in response to the spirochete is induced from within the phagosomal vacuole through the TLR8-MyD88 pathway (19, 30). In accord with this study, in 2015 Eigenbrod et al. demonstrated that human monocytes respond to bacterial RNA with the secretion of IL-6, TNF-α, and IFN-β, which is critically dependent on lysosomal maturation and TLR8 (20). Moreover, TLR8-dependent detection of bacterial RNA was critical for triggering monocyte activation in response to infection with Streptococcus pyogenes (20). More recently, Hafner et al. reported that the recognition of low multiplicities of infection (MOI) of S. pyogenes by murine macrophages and dendritic cells is completely dependent on the sensing of nucleic acids through endosomal TLRs. However, at higher MOIs, TLR2 also has a role in S. pyogenes recognition (31). Finally, through in vivo experiments, Hafner et al. demonstrated that nucleic acid-sensing is crucial to the early detection of S. pyogenes. The loss of this recognition leads to a reduced local containment of the bacteria and subsequent dissemination, which leads to significant systemic inflammation (31).
Overall, the studies discussed here show evidences that microbial RNA is a potent activator of monocytes/macrophages and dendritic cells, two key components of the innate immune system.
Prokaryotic RNA Induces Neutrophil and Endothelial Cells Activation Promoting Neutrophil Transmigration
Some years ago, Rodriguez-Rodrigues et al. showed that prokaryotic RNA is also capable of activating polymorphonuclear neutrophils (PMN) (32). In this study, the authors studied the effect of live vs. dead bacteria on inducing the activation of PMN. They could show that live E. coli but not heat-killed E. coli (HK-Ec) induces an increase in the forward-scatter (FSC) parameter and an up-regulation of CD11b surface expression, events corresponding to the spreading and early activation of PMN. Furthermore, only live bacteria managed to increase PMN chemotaxis and potentiate their bactericidal capacity, demonstrated by an increase in ROS production and neutrophil extracellular traps (NETs) formation (32). This phenomenon was not restricted to E. coli since they also observed similar effects when using other bacteria, such as Enterococcus faecalis and Klebsiella pneumoniae (32). Interestingly, stimulation with either E. coli RNA or supernatants of dead bacteria containing released RNA mimicked the responses observed with live bacteria. Treatment of the E. coli supernatants with RNase negatively impacted their ability to increase ROS generation and NETosis. However, RNase treatment did not influence PMN activation overall since an increase in FSC, CD11b up-regulation, and increased chemotaxis was still observed, suggesting that other molecules could be also involved in the recognition of viability. Furthermore, the authors were not able to determine the nucleic acid receptor/s involved in the recognition of viable E. coli. Regarding this, PMN only express endosomal TLR8 (33–35), NLRP3 (36, 37) and RIG-I. Elucidating which of these receptors is responsible for this effect would help in further understanding how the immune system distinguishes live pathogens and could be used to target therapies.
Since the interaction between endothelial cells and neutrophils is decisive in the resolution of infections and having shown that RNA activates PMN, Castillo et al. investigated the effects of bacterial RNA on endothelial cells and whether these effects could also modulate neutrophil-endothelial cell interaction (38). In this paper, the authors found that stimulation of Human Umbilical Vein Endothelial Cells (HUVEC) with E. coli RNA up-regulates the expression of ICAM-1 (or CD54) on these cells and the secretion of IL-8 and von-Willebrand factor. These effects are consequences of HUVEC activation. Moreover, the conditioned medium of RNA-treated HUVEC induces the migration of PMN and the activation of these cells, shown by the up-regulation of CD11b. Last, the authors found that by mimicking an infectious focus using a transwell system, HUVECs respond to RNA delivered from the basolateral side and this event triggers the adhesion and transmigration of PMN.
Despite showing for the first time the role of prokaryotic RNA as a stimulus of endothelial cell-PMN interactions, the authors were not able to determine the HUVEC nucleic acid receptor/s involved in this pathway. It is well known however that cytosolic RNA sensors such as MDA5 are fundamental in antiviral innate immunity (39). So, perhaps these receptors are involved in the activation of endothelial cells triggered by bacterial RNA.
Thus, live pathogens and bacterial RNA have been shown to act on different cell types other than antigen-presenting cells such as the endothelium and PMN and induce their activation. The fact that both these cell types are the first to encounter pathogens during an infection suggests that recognition of viability is essential for the immune system. Once the endothelium is activated by bacterial RNA, it will attract PMN to the site of infection. These PMN in turn will also be further activated by the bacteria itself and most importantly by microbial RNA and increase their bactericidal capacity. Killed bacteria and NETs will release more RNA, thus further amplifying the immune response and helping in the resolution of the infectious foci.
Microbial RNA as an Adjuvant
Given their capacity to alert the innate immune system and generate potent immune responses without the risk of infection, vita-PAMPs and microbial RNA could be considered the ideal adjuvants. Proof of concept was already provided by Sander et al. when first describing bacterial RNA as a viability-associated PAMP. The authors showed that administering heat-killed E. coli plus total bacterial RNA into mice enhanced the anti-E. coli antibody production to levels similar to live bacteria (2). The observed increase in IgG class-switched antibodies pointed to a specific role of bacterial RNA in benefiting B cell activation and promoting the generation of germinal centers. Mechanistically, a CD11c+ CX3CR1hi CCR2- Ly6C- monocyte population responded to bacterial RNA via TRIF and promoted the activation of T follicular helper cells (Tfh) through the secretion of IFN-β and IL-1β (40). The effect of bacterial RNA was shown to be restricted to an increase in Tfh differentiation and a concomitant increase in serum antibody titers in all B cell subtypes. However, whether this response persists in time or whether it can protect against disease was not addressed. Similarly, bacterial RNA can induce the secretion of TNF and IL-12 via TLR8 in human monocytes which in turn promotes the differentiation of Tfh and their IL-21 production (41).
Despite these promising reports, further studies are needed to establish whether bacterial RNA could be used as a vaccine adjuvant. Indeed, the immunogenic capacity of exogenous RNA is well-known in the field of mRNA vaccines. Thus, great efforts were put into identifying ways to reduce the pro-inflammatory effects of RNA, such as introducing modified nucleosides or adding carrier proteins (42). Nonetheless, if bacterial RNA is to be used as an adjuvant, its anti-inflammatory effects should also be considered. In the next sections, we will focus more on this topic, specifically on how bacterial RNA can impact antigen presentation.
Overall, in these sections, we discussed some interesting topics on the inflammatory properties of bacterial RNA and its potential use as a vaccine adjuvant. The most important points are summarized in Figure 1.
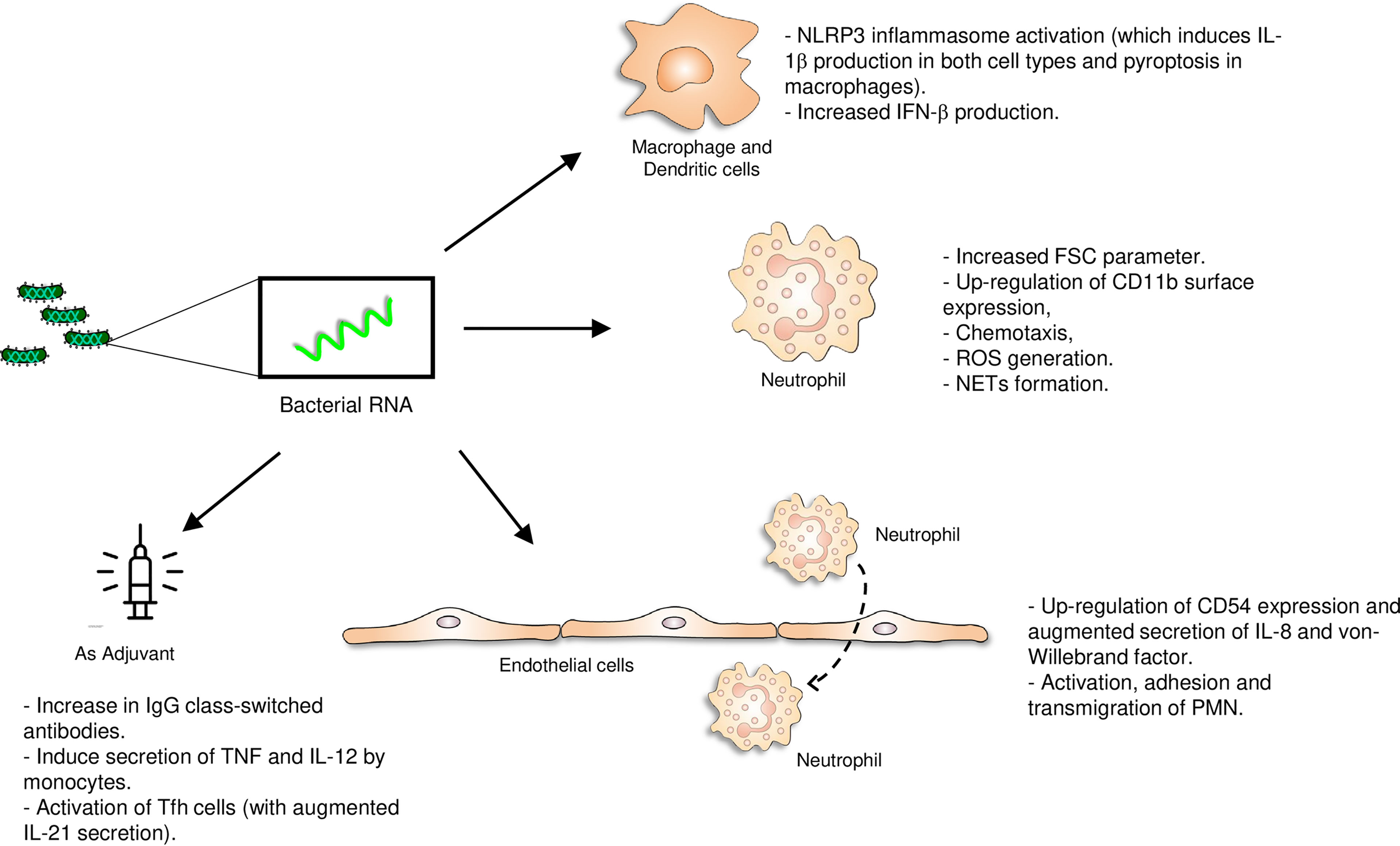
Figure 1 Pro-inflammatory role of bacterial RNA. The vita-PAMP RNA activates several immune cells (monocytes, macrophages, dendritic cells, polymorphonuclear cells) and endothelial cells and could act as an adjuvant in vaccine formulations. *Vaccine picture: created by Kiran Shastry from The Noun Project.
Down-Modulatory Capacity of Microbial Rna
Prokaryotic RNA Reduces MHC Class I and Class II Surface Expression on Monocytes/Macrophages
As shown in the previous sections, bacterial RNA can activate the innate immune system, and this characteristic makes it a potential vaccine adjuvant. In this section, we show evidence demonstrating that RNA is also a bacterial component used by specific pathogens to evade the immune system. A clear example of these pathogens is the intracellular bacterium Brucella abortus.
Several years ago, Barrionuevo et al. showed that infection of monocytes/macrophages with B. abortus hinders their ability to up-regulate the surface expression of MHC-I and MHC-II upon IFN-γ stimulation (43, 44). Thus, B. abortus-infected macrophages fail to present bacterial peptides to CD8+ and CD4+ T cells, respectively (43–45).
Interestingly, heat-killed B. abortus (HKBA) is not able to inhibit MHC-I expression, suggesting that such inhibition is dependent on bacterial viability (44). Furthermore, mutant strains in key virulence factors (such as the type IV secretion system and LPS) that cannot persist in macrophages for prolonged periods, can also inhibit MHC-I surface expression though only when they are alive (46, 47). The heat-killed forms of the mutant strains completely lost the ability to inhibit MHC-I expression (47), reinforcing the idea that Brucella-mediated MHC-I inhibition depends on bacterial viability but is independent of key virulence factors. In agreement with the results obtained with mutant strains, the kinetics of MHC-I inhibition showed that the phenomenon occurs early during infection when bacteria are actively replicating (46). Thus, Brucella was most likely employing a vita-PAMP to inhibit the expression of MHC-I, such as microbial RNA, which is exclusively present in viable bacteria (2, 6). Indeed, Brucella RNA was able to inhibit the MHC-I expression on the surface of macrophages, mimicking the effect observed during the infection with viable bacteria. Following these results, it was demonstrated that the incapacity of heat-killed Brucella to inhibit MHC-I surface expression is due to the absence of RNA in these bacterial preparations (47). In addition, RNA degradation products are also able to inhibit MHC-I expression. Furthermore, Brucella RNA and its degradation products mimic the retention of MHC-I molecules inside the Golgi apparatus previously observed by Brucella infection (44, 47). Interestingly, the phenomenon of MHC-I inhibition is not exclusive to Brucella RNA. Other prokaryotic RNAs, such as those from Salmonella typhimurium, Bacillus cereus, Escherichia coli, and Klebsiella pneumoniae had similar effects (47), suggesting that this immune modulation strategy can be extended to other infectious processes.
As mentioned, the most known receptors capable of detecting RNA are TLR3, TLR7, and TLR8, which are inside endosomes/phagolysosomes. Though initial candidates for the receptor involved in MHC-I inhibition, both TLR3 and human TLR7 were excluded using either BMDM from TRIF KO mice or specific inhibitors/agonists for their human counterparts. Therefore, human TLR8 was later shown to recognize Brucella RNA by using BMDM from TLR7 [the TLR that accomplishes the function of TLR8 in mice (24, 48, 49)] KO mice (47). Nonetheless, although not involved in MHC-I inhibition, TLR3 and TLR7 play an important role in sensing Brucella RNA to induce the production of pro-inflammatory cytokines and type I IFN expression in murine dendritic cells (50). As previously mentioned, TLR8 does not only recognize ssRNA but also RNA degradation products, specifically a uridine mononucleoside at one binding site and oligonucleotides like UG or UUG at a distinct second binding site (12). This would argue in favor of Brucella RNA degradation products as the real component involved in the inhibition of MHC-I surface expression. The reduced antigen presentation to MHC-I-restricted CD8+ cytotoxic T cells mediated by Brucella RNA was also abolished in BMDM from TLR7 KO mice (47). Thus, emphasizing the biological significance of RNA and its degradation products being employed as a molecular tool by Brucella to escape detrimental cytotoxic responses.
Regarding the inhibition of MHC-II surface expression on monocytes/macrophages mediated by B. abortus, unlike MHC-I inhibition, this phenomenon is mimicked by HKBA (43). A structural component, the outer membrane protein 19 (Omp19) [a prototypical B. abortus lipoprotein], diminishes the MHC-II surface expression (43). Moreover, the phenomenon of MHC-II inhibition could be extended to all Brucella lipoproteins since Pam3Cys (a synthetic lipohexapeptide that mimics the lipid moiety of lipoproteins) is also capable of inhibiting MHC-II surface expression (43). In addition, HKBA and lipidated Omp19 (L-Omp19) inhibited MHC-II surface expression through TLR2, and the soluble mediator involved in this phenomenon was IL-6 (43). Besides, the molecular mechanism exerted by B. abortus lipoproteins to inhibit MHC-II expression is actually at the level of MHC-II gene transcription (51). In detail, IL-6 induced by B. abortus lipoproteins-stimulation inhibits IFN-γ-induced IFN regulatory factor-1 (IRF-1) expression and activation. Then, this IRF-1 inhibition leads to a decrease in the transcription of the MHC-II master regulator (51).
In spite of what was known about MHC-II down-modulation by B. abortus, the fact that HKBA and B. abortus lipoproteins decreased MHC-II less than live bacteria (43, 51) led us to contemplate about the participation of another component associated with bacterial viability. In 2019, Milillo et al. demonstrated the participation of B. abortus RNA and its degradation products in the downregulation of IFN-γ-induced MHC-II expression on monocytes/macrophages (52). This result was confirmed using different models of monocytes/macrophages: THP-1 cells (a monocytic cell line), peripheral blood-purified human monocytes, and murine BMDM (52). As mentioned, B. abortus modified the expression of both MHC-I and MHC-II (47, 52). However, the MHC expression inhibition is not a generalized effect on all molecules induced by IFN-γ. Curiously, the IFN-γ- induced up-regulation of CD86 and CD40 was increased by B. abortus RNA, while the expression of CD80 was not modified by B. abortus RNA (52). However, the inhibition of MHC-II surface expression is not an exclusive phenomenon of B. abortus since it could be generalized to RNAs of other bacteria, such as Klebsiella pneumoniae, Staphylococcus aureus, and Escherichia coli. Additionally, the RNA of the parasite Trypanosoma cruzi was also able to inhibit MHC-II (52). These findings together with those observed for MHC-I inhibition by other prokaryotic RNAs, reinforce the idea that the MHC expression down-modulation is a phenomenon that can occur in multiple infectious contexts.
The evidence found in the study of Milillo et al., allowed us to understand that in the context of B. abortus infection there are two components participating in MHC-II down-modulation: RNA and lipoproteins. In line with this, we find a synergism between RNA and lipoproteins. Moreover, cells that were previously exposed to B. abortus RNA and that later received B. abortus lipoproteins presented higher MHC-II down-modulation compared to cells stimulated with the two ligands simultaneously. These results suggest that there could be a crosstalk between TLRs. Intricate TLR-TLR interactions have been described in the immune response against viral and bacterial pathogens (53, 54). In particular, stimulation of macrophages or dendritic cells with TLR8 alongside TLR3 or TLR4 ligands has a synergistic effect on NF-kB and IRF activation (53, 55). Moreover, Cervantes et al. demonstrated that after stimulation of monocytes with TLR8 ligands, TLR2 expression is up-regulated (56). For its part, Mureith et al. demonstrated that THP-1 cells pre-exposed to TLR8 ligand (3M-002) present an increased response to a subsequent TLR2 stimulation (57).
Finally, Milillo et al. demonstrated that B. abortus RNA-stimulated macrophages present a reduced capacity of antigen presentation to CD4+ T cells (even when B. abortus RNA increases the expression of co-stimulatory molecules). Furthermore, antigen presentation is even lower in macrophages stimulated with B. abortus RNA in combination with lipoproteins.
Here we present evidence demonstrating that bacterial RNA is a component associated with bacterial viability involved in the down-modulation of MHC-I and MHC-II surface expression. Consequently, through the inhibition of these molecules, the bacteria could avoid the recognition by CD8+ and CD4+ T cells and evade the surveillance of the immune system.
Down-Modulatory Properties of RNA in Other Infectious Contexts
Beyond B. abortus RNA, other authors also described that microbial RNA may have down-modulatory properties in the context of other infectious processes.
In 2016, Saha et al. demonstrated that infection of monocytes with Hepatitis C virus (HCV) induces their differentiation into polarized M2 macrophages that promote hepatic stellate cell activation via TGF-β. In this study, the authors also identified circulating monocytes expressing M2 markers and collagen in chronic HCV infection (58). Later, the same authors demonstrated that exosome-packaged HCV, cell-free HCV, or HCV ssRNA induces the differentiation of monocytes into macrophages with high expression of M2 surface markers and pro- and anti-inflammatory cytokines production (59). The activation of monocytes by HCV ssRNA could be prevented by TLR7 or TLR8 knockdown. Moreover, the stimulation of TLR7 or TLR8 by other TLR7/8 ligands (i.e., independent of HCV) promoted monocyte differentiation and M2 macrophages polarization. In chronic HCV-infected patients, the authors found circulating monocytes with high expression of TLR7/8 associated with increased procollagen intracellular expression. Finally, the authors demonstrated that knockdown of TLR8 completely attenuated the expression of collagen in monocytes exposed to HCV, and TLR7 knockdown partially attenuated this expression (59), suggesting an important role for TLR7/8 receptors in fibrocytes induction in the context of HCV infection.
As previously mentioned, TLR13 is only present in mice and recognizes a conserved sequence in 23S rRNA (13). More precisely, this sequence is the bacterial binding site for the antibiotics of the MLS (Macrolide, Lincosamide, and Streptogramin) group, including erythromycin. Remarkably, in this study Oldenburg et al. demonstrated that the clinical isolates from Staphylococcus aureus resistant to erythromycin have a 23S rRNA sequence unable to stimulate TLR13. Moreover, synthetic oligoribonucleotides with a modification that causes resistance to antibiotics of MLS group were unable to stimulate TLR13 as well. These results reveal that the same mechanisms that confer resistance to antibiotics allow the bacteria to deploy a powerful immune evasion strategy, preventing recognition via TLR13 (13, 60)
Overall, we discussed here evidence of the ability of microbial RNA to inhibit antigen presentation, direct monocyte differentiation into M2 macrophages, and abolish TLR13 recognition. These properties could be used by pathogens as potential immune evasion strategies. The more important points are summarized in Figure 2.
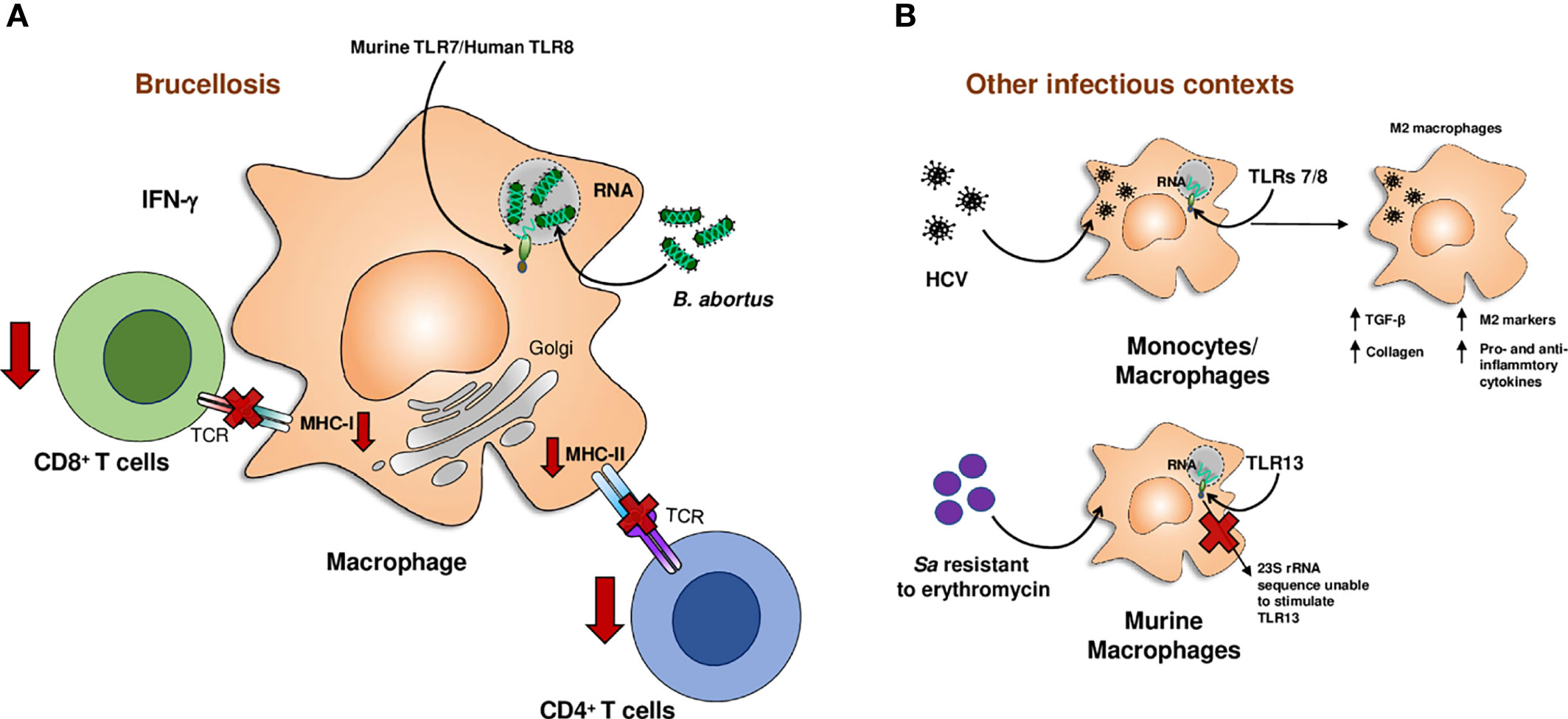
Figure 2 Down-modulatory role of microbial RNA. RNA from Brucella abortus (B. abortus) down-modulates IFN-γ-induced MHC-I and MHC-II expression on macrophages impairing antigen presentation to T lymphocytes (A). RNA from Hepatitis C virus (HCV) promotes the differentiation of human monocytes and macrophages to M2 macrophages. The rRNA 23S from Staphylococcus aureus (Sa) resistant to erythromycin cannot be sensed by the murine macrophage receptor, TLR13, evading immune system (B). *Image representing HCV: created by Marius edel from The Noun Project.
Final Remarks
In this review, we argue that microbial RNA can modulate the immune system in two manners: RNA has a pro-inflammatory capacity on numerous cells of the innate immune system, constituting an advantage for the host, even with potential use as a vaccine adjuvant; and, perhaps as a more challenging concept, microbial RNA has also down-modulatory properties constituting a crucial component in the complex set of immune evasion strategies employed by pathogens.
Author Contributions
MM, LV, and PB, conceptualization, writing, editing, and revising. All authors contributed to the article and have given approval to the final version of the manuscript.
Funding
This work was financed by PICT 2016-0356 and 2018-1969 grants from the Agencia Nacional de Promoción Científica y Tecnológica (ANPCYT-Argentina).
Conflict of Interest
The authors declare that the research was performed without any commercial or financial relationships that may represent a potential conflict of interest.
Publisher’s Note
All claims expressed in this article are solely those of the authors and do not necessarily represent those of their affiliated organizations, or those of the publisher, the editors and the reviewers. Any product that may be evaluated in this article, or claim that may be made by its manufacturer, is not guaranteed or endorsed by the publisher.
Acknowledgments
The authors would like to thank the authorities of the Institute of Experimental Medicine (https://imex.conicet.gov.ar/) for their continuous support of research in the Physiology of Inflammatory Processes Laboratory and the National Scientific and Technical Research Council (CONICET).
References
1. Kawamura I, Yang J, Takaesu Y, Fujita M, Nomoto K, Mitsuyama M. Antigen Provoking Gamma Interferon Production in Response to Mycobacterium Bovis BCG and Functional Difference in T-Cell Responses to This Antigen Between Viable and Killed BCG-Immunized Mice. Infect Immun (1994) 62:4396–403. doi: 10.1128/IAI.62.10.4396-4403.1994
2. Sander LE, Davis MJ, Boekschoten MV, Amsen D, Dascher CC, Ryffel B, et al. Detection of Prokaryotic mRNA Signifies Microbial Viability and Promotes Immunity. Nature (2011) 474:385–9. doi: 10.1038/nature10072
3. von Koenig CHW, Finger H, Hof H. Failure of Killed Listeria Monocytogenes Vaccine to Produce Protective Immunity. Nature (1982) 297:233–4. doi: 10.1038/297233A0
4. Lauvau G, Vijh S, Kong P, Horng T, Kerksiek K, Serbina N, et al. Priming of Memory But Not Effector CD8 T Cells by a Killed Bacterial Vaccine. Science (2001) 294:1735–9. doi: 10.1126/science.1064571
5. Blander JM, Sander LE. Beyond Pattern Recognition: Five Immune Checkpoints for Scaling the Microbial Threat. Nat Rev Immunol (2012) 12:215–25. doi: 10.1038/nri3167
6. Mourao-Sa D, Roy S, Blander JM. Vita-PAMPs: Signatures of Microbial Viability. Adv Exp Med Biol (2013) 785:1–8. doi: 10.1007/978-1-4614-6217-0_1
7. Moretti J, Roy S, Bozec D, Martinez J, Chapman JR, Ueberheide B, et al. STING Senses Microbial Viability to Orchestrate Stress-Mediated Autophagy of the Endoplasmic Reticulum. Cell (2017) 171:809–23.e13. doi: 10.1016/j.cell.2017.09.034
8. Burdette DL, Monroe KM, Sotelo-Troha K, Iwig JS, Eckert B, Hyodo M, et al. STING Is a Direct Innate Immune Sensor of Cyclic Di-GMP. Nature (2011) 478:515–8. doi: 10.1038/nature10429
9. Wu J, Sun L, Chen X, Du F, Shi H, Chen C, et al. Cyclic GMP-AMP is an Endogenous Second Messenger in Innate Immune Signaling by Cytosolic DNA. Science (2013) 339:826–30. doi: 10.1126/science.1229963
10. Ugolini M, Sander LE. Dead or Alive: How the Immune System Detects Microbial Viability. Curr Opin Immunol (2019) 56:60–6. doi: 10.1016/j.coi.2018.09.018
11. Dalpke A, Helm M. RNA Mediated Toll-Like Receptor Stimulation in Health and Disease. RNA Biol (2012) 9:828–42. doi: 10.4161/rna.20206
12. Tanji H, Ohto U, Shibata T, Taoka M, Yamauchi Y, Isobe T, et al. Toll-Like Receptor 8 Senses Degradation Products of Single-Stranded RNA. Nat Struct Mol Biol (2015) 22:109–15. doi: 10.1038/nsmb.2943
13. Oldenburg M, Kruger A, Ferstl R, Kaufmann A, Nees G, Sigmund A, et al. TLR13 Recognizes Bacterial 23S rRNA Devoid of Erythromycin Resistance-Forming Modification. Science (1979) (2012) 337:1111–5. doi: 10.1126/science.1220363
14. Hidmark A, von Saint Paul A, Dalpke AH. Cutting Edge: TLR13 Is a Receptor for Bacterial RNA. J Immunol (2012) 189:2717–21. doi: 10.4049/jimmunol.1200898
15. Schlee M, Hartmann G. Discriminating Self From Non-Self in Nucleic Acid Sensing. Nat Rev Immunol (2016) 16:566–80. doi: 10.1038/nri.2016.78
16. Vabret N, Blander JM. Sensing Microbial RNA in the Cytosol. Front Immunol (2013) 4:468. doi: 10.3389/fimmu.2013.00468
17. Abdullah Z, Schlee M, Roth S, Mraheil MA, Barchet W, Bottcher J, et al. RIG-I Detects Infection With Live Listeria by Sensing Secreted Bacterial Nucleic Acids. EMBO J (2012) 31:4153–64. doi: 10.1038/emboj.2012.274
18. Eigenbrod T, Dalpke AH. Bacterial RNA: An Underestimated Stimulus for Innate Immune Responses. J Immunol (2015) 195:411–8. doi: 10.4049/jimmunol.1500530
19. Cervantes JL, la Vake CJ, Weinerman B, Luu S, O’Connell C, Verardi PH, et al. Human TLR8 is Activated Upon Recognition of Borrelia Burgdorferi RNA in the Phagosome of Human Monocytes. J Leukoc Biol (2013) 94:1231–41. doi: 10.1189/jlb.0413206
20. Eigenbrod T, Pelka K, Latz E, Kreikemeyer B, Dalpke AH. TLR8 Senses Bacterial RNA in Human Monocytes and Plays a Nonredundant Role for Recognition of Streptococcus Pyogenes. J Immunol (2015) 195:1092–9. doi: 10.4049/jimmunol.1403173
21. Blander JM, Barbet G. Exploiting Vita-PAMPs in Vaccines. Curr Opin Pharmacol (2018) 41:128–36. doi: 10.1016/j.coph.2018.05.012
22. Hagmann CA, Herzner AM, Abdullah Z, Zillinger T, Jakobs C, Schuberth C, et al. RIG-I Detects Triphosphorylated RNA of Listeria Monocytogenes During Infection in non-Immune Cells. PLos One (2013) 8:e62872. doi: 10.1371/journal.pone.0062872
23. Alexopoulou L, Holt AC, Medzhitov R, Flavell RA. Recognition of Double-Stranded RNA and Activation of NF-kappaB by Toll-Like Receptor 3. Nature (2001) 413:732–8. doi: 10.1038/35099560
24. Heil F, Hemmi H, Hochrein H, Ampenberger F, Kirschning C, Akira S, et al. Species-Specific Recognition of Single-Stranded RNA via Toll-Like Receptor 7 and 8. Science (2004) 303:1526–9. doi: 10.1126/science.1093620
25. Gratz N, Hartweger H, Matt U, Kratochvill F, Janos M, Sigel S, et al. Type I Interferon Production Induced by Streptococcus Pyogenes-Derived Nucleic Acids Is Required for Host Protection. PLos Pathog (2011) 7:1001345. doi: 10.1371/journal.ppat.1001345
26. Mancuso G, Gambuzza M, Midiri A, Biondo C, Papasergi S, Akira S, et al. Bacterial Recognition by TLR7 in the Lysosomes of Conventional Dendritic Cells. Nat Immunol (2009) 10:587–94. doi: 10.1038/ni.1733
27. Deshmukh SD, Kremer B, Freudenberg M, Bauer S, Golenbock DT, Henneke P. Macrophages Recognize Streptococci Through Bacterial Single-Stranded RNA. EMBO Rep (2011) 12:71–6. doi: 10.1038/embor.2010.189
28. Eigenbrod T, Franchi L, Muñoz-Planillo R, Kirschning CJ, Freudenberg MA, Núñez G, et al. Bacterial RNA Mediates Activation of Caspase-1 and IL-1β Release Independently of TLRs 3, 7, 9 and TRIF But Is Dependent on UNC93B. J Immunol (2012) 189:328–36. doi: 10.4049/jimmunol.1103258
29. Signorino G, Mohammadi N, Patanè F, Buscetta M, Venza M, Venza I, et al. Role of Toll-Like Receptor 13 in Innate Immune Recognition of Group B Streptococci. Infect Immun (2014) 82:5013–22. doi: 10.1128/iai.02282-14
30. Cervantes JL, Hawley KL, Benjamin SJ, Weinerman B, Luu SM, Salazar JC. Phagosomal TLR Signaling Upon Borrelia Burgdorferi Infection. Front Cell Infect Microbiol (2014) 4:55. doi: 10.3389/fcimb.2014.00055
31. Hafner A, Kolbe U, Freund I, Castiglia V, Kovarik P, Poth T, et al. Crucial Role of Nucleic Acid Sensing via Endosomal Toll-Like Receptors for the Defense of Streptococcus Pyogenes In Vitro and In Vivo. Front Immunol (2019) 10:198. doi: 10.3389/fimmu.2019.00198
32. Rodriguez-Rodrigues N, Castillo LA, Landoni VI, Martire-Greco D, Milillo MA, Barrionuevo P, et al. Prokaryotic RNA Associated to Bacterial Viability Induces Polymorphonuclear Neutrophil Activation. Front Cell Infect Microbiol (2017) 7:306. doi: 10.3389/fcimb.2017.00306
33. Hayashi F, Means TK, Luster AD. Toll-Like Receptors Stimulate Human Neutrophil Function. Blood (2003) 102:2660–29. doi: 10.1182/blood-2003-04-1078
34. Janke M, Poth J, Wimmenauer V, Giese T, Coch C, Barchet W, et al. Selective and Direct Activation of Human Neutrophils But Not Eosinophils by Toll-Like Receptor 8. J Allergy Clin Immunol (2009) 123:1026–33. doi: 10.1016/j.jaci.2009.02.015
35. Berger M, Hsieh CY, Bakele M, Marcos V, Rieber N, Kormann M, et al. Neutrophils Express Distinct RNA Receptors in a non-Canonical Way. J Biol Chem (2012) 287:19409–17. doi: 10.1074/jbc.M112.353557
36. Bakele M, Joos M, Burdi S, Allgaier N, Pöschel S, Fehrenbacher B, et al. Localization and Functionality of the Inflammasome in Neutrophils. J Biol Chem (2014) 289:5320–9. doi: 10.1074/jbc.M113.505636
37. Pérez-Figueroa E, Torres J, Sánchez-Zauco N, Contreras-Ramos A, Alvarez-Arellano L, Maldonado-Bernal C. Activation of NLRP3 Inflammasome in Human Neutrophils by Helicobacter Pylori Infection. Innate Immun (2016) 22:103–12. doi: 10.1177/1753425915619475
38. Castillo LA, Birnberg Weiss F, Rodriguez-Rodrigues N, Pittaluga JR, Martire-Greco D, Milillo MA, et al. Prokaryotic RNA Activates Endothelial Cells Promoting Neutrophil Transmigration. Immunol Cell Biol (2019) 97:815–25. doi: 10.1111/imcb.12282
39. Guo X, Liu S, Yan R, Nguyen V, Zenati M, Billiar TR, et al. ADAR1 RNA Editing Regulates Endothelial Cell Functions via the MDA-5 RNA Sensing Signaling Pathway. Life Sci Alliance (2022) 5:e202101191. doi: 10.26508/lsa.202101191
40. Barbet G, Sander LE, Geswell M, Leonardi I, Cerutti A, Iliev I, et al. Sensing Microbial Viability Through Bacterial RNA Augments T Follicular Helper Cell and Antibody Responses. Immunity (2018) 48:584–98.e5. doi: 10.1016/j.immuni.2018.02.015
41. Ugolini M, Gerhard J, Burkert S, Jensen KJ, Georg P, Ebner F, et al. Recognition of Microbial Viability via TLR8 Drives T FH Cell Differentiation and Vaccine Responses. Nat Immunol (2018) 19:386–96. doi: 10.1038/s41590-018-0068-4
42. Pardi N, Hogan MJ, Porter FW, Weissman D. mRNA Vaccines - a New Era in Vaccinology. Nat Rev Drug Discovery (2018) 17:261–79. doi: 10.1038/nrd.2017.243
43. Barrionuevo P, Cassataro J, Delpino MV, Zwerdling A, Pasquevich KA, Garcia Samartino C, et al. Brucella Abortus Inhibits Major Histocompatibility Complex Class II Expression and Antigen Processing Through Interleukin-6 Secretion via Toll-Like Receptor 2. Infect Immun (2008) 76:250–62. doi: 10.1128/IAI.00949-07
44. Barrionuevo P, Delpino MV, Pozner RG, Velasquez LN, Cassataro J, Giambartolomei GH. Brucella Abortus Induces Intracellular Retention of MHC-I Molecules in Human Macrophages Down-Modulating Cytotoxic CD8(+) T Cell Responses. Cell Microbiol (2013) 15:487–502. doi: 10.1111/cmi.12058
45. Barrionuevo P, Giambartolomei GH. Inhibition of Antigen Presentation by Brucella: Many More Than Many Ways. Microbes Infect (2019) 21:136–42. doi: 10.1016/j.micinf.2018.12.004
46. Velasquez LN, Milillo MA, Delpino MV, Trotta A, Mercogliano MF, Pozner RG, et al. Inhibition of MHC-I by Brucella Abortus is an Early Event During Infection and Involves EGFR Pathway. Immunol Cell Biol (2017) 95:388–98. doi: 10.1038/icb.2016.111
47. Milillo MA, Velasquez LN, Trotta A, Delpino MV, Marinho FV, Balboa L, et al. B. Abortus RNA is the Component Involved in the Down-Modulation of MHC-I Expression on Human Monocytes via TLR8 and the EGFR Pathway. PLos Pathog (2017) 13:e1006527. doi: 10.1371/journal.ppat.1006527
48. Pelka K, Shibata T, Miyake K, Latz E. Nucleic Acid-Sensing TLRs and Autoimmunity: Novel Insights From Structural and Cell Biology. Immunol Rev (2016) 269:60–75. doi: 10.1111/imr.12375
49. Gorden KB, Gorski KS, Gibson SJ, Kedl RM, Kieper WC, Qiu X, et al. Synthetic TLR Agonists Reveal Functional Differences Between Human TLR7 and TLR8. J Immunol (2005) 174:1259–68. doi: 10.4049/jimmunol.174.3.1259
50. Campos PC, Gomes MT, Guimaraes ES, Guimaraes G, Oliveira SC. TLR7 and TLR3 Sense Brucella Abortus RNA to Induce Proinflammatory Cytokine Production But They Are Dispensable for Host Control of Infection. Front Immunol (2017) 8:28. doi: 10.3389/fimmu.2017.00028
51. Velasquez LN, Milillo MA, Delpino MV, Trotta A, Fernandez P, Pozner RG, et al. Brucella Abortus Down-Regulates MHC Class II by the IL-6-Dependent Inhibition of CIITA Through the Downmodulation of IFN Regulatory Factor-1 (IRF-1). J Leukoc Biol (2017) 101:759–73. doi: 10.1189/jlb.4A0416-196R
52. Milillo MA, Trotta A, Serafino A, Marin Franco JL, Marinho FV, Alcain J, et al. Bacterial RNA Contributes to the Down-Modulation of MHC-II Expression on Monocytes/Macrophages Diminishing CD4+ T Cell Responses. Front Immunol (2019) 10:2181. doi: 10.3389/fimmu.2019.02181
53. Ghosh TK, Mickelson DJ, Solberg JC, Lipson KE, Inglefield JR, Alkan SS. TLR-TLR Cross Talk in Human PBMC Resulting in Synergistic and Antagonistic Regulation of Type-1 and 2 Interferons, IL-12 and TNF-Alpha. Int Immunopharmacol (2007) 7:1111–21. doi: 10.1016/j.intimp.2007.04.006
54. Napolitani G, Rinaldi A, Bertoni F, Sallusto F, Lanzavecchia A. Selected Toll-Like Receptor Agonist Combinations Synergistically Trigger a T Helper Type 1-Polarizing Program in Dendritic Cells. Nat Immunol (2005) 6:769–76. doi: 10.1038/ni1223
55. Makela SM, Strengell M, Pietila TE, Osterlund P, Julkunen I. Multiple Signaling Pathways Contribute to Synergistic TLR Ligand-Dependent Cytokine Gene Expression in Human Monocyte-Derived Macrophages and Dendritic Cells. J Leukoc Biol (2009) 85:664–72. doi: 10.1189/jlb.0808503
56. Cervantes JL, Dunham-Ems SM, la Vake CJ, Petzke MM, Sahay B, Sellati TJ, et al. Phagosomal Signaling by Borrelia Burgdorferi in Human Monocytes Involves Toll-Like Receptor (TLR) 2 and TLR8 Cooperativity and TLR8-Mediated Induction of IFN-β. Proc Natl Acad Sci U S A (2011) 108:3683–8. doi: 10.1073/pnas.1013776108
57. Mureith MW, Chang JJ, Lifson JD, Ndung’u T, Altfeld M. Exposure to HIV-1-Encoded Toll-Like Receptor 8 Ligands Enhances Monocyte Response to Microbial Encoded Toll-Like Receptor 2/4 Ligands. Aids (2010) 24:1841–8. doi: 10.1097/QAD.0b013e32833ad89a
58. Saha B, Kodys K, Szabo G. Hepatitis C Virus-Induced Monocyte Differentiation Into Polarized M2 Macrophages Promotes Stellate Cell Activation via TGF-β. Cell Mol Gastroenterol Hepatol (2016) 2:302–316.e8. doi: 10.1016/j.jcmgh.2015.12.005
59. Saha B, Kodys K, Adejumo A, Szabo G. Circulating and Exosome-Packaged Hepatitis C Single-Stranded RNA Induce Monocyte Differentiation via TLR7/8 to Polarized Macrophages and Fibrocytes. J Immunol (2017) 198:1974–84. doi: 10.4049/jimmunol.1600797
Keywords: vita-PAMPs, microbial RNA sensors, RNA immunomodulation, adjuvants, immune evasion
Citation: Milillo MA, Velásquez LN and Barrionuevo P (2022) Microbial RNA, the New PAMP of Many Faces. Front. Trop. Dis 3:924719. doi: 10.3389/fitd.2022.924719
Received: 21 April 2022; Accepted: 08 June 2022;
Published: 07 July 2022.
Edited by:
Fabio Vitarelli Marinho, Federal University of Minas Gerais, BrazilReviewed by:
Erika Sousa Guimarães, Institute of Minas Gerais, BrazilCopyright © 2022 Milillo, Velásquez and Barrionuevo. This is an open-access article distributed under the terms of the Creative Commons Attribution License (CC BY). The use, distribution or reproduction in other forums is permitted, provided the original author(s) and the copyright owner(s) are credited and that the original publication in this journal is cited, in accordance with accepted academic practice. No use, distribution or reproduction is permitted which does not comply with these terms.
*Correspondence: Paula Barrionuevo, cGJhcnJpb24yMDA0QHlhaG9vLmNvbS5hcg==; M. Ayelén Milillo, YXllbGVuX21pbGlsbG9AaG90bWFpbC5jb20=