- 1Department of Medicine, University of California, San Francisco, San Francisco, CA, United States
- 2Department of Medicine, San Francisco Veterans Affairs Medical Center, San Francisco, CA, United States
Up to 90% of the global population has been infected with cytomegalovirus (CMV), a herpesvirus that remains latent for the lifetime of the host and drives immune dysregulation. CMV is a critical risk factor for poor outcomes after solid organ transplant, though lung transplant recipients (LTR) carry the highest risk of CMV infection, and CMV-associated comorbidities compared to recipients of other solid organ transplants. Despite potent antivirals, CMV remains a significant driver of chronic lung allograft dysfunction (CLAD), re-transplantation, and death. Moreover, the extended utilization of CMV antiviral prophylaxis is not without adverse effects, often necessitating treatment discontinuation. Thus, there is a critical need to understand the immune response to CMV after lung transplantation. This review identifies key elements of each arm of the CMV immune response and highlights implications for lung allograft tolerance and injury. Specific attention is paid to cellular subsets of adaptive and innate immune cells that are important in the lung during CMV infection and reactivation. The concept of heterologous immune responses is reviewed in depth, including how they form and how they may drive tissue- and allograft-specific immunity. Other important objectives of this review are to detail the emerging role of NK cells in CMV-related outcomes, in addition to discussing perturbations in CMV immune function stemming from pre-existing lung disease. Finally, this review identifies potential mechanisms whereby CMV-directed treatments may alter the cellular immune response within the allograft.
Introduction
Cytomegaloviruses (CMVs) are double-stranded DNA viruses that have co-evolved over millions of years with their mammalian hosts (1). CMV is ubiquitous and belongs to the beta subfamily of Herpesviridae (2). It possesses two sets of genetic material, a lipid envelope that encases its icosahedral nucleocapsid, and a proteinaceous tegument that is rich in viral phosphoproteins, including pp65 (3). During acute infection, human CMV (HCMV) interferes with antigen loading through major histocompatibility complex (MHC) downregulation, inhibits T cell receptor co-stimulation, produces viral peptides that imitate inhibitory cytokines which disrupt lymphocyte migration, and downregulates Natural Killer (NK) cell activating ligands while upregulating inhibitors of NK cell function (4). Following acute infection, CMV enters a dormant, or latent, state. While the complete viral genome remains within the host cell during latency, the virus's expression is severely restricted, resulting in very few viral antigens and no viral particles (5). CMV establishes latency in many cell types, though has notable tropisms for myeloid (CD34+) bone marrow progenitor cells, endothelial cells, circulating CD14+ monocytes, and lung and mucosal epithelial cells (6). After solid organ transplantation, reactivation of CMV is provoked by immunosuppression, other infections, or organ injury (7, 8). Reactivation of latent CMV can cause multiorgan disease, and has been associated with reduced graft survival, graft-vs.-host disease, post-transplant lymphoproliferative disorders, and increased mortality (9).
CMV is a leading cause of morbidity after lung transplant (LT) (10, 11), where up to 70% of lung transplant recipients experience CMV reactivation or primary infection within the first post-transplant year (12–16). CMV can lead to pneumonia, pneumonitis, and direct extra-pulmonary organ involvement (17). In addition, CMV has been associated with risk for several chronic lung transplant clinical syndromes including antibody-mediated rejection (AMR) and chronic lung allograft dysfunction (CLAD), the syndrome of chronic rejection (18–21). Lung transplant CMV complications increase care costs by 50% when compared to recipients who do not develop CMV disease after lung transplantation (22). Moreover, the extended utilization of CMV antiviral prophylaxis is not without drawbacks, often necessitating treatment discontinuation due to intolerance, drug interactions, leukopenia, or broader bone marrow suppression. There is emerging evidence that these poor CMV-related outcomes in lung transplant recipients are driven by a dysregulated immune response (23).
CMV exerts tissue-specific effects which impact tissue-level immune responses
CMV infection and its effects on allograft function are confounded by differences in cellular receptors and viral gene expression across tissues (8). The diversity of CMV strains and their adaptation to different tissue types is a complex phenomenon that likely involves a combination of factors, including genetic variation among CMV strains and viral evolution within the host (24, 25). Notably, different strains of the virus may have specific tropisms for different tissues. For example, certain strains of CMV may preferentially infect the lungs, while others may be more adept at infecting the liver or brain. This strain-specific tropism can contribute to the observed differences in CMV infection across different tissue types (26). In a study investigating differences in CMV genome by tissue, there were eighty viral genes with differential tissue expression levels (27). Because of its tissue specificity, CMV may activate different transcriptional processes in different cell types. Post-transcriptional events, such as mRNA stability, may also differ by target tissue or be influenced by local factors such as cytokines or microbes (28). Additional factors that influence the impact of viral infection include variations in immunosuppressive regimens and genetic vulnerability. It has also been shown that CMV microRNAs (miRNAs), short noncoding RNA species, differ by tissue type and may allow viral gene expression to be tissue specific (28–30). Certain miRNAs were also identified that reduce the cellular stress response, decrease cytokine release, and that downregulate host pattern recognition receptors (31–33). Finally, different tissues may exhibit variations in immune surveillance and response, which can influence the course of CMV infection and the selection pressures acting on the virus within each tissue microenvironment.
CMV is associated with a high burden of disease in lung transplant recipients
The incidence of CMV disease after lung transplantation ranges from 30% to 86%, with a CMV-attributable death rate of 2%–12% (34). This increased burden in LTRs may be a consequence of the lung as a reservoir for CMV latency (35) where higher viral loads are retained in the extensive pulmonary lymphatic system as compared to other solid organs transplants (33). It follows that CMV severity varies based on donor and recipient CMV serostatus (36). Donor seropositive and recipient seronegative (D+/R−) solid organ transplant recipients have the highest risk of CMV disease (37), while D−/R− recipients have the lowest risk for CMV disease (38). The other donor-recipient CMV pairings (D+/R+ and D−/R+) confer intermediate risk. Another driver of lung transplant CMV stems from the higher doses of induction and maintenance immunosuppression compared to other solid organ transplants (37). Antilymphocyte induction therapy agents, such as alemtuzumab or anti-thymocyte globulin have been associated with a nearly 6-fold increased incidence of early CMV reactivation after transplantation (39–41). Comparatively, non-depleting induction regimens, such as those that use basiliximab, may confer a reduced risk for CMV reactivation (42). Other factors prevalent in the lung transplant population can amplify the impact of CMV and lower the threshold for CMV reactivation. These include telomere biology disease, inherent disorders of immune function, and acute rejection or acute injury syndromes (43, 44).
Impact of immunosuppressive drugs on CMV
Immunosuppressive agents, vital for preventing organ rejection in transplant recipients, impact the risk and management of CMV infection. For example, Calcineurin Inhibitors (Cyclosporine and Tacrolimus) are cornerstone drugs in the transplant immunosuppression regimen and have been shown to predispose patients to viral infections, including CMV, by broadly suppressing the T cell immune response (45). Mycophenolate Mofetil (MMF) and Azathioprine inhibit the proliferation of T and B cells, reducing the immune response to both the transplanted organ and infectious agents like CMV. Notably, the degree of immunosuppression correlates with the risk of CMV infection (46). Mammalian Target of Rapamycin (mTOR) Inhibitors (Sirolimus and Everolimus) have impact on CMV infection (47). Alemtuzumab is a potent immunosuppressive agent but significantly increases the risk of infections, including CMV, by depleting lymphocytes, a critical component of the antiviral immune response (48).
Mean residual expression (MRE) could be a valuable tool in identifying lung allograft recipients who are at an increased risk of infection, by identifying the functional effects across immunosuppressive agents on the recipient immune response (49). This could potentially lead to more personalized immunosuppression regimens that balance the risk of rejection with the risk of infection, including CMV reactivation (48).
The early anti-CMV immune response is marked by monocyte and macrophage activity and immune evasion
CMV, along with other human herpesviruses, primarily infect fibroblasts, endothelial cells, epithelial cells, monocytes, and macrophages. It gains entry through the cell membrane via glycoprotein B (gB), which is a viral enveloped fusion glycoprotein; though, whether entry is mediated through gB-receptor dependent or independent processes is unknown (50). Potentially through caspase 2 activation, there is a subsequent release of inflammatory cytokines from these infected cells including TNF-α, IL-6, in addition to a robust type I interferon (IFN) response (51).
The heterogeneous macrophage (MΦ) population performs infection surveillance and maintains tissue homeostasis. MΦ function, and even reprogramming, occur in response to circulating mediators (52). Macrophages have been well described as hewing to two polarized differentiation pathways, M1 and M2, which have been named after CD4+ T helper (Th) cell subtypes (53). M1 (proinflammatory) MΦ's secretes IL-1β, IL-6, IL-12, and TNF-α, unlike M2 MΦ (anti-inflammatory) (54), which primarily secrete IL-10 and have other phenotypic and functional differences (55). The lungs have functionally distinct subsets of MΦ, notably, alveolar MΦ and interstitial MΦ that have been shown to be immunosuppressive with a poor ability to present antigens (56). Nonetheless, CMV infection triggers the production of pattern recognition receptor ligands. These ligands bind to TLR4 and TLR5 on macrophages and lead to intracellular MyD88 signalling and gene transcription of the proinflammatory cytokines TNF-α, IL-6, and IL-8 (57).
While the early inflammatory cascade of CMV infection is mediated by macrophages, HCMV has been shown to evade immune recognition by dampening the macrophage-specific response. MΦ's infected with HCMV downregulate MHC class I and class II molecules as well as chemokine receptors (56, 58). Concordantly, HCMV-infected monocytes differentiate away from an M1 phenotype and toward a mixed M1-M2 MΦ phenotype, which ensures successful viral survival and persistence (58). Persistent macrophage infection can overwhelm the conventional clearing mechanisms of the immune system (59). Further immune evasion occurs through reduction in the production of ligands to activating receptors on effector immune cells (60). The CMV protein UL141 directly binds to some of these ligands in macrophages and in other cell types (61). It has also been observed that noninfected bystander MΦ's are also impacted during CMV infection. This implies that tissue signals and soluble mediators may indirectly play a role in dampening the CMV-specific immune response (62).
In lung transplantation, CMV infection may drive a dysregulated macrophage response which contrasts to conventional macrophage responses to other viral infections (63, 64). This largely stems from the co-evolution of macrophages with CMV, as they are a primary host cell for CMV infection (65). Activation of TLR4, an important receptor in the early CMV immune response, has been closely linked to acute lung allograft rejection (66, 67). Bronchoalveolar lavage samples from lung transplant recipients with CMV pneumonia demonstrated increased gene expression of IL-1, IL-6 and serine esterase B, with concordant findings during acute rejection (68). Significant increases in the concentrations of cytokines, such as CXCL-10, CXCL-16, CCL-18, and CCL-20, in bronchoalveolar lavage have also been observed during HCMV replication in lung transplant recipients. The effector CD8+ and CD4+ T lymphocytes are selectively attracted by CXCL-10 and CXCL-16 (69). Antigen-presenting cells generate CCL-18, which acts as a monocyte activator and chemoattractant for B-cells, immature dendritic cells (DCs), and naïve and regulatory T cells. Pulmonary epithelial cells and monocytes secrete CCL-20, which binds to CCR6, a specific receptor on T cells and immature dendritic cells (DCs). CCR6 is prominently expressed on Th17 CD4 cells, making them particularly susceptible to the chemotactic effects of CCL-20. In addition, Th17 CD4 cells have been implicated in acute rejection (70, 71). IL-17 promotes neutrophil chemotaxis via the induction of IL-8. This leads to an increased presence of neutrophils and lymphocytes within the lung tissue, contributing to an inflammatory response characteristic of acute rejection (70). Thus, CMV-associated activation of macrophages may drive maladaptive T cell responses in the lung allograft.
Lymphocytes in CMV, in the lung and in transplantation
NK cells play an important role in mitigating CMV
Natural killer cells are lymphocytes and constituents of the innate immune system. Their primary role is to identify missing, transformed, or damaged self (72, 73). As opposed to T and B cells, where specificity for antigens occurs through genetic rearrangement, NK cell activity is dependent upon the integration of activating and inhibiting signals from somatically encoded receptors (74). There has been an increasing awareness that NK cells play a central role in many lung transplant conditions (75), and nowhere is their function in the lung more evident than during CMV infection.
NK cells are key mediators of CMV infection, where they eliminate CMV-infected cells through antibody-dependent cell-mediated cytotoxicity and through the modulation of T and B cells via the secretion of IFNγ (76). NK cells recognize CMV via the C-type lectin-like receptor CD94/NKG2C in humans and the Ly49H receptor in mice (77, 78). In humans, T cells also express the NKG2C receptor. Immunoreceptor tyrosine-based activation motifs (ITAMs) are located on the DAP12 signalling adaptor, which covalently binds NKG2C with the CD94 glycoprotein. This complex functions as a ligand-recognition mechanism for the invariant HLA-E protein (79). It is still unclear which CMV-related antigen is presented on HLA-E. While it is possible that HLA-E directly presents a dominant CMV peptide epitope, none has been identified to date (80). It is more likely that NKG2C+ NK cells may recognize changes in HLA-E stability and HLA-E-associated molecules that are presented during CMV infection (81). In parallel in the mouse, the NKR Ly49H receptor enables direct identification and killing of mouse CMV (mCMV) infected cells via recognition of the m157 protein (82–84).
In humans and mice, NKG2C or Ly49H activation leads to potent NK cell effector functions and results in memory-like capabilities. In a mouse model of CMV infection, mortality was improved in animals that received NK cells from mice previously infected with CMV as compared to mice with NK cells transferred from unexposed mice or those without NK cell transfers (85). In both human and mouse, these CMV-specific NK cells undergo avidity selection for NK cell clones that have the highest virus-specific receptor, expand, and persist as long-lived memory cells (83, 86). Recently, it has been shown that these long-lived CMV-specific NK cells localize to and persist in the lung and salivary gland tissue (87).
The NK cell response to CMV in lung transplantation
After transplantation, HCMV induces potent NKG2C+ NK cells (88–90). In kidney transplant recipients, CMV seropositive recipients demonstrated increased frequencies of baseline memory-like NK cells that expressed CD57 (88) and lacked FcεRIγ. Longitudinally, this population was dynamic and decreased in the circulation after transplant and after CMV reactivation. In this same group, a pre-memory NK cell population defined by CD57 and FcεRIγlow expression increased during CMV reactivation. This population had increased proliferative and cytotoxic capabilities. These findings demonstrate the interplay between transplantation and CMV in influencing CMV-specific cell populations and demonstrates that even in the presence of immunosuppression, transplant recipients can generate new innate effector memory (91).
There is evidence that NKG2C+ NK cells are important in constraining CMV infection in lung transplantation. A large percentage of the human population (∼70%) lacks the NKG2C encoding gene, KLRC2 (92). In a study of 98 lung transplant recipients, participants with intact KLRC2 alleles were less likely to experience CMV DNAemia and CMV-associated disease compared to participants harbouring the null allele (93). In a study of 130 lung transplant recipients, bronchoalveolar lavage NKG2C+ NK cells were increased in CMV seropositive individuals at baseline and expanded preceding CMV DNAemia (94). These studies suggests that NKG2C+ NK cells play a potentially crucial role in the CMV immune response following lung transplant.
The CD8 T cell response to CMV
In vivo data suggest CMV-specific CD8+ T lymphocytes are the primary population responsible for limiting viral cell replication (95). In genetically resistant mouse inbred strains (96), NK cells mediate early protection, whereas CD8 T cells acquire long-lasting protective memory and serve as primary antiviral effectors in susceptible strains (96, 97). Notably, studies using CMV tetramers, demonstrate that CMV-specific T cells are most abundant in blood (98) and lungs. Infection with CMV also results in the production of distinct CD8+ T-cell phenotypic population (99). Most CMV-specific CD8 T cells are effector memory cells (TEM), noted by the absence of CD62L and CCR7, 2 lymph node homing markers (100). CMV-specific CD8 TEM notably downregulate co-receptors (CD27 and CD28) and have markers of maturation (CD57, KLRG1) and increased effector functions (101) Memory CD8+ T cell responses to CMV have received a great deal of attention, owing to their atypical expansion, known as memory inflation (102), which occurs to a lesser extent in CMV-specific CD4+ T cell populations (103). Although these populations are thought to be associated with lifelong CMV infection, the mechanisms underlying these expansions and how they are maintained remain unknown.
In human lung transplant, CMV-specific CD8 T cells vary less in the blood than in the lungs where they have been reported to be increased preceding CMV replication (104). During CMV infection, studies have reported a profound influx of CD8 T cells into the lung allograft (105). These lung allograft CD8 T cells express an effector memory phenotype, demonstrate CMV-specificity, and undergo a contraction followed by long-term persistence after CMV infection. In a study of 41 lung transplant recipients, CMV DNAemia was associated with the expansion of 3 distinct populations of terminally differentiated effector memory (TEMRA). CD8 T cells in the circulation marked by differences in CD57 and KLRG1 expression. CD57 + KLRG1+ CD8 TEMRA cells were associated with CMV clearance and reduced risk for chronic lung allograft dysfunction or death (106, 107). Together, these data suggest that a robust CD8 T cell memory response can contribute to improved CMV control and better long-term outcomes after lung transplantation.
The γδ T cell response to CMV
Among lung transplant recipients with high-risk CMV mismatches (D+/R−), effector γδ T cells increased longitudinally after transplant, exhibit increased frequencies of the NK cell NKG2C receptor, and demonstrate increased T cell receptor diversity (108).
T cells with the γδ T cell receptor contribute to anti-cancer and anti-infection immune responses (109, 110). γδ T cells are different from αβ T cells in several ways, most notably in antigen detection and effector destiny development, even though they perform similar vital tasks (111). In contrast to αβ T cells, major histocompatibility complex (MHC) does not limit the activity of γδ T cells (112). Also, the variety of ligands recognized by the γδ TCR is remarkable and includes MHC-related proteins in addition to low molecular weight non-peptide ligands (113). Following CMV reactivation in transplant patients, γδ T cells increase and persist in the circulation (114). The observation that γδ T cells are protective from CMV-associated mortality in mice deficient in αβ T cells suggests that γδ T cells play a protective role in mitigating CMV disease (115). In lung transplant, it has been observed that recipients with high-risk CMV mismatches (D+/R−) have a robust effector γδ T cell response after transplant, increased expression of the NK cell NKG2C receptor, and increased T cell receptor diversity (108).
Other lymphocyte response to CMV
In the immunological response against CMV during lung transplantation, CD4+ T cells and B cells are essential components. Adoptive transfer of CMV-specific CD4+ T cells into sub-lethally irradiated mouse hosts show a protective effect. These cells are more effective when administered in conjunction with CD8+ T cells specific to the virus (116). A delayed emergence of CMV-specific CD4+ T cells is linked to longer viremia and more severe clinical illness in immunosuppressed solid organ recipients (117). In trials of cytotoxic T cell therapies in humans, CMV-specific CD4+ T cells are necessary for the persistence of CMV-specific CD8+ T cells (118). Further, CD4+ T cells may facilitate the entry of naïve CD8+ T cells and B cells to draining lymph nodes and serve to entice innate or antigen-specific effectors to viral replication sites through the secretion of local chemokines and the synthesis of IFN-γ. Antigen binding on CD4+ T cells triggers CD40L expression, which activates CD40 on B cells and promotes B cell proliferation and differentiation, initially in extra-follicular foci and then in lymph node germinal centers, producing memory B cells and plasma cells that generate anti-CMV antibodies (119). CD4+ T cells promote memory CD8+ T cell development through a variety of mechanisms, including downregulating the expression of TNF-related apoptosis-inducing ligand (TRAIL), producing cytokines like IL-2, or directly ligating CD40 on naïve CD8+ T cells (120, 121). The critical role that CD4+ T cells play in antiviral immunity is highlighted by the existence of viral genes that down-regulate MHC class II molecules and the expression of viral IL-10, both of which restrict antigen presentation to CD4+ T cells (104, 118).
In the spleen, B cells initiate the early interferon response to CMV in a lymphotoxin receptor-dependent manner (121). Moreover, they play a role in mitigating CMV infection via the production of anti-CMV antibodies. Though, the observation that B cells are decreased in lung transplant recipients with CMV and CLAD highlights the uncertainty in their role in the lung (122, 123). Additional investigation is needed to fully understand how B cells, specifically within the allograft, may contribute to CMV pathology.
The role of heterologous immune responses in promoting tissue- and allograft-specific immunity
Heterologous immunity as a phenomenon was recognized early in the infection literature but has profound implications for autoimmunity and allorecognition. The concept of heterologous immunity centres on the observation that previously encountered antigens can alter immunity to new or novel antigens (124). This heterologous immune response can be helpful or harmful to the host and depends largely on cellular and tissue contexts. Thus, the chronic immunological responses required to control CMV infection may cause graft harm by direct antibody-dependent cell-mediated cytotoxicity, the formation of heterologous alloimmune responses, and proinflammatory cytokine production (125).
CD8 T cells and heterologous immunity
The observation that there are more recognizable antigens than unique T cell receptors (TCRs) supports evidence of T-cell cross-reactivity (126). The mechanism whereby this is achieved remains to be fully elucidated but may result from the ability of the same TCR to recognize 2 different peptide-MHC complexes. Consequently, infection with CMV, in addition to a variety of other viruses, has been observed in C57BL/6 mice to promote CD8 T cell specificity to MHC I antigens in addition to viral antigens (127). In an allogeneic model of rat lung transplantation, infection with parainfluenza 1 virus broke tolerance and induced obliterative airway disease (128). Such findings suggest a heterologous component to lung transplant rejection following viral infection.
NK cells and trained immunity
While circulating and allograft NKG2C+ NK cells in lung transplant recipients expand with CMV reactivation, there is evidence that this cell population may have a dual role in allograft outcomes (9, 129). Among 130 lung transplant recipients, higher than the median NKG2C+ NK cells in the BAL conferred more than a 4-fold increased risk for CLAD or death (94). This suggests that NK cells may be recognizing low-level chronic CMV infection in the lung and potentiating chronic injury to the airways (18). In this same study, NKG2C+ NK cells were shown to have increased CD16, an activating-only Fc receptor. Indeed, NKG2C+ NK cells retain potent effector functions including the ability to participate in antibody-dependent cell-mediated cytotoxicity (ADCC) (130). In a longitudinal study of bronchoalveolar lavage NK cells after lung transplantation, CD16+ NK cells were associated with acute lung allograft dysfunction and antibody mediated rejection (AMR) (131). Further, increased CD16+ NK cells among recipients with AMR conferred an increased risk for chronic lung allograft dysfunction.
There is emerging evidence that CMV may influence the ability of NK cells to participate in antibody mediated rejection after lung transplantation. UL40 peptides, produced by CMV, have been shown to alter expression of HLA-E which binds NK cell receptors. Through a variety of potential mechanisms, UL40 may alter the NK cell response to CMV-infected cells, and polymorphic UL40 peptides induce variable NKG2C+ NK cell effector functions (132). Indeed, in a study of 150 lung transplant recipients, certain UL40 peptide variants were associated with AMR and induced proliferation of CD16 + NKG2C+ NK cells (92). Interestingly, in a cohort of 82 lung transplant recipients with at least 1 episode of CMV reactivation, one UL40 peptide variant was associated with CLAD and impeded immature NK cell proliferation. These data reveal a direct interaction between CMV peptides and NK cell functional phenotypes in mediating AMR and CLAD. While not meeting the true definition of heterologous immunity, these data illustrate how CMV infection may lead to off-target effects in lung allografts.
There is an interplay between CMV and allograft function
Lung allograft rejection is typically classified into acute cellular rejection and antibody-mediated rejection. Acute cellular rejection is characterized by perivascular and interstitial mononuclear cell infiltration, predominantly by lymphocytes. In contrast, antibody-mediated rejection involves capillary inflammation with neutrophils and evidence of antibody deposition, often detected through immunofluorescence. The diagnosis of rejection is based on histological examination of lung biopsy specimens. As discussed, CMV infection may cause direct and indirect allograft injury through a range of mechanisms (8). Consequently, acute allograft rejection also has implications for CMV pathogenesis directly. The production of tumour necrosis factor-alpha (TNF-α) in lung transplant recipients as been shown to promote CMV replication and is a critical driver for CMV reactivation from latency. Remarkably, the CMV viral protein pUL138 also amplifies the reaction to TNF-α by upregulating the receptor's cell surface expression (24).
Consequently, the pathogenesis of vascular injury observed in acute and chronic rejection is likely influenced by CMV infection of smooth muscle cells and vascular endothelium (133). The hallmark of CMV infection in lung transplant shows characteristic cytopathic effects, such as enlarged cells with intranuclear inclusions (“owl's eye” appearance) and intracytoplasmic inclusions. Real-time polymerase chain reaction (PCR) for CMV DNA quantification in blood or tissue samples is another crucial diagnostic tool, offering both high sensitivity and specificity for detecting viral replication. Though, our understanding of the pathology of heterologous CMV immunity specifically during lung transplant rejection is limited. In the future, tissue transcriptional analyses may yield insights into the CMV-specific T cell receptor repertoire or overall CMV immune response.
CMV causes an increase in the number of inflammatory cells in the graft by upregulating endothelial adhesion molecules like VCAM, ICAM, LFA-1, and VLA-4. A further mechanism of injury is molecular mimicry through induction of a glycoprotein homologous to MHC class I antigens. There is evidence of sequence homology and immunologic cross-reactivity between CMV immediate early antigens and the HLA-DR β chain (40). According to the “missing self-hypothesis,” loss of MHC class I may activate natural killer cell recognition and killing. As a result, HCMV promotes the expression of an HLA-E inhibitory receptor as well as several gene products that upregulate natural killer inhibitory receptors and disable natural killer activating receptors (59, 103).
A notable finding following CMV pulmonary infection is the development of numerous CMV plaques (134). These plaques have been shown to draw in inflammatory cells to the lung parenchyma. In a mouse model of bone marrow transplantation, CD3ε+ T cells were seen three weeks after viral infection co-localized to CMV-infected cells in the lung (135). The tertiary lymphatics have been shown to be central to tolerance in mouse allogeneic lung transplant (136). Such a CMV-related phenomenon could prime the tissue resident immune populations away from tolerance. Thus, the interplay between CMV and the lung allograft can significantly impact long-term outcomes.
Additionally, there is evidence that perturbations from underlying lung disease may alter the immune response to CMV. Mechanistically, short telomeres trigger ATM-dependent DNA damage repair pathways, which activate p53 signalling. In lung transplant recipients, this has shown to result in the cessation of proliferation and the release of pro-inflammatory cytokines (137). CMV exposure in lung transplant recipients with short telomeres had skewed distributions of conventional CD4 T cells and CD8 TEMRAs. Short recipient telomere lengths following transplantation have been sporadically associated with leukopenia, risk of cytomegalovirus (CMV) infection, reduced acute cellular rejection, and worsened chronic lung allograft dysfunction (CLAD)-free survival (138). A study of CD8 T cells in lung transplant recipients with short telomeres and idiopathic pulmonary fibrosis found several CD8 T cell impairments including reduced proliferation and effector functions (23). These data show how the CMV response after transplant may be influenced by systemic drivers of lung disease.
The impact of CMV-specific therapies on cellular immune populations
There are a host of available agents to manage CMV disease in the lung transplant population; though, CMV eradication is not possible currently. Thus, common CMV management strategies often focus on prophylaxis agents in high-risk lung transplant recipients to reduce the potential for reactivation (139). In addition, there are several strategies for the treatment of CMV disease or CMV DNAemia. Currently, most monitoring protocols involve BAL and plasma surveillance of CMV replication by PCR (140). Some centers have instituted CMV immune assays like quantiferon-CMV or commercial CMV ELISPOT assays designed to gauge cellular immunity (141).
The mainstays of CMV prophylaxis and treatment are antiviral agents, which include ganciclovir, valganciclovir, and the newer agents marabavir and letermovir (142, 143). Foscarnet has been used in the past but has fallen out of favor given its poor safety characteristics (143, 144). Valgancyclovir is the oral prodrug of ganciclovir which works through inhibition of viral DNA synthesis. Valganciclovir has been shown to influence NK cell frequencies and function. In a study of human herpesvirus-8, valganciclovir-treated participants had reduced NK cells in circulation and more pronounced KLRG1 expression (145). In a randomized study of valganciclovir compared to placebo in HIV+ patients, the valganciclovir group had reduced CD8 T cell activation relative to the placebo group (146). Among kidney transplant participants, lymphocyte proliferation and activated T cell counts were reduced with valganciclovir treatment (146, 147). Despite these changes, there is evidence that valganciclovir may preserve the CMV-specific T cell responses after lung transplantation (148).
In addition to antiviral agents, CMV hyperimmunoglobulin (CMVIg) has been used for several decades for prophylaxis against CMV reactivation in high-risk donor-recipient pairings (149). Proposed mechanisms for CMVIg efficacy include reduction of cytokine production, as observed in mixed lymphocyte reactions and anti-CD3 blastogenesis experiments, the reduction of ADCC, induction of CD8 T cell and NK cell apoptosis, and reduction in T-cell proliferation (150). Given these changes, one outstanding question is whether CMVIg may modulate graft outcomes. There is some indication that CMVIg may reduce acute rejection rates following heart or lung transplantation (151); though, the evidence is not conclusive and has not been verified for other solid organ transplant groups (152).
In summary, anti-CMV strategies influence immune populations known to be important in the CMV inflammatory response though, more study is needed to understand whether these occur independent from reduced CMV activity or directly influence allograft outcomes. Recently, it has been theorized that CMV-specific effector cells may be harnessed to treat refractory disease (115). It was demonstrated that expansion and infusion of autologous CMV-specific cytotoxic lymphocytes in a lung transplant recipient with ganciclovir-resistant CMV disease was safe (115). In a prospective trial of a similar strategy in solid organ transplant recipients, in vitro expanded autologous CMV-specific T cells were associated with an 84% response rate including blunting of CMV DNAemia (153). Several recipients showed long-term persistence of anti-CMV cellular immunity following this novel treatment. More work is needed to establish the safety and efficacy of this intriguing approach. Finally, the field may see the arrival of an effective CMV vaccine; though, efficacy in transplant populations and the long-term cellular implications for graft function will be important to understand (154).
Summary
Figure 1 depicts the impacts of CMV and CMV-specific effector immune cells on the lung allograft. Solid organ transplant recipients face a substantial clinical burden from CMV infection. Though, the rate of significant CMV disease is greater in lung transplant recipients than in recipients of all other solid organs owing to a variety of factors including high viral burden and need for more potent immunosuppression. Despite effective therapies, CMV remains a strong risk factor for CLAD following lung transplantation. While CMV can cause direct lung allograft damage, there is emerging evidence that poor outcomes are driven by effector immune system dysregulation. NK cells, CD8 T cells, and γδ T cells play important roles in mitigating CMV infection. However, after lung transplantation, heterologous immunity and off-target effects in these populations may predominate. Finally, several agents are used to prevent and treat CMV, and each may modulate the CMV immune response.
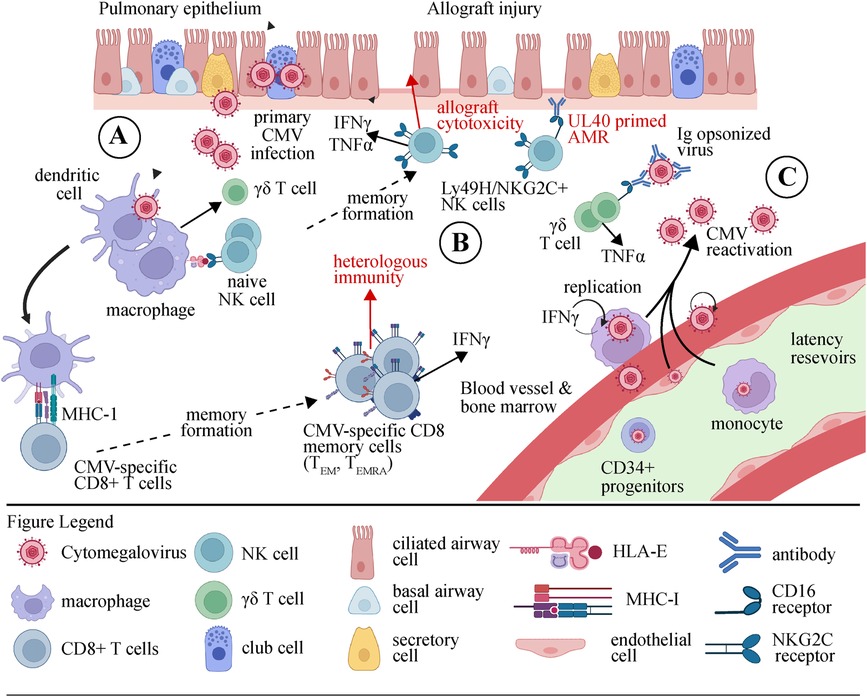
Figure 1. Illustration depicting the cellular response to CMV in the lung allograft. (A) During the initial stages of primary CMV infection, phagocytes, and dendritic cells (DCs) are stimulated by viral products through Toll-like receptors (TLRs) and nucleic acid sensors. As a result, they release pro-inflammatory cytokines (IFNαβ, IL-12, and IL-18) and present antigens that trigger the activation of natural killer (NK) cells and γδ T cells. (B) The activation of dendritic cells (DCs) results in their maturation and subsequent migration to lymph nodes. The process of presenting viral peptides to inactive CD8+ αβ T cells leads to their differentiation into either effector memory (TEM) or terminally differentiated effector memory (TEMRA) cells, as well as their expansion and acquisition of effector capabilities. Activated natural killer (NK) cells and αβ and γδ T cells can destroy and remove cells infected with cytomegalovirus (CMV) or regulate the replication of the virus by releasing anti-viral cytokines such as IFNγ and TNFα. Allograft injury may occur through this process. (C) Although an immune response is mounted, CMV continues to survive within its host. During viral reactivation, immune cells activated by CMV promptly respond to the presence of virions by recognizing m157/HLA-E, stress molecules, or viral peptides. Furthermore, the production of IFNγ by γδ T cells and NK cells stimulated by CMV can be triggered by the interaction between CD16 and Ig-opsonized viruses. CMV, cytomegalovirus; IFN, interferon; Ig, immunoglobulin; NK, natural killer cell; TEM, effector memory T cell; TEMRA, CD45RA+ effector memory T cell.
Author contributions
RB: Writing – original draft, Writing – review & editing. DC: Conceptualization, Funding acquisition, Resources, Writing – original draft, Writing – review & editing.
Funding
The authors declare financial support was received for the research, authorship, and/or publication of this article.
DC received funding for this project from CFF (CALABR19Q0) & VA ORD BLRD (BX005301). Figure was created in part with tools on BioRender.com with rights for publication licensed under agreement number FM26UX3MEV.
Conflict of interest
The authors declare that the research was conducted in the absence of any commercial or financial relationships that could be construed as a potential conflict of interest.
Publisher's note
All claims expressed in this article are solely those of the authors and do not necessarily represent those of their affiliated organizations, or those of the publisher, the editors and the reviewers. Any product that may be evaluated in this article, or claim that may be made by its manufacturer, is not guaranteed or endorsed by the publisher.
References
1. Klenerman P, Oxenius A. T cell responses to cytomegalovirus. Nat Rev Immunol. (2016) 16(6):367–77. doi: 10.1038/nri.2016.38
2. Hakki M, Ljungman P. Cytomegalovirus infection after stem cell transplantation. Transpl Infect: Fourth Edition. (2016):417–40. doi: 10.1007/978-3-319-28797-3_24
3. Lurain NS, Chou S. Antiviral drug resistance of human cytomegalovirus. Clin Microbiol Rev. (2010) 23(4):689–712. doi: 10.1128/CMR.00009-10
4. Griffiths P, Baraniak I, Reeves M. The pathogenesis of human cytomegalovirus. J Pathol. (2015) 235(2):288–97. doi: 10.1002/path.4437
5. Speck SH, Ganem D. Viral latency and its regulation: lessons from the γ-herpesviruses. Cell Host Microbe. (2010) 8(1):100–15. doi: 10.1016/j.chom.2010.06.014
6. Mullane KM. Human cytomegalovirus prophylaxis and treatment in lung transplantation in the current era. Curr Pulmonol Rep. (2020) 9:10–27. doi: 10.1007/s13665-020-00246-y
7. Griffiths P, Lumley S. Cytomegalovirus. Curr Opin Infect Dis. (2014) 27(6):554–9. doi: 10.1097/QCO.0000000000000107
8. Kaminski H, Fishman JA. The cell biology of cytomegalovirus: implications for transplantation. Am J Transplant. (2016) 16(8):2254–69. doi: 10.1111/ajt.13791
9. Leeaphorn N, Garg N, Thamcharoen N, Khankin EV, Cardarelli F, Pavlakis M. Cytomegalovirus mismatch still negatively affects patient and graft survival in the era of routine prophylactic and preemptive therapy: a paired kidney analysis. Am J Transplant. (2019) 19(2):573–84. doi: 10.1111/ajt.15183
10. Cannon MJ, Schmid DS, Hyde TB. Review of cytomegalovirus seroprevalence and demographic characteristics associated with infection. Rev Med Virol. (2010) 20(4):202–13. doi: 10.1002/rmv.655
11. Burguete SR, Maselli DJ, Fernandez JF, Levine SM. Lung transplant infection. Respirology. (2013) 18(1):22–38. doi: 10.1111/j.1440-1843.2012.02196.x
12. Azevedo LS, Pierrotti LC, Abdala E, Costa SF, Strabelli TMV, Campos SV, et al. Cytomegalovirus infection in transplant recipients. Clinics. (2015) 70:515–23. doi: 10.6061/clinics/2015(07)09
13. Rosenheck JP, Botros MM, Nunley DN. Cytomegalovirus in lung transplant. OBM Transplant. (2021) 5(2):1–18. doi: 10.21926/obm.transplant.2102145
14. Streblow DN, Dumortier J, Moses AV, Orloff SL, Nelson JA. Mechanisms of cytomegalovirus-accelerated vascular disease: induction of paracrine factors that promote angiogenesis and wound healing. Hum Cytomegalovirus. (2008):397–415. doi: 10.1007/978-3-540-77349-8_22
15. Cook CH. Cytomegalovirus reactivation in” immunocompetent” patients: a call for scientific prophylaxis. J Infect Dis. (2007) 196:1273–5. doi: 10.1086/522433
16. Bennett D, Bergantini L, Ferrara P, Cusi MG, Scolletta S, Montagnani F, et al. Cytomegalovirus infection is associated with development of chronic lung allograft dysfunction. Lung. (2022) 200(4):513–22. doi: 10.1007/s00408-022-00551-0
17. Restrepo-Gualteros SM, Jaramillo-Barberi LE, Gonzalez-Santos M, Rodriguez-Martinez CE, Perez GF, Gutierrez MJ, et al. Characterization of cytomegalovirus lung infection in non-HIV infected children. Viruses. (2014) 6(5):2038–51. doi: 10.3390/v6052038
18. Vietzen H, Jaksch P, Puchhammer-Stöckl E. The human cytomegalovirus-specific and UL40-mediated imprint in the natural killer cell repertoire is associated with antibody-mediated rejection in lung transplant recipients. J Heart Lung Transplant. (2023) 42(3):305–14. doi: 10.1016/j.healun.2022.10.014
19. Kraft CS, Armstrong WS, Caliendo AM. Interpreting quantitative cytomegalovirus DNA testing: understanding the laboratory perspective. Clin Infect Dis. (2012) 54(12):1793–7. doi: 10.1093/cid/cis212
20. Paraskeva M, Bailey M, Levvey BJ, Griffiths AP, Kotsimbos TC, Williams TP, et al. Cytomegalovirus replication within the lung allograft is associated with bronchiolitis obliterans syndrome. Am J Transplant. (2011) 11(10):2190–6. doi: 10.1111/j.1600-6143.2011.03663.x
21. Zhang Z, Li R, Chen Y, Zhang J, Zheng Y, Xu M, et al. Association between active cytomegalovirus infection and lung fibroproliferation in adult patients with acute respiratory distress syndrome: a retrospective study. BMC Infect Dis. (2022) 22(1):1–12. doi: 10.1186/s12879-021-07004-8
22. Ribeiro RVP, Samman A, Martinu T, Keshavjee S, Singer LG, Kumar D, et al. Incidence of post-transplant cytomegalovirus viremia in patients receiving lungs after ex vivo lung perfusion. J Heart Lung Transplant. (2022) 41(4):S399. doi: 10.1016/j.healun.2022.01.1562
23. Popescu I, Mannem H, Winters SA, Hoji A, Silveira F, McNally E, et al. Impaired cytomegalovirus immunity in idiopathic pulmonary fibrosis lung transplant recipients with short telomeres. Am J Respir Crit Care Med. (2019) 199(3):362–76. doi: 10.1164/rccm.201805-0825OC
24. Forte E, Zhang Z, Thorp EB, Hummel M. Cytomegalovirus latency and reactivation: an intricate interplay with the host immune response. Front Cell Infect Microbiol. (2020) 10:130. doi: 10.3389/fcimb.2020.00130
25. Noda T, Kawaoka Y. Structure of influenza virus ribonucleoprotein complexes and their packaging into virions. Rev Med Virol. (2010) 20(6):380–91. doi: 10.1002/rmv.666
26. Limaye AP, Babu TM, Boeckh M. Progress and challenges in the prevention, diagnosis, and management of cytomegalovirus infection in transplantation. Clin Microbiol Rev. (2020) 34(1):10–1128. doi: 10.1128/CMR.00043-19
27. Streblow DN, Nelson JA. Models of HCMV latency and reactivation. Trends Microbiol. (2003) 11(7):293–5. doi: 10.1016/S0966-842X(03)00149-5
28. Dunn W, Trang P, Zhong Q, Yang E, Van Belle C, Liu F. Human cytomegalovirus expresses novel microRNAs during productive viral infection. Cell Microbiol. (2005) 7(11):1684–95. doi: 10.1111/j.1462-5822.2005.00598.x
29. Wang FZ, Weber F, Croce C, Liu CG, Liao X, Pellett PE. Human cytomegalovirus infection alters the expression of cellular microRNA species that affect its replication. J Virol. (2008) 82(18):9065–74. doi: 10.1128/JVI.00961-08
30. Grey F, Meyers H, White EA, Spector DH, Nelson J. A human cytomegalovirus-encoded microRNA regulates expression of multiple viral genes involved in replication. PLoS Pathog. (2007) 3(11):e163. doi: 10.1371/journal.ppat.0030163
31. Stern-Ginossar N, Saleh N, Goldberg MD, Prichard M, Wolf DG, Mandelboim O. Analysis of human cytomegalovirus-encoded microRNA activity during infection. J Virol. (2009) 83(20):10684–93. doi: 10.1128/JVI.01292-09
32. Shen ZZ, Pan X, Miao LF, Ye HQ, Chavanas S, Davrinche C, et al. Comprehensive analysis of human cytomegalovirus microRNA expression during lytic and quiescent infection. PLoS One. (2014) 9(2):e88531. doi: 10.1371/journal.pone.0088531
33. Landais I, Pelton C, Streblow D, DeFilippis V, McWeeney S, Nelson JA. Human cytomegalovirus miR-UL112-3p targets TLR2 and modulates the TLR2/IRAK1/NFκB signaling pathway. PLoS Pathog. (2015) 11(5):e1004881. doi: 10.1371/journal.ppat.1004881
34. Humar A, Snydman D. Cytomegalovirus in solid organ transplant recipients. Am J Transplant. (2009) 9(s4). doi: 10.1111/j.1600-6143.2009.02897.x
35. Balthesen M, Messerle M, Reddehase MJ. Lungs are a major organ site of cytomegalovirus latency and recurrence. J Virol. (1993) 67(9):5360–6. doi: 10.1128/jvi.67.9.5360-5366.1993
36. Ramanan P, Razonable RR. Cytomegalovirus infections in solid organ transplantation: a review. Infect Chemother. (2013) 45(3):260–71. doi: 10.3947/ic.2013.45.3.260
37. Cui J, Zhao K, Sun Y, Wen R, Zhang X, Li X, et al. Diagnosis and treatment for the early stage of cytomegalovirus infection during hematopoietic stem cell transplantation. Front Immunol. (2022) 13:971156. doi: 10.3389/fimmu.2022.971156
38. Razonable RR, Hayden RT. Clinical utility of viral load in management of cytomegalovirus infection after solid organ transplantation. Clin Microbiol Rev. (2013) 26(4):703–27. doi: 10.1128/CMR.00015-13
39. Singh N. Preemptive therapy versus universal prophylaxis with ganciclovir for cytomegalovirus in solid organ transplant recipients. Clin Infect Dis. (2001) 32(5):742–51. doi: 10.1086/319225
40. Beck S, Barrell BG. Human cytomegalovirus encodes a glycoprotein homologous to MHC class-I antigens. Nature. (1988) 331(6153):269–72. doi: 10.1038/331269a0
41. Pardieck IN, Beyrend G, Redeker A, Arens R. Cytomegalovirus infection and progressive differentiation of effector-memory T cells. F1000Res. (2018) 7. doi: 10.12688/f1000research.15753.1
42. Anzivino E, Bellizzi A, Mitterhofer AP, Tinti F, Barile M, Colosimo MT, et al. Early monitoring of the human polyomavirus BK replication and sequencing analysis in a cohort of adult kidney transplant patients treated with basiliximab. Virol J. (2011) 8:1–10. doi: 10.1186/1743-422X-8-407
43. Stuart BD, Lee JS, Kozlitina J, Noth I, Devine MS, Glazer CS, et al. Effect of telomere length on survival in patients with idiopathic pulmonary fibrosis: an observational cohort study with independent validation. Lancet Respir Med. (2014) 2(7):557–65. doi: 10.1016/S2213-2600(14)70124-9
44. Newton CA, Batra K, Torrealba J, Kozlitina J, Glazer CS, Aravena C, et al. Telomere-related lung fibrosis is diagnostically heterogeneous but uniformly progressive. Eur Respir J. (2016) 48(6):1710–20. doi: 10.1183/13993003.00308-2016
45. Åsberg A, Jardine AG, Bignamini AA, Rollag H, Pescovitz MD, Gahlemann CC, et al. Effects of the intensity of immunosuppressive therapy on outcome of treatment for CMV disease in organ transplant recipients. Am J Transplant. (2010) 10(8):1881–8. doi: 10.1111/j.1600-6143.2010.03114.x
46. Roberts MB, Fishman JA. Immunosuppressive agents and infectious risk in transplantation: managing the “net state of immunosuppression”. Clin Infect Dis. (2021) 73(7):e1302–17. doi: 10.1093/cid/ciaa1189
47. Karami S, Roshandel E, Ghaffari Nazari H, Hajifathali A, Tavakoli F, Parkhideh S. In-depth summary over cytomegalovirus infection in allogeneic hematopoietic stem cell transplantation recipients. Virusdisease. (2021) 32(3):422–34. doi: 10.1007/s13337-021-00728-w
48. Luscalov S, Loga L, Dican L, Junie LM. Cytomegalovirus infection in immunosuppressed patients after kidney transplantation. Clujul Med. (2016) 89(3):343. doi: 10.15386/cjmed-587
49. Greenland JR, Chong T, Wang AS, Martinez E, Shrestha P, Kukreja J, et al. Suppressed calcineurin-dependent gene expression identifies lung allograft recipients at increased risk of infection. Am J Transplant. (2018) 18(8):2043–9. doi: 10.1111/ajt.14886
50. Vanarsdall AL, Ryckman BJ, Chase MC, Johnson DC. Human cytomegalovirus glycoproteins gB and gH/gL mediate epithelial cell-cell fusion when expressed either in cis or in trans. J Virol. (2008) 82(23):11837–50. doi: 10.1128/JVI.01623-08
51. Boehme KW, Guerrero M, Compton T. Human cytomegalovirus envelope glycoproteins B and H are necessary for TLR2 activation in permissive cells. J Immunol. (2006) 177(10):7094–102. doi: 10.4049/jimmunol.177.10.7094
52. Mosser DM, Edwards JP. Exploring the full spectrum of macrophage activation. Nat Rev Immunol. (2008) 8(12):958–69. doi: 10.1038/nri2448
53. Tang J, Frascaroli G, Lebbink RJ, Ostermann E, Brune W. Human cytomegalovirus glycoprotein B variants affect viral entry, cell fusion, and genome stability. Proc Natl Acad Sci USA. (2019) 116(36):18021–30. doi: 10.1073/pnas.1907447116
54. Romo N, Magri G, Muntasell A, Heredia G, Baía D, Angulo A, et al. Natural killer cell-mediated response to human cytomegalovirus-infected macrophages is modulated by their functional polarization. J Leukoc Biol. (2011) 90(4):717–26. doi: 10.1189/jlb.0311171
55. Goerdt S, Orfanos CE. Other functions, other genes: alternative activation of antigen-presenting cells. Immunity. (1999) 10(2):137–42. doi: 10.1016/S1074-7613(00)80014-X
56. Sinzger C, Eberhardt K, Cavignac Y, Weinstock C, Kessler T, Jahn G, et al. Macrophage cultures are susceptible to lytic productive infection by endothelial-cell-propagated human cytomegalovirus strains and present viral IE1 protein to CD4+ T cells despite late downregulation of MHC class II molecules. J Gen Virol. (2006) 87(7):1853–62. doi: 10.1099/vir.0.81595-0
57. Smith PD, Shimamura M, Musgrove LC, Dennis EA, Bimczok D, Novak L, et al. Cytomegalovirus enhances macrophage TLR expression and MyD88-mediated signal transduction to potentiate inducible inflammatory responses. J Immunol. (2014) 193(11):5604–12. doi: 10.4049/jimmunol.1302608
58. Frascaroli G, Varani S, Blankenhorn N, Pretsch R, Bacher M, Leng L, et al. Human cytomegalovirus paralyzes macrophage motility through down-regulation of chemokine receptors, reorganization of the cytoskeleton, and release of macrophage migration inhibitory factor. J Immunol. (2009) 182(1):477–88. doi: 10.4049/jimmunol.182.1.477
59. Bestard O, Kaminski H, Couzi L, Fernández-Ruiz M, Manuel O. Cytomegalovirus cell-mediated immunity: ready for routine use? Transpl Int. (2023) 36:11963. doi: 10.3389/ti.2023.11963
60. Ferlazzo G, Tsang ML, Moretta L, Melioli G, Steinman RM, Munz C. Human dendritic cells activate resting natural killer (NK) cells and are recognized via the NKp30 receptor by activated NK cells. J Exp Med. (2002) 195(3):343–51. doi: 10.1084/jem.20011149
61. Pende D, Castriconi R, Romagnani P, Spaggiari GM, Marcenaro S, Dondero A, et al. Expression of the DNAM-1 ligands, Nectin-2 (CD112) and poliovirus receptor (CD155), on dendritic cells: relevance for natural killer-dendritic cell interaction. Blood. (2006) 107(5):2030–6. doi: 10.1182/blood-2005-07-2696
62. Smith W, Tomasec P, Aicheler R, Loewendorf A, Nemčovičová I, Wang ECY, et al. Human cytomegalovirus glycoprotein UL141 targets the TRAIL death receptors to thwart host innate antiviral defenses. Cell Host Microbe. (2013) 13(3):324–35. doi: 10.1016/j.chom.2013.02.003
63. Li H, Wang A, Zhang Y, Wei F. Diverse roles of lung macrophages in the immune response to influenza a virus. Front Microbiol. (2023) 14:1260543. doi: 10.3389/fmicb.2023.1260543
64. Bai X, Yang W, Li H, Zhao Y, Fan W, Zhang H, et al. Cyclosporine a regulates influenza a virus-induced macrophages polarization and inflammatory responses by targeting cyclophilin a. Front Immunol. (2022) 13:861292. doi: 10.3389/fimmu.2022.861292
65. Baasch S, Ruzsics Z, Henneke P. Cytomegaloviruses and macrophages—friends and foes from early on? Front Immunol. (2020) 11:521287. doi: 10.3389/fimmu.2020.00793
66. Palmer SM, Burch LH, Trindade AJ, Davis RD, Herczyk WF, Reinsmoen NL, et al. Innate immunity influences long-term outcomes after human lung transplant. Am J Respir Crit Care Med. (2005) 171(7):780–5. doi: 10.1164/rccm.200408-1129OC
67. Palmer SM, Burch LH, Davis RD, Herczyk WF, Howell DN, Reinsmoen NL, et al. The role of innate immunity in acute allograft rejection after lung transplantation. Am J Respir Crit Care Med. (2003) 168(6):628–32. doi: 10.1164/rccm.200303-447OC
68. Humbert M, Devergne O, Cerrina J, Rain B, Simonneau G, Dartevelle P, et al. Activation of macrophages and cytotoxic cells during cytomegalovirus pneumonia complicating lung transplantations. Am Rev Respir Dis. (1992) 145(5):1178–84. doi: 10.1164/ajrccm/145.5.1178
69. Weseslindtner L, Görzer I, Roedl K, Küng E, Jaksch P, Klepetko W, et al. Intrapulmonary human cytomegalovirus replication in lung transplant recipients is associated with a rise of CCL-18 and CCL-20 chemokine levels. Transplantation. (2017) 101(1):197–203. doi: 10.1097/TP.0000000000001065
70. Vanaudenaerde BM, Dupont LJ, Wuyts WA, Verbeken EK, Meyts I, Bullens DM, et al. The role of interleukin-17 during acute rejection after lung transplantation. Eur Respir J. (2006) 27(4):779–87. doi: 10.1183/09031936.06.00019405
71. Snell GI, Levvey BJ, Zheng L, Bailey M, Orsida B, Williams TJ, et al. Interleukin-17 and airway inflammation: a longitudinal airway biopsy study after lung transplantation. J Heart Lung Transplant. (2007) 26(7):669–74. doi: 10.1016/j.healun.2007.05.004
72. Fildes JE, Yonan N, Leonard CT. Natural killer cells and lung transplantation, roles in rejection, infection, and tolerance. Transpl Immunol. (2008) 19(1):1–11. doi: 10.1016/j.trim.2008.01.004
73. Benichou G, Yamada Y, Aoyama A, Madsen JC. Natural killer cells in rejection and tolerance of solid organ allografts. Curr Opin Organ Transplant. (2011) 16(1):47. doi: 10.1097/MOT.0b013e32834254cf
74. Vivier E, Raulet DH, Moretta A, Caligiuri MA, Zitvogel L, Lanier LL, et al. Innate or adaptive immunity? The example of natural killer cells. Science (1979). (2011) 331(6013):44–9. doi: 10.1126/science.1198687
75. Calabrese DR, Lanier LL, Greenland JR. Natural killer cells in lung transplantation. Thorax. (2018) 74(4):397–404. doi: 10.1136/thoraxjnl-2018-212345
76. Mujal AM, Delconte RB, Sun JC. Natural killer cells: from innate to adaptive features. Annu Rev Immunol. (2021) 39:417–47. doi: 10.1146/annurev-immunol-101819-074948
77. Schuster IS, Coudert JD, Andoniou CE, Degli-Esposti MA. “Natural regulators”: NK cells as modulators of T cell immunity. Front Immunol. (2016) 7:235. doi: 10.3389/fimmu.2016.00235
78. Su HC, Nguyen KB, Salazar-Mather TP, Ruzek MC, Dalod MY, Biron CA. NK cell functions restrain T cell responses during viral infections. Eur J Immunol. (2001) 31(10):3048–55. doi: 10.1002/1521-4141(2001010)31:10%3C3048::AID-IMMU3048%3E3.0.CO;2-1
79. Braud VM, Allan DSJ, O’Callaghan CA, Söderström K, D’Andrea A, Ogg GS, et al. HLA-E binds to natural killer cell receptors CD94/NKG2A, B and C. Nature. (1998) 391(6669):795–9. doi: 10.1038/35869
80. Sharpe HR, Bowyer G, Brackenridge S, Lambe T. HLA-E: exploiting pathogen-host interactions for vaccine development. Clin Exp Immunol. (2019) 196(2):167–77. doi: 10.1111/cei.13292
81. Pupuleku A, Costa-García M, Farré D, Hengel H, Angulo A, Muntasell A, et al. Elusive role of the CD94/NKG2C NK cell receptor in the response to cytomegalovirus: novel experimental observations in a reporter cell system. Front Immunol. (2017) 8:1317. doi: 10.3389/fimmu.2017.01317
82. McQueen KL, Freeman JD, Takei F, Mager DL. Localization of five new Ly49 genes, including three closely related to Ly49c. Immunogenetics. (1998) 48:174–83. doi: 10.1007/s002510050421
83. Daniels KA, Devora G, Lai WC, O’Donnell CL, Bennett M, Welsh RM. Murine cytomegalovirus is regulated by a discrete subset of natural killer cells reactive with monoclonal antibody to Ly49H. J Exp Med. (2001) 194(1):29–44. doi: 10.1084/jem.194.1.29
84. Smith HRC, Heusel JW, Mehta IK, Kim S, Dorner BG, Naidenko OV, et al. Recognition of a virus-encoded ligand by a natural killer cell activation receptor. Proc Natl Acad Sci USA. (2002) 99(13):8826–31. doi: 10.1073/pnas.092258599
85. Sun JC, Beilke JN, Lanier LL. Adaptive immune features of natural killer cells. Nature. (2009) 457(7229):557–61. doi: 10.1038/nature07665
86. Adams NM, Geary CD, Santosa EK, Lumaquin D, Le Luduec JB, Sottile R, et al. Cytomegalovirus infection drives avidity selection of natural killer cells. Immunity. (2019) 50(6):1381–90. doi: 10.1016/j.immuni.2019.04.009
87. Schuster IS, Sng XYX, Lau CM, Powell DR, Weizman OE, Fleming P, et al. Infection induces tissue-resident memory NK cells that safeguard tissue health. Immunity. (2023) 56(3):531–46. doi: 10.1016/j.immuni.2023.01.016
88. Ishiyama K, Arakawa-Hoyt J, Aguilar OA, Damm I, Towfighi P, Sigdel T, et al. Mass cytometry reveals single-cell kinetics of cytotoxic lymphocyte evolution in CMV-infected renal transplant patients. Proc Natl Acad Sci USA. (2022) 119(8):e2116588119. doi: 10.1073/pnas.2116588119
89. Gumá M, Angulo A, Vilches C, Gómez-Lozano N, Malats N, López-Botet M. Imprint of human cytomegalovirus infection on the NK cell receptor repertoire. Blood. (2004) 104(12):3664–71. doi: 10.1182/blood-2004-05-2058
90. Foley B, Cooley S, Verneris MR, Pitt M, Curtsinger J, Luo X, et al. Cytomegalovirus reactivation after allogeneic transplantation promotes a lasting increase in educated NKG2C+ natural killer cells with potent function. Blood. J Am Soc Hematol. (2012) 119(11):2665–74. doi: 10.1182/blood-2011-10-386995
91. Gumá M, Budt M, Sáez A, Brckalo T, Hengel H, Angulo A, et al. Expansion of CD94/NKG2C+ NK cells in response to human cytomegalovirus-infected fibroblasts. Blood. (2006) 107(9):3624–31. doi: 10.1182/blood-2005-09-3682
92. Vietzen H, Zoufaly A, Traugott M, Aberle J, Aberle SW, Puchhammer-Stöckl E. Deletion of the NKG2C receptor encoding KLRC2 gene and HLA-E variants are risk factors for severe COVID-19. Genet Med. (2021) 23(5):963–7. doi: 10.1038/s41436-020-01077-7
93. Vietzen H, Pollak K, Honsig C, Jaksch P, Puchhammer-Stöckl E. NKG2C deletion is a risk factor for human cytomegalovirus viremia and disease after lung transplantation. J Infect Dis. (2018) 217(5):802–6. doi: 10.1093/infdis/jix608
94. Calabrese DR, Chong T, Wang A, Singer JP, Gottschall M, Hays SR, et al. NKG2C natural killer cells in bronchoalveolar lavage are associated with cytomegalovirus viremia and poor outcomes in lung allograft recipients. Transplantation. (2019) 103(3):493–501. doi: 10.1097/TP.0000000000002450
95. Zhang J, Cao J, Zheng R, Yu M, Lin Z, Wang C, et al. The establishment of a cytomegalovirus-specific CD8+ T-cell threshold by kinetic modeling for the prediction of post-hemopoietic stem cell transplant reactivation. iScience. (2022) 25(11):105340. doi: 10.1016/j.isci.2022.105340
96. Chan G, Bivins-Smith ER, Smith MS, Yurochko AD. NF-κB and phosphatidylinositol 3-kinase activity mediates the HCMV-induced atypical M1/M2 polarization of monocytes. Virus Res. (2009) 144(1–2):329–33. doi: 10.1016/j.virusres.2009.04.026
97. Reddehase MJ, Weiland F, Münch K, Jonjic S, Lüske A, Koszinowski UH. Interstitial murine cytomegalovirus pneumonia after irradiation: characterization of cells that limit viral replication during established infection of the lungs. J Virol. (1985) 55(2):264–73. doi: 10.1128/jvi.55.2.264-273.1985
98. Akulian JA, Pipeling MR, John ER, Orens JB, Lechtzin N, McDyer JF. High-quality CMV-specific CD4+ memory is enriched in the lung allograft and is associated with mucosal viral control. Am J Transplant. (2013) 13(1):146–56. doi: 10.1111/j.1600-6143.2012.04282.x
99. Khan N, Cobbold M, Keenan R, Moss PAH. Comparative analysis of CD8+ T cell responses against human cytomegalovirus proteins pp65 and immediate early 1 shows similarities in precursor frequency, oligoclonality, and phenotype. J Infect Dis. (2002) 185(8):1025–34. doi: 10.1086/339963
100. Martin MD, Badovinac VP. Defining memory CD8 T cell. Front Immunol. (2018) 9:2692. doi: 10.3389/fimmu.2018.02692
101. Appay V, Dunbar PR, Callan M, Klenerman P, Gillespie GMA, Papagno L, et al. Memory CD8+ T cells vary in differentiation phenotype in different persistent virus infections. Nat Med. (2002) 8(4):379–85. doi: 10.1038/nm0402-379
102. Libri V, Azevedo RI, Jackson SE, Di Mitri D, Lachmann R, Fuhrmann S, et al. Cytomegalovirus infection induces the accumulation of short-lived, multifunctional CD4+ CD45RA+ CD27− T cells: the potential involvement of interleukin-7 in this process. Immunology. (2011) 132(3):326–39. doi: 10.1111/j.1365-2567.2010.03386.x
103. La Rosa C, Diamond DJ. The immune response to human CMV. Future Virol. (2012) 7(3):279–93. doi: 10.2217/fvl.12.8
104. Westall G, Kotsimbos T, Brooks A. CMV-specific CD8+ T-cell dynamics in the blood and the lung allograft reflect viral reactivation following lung transplantation. Am J Transplant. (2006) 6(3):577–84. doi: 10.1111/j.1600-6143.2005.01212.x
105. Pipeling MR, West EE, Osborne CM, Whitlock AB, Dropulic LK, Willett MH, et al. Differential CMV-specific CD8+ effector T cell responses in the lung allograft predominate over the blood during human primary infection. J Immunol. (2008) 181(1):546–56. doi: 10.4049/jimmunol.181.1.546
106. Pickering H, Arakawa-Hoyt J, Llamas M, Ishiyama K, Sun Y, Parmar R, et al. CMV-expanded, phenotypically heterogenous CD8 TEMRA differentially associate with viral control and allograft outcomes. J Heart Lung Transplant. (2023) 42(4):S109. doi: 10.1016/j.healun.2023.02.1525
107. Schulz U, Solidoro P, Müller V, Szabo A, Gottlieb J, Wilkens H, et al. CMV immunoglobulins for the treatment of CMV infections in thoracic transplant recipients. Transplantation. (2016) 100(Suppl 3):S5. doi: 10.1097/TP.0000000000001097
108. Stankovic S, Davey MS, Shaw EM, von Borstel A, Cristiano Y, Levvey BJ, et al. Cytomegalovirus replication is associated with enrichment of distinct γδ T cell subsets following lung transplantation: a novel therapeutic approach? J Heart Lung Transplant. (2020) 39(11):1300–12. doi: 10.1016/j.healun.2020.08.014
109. Scheper W, Sebestyen Z, Kuball J. Cancer immunotherapy using γδ T cells: dealing with diversity. Front Immunol. (2014) 5:601. doi: 10.3389/fimmu.2014.00601
110. Wu YL, Ding YP, Tanaka Y, Shen LW, Wei CH, Minato N, et al. γδ T cells and their potential for immunotherapy. Int J Biol Sci. (2014) 10(2):119. doi: 10.7150/ijbs.7823
111. Kabelitz D, Déchanet-Merville J. Recent advances in gamma/delta T cell biology: new ligands, new functions, and new translational perspectives. Front Immunol. (2015) 6:371. doi: 10.3389/fimmu.2015.00371
112. Chien Yh, Meyer C, Bonneville M. γδ T cells: first line of defense and beyond. Annu Rev Immunol. (2014) 32:121–55. doi: 10.1146/annurev-immunol-032713-120216
113. Guan P, Bassiri H, Patel NP, Nichols KE, Das R. Invariant natural killer T cells in hematopoietic stem cell transplantation: killer choice for natural suppression. Bone Marrow Transplant. (2016) 51(5):629–37. doi: 10.1038/bmt.2015.335
114. Lafarge X, Merville P, Cazin MC, Bergé F, Potaux L, Moreau JF, et al. Cytomegalovirus infection in transplant recipients resolves when circulating γδ T lymphocytes expand, suggesting a protective antiviral role. J Infect Dis. (2001) 184(5):533–41. doi: 10.1086/322843
115. Khairallah C, Netzer S, Villacreces A, Juzan M, Rousseau B, Dulanto S, et al. γδ T cells confer protection against murine cytomegalovirus (MCMV). PLoS Pathog. (2015) 11(3):e1004702. doi: 10.1371/journal.ppat.1004702
116. Jeitziner SM, Walton SM, Torti N, Oxenius A. Adoptive transfer of cytomegalovirus-specific effector CD 4+ T cells provides antiviral protection from murine CMV infection. Eur J Immunol. (2013) 43(11):2886–95. doi: 10.1002/eji.201343690
117. Widmann T, Sester U, Gärtner BC, Schubert J, Pfreundschuh M, Köhler H, et al. Levels of CMV specific CD4 T cells are dynamic and correlate with CMV viremia after allogeneic stem cell transplantation. PLoS One. (2008) 3(11):e3634. doi: 10.1371/journal.pone.0003634
118. Lim EY, Jackson SE, Wills MR. The CD4+ T cell response to human cytomegalovirus in healthy and immunocompromised people. Front Cell Infect Microbiol. (2020) 10:202. doi: 10.3389/fcimb.2020.00202
119. Juno JA, Van Bockel D, Kent SJ, Kelleher AD, Zaunders JJ, Munier CML. Cytotoxic CD4 T cells—friend or foe during viral infection? Front Immunol. (2017) 8:19. doi: 10.3389/fimmu.2017.00019
120. Sant AJ, McMichael A. Revealing the role of CD4+ T cells in viral immunity. J Exp Med. (2012) 209(8):1391–5. doi: 10.1084/jem.20121517
121. Swain SL, McKinstry KK, Strutt TM. Expanding roles for CD4+ T cells in immunity to viruses. Nat Rev Immunol. (2012) 12(2):136–48. doi: 10.1038/nri3152
122. Matsuda Y, Watanabe T, Li XK. Approaches for controlling antibody-mediated allograft rejection through targeting B cells. Front Immunol. (2021) 12:682334. doi: 10.3389/fimmu.2021.682334
123. Zeng Q, Ng YH, Singh T, Jiang K, Sheriff KA, Ippolito R, et al. B cells mediate chronic allograft rejection independently of antibody production. J Clin Invest. (2014) 124(3):1052–6. doi: 10.1172/JCI70084
124. Sharma S, Thomas PG. The two faces of heterologous immunity: protection or immunopathology. J Leukoc Biol. (2014) 95(3):405–16. doi: 10.1189/jlb.0713386
125. Cantisán S, Torre-Cisneros J, Lara R, Rodríguez-Benot A, Santos F, Gutiérrez-Aroca J, et al. Age-dependent association between low frequency of CD27/CD28 expression on pp65 CD8+ T cells and cytomegalovirus replication after transplantation. Clin Vaccine Immunol. (2009) 16(10):1429–38. doi: 10.1128/CVI.00214-09
126. Mason D. A very high level of crossreactivity is an essential feature of the T-cell receptor. Immunol Today. (1998) 19(9):395–404. doi: 10.1016/S0167-5699(98)01299-7
127. Yang HY, Dundon PL, Nahill SR, Welsh RM. Virus-induced polyclonal cytotoxic T lymphocyte stimulation. J Immunol. (1989) 142(5):1710–8. doi: 10.4049/jimmunol.142.5.1710
128. Winter JB, Gouw ASH, Groen M, Wildevuur C, Prop J. Respiratory viral infections aggravate airway damage caused by chronic rejection in rat lung allografts. Transplantation. (1994) 57(3):418–22. doi: 10.1097/00007890-199402150-00018
129. Bayard C, Lepetitcorps H, Roux A, Larsen M, Fastenackels S, Salle V, et al. Coordinated expansion of both memory T cells and NK cells in response to CMV infection in humans. Eur J Immunol. (2016) 46(5):1168–79. doi: 10.1002/eji.201546179
130. Tomescu C, Kroll K, Colon K, Papasavvas E, Frank I, Tebas P, et al. Identification of the predominant human NK cell effector subset mediating ADCC against HIV-infected targets coated with BNAbs or plasma from PLWH. Eur J Immunol. (2021) 51(8):2051–61. doi: 10.1002/eji.202149188
131. Calabrese DR, Chong T, Singer JP, Rajalingam R, Hays SR, Kukreja J, et al. CD16+ natural killer cells in bronchoalveolar lavage are associated with antibody-mediated rejection and chronic lung allograft dysfunction. Am J Transplant. (2023) 23(1):37–44. doi: 10.1016/j.ajt.2022.10.006
132. Wang ECY, McSharry B, Retiere C, Tomasec P, Williams S, Borysiewicz LK, et al. UL40-mediated NK evasion during productive infection with human cytomegalovirus. Proc Natl Acad Sci USA. (2002) 99(11):7570–5. doi: 10.1073/pnas.112680099
133. Reeves MB, Coleman H, Chadderton J, Goddard M, Sissons JGP, Sinclair JH. Vascular endothelial and smooth muscle cells are unlikely to be major sites of latency of human cytomegalovirus in vivo. J Gen Virol. (2004) 85(11):3337–41. doi: 10.1099/vir.0.80285-0
134. Fonseca Brito L, Brune W, Stahl FR. Cytomegalovirus (CMV) pneumonitis: cell tropism, inflammation, and immunity. Int J Mol Sci. (2019) 20(16):3865. doi: 10.3390/ijms20163865
135. Holtappels R, Podlech J, Geginat G, Steffens HP, Thomas D, Reddehase MJ. Control of murine cytomegalovirus in the lungs: relative but not absolute immunodominance of the immediate-early 1 nonapeptide during the antiviral cytolytic T-lymphocyte response in pulmonary infiltrates. J Virol. (1998) 72(9):7201–12. doi: 10.1128/JVI.72.9.7201-7212.1998
136. Li W, Gauthier JM, Higashikubo R, Hsiao HM, Tanaka S, Vuong L, et al. Bronchus-associated lymphoid tissue–resident Foxp3+ T lymphocytes prevent antibody-mediated lung rejection. J Clin Invest. (2019) 129(2):556–68. doi: 10.1172/JCI122083
137. Wang P, Leung J, Lam A, Lee S, Calabrese DR, Hays SR, et al. Lung transplant recipients with idiopathic pulmonary fibrosis have impaired alloreactive immune responses. J Heart Lung Transplant. (2022) 41(5):641–53. doi: 10.1016/j.healun.2021.11.012
138. Tokman S, Singer JP, Devine MS, Westall GP, Aubert JD, Tamm M, et al. Clinical outcomes of lung transplant recipients with telomerase mutations. J Heart Lung Transplant. (2015) 34(10):1318–24. doi: 10.1016/j.healun.2015.05.002
139. Kotton CN, Kumar D, Caliendo AM, Huprikar S, Chou S, Danziger-Isakov L, et al. The third international consensus guidelines on the management of cytomegalovirus in solid-organ transplantation. Transplantation. (2018) 102(6):900–31. doi: 10.1097/TP.0000000000002191
140. Grossi PA, Kamar N, Saliba F, Baldanti F, Aguado JM, Gottlieb J, et al. Cytomegalovirus management in solid organ transplant recipients: a pre-COVID-19 survey from the working group of the European society for organ transplantation. Transpl Int. (2022) 35:10332. doi: 10.3389/ti.2022.10332
141. Sester M, Leboeuf C, Schmidt T, Hirsch HH. The “ABC” of virus-specific T cell immunity in solid organ transplantation. Am J Transplant. (2016) 16(6):1697–706. doi: 10.1111/ajt.13684
142. Papanicolaou GA, Silveira FP, Langston AA, Pereira MR, Avery RK, Uknis M, et al. Maribavir for refractory or resistant cytomegalovirus infections in hematopoietic-cell or solid-organ transplant recipients: a randomized, dose-ranging, double-blind, phase 2 study. Clin Infect Dis. (2019) 68(8):1255–64. doi: 10.1093/cid/ciy706
143. Veit T, Munker D, Barton J, Milger K, Kauke T, Meiser B, et al. Letermovir in lung transplant recipients with cytomegalovirus infection: a retrospective observational study. Am J Transplant. (2021) 21(10):3449–55. doi: 10.1111/ajt.16718
144. Avery RK, Arav-Boger R, Marr KA, Kraus E, Shoham S, Lees L, et al. Outcomes in transplant recipients treated with foscarnet for ganciclovir-resistant or refractory cytomegalovirus infection. Transplantation. (2016) 100(10):e74. doi: 10.1097/TP.0000000000001418
145. Flores-Gonzalez J, Ramon-Luing LA, Ocaña-Guzman R, Buendia-Roldan I, Islas-Muñoz B, Volkow-Fernández P, et al. Valganciclovir as add-on therapy modifies the frequency of NK and NKT cell subpopulations in disseminated kaposi sarcoma patients. Cancers. (2022) 14(2):412. doi: 10.3390/cancers14020412
146. Hunt PW, Martin JN, Sinclair E, Epling L, Teague J, Jacobson MA, et al. Valganciclovir reduces T cell activation in HIV-infected individuals with incomplete CD4+ T cell recovery on antiretroviral therapy. J Infect Dis. (2011) 203(10):1474–83. doi: 10.1093/infdis/jir060
147. Zieliński M, Tarasewicz A, Zielińska H, Jankowska M, Moszkowska G, Dębska-Ślizień A, et al. Impact of donor and recipient human cytomegalovirus status on kidney transplantation. Int Immunol. (2017) 29(12):541–9. doi: 10.1093/intimm/dxx062
148. Snyder LD, Medinas R, Chan C, Sparks S, Davis WA, Palmer SM, et al. Polyfunctional cytomegalovirus-specific immunity in lung transplant recipients receiving valganciclovir prophylaxis. Am J Transplant. (2011) 11(3):553–60. doi: 10.1111/j.1600-6143.2010.03405.x
149. Hoetzenecker K, Hacker S, Hoetzenecker W, Sadeghi K, Sachet M, Pollreisz A, et al. Cytomegalovirus hyperimmunoglobulin: mechanisms in allo-immune response in vitro. Eur J Clin Invest. (2007) 37(12):978–86. doi: 10.1111/j.1365-2362.2007.01881.x
150. van Gent R, Jaadar H, Tjon ASW, Mancham S, Kwekkeboom J. T-cell inhibitory capacity of hyperimmunoglobulins is influenced by the production process. Int Immunopharmacol. (2014) 19(1):142–4. doi: 10.1016/j.intimp.2014.01.007
151. Solidoro P, Libertucci D, Delsedime L, Ruffini E, Bosco M, Costa C, et al. Combined cytomegalovirus prophylaxis in lung transplantation: effects on acute rejection, lymphocytic bronchitis/bronchiolitis, and herpesvirus infections. In: Hong JC, editor. Transplantation Proceedings. Hershey, PA: Elsevier (2008). p. 2013–4.
152. Kwekkeboom J, Tha-In T, Tra WMW, Hop W, Boor PPC, Mancham S, et al. Hepatitis B immunoglobulins inhibit dendritic cells and T cells and protect against acute rejection after liver transplantation. Am J Transplant. (2005) 5(10):2393–402. doi: 10.1111/j.1600-6143.2005.01029.x
153. Smith C, Beagley L, Rehan S, Neller MA, Crooks P, Solomon M, et al. Autologous adoptive T-cell therapy for recurrent or drug-resistant cytomegalovirus complications in solid organ transplant recipients: a single-arm open-label phase I clinical trial. Clin Infect Dis. (2019) 68(4):632–40. doi: 10.1093/cid/ciy549
154. Hu X, Karthigeyan KP, Herbek S, Valencia SM, Jenks JA, Webster H, et al. Human cytomegalovirus mRNA-1647 vaccine candidate elicits potent and broad neutralization and higher antibody-dependent cellular cytotoxicity responses than that of the partially effective gB/MF59 vaccine. medRxiv. (2023):2008–23. doi: 10.1093/infdis/jiad593
Keywords: lung transplantation, cytomegalovirus, heterologous immunity, allograft injury, effector immune response, NK cells
Citation: Bharti R and Calabrese DR (2024) Innate and adaptive effector immune drivers of cytomegalovirus disease in lung transplantation: a double-edged sword. Front. Transplant. 3:1388393. doi: 10.3389/frtra.2024.1388393
Received: 19 February 2024; Accepted: 24 April 2024;
Published: 10 June 2024.
Edited by:
Xian C. Li, Houston Methodist Hospital, United StatesReviewed by:
Paul Schroder, University of Wisconsin Health, United StatesMaciej Zieliński, Medical University of Gdańsk, Poland
© 2024 Bharti and Calabrese. This is an open-access article distributed under the terms of the Creative Commons Attribution License (CC BY). The use, distribution or reproduction in other forums is permitted, provided the original author(s) and the copyright owner(s) are credited and that the original publication in this journal is cited, in accordance with accepted academic practice. No use, distribution or reproduction is permitted which does not comply with these terms.
*Correspondence: Daniel R. Calabrese, RGFuaWVsLmNhbGFicmVzZUB1Y3NmLmVkdQ==