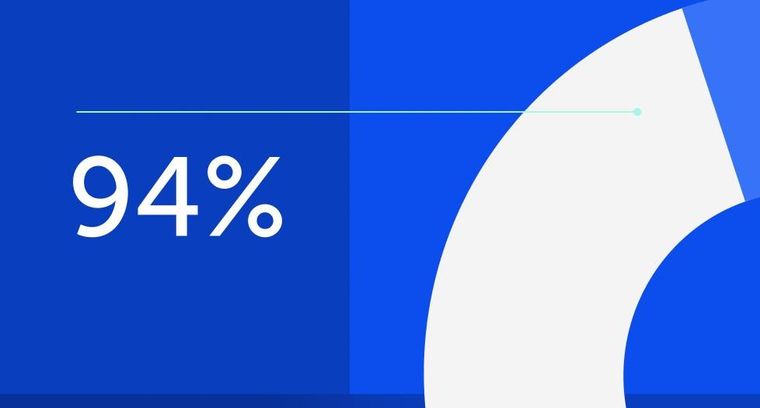
94% of researchers rate our articles as excellent or good
Learn more about the work of our research integrity team to safeguard the quality of each article we publish.
Find out more
ORIGINAL RESEARCH article
Front. Trop. Dis., 16 September 2021
Sec. Vaccines for Tropical Diseases
Volume 2 - 2021 | https://doi.org/10.3389/fitd.2021.742804
This article is part of the Research TopicVaccines against Enteric InfectionsView all 4 articles
Typhoid Vi-conjugate vaccines (Vi-TCV) have been developed to control typhoid fever in children in endemic regions. Previously, in a human challenge model of typhoid, Vi-TCV was administered prior to deliberate ingestion of Salmonella Typhi by healthy adult volunteers in the UK. Vi-specific antibody-dependent neutrophil phagocytosis (ADNP) was associated with protection against enteric fever in this model, but it is not known if ADNP is induced by vaccination of children. We measured ADNP in a cohort of Nepalese children receiving a Vi-TCV in a field study to investigate whether functional antibody responses were also present in children in an endemic setting. Furthermore, we investigated relationships between the functional antibody measures and other properties of the antibody response, including Vi-IgG and IgA titres, and Fc region glycosylation. Antibody-dependent neutrophil phagocytosis significantly increased in children aged 9 months to 15 years between the day of vaccination and 28 days following administration of Vi-TCV (D28). The magnitude of ADNP was also comparable with the levels of ADNP induced by plasma from vaccinated UK adults. Neither IgG nor IgA antibody titres significantly correlated with ADNP scores at D28; however, increased vaccine-induced ADNP was associated with decreased levels of IgG1 sialylation. These data suggest that vaccination with Vi-TCV produces functional antibody responses in children, which associate with specific glycosylation patterns of the Fc region.
Typhoid fever is a gastrointestinal infection caused by the bacterium Salmonella enterica serovar Typhi (S. Typhi), which invades through the gastrointestinal mucosa to cause systemic disease. Typhoid fever predominately affects children in low and middle-income countries where access to clean drinking water is limited (1, 2). In 2017 there were an estimated 10.9 million cases of typhoid fever resulting in approximately 116,000 deaths (3). Within Nepal, S. Typhi is the dominant cause of bloodstream bacterial infections (4), and annual incidence has been estimated up to 449 cases per 100,000 (95% CI, 383 to 521) (3). Antibiotic resistance for S. Typhi is on the rise in several South Asian countries, with nearly 65% of S. Typhi strains in Pakistan being reported as extensively drug-resistant (XDR) between 2012 and 2018 (5, 6). Given the high prevalence of typhoid fever, and increasing antimicrobial resistance, effective vaccination campaigns are needed now more than ever (7, 8). In 2018, a Vi-conjugate vaccine (Vi-TCV), which targets the Vi polysaccharide capsule of S. Typhi, was approved by the WHO, and recently demonstrated clinical efficacy of 81.6% in a field study involving over 20,000 Nepalese children (9, 10).
If correlates of protection can be identified, smaller immunogenicity cohorts can be used to bridge likely vaccine efficacy to new populations, bypassing larger scale efficacy studies and aiding new product development and vaccine deployment (11). In a human challenge model of typhoid fever, potential correlates of protection were assessed, including; Vi-antibody levels, cellular responses to vaccination, antibody-antigen binding, and Fc-mediated (fragment crystallisable) antibody effector functions (12, 13). A higher fold change in antibody-dependent phagocytosis (ADNP) was measured in participants who appeared protected from typhoid fever when compared with diagnosed individuals, from baseline to 28 and 114 days post-Vi-TCV vaccination (p=0.06, and p=0.047 respectively) (11). An increase in the neutrophil oxidative burst, which occurs downstream of phagocytosis, was also significantly higher in protected individuals at D28 (p=0.014), suggesting that antibody-induced neutrophil function may contribute to vaccine-mediated protection.
Fc-mediated effector functions, such as ADNP, are determined in part by antibody subclass and isotype, which allows the selective binding of antibodies to Fc receptors. The Fc region is modified co- and post-translationally by the glycosylation pathway which induces a large structural and functional diversification of the proteome. For IgG antibodies, N-linked glycosylation occurs on the conserved asparagine located in position 297 of the constant heavy domain (14). IgG glycosylation gives rise to a diverse range of possible IgG glycoforms, including diantennary glycans with varying levels of galactosylation, fucosylation, sialylation and bisecting N-acetylglucosamine (GlcNAc).
Through conformational changes, Fc glycosylation can influence the binding strength of antibodies to Fc receptors, and thus the magnitude of downstream cellular activity (13, 14). Generally, a loss of galactose, sialic acid and fucose is associated with increased inflammation and disease severity (15). For example, afucosylated IgG has been shown to associate with severe COVID disease and increased macrophage inflammation (16, 17). Whilst the manipulation of Fc glycoforms to alter function has long been utilised in monoclonal therapeutics, the implications of Fc glycosylation following vaccination is only just being investigated (18, 19). In 2016, Mahan et al. showed that HIV vaccination induced more inflammatory IgG glycoforms, including agalactosylation and asialylation, that were associated with higher antibody-dependent cellular cytotoxicity (ADCC) (20). However, it is not yet well understood how glycosylation influences ADNP in either infection or vaccine induced responses.
In this study we measure the capacity of vaccine induced antibodies to mediate neutrophil phagocytosis in a subset of children aged 9 months - 15 years of age in the Phase 3 TyVAC Nepal Study who are to be targeted for Vi-TCV vaccination (10). In addition, we compare antibody driven neutrophil responses in this cohort with those adults vaccinated in the UK to see if there are differences in ADNP post-vaccination between populations. Furthermore, in order to understand factors which may influence ADNP, we examine the relationships between participant age, Vi-antibody titres and Fc antibody glycosylation, and ADNP.
To examine the efficacy of the Vi-TCV vaccine amongst populations endemic for typhoid fever, children aged 9 months-16 years were vaccinated with Vi-TCV (Typbar-TCV, Bharat Biotech) in a Phase 3, double-blind, randomized controlled trial. For full details of the study see Shakya et al. (CTR: ISRCTN43385161) (10). Vaccinations took place in Lalitpur Metropolitan City, Kathmandu Valley, Nepal between the 20th of November 2017 and the 9th of April 2018. Children enrolled in the study were randomised in a 1:1 ratio to receive either the Vi-TCV or control meningitis vaccine (MenAfriVac, Serum Institute of India PVT Ltd). Blood sampling was performed at day 0 (pre-vaccination), and 28 days following vaccination for participants in the immunogenicity cohort. For our study we randomly selected 77 participants from the immunogenicity cohort who received a Vi-TCV vaccine. These participants also included 24 children who had glycosylation profiles measured who had previously been randomly selected for analysis.
Healthy UK-based adults aged between 18 and 60 years with no history of typhoid fever or vaccination, were given a single dose of either a Vi tetanus conjugate vaccine (Typbar-TCV, Bharat Biotech), Vi-PS vaccine (Typhim Vi; Sanofi Pasteur), or a control (MenACWY) vaccine (MENVEO; GlaxoSmithKline), randomised 1:1:1 in a double-blind trial as previously described (CTR: NCT02324751) (21). In order to assess efficacy of the Vi-TCV vaccine, 28 days after vaccination, participants were challenged with a dose of 1-5 x 104 CFU of S. Typhi in bicarbonate solution and monitored for a diagnosis of typhoid fever.
Participants were diagnosed with typhoid infection on presentation with a continuous fever of 38°C or higher for 12 h or longer, or by positive S. Typhi blood culture within 14 days after challenge. Blood samples were collected for serum and plasma separation at day 0 (pre-vaccination) and 28 days following vaccination (D28), before S. Typhi challenge. Thirty-seven participants received the Vi-conjugate vaccine. For the ADNP assay in our study, we randomly selected plasma from a subset of 16 Vi-TCV vaccinated individuals who were not diagnosed with typhoid fever during the study.
Anti-Vi IgG titers were measured using a commercial vaccine enzyme-linked immunosorbent assay (ELISA) kit (VaccZyme, Binding Site) according to the manufacturer’s instructions. Anti-IgA titres were measured using a modification to the same commercially available kit, replacing the Typhi Vi-IgG conjugate with a Vi-IgA conjugate. ELISA titres were measured in plasma obtained on day 0 (pre-vaccination), and 28 days post-vaccination and the data has been published previously (21, 22).
2.5 µl of biotinylated 1 mg/ml Vi-antigen (NIBSC) was added to 10 µl of red fluorescent NeutrAvidin™-Labelled microspheres (Thermofisher) per plate and incubated overnight at 4°C, or for two hours at 37°C. Prior to the assay, the beads are washed twice with 0.1%, and 5% BSA, before being resuspended in 1 ml of RPMI per plate.
Plasma samples were first heat inactivated at 56°C for 30 min. Plasma was diluted in RPMI at 1:320 for assessment of antibody-mediated neutrophil phagocytosis. Samples were then incubated for 2 hours with Vi-coupled red fluorescent microspheres (Thermofisher) in 96-well plates.
Leukocytes, including neutrophils were isolated from sodium heparin treated blood from healthy anonymised UK donors using ammonium-chloride-potassium (ACK) lysis buffer (Thermofisher). 5 x 104 leukocytes were added to each well of antibody-opsonised beads. Cells were pelleted and stained with APC-Cy7 anti-CD14 (Clone MyP9, BD Biosciences, Franklin Lakes, USA), Alexa Fluor 700 anti-CD3 (Clone UCHT1700, BD Biosciences, Franklin Lakes, USA) and Pacific Blue anti-CD66b (Clone G10F5, Biolegend, San Diego, USA).
Leukocytes were washed and analysed using a BD LSRFortessa X-20 for flow cytometry. FlowJo v10.6 software was used for gating cell populations. The phagocytic score was calculated as the mean fluorescent intensity (MFI) of red beads within gated neutrophils (CD14, CD3 negative, CD66b positive), multiplied by the percentage of bead-positive gated neutrophils.
Vi-specific IgG was captured using NeutrAvidin plates (Thermofisher), coated for 2 hours at room temperature with 100 µl biotinylated Vi (commercially biotinylated WHO international Standard Vi polysaccharide of C. freundii NIBSC code: 12/244) at 1.25 µg/ml in 0.5X PBS. After washing three times with 200 µl 0.5X PBS, 200 µl of 1:20 diluted (in 0.5X PBS) plasma was incubated for 1 hour at room temperature and then the sample transferred to another Vi-coated blank well for another 1 hour incubation. After each incubation, wells were washed once with 200 µl 0.5X PBS and three times with 200 µl freshly made 25 mM ammonium bicarbonate (Sigma). Following the washes, 50 µl of 100 mM formic acid (Sigma) was added and incubated at room temperature for 5 min with agitation. After transferring the 50 µl eluate to a clean V-bottom 96-well plate, another 50 µl 100 mM formic acid was added, incubated for 5 min with agitation and the 2 eluates from the same sample were pooled.
Samples were dried using a vacuum centrifuge for 2-3 hours at 50/60°C, and shipped to LUMC for processing as below for total IgG. Briefly, samples were resolubilised in 20 μl 50 mM ammonium bicarbonate (Sigma) while shaking for 5 min. Twenty μl of 0.05 μg/μl tosyl phenylalanyl chloromethyl ketone (TPCK)-treated trypsin (Sigma) in ice-cold purified water was added per well, and the samples were incubated at 37°C overnight.
For IgG purification, 2 μl of Protein G Sepharose 4 Fast Flow beads (GE Healthcare) were added per well on an Orochem filter plate and washed three times with PBS. 20 μl of 1:20 diluted plasma (in PBS) was added to each well. Plates were incubated for 1 hour with shaking at 750rpm. Using a vacuum manifold, the samples were washed three times by adding 400 μl PBS, followed by three times 400 μl of purified water. Antibodies were eluted from the beads by addition of 100 μl of 100 mM formic acid (Sigma) incubating for 5 min with shaking, and centrifuging at 100 × g for 2 min. Samples were dried for 2 hours at 60°C in a vacuum centrifuge before resolubilisation in 20 μl 50 mM ammonium bicarbonate (Sigma) while shaking for 5 min. Twenty μl of 0.05 μg/μl tosyl phenylalanyl chloromethyl ketone (TPCK)-treated trypsin (Sigma) in ice-cold purified water was added per well, incubated at 37°C overnight, and stored at -20°C until MS analysis.
Samples were thawed and thoroughly mixed. Two hundred nl of total IgG or 5 μl of Vi-specific IgG glycopeptide samples were injected into an Ultimate 3000 RSLCnano system (Dionex/Thermo Scientific, Breda, the Netherlands) coupled to a quadrupole-TOF-MS (MaXis HD; Bruker Daltonics, Bremen, Germany). The LC system was equipped with an Acclaim PepMap 100 trap column (particle size 5 μm, pore size 100 Å, 100 μm × 20 mm, Dionex/Thermo Scientific) and an Acclaim PepMap C18 nano analytical column (particle size 2 μm, pore size 100 Å, 75 μm × 150 mm, Dionex/Thermo Scientific). Glycopeptides were identified based on their m/z, retention time and literature (23).
Data from this study was analysed using R version 4.00. Phagocytosis data were first tested for normality using Shapiro-Wilk normality test. The Wilcoxon paired test used for comparisons between non-parametric groups, including comparisons between time points and the Wilcoxon test (unpaired) was used to test for comparisons between study cohorts. Correlation analyses of glycosylation data used Spearman’s r correlation, with 95% CI. Fold change in ADNP from baseline to D28 was calculated using the formula:
To assess the Vi-specific ADNP response in children vaccinated with Vi-TCV in Nepal, plasma samples from children vaccinated in the TyVAC study were analysed for their capacity to induce neutrophil phagocytosis at baseline and 28 days post-vaccination (n = 77). These data show that ADNP significantly increased 28 days following Vi-conjugate vaccination (p <0.0001) (Figure 1), with an average 3.6-fold increase in ADNP from baseline.
Figure 1 ADNP measured 28 days following Vi-TCV vaccination in Nepalese children. Anti-Vi ADNP was measured for 77 participants prior to and 28 days post-vaccination. The Y axis shows the phagocytic score which was calculated from bead+ neutrophils, multiplied by the MFI of the red fluorescent beads. Samples were run in technical duplicate and presented are the mean values. Each box plot shows the median and interquartile ranges per time point, and the Wilcoxon paired test was used to determine statistical significance between time points **** = (p <0.0001).
In order to compare anti-Vi ADNP responses between an adult population vaccinated in the UK and children vaccinated in Nepal, we measured ADNP for a subset of both Nepalese and UK vaccinated volunteers within the same assay (n = 17, n =16, respectively). UK participants were selected who had received the Vi-TCV vaccine and who were not diagnosed with typhoid fever in the human challenge study. Participants from the TyVAC study were selected randomly from the initial ADNP cohort. As previous functional antibody assays had been published using serum that was not collected in the TyVAC Nepal study, we reran the ADNP assay using UK participant plasma, alongside the Nepal samples.
Twenty eight days following Vi-TCV vaccination, ADNP increased in both groups when compared with baseline (p<0.0001, p < 0.001) (Figure 2). When comparing ADNP responses between cohorts there was no significant difference between the median ADNP responses at D28 in Nepalese children when compared with UK adults (Figure 2). In addition, whilst there were higher baseline ADNP levels in the UK adults compared with Nepalese children (p=0.02), the fold changes between baseline ADNP and D28 were not significantly different between study cohorts. Therefore, ADNP is induced by plasma antibodies from children vaccinated in Nepal in a similar magnitude as seen in UK adult vaccinees.
Figure 2 Comparison of ADNP between Nepalese and UK vaccines. Anti-Vi ADNP was measured pre, and 28 days post Vi-TCV vaccination in participants from the TyVAC Nepal study (n =17) and adults vaccinated in the UK (n = 16). The phagocytic scores were calculated from bead+ neutrophils, multiplied by the MFI of the red fluorescent beads. Samples were run on the same day and in technical duplicate. Presented are the mean phagocytic score per participant, pre and 28-days post vaccination. Box plot shows the median and interquartile ranges for each time point, and the Wilcoxon paired test was used to determine statistical significance between time points, **** = (p < 0.0001), *** = (p < 0.001).
When measuring anti Vi-IgG and Vi-IgA titres at baseline, there was no significant difference between our subset of UK and Nepal based vaccinees; however the titres were largely at the lower limit of detection of the ELISA assay (data not shown). Twenty eight days following vaccination, Vi-IgG titres were significantly higher in the Nepalese cohort, while no difference was observed in Vi-IgA titres (Supplementary Figure 1).
To better understand ADNP responses at both baseline and 28 days following vaccination in Nepalese children, we investigated if anti-Vi ADNP responses differed by age. We compared phagocytosis scores prior to, and 28-days after Vi-TCV vaccination in children between 0-4, 5-9 and 10-15 years of age (Figure 3) who received typhoid vaccination. At baseline, prior to vaccination, ADNP activity was higher along with increasing participant age (Figure 3A); the oldest children, 10-15 years of age, had significantly higher levels of ADNP when compared with children aged 5-9 (p<0.05) and children aged 0-4 (p<0.0001). These children also had higher levels of Vi-IgG at baseline when compared with younger children aged 5-9 (Supplementary Figure 2A). Twenty eight days following vaccination, however, plasma from all children had a similar capacity to induce neutrophil phagocytic activity, and there was no significant difference in ADNP between age groups (Figure 3B). Subsequently, due to the lower baseline in the younger children, the fold change in ADNP between baseline and D28 also differed between age groups (Figure 3C).
Figure 3 Differences in ADNP by age of vaccinated children in Nepal. (A, B) Participants were separated into groups aged 0-4 (n= 21), 5-9 (n= 24) and 10-15 years of age (n= 32). Phagocytic scores were then calculated from bead+ neutrophils, multiplied by the MFI of the red fluorescent beads, at pre and post-vaccination time points, respectively. Box plots display the median and interquartile range for each age group, and differences in anti-Vi ADNP responses between age groups were compared using the Wilcoxon test, **** = (p < 0.0001), *** = (p < 0.001). ** = (p < 0.01) * = (p < 0.05). Samples were run in technical duplicate and displayed are the mean phagocytic scores (C) The fold change in anti-Vi ADNP calculated for children in each age group following Vi-TCV vaccination. Fold change was calculated using the mean phagocytic scores pre and 28-days post Vi-TCV vaccination.
Higher levels of serum Vi-antibodies are associated with increased exposure to S. Typhi (24, 25). We therefore postulated that higher Vi-IgG or Vi-IgA titres could be driving the higher pre-vaccination ADNP levels observed in children as they age. For Vi-IgG, we did measure higher antibody titres in the older age group (10-15 years) when compared with younger children (5-9 years) at baseline (Supplementary Figure 2A). However, the correlation between Vi-IgG titres and ADNP responses pre-vaccination was weak (R =0.24, p=0.044) (Figure 4A). Additionally we did not observe a significant difference in baseline Vi-IgA titres between children as they aged in our cohort of children (Supplementary Figure 2B). No correlation was observed between baseline ADNP and Vi-IgA titres (R =-0.075, p=0.54) (Figure 4B).
Figure 4 ADNP does not correlate with Vi-IgG or Vi-IgA titre. (A, B) Scatterplot displaying anti-Vi phagocytic scores with Vi-IgG, and Vi-IgA titres at baseline (pre-vaccination), and 28 days post-vaccination. (C, D) Scatterplot displaying anti-Vi phagocytic scores and Vi-IgG and Vi-IgA and titres 28 days post-vaccination, respectively. The phagocytic scores were calculated from bead+ neutrophils, multiplied by the MFI of the red fluorescent beads. Samples were run in technical duplicates and presented are the mean phagocytic scores and ELISA units (EU/ml) per participant (n = 77).
Following vaccination, we investigated the relationship between Vi-antibody titre and ADNP. Twenty eight days after vaccination there is both an increase in Vi-antibody titres and phagocytosis scores (Supplementary Figure 3). Vi-IgG titre also increased with age (Supplementary Figure 2D). Yet as with pre-vaccination ADNP, there was no correlation between ADNP and Vi-IgG (R =0.018, p=0.89) and Vi-IgA (R =0.17, p=0.16) antibody titres (Figures 4C, D). Therefore, differences in ADNP magnitude at baseline and following vaccination are not solely due to antibody quantity and may be driven by other changes in antibody quality such as Fc receptor binding or modulation by Fc glycosylation.
Glycosylation profiles including galactosylation, sialyation and fucosylation, were measured in a randomly selected subset (n =24) of plasma samples from Nepalese children aged 9 months – 15 years. Due to the similarities in amino acid sequence in the Fc region proximal to the asparagine at position 297, IgG2 and IgG3 cannot be distinguished from each other, and were therefore recorded together. LC-MS was used to measure Fc glycopeptides, providing IgG subclass-specific glycosylation profiles for both Vi-specific antibodies and the total IgG antibody compartment (26).
We first assessed the correlation between Fc glycan traits of Vi-specific IgG1 and Vi-IgG2/3 at D28 with ADNP fold change from baseline (Figures 5A, B). We observed that lower percentages of sialylated Vi-IgG1 antibodies correlated with higher levels of ADNP induced at D28 from baseline (R = 0.47, p=0.021), (Figure 5C). A lower percentage of terminal galactose residues, on the Fc domain of Vi-IgG1 28 days post-vaccination, also correlated with ADNP at D28 (R = 0.38, p=0.067) (Figure 5D), however this was not statistically significant. Moreover, when breaking down galactosylation levels into the percentage of agalactosylated (G0), monogalactosylated (G1), and digalactosylated (G2) Vi-IgG1 antibodies; a higher percentage of monogalactosylated Vi-IgG1 correlated with levels of ADNP at D28 from baseline (R = 0.48, p=0.028), whereas the percentage of digalactosylated Vi-IgG1 inversely correlated with ADNP (R = -0.047, p=0.021) (Supplementary Figures 4B, C).
Figure 5 Correlations of Vi-IgG glycosylation states with ADNP fold change. Spearman’s correlation of the percentage of Vi-IgG1 (A), and Vi-IgG2/3 (B) sialylation, galactosylation, and GlcNAc bisection at day 28, with the fold change in ADNP between baseline and D28, as well as the participant age (n =24). The colour of the square indicates the strength of the correlation (Rho) and the p-value is noted within each box. Correlation of the percentage of Vi-IgG1 sialylation (C), galactosylation (D), and sialic acid per galactose residues (S/G) (E) at day 28, respectively, with the fold change in ADNP between baseline and D28 (n =24). Shown for each graph are the Spearman’s Rho and p-values for significance. In addition, the black line indicates the linear regression, and the shaded area shows 95% confidence intervals for the model.
When measuring Fc glycosylation of Vi-IgG2 and 3 at D28, we observed similar patterns as seen with Vi-IgG1 and ADNP, whereby lower percentages of sialylation and galactosylation were associated with an increase in ADNP from baseline, however these were non-significant (Figure 5B). Furthermore, when investigating the associations of G0, G1 and G2 galactosylation levels with ADNP, monogalactosylation of Vi-IgG2/3 also correlated with ADNP fold change (R= 0.43, p=0.038) (Supplementary Figure 4E).
As sialyation biosynthetically occurs following galactosylation, and the two have been shown to correlate in previous studies (27), we investigated if sialyation and galactosylation of Vi-specific antibodies correlated. We observed a strong correlation between the percentage of galactosylation and sialyation of Vi-IgG1 (p<0.0001) (Figure 5A), confirming that the two processes are linked. To understand if there was a sialyation specific effect on ADNP, we correlated the number of sialic acid residues per galactose (S/G) with ADNP fold change from baseline. For Vi-IgG1, we observed a significant correlation between lower percentages of sialyation per galactose and ADNP at D28 (R = 0.43, p=0.035) (Figure 5E). Thus, suggesting, that for Vi-IgG1, sialyation of the Fc region may have a specific effect on the capacity for antibodies to induce ADNP following vaccination, in conjunction with galactosylation.
To confirm that these associations with ADNP were driven by Vi-specific antibodies and not by glycosylation profiles of all non-Vi specific IgG antibodies, we correlated total IgG glycosylation profiles at D28 with ADNP. No associations with antibody-induced neutrophil function were observed (data not shown). Furthermore, we did not correlate fucosylation levels of the Fc domain 28 days following vaccination with ADNP due to the consistently high fucosylation levels across participants (Supplementary Figure 5). We also examined if age was a factor in driving glycosylation states at D28 and associations with ADNP, as glycosylation states at baseline have been known to vary with the age of children and adolescents (28). Therefore, we tested for interactions between age and Vi-IgG1 galactosylation and sialylation in a linear model to predict ADNP fold change. This model showed that the interaction between the two glycosylation profiles with age, respectively, was non-significant (p=0.95, p=0.64).
The capacity of antibodies to induce neutrophil activity, including phagocytosis, has been shown to associate with protection following Vi-TCV vaccination prior to S. Typhi exposure in a UK based human challenge model (21). Our findings demonstrate that Vi-TCV vaccination also gives rise to a significant increase in capacity for Vi-specific antibody-dependent neutrophil phagocytosis amongst Nepalese children aged 9 months - 15 years. We found that the level of ADNP activity reached after vaccination was unaffected by age, despite differences in pre-vaccination Vi-IgG titres and ADNP levels. Encouragingly, 28 days after vaccination, ADNP activity measured in plasma from these children was present at similar levels to ADNP activity measured in plasma from UK adults. In addition, we found that lower abundances of sialic acid of Vi-specific IgG1 correlated with higher levels of ADNP 28 days following immunisation.
Antibody-induced neutrophil effector functions provide important immune responses against S. Typhimurium infections, localizing Salmonellae to the gut (29). Conversely, during infection with S. Typhi, the Vi capsule enables the bacterium to evade antibody-mediated neutrophil responses (30, 31), and in vitro models of S. Typhi have shown its ability to evade the oxidative burst and phagosome maturation within monocytes and neutrophils after phagocytosis (32, 33), ultimately resulting in bacterial dissemination. Vaccination with Vi-TCV however induces gut homing plasma cells that produce Vi-specific antibodies (34). Given the increase in ADNP following vaccination, these antibodies may have a role in activating Fc receptors on neutrophils to bring about phagocytosis, thus overcoming the natural ability of S. Typhi to evade neutrophil-mediated responses. Consequently, ADNP may be playing a mechanistic role in vaccine-mediated protection.
In order to better understand which antibody isotypes may be driving neutrophil phagocytosis, we correlated Vi-IgA and Vi-IgG titres with ADNP. Previous analyses demonstrated that Vi-IgA antibody levels were associated with vaccine-mediated protection (13). Neutrophils express the highest levels of the IgA receptor, CD89, of any phagocytic immune cell type (35, 36) and IgA has also been shown to be the primary isotype responsible for monoclonal-induced phagocytosis of tumour cells by neutrophils (37). In the context of SARS-CoV-2 infection, high levels of IgA and ADNP have both been associated with increased inflammation (38), highlighting the potentially synergistic effect these pathways may play in disease as well as protection. It is therefore possible that Vi-IgA and ADNP could be acting in tandem, with Vi-IgA mediating ADNP following vaccination. Whilst there is an increase in ADNP from baseline following vaccination, together with increasing Vi-IgA and IgG titres, neither Vi-IgA nor Vi-IgG titres correlated strongly with post-vaccination or baseline anti-Vi ADNP responses in Nepalese children.
These data suggest that qualitative antibody properties such as Fc glycosylation may be affecting the magnitude of antibody-dependent neutrophil phagocytosis. We observed distinct Fc glycan characteristics of Vi-specific IgG1 antibodies measured 28 days following vaccination, which associated with an increase in ADNP post-vaccination, featuring a lower abundance of sialic acid on the Vi-IgG1 Fc domain. Furthermore, an increase in the number of sialic acid residues per galactose, also correlated with reducing ADNP levels. Sialylation of IgG Fc has been associated with reduced FcγRIIIa binding and ADCC function of IgG1 (39, 40), and sialylation is also responsible for anti-inflammatory effects of intravenous immunoglobulin treatments (41).
As well as IgG sialylation, antigen-specific Fc galactosylation has been demonstrated to influence Fc-mediated antibody effector functions against pathogens such as HIV (42), and agalactosylation has been implicated in increasing inflammation within active tuberculosis (43, 44). In this context we show that both Vi-IgG sialylation, and, to a lesser extent, galactosylation levels associate with ADNP activity after Vi-TCV vaccination. Removal of sialic acid and galactose on IgG Fc has been shown to induce a conformational change in the IgG Fc hinge region, resulting in antibody structures that are more accessible to Fc receptors, and thus stronger binding of IgG to Fc receptors (45). Through greater cross-linking of Fc receptors, increased binding of immunoglobulin to Fc receptors has been shown to impact responses downstream from ADNP in other studies, including the oxidative burst (46, 47). We did not find significant associations with Vi-IgG2 and 3 sialylation with ADNP at Day 28, however glycosylation states displayed a similar relationship as seen in with Vi-IgG1. IgG1 has the highest affinity for Fc γ receptors, therefore it is possible that this is a greater driver of ADNP compared with Vi-IgG2 and 3 (48).
While our study did not measure IgA Fc glycosylation, reduced sialylation on IgA has been demonstrated to increase Fc-mediated neutrophil and monocyte responses within inflammatory rheumatoid arthritis (49, 50). Given the role IgA plays in limiting S. Typhi infection, and its associations with vaccine mediated-protection and neutrophil responses (51, 52), future studies investigating the role of IgA Fc glycosylation and downstream effector functions post-vaccination could help understand the potential relationship between IgA and ADNP further. Our study also used neutrophils from healthy adults in the UK. Neutrophil function in target vaccine populations may differ, as neutropenia, for example, may be more common in African and South Asian populations where HIV prevalence is high (53). Monitoring vaccine efficacy in these subgroups should therefore be considered when deploying the Vi-conjugate vaccine in different regions.
Due to the low abundance of Vi-antibodies at baseline, we did not examine changes in glycosylation between baseline and Day 28 with ADNP in this study. Several studies have shown that antigen-specific IgG Fc sialylation and galactosylation increases after vaccination (54, 55). Future work evaluating the impact of Vi-TCV vaccination on Fc glycosylation states from baseline will therefore help understand the influence vaccination has on this response.
In this study, we show that vaccination increases ADNP in Nepalese children, and that ADNP associated with Vi-IgG1 Fc sialyation measured at Day 28. Fc-mediated antibody functions are increasingly recognised in evaluating vaccine immunity to many infections, including typhoid fever, HIV, and SARS-CoV-2 (40, 56). Furthermore, recent research has shown that the adjuvant and site of vaccine delivery can alter antibody glycosylation profiles, and thus function (57). Decoding factors driving Fc-mediated effector functions could therefore provide pathways for targeted vaccine design and implementation, including emerging typhoid and paratyphoid vaccine candidates (58).
The raw data supporting the conclusions of this article will be made available by the authors, without undue reservation.
The TyVAC Nepal study was approved by the Oxford Tropical Research Ethics Committee and the Nepal Health Research Council. The VAST human challenge study was approved by the sponsor (University of Oxford), the South Central Oxford A Ethics Committee (14/SC/1427), and the Medicines and Healthcare Products Regulatory Agency (Eudract 2014-002978-36). Written informed consent to participate in this study was provided by the participants’ legal guardian/next of kin.
MJ, LS, NH, MW, JH, and AP conceived and designed the work. Methodology was developed by MJ, LS, JH, NH, MW, JN, CK. CJ, EJ, JH, and AP carried out the VAST clinical trial. MS, RC-J, KT-N, MV, DP, SK, SD, AK, SS, BB, and AP carried out the TyVAC clinical trial. MJ, LS, NH, JN, CK, JC, SM, and EJ acquired data for the study. MJ performed statistical analysis of the study, and MV also provided statistical support and database management. MJ wrote the original draft of the manuscript. LS, NH, MW, JN, MV, CJ, SS, JH, and AP edited and contributed to the manuscript. LS, JH, and AP provided project supervision. All authors contributed to the article and approved the submitted version.
The views expressed in this article do not necessarily represent the views of DHSC, JCVI, NIHR or WHO.
AP is Chair of UK Dept. Health and Social Care’s (DHSC) Joint Committee on Vaccination & Immunisation (JCVI). He is a member of the WHO’s SAGE. AP is an NIHR Senior Investigator. Oxford University has entered a joint COVID19 vaccine development partnership with Astra Zeneca.
The remaining authors declare that the research was conducted in the absence of any commercial or financial relationships that could be construed as a potential conflict of interest.
All claims expressed in this article are solely those of the authors and do not necessarily represent those of their affiliated organizations, or those of the publisher, the editors and the reviewers. Any product that may be evaluated in this article, or claim that may be made by its manufacturer, is not guaranteed or endorsed by the publisher.
The Supplementary Material for this article can be found online at: https://www.frontiersin.org/articles/10.3389/fitd.2021.742804/full#supplementary-material
1. Ochiai RL, Acosta CJ, Danovaro-Holliday MC, Baiqing D, Bhattacharya SK, Agtini MD, et al. A Study of Typhoid Fever in Five Asian Countries: Disease Burden and Implications for Controls. Bull World Health Organ (2008) 86:260–8. doi: 10.2471/BLT.06.039818
2. Antillón M, Warren JL, Crawford FW, Weinberger DM, Kürüm E, Pak GD, et al. The Burden of Typhoid Fever in Low- and Middle-Income Countries: A Meta-Regression Approach. PloS Negl Trop Dis (2017) 11:e0005376. doi: 10.1371/journal.pntd.0005376
3. Stanaway JD, Reiner RC, Blacker BF, Goldberg EM, Khalil IA, Troeger CE, et al. The Global Burden of Typhoid and Paratyphoid Fevers: A Systematic Analysis for the Global Burden of Disease Study. Lancet Infect Dis (2019) 19(4):369–81. doi: 10.1016/S1473-3099(18)30685-6
4. Zellweger RM, Basnyat B, Shrestha P, Prajapati KG, Dongol S, Sharma PK, et al. A 23-Year Retrospective Investigation of Salmonella Typhi and Salmonella Paratyphi Isolated in a Tertiary Kathmandu Hospital. PloS Negl Trop Dis (2017) 11(11):e0006051. doi: 10.1371/journal.pntd.0006051
5. Dyson ZA, Klemm EJ, Palmer S, Dougan G. Antibiotic Resistance and Typhoid. Clin Infect Dis (2019) 68:S165–70. doi: 10.1093/cid/ciy1111
6. Umair M, Siddiqui SA. Antibiotic Susceptibility Patterns of Salmonella Typhi and Salmonella Paratyphi in a Tertiary Care Hospital in Islamabad. Cureus (2020) 12(9):e10228. doi: 10.7759/cureus.10228
7. Marchello CS, Birkhold M, Crump JA. Complications and Mortality of Typhoid Fever: A Global Systematic Review and Meta-Analysis. J Infect (2020) 81:902–10. doi: 10.1016/j.jinf.2020.10.030
8. O’Reilly PJ, Pant D, Shakya M, Basnyat B, Pollard AJ. Progress in the Overall Understanding of Typhoid Fever: Implications for Vaccine Development. Expert Rev Vaccines (2020) 19:367–82. doi: 10.1080/14760584.2020.1750375
9. World Health Organization. Typhoid Vaccines: WHO Position Paper, March 2018 – Recommendations. Vaccine (2019) 37:214–6. doi: 10.1016/j.vaccine.2018.04.022
10. Shakya M, Colin-Jones R, Theiss-Nyland K, Voysey M, Pant D, Smith N, et al. Phase 3 Efficacy Analysis of a Typhoid Conjugate Vaccine Trial in Nepal. N Engl J Med (2019) 381:2209–18. doi: 10.1056/NEJMoa1905047
11. Blohmke CJ, O’Connor D, Pollard AJ. The Use of Systems Biology and Immunological Big Data to Guide Vaccine Development. Genome Med (2015) 7:114. doi: 10.1186/s13073-015-0236-1
12. Jin C, Hill J, Gunn BM, Yu W-H, Dahora LC, Jones E, et al. Vi-Specific Serological Correlates of Protection for Typhoid Fever. J Exp Med (2020) 218(2):e20201116. doi: 10.1084/jem.20201116
13. Dahora LC, Jin C, Spreng RL, Feely F, Mathura R, Seaton KE, et al. Iga and Igg1 Specific to Vi Polysaccharide of Salmonella Typhi Correlate With Protection Status in a Typhoid Fever Controlled Human Infection Model. Front Immunol (2019) 10:2582. doi: 10.3389/fimmu.2019.02582
14. Rudd PM, Leatherbarrow RJ, Rademacher TW, Dwek RA. Diversification of the Igg Molecule by Oligosaccharides. Mol Immunol (1991) 28:1369–78. doi: 10.1016/0161-5890(91)90039-M
15. Irvine EB, Alter G. Understanding the Role of Antibody Glycosylation Through the Lens of Severe Viral and Bacterial Diseases. Glycobiol (2020) 30:241–53. doi: 10.1093/glycob/cwaa018
16. Chakraborty S, Gonzalez J, Edwards K, Mallajosyula V, Buzzanco AS, Sherwood R, et al. Proinflammatory Igg Fc Structures in Patients With Severe COVID-19. Nat Immunol (2021) 22:67–73. doi: 10.1038/s41590-020-00828-7
17. Hoepel W, Chen H-J, Geyer CE, Allahverdiyeva S, Manz XD, de Taeye SW, et al. High Titers and Low Fucosylation of Early Human Anti-SARS-Cov-2 Igg Promote Inflammation by Alveolar Macrophages. Sci Transl Med (2021) 13(596):eabf8654. doi: 10.1126/scitranslmed.abf8654
18. Golay J, Da Roit F, Bologna L, Ferrara C, Leusen JH, Rambaldi A, et al. Glycoengineered CD20 Antibody Obinutuzumab Activates Neutrophils and Mediates Phagocytosis Through CD16B More Efficiently Than Rituximab. Blood (2013) 122:3482–91. doi: 10.1182/blood-2013-05-504043
19. Kuhns S, Shu J, Xiang C, De Guzman R, Zhang Q, Bretzlaff W, et al. Differential Influence on Antibody Dependent Cellular Phagocytosis by Different Glycoforms on Therapeutic Monoclonal Antibodies. J Biotechnol (2020) 317:5–15. doi: 10.1016/j.jbiotec.2020.04.017
20. Mahan AE, Jennewein MF, Suscovich T, Dionne K, Tedesco J, Chung AW. Antigen-Specific Antibody Glycosylation Is Regulated via Vaccination. PLoS Pathog (2016) 12(3):e1005456. doi: 10.1371/journal.ppat.1005456
21. Jin C, Gibani MM, Moore M, Juel HB, Jones E, Meiring J, et al. Efficacy and Immunogenicity of a Vi-Tetanus Toxoid Conjugate Vaccine in the Prevention of Typhoid Fever Using a Controlled Human Infection Model of Salmonella Typhi: A Randomised Controlled, Phase 2b Trial. Lancet (2017) 390:2472–80. doi: 10.1016/S0140-6736(17)32149-9
22. Voysey M, Pollard AJ. Seroefficacy of VI Polysaccharide–Tetanus Toxoid Typhoid Conjugate Vaccine (Typbar TCV). Clin Infect Dis (2018) 67:18–24. doi: 10.1093/cid/cix1145
23. Falck D, Jansen BC, de Haan N, Wuhrer M. High-Throughput Analysis of Igg Fc Glycopeptides by LC-MS. In: Methods in Molecular Biology, vol. 1503. New York: Humana Press Inc. (2017). p. 31–47.
24. Watson CH, Baker S, Lau CL, Rawalai K, Taufa M, Coriakula J, et al. A Cross-Sectional Seroepidemiological Survey of Typhoid Fever in Fiji. PloS Negl Trop Dis (2017) 11(7):e0005786. doi: 10.1371/journal.pntd.0005786
25. House D, et al. Antibodies to the Vi Capsule of Salmonella Typhi in the Serum of Typhoid Patients and Healthy Control Subjects From a Typhoid Endemic Region. J Infect Dev Ctries (2008) 2:308–12. doi: 10.3855/jidc.227
26. Chandler KB, Mehta N, Leon DR, Suscovich TJ, Alter G, Costello CE. Multi-Isotype Glycoproteomic Characterization of Serum Antibody Heavy Chains Reveals Isotype- and Subclass-Specific N-Glycosylation Profiles. Mol Cell Proteomics (2019) 18:686–703. doi: 10.1074/mcp.RA118.001185
27. Van de Geijn FE, Wuhrer M, Selman MHJ, Willemsen SP, de Man YA, Deelder AM, et al. Immunoglobulin G Galactosylation and Sialylation Are Associated With Pregnancy-Induced Improvement of Rheumatoid Arthritis and the Postpartum Flare: Results From a Large Prospective Cohort Study. Arthritis Res Ther (2009) 11:R193. doi: 10.1186/ar2892
28. de Haan N, Reiding KR, Driessen G, van der Burg M, Wuhrer M. Changes in Healthy Human Igg Fc-Glycosylation After Birth and During Early Childhood. J Proteome Res (2016) 15:1853–61. doi: 10.1021/acs.jproteome.6b00038
29. Broz P, Ohlson MB, Monack DM. Innate Immune Response to Salmonella Typhimurium, a Model Enteric Pathogen. Gut Microbes (2012) 3:62–70. doi: 10.4161/gmic.19141
30. Wangdi T, Lee CY, Spees AM, Yu C, Kingsbury DD, Winter SE, et al. The Vi Capsular Polysaccharide Enables Salmonella Enterica Serovar Typhi to Evade Microbe-Guided Neutrophil Chemotaxis. PloS Pathog (2014) 10(8):e1004306. doi: 10.1371/journal.ppat.1004306
31. Hiyoshi H, Wangdi T, Lock G, Saechao C, Raffatellu M, Cobb BA, et al. Mechanisms to Evade the Phagocyte Respiratory Burst Arose by Convergent Evolution in Typhoidal Salmonella Serovars. Cell Rep (2018) 22:1787. doi: 10.1016/j.celrep.2018.01.016
32. Vazquez-Torres A, Xu Y, Jones-Carson J, Holden DW, Lucia SM, Dinauer MC, et al. Salmonella Pathogenicity Island 2-Dependent Evasion of the Phagocyte NADPH Oxidase. Sci (80-) (2000) 287:1655–8. doi: 10.1126/science.287.5458.1655
33. Crawford RW, Wangdi T, Spees AM, Xavier MN, Tsolis RM, Bäumler AJ. Loss of Very-Long O-Antigen Chains Optimizes Capsule-Mediated Immune Evasion by Salmonella Enterica Serovar Typhi. MBio (2013) 4(4):e00232–13. doi: 10.1128/mBio.00232-13
34. Cross DL, Verheul MK, Leipold MD, Obermoser G, Jin C, Jones E, et al. Vi-Vaccinations Induce Heterogeneous Plasma Cell Responses That Associate With Protection From Typhoid Fever. Front Immunol (2020) 11:3020. doi: 10.3389/fimmu.2020.574057.
35. Cheeseman HM, Carias AM, Evans AB, Olejniczak NJ, Ziprin P, King DFL, et al. Expression Profile of Human Fc Receptors in Mucosal Tissue: Implications for Antibody-Dependent Cellular Effector Functions Targeting HIV-1 Transmission. PloS One (2016) 11(5):e0154656. doi: 10.1371/journal.pone.0154656
36. Morton HC, Brandtzaeg P. CD89: The Human Myeloid Iga Fc Receptor. Arch Immunol Ther Exp (2001) 49:217–29.
37. Heemskerk N, van Egmond M. Monoclonal Antibody-Mediated Killing of Tumour Cells by Neutrophils. Eur J Clin Invest (2018) 48 Suppl 2(Suppl Suppl 2):e12962. doi: 10.1111/eci.12962
38. Zohar T, Loos C, Fischinger S, Atyeo C, Wang C, Slein MD, et al. Compromised Humoral Functional Evolution Tracks With SARS-Cov-2 Mortality. Cell (2020) 183:1508–19.e12. doi: 10.1016/j.cell.2020.10.052
39. Roberts JT, Patel KR, Barb AW. Site-Specific N-Glycan Analysis of Antibody-Binding Fc γ Receptors From Primary Human Monocytes. Mol Cell Proteomics (2020) 19:362–74. doi: 10.1074/mcp.RA119.001733
40. Barrett JR, Belij-Rammerstorfer S, Dold C, Ewer KJ, Folegatti PM, Gilbride C, et al. Phase 1/2 Trial of SARS-Cov-2 Vaccine Chadox1 Ncov-19 With a Booster Dose Induces Multifunctional Antibody Responses. Nat Med (2020) 27:279–88. doi: 10.1038/s41591-020-01179-4
41. Ahmed AA, Giddens J, Pincetic A, Lomino JV, Ravetch JV, Wang LX, et al. Structural Characterization of Anti-Inflammatory Immunoglobulin G Fc Proteins. J Mol Biol (2014) 426:3166–79. doi: 10.1016/j.jmb.2014.07.006
42. Muenchhoff M, Chung AW, Roider J, Dugast A-S, Richardson S, Kløverpris H, et al. Distinct Immunoglobulin Fc Glycosylation Patterns Are Associated With Disease Nonprogression and Broadly Neutralizing Antibody Responses in Children With HIV Infection. mSphere (2020) 5(6):e00880–20. doi: 10.1128/mSphere.00880-20
43. Pilkington C, Yeung E, Isenberg D, Lefvert AK, Rook GAW. Agalactosyl Igg and Antibody Specificity in Rheumatoid Arthritis, Tuberculosis, Systemic Lupus Erythematosus and Myasthenia Gravis. Autoimmunity (1995) 22:107–11. doi: 10.3109/08916939508995306
44. Lu LL, et al. Antibody Fc Glycosylation Discriminates Between Latent and Active Tuberculosis. J Infect Dis (2020) 222:2093–102. doi: 10.1093/infdis/jiz643
45. Krapp S, Mimura Y, Jefferis R, Huber R, Sondermann P. Structural Analysis of Human Igg-Fc Glycoforms Reveals a Correlation Between Glycosylation and Structural Integrity. J Mol Biol (2003) 325:979–89. doi: 10.1016/S0022-2836(02)01250-0
46. Strohmeier GR, Brunkhorst BA, Seetoo KF, Bernardo J, Weil GJ, Simons ER. Neutrophil Functional Responses Depend on Immune Complex Valency. J Leukoc Biol (1995) 58:403–14. doi: 10.1002/jlb.58.4.403
47. Nesspor TC, Raju TS, Chin C-N, Vafa O, Brezski RJ. Avidity Confers Fcgr Binding and Immune Effector Function to Aglycosylated Immunoglobulin G1. (2012) 25:147–54. doi: 10.1002/jmr.2155
48. Gunn BM, Alter G. Modulating Antibody Functionality in Infectious Disease and Vaccination. Trends Mol Med (2016) 22:969–82. doi: 10.1016/j.molmed.2016.09.002
49. Steffen U, Koeleman CA, Sokolova MV, Bang H, Kleyer A, Rech J, et al. Iga Subclasses Have Different Effector Functions Associated With Distinct Glycosylation Profiles. Nat Commun (2020) 11(1):120. doi: 10.1038/s41467-019-13992-8
50. Breedveld A, Van Egmond M. Iga and Fcαri: Pathological Roles and Therapeutic Opportunities. Front Immunol (2019) 10:553. doi: 10.3389/fimmu.2019.00553
51. Michetti P, Mahan MJ, Slauch JM, Mekalanos JJ, Neutra MR. Monoclonal Secretory Immunoglobulin a Protects Mice Against Oral Challenge With the Invasive Pathogen Salmonella Typhimurium. Infect Immun (1992) 60:1786–92. doi: 10.1128/iai.60.5.1786-1792.1992
52. Wahid R, Pasetti MF, Maciel M, Simon JK, Tacket CO, Levine MM, et al. Oral Priming With Salmonella Typhi Vaccine Strain CVD 909 Followed by Parenteral Boost With the s. Typhi Vi Capsular Polysaccharide Vaccine Induces CD27+Igd- s. Typhi-Specific Iga and Igg B Memory Cells in Humans. Clin Immunol (2011) 138:187–200. doi: 10.1016/j.clim.2010.11.006
53. Shi X, Sims MD, Hanna MM, Xie M, Gulick PG, Zheng Y-H, et al. Neutropenia During HIV Infection: Adverse Consequences and Remedies. Int Rev Immunol (2014) 33:511. doi: 10.3109/08830185.2014.893301
54. Selman MHJ, de Jong SE, Soonawala D, Kroon FP, Adegnika AA, Deelder AM, et al. Changes in Antigen-Specific Igg1 Fc N-Glycosylation Upon Influenza and Tetanus Vaccination. Mol Cell Proteomics (2012) 11(4):M111.014563. doi: 10.1074/MCP.M111.014563
55. Vestrheim AC, Moen A, Egge-Jacobsen W, Reubsaet L, Halvorsen TG, Bratlie DB, et al. A Pilot Study Showing Differences in Glycosylation Patterns of Igg Subclasses Induced by Pneumococcal, Meningococcal, and Two Types of Influenza Vaccines. Immun Inflamm Dis (2014) 2(2):76–91. doi: 10.1002/iid3.22
56. Arnold KB, Chung AW. Prospects From Systems Serology Research. Immunol (2018) 153:279–89. doi: 10.1111/imm.12861
57. Bartsch YC, Eschweiler S, Leliavski A, Lunding HB, Wagt S, Petry J, et al. Igg Fc Sialylation Is Regulated During the Germinal Center Reaction Following Immunization With Different Adjuvants. J Allergy Clin Immunol (2020) 146:652–66.e11. doi: 10.1016/j.jaci.2020.04.059
Keywords: vaccination, typhoid fever, glycosylation, Fc-mediated effector function, correlates of protection, antibody-dependent neutrophil phagocytosis, Salmonella
Citation: Johnson M, Stockdale L, de Haan N, Wuhrer M, Nouta J, Koeleman CAM, Clarke J, Marinou S, Shakya M, Colin-Jones R, Theiss-Nyland K, Voysey M, Jin C, Pant D, Jones E, Kelly S, Dongol S, Karkey A, Shrestha S, Basnyat B, Hill J and Pollard AJ (2021) Association of Antibody-Dependent Neutrophil Phagocytosis With Distinct Antibody Glycosylation Profiles Following Typhoid Vaccination. Front. Trop. Dis 2:742804. doi: 10.3389/fitd.2021.742804
Received: 16 July 2021; Accepted: 31 August 2021;
Published: 16 September 2021.
Edited by:
Sushant Sahastrabuddhe, International Vaccine Institute, South KoreaReviewed by:
Malcolm Scott Duthie, HDT Biotech Corporation, United StatesCopyright © 2021 Johnson, Stockdale, de Haan, Wuhrer, Nouta, Koeleman, Clarke, Marinou, Shakya, Colin-Jones, Theiss-Nyland, Voysey, Jin, Pant, Jones, Kelly, Dongol, Karkey, Shrestha, Basnyat, Hill and Pollard. This is an open-access article distributed under the terms of the Creative Commons Attribution License (CC BY). The use, distribution or reproduction in other forums is permitted, provided the original author(s) and the copyright owner(s) are credited and that the original publication in this journal is cited, in accordance with accepted academic practice. No use, distribution or reproduction is permitted which does not comply with these terms.
*Correspondence: Mari Johnson, bWFyaS5qb2huc29uQGxtaC5veC5hYy51aw==
†ORCID: Lisa Stockdale, orcid.org/0000-0002-2576-8783
‡These authors share senior authorship
Disclaimer: All claims expressed in this article are solely those of the authors and do not necessarily represent those of their affiliated organizations, or those of the publisher, the editors and the reviewers. Any product that may be evaluated in this article or claim that may be made by its manufacturer is not guaranteed or endorsed by the publisher.
Research integrity at Frontiers
Learn more about the work of our research integrity team to safeguard the quality of each article we publish.