- 1Benaki Phytopathological Institute, Scientific Directorate of Entomology and Agricultural Zoology, Athens, Greece
- 2Laboratory of Entomology and Agricultural Zoology, School of Agricultural Science, Department of Agriculture Crop Production and Rural Environment, University of Thessaly, Volos, Greece
- 3Ygeionomiki Kritis, Chania, Greece
Aedes albopictus, also known as the Asian tiger mosquito, is an aggressive invasive mosquito species that transmits parasites that cause several major human diseases such as dengue, Chikungunya, and Zika. Its recent establishment in many European countries and the reported autochthonous cases of Aedes-transmitted arboviral diseases highlight the importance of effective surveillance programs to plan and implement efficient mosquito-control interventions. Aedes albopictus invaded the Greek island of Crete in 2014 and rapidly spread throughout; however, there are no comprehensive population-related data yet available. Our study focused on spatial and temporal dynamics of Ae. albopictus populations in rural and agricultural areas in Chania (western part of the Crete Island). An oviposition surveillance network, consisting of 51 ovitraps, was established in 2017 and systematically inspected for 2 years. Thirty ovitraps were established in rural areas and 21 in the surrounding agricultural areas. Eggs were collected weekly and transferred to the laboratory for counting and assessment of hatching rates. The spatial and temporal egg distribution was assessed by using geographical information systems and spatial statistical analysis tools. Kernel density and hot spot analysis were employed to identify clusters of high populations of eggs. Oviposition activity (eggs in traps) was recorded during April–May and December (of both years), while the maximum egg-laying rates were observed during September–October. The proportion of traps with eggs was similar between rural and agricultural areas, while the higher number of eggs was recorded in rural compared to agricultural areas during 2017. Egg hatch rates were high (>90%) until the end of September. The proportion of diapausing non-hatched eggs rapidly increased at the end of November. All hatched larvae were identified as Ae. Albopictus, indicating a negative effect of the invasion on the population of the local species Ae. cretinus. This was a systematic attempt to monitor the seasonal and spatial patterns of the Asian tiger mosquito in the island of Crete, the southernmost area of Europe, and a study in Europe that assessed temporal and spatial dynamics of Ae. albopictus soon after its invasion and establishment in an area.
Introduction
Aedes albopictus, also known as Asian tiger mosquito, is a daytime-biting, container-inhabiting mosquito species (1, 2). The females lay their eggs onto the moist substrates of breeding sites (3), while as a container-breeding species, it tends to breed in various artificial containers frequently found in urban areas (4). Urban areas include many private areas where water containers can be found and often act as ideal breeding sites for Ae. albopictus.
Because of its high ecological plasticity, Ae. albopictus has dispersed from the ancient habitats of southeastern Asia to almost all temperate areas of the globe in the last five decades (5). It was first reported in Europe in 1979, in Albania (6), it was then found in Italy in 1990 and has since spread in various areas of Europe (5, 7). In Greece, the establishment of Ae. albopictus was confirmed in 2005 (8). It was detected for the first time in the regional unit of Chania, Crete, in 2014 (9).
While the presence of Ae. albopictus is well documented in many European habitats, abundance and population dynamics are less studied (10). Ae. albopictus is a competent vector of at least 23 arboviruses including Chikungunya, dengue, Zika, and yellow fever virus (11). Its vectorial capacity is directly related to population growth, dispersion, and density. Temperate populations of Ae. albopictus are affected by seasonal temperature and photoperiodicity and, in response to these factors, can overwinter by producing eggs that undergo a winter diapause (12). However, this is not true for subtropical and tropical populations (13).
Surveillance of container-breeding mosquitoes is made, among others, with the use of ovitraps. In the management plan suggested for Ae. albopictus, monitoring is best conducted using ovitraps that attract females to lay their eggs on the provided oviposition substrate (5). Furthermore, ovitraps are widely used for entomological surveillance of Aedes species because they are cheap and easy to handle (14). Studies on surveillance of Aedes albopictus were also implemented in Switzerland (15, 16), Germany (17), France (18), Spain (19), and Italy (20).
A comprehensive vector surveillance system is essential for locally appropriate decision-making (21) toward vector control. Entomological surveillance in space and time aims, among others, at early detection, identification of the vector species, and optimization of the vector control measures in time and space (22). Knowledge of vector population peaks enables effective and timely response measures. This is reinforced by the fact that urban species such as Ae. albopictus exhibit strong density heterogenicity across districts of the same town/city (22).
Study of the spatial and temporal dynamics could be useful to determine timing, need, and area of interventions, while it could also drive to the identification of distribution patterns and clusters. Furthermore, the assessment of surveillance data at a fine spatial scale may help reveal the specific geographical features that contribute to hot spot generation (23, 24). Ae. albopictus distribution spatial patterns have been assessed earlier in terms of West Nile virus risk assessment (23, 25) or in terms of environmental and landscape effects on the distribution dynamics (26; 27, 28). Especially when the available resources for mosquitoes’ management are limited, the evaluation of spatiotemporal dynamics can provide a supporting tool for decision makers on how and when to allocate resources. Although there are some universal insights on where increased populations may be found, there is a consistent need for local spatial analysis that provides, apart from efficient mapping of the surveillance data, statistical backing of a particular hypothesis (25). There is some evidence that socioeconomic factors are important for determining the abundance of Ae. albopictus at the local scale (29); however, other studies suggest that local land use is the most important determinant of Ae. albopictus abundance, outweighing socioeconomic factors (e.g., income) (30).
In the current study, we assess the spatial and temporal population dynamics of Ae. albopictus in Chania 2 years after its introduction and establishment in the area and its invasion to other Regional Units in Crete, in the Regional Unit of Iraklio in 2015 and in the Regional Unit of Rethymno in 2016 (31). Our aim was to assess the temporal and spatial population dynamics of Ae. albopictus in rural and the surrounding agricultural areas in a selected region in Crete, which is regarded as an at-risk point of entry for invasive mosquito species (32). Understanding population dynamics at early stages of invasion and establishment is important for efficient decision-making on vector management practices.
Materials and Methods
Description of Study Site
The study focused on a part of the Municipality of Chania, which is located in the northwestern part of the Mediterranean island of Crete (35.48°N, 24.02°E), Greece (Figure 1). Chania is the second largest city in Crete. The study area covers most of the rural and agricultural areas around the airport of Chania. The agricultural parts of the study area include olive groves and greenhouses. The rural areas include small villages around the airport with a population of up to 600 inhabitants each, according to the 2011 census. The rural areas had similar patterns with small family houses and private gardens with vegetation (Mediterranean) and in some cases with domestic animals. The mean daily temperature, from May until December 2017, in the study area was 21.62°C and 21.72°C for the same period in 2018. The total rainfall from May until December was 194 mm in 2017 and 462 mm in 2018. The study area covers an area of almost 13 km2, while the Municipality of Chania has around 108.000 inhabitants (according to the last census, in 2011). The climate of the area according to Köppen–Geiger classification system is hot-summer Mediterranean (Csa). During the period that the current study was implemented (2017–2018), the mosquito control program in all Regional Units of Crete was focused only on native mosquitoes. The Municipality of Chania has no previous experience of locally transmitted vector-borne diseases (VBDs). No cases of VBDs related to mosquitoes of Aedes species have been recorded in this Municipality (18).
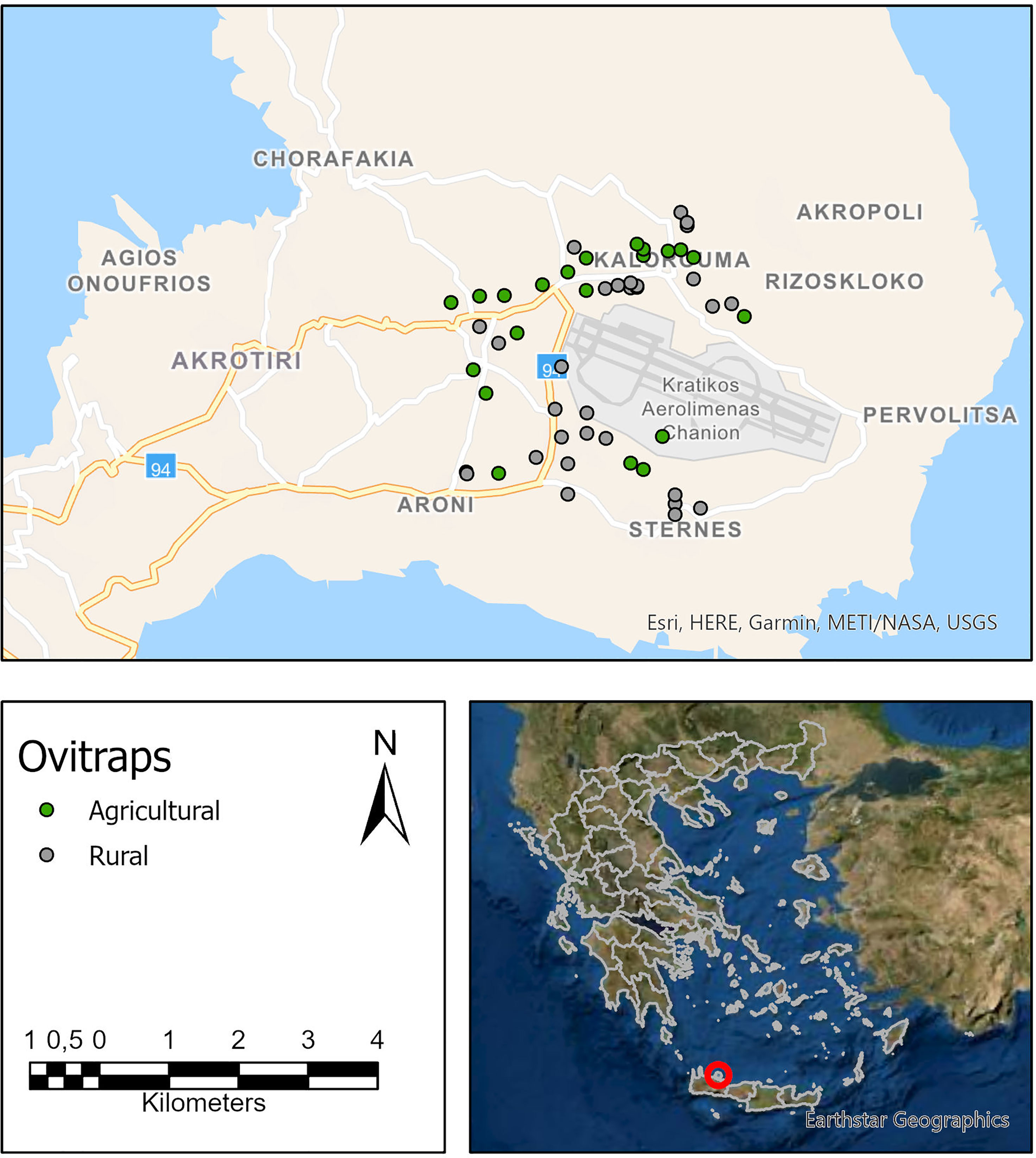
Figure 1 The study area in Chania, island of Crete, Greece. Green and gray dots indicate the position of the ovitraps in rural and agricultural areas, respectively.
Entomological Surveillance
An oviposition surveillance network, consisting of 51 ovitraps, was established and inspected for almost 2 years. Ovitraps were established on the first week of May 2017 and kept until the end of December 2018. Thirty ovitraps were established in rural areas, and 21 were set up in the surrounding agricultural areas. Ovitraps were put close to private areas but always in public places to ensure easy access. The exact location of the ovitraps (Figure 1) was determined with a Global Positioning System (GPS) device, and the ovitrap was not moved during the rest of the surveillance period.
Although the absence of a global standard to delineate cities, urban, and rural areas for international statistical comparisons (34) makes it difficult to define rural areas, we used the human population density criteria (35) according to which when density is greater than or equal to 1.000 people per square kilometer, we have an indication of intense urbanization (36). Agricultural areas describe areas used for farming. In our dataset, the smallest distance between a trap in a rural area and a trap in an agricultural area is 250 m in case the “rural” trap was located in the outer part of a village (usually in the cemetery) and the “agricultural” trap was located out of the village in the nearby fields.
The ovitraps used were black plastic containers, 15 cm high and 11.5 cm in diameter. Each ovitrap was filled with water (around 0.7 L). A hole was made 2.5 cm below the rim to avoid flooding by rainwater. A wooden strip was placed inside the ovitrap as an oviposition substrate. For ovitrap field management, the Standard Operational Procedures were followed (5). Therefore, ovitraps were placed in shaded and wind-protected sites surrounded by vegetation. The wooden strips were collected weekly and transferred to the laboratory. In the laboratory, the substrates were inspected with a stereoscope and the total number of eggs was counted.
Egg-Hatching Procedure
To avoid confusion with other Aedes species that also lay their eggs in small water containers, all eggs were hatched into larvae and then emerged adults were identified.
The collected eggs were transferred and hatched at the Benaki Phytopathological Institute, Athens, Greece, following a well-established methodology (37, 38).
Data Analysis
The positive ovitrap index (POI) and the mean eggs per trap (MET) were used to measure infestation of population as in Serpa et al. (39). The POI represents the mosquitoes’ distribution, and the MET describes the abundance of the population (40).
The POI and the MET were estimated as follows:
The MET was estimated as the number of eggs to the number of positive traps.
Normality of POI and MET data for rural and agricultural areas were visually tested with qq-plots and with Shapiro–Wilk test using SPSS (IBM SPSS Statistics for Windows, Version 22.0, IBM Corp., Armonk, New York, NY, USA). The Kruskal–Wallis test was used to evaluate the weekly POI and MET distributions in rural and agricultural areas.
Spatial Analysis
For the analysis of space and time patterns, kernel density analysis was used to produce monthly surface maps and identify high and low egg densities. Kernel density estimation (KDE) represents a simple alternative to analyze focal behavioral patterns (41). For the implementation of the KDE, ArcGIS Pro was used. For the implementation of the KDE, a quadratic function with a bandwidth of 1.000 m was used.
Hot spot analysis (Getis-Ord Gi* statistic) was also used to shed light upon local statistics (42). This kind of analysis assesses each feature within the context of neighboring features and compares the local situation to the global situation (43). The Getis-Ord Gi* statistic, applied within ArcGIS pro 2.7.0 (ESRI Inc., Redlands, USA) was used to identify hot spots for each month of the 2 years of surveillance. The Getis-Ord Gi* was used for each feature in the dataset, and the resulting z-scores and p-values indicate where spatial clusters of high or low values exist. For statistically significant positive z-scores, the larger the z-score is, the more intense the clustering of high values—hot spot. For statistically significant negative z-scores, the smaller the z-score is, the more intense the clustering of low values—cold spot (44).
The KDE is commonly used to smooth out the information represented by a collection of points in a way that it is understandable (45). However, it is the Getis-Ord Gi* statistic of the hot spot analysis that detects the significant clusters of high and low values.
Both spatial analyses were based on the average weekly number of eggs per trap for each of the studied month.
Results
Positive Ovitrap Index and Mean Eggs Per Trap
The monthly values of POI are presented in Figure 2 from May 2017 until December 2017 and from April 2018 until December 2018. From January 2018 until March 2018, the collected eggs were almost zero for consecutive weeks, and for this reason, the number of active traps was kept to a minimum, aiming only at determining the time on which eggs would reappear in the collected substrates. Ae. albopictus oviposition occurred during all samplings from May 2017 until December 2017 and from April 2018 until December 2018. In 2017, the peak of POI was observed in September for both agricultural (95.24%) and rural areas (96.67%); while in 2018, two peaks (100%) were observed in rural areas, the first one in July (100%) and the second in October (100%). The peak of POI in the agricultural areas, in 2018, was observed in October (100%). It should be noted though that the POI in rural areas during September 2018 reached also 96.67%.
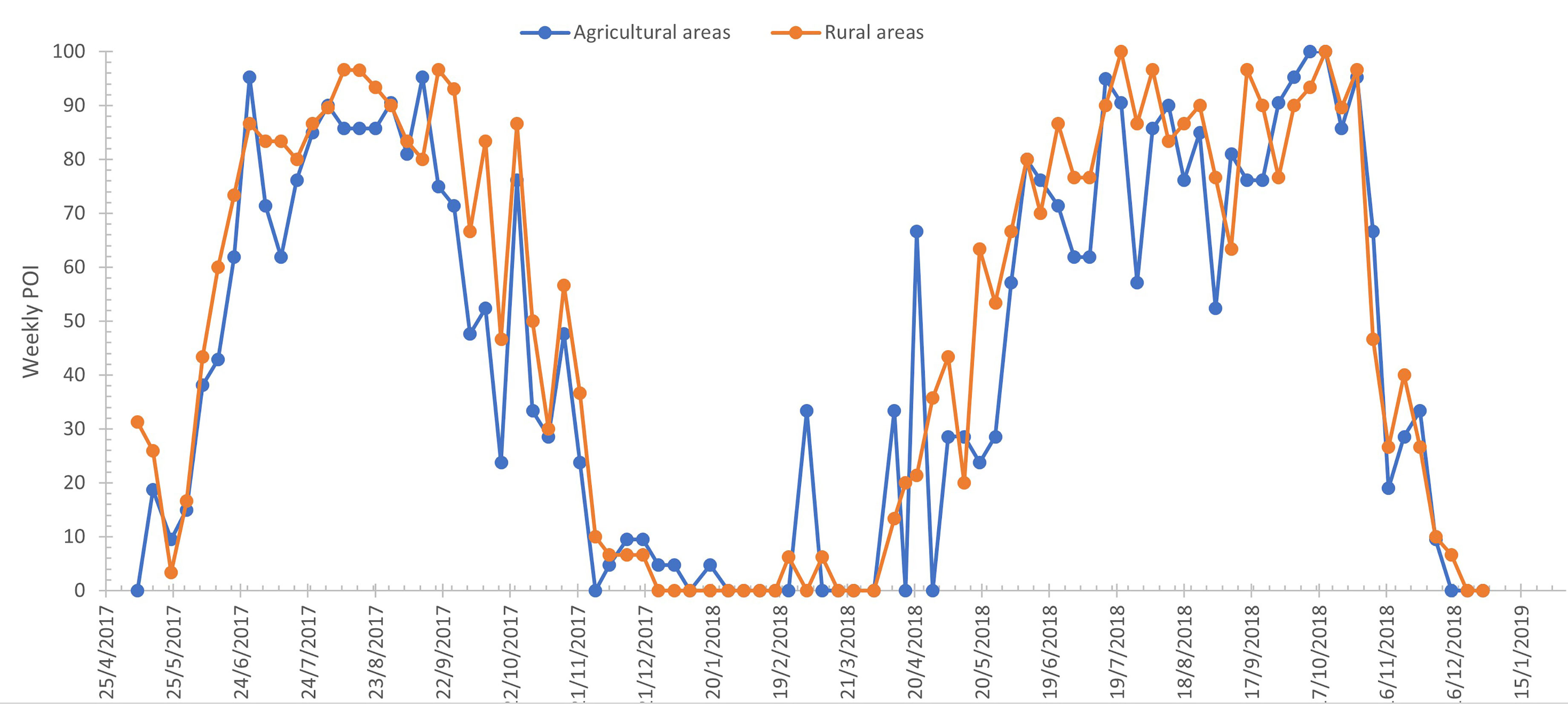
Figure 2 Weekly distribution of positive ovitrap index (POI) in agricultural and rural areas for Aedes albopictus.
The abundance of eggs was measured with MET (Figure 3). In 2017, the highest number of MET was recorded in September (99.45 ± 9.98) for the rural areas and during the last week of May (85.00 ± 3.93) for the agricultural areas. The lowest values of MET were recorded in December for both years and areas and were close to zero. In 2018, the highest value of MET was recorded in October (agricultural areas: 97.38 ± 13.80; rural areas: 102.64 ± 10.15).
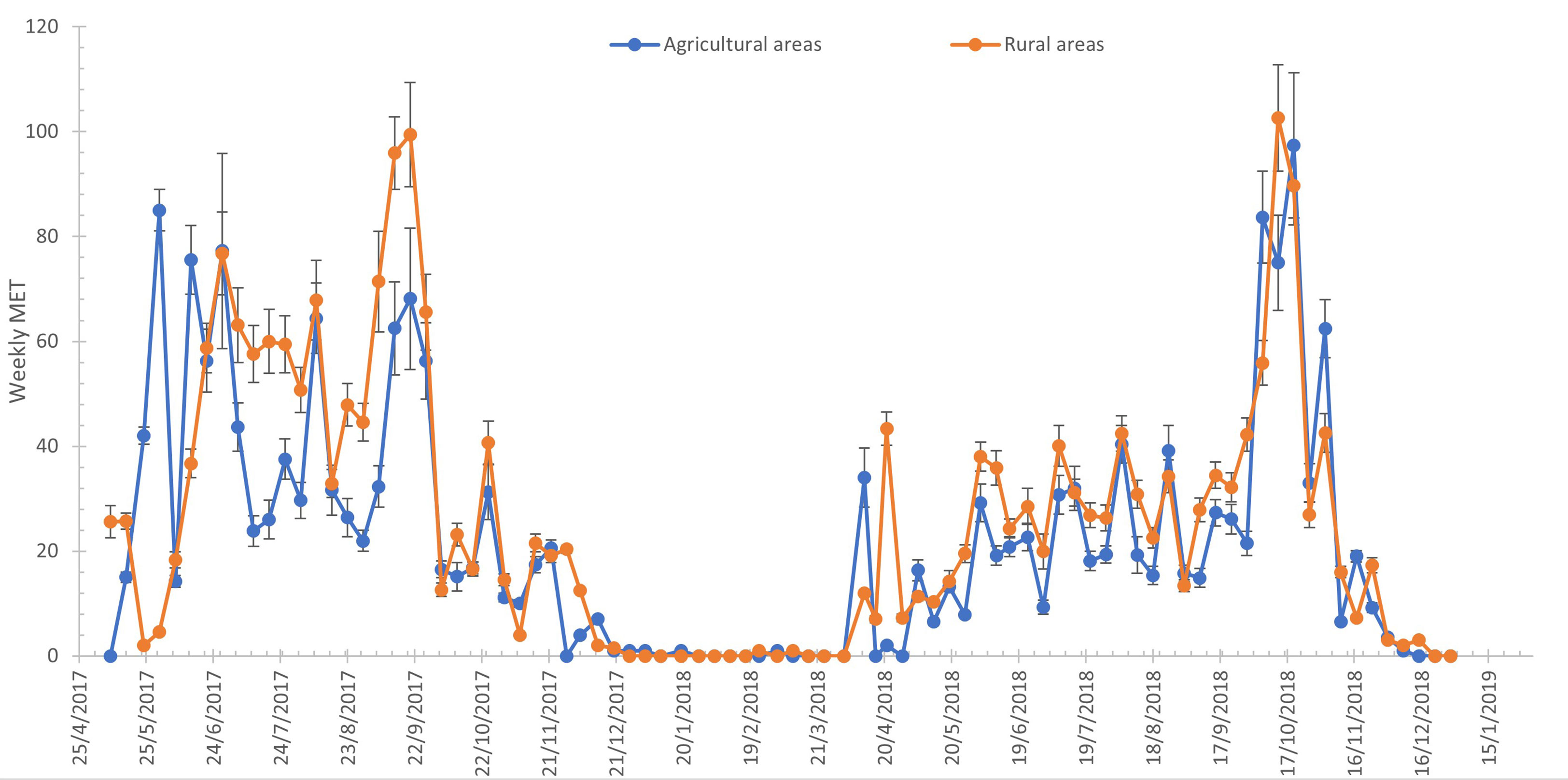
Figure 3 Weekly distribution of mean eggs per trap (MET) in agricultural and rural areas for Aedes albopictus.
Weekly POI and MET distributions in rural and agricultural areas were statistically evaluated. The MET in the rural areas was significantly higher than the MET in agricultural areas (H = 8.798, p < 0.01). On the contrary, there was no significant difference between the POI in rural and agricultural areas (H = 1.295, p = 0.255).
Hatchability
All hatched larvae were identified as Ae. albopictus. Figure 4 presents the average rates of egg hatching. The hatchability was above 90% from June to September in both years. The highest hatching rate (>98%) was observed in early September for both years. The hatching rate started to decrease below 70% in mid-October 2017 and early November 2018.
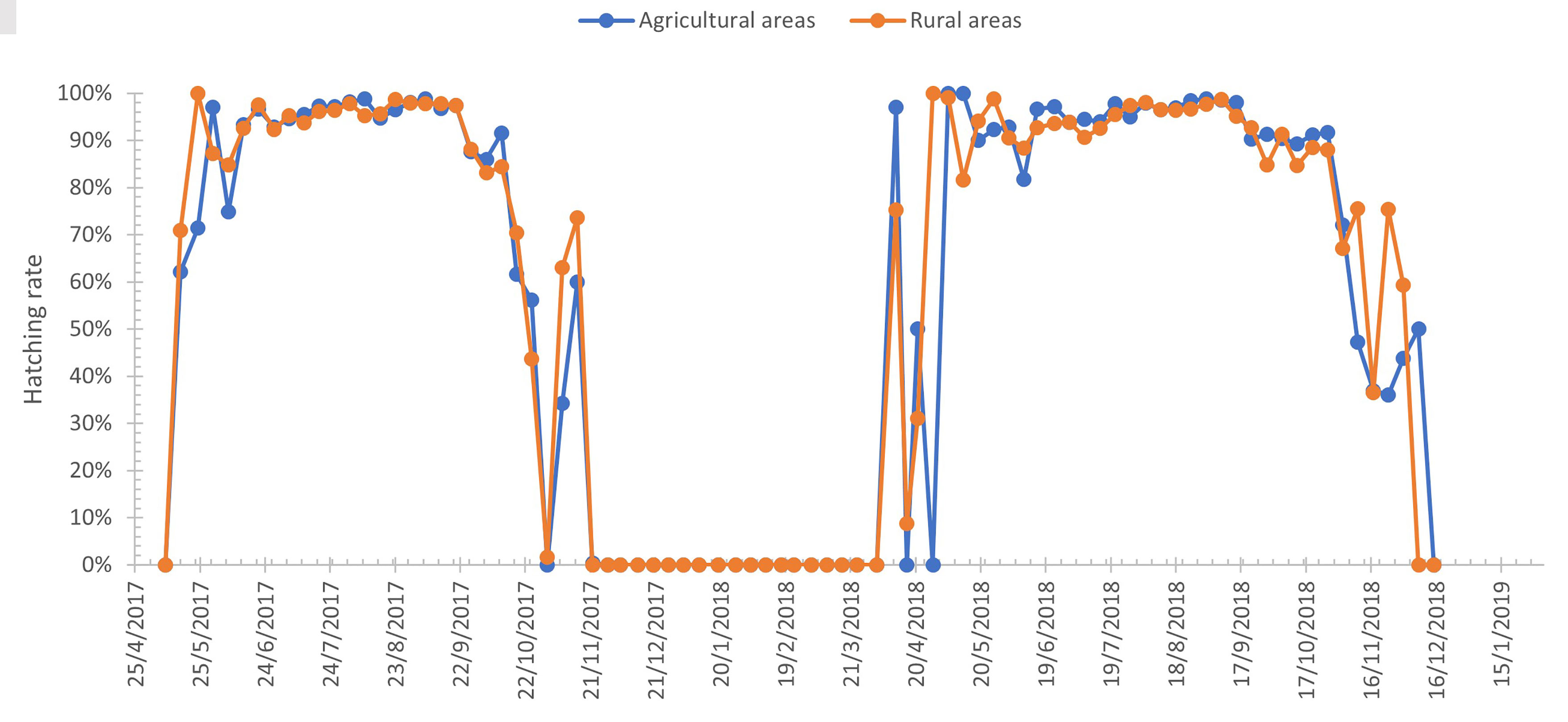
Figure 4 Hatchability rate in 2017 and 2018. The collected eggs (oviposition substrates) from 51 ovitraps were hatched weekly.
Spatial Analysis
The weekly number of eggs was used for the assessment of the spatiotemporal distribution of Ae. albopictus. Figures 5, 6 present the combined kernel density clusters and the Getis-Ord Gi* statistic of the hot spot analysis for 2017 and 2018. The maps illustrate the spatial and temporal evolution in distribution of Ae. albopictus. Kernel density maps and hot spot analysis were produced with the same legend for all studied months to enable the visual identification of trends in egg densities.
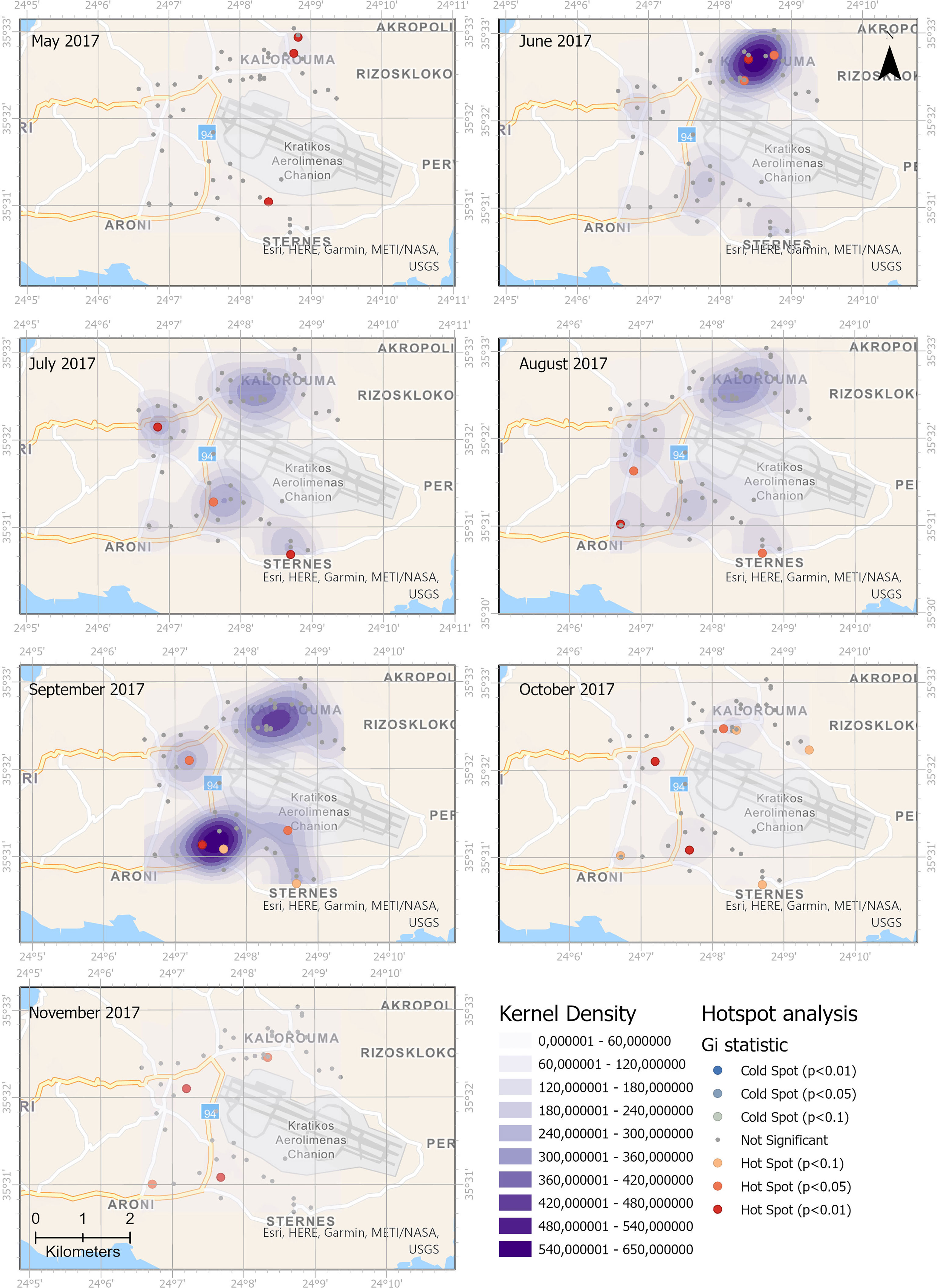
Figure 5 Spatial and temporal distribution of Aedes albopictus eggs in 2017. Monthly comparison of egg densities and hot spot analysis.
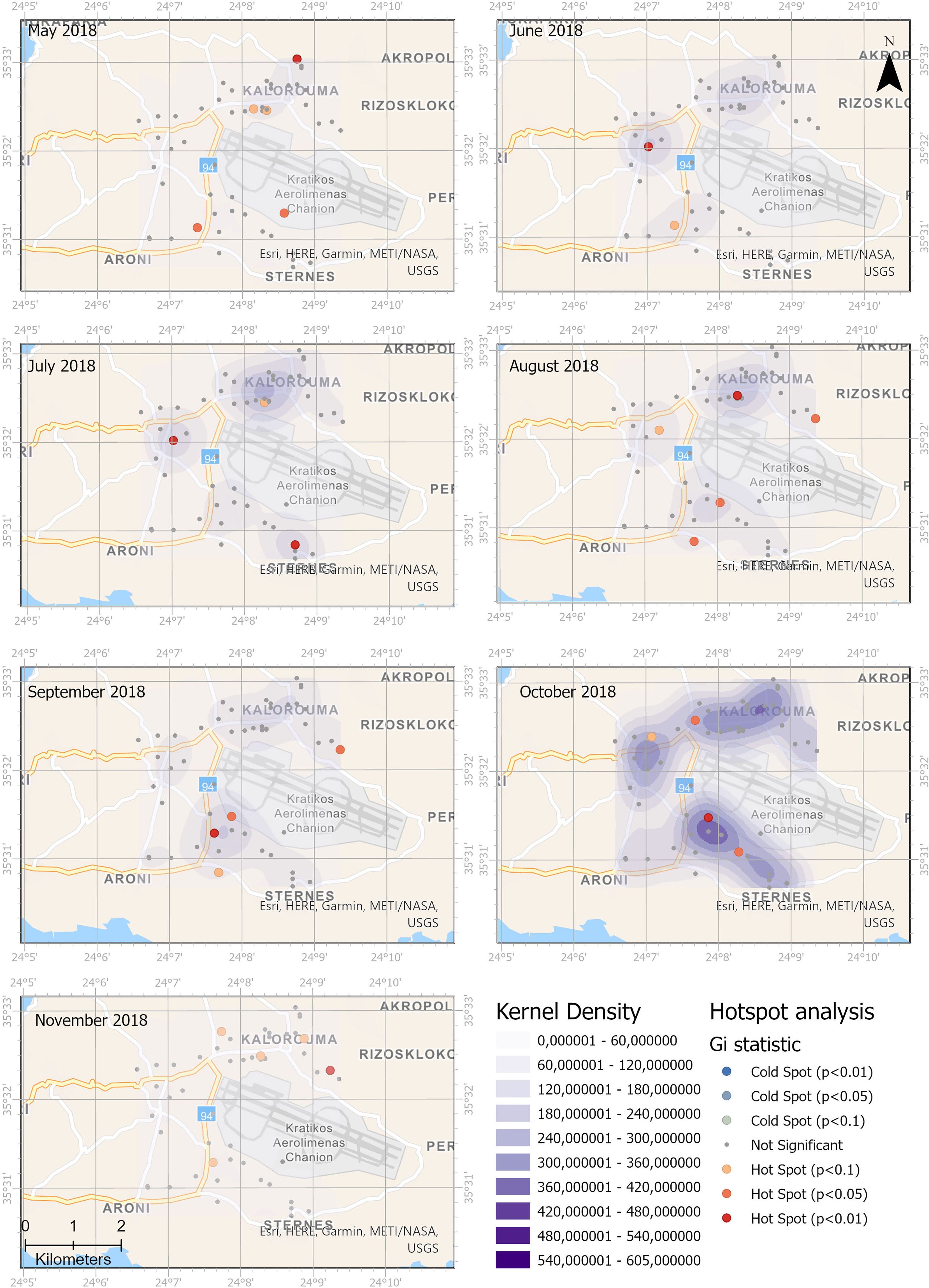
Figure 6 Spatial and temporal distribution of Aedes albopictus eggs in 2018. Monthly comparison of egg densities and hot spot analysis.
In 2017, high values of kernel densities were observed in the northern part of the study area during June, while during the following 2 months, the kernel density in this part of the area was lower and an expansion to the southwestern areas was observed. During September 2017, the highest kernel densities were observed in the southern part of the study area. During 2018, the respective spatial pattern, from northern to southern areas, was observed but with lower kernel densities.
The hot spot analysis revealed that significant hot spots (p < 0.01) in 2017 start from the northern areas and move to the southwestern part of the study area. During 2018, the hot spot analysis revealed a prevalence of significant hot spots (p > 0.05) over the rural areas. No statistically significant cold spot was observed either in 2017 or in 2018.
Discussion
Our study that has been conducted soon after Ae. albopictus introduction and establishment in the area reveals that Ae. albopictus is active and oviposits for a long period starting from May until December in the study area. Population abundance measured with MET revealed that was significantly higher in rural areas than in agricultural areas. Agricultural and rural areas though do not differ in terms of population distribution of Ae. albopictus measured with POI. The spatial analysis shows that the southern rural area presented the highest kernel densities in September 2017 and October 2018 and are identified as a persistent hot spot.
The surveillance network provided continuous monitoring of ovitraps from May 2017 until December 2018. The highest POI (>60%) was recorded in the rural areas from July until September and from June until October for 2017 and 2018, respectively. These results reveal a widely established mosquito population during the abovementioned periods probably due to favorable climatic conditions, such as increased rainfall (Supplementary Data). In a similar study (39) conducted in peridomicile and intradomicile environments in Brazil, the maximum value of POI was nearly 10%. Furthermore, this oviposition pattern is in accordance with previous studies in Greece (4, 46, 47).
For the rural areas, the abundance of the population (MET) presented the highest values in September 2017 and October 2018. The highest MET values in agricultural areas were recorded in May 2017 and October 2018. A possible explanation for these significantly higher MET values is probably due to the increased number of microhabitats that are commonly present in rural areas (1, 48). The Asian tiger mosquito can develop in a wide range of artificial breeding sites (microhabitats), such as structural containers or trash, often found in rural areas due to human activities compared with other types of landscapes (5, 26, 48). Moreover, a higher density of human population means more opportunities for Ae. albopictus blood feeding (49), while its spread could be enhanced by urbanization in combination with other socioecological challenges (4, 36, 49, 50). Nevertheless, in previous studies that compared Ae. aegypti and Ae. albopictus distribution in urban vs. rural environments, the Asian tiger mosquito was considered as a rural vector (51) due to its preference to landscapes with a high vegetation. In another similar study, examining peridomicile and intradomicile environments in Brazil, the maximum value of MET for the peridomicile environment was equal to 5 (39).
The hatching rates remained high (>90%) during the period between June and September for both years, which coincide with the findings for other areas in Greece (52). In almost all the European countries, Ae. albopictus population enters diapause and the exact period is depending on local seasonal conditions, such as temperature (53, 54). In the present study, during winter period (January–March), few eggs (in total 4) were collected but did not hatch (diapause eggs). This result demonstrates the presence of an overwintering adult population in the study area, a phenomenon similar in other European countries such as Germany (55) and Italy (56). However, in Murcia Province (Southeastern Spain), the continuity of Ae. albopictus life cycle (no diapause eggs) all over the year was recorded for the first time in Europe (57, 58), revealing a potential public health problem for southern Mediterranean countries.
In a previous study, the risk of outbreaks (R0) in four Greek localities was assessed based on Ae. albopictus population density (based on the number of eggs laid in ovitraps; 59). Results revealed that 1 year after the first detection of Ae. albopictus in Chania (2015), the R0 for the mutated Chikungunya virus was similar to that of Athens (first detection in 2008). This could indicate that Ae. albopictus invasion found suitable conditions for establishment and further expansion (31). Another interesting finding from hatching procedures is that all hatched larvae were identified as Ae. albopictus. This result is clearly indicating that the new invasion of Ae. albopictus had a negative effect on Ae. cretinus population probably due to interspecific competitive interactions, a phenomenon already known for Ae. aegypti and Ae. albopictus (60–62). Ae. cretinus, also a container-breeding mosquito, is a local species that was found in the island of Crete before invasion of Ae. albopictus, and for the last 5 years, its presence has been reported only in Rethymno (Crete) and Leros island in 2018 (3, 46, 63, 64). Furthermore, according to the findings of a recent study (62), there is evidence that Ae. albopictus is a superior competitor to Ae. cretinus, primarily at limited larval food resources (under laboratory conditions), and this may account for the expansion of Ae. albopictus and the limited presence of Ae. cretinus in areas of Athens, Greece, where these related species still coexist.
In the current study, KDE and Getis-Ord Gi* were used to reveal the spatial structure of month-to-month distribution and identify the temporal stability of Ae. albopictus presence. Although KDE and hot spot analysis may often give the same results, they are different analyses. KDE detects clusters of high values within the data, while hot spot analysis not only detects but also deepens the understanding of spatial clusters (65). A feature with a high value is certainly interesting, but it is the statistically significant hot spots that will shed light upon potential underlying spatial processes.
The produced spatial distribution revealed a heterogeneous spatial distribution of ovipositions, with increased densities over rural areas and during the months with higher POI (e.g., September 2017). In 2017, the high kernel densities were observed in June in the northern part of the study area that is a small relatively isolated village mainly focused on agricultural activities. During September of the same year, the highest kernel densities were observed in the southern part of the study area, over an urban area that is a large village, passage to the airport, with a few touristic lodges. The same south–north trend was verified also by the hot spot analysis. The southern urban area that presented the highest kernel densities in September 2017 and October 2018 is identified as a persistent hot spot mainly during the peak period of September–October for both studied years.
Conclusions
This is a thorough assessment of temporal and spatial dynamics of Ae. albopictus conducted soon after its invasion and establishment in the area. The obtained outcomes could be valuable for efficient management, while spatial and temporal identification of Ae. albopictus distribution in an area may reveal trends for maximizing surveillance efforts. Stakeholders, responsible for mosquito control, can deploy focal management strategies that are more efficient and cost-effective. Therefore, ovitraps’ surveillance can reveal specific areas that act persistently as statistically significant hot spots. Door-to-door intervention is recommended, as well as information campaigns (to raise community awareness) that can effectively reduce the initial adult mosquito population. This methodology for effective vector management can be transferred and applied to other areas with similar climatic conditions in the Mediterranean Basin. Identification of high-risk areas and focused interventions in these areas, in contrast to the usual uniform control strategies, may provide better results in vector management (66).
Data Availability Statement
The raw data supporting the conclusions of this article will be made available by the authors without undue reservation. Meteorological data were retrieved from the National Observatory of Athens (http://meteosearch.meteo.gr).
Author Contributions
Conceptualization: AS and AM. Investigation: AS, GB, DeD, DiD, and AC. Data curation: AS and DP. Writing: AS and GB. Review and editing: AS, NP, PM, DP, and AM. Funding acquisition: AM. All authors contributed to the article and approved the submitted version.
Funding
This study was supported by LIFE CONOPS project and International Atomic Energy Agency (IAEA). The project LIFE CONOPS (LIFE12 ENV/GR/000466) was funded by the European Commission in the framework of the program LIFE + Environment Policy and Governance (www.conops.gr; accessed on April 20, 2022). The TC Project RER/5/022 “Establishing Genetic Control Programs for Aedes Invasive Mosquitoes” was financed by IAEA. The funders had no role in the study design, data collection and analysis, decision to publish, or preparation of the article.
Conflict of Interest
The authors declare that the research was conducted in the absence of any commercial or financial relationships that could be construed as a potential conflict of interest.
Publisher’s Note
All claims expressed in this article are solely those of the authors and do not necessarily represent those of their affiliated organizations, or those of the publisher, the editors and the reviewers. Any product that may be evaluated in this article, or claim that may be made by its manufacturer, is not guaranteed or endorsed by the publisher.
Acknowledgments
We gratefully acknowledge Mrs. Marina Bisia and Dr. Petros Vahamidis for contributing to data preparation and statistical analysis. We also gratefully acknowledge the Municipality of Chania and Mr. Dimitrios Nikolakakis for supporting the implementation of the experiment.
Supplementary Material
The Supplementary Material for this article can be found online at: https://www.frontiersin.org/articles/10.3389/fitd.2022.811945/full#supplementary-material
References
1. Stefopoulou A., Balatsos G, Petraki A, LaDeau SL, Papachristos D, Michaelakis A. Reducing Aedes Albopictus Breeding Sites Through Education: A Study in Urban Area. PloS One (2018) 13:(11):e0202451. doi: 10.1371/journalpone.0202451
2. Estrada-Franco JG, Craig GB. Biology, disease relationships, and control of Aedes albopictus. In: OPS Cuaderno Tecnico (No. 42). Washington, DC: Organizacio´n Panamericana de la Salud (1995).
3. Becker N, Petrić D, Zgomba M, Boase C, Madon M, Dahl C, et al. Mosquitoes and Their Control. Heidelberg/Dordrecht/London/New York: Springer (2010). pp 577.
4. Stefopoulou A, LaDeau SL, Syrigou N, Balatsos G, Karras V, Papachristos DP, et al. Knowledge, Attitude and Practices Survey in Greece Before the Implementation of Sterile Insect Technique Against Aedes Albopictus. Insects (2021) 12:212. doi: 10.3390/insects12030212
5. Bellini R, Michaelakis A, Petrić D, Schaffner F, Alten B, Angelini P, et al. Practical Management Plan for Invasive Mosquito Species in Europe: I. Asian Tiger Mosquito (Aedes Albopictus). Travel Med. Infect. Dis (2020) 35:101691. doi: 10.1016/j.tmaid.2020.101691
6. Adhami J, Reiter P. Introduction and Establishment of Aedes (Stegomyia) Albopictus Skuse (Diptera: Culicidae) in Albania. J Am Mosq Control Assoc (1998) 14(3):340–3.
7. European Centre for Disease Prevention and Control and European (ECDC). Food Safety Authority. Stockholm Mosquito Maps. (2021). Available at: https://ecdc.europa.eu/en/disease-vectors/surveillance-and-disease-data/mosquito-maps.
8. Samanidou-Voyadjoglou A, Patsoula E, Spanakos G, Vakalis NC. Confirmation of Aedes Albopictus (Skuse) (Diptera: Culicidae) in Greece. Eur Mosq Bullet (2005) 19:10–1. https://e-m-b.myspecies.info/sites/e-m-b.org/files/EMB19_03.pdf
9. Patsoula E, Beleri S, Vakali A, Pervanidou D, Tegos N, Nearchou A, et al. Records of Aedes Albopictus (Skuse 1894) (Diptera; Culicidae) and Culex Tritaeniorhynchus (Diptera; Culicidae). Expans. areas mainland Greece islands Vector–borne Zoonot. (2017) 17(3):217–23. doi: 10.1089/vbz.2016.1974
10. Petrić M, Ducheyne E, Gossner CM, Marsboom C, Nicolas G, Venail C, et al. Seasonality and Timing of Peak Abundance of Aedes Albopictus in Europe: Implications to Public and Animal Health. Geospat Health (2021) 16(1):194–204. doi: 10.4081/gh.2021.996
11. Becker N, Schön S, Klein A-M, Ferstl I, Kizgin A, Tannich E, et al. First Mass Development of Aedes Albopictus (Diptera: Culicidae)—its Surveillance and Control in Germany. Parasitol Res (2017) 116(3):847–58. doi: 10.1007/s00436-016-5356-z
12. Medlock JM, Avenell D, Barrass I, Leach S. Analysis of the Potential for Survival and Seasonal Activity of Aedes Albopictus (Diptera: Culicidae) in the United Kingdom. J Vector Ecol (2006) 31(2):292–304. doi: 10.3376/1081-1710(2006)31[292:AOTPFS]2.0.CO;2
13. Gatt P, Deeming JC, Schaffner F. First Records of Aedes (Stegomyia) Albopictus (Skuse) (Diptera: Culicidae) in Malta. Eu Mosq Bull (2009) 2009:27 56–64. doi: 10.5167/uzh-23672
14. European Centre for Disease Prevention and Control (ECDC). Guidelines for the Surveillance of Native Mosquitoes in Europe. Stockholm: ECDC. (2014) https://www.ecdc.europa.eu/sites/default/files/media/en/publications/Publications/surveillance-of%20native-mosquitoes%20-guidelines.pdf.
15. Suter TT, Flacio E, Feijoó Fariña B, Engeler L, Tonolla M, Regis LN, et al. Surveillance and Control of Aedes Albopictus in the Swiss-Italian Border Region: Differences in Egg Densities Between Intervention and Non-Intervention Areas. PloS Negl Trop Dis (2016) 10(1):e0004315. doi: 10.1371/journal.pntd.0004315
16. Ravasi D, Mangili F, Huber D, Azzimonti L, Engeler L, Vermes N, et al. Risk-Based Mapping Tools for Surveillance and Control of the Invasive Mosquito Aedes Albopictus in Switzerland. Int J Environ Res Public Health (2022) 19:3220. doi: 10.3390/ijerph19063220
17. Kulisch C, Kampen H, Walther D. The Asian Tiger Mosquito Aedes Albopictus (Diptera: Culicidae) in Central Germany: Surveillance in its Northernmost Distribution Area. Acta Tropica Vol (2018) 188. Pp:78–85. doi: 10.1016/j.actatropica.2018.08.019
18. Roche B, Léger L, L’Ambert G, Lacour G, Foussadier R, Besnard G, et al. The Spread of Aedes Albopictus in Metropolitan France: Contribution of Environmental Drivers and Human Activities and Predictions for a Near Future. PloS One (2015) 10(5):e0125600. doi: 10.1371/journal.pone.0125600
19. Aranda C, Martínez Miguel J, Montalvo T, Eritja R, Navero-Castillejos J, Herreros E, et al. Arbovirus Surveillance: First Dengue Virus Detection in Local Aedes Albopictus Mosquitoes in Europe, Catalonia, Spain 2015. Euro Surveill. (2018) 23(47):pii=1700837. doi: 10.2807/1560-7917.ES.2018.23.47.1700837
20. Albieri A, Carrieri M, Angelini P, Baldacchini F, Venturelli C, Zeo SM, et al. Quantitative Monitoring of Aedes Albopictus in Emilia-Romagna, Northern Italy: Cluster Investigation and Geostatistical Analysis. Bull Insectol (2010) Vol. 63(2):209–216.
21. World Health Organisation. Handbook for Integrated Vector Management. Geneva: World Health Organization (2012). Available at: https://apps.who.int/iris/handle/10665/44768.
22. Jourdain F, Samy AM, Hamidi A, Bouattour A, Alten B, Faraj C, et al. Towards Harmonisation of Entomological Surveillance in the Mediterranean Area. PloS Negl Trop Dis (2019) 13(6):e0007314. doi: 10.1371/journal.pntd.0007314
23. Curtis A, Ye X, Heob E, Targhetta J, Salvato V, Reyna M, et al. A Comparison of Three Approaches to Identify West Nile Virus Mosquito Space-Time Hotspots in the Houston Vicinity for the Period 2002-2011. Appl Geograp (2014) 51:58–64. doi: 10.1016/j.apgeog.2014.02.003
24. Amore G, Bertolotti L, Hamer GL, Kitron UD, Walker ED, Ruiz MO, et al. Multi-Year Evolutionary Dynamics of West Nile Virus in Suburban Chicago, USA 2005-2007. Philos Trans R Soc B (2010) 365(1548):1871–8. doi: 10.1098/rstb.2010.0054
25. Carnes A, Ogneva-Himmelberger Y. Temporal Variations in the Distribution of West Nile Virus Within the United States; 2000–2008. Appl Spatial Anal (2012) 5:211–29. doi: 10.1007/s12061-011-9067-7
26. Roiz D, Ruiz S, Soriguer R, Figuerola J. Landscape Effects on the Presence, Abundance and Diversity of Mosquitoes in Mediterranean Wetlands. PloS One (2015) 10(6):e0128112. doi: 10.1371/journal.pone.0128112
27. Barrera R. Spatial Stability of Adult Aedes Aegypti Populations. Am J Trop Med Hyg (2011) 85:1087–92. doi: 10.4269/ajtmh.2011.11-0381
28. Johansson MA, Dominici F, Glass GE. Local and Global Effects of Cli-Mate on Dengue Transmission in Puerto Rico. PloS Negl Trop Dis (2009) 3(2):e382. doi: 10.1371/journal.pntd.0000382
29. Reiskin MH, Styers DM, Hayes I, Richards SL, Doyle MS, Reed EMX, et al. Short-Term, Large-Area Survey of Container Aedes Spp. (Diptera: Culicidae): Presence and Abundance is Associated With Fine-Scale Landscape Factors in North Carolina, USA. Environ Health Insights Vol (2020) 14:1–9. doi: 10.1177/1178630220952806
30. Little E, Bajwa W, Shaman J. Local Environmental and Meteorological Conditions Influencing the Invasive Mosquito Ae. Albopictus and Arbovirus Transmissionrisk in New York City. PloS Negl Trop Dis (2017) 11:e0005828. doi: 10.1371/journal.pntd.0005828
31. Badieritakis Ε., Papachristos D., Latinopoulos D., Stefopoulou A., Kolimenakis A., Bithas Κ., et al. (2017). Aedes albopictus (Skuse, 1895) (Diptera: Culicidae) in Greece: 13 years of living with the Asian tiger mosquito. Parasitology Research 117(2):453-460. doi: 10.1007/s00436-017-5721-6
32. Tagaris E, Sotiropoulou REP, Sotiropoulos A, Spanos I, Milonas P, Michaelakis A. Climate Change Impact on the Establishment of the Invasive Mosquito Species (IMS). In: Perspectives on Atmospheric Sciences. Cham: Springer (2017). p. 689–94.
33. National Public Health Organization (NPHO). Available at: https://eody.gov.gr/en/epidemiological-statistical-data/annual-epidemiological-data// (Accessed August 19, 2021).
34. UN Statistical Commission. A Recommendation on the Method to Delineate Cities, Urban and Rural Areas for International Statistical Comparisons. European Commission. (2020) https://ec.europa.eu/eurostat/cros/system/files/bg-item3j-recommendation-e.pdf.
35. Bhatt S, Gething PW, Brady OJ, Messina JP, Farlow AW, Moyes CL, et al. The Global Distribution and Burden of Dengue. Nature (2013) 496(7446):504–7. doi: 10.1038/nature12060
36. Kolimenakis A, Heinz S, Wilson ML, Winkler V, Yakob L, Michaelakis A, et al. The Role of Urbanisation in the Spread of Aedes Mosquitoes and the Diseases They Transmit—A Systematic Review. PloS Neglected Trop Dis (2021) 15(9):e0009631. doi: 10.1371/journal.pntd.000963
37. Bellini R, Calvitti M, Medici A, Carrieri M, Celli G, Maini S. Use of the Sterile Insect Technique Against Aedes Albopictus in Italy: First Results of a Pilot Trial. In: Area-Wide Control of Insect Pests: From Research to Field Implementation. Dordrecht, The Netherlands: Springer (2007). p. 505–15.
38. Bellini R, Medici A, Puggioli A, Balestrino F, Carrieri M. Pilot Field Trials With Aedes Albopictus Irradiated Sterile Males in Italian Urban Areas. J Med Entomol (2013) 50:317–25. doi: 10.1603/ME12048
39. Serpa LL, Marques GR, de Lima AP, Voltolini JC, de Brito Arduino M, Barbosa GL, et al. Study of the Distribution and Abundance of the Eggs of Aedes Aegypti and Aedes Albopictus According to the Habitat and Meteorological Variables, Municipality of São Sebastião, São Paulo State, Brazil. Parasit. Vectors (2013) 6(1):321. doi: 10.1186/1756-3305-6-321
40. Hasnan A, Che Dom N, Rosly H, Say Tiong C. Quantifying the Distribution and Abundance of Aedes Mosquitoes in Dengue Risk Areas in Shah Alam, Selangor. Proc - Soc Behav Sci (2016) 234:154–63. doi: 10.1016/j.sbspro.2016.10.230
41. Regis LN, Acioli RV, Silveira JC Jr, Melo-Santos MAV, Souza WV, Ribeiro CMN, et al. Sustained Reduction of the Dengue Vector Population Resulting From an Integrated Control Strategy Applied in Two Brazilian Cities. PloS One (2013) 8(7):e67682. doi: 10.1371/journal.pone.0067682
42. Getis A, Ord JK. The Analysis of Spatial Association by Use of Distance Statistics. Geogr Anal (1992) 4:189–206. doi: 10.1111/j.1538-4632.1992.tb00261.x
43. ESRI. How Spatial Autocorrelation (Global Moran's I) Works (2021). Available at: https://pro.arcgis.com/en/pro-app/latest/tool-reference/spatial-statistics/h-how-spatial-autocorrelation-moran-s-i-spatial-st.htm (Accessed November 8, 2021).
44. ESRI. How Hot Spot Analysis (Getis-Ord Gi*) Works (2021). Available at: https://desktop.arcgis.com/en/arcmap/latest/tools/spatial-statistics-toolbox/h-how-hot-spot-analysis-getis-ord-gi-spatial-stati.htm (Accessed November 8, 2021).
45. ESRI. How Should I Interpret the Output of Density Tools? (2021). Available at: https://www.esri.com/arcgis-blog/products/product/analytics/how-should-i-interpret-the-output-of-density-tools/ (Accessed November 8, 2021).
46. Giatropoulos A, Emmanouel N, Koliopoulos G, Michaelakis A. A Study on Distribution and Seasonal Abundance of Aedes Albopictus (Diptera: Culicidae) Population in Athens, Greece. J Med Entomol (2012) 49(2):262–9. doi: 10.1603/ME11096
47. Beleri S, Balatsos G, Karras V, Tegos N, Sereti F, Rachiotis G, et al. Seasonal Phenological Patterns and Flavivirus Vectorial Capacity of Medically Important Mosquito Species in a Wetland and an Urban Area of Attica, Greece. Trop Med Infect Dis (2021) 6(4):176. doi: 10.3390/tropicalmed6040176
48. Bodner D., LaDeau S.L., Biehler D., Kirchoff N., Leisnham P.T. Effectiveness of Print Education at Reducing Urban Mosquito Infestation through Improved Resident-Based Management. PLoS One 2016 11(5):e0155011. doi: 10.1371/journal.pone.0155011
49. Li Y, Kamara F, Zhou G, Puthiyakunnon S, Li C, Liu Y, et al. Urbanization Increases Aedes Albopictus Larval Habitats and Accelerates Mosquito Development and Survivorship. PloS Negl Trop Dis (2014) 8(11):e3301. doi: 10.1371/journal.pntd.0003301
50. Lim KW, Sit NW, Norzahira R, Sing KW, Wong HM, Chew HS, et al. Dengue Vector Surveillance in Insular Settlements of Pulau Ketam, Selangor Malaysia. Trop Biomed (2010) 27:185–92.
51. Higa Y. Dengue Vectors and Their Spatial Distribution. Trop Med Health (2011) 39:17–27. doi: 10.2149/tmh.2011-S04
52. Balatsos G, Puggioli A, Karras V, Lytra I, Mastronikolos G, Carrieri M, et al. Reduction in Egg Fertility of Aedes Albopictus Mosquitoes in Greece Following Releases of Imported Sterile Males. Insects (2021) 12:110. doi: 10.3390/insects12020110
53. Lacour G, Chanaud L, L’Ambert G, Hance T. Seasonal Synchronization of Diapause Phases in Aedes Albopictus (Diptera: Culicidae). PloS One (2015) 10(12):e0145311. doi: 10.1371/journal.pone.0145311
54. Tisseuil C, Velo E, Bino S, Kadriaj P, Mersini K, Shukullari A, et al. Forecasting the Spatial and Seasonal Dynamic of Aedes Albopictus Oviposition Activity in Albania and Balkan Countries. PloS Negl Trop Dis (2018) 12(2):e0006236. doi: 10.1371/journal.pntd.0006236
55. Pluskota B, Jöst A, Augsten X, Stelzner L, Ferstl I, Becker N. Successful Overwintering of Aedes Albopictus in Germany. Parasitol Res (2016) 115(8):3245–7. doi: 10.1007/s00436-016-5078-2
56. Toma L, Severini F, Di Luca M, Bella A, Romi R. Seasonal Patterns of Oviposition and Egg Hatching Rate of Aedes Albopictus in Rome. J Am Mosq Control Assoc (2003) 19(1):19–22.
57. Collantes F, Delgado JA, Alarcón-Elbal PM, Delacour S, Lucientes J. First Confirmed Outdoor Winter Reproductive Activity of Asian Tiger Mosquito (Aedes Albopictus) in Europe. In Anales Biología. Servicio Publicaciones la Universidad Murcia. No. (2014) 36:71–6. doi: 10.6018/analesbio.36.12
58. Bueno-Marí R, Jiménez-Peydró R. First Observations of Homodynamic Populations of Aedes Albopictus (Skuse) in Southwest Europe. J vector borne Dis (2015) 52(2):175.
59. Bellini R, Bonilauri P, Puggioli A, Lelli D, Gaibani P. Chikungunya and Dengue Risk Assessment in Greece. Vector Biol J (2016) 1(2):28–1. doi: 10.4172/2473-4810.1000108
61. Daugherty MP, Alto BW, Juliano SA. Invertebrate Carcasses as a Resource for Competing Aedes Albopictus and Aedes Aegypti (Diptera: Culicidae). J Med Entom (2000) 37:364–72. doi: 10.1093/jmedent/37.3.364
62. Giatropoulos A, Papachristos D, Michaelakis A, Kapranas A, Emmanouel N. Laboratory Study on Larval Competition Between Two Related Mosquito Species: Aedes (Stegomyia) Albopictus and Aedes (Stegomyia) Cretinus. Acta Tropica (2022) 230:106389. doi: 10.1016/j.actatropica.2022.106389
63. Giatropoulos AK, Michaelakis AN, Koliopoulos GT, Pontikakos CM. Records of Aedes Albopictus and Aedes Cretinus (Diptera: Culicidae) in Greece From 2009 to 2011. Hellenic Plant Prot J (2012) 5(2):49–56. https://www.cabi.org/isc/FullTextPDF/2012/20123271138.pdf
64. Lytra I, Balatsos G, Karras V, Stefopoulou A., Papachristos D, Michaelakis A. (2018). Finding Aedes cretinus: current state in Greece. 21st European Conference of the Society for Vector Ecology (SOVE), Palermo (Italy), 22nd –26th October 2018.
65. Kalinic M, Krisp JM. Kernel Density Estimation (KDE) vs. Hot-Spot Analysis – Detecting Criminal Hot Spots in the City of San Francisco. In AGILE Conference 2018 - 21st Conference on Geo-Information Science At: Lund. Sweden June (2018) 12-15:2018.
Keywords: mosquito surveillance, oviposition network, Geographic Information System (GIS), spatial analysis, entomological indices
Citation: Stefopoulou A, Balatsos G, Papadopoulos NT, Daskalakis D, Daskalakis D, Chatzidaki A, Milonas P, Papachristos D and Michaelakis A (2022) Spatial and Temporal Dynamics of Aedes albopictus Populations in Rural and Agricultural Areas in Chania, Greece, After Its Invasion. Front. Trop. Dis 3:811945. doi: 10.3389/fitd.2022.811945
Received: 09 November 2021; Accepted: 02 May 2022;
Published: 04 July 2022.
Edited by:
Rubén Bueno-Marí, Lokimica Laboratorios, SpainReviewed by:
Constância Flávia Junqueira Ayres, Oswaldo Cruz Foundation (Fiocruz), BrazilEleonora Flacio, University of Applied Sciences and Arts of Southern Switzerland (SUPSI), Switzerland
Copyright © 2022 Stefopoulou, Balatsos, Papadopoulos, Daskalakis, Daskalakis, Chatzidaki, Milonas, Papachristos and Michaelakis. This is an open-access article distributed under the terms of the Creative Commons Attribution License (CC BY). The use, distribution or reproduction in other forums is permitted, provided the original author(s) and the copyright owner(s) are credited and that the original publication in this journal is cited, in accordance with accepted academic practice. No use, distribution or reproduction is permitted which does not comply with these terms.
*Correspondence: Angeliki Stefopoulou, YS5zdGVmb3BvdWxvdUBicGkuZ3I=