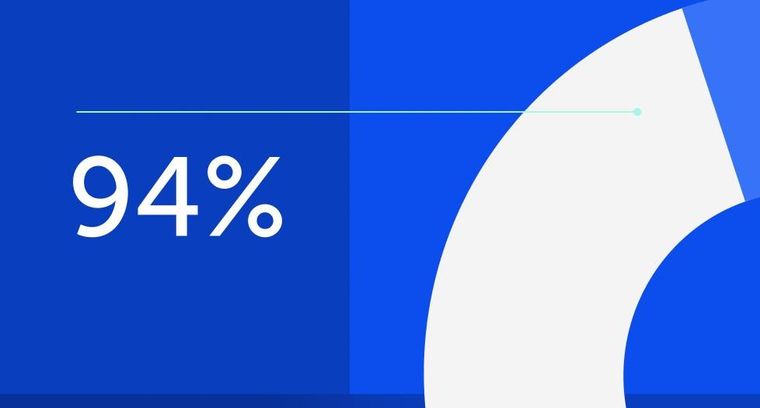
94% of researchers rate our articles as excellent or good
Learn more about the work of our research integrity team to safeguard the quality of each article we publish.
Find out more
REVIEW article
Front. Transplant., 20 December 2023
Sec. Vascularized Composite Allotransplantation
Volume 2 - 2023 | https://doi.org/10.3389/frtra.2023.1323387
This article is part of the Research TopicBio-engineered Organs and Grafts for Clinical TransplantationView all articles
The applications of Vascularized composite allotransplantation (VCA) are increasing since the first successful hand transplantation in 1998. However, the abundance of muscle tissue makes VCA's vulnerable to ischemia-reperfusion injury (IRI), which has detrimental effects on the outcome of the procedure, restricting allowable donor-to-recipient time and limiting its widespread use. The current clinical method is Static cold storage (SCS) and this allows only 6 h before irreversible damage occurs upon reperfusion. In order to overcome this obstacle, the focus of research has been shifted towards the prospect of ex-vivo perfusion preservation which already has an established clinical role in solid organ transplants especially in the last decade. In this comprehensive qualitative review, we compile the literature on all VCA machine perfusion models and we aim to highlight the essentials of an ex vivo perfusion set-up, the different strategies, and their associated outcomes.
The history of organ preservation using machine perfusion dates back to the 1930s with the work of Carrell (1) and Lindbergh (2). The first successful clinical transplantation of machine-perfused donor organs was in the late 1960s with both the kidney (3) and liver (4) organs. However, this approach fell out of popularity for a period of time due to a better understanding of the benefits of cooling (5) and the development of preservation solutions (6–8) that provided an easier yet still effective method for organ preservation. This method is Static Cold Storage (SCS) and involves flushing the organ with a preservation solution and immersing it in the solution at 4°C. This allows up to 24-h preservation for kidneys (9) and around 12 h for livers (10, 11) without significant post-operative graft dysfunction.
To match the increasing need for organs, extended criteria donors (ECD) and donors after circulatory death (DCD) are used in increasing numbers (12, 13). These grafts are frail by definition, and machine perfusion systems come into play by allowing graft assessment and reconditioning, which cannot be achieved by SCS. For instance, ex vivo perfusion of lungs now allows high-risk organs or even discarded organs to be assessed and transplanted successfully with longer preservation times (14–16). Again, successful transplantation of declined or marginal livers can now be performed after resuscitation with machine perfusion (17, 18). Quality assessment of kidneys can also be performed before transplantation (19). Moreover, machine-perfused kidneys have been shown to have less frequent delayed graft function when compared to static cold preserved kidneys in the clinical setting (20). Machine perfusion systems now have an increasing clinical application in solid organ transplantations, exceeding the limits of SCS.
The relatively new field of vascularized composite tissue allotransplantation (VCA)—which is the simultaneous transfer of multiple types of tissues such as that of the skin, muscle, nerve, and bone as a single functioning unit—faces a distinct obstacle in terms of preservation. The obstacle is due to the abundance of muscle tissue that is highly metabolically active and sensitive to Ischemia-Reperfusion injury (IRI) (21, 22). IRI defines a series of well-studied predictable events that occur when the blood supply is cut in any given organ (23). In general terms, the depletion of ATP during ischemia results in the disruption of various membrane antiports (24), the mitochondrial electron transport chain (25), and various enzymes (26), resulting in acidosis and cellular swelling. Upon reperfusion, the accumulated cations continue to create an osmotic gradient after normalization of the extracellular space, which further induces cellular swelling and death (27). In addition, reactive oxygen species (ROS) reappear, and the reduced capability of the cell to withstand oxidative stress (28) again leads to cellular death, which clinically manifests as diminished function of the muscle (27). Also, the reperfusion phase initiates an inflammatory response (29), which is associated with sensitization and acute rejections (30). The SCS method here is not as successful as solid organs and allows only a period of 4–6 h before the detrimental effects of IRI are irreversible (27, 31).
In its third decade, the field of VCA is expanding, reaching over 140 hand–upper extremity transplantations (32) and 40 craniofacial transplantations (33) worldwide. As the clinical applications of VCA increase, and with respect to the shortcomings of SCS in muscle preservation, there has been a coincidental increase in the amount of research on the prospect of machine perfusion of limbs and other VCA models specifically in the last decade. This comprehensive review compiles the literature of all VCA machine perfusion models (Tables 1, 2) and aims to highlight the essentials of an ex vivo perfusion setup, the different strategies, and their associated outcomes.
An example of a standard ex vivo machine perfusion setup is shown in Figure 1. The limb or the composite tissue is procured with its vascular pedicle and the artery is cannulated. The circuit starts with a reservoir containing the perfusate. The perfusate is driven by a pump to a membrane oxygenator attached to a heat exchanger for the delivery of perfusate at the desired temperature. Continuous pressure monitoring is made at the level of the artery. The venous return is gravity-fed back to the reservoir to complete the circuit.
Figure 1. The essentials of ex vivo limb perfusion setup. Created with BioRender.com.
The temperature is the key determinant in the ex vivo perfusion of a limb or other composite tissue models as the metabolic activity changes according to temperature. Every 10°C drop in temperature results in about a two-fold decrease in metabolic activity (73). This relationship also impacts the composition of the perfusate and other parameters to meet the demand of the tissue at a given temperature. Currently, there is no consensus regarding the optimal perfusion temperature, and a wide range (4°C–39°C) has been used in experimental models (Tables 1, 2). Perfusion temperatures fall into one of the four categories suggested by Karangwa et al. (74): Hypothermic (0°C–12°C), Mid-thermic (13°C–24°C), Subnormothermic (25°C–34°C), and Normothermic (35°C–38°C). Experiments should be assessed in their temperature context, and this nomenclature will be used to group and review studies in the following sections.
Since the first attempts at organ preservation with machine perfusion using autologous blood in solid organs, a wide array of commercially available preservation solutions has been developed and experimented with, and custom-made cellular/acellular mixes have also been reported. Thus far, for limb and composite tissue machine perfusion experiments, there have been adaptations from solid organ preservations (Tables 1, 2). In broad terms, a perfusate can be formulated as Colloid + Electrolytes ± Oxygen carrier & Additives. This can be thought of as a mimicry of mammalian blood in which plasma contains proteins that create colloid oncotic pressure along with electrolytes and oxygen is delivered to the tissues via Hemoglobin in red blood cells (RBCs).
Colloids are macromolecules that cannot move through membranes and help to decrease the fluid escape to prevent edema. One of the most common colloids that were used in VCA machine perfusion is dextran 40, a polysaccharide that has been used for plasma volume expansion. It is the colloid used in commercial preservation solutions like Low-potassium dextran (LPD-Perfadex) and Steen solution (LPD solution with albumin) as well as in custom-made RBC-based perfusates. Albumin is the common colloid of choice in RBC-based custom perfusates as well as the aforementioned Steen solution. Pentafraction is a form of hydroxyethyl starch (HAES), which is another polysaccharide, and is the colloid used in UW-MP solution. Another compound used in machine perfusion solutions is polyethylene glycol (PEG), which is used in the enrichment of custom-made perfusates (Tables 1, 2, “Perfusate base” section). The composition of commercial products that have been tested in VCA machine perfusions can be seen in Table 3.
In RBC-based perfusates for electrolytes, crystalloid solutions have been used such as Ringer's Lactate, Plasmalyte (a crystalloid solution that mimics plasma contents closely) (75) or plasma. The mentioned commercial preservation solutions each have different electrolyte compositions, and the important difference is the Na+/K+ ratio. Solutions that have high Na+ are extracellular types while those with high K+ are intracellular types. Extracellular solutions mimic the post-ischemic environment and help the recovery of Na+/K+ -ATPase (76), whereas intracellular solutions compensate for the lack of active transport in an attempt to create cation balance (73) (Table 3).
Mammalians have an average body temperature of 37.5°C (77), and oxygen delivery is done via hemoglobin. When temperatures are lower, the metabolic rate decreases and the solubility of oxygen increases (78). With respect to this relationship, different oxygenation strategies were derived for different temperature settings.
Due to the decreased metabolic need under hypothermic conditions, perfusion without an oxygen carrier can be attempted. Direct oxygenation of the perfusate is typically done with a carbogen mixture (%95 O2/%5 CO2) (45, 50, 51), which is also used in other temperature ranges in both limb and flap models, and the perfusate partial O2 pressure is maintained around 300–500 mmHg (45, 46).
For mid-thermic and subnormothermic conditions, perfusion without an oxygen carrier has been attempted by Pendexter (34), Veraza (37), and Kruit (42) in limb models and by Taeger et al. in multiple studies (65, 67, 69) (Tables 1, 2). Most of these studies do not include SCS controls except for that of Kruit et al. in which they report worse outcomes after 18 h of perfusion in histology vs. SCS controls yet preserved muscle contractility after replantation. The effect of adding an oxygen carrier under these conditions (21°C) was tested by Burlage et al. (36) in rat hindlimbs by adding HBOC-201, a hemoglobin-based oxygen carrier polymer (250 kDa) derived from bovine hemoglobin (79) to their custom-made perfusate consisting of BSA, PEG, and muscle cell media. They reported a decrease in weight gain from a mean of 27.3% to 4.9% and better histological outcomes. The idea of preserving a limb at room temperature without any oxygen carrier is appealing because every intervention closing towards normal physiology increases the complexity and the cost of the procedure. It should, however, be noted that due to the high metabolic need of muscle, this idea may only apply for a certain range of temperatures and most probably temperatures approaching the hypothermic range. This would provide only a modest increase in preservation time compared to common SCS conditions.
For normothermic conditions, the need for an oxygen carrier is obvious and reflected in the literature (Tables 1, 2). The most common is the use of RBCs, which is typically arranged to provide a hematocrit range of 10%–15%. Said et al. (44) used HBOC-201 as an oxygen carrier in swine forelimbs and found comparable results to their previous study using RBCs as carriers. Moreover, Figueroa et al. (40) tested HBOC-201 vs. RBC based perfusate in swine forelimbs at normothermic temperatures and reported similar results for histology, although RBCs showed slightly better outcomes in weight increase and compartment pressures (13.18% ± 22.70./29 mmHg ± 15 vs. 23.10% ± 3.00/32 mmHg ± 23). Both groups showed significantly better outcomes than SCS controls.
Hemoglobin-based oxygen carriers (HBOC) are a good prospect in replacing RBCs and should be further studied as an oxygen carrier. RBCs have a significantly lower shelf life and necessitate special storage conditions and are a valuable resource. Moreover, mechanical hemolysis, the need for cross-matching, and the risk of sensitization and transmission of infectious diseases could represent issues. HBOCs, however, are acellular, have low immunogenicity, have a longer shelf life, and can be stored for up to 3 years at room temperature (80, 81).
Heparin is a common additive used in most perfusion experiments. This is used in the first batch of the perfusate to remove the residual thrombi that might form after procurement and flushing. Steroids such as methylprednisolone and dexamethasone are also commonly used additives for their effect of decreasing capillary leak and edema, but their relative effect has not been tested in the machine perfusion setting. Antibiotics such as vancomycin, cefazolin, and streptomycin have been used by some groups. Colonization may be a problem, especially for extended perfusion runs, as the perfusion system creates foreign surfaces. Currently, no published data have analyzed bacterial growth during VCA machine perfusions. Further testing will provide insights as to which antibiotics may be needed. Another common additive is the 50% Dextrose-Regular insulin combination. As the amputated limb lacks endocrine control, insulin may be used to enhance glucose uptake to the cells.
To sum up, currently, there is no optimal perfusate composition that has shown consistent results in every given temperature setting and model. Assessments should be made in the context of temperature and procedure. For instance, our group has previously tested the differences between SCS with Heparinized saline, UW, HTK, and Perfadex in an allogeneic rat model and found better results with UW and Perfadex (82). Similar experiments may be attempted especially for hypothermic and mid-thermic perfusion strategies. Studying the limits of mid-thermic perfusion without oxygen carriers can be another good focus due to the relative simplicity of the approach.
Generally, the flow is adjusted to maintain a pressure goal. The pressure goals vary but for hypothermic perfusions, a 30–40 mmHg perfusion pressure is typically used in all models, including rat, swine, and human. Kueckelhaus et al. (54) report in their pilot studies less endothelial sheer and better structural integrity of the muscle at 30 mmHg compared to 60 mmHg. The same pressure range was also used in a mid-thermic setting by Burlage (36) and Pendexter (34) in rat models. Under the subnormothermic range, Müller et al. (57) and Constantinescu (58) have used 100–150 ml/min with an RBC-based perfusate, which corresponded to 30 mmHg pressure. They reported physiologic pressures resulting in significantly more edema, but numerical data of extremities perfused at physiological pressures were not included in the publication. On the other hand, Özer et al. (55, 56) used pulsatile perfusion. The pulses were driven at 60–80 mmHg with RBC-based perfusate under the same temperatures. In normothermic perfusions, 90 mmHg, which falls into the physiologic level of mean arterial pressure, is commonly used (35, 39, 40, 47).
There are no studies that directly assess different perfusion pressures. However, in a study by Amin et al. (43), four different modalities in swine forelimbs were tested while keeping the perfusate constant (RBC + Albumin based). The first group was hypothermic (10°C) 30 mmHg (HMP-30), the second was subnormothermic (28°C) 50 mmHg (SNMP-50), the third was SNMP-70 mmHg, and the fourth group was normothermic (38°C) at 70 mmHg (NMP-70). Results were reported to be better in terms of histology and weight increase in the NMP-70 group, but they do not discuss differences between the SNMP-50 and SNMP-70 groups. More studies are required to provide better insights in the optimal perfusion pressure for each temperature setting.
Ex vivo perfusion platforms allow donor quality assessment during organ preservation. The liver, bile, urea, and coagulation cofactor productions can be monitored (83, 84). In kidney perfusions, urine production can be observed during perfusion, and kidney-specific markers such as NGAL (neutrophil gelatinase-associated lipocalin) can be measured to assess injury in the organ (85). In lungs, ventilation parameters can be analyzed as airway resistance and pulmonary compliance during reperfusion (86). On the other hand, the tissue of interest is not a single specific organ that has a pre-determined, gradable internal function for the continuation of homeostasis in VCA. The overwhelming majority of clinical applications of VCA involve extremity and craniofacial transplantations (87). In limb transplants, the goal is to achieve a viable limb with good motor and sensory function that allows daily activities to be accomplished independently. In craniofacial transplants, the goals are to improve airway stability, mastication, speech, and overall cosmesis depending on the pre-transplant condition of the patient. A common feature in these grafts is the transfer of functional muscle tissue with motor nerve coaptation to the recipient motor nerve ends. Long-term functional outcomes also depend on nerve repair level, regeneration, and rehabilitation (32, 87). In that context, graft monitoring during ex vivo perfusion differs from other organs. To document the function of the graft during preservation, assessment of muscle contraction in response to nerve stimulation has been a frequent practice (12 studies in extremity models, 40%) (Table 1). However, it must be emphasized that the depolarization of a muscle fiber at a given time is influenced by factors such as temperature, electrolyte imbalances, and pH (88). A negative response does not mean that the limb is “failing” or a perfusion without any contraction response is worse than a perfusion with contraction. There is no limb specific marker that can be used for every setting; however, regular perfusate gas analysis is important to follow markers such as lactate and potassium. Increased lactate indicates poor tissue oxygenation and a shift to anaerobic respiration in any tissue in the body (89). Potassium is abundant intracellularly (90) and is indicative of cellular damage; however, the models involve cut ends of muscle bodies and some increase is usually observed. Weight increase and compartment pressure are also monitoring modalities that do not require histopathological and metabolic analysis. There is currently no commonly accepted monitoring protocol in ex vivo VCA perfusion studies. Studies so far show the following: weight gain, perfusate gas analysis, and histopathological analysis as a common practices in monitoring VCA ex vivo perfusions. Compartment pressure, nerve stimulation, and metabolic analysis have emerged as frequent but not universal practices in monitoring VCA ex-vivo perfusion.
A common finding in all limb and VCA perfusions is the weight increase over time due to the fluid escape to the interstitium and the inevitable increase in vascular resistance and compartment pressures. An extremely wide range of weight increases have been reported (0%–99%) (Tables 1, 2).
For hypothermic conditions, Kueckelhaus et al. (54) report a 44.06% mean weight increase in swine hindlimbs after 12 h of perfusion with Perfadex. A subsequent study again by Kueckelhaus et al. (51) reported a 10% mean weight increase in swine forelimbs after 12 h of perfusion with Perfadex. These were replanted with a 7-day follow-up and compared to limbs replanted after 4 h of SCS. The perfusion group had significantly better outcomes in terms of muscle histology after replantation. Krezdorn et al. (50) perfused swine forelimbs for 24 h with Steen solution and observed a 41% mean weight increase. After replantation, perfused limbs showed better histology compared to the 4-h SCS + replantation control group. There was no information on the post-reperfusion weight or compartment examination. Haug et al. (45) reported only a 4.3% weight increase in human limbs when perfused with Steen solution for 24 h with similar perfusion parameters (30 mmHg PP goal, at 10°C).
For flap models, Brouwers et al. (64) perfused swine rectus abdominis myocutaneous flaps for 24 h at 10°C and reported a weight decrease in UW perfused flaps (−6% and −7%), whereas HTK perfused flaps had a 97% and a 60% increase in weight. After replantation and follow-up, both groups showed degenerative changes in muscle histology (Table 2).
Kruit et al. (42) perfused swine forelimbs with UW-MP solution for 18 h at mid-thermic temperature (13.5°C) and observed a mean weight gain of −2.7% for perfused limbs vs. +1.6% for SCS controls. The limbs were replanted and followed for 12 h. At the end of the follow-up, perfused limbs had a 19% weight increase, whereas SCS controls had an increase of 11.6%. The perfused limbs showed worse outcomes in histology, but they preserved contractility at the end of reperfusion. Tawa et al. (38) conducted their experiments at 21°C using a modified Steen solution (further enrichment with PEG and albumin) and observed a mean weight increase of 14.48% with continuous flow after 24 h of perfusion in swine partial hindlimbs.
Gök et al. (49) reported a 3.1% weight increase after 6 h in a rat hind limb model at 30°C–35°C using a Steen and RBC mixture. Werner et al. (53) reported a mean 0.4% decrease in weight using a RBC-plasma-based perfusate in human forearms at 30°C–33°C after 24 h of perfusion. Constantinescu et al. (58) reported a 1.32% weight increase after 12 h of perfusion with autologous blood.
Taeger et al. (65, 67, 68), in their multiple studies, reported a wide range of weight gain (49.5%–99%) after 6 h of perfusion in swine rectus abdomins flaps. They reported better tissue preservation with perfusion. However, it should be emphasized that the control groups in these studies were subjected to ischemia at room temperature rather than at 4°C (Table 3).
Under normothermic conditions, Duraes et al. (52) reported a 0.54% mean weight increase at 12 h with an RBC + albumin-based perfusate. In the subsequent studies, from the same group (39, 40, 44) they put forth the following as a discontinuation criteria for limb perfusions: (1) Arterial pressure ≥115 mmHg, (2) 20% drop in tissue O2 saturation, and (3) Compartment pressure ≥30 mmHg.
In a recent 2023 study, Meyers et al. (35) reported a positive correlation between weight increase, myocyte injury score (MIS), and potassium and lactate levels. There was a negative correlation with muscle contractility under normothermic conditions. In their experiments, they reached a 2% weight increase after 13 ± 5 h, 5% after 15 ± 6 h, 10% after 16 ± 6 h, and 20% after 19 ± 4 h of perfusion. MIS was significantly higher than the baseline at 5% weight increase, and contractility was significantly lower at 20% weight increase, when compared to baseline values. Also, they reported a significant increase in compartment pressures upon the termination of the perfusion compared to SCS-preserved limbs (56.5 mmHg vs. 10.5 mmHg).
The clinical and pathological results after reperfusion are critically important to evaluate the efficacy of machine perfusion. Thus far, 11 studies (36%) in extremity models have included some form of reperfusion. They used blood from unrelated donors (swine) in one study, replantation in five studies (four swine and one canine), and transplantation in five studies (one rat study syngeneic; two rat studies unspecified; two swine studies unspecified). Reperfusion follow-up periods ranged from 4 h to 12 weeks) (Table 1).
Under hypothermic conditions, Kueckelhaus et al. (51) observed higher heart rates, which were accompanied by arrhythmias and a drop in oxygen saturation in static cold stored preserved limb recipients vs. perfused limb (swine, 12 h, 10°C, low-potassium dextran) recipients following replantation. This clinical finding was also accompanied by higher markers of muscle injury (myoglobin, K) in the static cold storage group. Histopathology also showed segmental depletion and vacuolization of the fibers in the cold storage group after 7 days post-reperfusion. Similarly, Krezdorn et al. (50) observed that heart and respiratory rates after replantation were increased in the static cold storage group. There was increased damage in muscle biopsy specimens obtained from animals in the static cold storage group after 7 days when compared with those from animals in the perfusion group (swine, 24 h, 8°C, Steen). Furthermore, Gök et al. (49) observed that at 12 weeks post-transplantation the perfusion group (rat, 6 h, 8°C, HTK) showed similar results to the immediate transplantation group in terms of muscle injury scores and muscle contractility while static cold stored transplantations showed worse outcomes.
Under mid-thermic conditions, Kruit et al. (42) observed higher muscle injury scores in perfused limbs (18 h, 15°C, UW) at 12 h post-replantation when compared to SCS, which was attributed to edema formation during preservation. The mean threshold stimulus for muscle contraction did not differ between cold storage and perfusion groups. Clinical outcomes post-reperfusion were not assessed in this study. In the study from Burlage et al. (36), perfused limbs (rat, 6 h, 21°C, HBOC-201) showed higher transplant survival rates in comparison to SCS controls and were similar to the immediate transplant group at 30 days post-reperfusion.
In the sub-normothermic range, Özer et al. (55) observed similar outcomes in single fiber contractility tests between perfusion (swine, 12 & 24 h, RBC based) 27°C–32°C and normal control groups at 12 h post-transplant. However, SCS-preserved muscle showed a decrease in the contractility test.
Reperfusion after normothermic perfusion was tested by Amin et al. (43) and was achieved by an additional 4 h of perfusion with unrelated donor blood. Perfused limbs were hemodynamically and biochemically stable on reperfusion in comparison to those subjected to SCS, showing lower lactate, normal pH, and less edema.
In flap ex vivo perfusion models, two studies have included a reperfusion period (replantation of the flap) using swine rectus abdominis myocutaneous flaps as models (Table 2). Brouwers et al. (64) observed better outcomes of muscle injury in HTK perfused flaps when compared to UW-perfused and cold-stored flaps. Perfusion preservation was under hypothermic conditions for 24 h followed by reperfusion for 7 days. Kruit et al. (66) aimed to analyze the gene expression patterns in perfusion-preserved flaps, using HTK and UW with SCS controls. Their perfusion duration was 18 h followed by a reperfusion period of 12 h while the SCS duration was 4 h. The expression of genes related to ischemia, apoptosis, and inflammation was comparable between the ex-vivo perfusion and static cold storage groups.
Four major strategies (Hypothermic, Midthermic, Subnormothermic, and Normothermic) have emerged in VCA preservation as in solid organs with each one having advantages and limitations. Similar strategies have resulted in different outcomes in different studies, and current literature lacks evidence to make conclusions mainly due to the complex nature of these studies. Weight increase and compartment pressure increases are the common consequences of ex vivo perfusion, especially after 12 h. The current literature shows that the simulation of physiology (Normothermic/RBC) seems to have better outcomes in terms of edema. However, promising results have also been obtained in other settings even without oxygen carriers.
It must also be noted again that most of the studies so far do not include a reperfusion phase, which is important to fully assess the preservation method (Tables 1, 2, reperfusion outcomes section). To move one step further for clinical translation, the net effect of weight increase on compartment pressures and the possible early post-operative consequences of reperfusing an extremity that already has increased weight/pressures should be thoroughly studied to provide stringent criteria for discontinuation for each model used. After this, optimizing and comparing approaches will be an easier exercise. More studies are needed with reperfusion, especially allogeneic, to better understand the course of machine-preserved limbs against SCS-preserved limbs.
Currently, there is no optimal perfusate composition for limbs and muscle containing composite flaps that has shown consistent results in every given temperature setting and model. Assessments should be made in the context of temperature and procedure. For instance, our group has previously tested the differences between SCS with Heparinized saline, UW, HTK, and Perfadex in an allogeneic rat model and found better results with UW and Perfadex (82). Similar experiments may be attempted especially for hypothermic and mid-thermic perfusion strategies. Studying the limits of mid-thermic perfusion without oxygen carriers can be another good focus due to the relative simplicity of the approach.
Another important aspect of ex vivo limb perfusion studies is the sampling of muscle tissues, especially for studies that do not have a reperfusion phase. The weight increase in the proximal part of the limb will not translate into an increase in compartment pressure as the fascial compartment is released during procurement, and over the course of ex vivo perfusion this may create differences in viability compared to the distal muscles in the limb confined in their fascial compartments. There have been no studies investigating the possible differences in histopathological or metabolic outcomes of the different levels of the limb. We acknowledge that these experiments are time and resource consuming. However, this will be a good initial step to better understand the effects of weight and compartment pressure increases of ex vivo perfusions.
We also acknowledge that the perfusion duration goals in the given examples are arbitrary and designed to demonstrate how much longer an extremity or VCA can be preserved by using machine perfusion. In a future clinical scenario completing the whole procedure “as soon as possible” will remain a goal both for transplantation and replantation cases. Nevertheless, ex vivo perfusion as a method of preservation for VCA is an exciting field of research with a high potential for clinical translation.
ÇD: Conceptualization, Data curation, Investigation, Methodology, Writing – original draft. FB: Data curation, Investigation, Writing – original draft. NH: Data curation, Investigation, Writing – original draft. AD: Data curation, Investigation, Writing – original draft. VS: Data curation, Investigation, Writing – original draft. SH: Conceptualization, Investigation, Supervision, Writing – review & editing.
The author(s) declare that no financial support was received for the research, authorship, and/or publication of this article.
The authors declare that the research was conducted in the absence of any commercial or financial relationships that could be construed as a potential conflict of interest.
All claims expressed in this article are solely those of the authors and do not necessarily represent those of their affiliated organizations, or those of the publisher, the editors and the reviewers. Any product that may be evaluated in this article, or claim that may be made by its manufacturer, is not guaranteed or endorsed by the publisher.
1. Carrel A, Lindbergh CA. The culture of whole organs. Science. (1935) 81(2112):621–3. doi: 10.1126/science.81.2112.621
2. Lindbergh CA. An apparatus for the culture of whole organs. J Exp Med. (1935) 62(3):409–31. doi: 10.1084/jem.62.3.409
3. Belzer FO, Ashby BS, Gulyassy PF, Powell M. Successful seventeen-hour preservation and transplantation of human-cadaver kidney. N Engl J Med. (1968) 278(11):608–10. doi: 10.1056/NEJM196803142781108
4. Starzl TE, Groth CG, Brettschneider L, Penn I, Fulginiti VA, Moon JB, et al. Orthotopic homotransplantation of the human liver. Ann Surg. (1968) 168(3):392–415. doi: 10.1097/00000658-196809000-00009
5. Robinson WR, Peters RH, Zimmermann J. The effects of body size and temperature on metabolic rate of organisms. Can J Zool. (1983) 61(2):281–8. doi: 10.1139/z83-037
6. Wahlberg JA, Southard JH, Belzer FO. Development of a cold storage solution for pancreas preservation. Cryobiology. (1986) 23(6):477–82. doi: 10.1016/0011-2240(86)90056-8
7. Aydin G, Okiye SE, Zincke H. Successful 24-hour preservation of the ischemic canine kidney with euro-collins solution. J Urol. (1982) 128(6):1401–3. doi: 10.1016/S0022-5347(17)53517-X
8. Collins GM, Bravo-Shugarman M, Terasaki PI. Kidney preservation for transportation. Initial perfusion and 30 hours’ ice storage. Lancet. (1969) 2(7632):1219–22. doi: 10.1016/S0140-6736(69)90753-3
9. Groenewoud AF, Thorogood J. A preliminary report of the HTK randomized multicenter study comparing kidney graft preservation with HTK and EuroCollins solutions. HTK study group. Transpl Int. (1992) 5(Suppl 1):S429–32. doi: 10.1111/tri.1992.5.s1.429
10. Adam R, Bismuth H, Diamond T, Ducot B, Morino M, Astarcioglu I, et al. Effect of extended cold ischaemia with UW solution on graft function after liver transplantation. Lancet. (1992) 340(8832):1373–6. doi: 10.1016/0140-6736(92)92559-X
11. Sibulesky L, Li M, Hansen RN, Dick AAS, Montenovo MI, Rayhill SC, et al. Impact of cold ischemia time on outcomes of liver transplantation: a single center experience. Ann Transplant. (2016) 21:145–51. doi: 10.12659/AOT.896190
12. Gopalakrishnan G, Gourabathini SP. Marginal kidney donor. Indian J Urol. (2007) 23(3):286–93. doi: 10.4103/0970-1591.33726
13. Detelich D, Markmann JF. The dawn of liver perfusion machines. Curr Opin Organ Transplant. (2018) 23(2):151–61. doi: 10.1097/MOT.0000000000000500
14. Steen S, Ingemansson R, Eriksson L, Pierre L, Algotsson L, Wierup P, et al. First human transplantation of a nonacceptable donor lung after reconditioning ex vivo. Ann Thorac Surg. (2007) 83(6):2191–4. doi: 10.1016/j.athoracsur.2007.01.033
15. Cypel M, Yeung JC, Liu M, Anraku M, Chen F, Karolak W, et al. Normothermic ex vivo lung perfusion in clinical lung transplantation. N Engl J Med. (2011) 364(15):1431–40. doi: 10.1056/NEJMoa1014597
16. Machuca TN, Mercier O, Collaud S, Tikkanen J, Krueger T, Yeung JC, et al. Lung transplantation with donation after circulatory determination of death donors and the impact of ex vivo lung perfusion. Am J Transplant. (2015) 15(4):993–1002. doi: 10.1111/ajt.13124
17. Mergental H, Perera MTPR, Laing RW, Muiesan P, Isaac JR, Smith A, et al. Transplantation of declined liver allografts following normothermic ex-situ evaluation. Am J Transplant. (2016) 16(11):3235–45. doi: 10.1111/ajt.13875
18. Perera T, Mergental H, Stephenson B, Roll GR, Cilliers H, Liang R, et al. First human liver transplantation using a marginal allograft resuscitated by normothermic machine perfusion. Liver Transpl. (2016) 22:120–4. doi: 10.1002/lt.24369
19. Hosgood SA, Barlow AD, Hunter JP, Nicholson ML. Ex vivo normothermic perfusion for quality assessment of marginal donor kidney transplants. Br J Surg. (2015) 102(11):1433–40. doi: 10.1002/bjs.9894
20. Nicholson ML, Hosgood SA. Renal transplantation after ex vivo normothermic perfusion: the first clinical study. Am J Transplant. (2013) 13(5):1246–52. doi: 10.1111/ajt.12179
21. Thomason PR, Matzke HA. Effects of ischemia on the hind limb of the rat. Am J Phys Med. (1975) 54(3):113–31.1137010
22. Datta S, Fitzpatrick AM, Haykal S. Preservation solutions for attenuation of ischemia-reperfusion injury in vascularized composite allotransplantation. SAGE Open Med. (2021) 9:20503121211034924. doi: 10.1177/20503121211034924
23. Kalogeris T, Baines CP, Krenz M, Korthuis RJ. Ischemia/reperfusion. Compr Physiol. (2016) 7(1):113–70. doi: 10.1002/cphy.c160006
24. Fernández ÁF, Liu Y, Ginet V, Shi M, Nah J, Zou Z, et al. Interaction between the autophagy protein Beclin 1 and Na+, K+-ATPase during starvation, exercise, and ischemia. JCI Insight. (2020) 5(1):133282. doi: 10.1172/jci.insight.133282
25. Chen Q, Younus M, Thompson J, Hu Y, Hollander JM, Lesnefsky EJ. Intermediary metabolism and fatty acid oxidation: novel targets of electron transport chain-driven injury during ischemia and reperfusion. Am J Physiol Heart Circ Physiol. (2018) 314(4):H787–95. doi: 10.1152/ajpheart.00531.2017
26. Granger DN, Kvietys PR. Reperfusion injury and reactive oxygen species: the evolution of a concept. Redox Biol. (2015) 6:524–51. doi: 10.1016/j.redox.2015.08.020
27. Paradis S, Charles AL, Meyer A, Lejay A, Scholey JW, Chakfé N, et al. Chronology of mitochondrial and cellular events during skeletal muscle ischemia-reperfusion. Am J Physiol Cell Physiol. (2016) 310(11):C968–82. doi: 10.1152/ajpcell.00356.2015
28. Wu L, Xiong X, Wu X, Ye Y, Jian Z, Zhi Z, et al. Targeting oxidative stress and inflammation to prevent ischemia-reperfusion injury. Front Mol Neurosci. (2020) 13:28. doi: 10.3389/fnmol.2020.00028
29. Yu H, Kalogeris T, Korthuis RJ. Reactive species-induced microvascular dysfunction in ischemia/reperfusion. Free Radic Biol Med. (2019) 135:182–97. doi: 10.1016/j.freeradbiomed.2019.02.031
30. Slegtenhorst BR, Dor FJ, Rodriguez H, Voskuil FJ, Tullius SG. Ischemia/reperfusion injury and its consequences on immunity and inflammation. Curr Transplant Rep. (2014) 1(3):147–54. doi: 10.1007/s40472-014-0017-6
31. Eriksson E, Anderson WA, Replogle RL. Effects of prolonged ischemia on muscle microcirculation in the cat. Surg Forum. (1974) 25(0):254–5.4439181
32. Wells MW, Rampazzo A, Papay F, Gharb BB. Two decades of hand transplantation: a systematic review of outcomes. Ann Plast Surg. (2022) 88:335–44. doi: 10.1097/SAP.0000000000003056
33. Alberti FB, Hoyle V. Face transplants: an international history. J Hist Med Allied Sci. (2021) 76(3):319–45. doi: 10.1093/jhmas/jrab019
34. Pendexter CA, Haque O, Mojoudi M, Maggipinto S, Goutard M, Baicu S, et al. Development of a rat forelimb vascularized composite allograft (VCA) perfusion protocol. PLoS One. (2023) 18(1):1–13. doi: 10.1371/journal.pone.0266207
35. Meyers A, Pandey S, Kopparthy V, Sadeghi P, Clark RC, Figueroa B, et al. Weight gain is an early indicator of injury in ex vivo normothermic limb perfusion (EVNLP). Artif Organs. (2023) 47(2):290–301. doi: 10.1111/aor.14442
36. Burlage LC, Lellouch AG, Taveau CB, Tratnig-Frankl P, Pendexter CA, Randolph MA, et al. Optimization of ex vivo machine perfusion and transplantation of vascularized composite allografts. J Surg Res. (2022) 270:151–61. doi: 10.1016/j.jss.2021.09.005
37. Veraza RJ, Lopez R, Parry O, Sleeter J, Cano I, Bohara U, et al. Proof of concept study for a closed ex vivo limb perfusion system for 24-hour subnormothermic preservation using acellular perfusate. J Trauma Acute Care Surg. (2022) 93(2):S102–9. doi: 10.1097/TA.0000000000003688
38. Tawa P, Goutard M, Andrews AR, De Vries RJ, Rosales IA, Yeh H, et al. Sciencedirect continuous versus pulsatile flow in 24-hour vascularized composite allograft machine perfusion in swine: a pilot study. J Surg Res. (2022) 283:1145–53. doi: 10.1016/j.jss.2022.11.003
39. Rezaei M, Ordenana C, Figueroa BA, Said SA, Fahradyan V, Dalla Pozza E, et al. Ex vivo normothermic perfusion of human upper limbs. Transplantation. (2022) 106(8):1638–46. doi: 10.1097/TP.0000000000004045
40. Figueroa BA, Said SA, Ordenana C, Rezaei M, Orfahli LM, Dubé GP, et al. Ex vivo normothermic preservation of amputated limbs with a hemoglobin-based oxygen carrier perfusate. J Trauma Acute Care Surg. (2022) 92(2):388–97. doi: 10.1097/TA.0000000000003395
41. Rohde E, Goudarzi M, Madajka M, Said SAD, Ordenana C, Rezaei M, et al. Metabolic profiling of skeletal muscle during ex-vivo normothermic limb perfusion. Mil Med. (2021) 186(Suppl 1):358–63. doi: 10.1093/milmed/usaa268
42. Kruit AS, Brouwers K, van Midden D, Zegers H, Koers E, van Alfen N, et al. Successful 18-h acellular extracorporeal perfusion and replantation of porcine limbs—histology versus nerve stimulation. Transpl Int. (2021) 34(2):365–75. doi: 10.1111/tri.13802
43. Amin KR, Stone JP, Kerr J, Geraghty A, Joseph L, Montero-Fernandez A, et al. Randomized preclinical study of machine perfusion in vascularized composite allografts. Br J Surg. (2021) 108(5):574–82. doi: 10.1002/bjs.11921
44. Said SA, Ordeñana CX, Rezaei M, Figueroa BA, Dasarathy S, Brunengraber H, et al. Ex-vivo normothermic limb perfusion with a hemoglobin-based oxygen carrier perfusate. Mil Med. (2020) 185(Suppl 1):110–20. doi: 10.1093/milmed/usz314
45. Haug V, Kollar B, Tasigiorgos S, Endo Y, Kauke M, Safi AF, et al. Hypothermic ex situ perfusion of human limbs with acellular solution for 24 hours. Transplantation. (2020) 104(9):e260–70. doi: 10.1097/TP.0000000000003221
46. Haug V, Kollar B, Endo Y, Kadakia N, Veeramani A, Kauke M, et al. Comparison of acellular solutions for ex-situ perfusion of amputated limbs. Mil Med. (2020) 185(11–12):e2004–12. doi: 10.1093/milmed/usaa160
47. Fahradyan V, Said SAD, Ordenana C, Dalla Pozza E, Frautschi R, Duraes EFR, et al. Extended ex vivo normothermic perfusion for preservation of vascularized composite allografts. Artif Organs. (2020) 44(8):846–55. doi: 10.1111/aor.13678
48. Gok E, Alghanem F, Moon R, Guy E, Rojas-Pena A, Bartlett RH, et al. Development of an ex-situ limb perfusion system for a rodent model. ASAIO J. (2019) 65(2):167–72. doi: 10.1097/MAT.0000000000000786
49. Gok E, Kubiak CA, Guy E, Ponder M, Hoenerhoff MJ, Rojas-Pena A, et al. Long-term effects of hypothermic ex situ perfusion on skeletal muscle metabolism, structure, and force generation after transplantation. Transplantation. (2019) 103(10):2105–12. doi: 10.1097/TP.0000000000002800
50. Krezdorn N, Macleod F, Tasigiorgos S, Turk M DM, Wo L, Kiwanuka B AH, et al. Twenty-four-hour ex vivo perfusion with acellular solution enables successful replantation of porcine forelimbs. Plast Reconstr Surg. (2019) 144(4):608e–18e. doi: 10.1097/PRS.0000000000006084
51. Kueckelhaus M, Dermietzel A, Alhefzi M, Aycart MA, Fischer S, Krezdorn N, et al. Acellular hypothermic extracorporeal perfusion extends allowable ischemia time in a porcine whole limb replantation model. Plast Reconstr Surg. (2017) 139(4):922e–32e. doi: 10.1097/PRS.0000000000003208
52. Duraes EFR, Madajka M, Frautschi R, Soliman B, Cakmakoglu C, Barnett A, et al. Developing a protocol for normothermic ex-situ limb perfusion. Microsurgery. (2018) 38(2):185–94. doi: 10.1002/micr.30252
53. Werner NL, Alghanem F, Rakestraw SL, Sarver DC, Nicely B, Pietroski RE, et al. Ex situ perfusion of human limb allografts for 24 hours. Transplantation. (2017) 101(3):e68–74. doi: 10.1097/TP.0000000000001500
54. Kueckelhaus M, Fischer S, Sisk G, Kiwanuka H, Bueno EM, Dermietzel A, et al. A mobile extracorporeal extremity salvage system for replantation and transplantation. Ann Plast Surg. (2016) 76(3):355–60. doi: 10.1097/SAP.0000000000000681
55. Ozer K, Rojas-pena A, Mendias CL, Bryner BS, Toomasian C, Bartlett RH. The effect of ex situ perfusion in a swine limb. J Hand Surg. (2016) 41(1):3–12. doi: 10.1016/j.jhsa.2015.11.003
56. Ozer K, Rojas-Pena A, Mendias CL, Bryner B, Toomasian C, Bartlett RH. Ex situ limb perfusion system to extend vascularized composite tissue allograft survival in swine. Transplantation. (2015) 99(10):2095–101. doi: 10.1097/TP.0000000000000756
57. Müller S, Constantinescu MA, Kiermeir DM, Gajanayake T, Bongoni AK, Vollbach FH, et al. Ischemia/reperfusion injury of porcine limbs after extracorporeal perfusion. J Surg Res. (2013) 181(1):170–82. doi: 10.1016/j.jss.2012.05.088
58. Constantinescu MA, Knall E, Xu X, Kiermeir DM, Jenni H, Gygax E, et al. Preservation of amputated extremities by extracorporeal blood perfusion; a feasibility study in a porcine model. J Surg Res. (2011) 171(1):291–9. doi: 10.1016/j.jss.2010.01.040
59. Tsuchida T, Kato T, Yamaga M, Ikebe K, Oniki Y, Irie H, et al. The effect of perfusion with UW solution on the skeletal muscle and vascular endothelial exocrine function in rat hindlimbs. J Surg Res. (2003) 110(1):266–71. doi: 10.1016/S0022-4804(02)00067-7
60. Tsuchida T, Kato T, Yamaga M, Ikebe K, Oniki Y, Irie H, et al. Effect of perfusion during ischemia on skeletal muscle. J Surg Res. (2001) 101(2):238–41. doi: 10.1006/jsre.2001.6278
61. Yabe Y, Ishiguro N, Shimizu T, Tamura Y, Wakabayashi T, Miura T. Morphologic and metabolic study of the effect of oxygenated perfluorochemical perfusion on amputated rabbit limbs. J Reconstr Microsurg. (1994) 10(3):185–91. doi: 10.1055/s-2007-1006586
62. Gordon L, Levinsohn DG, Borowsky CD, Manojlovic RD, Sessler DI, Weiner MW, et al. Improved preservation of skeletal muscle in amputated limbs using pulsatile hypothermic perfusion with university of Wisconsin solution. A preliminary study. J Bone Joint Surg Am. (1992) 74(9):1358–66. doi: 10.2106/00004623-199274090-00009
63. Domingo-Pech J, Garriga JM, Toran N, Rusinol M, Girvent F, Rosines D, et al. Preservation of the amputated canine hind limb by extracorporeal perfusion. Int Orthop. (1991) 15(4):289–91. doi: 10.1007/BF00186863
64. Brouwers K, Thijssen MF, Kruit AS, van Midden D, Koers EJ, Zegers HJH, et al. 24-hour perfusion of porcine myocutaneous flaps mitigates reperfusion injury: a 7-day follow-up study. Plast Reconstr Surg Glob Open. (2022) 10(2):e4123. doi: 10.1097/GOX.0000000000004123
65. Taeger CD, Friedrich O, Horch RE, Distler C, Kengelbach-Weigand A, Wenzel C, et al. Tissue viability of free flaps after extracorporeal perfusion using a modified hydroxyethyl starch solution. J Clin Med. (2020) 9(12):3929. doi: 10.3390/jcm9123929
66. Kruit AS, Smits L, Pouwels A, Schreinemachers MCJM, Hummelink SLM, Ulrich DJO. Ex-vivo perfusion as a successful strategy for reduction of ischemia-reperfusion injury in prolonged muscle flap preservation—a gene expression study. Gene. (2019) 701:89–97. doi: 10.1016/j.gene.2019.03.021
67. Taeger CD, Friedrich O, Drechsler C, Weigand A, Hobe F, Geppert CI, et al. Hydroxyethyl starch solution for extracorporeal tissue perfusion. Clin Hemorheol Microcirc. (2016) 64(1):91–103. doi: 10.3233/CH-162049
68. Taeger CD, Friedrich O, Dragu A, Weigand A, Hobe F, Drechsler C, et al. Assessing viability of extracorporeal preserved muscle transplants using external field stimulation: a novel tool to improve methods prolonging bridge-to-transplantation time. Sci Rep. (2015) 5:11956. doi: 10.1038/srep11956
69. Taeger CD, Müller-Seubert W, Horch RE, Präbst K, Münch F, Geppert CI, et al. Ischaemia-related cell damage in extracorporeal preserved tissue—new findings with a novel perfusion model. J Cell Mol Med. (2014) 18(5):885–94. doi: 10.1111/jcmm.12238
70. Dragu A, Taeger CD, Buchholz R, Sommerfeld B, Hübner H, Birkholz T, et al. Online oxygen measurements in ex vivo perfused muscle tissue in a porcine model using dynamic quenching methods. Arch Orthop Trauma Surg. (2012) 132(5):655–61. doi: 10.1007/s00402-011-1458-3
71. Dragu A, Kleinmann JA, Taeger CD, Birkholz T, Schmidt J, Geppert CI, et al. Immunohistochemical evaluation after ex vivo perfusion of rectus abdominis muscle flaps in a porcine model. Plast Reconstr Surg. (2012) 130(2):265e–73e. doi: 10.1097/PRS.0b013e3182589c2d
72. Dragu A, Birkholz T, Kleinmann JA, Schnürer S, Münch F, Cesnjevar R, et al. Extracorporeal perfusion of free muscle flaps in a porcine model using a miniaturized perfusion system. Arch Orthop Trauma Surg. (2011) 131(6):849–55. doi: 10.1007/s00402-010-1251-8
73. Jing L, Yao L, Zhao M, Peng LP, Liu M. Organ preservation: from the past to the future. Acta Pharmacol Sin. (2018) 39(5):845–57. doi: 10.1038/aps.2017.182
74. Karangwa SA, Dutkowski P, Fontes P, Friend PJ, Guarrera JV, Markmann JF, et al. Machine perfusion of donor livers for transplantation: a proposal for standardized nomenclature and reporting guidelines. Am J Transplant. (2016) 16(10):2932–42. doi: 10.1111/ajt.13843
76. Petrenko A, Carnevale M, Somov A, Osorio J, Rodríguez J, Guibert E, et al. Organ preservation into the 2020s: the era of dynamic intervention. Transfus Med Hemother. (2019) 46(3):151–72. doi: 10.1159/000499610
77. Refinetti R. Circadian rhythmicity of body temperature and metabolism. Temperature (Austin). (2020) 7(4):321–62. doi: 10.1080/23328940.2020.1743605
78. Christmas KM, Bassingthwaighte JB. Equations for O(2) and CO(2) solubilities in saline and plasma: combining temperature and density dependences. J Appl Physiol (1985). (2017) 122(5):1313–20. doi: 10.1152/japplphysiol.01124.2016
79. Cao M, Zhao Y, He H, Yue R, Pan L, Hu H, et al. New applications of HBOC-201: a 25-year review of the literature. Front Med (Lausanne). (2021) 8:794561. doi: 10.3389/fmed.2021.794561
80. Laing RW, Bhogal RH, Wallace L, Boteon Y, Neil DAH, Smith A, et al. The use of an acellular oxygen carrier in a human liver model of normothermic machine perfusion. Transplantation. (2017) 101(11):2746–56. doi: 10.1097/TP.0000000000001821
81. Jahr JS, Akha AS, Holtby RJ. Crosslinked, polymerized, and PEG-conjugated hemoglobin-based oxygen carriers: clinical safety and efficacy of recent and current products. Curr Drug Discov Technol. (2012) 9(3):158–65. doi: 10.2174/157016312802650742
82. Rostami S, Xu M, Datta S, Haykal S. Evaluation of early markers of ischemia-reperfusion injury and preservation solutions in a modified hindlimb model of vascularized composite allotransplantation. Transplant Direct. (2022) 8(1):e1251. doi: 10.1097/TXD.0000000000001251
83. Serifis N, Matheson R, Cloonan D, Rickert CG, Markmann JF, Coe TM. Machine perfusion of the liver: a review of clinical trials. Front Surg. (2021) 8:625394. doi: 10.3389/fsurg.2021.625394
84. Brüggenwirth IMA, de Meijer VE, Porte RJ, Martins PN. Viability criteria assessment during liver machine perfusion. Nat Biotechnol. (2020) 38(11):1260–2. doi: 10.1038/s41587-020-0720-z
85. Hamelink TL, Ogurlu B, de Beule J, Lantinga VA, Pool MBF, Venema LH, et al. Renal normothermic machine perfusion: the road toward clinical implementation of a promising pretransplant organ assessment tool. Transplantation. (2022) 106:268–79. doi: 10.1097/TP.0000000000003817
86. Reeb J, Cypel M. Ex vivo lung perfusion. Clin Transplant. (2016) 30:183–94. doi: 10.1111/ctr.12680
87. Milek D, Reed LT, Echternacht SR, Shanmugarajah K, Cetrulo CL, Lellouch AG, et al. A systematic review of the reported complications related to facial and upper extremity vascularized composite allotransplantation. J Surg Res. (2023) 281:164–75. doi: 10.1016/j.jss.2022.08.023
88. Mukund K, Subramaniam S. Skeletal muscle: a review of molecular structure and function, in health and disease. Wiley Interdiscip Rev Syst Biol Med. (2020) 12:1462. doi: 10.1002/wsbm.1462
89. Brooks GA. Lactate as a fulcrum of metabolism. Redox Biol. (2020) 35:101454. doi: 10.1016/j.redox.2020.101454
Keywords: ex-vivo perfusion, ex-vivo limb perfusion, vascularized composite allograft (VCA), ischemia reperfusion (I/R) injury, hand transplantation
Citation: Duru Ç, Biniazan F, Hadzimustafic N, D'Elia A, Shamoun V and Haykal S (2023) Review of machine perfusion studies in vascularized composite allotransplant preservation. Front. Transplant. 2:1323387. doi: 10.3389/frtra.2023.1323387
Received: 17 October 2023; Accepted: 4 December 2023;
Published: 20 December 2023.
Edited by:
Kagan Ozer, University of Cincinnati, United StatesReviewed by:
Theresa Hautz, Innsbruck Medical University, Austria© 2023 Duru, Biniazan, Hadzimustafic, D'Elia, Shamoun and Haykal. This is an open-access article distributed under the terms of the Creative Commons Attribution License (CC BY). The use, distribution or reproduction in other forums is permitted, provided the original author(s) and the copyright owner(s) are credited and that the original publication in this journal is cited, in accordance with accepted academic practice. No use, distribution or reproduction is permitted which does not comply with these terms.
*Correspondence: Siba Haykal c2liYS5oYXlrYWxAdWhuLmNh
Disclaimer: All claims expressed in this article are solely those of the authors and do not necessarily represent those of their affiliated organizations, or those of the publisher, the editors and the reviewers. Any product that may be evaluated in this article or claim that may be made by its manufacturer is not guaranteed or endorsed by the publisher.
Research integrity at Frontiers
Learn more about the work of our research integrity team to safeguard the quality of each article we publish.