- Clem Jones Centre for Regenerative Medicine, Bond University, Gold Coast, QL, Australia
Stem cell therapies can potentially treat various retinal degenerative diseases, including age-related macular degeneration (AMD) and inherited retinal diseases like retinitis pigmentosa. For these diseases, transplanted cells may include stem cell-derived retinal pigmented epithelial (RPE) cells, photoreceptors, or a combination of both. Although stem cell-derived RPE cells have progressed to human clinical trials, therapies using photoreceptors and other retinal cell types are lagging. In this review, we discuss the potential use of human pluripotent stem cell (hPSC)-derived photoreceptors for the treatment of retinal degeneration and highlight the progress and challenges for their efficient production and clinical application in regenerative medicine.
1. Introduction
The global burden of vision loss is significant, with an estimated 61 million people predicted to be blind and another 474 million people experiencing moderate to severe vision impairment by 2050 (1). The root cause of irreparable vision loss is often the degeneration of photoreceptor cells in the retina, which can occur as a primary or secondary disease process. Therefore, developing effective therapeutic approaches for retinal degenerative diseases is crucial in the fight against vision loss.
The retina is a layer of nervous tissue in the eye responsible for vision. The outer nuclear layer of the human retina contains light-sensitive cells called photoreceptors that convert light signals into electrical impulses (2). The inner nuclear retinal layer consists of bipolar, horizontal and amacrine cells that receive and modulate visual information before synapsing with the ganglion cell layer to transmit electrical impulses sent to the brain for visual processing (3).
The human retina contains approximately 110 million rods and 4.6 million cones (4). Rods account for 95% of photoreceptor neurons and are concentrated in the peripheral retina, largely absent in the fovea. They are specialized cells that provide scotopic vision, having low contrast sensitivity and acuity. Rods are extremely sensitive to a single photon of light and can become light-saturated in bright light (5).
Cones constitute 5% of photoreceptors in humans. The macula is a small region of the retina that contains the highest density of cones, resulting in high visual acuity. Humans and some primates have three cone subtypes (blue, green, red) for trichromatic vision (6). Unlike rods, cones are not light-saturated and can recover their membrane current from photobleaching within milliseconds (7).
The health and function of photoreceptors are carefully maintained within the retinal ecosystem. Retinal pigment epithelial (RPE) cells interdigitate with photoreceptors and perform critical functions, such as the phagocytosis of photoreceptor outer segments, recycling of opsins (visual cycle), barrier function, nutrient supply, waste removal, and cytokine secretion to preserve retinal function (8). Müller glial cells of the retina provide structural support and help balance neurotransmitters, trophic factors, and metabolites (9). Cones also depend on rods for their survival through the regulation of glucose uptake (10). Disturbance of the retinal ecosystem can cascade toward retinal degeneration when cell functions are compromised.
Age-related macular degeneration (AMD) is a leading cause of blindness in elderly individuals in Western nations, affecting approximately 200 million people worldwide (11, 12). AMD begins as yellowish deposits called drusen in the retina of the macula and can progress to either dry AMD (geographic atrophy) or wet AMD (choroidal neovascularization). Late-stage AMD leads to severe vision impairment and irreversible loss of central vision due to the death or dysfunction of the RPE in the macula, followed by secondary loss of cone photoreceptors (13). Multiple contributing factors to AMD, including age, light exposure, genetics and lifestyle, lead to dysregulation of metabolic, redox and complement systems (14). While current treatments can slow the progression of AMD, approximately 15% of patients will ultimately lose their central vision (15) (Figure 1).
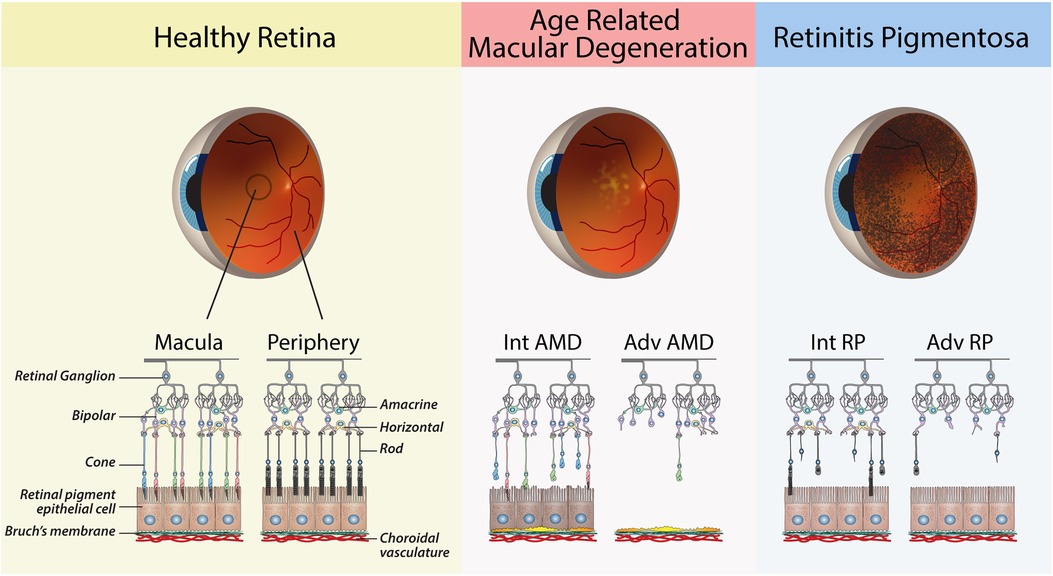
Figure 1. Schematic diagrams of retinas from healthy and diseased eyes. The healthy retina shows the major cells of the retina, with a clear distinction between the photoreceptors of the macula vs. the peripheral retina. Age-related Macular Degeneration: Retinal schematic of the macula, as shown by the presence of cone photoreceptors. Intermediate (Int) AMD—The presence of sub-RPE drusen deposits and early signs of RPE dysfunction. Advanced (Adv) AMD—loss of RPE cells and cones in the macula. Retinitis Pigmentosa: Retinal schematic showing the progressive degeneration of rods in the peripheral retina. Intermediate (Int) RP—partial loss of rod. Advanced (Adv) RP—widespread loss of rods in the peripheral retina.
Inherited retinal diseases (IRDs) are a group of genetic disorders that result in blindness due to the primary death or dysfunction of photoreceptors. There are over 300 known mutations that can cause IRDs, resulting in conditions such as retinitis pigmentosa (RP), Stargardt disease (SD), cone-rod dystrophy (COD) and Leber congenital amaurosis (LCA) (16). RP is the most common type of inherited retinal disease, affecting approximately 1 in 5,000 births, and typically causes progressive vision loss beginning in the third or fourth decade of life due to the loss of rod photoreceptors in the peripheral retina (17) (Figure 1). This leads to night blindness and narrowing of vision, and ultimately complete blindness due to secondary loss of cones (18).
Current treatment options for AMD and IRDs are limited and primarily focused on slowing the progression of degeneration (19, 20). The standard treatment for neovascular AMD is anti-VEGF therapy to reduce abnormal blood vessel growth and leakage (21). In early 2023 the FDA approved Pegcetacoplan (marketed as Syfovre) as the first treatment for geographic atrophy (GA). Syfovre works by inhibiting complement factor 3 (22) and can reduce the growth of geographic atrophic regions by up to 30% compared to controls (23, 24). In addition to limited efficacy, patients with dry AMD are at increased risk of developing wet AMD (25).
Several alternative therapeutic approaches to target early and intermediate-stage retinal degeneration, including gene therapies to restore normal cell function, neuroprotection to support cell survival and reduce inflammatory signalling, pharmacotherapies to compensate for metabolic and biochemical imbalances (26), exosome therapies to deliver packages of mRNAs, miRNAs, proteins to augment gene expression and cell function (27) and optogenetics to confer light sensitivity on other retinal cells (28). Luxturna is the first and only FDA-approved gene therapy for the treatment of an IRD (LCA), however, other disease targets are under development, including AMD and RP using gene-specific and gene-indifferent approaches (16, 29, 30). The long-term efficacy of these therapies is yet to be determined in patients.
Human photoreceptors do not have the capacity to regenerate, and there are currently no treatments to restore vision once they are lost. Cell replacement is a promising approach to treat various types of retinal degeneration. Human pluripotent stem cells offer the potential for unlimited amounts of transplantable retinal cells and photoreceptors. This review discusses progress and challenges in the efficient production of photoreceptors from human pluripotent stem cells and their clinical translation.
1.1 Cell transplantation for retinal degenerative diseases
The purpose and goal of cell therapy for retinal diseases is to restore or improve vision in individuals who suffer from various retinal disorders. In the early stages of age-related macular degeneration (AMD), cell therapies aim to preserve cone photoreceptors by transplanting retinal pigment epithelial (RPE) cells (31). In advanced stages of AMD, transplantation of both RPE and cones may be required to restore vision, while other retinal degenerative diseases may require the transplantation of rods and/or cones.
The possibility of photoreceptor integration depends on the structural and functional preservation of the remaining retina, particularly the ONL and retinal ganglia (32, 33) to process and transmit visual information to the brain (34). To achieve retinal integration, donor cells are transplanted into the subretinal space above the RPE layer and must then migrate and extend processes towards the host ONL while overcoming the barriers of the outer limiting membrane (OLM) (35). Subsequently, photoreceptors must mature and function normally through interactions within the surrounding retinal environment.
The retina is an ideal anatomical location for cell transplantation as it is relatively simple to access and monitor and requires relatively few cells to achieve a therapeutic effect (36). Furthermore, the eye is an immune-privileged site with a reduced risk of graft rejection (37). Graft function can be assessed using several non-invasive techniques, such as optical coherence tomography, fundus autofluorescence, fluorescein angiography and visual field tests (38, 39). In the past four decades, the potential for cell therapies to treat various retinal conditions has spurred the development of surgical techniques and has led to numerous clinical trials (40–45).
The transplantation of cells into the retina to treat degenerative diseases has been pursued since the early 1980s. Proof-of-concept was first established in owl monkeys by Peter Gouras and others, who subsequently published a series of studies demonstrating photoreceptor rescue by RPE transplantation in rodents (46). Photoreceptor transplantation was pioneered using retinal microaggregates and enriched photoreceptors in rodents and pigs (47–51). Studies focused on the fundamentals of surgery, preparation of cells and tissues for grafting, and labelling methods to track grafted cells in vivo. The feasibility and short-term efficacy of retinal tissue grafting were tested by transplanting retinal clumps from newborn rats into the subretinal space of adult rats with outer retinal lesions (48, 52–55). Studies in wild-type and degenerative model rodents demonstrated graft survival for up to nine months, and preliminary evidence of integration, suggested by the formation of primitive outer segments and synapses (48, 56, 57), and in some cases demonstrated observable behavioral changes such as light avoidance in rodents (58).
Transplantation of retinal tissues and cells of various developmental ages suggested that younger cells provided better outcomes than more developed cells (59). In 2006, Maclaren determined that the optimal developmental age of photoreceptors in mice was post-mitotic precursors, derived from postnatal days 3 and 7 in mice (60). This preference may be attributed to the enhanced resilience exhibited by these precursors relative to fully matured cells, characterized by elongated and delicate structures that may render them vulnerable to dissociation techniques. Additionally, precursors may be better suited to migrate from the subretinal space to establish synaptic connections with the ONL.
Preclinical studies in large animal studies showed subretinally transplanted retinal sheets survived and improved cone responses, despite a lack of evidence of retinal integration (51, 61). However, several human trials conducted between the late 1990s and early 2000s using fetal retinal sheets and cells resulted in limited or transient visual benefit in RP patients (62–65).
Despite their lack of clinical efficacy, these pioneering studies demonstrated that photoreceptor replacement could potentially be developed into a therapy for retinal degenerative diseases by overcoming the obstacles of survival and retinal integration, as well as a reliable source of cells.
1.2. Human pluripotent stem cells in cell therapy
The isolation of human embryonic stem cells (hESCs) in 1998 (66) and the subsequent generation of human induced pluripotent stem cells (hIPSCs) by somatic cell reprogramming in 2007 (67, 68) opened a multitude of opportunities for regenerative medicine.
The critical properties of hESCs and hIPSCs (together, hPSCs) include pluripotent differentiation potential (the ability to form any cell type of the body) and self-renewal (maintenance of an undifferentiated state during mitosis), making them an ideal and possibly limitless source of cells for therapeutic purposes. Unlike hESCs, hIPSCs do not require human embryos and can be generated by reprogramming somatic cells. Reprogramming allows for the generation of hIPSCs with patient-specific genetics for personalized medicine and disease modelling and avoids the ethical concerns associated with human embryo research (69).
One of the most promising applications of hPSCs is cell replacement therapies for retinal degenerative diseases. The earliest completed human trials to use hPSC-derived cell products were hESC and hIPSC-derived RPE cells in AMD patients (70, 71). Over the past decade, hPSC-RPE transplantation accounted more human trials than any other hPSC-derived product (22/102) (72). Moreover, the first clinical trial for RP using hIPSC-derived retinal organoid sheets (jRCTa050200020) recently began in Japan (73).
Most clinical trials for AMD have generated hPSC-RPE by spontaneous differentiation (70, 74–76). This approach is inefficient, time-consuming and highly variable across cell lines (77). Furthermore, hPSC-RPE cell stocks must be expanded to generate sufficient cell numbers, leading to reduced cell function and increased risk of mutations (78). By contrast, the directed differentiation of hPSC-RPE cells using cytokines and small molecules results in higher efficiencies and final yields (79–81). Some groups have refined their protocols to produce hPSC-RPE under current good manufacturing practice (cGMP) conditions for human trials (70, 75, 82–84), and commercial approval, though not yet granted, is anticipated (44).
Although photoreceptors and RPE cells are derived from the same progenitor cell type, photoreceptors are more challenging to generate efficiently and are post-mitotic. These two factors make producing large numbers of cells difficult for clinical use. It is, therefore, critical that efficient methods for the production and harvest of photoreceptors can be developed for therapeutic applications (85).
1.3. Retinal development in vivo
Developing differentiation protocols for specific retinal cell types requires knowledge of retinal development in vivo. While the key drivers of early retinal development are known, the extrinsic factors that drive the development of specific neuronal cell subtypes are not yet fully understood.
Ontologically, the retina is part of the brain and follows early forebrain development in vivo. During mammalian embryogenesis, this is achieved by dual SMAD inhibition of TGFβ and BMP signalling pathways, as well as inhibition of WNT signalling along an anterior-posterior gradient that defines this axis, resulting in the anterior neurectoderm (Figure 2) (86, 87). hPSCs can be directed to the same fate using small molecules or cytokine inhibition of the same pathways (81, 88, 89). Eyefield progenitor cells (EFPC) are multipotent and have the potential to form the RPE, neural retina and associated progeny cells, including photoreceptors. Under appropriate conditions, EFPCs can be generated with nearly 100% efficiency, as determined by the expression of specific eyefield markers like RAX, LHX2 and PAX6 (90). The efficient formation of hPSC-derived EFPCs in vitro is thus a critical platform for producing the RPE or neural retinal progenitor cells.
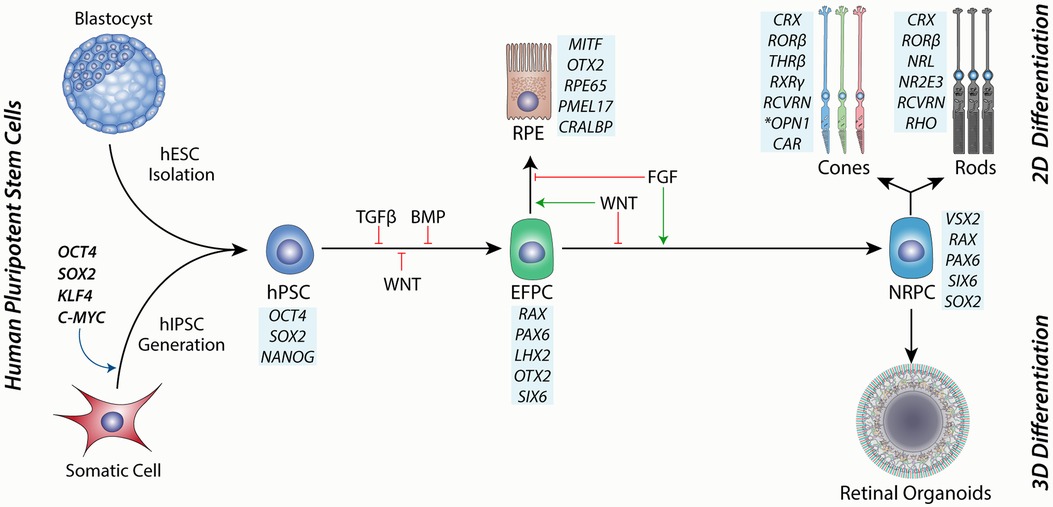
Figure 2. A schematic showing the source of human pluripotent stem cells from either a blastocyst-stage embryo or through hIPSC cell induction by reprogramming factors. hPSCs are then directed toward the eye field progenitor cells (EFPCs) by inhibition of TGFβ, BMP and WNT signalling Progression of differentiation to the RPE is governed by WNT signalling and is enhanced through inhibition of FGF signalling. Conversely, the differentiation of EFPCs towards neural retinal progenitor cells (NRPCs) is driven by FGF signalling and inhibition of WNT signalling. NRPCs have the differentiation potential to form all cells of the neural retina and can be cultured in suspension as 3D aggregates to form retinal organoids or under 2D conditions under directed differentiation towards rod or cone photoreceptors. Blue boxes show markers for each cell type.
Bifurcation of the eyefield to the RPE or neural retina is governed by the expression of MITF and VSX2, respectively (91). Extrinsic factors that regulate this process include FGF and WNT signalling (92). Support for this model can be observed in vitro and in vivo, where FGF signalling is required for the development of the neural retina in the developing chick (93) and is sufficient for the transdifferentiation of the RPE to the neural retinal in vitro (94). This model complements studies showing that loss of WNT signalling during mouse development results in the transdifferentiation of the presumptive RPE to the neural retina (95) and is also supported by retinal differentiation studies using hPSCs (91). Thus, the directed differentiation of EFPCs to neural retinal progenitor cells (NRPCs) has the potential to yield photoreceptors with high efficiency in vitro.
1.4. Towards photoreceptor production
Early studies on the directed differentiation of mouse embryonic stem cells (mESCs) and hPSCs towards retinal cells used a combination of 3D/2D hybrid protocols to achieve retinal differentiation and, in some cases, showed the production of photoreceptors after extended culture periods. In 2005, a landmark study demonstrated the generation of retinal precursors by culturing mESCs as embryoid bodies in serum-free conditions in the presence of Dkk1 and LeftyA, followed by the addition of serum and Activin, resulting in about 6% Rx+/Pax6+ cells (96). Overexpression of Crx in these cells through lentiviral transduction, or co-culture with retinal explants, resulted in the upregulation of rhodopsin (Rho) and recoverin (Rcvrn).
Shortly after, Lamba et al. (97) demonstrated retinal progenitor differentiation by culturing hESCs as embryoid bodies in serum-free media (SFEB) with IGF-1, DKK1 and Noggin. After three weeks, cells expressed PAX6 and VSX2 (CHX10) in approximately 80% of cells (97). In 2008, Osakada et al. adapted and refined SFEB differentiation in mouse, monkey and human ESCs using retinoic acid and taurine to enhance rod production by day 130 (98). This study also marked the first-time photoreceptor progenitors were generated in the absence of mature retinal tissue, with photoreceptors representing about 20% of cultured cells.
Lamba et al. (99) also demonstrated the differentiation of multiple hESC and hIPSC lines towards retinal progenitors through the suspension culture of aggregates in DKK-1, IGF-1 and Noggin. These cultures showed patches of retinal pigment epithelial, amacrine and neural ganglion cells. After two months of culture, photoreceptor markers, including Crx and Otx2, were detected in about 10% of cells. Approximately 30% of the photoreceptors produced were rods, as indicated by the expression of Nrl, and about 1% of cells expressed more mature photoreceptor markers such as recoverin, AIPL-1, Rho and S-Opsin (99).
By contrast, Meyer et al. (100) induced retinal differentiation using a 3D/2D/3D protocol by culturing hIPSCs without specific factors. For this, hIPSCs were cultured in a neural induction medium (N2 and heparin) from day 2 to day 16, followed by a retinal induction medium (B27) thereafter. Floating aggregates were plated to form neural rosettes, which were lifted to form neurospheres and, over time, proceeded towards photoreceptors via a neural retinal intermediate stage. Despite the absence of specific factors, some cells expressed PAX6 and RAX at day 10, CHX10 by day 40, followed by cells expressing CRX, RCVRN and OPSIN by day 80 (100).
These early studies demonstrated the potential for generating retinal tissue from hPSCs by directed (guided) or unguided methods and laid the foundations for new methods to generate retinal cells and photoreceptors.
1.5. Retinal organoid differentiation
The Sasai group advanced retinal differentiation through the generation of 3D self-folding optic cups from mouse (101) and human ESCs (102), leading to the field of retinal organoid research.
Retinal organoids are 3D multi-layered structures derived from hPSCs that can faithfully recapitulate key elements of retinal development, disease and function in the dish (103). Mature retinal organoids contain photoreceptors, bipolar, horizontal, amacrine, retinal muller and ganglion cells and exhibit aspects of native connectivity, apical and basal polarity, cellular architecture, and metabolic interactions (104) and, in some cases, are light responsive (105).
Retinal organoids have since emerged as valuable tools for the investigation of retinal development (106) and diseases in vitro (107), therapeutic drug screening (108), exploration of gene therapies (109), and evaluation of neuroprotective agents (110). Furthermore, retinal organoid technologies have reached human trials to treat RP (111).
Over the past decade, many research groups have adapted and explored new protocols for retinal organoid generation. Here, we discuss the common features of retinal organoid protocols and highlight fundamental research that has improved organoid development's robustness and quality and increased photoreceptor yields and quality.
Retinal organoid protocols generally share features of neural induction, isolation or selection of developing neural retinal tissue, followed by culture to support retinal development. Neural induction can be performed using three-dimensional (3D) (112) or two-dimensional methods (2D) (113) (Figure 3A) and may be directed (guided) using specific signalling factors or unguided (spontaneous) (100, 114, 115), which is consistent with the default model of development (116). While unguided protocols may be sufficient for some cell lines, inherent differences between lines, such as epigenetics (117, 118), may affect retinal differentiation (99, 119, 120).
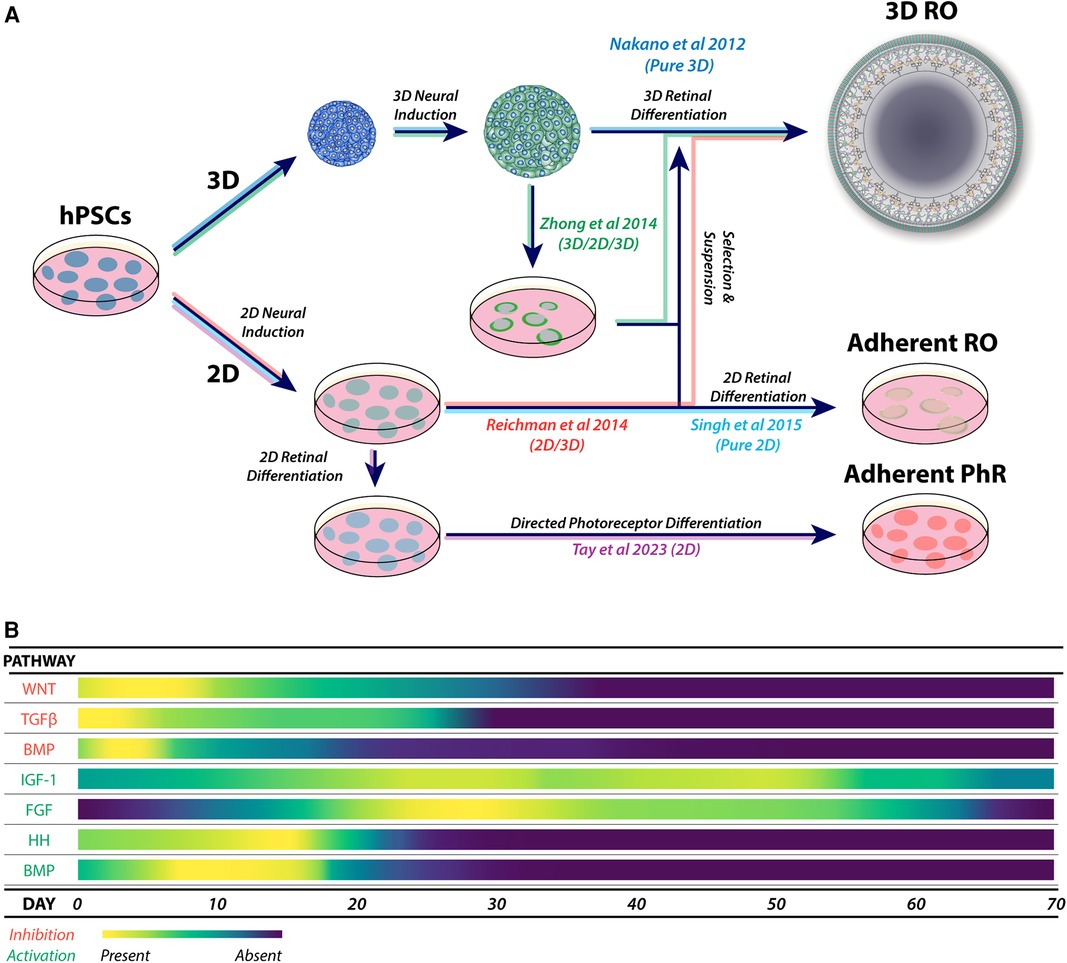
Figure 3. (A) Major variations in the physical formats for retinal organoid and photoreceptor differentiation, showing combinations of 3D, 2D/3D, 3D/2D/3D and 2D culture systems. These approaches form the basis of derived and adapted protocols for the generation of retinal organoids and enhanced photoreceptor differentiation. (B) A heat map of the most utilized pathways during guided neural induction and early-to-mid retinal differentiation. Pathway activation or inhibition is commonly achieved using small molecules and exogenous cytokines. Adaptations of protocols are commonly explored by using additional specific molecules to enhance or promote target cell types, including photoreceptors.
Guided protocols provide a form of controlled neural induction that may override the intrinsic bias of hPSC lines (121). Guided neural induction typically involves inhibiting the TGFβ, BMP (122–126) and WNT signalling pathways to enhance anterior neural differentiation (123, 127–129) (Figure 3B). Recently, nicotinamide was shown to enhance neural induction through BMP inhibition (130) and possibly also through inhibition of the WNT pathway (131).
Retinal differentiation may be enhanced by promoting neural retinal differentiation and development and inhibiting RPE differentiation. A common approach is stimulation of the FGF pathway using FGF2 (132–133) or FGF9 (94, 134, 135). Other approaches include the direct inhibition of WNT signalling with small molecules or factors (102, 125, 134, 136).
Supplementation of retinal organoid cultures with IGF-1 promotes optic vesicle and optic cup formation, retinal lamination and the appearance of photoreceptors (112, 137). Various protocols employ BMP4 soon after neural induction to enhance retinal differentiation (138–140). However, the effects of IGF-1 and BMP4 on retinal organoid differentiation may be protocol and cell line specific (141). Despite these observations, retinal induction with BMP4 is reproducible across multiple hPSC lines (73, 139, 142, 143).
Amongst the myriad of retinal organoid protocols, a unique approach to neural retinal development by Kuwahara et al. (138) used the directed differentiation of hPSCs to RPE cells followed by induction reversal to the form highly organized organoids with a stratified neural retina that were rich in photoreceptors (138). This protocol has been further optimized by preconditioning hPSCs through SHH activation and TGFβ inhibition in stem cell maintenance medium for 24 h (142).
1.6. Photoreceptors from retinal organoids
Strategies for promoting photoreceptor differentiation in retinal organoids vary from improving overall organoid quality, increasing photoreceptor yields, biasing photoreceptor sub-types and improving final photoreceptor maturity and function.
Notch inhibition causes retinal progenitors to exit the cell cycle, which triggers the early appearance of photoreceptors and increases yields (127, 129, 133). While most studies have used DAPT to inhibit Notch signalling, one study demonstrated that Notch inhibition for three days using PF-03084014, but not DAPT, at different time points (day 45 or day 60 and day 90) promoted differentiation of cones and rods, respectively (144). However, neither cone nor rod photoreceptors did not express mature markers, even after prolonged culture. However, Zerti et al. (145) found that exposure of retinal organoids to DAPT from days 28–42 and retinoic acid (RA) from day 30 to day 120 significantly increased M/l cone differentiation at the expense of rods (145).
Although RA is commonly used to enhance photoreceptor development (114, 127, 146, 147), the timing and concentration of RA can affect the timing of photoreceptors, subtype bias, and maturation (145, 148, 149). Moreover, the type of retinoid can also affect photoreceptor differentiation. Zerti et al. (145) found that RA enhanced expression of mature rod and cone markers, while 9-cis-retinal and 11-cis-retinal reduced expression below control values. Conversely, the Swaroop lab found 9-cis-retinal accelerated rod differentiation (150, 151).
COCO simultaneously inhibits the TGFβ, BMP and WNT (152) signalling pathways and enhances cone photoreceptor differentiation. In an adapted protocol, Pan et al. (125) showed that COCO significantly increased photoreceptor precursors by day 45 but not final photoreceptor yields. However, COCO increased the ratio of cones vs. rods by day 90 (125).
Other studies have used thyroid hormone to bias photoreceptor sub-types. Thyroid hormone determines the development of rod vs. cone subtypes when used in a temporal and concentration-dependent manner and causes a shift away from S-Opsin cones towards M/l-Opsin cones (128, 153, 154). Finally, somatostatin signalling was recently found to promote rod differentiation and maturation (155).
In addition to culture media and signalling molecules, physical conditions can enhance photoreceptor development in organoids. For example, Völkner et al. (156) utilized a combination of hypoxic culture and tri-sectioning, which led to the development of stratified retinal organoids (ROs) expressing markers related to phototransduction and synaptic vesicle proteins, along with functional responses to light (156). Furthermore, improved RO development can be achieved by cultivating them on plate shakers or in spinner flasks (157) and using rotating vessels (158). These approaches facilitate the efficient exchange of oxygen and nutrients, enhancing retinal development.
Overall, the discovery of retinal organoids has led to various methods to create retinal tissues containing photoreceptors of different stages of development, with increasing control over rod and cone differentiation and higher yields that will hasten their clinical translation.
1.7. Directed differentiation of photoreceptors
Whereas retinal organoids are intended to recapitulate complexity of the developing retina, directed differentiation protocols aim to generate photoreceptor precursors with high efficiency and may be useful for large-scale production.
Following classical dual SMAD and WNT inhibition using COCO in 3D/2D culture, Zhou et al. (88) generated photoreceptor precursors for 21 days at high efficiency. Neural induction was performed using EBs by using COCO, FGF2 and IGF-1, which were plated onto Matrigel and cultured for a further four weeks, resulting in approximately 90% CRX+ and 40% S-Opsin+ cells by day 21. The addition of thyroid hormone shifted cone identity from S-Opsin to M/l-Opsin cones (88). The remarkable ability of COCO to direct cone differentiation has since been tested in rat retinal progenitor cells (159) and human retinal organoids (125, 126).
Tay et al. (160) explored the effect of culture substrate on photoreceptor differentiation efficiencies (160). hESC monolayers were seeded onto LN521, LN323 + LN521 (2:1 ratio) or LN523 + LN521 (2:1 ratio) and cultured in neural induction medium (N2, B27, SB431542, and CKI-7) until day 9, followed by photoreceptor differentiation medium containing (N2, B27, BDNF, CNTF, RA, and DAPT) until day 32. By day 32, approximately 35% of cells on LN523 + LN521 co-expressed CRX and RCVRN, compared to -10% on LN323 + LN521 and >1% on LN521 alone. This is consistent with the presence of laminin γ3 in the interphotoreceptor matrix (161–163).
Direct conversion by transcription factor overexpression presents new ways to generate target cell types from hPSCs (164). This strategy generated photoreceptors from hPSCs by inducible expression of CRX and NEUROD1 under 2D and 3D (165). By day 28, RCVRN was expressed in approximately 34% and 44% of cells in 2D and 3D cultures, respectively. Moreover, RHO and CAR were elevated in 3D compared to 2D culture. While the future clinical application of genetically modified cells remains uncertain, this may be overcome by the direct conversion of hPSCs to photoreceptors using non-integrative techniques, such as mRNA transfection (166).
1.8. Co-culture methods
The optic cup stage of retinogenesis involves interactions between the nascent neural retinal layer and the RPE that promote their mutual development and maturation in vivo (167, 168). Co-culture systems partially recapitulate this by bringing retinal tissue or photoreceptors into proximity with the RPE, facilitating dynamic signalling between cells in vitro.
The RPE is a central source of factors involved in the development, maturation, function and maintenance of photoreceptors, including IGF-1, FGF, CNTF, TGFα, CNTF, PEDF, BDNF and HB-EGF (169). While RPE-conditioned medium can enhance retinal cell differentiation and survival (170–173), co-culture systems restore cell-to-cell contact to potentially enhance differentiation and maturation of retinal cells and the RPE (174–177).
Various combinations of RPE cells, retinal explant sheets, neurospheres, isolated cells, stem cell-derived retinal cells, retinal organoids and retinal cell lines are used in co-culture systems (178). Additionally, co-culture systems may combine cells from different species (179).
Basic co-culture systems typically use RPE monolayers on dishes or transwell systems layered with dissociated retinal cells, resulting in higher maturation than mono-culture controls (180). Studies using hPSC-derived RPE/neuroretinal co-cultures demonstrate enhanced photoreceptor differentiation. hESC-derived 3D neuroretinal progenitors cultured on primary rabbit RPE monolayers led to contact-dependent photoreceptor maturation (181). A proximity effect of RPE and retinal co-culture was shown using RPE cells and retinal progenitors derived from hESCs, resulting in photoreceptor differentiation near the RPE and upregulation of ganglion cell markers on the opposite side, as seen during retinal development (134). Similarly, hIPSC-derived retinal progenitor cells seeded on a scaffold (GCH-521: gelatin, chondroitin sulphate, hyaluronic acid and laminin 521) in contact with mature human fetal RPE monolayers developed a laminar structure with immature photoreceptors and immature inner nuclear layer, as well as increased RPE maturation (182). Subsequent research by the same group revealed that this co-culture system led to the deposition of drusen-like mounds by the RPE, offering a model of early AMD (183).
The co-culture of retinal organoids on RPE cells enables interaction between committed photoreceptor precursors rather than the nascent neural retina and can accelerate the upregulation of mature photoreceptor markers (184). Achberger et al. (178) co-cultured retinal organoids and RPE cells using a microfluidic device, generating mature photoreceptors and recapitulating native interactions, including outer-segment phagocytosis (178).
Thus, co-culture systems demonstrate the potential for RPE cells to improve retinal development and enhance photoreceptor differentiation by reconstructing the native configuration of the developing retina in vitro and may also improve therapeutic efficacy when co-transplanted (135, 185, 186).
2. Challenges for the clinical use of hPSC-derived photoreceptors
Photoreceptor therapies face several hurdles before reaching the clinic. One of the most important is the accurate determination and optimisation of structural integration into the retina. Even without integration, therapeutic benefit can occur through indirect mechanisms such as the release of supportive factors. Since results from preclinical studies support the clinical translation, the degree, contribution and duration of such mechanisms to therapeutic outcomes should be understood so they can potentially be overcome or exploited (187).
2.1. Cell tracking
Early studies labelled donor cell nuclei with triturated thymidine (57) or genetically modified β-galactosidase reporters (56). However, thymidine labelling does not provide structural information and can create false positives through host photoreceptor uptake of labelled DNA from dead donor cells (188), while β-galactosidase/Lac Z + can be taken up by host cells. It was, however, recognized that dual cytoplasmic and nuclear labelling might be required to interpret transplantation outcomes accurately.
The advent of fluorescent reporters gave rise to new methods for cell tracing and allowed for cell sorting prior to transplantation. Swaroop and MacLaren developed the NRL-GFP model in 2006 for rod transplantation studies (60, 189). Many groups have created photoreceptor reporter lines or used viral delivery to identify and isolate photoreceptors by FACS and follow cell survival and integration in transplanted animals. Reporter lines have also enabled visualization of photoreceptors during in vitro differentiation, commonly using CRX, RCVRN, Opsin (102, 190–197),. Alternatively, cell cultures can be transduced with viral reporters to label and isolate specific cells and track them post-transplantation (191, 198–201).
Several groups have transplanted fluorescently labelled photoreceptors into animal models and reported integration based on its co-localisation with synaptic markers or presence in the ONL (99, 153, 194, 198, 200, 202–208). While photoreceptor integration rates are typically below 1%, some studies have also shown significant improvements retinal electrophysiology and animal behaviour (61, 73, 200, 203, 207, 209–211), suggesting the possibility of therapeutic benefit.
2.2. Cytoplasmic transfer
Prior evidence for photoreceptor integration was called into question in 2016 and 2017 after a series of studies showed that male GFP+ photoreceptors transplanted into female DsRed mice resulted in a high proportion of cells that were GFP+/DsRed+, suggesting that donor and host photoreceptors shared cytoplasmic contents (190, 212–214). Further analysis showed that cytoplasmic exchange was bidirectional between host and donor cells and mediated through direct contact. However, cell fusion was ruled out by the absence of binuclear cells or fused membranes. The uptake of GFP from the extracellular space was also ruled out by the injection of GFP into the subretinal space (212). Subsequent studies confirmed that material exchange between photoreceptors occurred in vitro and in vivo post-transplantation via photoreceptor nanotubes rather than EV release (215, 216). Exchanged material between donor and host photoreceptors included miRNA, mRNA, proteins and mitochondria.
The Pearson group demonstrated that rates of donor cell integration and cytoplasmic transfer were affected by donor age and the host background using GFP+ mouse cone precursors from male mice or mESC-derived retinal organoids (217). Male GFP+ cone precursors were transplanted into adult wild-type (WT) and retinal degenerative backgrounds expressing dsRed. Consistent with previous studies, they showed that the developmental stage of donor cells affected integration into wild-type retinas. Specifically, GFP+ cone precursors harvested at E15 integrated less effectively than their counterparts from P1, P8 and mESC-derived ROs. The apparent integration rates of photoreceptors varied significantly across genetic backgrounds, with very few GFP+ cells found in the WT retina and almost 10-fold higher numbers in the NRL-/- retina, compared with other models, suggesting a role of the host's outer limiting membrane (OLM) on transplantation outcomes. Using FACS and fluorescent in situ hybridization (FISH), they found that up to 99% of GFP+ cells in the WT retinas were of host origin, compared to approximately 75% in NRL-/- retinas. Taken together, these experiments showed that rates of integration and cytoplasmic exchange can co-occur and are directly affected by the developmental age of donor cells and the host retinal environment, particularly the integrity of the OLM. Finally, cytoplasmic exchange between photoreceptors via nanotubes has been shown to occur naturally in the adult mouse retina and may be a natural homeostatic process (215, 218).
Cytoplasmic exchange via nanotubes between transplanted human and host animals remains underexplored. However, tunneling nanotubes have been known to mediate transfer between various human cells in vitro (219). Moreover, such exchanges have also been observed between transplanted hPSC-derived MSCs into mouse cells (220). Thus, it is possible that nanotube-mediated cytoplasmic exchange may also occur in pre-clinical studies of hPSC-photoreceptor transplantation.
The apparent setback of cytoplasmic exchange may also be an opportunity to develop new therapies, including mitochondrial delivery via cell transplantation (221, 222). By mapping the endogenous networks of mitochondrial transfer within the retina, stem cell-derived products could be utilized as mitochondrial delivery vectors to specific retinal cell types. Of particular interest will be rare diseases caused by mutations in the mitochondrial genome (mtDNA) (223). Another application is the delivery of engineered mitochondria (224, 225) to increase the survival of RPE, photoreceptors and other retinal cells with compromised capacity for redox homeostasis (226, 227).
2.3. Photoreceptor isolation
Photoreceptor isolation from heterogeneous cultures is challenging, especially for clinical applications. Currently, two main approaches are used for photoreceptor isolation: fluorescent reporter-based fluorescence-activated cell sorting (FACS) or antibody labelling followed by FACS or magnetic-activated cell sorting (MACS) (228, 229).
Fluorescent reporters, under photoreceptor-specific gene regulation, enable isolation from heterogeneous cultures, facilitating quantification of differentiation outcomes, characterization, animal transplantation, real-time survival monitoring, and retinal integration studies (102, 153, 192, 196, 197, 200, 202). However, their clinical use is limited due to potential risks from genetic manipulation and fluorophores' cytotoxic and immunogenic effects (230). Hence, it is crucial to isolate photoreceptors based on native characteristics, like surface markers.
The surface marker CD73 was initially considered a promising target for photoreceptor isolation (231). CD73+ selection of day 120 ROs resulted in approximately two-fold enrichment of CRX+/RCVRN+ photoreceptor precursors, capable of producing cones and rods in vitro and in vivo (229). However, different studies' attempts to isolate photoreceptors from ROs demonstrated vastly different results.
Lakowski et al. (232) found few CD73+ cells in day 100 ROs and human fetal retinas. Instead, CD73 was highly prominent in day 200 ROs and human adult retinas. Unexpectedly, CD73+ sorting of day 200 ROs resulted in an approximately six-fold depletion of CRX+/RCVRN+ photoreceptor precursors from the total RO cell pool, suggesting that CD73 is a poor marker of early photoreceptor differentiation dynamic changes in the co-expression of CD73, CRX and RCVRN during early development and poor cell type specificity (232). A similar study sorted day 150 ROs using RCVRN-eGFP reporter and found almost no detectable transcript for NT5E (gene coding for CD73) and poor CD73 co-localization with eGFP+ cells by immunofluorescence. Rather, eGFP expression was colocalized with rod and cone photoreceptor markers and CD133 (196).
In a concerted effort to establish a robust and reliable photoreceptor marker panel, Welby et al. (192) conducted a high throughput study of 242 markers on developing human foetal retinas transduced with a cone-GFP reporter. The screen identified several candidate markers but none that were unique to cones. Candidate markers were tested against cone-GFP transduced ROs (∼day 120), resulting in a sorting strategy (SSEA1-/CD26+/CD133+/CD147+) with approximately seven-fold enrichment. However, only 30% ± 15% of the isolated cells expressed cone markers (192).
These studies underscore the complexity of photoreceptor isolation using antibody-based methods, especially for clinical applications. In addition to exploring high-yielding marker panels, novel purification methods like label-free isolation techniques may also prove useful (233–235).
2.4. Further considerations for transplantation
The outer limiting membrane is a physical barrier to photoreceptor integration—studies using models of photoreceptors integrated at far higher efficiencies when coupled with OLM disruption. Pearson et al. (212) showed that approximately 23% of photoreceptors integrated into such models, compared with models with an intact OLM. This is consistent with previous research showing enhanced integration through transient chemical disruption of the OLM (236) or through genetic disruption (237). However, the severity of OLM disruption is also linked to disease progression, which may hinder integration due to retinal remodelling, glial scarring, and inflammation (239, 240). In the context of cell therapy, this would imply an optimal therapeutic window, or pre-treatment of the retina to limit retinal remodelling and gliosis.
Strategies for clinical therapies must reflect disease progression and the state of patient retinas. For instance, the transplantation of hPSC-RPE cells may be sufficient for AMD patients with viable cones; however, advanced stages of AMD will likely require co-transplantation of both hPSC-derived RPE cells and cones prior to retinal remodelling (238). On the other hand, low photoreceptor integration rates and widespread degeneration limit the potential of recovery of full-field vision rescue in RP patients. However, an appropriate strategy might be to transplant rods around the macula to protect cones and save central vision. This approach would not necessarily depend on the integration and maturation of rod cells for light detection but would instead rely on the neurotropic effect of rod-derived cone viability factor (RdCVF) to enhance the survival of cones via increased glucose uptake (10). Proof of concept for this approach has been demonstrated in a porcine model of retinitis pigmentosa, where photoreceptor precursors from pig embryos and hPSCs prevented the loss of cones up to 1,000 μm from the site of injection and reactivated dormant cones when performed prior to cone inner segment disassembly (239). Thus, therapeutic strategies should be designed in a disease specific manner to achieve the best outcome possible.
The immune privilege of the eye is not absolute in health and is often compromised in the diseased state. Therefore, one major hurdle in the utilization of hPSCs for cell therapies is a requirement for human leukocyte antigen (HLA) matching between the donor cells and the recipient, as mismatched HLA can lead to immune rejection and decreased therapeutic efficacy (240). Using autologous hIPSC lines offer a perfect HLA match for the patient, however, the current technological limitations, including the time and effort required to generate and validate patient-specific hIPSC clones and the need for genetic modification of disease alleles before differentiation, make this approach impractical (84, 243).
A truly universal donor stem cell line would allow off-the-shelf therapies to be available to the entire population. At present, gene editing is used to suppress surface expression of HLA-I and HLA-II proteins, while simultaneously preventing attack by Natural Killer (NK) cells (244). This is primarily achieved in hPSCs by deleting the genes encoding for Beta-2 Microglobulin (B2M, encoding the subunit required for surface expression HLA-class I proteins) and CTIIA, which encodes a transcription factor necessary for HLA-class II proteins (245). Complimentary or combinatorial approaches have also been used to prevent NK attack, reduce immune co-stimulation or enhance tolerance by overexpression of other molecules like CD47, HLA-E, HLA-G, PD-L1, CTLA4-Ig (246–248).
Alternatively, HLA homozygote superdonor stem cell banks can be generated to match the HLA-A, HLA-B, and HLA-DR loci of populations for transplant compatibility (249). The degree of population compatibility depends on the genetic diversity within a given population. For instance, it is estimated that 30–55 HLA homozygous lines cover 80% of the Japanese population (250), while 150 lines would be needed for 93% compatibility in the UK (251) and 80 lines for 50% compatibility in California (252).
Each approach towards immunocompatible cells is imperfect and comes with its own compromises. For instance, patient specific hIPSC lines are a perfect HLA match to the donor but are expensive and time consuming to create product cells from the initial biopsy. HLA homozygous lines achieve compatibility with limited subsets of any given population without immune evasion or suppression, and thus may may present viral or cancer antigens in a normally. Finally, universal HLA-edited lines may be compatible with the entire population, although there are some safety concerns about compromised antigen presentation and mimicry of cancer survival strategies.
3. Conclusions
Advancing cell-based therapies to address retinal degenerative diseases necessitates interdisciplinary progress. Over the past five decades, significant advancements in areas such as transplantation, immunology, molecular, cell biology, and materials science have increased the possibility of cell therapies to treat retinal degeneration—and perhaps restore vision—within our lifetimes. This is particularly evident in the rapid progress in cell production and supportive technologies like GMP facilities and the establishment of hPSC banks.
However, recent insights into cytoplasmic exchange between donor and host photoreceptors reveal that genuine integration rates are notably lower than initially estimated, thereby elevating the evidential threshold for researchers. Such findings underscore the need to revisit critical facets of photoreceptor transplantation, including the optimal differentiation state and delivery modality, be it through injections of singular cells, retinal sheets, or concurrent delivery of RPE with retinal cells. Given the inconsistencies in transplantation outcomes across animal models, novel models may be needed to assess and predict photoreceptor integration in the context of human retinal pathology.
Complementary therapeutic strategies, such as neuroprotection, EV therapies, gene interventions, immune modulation, and optogenetics may reduce the need for cell replacement, delay regeneration or extend the optimal window for cell transplantation. Such approaches may be complementary or necessary to pre-treat the retina before cell transplantation for optimized therapeutic outcomes. Such strategies might be indispensable for pre-treatment before cell transplantation to maximize therapeutic efficacy. Inevitably, human clinical trials will play a pivotal role in establishing human specific approaches and widespread adoption of photoreceptor transplantation therapies.
Author contributions
IJL corresponding author, co-wrote the first draft paper and wrote the final paper, figures, final approval. DB co-wrote the first draft paper, final approval. All authors contributed to the article and approved the submitted version.
Funding
This work was supported by the Clem Jones Foundation, a bequest from the Estate of Cora Cutmore and a donation from Roslyn and Allan Bull.
Acknowledgments
We would like to thank Professor Helen O'Neill for her helpful discussions and contributions to this work.
Conflict of interest
The authors declare that the research was conducted in the absence of any commercial or financial relationships that could be construed as a potential conflict of interest.
Publisher's note
All claims expressed in this article are solely those of the authors and do not necessarily represent those of their affiliated organizations, or those of the publisher, the editors and the reviewers. Any product that may be evaluated in this article, or claim that may be made by its manufacturer, is not guaranteed or endorsed by the publisher.
References
1. GBD 2019 Blindness and Vision Impairment Collaborators; Bourne R, Steinmetz JD, Flaxman S, Briant PS, Taylor HR, et al. Trends in prevalence of blindness and distance and near vision impairment over 30 years: an analysis for the global burden of disease study. Lancet Global Heal. (2020) 9:e130–43. doi: 10.1016/s2214-109x(20)30425-3
2. Veleri S, Lazar CH, Chang B, Sieving PA, Banin E, Swaroop A. Biology and therapy of inherited retinal degenerative disease: insights from mouse models. Dis Model Mech. (2015) 8:109–29. doi: 10.1242/dmm.017913
3. Ptito M, Bleau M, Bouskila J. The retina: a window into the brain. Cells. (2021) 10:3269. doi: 10.3390/cells10123269
4. Curcio CA, Sloan KR, Kalina RE, Hendrickson AE. Human photoreceptor topography. J Comp Neurol. (1990) 292:497–523. doi: 10.1002/cne.902920402
5. Pugh EN. The discovery of the ability of rod photoreceptors to signal single photons. J Gen Physiol. (2018) 150:383–8. doi: 10.1085/jgp.201711970
6. Carvalho LS, Pessoa DMA, Mountford JK, Davies WIL, Hunt DM. The genetic and evolutionary drives behind primate color vision. Frontiers Ecol Evol. (2017) 5:34. doi: 10.3389/fevo.2017.00034
7. Masland RH. Cell populations of the retina: the Proctor lecture. Invest Ophth Vis Sci. (2011) 52:4581–91. doi: 10.1167/iovs.10-7083
8. Sparrrow JR, Hicks D, Hamel CP. The retinal pigment epithelium in health and disease. Curr Mol Med. (2010) 10:802–23. doi: 10.2174/156652410793937813
9. Bringmann A, Reichenbach A. Role of Muller cells in retinal degenerations. Front Biosci J Virtual Libr. (2001) 6:E72–92. doi: 10.2741/bringman
10. Aït-Ali N, Fridlich R, Millet-Puel G, Clérin E, Delalande F, Jaillard C, et al. Rod-derived cone viability factor promotes cone survival by stimulating aerobic glycolysis. Cell. (2015) 161:817–32. doi: 10.1016/j.cell.2015.03.023
11. Bourne RRA, Jonas JB, Flaxman SR, Keeffe J, Leasher J, Naidoo K, et al. Prevalence and causes of vision loss in high-income countries and in eastern and central Europe: 1990–2010. Br J Ophthalmol. (2014) 98:629–38. doi: 10.1136/bjophthalmol-2013-304033
12. Flaxman SR, Bourne RRA, Resnikoff S, Ackland P, Braithwaite T, Cicinelli MV, et al. Global causes of blindness and distance vision impairment 1990–2020: a systematic review and meta-analysis. Lancet Glob Health. (2017) 5(12):e1221–34. doi: 10.1016/s2214-109x(17)30393-5
13. Priore LVD, Kaplan HJ. Pathogenesis of AMD. Ophthalmology. (1995) 102:1125. doi: 10.1016/s0161-6420(95)30903-7
14. Thomas CJ, Mirza RG, Gill MK. Age-related macular degeneration. Med Clin N Am. (2021b) 105:473–91. doi: 10.1016/j.mcna.2021.01.003
15. Ong JM, da Cruz L. A review and update on the current status of stem cell therapy and the retina. Brit Med Bull. (2012) 102:133–46. doi: 10.1093/bmb/lds013
16. Hu ML, Edwards TL, O’Hare F, Hickey DG, Wang J-H, Liu Z, et al. Gene therapy for inherited retinal diseases: progress and possibilities. Clin Exp Optom. (2021) 104:444–54. doi: 10.1080/08164622.2021.1880863
17. Schneider N, Sundaresan Y, Gopalakrishnan P, Beryozkin A, Hanany M, Levanon EY, et al. Inherited retinal diseases: linking genes, disease-causing variants, and relevant therapeutic modalities. Prog Retin Eye Res. (2022) 89:101029. doi: 10.1016/j.preteyeres.2021.101029
18. Newton F, Megaw R. Mechanisms of photoreceptor death in retinitis Pigmentosa. Genes (Basel). (2020) 11:1120. doi: 10.3390/genes11101120
19. Duncan JL, Pierce EA, Laster AM, Daiger SP, Birch DG, Ash JD, et al. Inherited retinal degenerations: current landscape and knowledge gaps. Transl Vis Sci Technol. (2018) 7:6. doi: 10.1167/tvst.7.4.6
20. Stahl A. The diagnosis and treatment of age-related macular degeneration. Dtsch Ärzteblatt Int. (2020) 117:513–20. doi: 10.3238/arztebl.2020.0513
21. Sharma D, Zachary I, Jia H. Mechanisms of acquired resistance to anti-VEGF therapy for neovascular eye diseases. Investig Ophthalmol Vis Sci. (2023) 64:28. doi: 10.1167/iovs.64.5.28
22. Hoy SM. Pegcetacoplan: first approval. Drugs. (2021) 81:1423–30. doi: 10.1007/s40265-021-01560-8
23. Steinle NC, Pearce I, Monés J, Metlapally R, Saroj N, Hamdani M, et al. Impact of baseline characteristics on geographic atrophy progression in the FILLY trial evaluating the complement C3 inhibitor pegcetacoplan. Am J Ophthalmol. (2021) 227:116–24. doi: 10.1016/j.ajo.2021.02.031
24. Liao DS, Metlapally R, Joshi P. Pegcetacoplan treatment for geographic atrophy due to age-related macular degeneration: a plain language summary of the FILLY study. Immunotherapy. (2022) 14:995–1006. doi: 10.2217/imt-2022-0078
25. Wykoff CC, Rosenfeld PJ, Waheed NK, Singh RP, Ronca N, Slakter JS, et al. Characterizing new-onset exudation in the randomized phase 2 FILLY trial of complement inhibitor pegcetacoplan for geographic atrophy. Ophthalmology. (2021) 128:1325–36. doi: 10.1016/j.ophtha.2021.02.025
26. Scholl HPN, Strauss RW, Singh MS, Dalkara D, Roska B, Picaud S, et al. Emerging therapies for inherited retinal degeneration. Sci Transl Med. (2016) 8:368rv6. doi: 10.1126/scitranslmed.aaf2838
27. Chow LLC, Mead B. Extracellular vesicles as a potential therapeutic for age-related macular degeneration. Neural Regen Res. (2023) 18:1876–80. doi: 10.4103/1673-5374.367835
28. Sakai D, Tomita H, Maeda A. Optogenetic therapy for visual restoration. Int J Mol Sci. (2022) 23:15041. doi: 10.3390/ijms232315041
29. Cheng S-Y, Punzo C. Update on viral gene therapy clinical trials for retinal diseases. Hum Gene Ther. (2022) 33:865–78. doi: 10.1089/hum.2022.159
30. Khanani AM, Thomas MJ, Aziz AA, Weng CY, Danzig CJ, Yiu G, et al. Review of gene therapies for age-related macular degeneration. Eye. (2022) 36:303–11. doi: 10.1038/s41433-021-01842-1
31. Rohowetz LJ, Koulen P. Stem cell-derived retinal pigment epithelium cell therapy: past and future directions. Front Cell Dev Biol. (2023) 11:1098406. doi: 10.3389/fcell.2023.1098406
32. Stone JL, Barlow WE, Humayun MS, de Juan E, Milam AH. Morphometric analysis of macular photoreceptors and ganglion cells in retinas with retinitis Pigmentosa. Arch Ophthalmol. (1992) 110:1634–9. doi: 10.1001/archopht.1992.01080230134038
33. Santos A, Humayun MS, de Juan E, Greenburg RJ, Marsh MJ, Klock IB, et al. Preservation of the inner retina in retinitis Pigmentosa: a morphometric analysis. Arch Ophthalmol. (1997) 115:511–5. doi: 10.1001/archopht.1997.01100150513011
34. Humayun MS, de Juan E, Dagnelie G, Greenberg RJ, Propst RH, Phillips DH. Visual perception elicited by electrical stimulation of retina in blind humans. Arch Ophthalmol. (1996) 114:40–6. doi: 10.1001/archopht.1996.01100130038006
35. Warre-Cornish K, Barber AC, Sowden JC, Ali RR, Pearson RA. Migration, integration and maturation of photoreceptor precursors following transplantation in the mouse retina. Stem Cells Dev. (2014) 23:941–54. doi: 10.1089/scd.2013.0471
36. Maeda T, Mandai M, Sugita S, Kime C, Takahashi M. Strategies of pluripotent stem cell-based therapy for retinal degeneration: update and challenges. Trends Mol Med. (2022) 28:388–404. doi: 10.1016/j.molmed.2022.03.001
37. Keino H, Horie S, Sugita S. Immune privilege and eye-derived T-regulatory cells. J Immunol Res. (2018) 2018:1–12. doi: 10.1155/2018/1679197
38. Das T, del Cerro M, Jalali S, Rao VS, Gullapalli VK, Little C, et al. The transplantation of human fetal neuroretinal cells in advanced retinitis Pigmentosa patients: results of a long-term safety study. Exp Neurol. (1999) 157:58–68. doi: 10.1006/exnr.1998.6992
39. Tu H-Y, Watanabe T, Shirai H, Yamasaki S, Kinoshita M, Matsushita K, et al. Medium- to long-term survival and functional examination of human iPSC-derived retinas in rat and primate models of retinal degeneration. EBioMedicine. (2019) 39:562–74. doi: 10.1016/j.ebiom.2018.11.028
40. Aziz K, Zarbin MA, Singh MS. Cell-Based therapy for degenerative retinal disease. Cham: Springer Nature Switzerland AG (2019) p. 245–65. doi: 10.1007/978-3-030-05222-5_13
41. Zarbin M, Sugino I, Townes-Anderson E. Concise review: update on retinal pigment epithelium transplantation for age-related macular degeneration. Stem Cells Transl Med. (2019) 8(5):466–77. doi: 10.1002/sctm.18-0282
42. O’Neill HC, Limnios IJ, Barnett NL. Advancing a stem cell therapy for age-related macular degeneration. Curr Stem Cell Res Ther. (2020) 15:89–97. doi: 10.2174/1574888x15666191218094020
43. Thompson DA, Iannaccone A, Ali RR, Arshavsky VY, Audo I, Bainbridge JWB, et al. Advancing clinical trials for inherited retinal diseases: recommendations from the second monaciano symposium. Transl Vis Sci Technol. (2020) 9:2. doi: 10.1167/tvst.9.7.2
44. Hinkle JW, Mahmoudzadeh R, Kuriyan AE. Cell-based therapies for retinal diseases: a review of clinical trials and direct to consumer “cell therapy” clinics. Stem Cell Res Ther. (2021) 12:538. doi: 10.1186/s13287-021-02546-9
45. Enosawa S. Clinical trials of stem cell therapy in Japan: the decade of progress under the national program. J Clin Med. (2022) 11:7030. doi: 10.3390/jcm11237030
46. Gouras P, Flood MT, Kjeldbye H, Bilek MK, Eggers H. Transplantation of cultured human retinal epithelium to bruch’s membrane of the owl monkey’s eye. Curr Eye Res. (1985) 4:253–65. doi: 10.3109/02713688509000857
47. del Cerro M, Gash DM, Rao GN, Notter MF, Wiegand SJ, Gupta M. Intraocular retinal transplants. Invest Ophth Vis Sci. (1985) 26:1182–5.
48. del Cerro M, Notter MFD, Wiegand SJ, Jiang LQ, del Cerro C. Intraretinal transplantation of fluorescently labeled retinal cell suspensions. Neurosci Lett. (1988) 92:21–6. doi: 10.1016/0304-3940(88)90735-5
49. Aramant R, Seiler M, Ehinger B, Bergström A, Gustavii B, Brundin P, et al. Transplantation of human embryonic retina to adult rat retina. Restor Neurol Neurosci. (1990) 2:9–22. doi: 10.3233/rnn-1990-2102
50. Gouras P, Du J, Gelanze M, Lopez R, Kwun R, Kjeldbye H, et al. Survival and synapse formation of transplanted rat rods. Neural Plast. (1991) 2:91–100. doi: 10.1155/np.1991.91
51. Engelsberg K, Ghosh F. Transplantation of cultured adult porcine full-thickness retina. Cell Transplant. (2007) 16:31–9. doi: 10.3727/000000007783464506
52. Turner JE, Blair JR. Newborn rat retinal cells transplanted into a retinal lesion site in adult host eyes. Dev Brain Res. (1986) 26:91–104. doi: 10.1016/0165-3806(86)90011-8
53. Blair JR, Turner JE. Optimum conditions for successful transplantation of immature rat retina to the lesioned adult retina. Dev Brain Res. (1987) 36:257–70. doi: 10.1016/0165-3806(87)90029-0
54. del Cerro M, Lazar ES, Diloreto D Jr. The first decade of continuous progress in retinal transplantation. Microsc Res Tech. (1997) 36:130–41. doi: 10.1002/(sici)1097-0029(19970115)36:2%3C130::aid-jemt6%3E3.0.co;2-t
55. del Manuel CDN, Mary F, del Coca CJJ, Stanley A, Donald G, Eliot L. Intraretinal transplantation for rod-cell replacement in light-damaged retinas. Neural Plast. (1989) 1:1–10. doi: 10.1155/np.1989.1
56. Gouras P, Du J, Kjeldbye H, Kwun R, Lopez R, Zack DJ. Transplanted photoreceptors identified in dystrophic mouse retina by a transgenic reporter gene. Investig Ophthalmol Vis Sci. (1991b) 32:3167–74.
57. Gouras P, Du J, Gelanze M, Kwun R, Kjeldbye H, Lopez R. Transplantation of photoreceptors labeled with tritiated thymidine into RCS rats. Investig Ophthalmol Vis Sci. (1991a) 32:1704–7.
58. Kwan ASL, Wang S, Lund RD. Photoreceptor layer reconstruction in a rodent model of retinal degeneration. Exp Neurol. (1999) 159:21–33. doi: 10.1006/exnr.1999.7157
59. Aramant R, Seiler M, Turner JE. Donor age influences on the success of retinal grafts to adult rat retina. Investig Ophthalmol Vis Sci. (1988) 29:498–503.
60. MacLaren RE, Pearson RA, MacNeil A, Douglas RH, Salt TE, Akimoto M, et al. Retinal repair by transplantation of photoreceptor precursors. Nature. (2006) 444:203–7. doi: 10.1038/nature05161
61. Ghosh F, Engelsberg K, English RV, Petters RM. Long-term neuroretinal full-thickness transplants in a large animal model of severe retinitis Pigmentosa. Graefe’s Arch Clin Exp Ophthalmol. (2007) 245:835–46. doi: 10.1007/s00417-006-0437-9
62. Kaplan HJ, Tezel TH, Berger AS, Wolf ML, Priore LVD. Human photoreceptor transplantation in retinitis Pigmentosa: a safety study. Arch Ophthalmol. (1997) 115:1168–72. doi: 10.1001/archopht.1997.01100160338012
63. Radtke ND, Aramant RB, Seiler M, Petry HM. Preliminary report: indications of improved visual function after retinal sheet transplantation in retinitis pigmentosa patients. Am J Ophthalmol. (1999) 128:384–7. doi: 10.1016/s0002-9394(99)00250-0
64. Radtke ND, Aramant RB, Seiler MJ, Petry HM, Pidwell D. Vision change after sheet transplant of fetal retina with retinal pigment epithelium to a patient with retinitis Pigmentosa. Arch Ophthalmol. (2004) 122:1159–65. doi: 10.1001/archopht.122.8.1159
65. Berger AS, Tezel TH, Priore LVD, Kaplan HJ. Photoreceptor transplantation in retinitis Pigmentosa: short-term follow-up. Ophthalmology. (2003) 110:383–91. doi: 10.1016/s0161-6420(02)01738-4
66. Thomson JA, Itskovitz-Eldor J, Shapiro SS, Waknitz MA, Swiergiel JJ, Marshall VS, et al. Embryonic stem cell lines derived from human blastocysts. Science. (1998) 282:1145–7. doi: 10.1126/science.282.5391.1145
67. Takahashi K, Tanabe K, Ohnuki M, Narita M, Ichisaka T, Tomoda K, et al. Induction of pluripotent stem cells from adult human fibroblasts by defined factors. Cell. (2007) 131:861–72. doi: 10.1016/j.cell.2007.11.019
68. Yu J, Vodyanik MA, Smuga-Otto K, Antosiewicz-Bourget J, Frane JL, Tian S, et al. Induced pluripotent stem cell lines derived from human somatic cells. Science. (2007) 318:1917–20. doi: 10.1126/science.1151526
69. Walker MJ, Bourke J, Hutchison K. Evidence for personalised medicine: mechanisms, correlation, and new kinds of black box. Theor Med Bioeth. (2019) 40:103–21. doi: 10.1007/s11017-019-09482-z
70. Schwartz SD, Hubschman J-P, Heilwell G, Franco-Cardenas V, Pan CK, Ostrick RM, et al. Embryonic stem cell trials for macular degeneration: a preliminary report. Lancet. (2012) 379:713–20. doi: 10.1016/s0140-6736(12)60028-2
71. Mandai M, Watanabe A, Kurimoto Y, Hirami Y, Morinaga C, Daimon T, et al. Autologous induced stem-cell–derived retinal cells for macular degeneration. N Engl J Med. (2017b) 376:1038–46. doi: 10.1056/nejmoa1608368
72. Kobold S, Bultjer N, Stacey G, Mueller SC, Kurtz A, Mah N. History and current status of clinical studies using human pluripotent stem cells. Stem Cell Rep. (2023) 18(8):1592–8. doi: 10.1016/j.stemcr.2023.03.005
73. Watari K, Yamasaki S, Tu H-Y, Shikamura M, Kamei T, Adachi H, et al. Self-organization, quality control, and preclinical studies of human iPSC-derived retinal sheets for tissue-transplantation therapy. Commun Biol. (2023) 6:164. doi: 10.1038/s42003-023-04543-5
74. Song W, Park K-M, Kim H-J, Lee J, Choi J, Chong S, et al. Treatment of macular degeneration using embryonic stem cell-derived retinal pigment epithelium: preliminary results in Asian patients. Stem Cell Rep. (2015) 4:860–72. doi: 10.1016/j.stemcr.2015.04.005
75. da Cruz L, Fynes K, Georgiadis O, Kerby J, Luo YH, Ahmado A, et al. Phase 1 clinical study of an embryonic stem cell–derived retinal pigment epithelium patch in age-related macular degeneration. Nat Biotechnol. (2018) 36:328–37. doi: 10.1038/nbt.4114
76. Kashani AH, Lebkowski JS, Rahhal FM, Avery RL, Salehi-Had H, Dang W, et al. A bioengineered retinal pigment epithelial monolayer for advanced, dry age-related macular degeneration. Sci Transl Med. (2018) 10:eaao4097. doi: 10.1126/scitranslmed.aao4097
77. Lane A, Philip LR, Ruban L, Fynes K, Smart M, Carr A, et al. Engineering efficient retinal pigment epithelium differentiation from human pluripotent stem cells. Stem Cell Transl Med. (2014) 3:1295–304. doi: 10.5966/sctm.2014-0094
78. da Cruz L, Chen FK, Ahmado A, Greenwood J, Coffey P. RPE Transplantation and its role in retinal disease. Prog Retinal Eye Res. (2007) 26:598–635. doi: 10.1016/j.preteyeres.2007.07.001
79. Buchholz DE, Pennington BO, Croze RH, Hinman CR, Coffey PJ, Clegg DO. Rapid and efficient directed differentiation of human pluripotent stem cells into retinal pigmented epithelium. Stem Cells Transl Med. (2013) 2:384–93. doi: 10.5966/sctm.2012-0163
80. Fazzina R, Mariotti A, Procoli A, Fioravanti D, Iudicone P, Scambia G, et al. A new standardized clinical-grade protocol for banking human umbilical cord tissue cells. Transfusion. (2015) 55:2864–73. doi: 10.1111/trf.13277
81. Limnios IJ, Chau Y-Q, Skabo SJ, Surrao DC, O’Neill HC. Efficient differentiation of human embryonic stem cells to retinal pigment epithelium under defined conditions. Stem Cell Res Ther. (2021) 12:248. doi: 10.1186/s13287-021-02316-7
82. McGill TJ, Bohana-Kashtan O, Stoddard JW, Andrews MD, Pandit N, Rosenberg-Belmaker LR, et al. Long-term efficacy of GMP grade Xeno-free hESC-derived RPE cells following transplantation. Transl Vis Sci Technol. (2017) 6:17. doi: 10.1167/tvst.6.3.17
83. Mehat MS, Sundaram V, Ripamonti C, Robson AG, Smith AJ, Borooah S, et al. Transplantation of human embryonic stem cell-derived retinal pigment epithelial cells in macular degeneration. Ophthalmology. (2018) 125:1765–75. doi: 10.1016/j.ophtha.2018.04.037
84. Takagi S, Mandai M, Gocho K, Hirami Y, Yamamoto M, Fujihara M, et al. Evaluation of transplanted autologous induced pluripotent stem cell-derived retinal pigment epithelium in exudative age-related macular degeneration. Ophthalmol Retin. (2019) 3:850–9. doi: 10.1016/j.oret.2019.04.021
85. Ludwig AL, Gamm DM. Outer retinal cell replacement: putting the pieces together. Transl Vis Sci Technol. (2021) 10:15. doi: 10.1167/tvst.10.10.15
86. Wilson PA, Hemmati-Brivanlou A. Vertebrate neural induction: inducers, inhibitors, and a new synthesis. Neuron. (1997) 18:699–710. doi: 10.1016/s0896-6273(00)80311-6
87. Hemmati-Brivanlou A, Melton D. Vertebrate embryonic cells will become nerve cells unless told otherwise. Cell. (2000) 88:13–7. doi: 10.1016/s0092-8674(00)81853-x
88. Zhou S, Flamier A, Abdouh M, Tétreault N, Barabino A, Wadhwa S, et al. Differentiation of human embryonic stem cells into cone photoreceptors through simultaneous inhibition of BMP, TGFβ and Wnt signaling. Development. (2015) 142:3294–306. doi: 10.1242/dev.125385
89. Chavali VRM, Haider N, Rathi S, Vrathasha V, Alapati T, He J, et al. Dual SMAD inhibition and Wnt inhibition enable efficient and reproducible differentiations of induced pluripotent stem cells into retinal ganglion cells. Sci Rep. (2020) 10:11828. doi: 10.1038/s41598-020-68811-8
90. Heavner W, Pevny L. Eye development and retinogenesis. Csh Perspect Biol. (2012) 4:a008391. doi: 10.1101/cshperspect.a008391
91. Capowski EE, Wright LS, Liang K, Phillips MJ, Wallace K, Petelinsek A, et al. Regulation of WNT signaling by VSX2 during optic vesicle patterning in human induced pluripotent stem cells. Stem Cells. (2016) 34:2625–34. doi: 10.1002/stem.2414
92. Fujimura N, Taketo MM, Mori M, Korinek V, Kozmik Z. Spatial and temporal regulation of Wnt/β-catenin signaling is essential for development of the retinal pigment epithelium. Dev Biol. (2009) 334:31–45. doi: 10.1016/j.ydbio.2009.07.002
93. Pittack C, Grunwald GB, Reh TA. Fibroblast growth factors are necessary for neural retina but not pigmented epithelium differentiation in chick embryos. Development. (1997) 124:805–16. doi: 10.1242/dev.124.4.805
94. Gamm DM, Clark E, Capowski EE, Singh R. The role of FGF9 in the production of neural retina and RPE in a pluripotent stem cell model of early human retinal development. Am J Ophthalmol. (2019) 206:113–31. doi: 10.1016/j.ajo.2019.04.033
95. Fujimura N. WNT/β-catenin signaling in vertebrate eye development. Front Cell Dev Biol. (2016) 4:138. doi: 10.3389/fcell.2016.00138
96. Ikeda H, Osakada F, Watanabe K, Mizuseki K, Haraguchi T, Miyoshi H, et al. Generation of rx+/Pax6+ neural retinal precursors from embryonic stem cells. Proc National Acad Sci. (2005) 102:11331–6. doi: 10.1073/pnas.0500010102
97. Lamba DA, Karl MO, Ware CB, Reh TA. Efficient generation of retinal progenitor cells from human embryonic stem cells. Proc National Acad Sci. (2006) 103:12769–74. doi: 10.1073/pnas.0601990103
98. Osakada F, Ikeda H, Mandai M, Wataya T, Watanabe K, Yoshimura N, et al. Toward the generation of rod and cone photoreceptors from mouse, monkey and human embryonic stem cells. Nat Biotechnol. (2008) 26:215–24. doi: 10.1038/nbt1384
99. Lamba DA, McUsic A, Hirata RK, Wang P-R, Russell D, Reh TA. Generation, purification and transplantation of photoreceptors derived from human induced pluripotent stem cells. PLoS One. (2010) 5(1):e8763. doi: 10.1371/journal.pone.0008763
100. Meyer JS, Shearer RL, Capowski EE, Wright LS, Wallace KA, McMillan EL, et al. Modeling early retinal development with human embryonic and induced pluripotent stem cells. Proc Natl Acad Sci U S A. (2009) 106:16698–703. doi: 10.1073/pnas.0905245106
101. Eiraku M, Takata N, Ishibashi H, Kawada M, Sakakura E, Okuda S, et al. Self-organizing optic-cup morphogenesis in three-dimensional culture. Nature. (2011) 472:51. doi: 10.1038/nature09941
102. Nakano T, Ando S, Takata N, Kawada M, Muguruma K, Sekiguchi K, et al. Self-formation of optic cups and storable stratified neural retina from human ESCs. Cell Stem Cell. (2012) 10:771–85. doi: 10.1016/j.stem.2012.05.009
103. Bell CM, Zack DJ, Berlinicke CA. Human organoids for the study of retinal development and disease. Annu Rev Vis Sc. (2020) 6:91–114. doi: 10.1146/annurev-vision-121219-081855
104. Li B, Zhang T, Liu W, Wang Y, Xu R, Zeng S, et al. Metabolic features of mouse and human retinas: rods vs. cones, macula vs. periphery retina versus RPE. Iscience. (2020) 23:101672. doi: 10.1016/j.isci.2020.101672
105. Onyak JR, Vergara MN, Renna JM. Retinal organoid light responsivity: current status and future opportunities. Transl Res. (2022) 250:98–111. doi: 10.1016/j.trsl.2022.06.001
106. Kang J, Gong J, Yang C, Lin X, Yan L, Gong Y, et al. Application of human stem cell derived retinal organoids in the exploration of the mechanisms of early retinal development. Stem Cell Rev Rep. (2023) 19(6):1755–72. doi: 10.1007/s12015-023-10553-x
107. Watson A, Lako M. Retinal organoids provide unique insights into molecular signatures of inherited retinal disease throughout retinogenesis. J Anat. (2023) 243(2):186–203. doi: 10.1111/joa.13768
108. Aasen DM, Vergara MN. New drug discovery paradigms for retinal diseases: a focus on retinal organoids. J Ocul Pharmacol Ther. (2020) 36:18–24. doi: 10.1089/jop.2018.0140
109. Belova L, Lavrov A, Smirnikhina S. Organoid transduction using recombinant adeno-associated viral vectors: challenges and opportunities. BioEssays. (2022) 44:2200055. doi: 10.1002/bies.202200055
110. Zhu Y, Cao B, Tolone A, Yan J, Christensen G, Arango-Gonzalez B, et al. In vitro model systems for studies into retinal neuroprotection. Front Neurosci-Switz. (2022) 16:938089. doi: 10.3389/fnins.2022.938089
111. Mandai M. Pluripotent stem cell-derived retinal organoid/cells for retinal regeneration therapies: a review. Regen Ther. (2023) 22:59–67. doi: 10.1016/j.reth.2022.12.005
112. Mellough CB, Collin J, Khazim M, White K, Sernagor E, Steel DHW, et al. IGF-1 signaling plays an important role in the formation of three-dimensional laminated neural retina and other ocular structures from human embryonic stem cells. Stem Cells. (2015) 33:2416–30. doi: 10.1002/stem.2023
113. Reichman S, Terray A, Slembrouck A, Nanteau C, Orieux G, Habeler W, et al. From confluent human iPS cells to self-forming neural retina and retinal pigmented epithelium. Proc Natl Acad Sci U S A. (2014) 111:8518–23. doi: 10.1073/pnas.1324212111
114. Zhong X, Gutierrez C, Xue T, Hampton C, Vergara MN, Cao L-H, et al. Generation of three-dimensional retinal tissue with functional photoreceptors from human iPSCs. Nat Commun. (2014) 5:4047. doi: 10.1038/ncomms5047
115. Lowe A, Harris R, Bhansali P, Cvekl A, Liu W. Intercellular adhesion-dependent cell survival and ROCK-regulated actomyosin-driven forces mediate self-formation of a retinal organoid. Stem Cell Rep. (2016) 6:743–56. doi: 10.1016/j.stemcr.2016.03.011
116. Levine AJ, Brivanlou AH. Proposal of a model of mammalian neural induction. Dev Biol. (2007) 308:247–56. doi: 10.1016/j.ydbio.2007.05.036
117. Nazor KL, Altun G, Lynch C, Tran H, Harness JV, Slavin I, et al. Recurrent variations in DNA methylation in human pluripotent stem cells and their differentiated derivatives. Cell Stem Cell. (2012) 10:620–34. doi: 10.1016/j.stem.2012.02.013
118. Choi J, Lee S, Mallard W, Clement K, Tagliazucchi GM, Lim H, et al. A comparison of genetically matched cell lines reveals the equivalence of human iPSCs and ESCs. Nat Biotechnol. (2015) 33:1173–81. doi: 10.1038/nbt.3388
119. Wang L, Hiler D, Xu B, AlDiri I, Chen X, Zhou X, et al. Retinal cell type DNA methylation and histone modifications predict reprogramming efficiency and retinogenesis in 3D organoid cultures. Cell Rep. (2018) 22:2601–14. doi: 10.1016/j.celrep.2018.01.075
120. Slembrouck-Brec A, Rodrigues A, Rabesandratana O, Gagliardi G, Nanteau C, Fouquet S, et al. Reprogramming of adult retinal Müller glial cells into human-induced pluripotent stem cells as an efficient source of retinal cells. Stem Cells Int. (2019) 2019:7858796. doi: 10.1155/2019/7858796
121. Ito A, Ye K, Onda M, Morimoto N, Osakada F. Efficient and robust induction of retinal pigment epithelium cells by tankyrase inhibition regardless of the differentiation propensity of human induced pluripotent stem cells. Biochem Bioph Res Co. (2021) 552:66–72. doi: 10.1016/j.bbrc.2021.03.012
122. Browne AW, Arnesano C, Harutyunyan N, Khuu T, Martinez JC, Pollack HA, et al. Structural and functional characterization of human stem-cell-derived retinal organoids by live imaging. Investig Ophthalmol Vis Sci. (2017) 58:3311–8. doi: 10.1167/iovs.16-20796
123. Zhu J, Reynolds J, Garcia T, Cifuentes H, Chew S, Zeng X, et al. Generation of transplantable retinal photoreceptors from a current good manufacturing practice-manufactured human induced pluripotent stem cell line. Stem Cells Transl Med. (2018) 7:210–9. doi: 10.1002/sctm.17-0205
124. Döpper H, Menges J, Bozet M, Brenzel A, Lohmann D, Steenpass L, et al. Differentiation protocol for 3D retinal organoids, immunostaining and signal quantitation. Curr Protoc Stem Cell Biol. (2020) 55:e120. doi: 10.1002/cpsc.120
125. Pan D, Xia X-X, Zhou H, Jin S-Q, Lu Y-Y, Liu H, et al. COCO enhances the efficiency of photoreceptor precursor differentiation in early human embryonic stem cell-derived retinal organoids. Stem Cell Res Ther. (2020) 11:366. doi: 10.1186/s13287-020-01883-5
126. Zhang X, Jin Z-B. Directed induction of retinal organoids from human pluripotent stem cells. J Vis Exp. (2021) (170). doi: 10.3791/62298
127. Wahlin KJ, Maruotti JA, Sripathi SR, Ball J, Angueyra JM, Kim C, et al. Photoreceptor outer segment-like structures in long-term 3D retinas from human pluripotent stem cells. Sci Rep. (2017) 7:766. doi: 10.1038/s41598-017-00774-9
128. Eldred KC, Hadyniak SE, Hussey KA, Brenerman B, Zhang P-W, Chamling X, et al. Thyroid hormone signaling specifies cone subtypes in human retinal organoids. Science. (2018) 362:eaau6348. doi: 10.1126/science.aau6348
129. Lu Y, Shiau F, Yi W, Lu S, Wu Q, Pearson JD, et al. Single-cell analysis of human retina identifies evolutionarily conserved and Species-specific mechanisms controlling development. Dev Cell. (2020) 53:473–491.e9. doi: 10.1016/j.devcel.2020.04.009
130. Regent F, Batz Z, Kelley RA, Gieser L, Swaroop A, Chen HY, et al. Nicotinamide promotes formation of retinal organoids from human pluripotent stem cells via enhanced neural cell fate commitment. Front Cell Neurosci. (2022) 16:878351. doi: 10.3389/fncel.2022.878351
131. Meng Y, Ren Z, Xu F, Zhou X, Song C, Wang VY-F, et al. Nicotinamide promotes cell survival and differentiation as kinase inhibitor in human pluripotent stem cells. Stem Cell Rep. (2018) 11:1347–56. doi: 10.1016/j.stemcr.2018.10.023
132. Reichman S, Slembrouck A, Gagliardi G, Chaffiol A, Terray A, Nanteau C, et al. Generation of storable retinal organoids and retinal pigmented epithelium from adherent human iPS cells in Xeno-free and feeder-free conditions. Stem Cells. (2017) 35:1176–88. doi: 10.1002/stem.2586
133. Garita-Hernandez M, Guibbal L, Toualbi L, Routet F, Chaffiol A, Winckler C, et al. Optogenetic light sensors in human retinal organoids. Front Neurosci. (2018) 12:789. doi: 10.3389/fnins.2018.00789
134. Singh RK, Mallela RK, Cornuet PK, Reifler AN, Chervenak AP, West MD, et al. Characterization of three-dimensional retinal tissue derived from human embryonic stem cells in adherent monolayer cultures. Stem Cells Dev. (2015) 24:2778–95. doi: 10.1089/scd.2015.0144
135. Singh RK, Occelli LM, Binette F, Petersen-Jones SM, Nasonkin IO. Transplantation of human embryonic stem cell-derived retinal tissue in the subretinal space of the cat eye. Stem Cells Dev. (2019) 28:1151–66. doi: 10.1089/scd.2019.0090
136. Lane A, Jovanovic K, Shortall C, Ottaviani D, Panes AB, Schwarz N, et al. Modeling and rescue of RP2 retinitis Pigmentosa using iPSC-derived retinal organoids. Stem Cell Rep. (2020) 15:67–79. doi: 10.1016/j.stemcr.2020.05.007
137. Zerti D, Molina MM, Dorgau B, Mearns S, Bauer R, Al-Aama J, et al. IGFBPs mediate IGF-1’s functions in retinal lamination and photoreceptor development during pluripotent stem cell differentiation to retinal organoids. Stem Cells. (2021b) 39:458–66. doi: 10.1002/stem.3331
138. Kuwahara A, Ozone C, Nakano T, Saito K, Eiraku M, Sasai Y. Generation of a ciliary margin-like stem cell niche from self-organizing human retinal tissue. Nat Commun. (2015) 6:6286. doi: 10.1038/ncomms7286
139. Capowski EE, Samimi K, Mayerl SJ, Phillips MJ, Pinilla I, Howden SE, et al. Reproducibility and staging of 3D human retinal organoids across multiple pluripotent stem cell lines. Development. (2018) 146:dev.171686. doi: 10.1242/dev.171686
140. Yamasaki S, Kuwahara A, Kishino A, Kimura T, Takahashi M, Mandai M. Addition of Chk1 inhibitor and BMP4 cooperatively promotes retinal tissue formation in self-organizing human pluripotent stem cell differentiation culture. Regen Ther. (2022) 19:24–34. doi: 10.1016/j.reth.2021.12.003
141. Chichagova V, Hilgen G, Ghareeb A, Georgiou M, Carter M, Sernagor E, et al. Human iPSC differentiation to retinal organoids in response to IGF1 and BMP4 activation is line- and method-dependent. Stem Cells Dayt Ohio. (2020) 38:195–201. doi: 10.1002/stem.3116
142. Kuwahara A, Yamasaki S, Mandai M, Watari K, Matsushita K, Fujiwara M, et al. Preconditioning the initial state of feeder-free human pluripotent stem cells promotes self-formation of three-dimensional retinal tissue. Sci Rep. (2019) 9:18936. doi: 10.1038/s41598-019-55130-w
143. Saha A, Capowski E, Zepeda MAF, Nelson EC, Gamm DM, Sinha R. Cone photoreceptors in human stem cell-derived retinal organoids demonstrate intrinsic light responses that mimic those of primate fovea. Cell Stem Cell. (2022) 29:460–471.e3. doi: 10.1016/j.stem.2022.01.002
144. Chew SH, Martinez C, Chirco KR, Kandoi S, Lamba DA. Timed notch inhibition drives photoreceptor fate specification in human retinal organoids. Invest Ophth Vis Sci. (2022) 63:12. doi: 10.1167/iovs.63.10.12
145. Zerti D, Dorgau B, Felemban M, Ghareeb AE, Yu M, Ding Y, et al. Developing a simple method to enhance the generation of cone and rod photoreceptors in pluripotent stem cell-derived retinal organoids. Stem Cells. (2020) 38:45–51. doi: 10.1002/stem.3082
146. Kelley MW, Turner JK, Reh TA. Retinoic acid promotes differentiation of photoreceptors in vitro. Development. (1994) 120:2091–102. doi: 10.1242/dev.120.8.2091
147. Chichagova V, Dorgau B, Felemban M, Georgiou M, Armstrong L, Lako M. Differentiation of retinal organoids from human pluripotent stem cells. Curr Protoc Stem Cell Biol. (2019) 50:e95. doi: 10.1002/cpsc.95
148. Isla-Magrané H, Zufiaurre-Seijo M, García-Arumí J, Duarri A. All-trans retinoic acid modulates pigmentation, neuroretinal maturation, and corneal transparency in human multiocular organoids. Stem Cell Res Ther. (2022) 13:376. doi: 10.1186/s13287-022-03053-1
149. Sanjurjo-Soriano C, Erkilic N, Damodar K, Boukhaddaoui H, Diakatou M, Garita-Hernandez M, et al. Retinoic acid delays initial photoreceptor differentiation and results in a highly structured mature retinal organoid. Stem Cell Res Ther. (2022) 13:478. doi: 10.1186/s13287-022-03146-x
150. Kaya KD, Chen HY, Brooks MJ, Kelley RA, Shimada H, Nagashima K, et al. Transcriptome-based molecular staging of human stem cell-derived retinal organoids uncovers accelerated photoreceptor differentiation by 9-cis retinal. Mol Vis. (2019) 25:663–78.31814692
151. Kelley RA, Chen HY, Swaroop A, Li T. Accelerated development of rod photoreceptors in retinal organoids derived from human pluripotent stem cells by supplementation with 9-cis retinal. Star Protoc. (2020) 1:100033. doi: 10.1016/j.xpro.2020.100033
152. Bell E, Muñoz-Sanjuán I, Altmann CR, Vonica A, Brivanlou AH. Cell fate specification and competence by Coco, a maternal BMP, TGFβ and Wnt inhibitor. Development. (2003) 130:1381–9. doi: 10.1242/dev.00344
153. Kruczek K, Gonzalez-Cordero A, Goh D, Naeem A, Jonikas M, Blackford SJI, et al. Differentiation and transplantation of embryonic stem cell-derived cone photoreceptors into a mouse model of end-stage retinal degeneration. Stem Cell Rep. (2017) 8:1659–74. doi: 10.1016/j.stemcr.2017.04.030
154. McNerney C, Johnston RJ. Thyroid hormone signaling specifies cone photoreceptor subtypes during eye development: insights from model organisms and human stem cell-derived retinal organoids. Vitam Horm. (2021) 116:51–90. doi: 10.1016/bs.vh.2021.03.001
155. Chen M, Mao X, Huang D, Jing J, Zou W, Mao P, et al. Somatostatin signalling promotes the differentiation of rod photoreceptors in human pluripotent stem cell-derived retinal organoid. Cell Prolif. (2022) 55:e13254. doi: 10.1111/cpr.13254
156. Völkner M, Zschätzsch M, Rostovskaya M, Overall RW, Busskamp V, Anastassiadis K, et al. Retinal organoids from pluripotent stem cells efficiently recapitulate retinogenesis. Stem Cell Rep. (2016) 6:525–38. doi: 10.1016/j.stemcr.2016.03.001
157. Ovando-Roche P, West EL, Branch MJ, Sampson RD, Fernando M, Munro P, et al. Use of bioreactors for culturing human retinal organoids improves photoreceptor yields. Stem Cell Res Ther. (2018) 9:156. doi: 10.1186/s13287-018-0907-0
158. DiStefano TJ, Chen HY, Panebianco C, Kaya KD, Brooks MJ, Gieser L, et al. Accelerated and improved differentiation of retinal organoids from pluripotent stem cells in rotating-wall vessel bioreactors. Stem Cell Rep. (2021) 16:224. doi: 10.1016/j.stemcr.2020.12.006
159. Khalili S, Ballios BG, Belair-Hickey J, Donaldson L, Liu J, Coles BLK, et al. Induction of rod vs. cone photoreceptor-specific progenitors from retinal precursor cells. Stem Cell Res. (2018) 33:215–27. doi: 10.1016/j.scr.2018.11.005
160. Tay HG, Andre H, Chrysostomou V, Adusumalli S, Guo J, Ren X, et al. Photoreceptor laminin drives differentiation of human pluripotent stem cells to photoreceptor progenitors that partially restore retina function. Mol Ther. (2023) 31:825–46. doi: 10.1016/j.ymthe.2022.12.012
161. Libby RT, Champliaud M-F, Claudepierre T, Xu Y, Gibbons EP, Koch M, et al. Laminin expression in adult and developing retinae: evidence of two novel CNS laminins. J Neurosci. (2000) 20:6517–28. doi: 10.1523/jneurosci.20-17-06517.2000
162. Zenker M, Aigner T, Wendler O, Tralau T, Müntefering H, Fenski R, et al. Human laminin β2 deficiency causes congenital nephrosis with mesangial sclerosis and distinct eye abnormalities. Hum Mol Genet. (2004) 13:2625–32. doi: 10.1093/hmg/ddh284
163. Dorgau B, Felemban M, Sharpe A, Bauer R, Hallam D, Steel DH, et al. Laminin γ3 plays an important role in retinal lamination, photoreceptor organisation and ganglion cell differentiation. Cell Death Dis. (2018) 9:615. doi: 10.1038/s41419-018-0648-0
164. Zhang Y, Pak C, Han Y, Ahlenius H, Zhang Z, Chanda S, et al. Rapid single-step induction of functional neurons from human pluripotent stem cells. Neuron. (2013) 78:785–98. doi: 10.1016/j.neuron.2013.05.029
165. Otsuka Y, Imamura K, Oishi A, Kondo T, Suga M, Yada Y, et al. One-step induction of photoreceptor-like cells from human iPSCs by delivering transcription factors. Iscience. (2022) 25:103987. doi: 10.1016/j.isci.2022.103987
166. Connor B, Firmin E, McCaughey-Chapman A, Monk R, Lee K, Liot S, et al. Conversion of adult human fibroblasts into neural precursor cells using chemically modified mRNA. Heliyon. (2018) 4:e00918. doi: 10.1016/j.heliyon.2018.e00918
167. Hollyfield JG, Witkovsky P. Pigmented retinal epithelium involvement in photoreceptor development and function. J Exp Zoöl. (1974) 189:357–77. doi: 10.1002/jez.1401890309
168. Raymond SM, Jackson IJ. The retinal pigmented epithelium is required for development and maintenance of the mouse neural retina. Curr Biol. (1995) 5:1286–95. doi: 10.1016/s0960-9822(95)00255-7
169. Dutt K, Douglas P, Cao Y. RPE-secreted factors: influence differentiation in human retinal cell line in dose- and density-dependent manner. J Ocul Biol Dis Inform. (2010) 3:144–60. doi: 10.1007/s12177-011-9076-4
170. Gaur VP, Liu Y, Turner JE. RPE conditioned medium stimulates photoreceptor cell survival, neurite outgrowth and differentiation in vitro. Exp Eye Res. (1992) 54:645–59. doi: 10.1016/0014-4835(92)90020-s
171. Sheedlo HJ, Li L, Turner JE. Effects of RPE-cell factors secreted from permselective fibers on retinal cells in vitro. Brain Res. (1992) 587:327–37. doi: 10.1016/0006-8993(92)91015-7
172. Sheedlo HJ, Fan W, Li L, Turner JE. Retinal pigment epithelial cell support of photoreceptor survival in vitro. Vitro Cell Dev Biol Anim. (1995) 31:330–3. doi: 10.1007/bf02634278
173. Sheedlo HJ, Turner JE. Effects of retinal pigment epithelial cell-secreted factors on neonatal rat retinal explant progenitor cells. J Neurosci Res. (1996) 44:519–31. doi: 10.1002/(sici)1097-4547(19960615)44:6%3C519::aid-jnr2%3E3.0.co;2-e
174. Li M, Sakaguchi DS. Inhibition of integrin-mediated adhesion and signaling disrupts retinal development. Dev Biol. (2004) 275:202–14. doi: 10.1016/j.ydbio.2004.08.005
175. Ha T, Moon KH, Dai L, Hatakeyama J, Yoon K, Park H-S, et al. The retinal pigment epithelium is a notch signaling niche in the mouse retina. Cell Rep. (2017) 19:351–63. doi: 10.1016/j.celrep.2017.03.040
176. Yang X, Chung J-Y, Rai U, Esumi N. Cadherins in the retinal pigment epithelium (RPE) revisited: p-cadherin is the highly dominant cadherin expressed in human and mouse RPE in vivo. PLoS One. (2018) 13:e0191279. doi: 10.1371/journal.pone.0191279
177. Papal S, Monti CE, Tennison ME, Swaroop A. Molecular dissection of cone photoreceptor-enriched genes encoding transmembrane and secretory proteins. J Neurosci Res. (2019) 97:16–28. doi: 10.1002/jnr.24329
178. Achberger K, Probst C, Haderspeck J, Bolz S, Rogal J, Chuchuy J, et al. Merging organoid and organ-on-a-chip technology to generate complex multi-layer tissue models in a human retina-on-a-chip platform. Elife. (2019) 8:e46188. doi: 10.7554/elife.46188
179. Schnichels S, Paquet-Durand F, Löscher M, Tsai T, Hurst J, Joachim SC, et al. Retina in a dish: cell cultures, retinal explants and animal models for common diseases of the retina. Prog Retin Eye Res. (2021) 81:100880. doi: 10.1016/j.preteyeres.2020.100880
180. German OL, Buzzi E, Rotstein NP, Rodríguez-Boulan E, Politi LE. Retinal pigment epithelial cells promote spatial reorganization and differentiation of retina photoreceptors. J Neurosci Res. (2008) 86:3503–14. doi: 10.1002/jnr.21813
181. Amirpour N, Nasr-Esfahani MH, Esfandiari E, Razavi S, Karamali F. Comparing three methods of co-culture of retinal pigment epithelium with progenitor cells derived human embryonic stem cells. Int J Prev Med. (2013) 4:1243–50.24404357
182. Singh D, Chen X, Xia T, Ghiassi-Nejad M, Tainsh L, Adelman RA, et al. Partially differentiated neuroretinal cells promote maturation of the retinal pigment epithelium. Invest Ophth Vis Sci. (2020) 61:9. doi: 10.1167/iovs.61.13.9
183. Chen X, Singh D, Adelman RA, Rizzolo LJ. Unstimulated, Serum-free cultures of retinal pigment epithelium excrete large mounds of drusen-like deposits. Curr Eye Res. (2020) 45:1390–4. doi: 10.1080/02713683.2020.1740744
184. Akhtar T, Xie H, Khan MI, Zhao H, Bao J, Zhang M, et al. Accelerated photoreceptor differentiation of hiPSC-derived retinal organoids by contact co-culture with retinal pigment epithelium. Stem Cell Res. (2019) 39:101491. doi: 10.1016/j.scr.2019.101491
185. Radtke ND, Aramant RB, Petry HM, Green PT, Pidwell DJ, Seiler MJ. Vision improvement in retinal degeneration patients by implantation of retina together with retinal pigment epithelium. Am J Ophthalmol. (2008) 146:172–182.e1. doi: 10.1016/j.ajo.2008.04.009
186. Thomas BB, Lin B, Martinez-Camarillo JC, Zhu D, McLelland BT, Nistor G, et al. Co-grafts of human embryonic stem cell derived retina organoids and retinal pigment epithelium for retinal reconstruction in immunodeficient retinal degenerate royal college of surgeons rats. Front Neurosci-Switz. (2021a) 15:752958. doi: 10.3389/fnins.2021.752958
187. Léveillard T, Klipfel L. Mechanisms underlying the visual benefit of cell transplantation for the treatment of retinal degenerations. Int J Mol Sci. (2019) 20:557. doi: 10.3390/ijms20030557
188. Gouras P, Du J, Kjeldbye H, Yamamoto S, Zack DJ. Long-term photoreceptor transplants in dystrophic and normal mouse retina. Investig Ophthalmol Vis Sci. (1994) 35:3145–53.
189. Akimoto M, Cheng H, Zhu D, Brzezinski JA, Khanna R, Filippova E, et al. Targeting of GFP to newborn rods by Nrl promoter and temporal expression profiling of flow-sorted photoreceptors. Proc Natl Acad Sci. (2006) 103:3890–5. doi: 10.1073/pnas.0508214103
190. Decembrini S, Martin C, Sennlaub F, Chemtob S, Biel M, Samardzija M, et al. Cone genesis tracing by the Chrnb4-EGFP mouse line: evidences of cellular material fusion after cone precursor transplantation. Mol Ther. (2017) 25:634–53. doi: 10.1016/j.ymthe.2016.12.015
191. Gonzalez-Cordero A, Kruczek K, Naeem A, Fernando M, Kloc M, Ribeiro J, et al. Recapitulation of human retinal development from human pluripotent stem cells generates transplantable populations of cone photoreceptors. Stem Cell Rep. (2017) 9:820–37. doi: 10.1016/j.stemcr.2017.07.022
192. Welby E, Lakowski J, Foggia VD, Budinger D, Gonzalez-Cordero A, Lun ATL, et al. Isolation and comparative transcriptome analysis of human fetal and iPSC-derived cone photoreceptor cells. Stem Cell Rep. (2017) 9:1898–915. doi: 10.1016/j.stemcr.2017.10.018
193. Nickerson PEB, Ortin-Martinez A, Wallace VA. Material exchange in photoreceptor transplantation: updating our understanding of donor/host communication and the future of cell engraftment science. Front Neural Circuit. (2018) 12:17. doi: 10.3389/fncir.2018.00017
194. Collin J, Zerti D, Queen R, Santos-Ferreira T, Bauer R, Coxhead J, et al. CRX expression in pluripotent stem cell-derived photoreceptors marks a transplantable subpopulation of early cones. Stem Cells. (2019) 37:609–22. doi: 10.1002/stem.2974
195. Lam PT, Gutierrez C, Rio-Tsonis KD, Robinson ML. Generation of a retina reporter hiPSC line to label progenitor, ganglion, and photoreceptor cell types. Transl Vis Sci Technol. (2020) 9:21. doi: 10.1167/tvst.9.3.21
196. Guan Y, Wang Y, Zheng D, Xie B, Xu P, Gao G, et al. Generation of an RCVRN-eGFP reporter hiPSC line by CRISPR/Cas9 to monitor photoreceptor cell development and facilitate the cell enrichment for transplantation. Front Cell Dev Biol. (2022) 10:870441. doi: 10.3389/fcell.2022.870441
197. Nazlamova L, Cassidy E-J, Sowden JC, Lotery A, Lakowski J. Generation of a cone photoreceptor-specific GNGT2 reporter line in human pluripotent stem cells. Stem Cells. (2022) 40:190–203. doi: 10.1093/stmcls/sxab015
198. Gonzalez-Cordero A, West EL, Pearson RA, Duran Y, Carvalho LS, Chu CJ, et al. Photoreceptor precursors derived from three-dimensional embryonic stem cell cultures integrate and mature within adult degenerate retina. Nat Biotechnol. (2013) 31:nbt.2643. doi: 10.1038/nbt.2643
199. Markus A, Shamul A, Chemla Y, Farah N, Shaham L, Goldstein RS, et al. An optimized protocol for generating labeled and transplantable photoreceptor precursors from human embryonic stem cells. Exp Eye Res. (2018) 180:29–38. doi: 10.1016/j.exer.2018.11.013
200. Ribeiro J, Procyk CA, West EL, O’Hara-Wright M, Martins MF, Khorasani MM, et al. Restoration of visual function in advanced disease after transplantation of purified human pluripotent stem cell-derived cone photoreceptors. Cell Rep. (2021) 35:109022. doi: 10.1016/j.celrep.2021.109022
201. McClements ME, Steward H, Atkin W, Goode EA, Gándara C, Chichagova V, et al. Tropism of AAV vectors in photoreceptor-like cells of human iPSC-derived retinal organoids. Transl Vis Sci Technol. (2022) 11:3. doi: 10.1167/tvst.11.4.3
202. Lakowski J, Han Y-T, Pearson RA, Gonzalez-Cordero A, West EL, Gualdoni S, et al. Effective transplantation of photoreceptor precursor cells selected via cell surface antigen expression. Stem Cells. (2011) 29:1391–404. doi: 10.1002/stem.694
203. Seiler MJ, Aramant RB. Cell replacement and visual restoration by retinal sheet transplants. Prog Retin Eye Res. (2012) 31:661–87. doi: 10.1016/j.preteyeres.2012.06.003
204. Barnea-Cramer AO, Wang W, Lu S-J, Singh MS, Luo C, Huo H, et al. Function of human pluripotent stem cell-derived photoreceptor progenitors in blind mice. Sci Rep. (2016) 6:29784. doi: 10.1038/srep29784
205. Shirai H, Mandai M, Matsushita K, Kuwahara A, Yonemura S, Nakano T, et al. Transplantation of human embryonic stem cell-derived retinal tissue in two primate models of retinal degeneration. Proc Natl Acad Sci. (2016) 113:E81–90. doi: 10.1073/pnas.1512590113
206. Chao JR, Lamba DA, Klesert TR, Torre AL, Hoshino A, Taylor RJ, et al. Transplantation of human embryonic stem cell-derived retinal cells into the subretinal space of a non-human primate. Transl Vis Sci Technol. (2017) 6:4. doi: 10.1167/tvst.6.3.4
207. Mandai M, Fujii M, Hashiguchi T, Sunagawa GA, Ito S, Sun J, et al. iPSC-Derived retina transplants improve vision in rd1 end-stage retinal-degeneration mice. Stem Cell Rep. (2017a) 8:69–83. doi: 10.1016/j.stemcr.2016.12.008
208. Lingam S, Liu Z, Yang B, Wong W, Parikh BH, Ong JY, et al. cGMP-grade human iPSC-derived retinal photoreceptor precursor cells rescue cone photoreceptor damage in non-human primates. Stem Cell Res Ther. (2021) 12:464. doi: 10.1186/s13287-021-02539-8
209. Lamba DA, Gust J, Reh TA. Transplantation of human embryonic stem cell-derived photoreceptors restores some visual function in crx-deficient mice. Cell Stem Cell. (2009) 4:73–9. doi: 10.1016/j.stem.2008.10.015
210. McLelland BT, Lin B, Mathur A, Aramant RB, Thomas BB, Nistor G, et al. Transplanted hESC-derived retina organoid sheets differentiate. Integrate, and Improve Visual Function in Retinal Degenerate Rats. Invest Ophth Vis Sci. (2018) 59:2586–603. doi: 10.1167/iovs.17-23646
211. Zerti D, Hilgen G, Dorgau B, Collin J, Ader M, Armstrong L, et al. Transplanted pluripotent stem cell-derived photoreceptor precursors elicit conventional and unusual light responses in mice with advanced retinal degeneration. Stem Cells. (2021a) 39:882–96. doi: 10.1002/stem.3365
212. Pearson RA, Gonzalez-Cordero A, West EL, Ribeiro JR, Aghaizu N, Goh D, et al. Donor and host photoreceptors engage in material transfer following transplantation of post-mitotic photoreceptor precursors. Nat Commun. (2016) 7:13029. doi: 10.1038/ncomms13029
213. Santos-Ferreira T, Llonch S, Borsch O, Postel K, Haas J, Ader M. Retinal transplantation of photoreceptors results in donor–host cytoplasmic exchange. Nat Commun. (2016) 7:ncomms13028. doi: 10.1038/ncomms13028
214. Singh MS, Balmer J, Barnard AR, Aslam SA, Moralli D, Green CM, et al. Transplanted photoreceptor precursors transfer proteins to host photoreceptors by a mechanism of cytoplasmic fusion. Nat Commun. (2016) 7:13537. doi: 10.1038/ncomms13537
215. Kalargyrou AA, Basche M, Hare A, West EL, Smith AJ, Ali RR, et al. Nanotube-like processes facilitate material transfer between photoreceptors. EMBO Rep. (2021) 22:e53732. doi: 10.15252/embr.202153732
216. Ortin-Martinez A, Yan NE, Tsai ELS, Comanita L, Gurdita A, Tachibana N, et al. Photoreceptor nanotubes mediate the in vivo exchange of intracellular material. EMBO J. (2021) 40:e107264. doi: 10.15252/embj.2020107264
217. Waldron PV, Marco FD, Kruczek K, Ribeiro J, Graca AB, Hippert C, et al. Transplanted donor- or stem cell-derived cone photoreceptors can both integrate and undergo material transfer in an environment-dependent manner. Stem Cell Rep. (2018) 10:406–21. doi: 10.1016/j.stemcr.2017.12.008
218. Heisterkamp P, Borsch O, Lezama ND, Gasparini S, Fathima A, Carvalho LS, et al. Evidence for endogenous exchange of cytoplasmic material between a subset of cone and rod photoreceptors within the adult mammalian retina via direct cell-cell connections. Exp Eye Res. (2022) 219:109033. doi: 10.1016/j.exer.2022.109033
219. Dong L-F, Rohlena J, Zobalova R, Nahacka Z, Rodriguez A-M, Berridge MV, et al. Mitochondria on the move: horizontal mitochondrial transfer in disease and health. J Cell Biol. (2023) 222:e202211044. doi: 10.1083/jcb.202211044
220. Yao Y, Fan X-L, Jiang D, Zhang Y, Li X, Xu Z-B, et al. Connexin 43-mediated mitochondrial transfer of iPSC-MSCs alleviates asthma inflammation. Stem Cell Rep. (2018) 11:1120–35. doi: 10.1016/j.stemcr.2018.09.012
221. Chinnery HR, Keller KE. Tunneling nanotubes and the eye: intercellular communication and implications for ocular health and disease. BioMed Res Int. (2020) 2020:7246785. doi: 10.1155/2020/7246785
222. Qin Y, Jiang X, Yang Q, Zhao J, Zhou Q, Zhou Y. The functions, methods, and mobility of mitochondrial transfer between cells. Front Oncol. (2021) 11:672781. doi: 10.3389/fonc.2021.672781
223. Chen BS, Harvey JP, Gilhooley MJ, Jurkute N, Yu-Wai-Man P. Mitochondria and the eye—manifestations of mitochondrial diseases and their management. Eye. (2023) 37(12):2416–25. doi: 10.1038/s41433-023-02523-x
224. Caicedo A, Aponte PM, Cabrera F, Hidalgo C, Khoury M. Artificial mitochondria transfer: current challenges, advances, and future applications. Stem Cells Int. (2017) 2017:1–23. doi: 10.1155/2017/7610414
225. Barrera-Paez JD, Moraes CT. Mitochondrial genome engineering coming-of-age. Trends Genet. (2022) 38:869–80. doi: 10.1016/j.tig.2022.04.011
226. Lefevere E, Toft-Kehler AK, Vohra R, Kolko M, Moons L, Hove IV. Mitochondrial dysfunction underlying outer retinal diseases. Mitochondrion. (2017) 36:66–76. doi: 10.1016/j.mito.2017.03.006
227. Eells JT. Mitochondrial dysfunction in the aging retina. Biology (Basel). (2019) 8:31. doi: 10.3390/biology8020031
228. Eberle D, Santos-Ferreira T, Grahl S, Ader M. Subretinal transplantation of MACS purified photoreceptor precursor cells into the adult mouse retina. J Vis Exp (2014) (84):e50932. doi: 10.3791/50932
229. Gagliardi G, M’Barek KB, Chaffiol A, Slembrouck-Brec A, Conart J-B, Nanteau C, et al. Characterization and transplantation of CD73-positive photoreceptors isolated from human iPSC-derived retinal organoids. Stem Cell Rep. (2018) 11:665–80. doi: 10.1016/j.stemcr.2018.07.005
230. Ansari AM, Ahmed AK, Matsangos AE, Lay F, Born LJ, Marti G, et al. Cellular GFP toxicity and immunogenicity: potential confounders in in vivo cell tracking experiments. Stem Cell Rev Rep. (2016) 12:553–9. doi: 10.1007/s12015-016-9670-8
231. Koso H, Minami C, Tabata Y, Inoue M, Sasaki E, Satoh S, et al. CD73, a novel cell surface antigen that characterizes retinal photoreceptor precursor cells. Invest Ophthalmol Visual Sci. (2009) 50:5411–8. doi: 10.1167/iovs.08-3246
232. Lakowski J, Welby E, Budinger D, Marco FD, Foggia VD, Bainbridge JWB, et al. Isolation of human photoreceptor precursors via a cell surface marker panel from stem cell-derived retinal organoids and fetal retinae. Stem Cells Dayt Ohio. (2018) 36:709–22. doi: 10.1002/stem.2775
233. Santos-Ferreira T, Herbig M, Otto O, Carido M, Karl MO, Michalakis S, et al. Morpho-rheological fingerprinting of rod photoreceptors using real-time deformability cytometry. Cytom A. (2019) 95:1145–57. doi: 10.1002/cyto.a.23798
234. Stone NE, Voigt AP, Mullins RF, Sulchek T, Tucker BA. Microfluidic processing of stem cells for autologous cell replacement. Stem Cells Transl Med. (2021) 10:1384–93. doi: 10.1002/sctm.21-0080
235. Herbig M, Tessmer K, Nötzel M, Nawaz AA, Santos-Ferreira T, Borsch O, et al. Label-free imaging flow cytometry for analysis and sorting of enzymatically dissociated tissues. Sci Rep. (2022) 12:963. doi: 10.1038/s41598-022-05007-2
236. West EL, Pearson RA, Tschernutter M, Sowden JC, MacLaren RE, Ali RR. Pharmacological disruption of the outer limiting membrane leads to increased retinal integration of transplanted photoreceptor precursors. Exp Eye Res. (2008) 86:601–11. doi: 10.1016/j.exer.2008.01.004
237. Barber AC, Hippert C, Duran Y, West EL, Bainbridge JWB, Warre-Cornish K, et al. Repair of the degenerate retina by photoreceptor transplantation. Proc Natl Acad Sci. (2013) 110:354–9. doi: 10.1073/pnas.1212677110
238. Jones BW, Watt CB, Frederick JM, Baehr W, Chen C, Levine EM, et al. Retinal remodeling triggered by photoreceptor degenerations. J Comp Neurol. (2003) 464:1–16. doi: 10.1002/cne.10703
239. West EL, Pearson RA, Duran Y, Gonzalez-Cordero A, Maclaren RE, Smith AJ, et al. Manipulation of the recipient retinal environment by ectopic expression of neurotrophic growth factors can improve transplanted photoreceptor integration and survival. Cell Transplant. (2012) 21:871–87. doi: 10.3727/096368911x623871
240. Light JG, Fransen JW, Adekunle AN, Adkins A, Pangeni G, Loudin J, et al. Inner retinal preservation in rat models of retinal degeneration implanted with subretinal photovoltaic arrays. Exp Eye Res. (2014) 128:34–42. doi: 10.1016/j.exer.2014.09.004
241. Wang W, Lee SJ, Scott PA, Lu X, Emery D, Liu Y, et al. Two-step reactivation of dormant cones in retinitis Pigmentosa. Cell Rep. (2016) 15:372–85. doi: 10.1016/j.celrep.2016.03.022
242. Kashani AH, Lebkowski JS, Hinton DR, Zhu D, Faynus MA, Chen S, et al. Survival of an HLA-mismatched, bioengineered RPE implant in dry age-related macular degeneration. Stem Cell Rep. (2022) 17:448–58. doi: 10.1016/j.stemcr.2022.01.001
243. Sugita S, Mandai M, Hirami Y, Takagi S, Maeda T, Fujihara M, et al. HLA-matched allogeneic iPS cells-derived RPE transplantation for macular degeneration. J Clin Med. (2020) 9:2217. doi: 10.3390/jcm9072217
244. Koga K, Wang B, Kaneko S. Current status and future perspectives of HLA-edited induced pluripotent stem cells. Inflamm Regen. (2020) 40:23. doi: 10.1186/s41232-020-00132-9
245. Riolobos L, Hirata RK, Turtle CJ, Wang P-R, Gornalusse GG, Zavajlevski M, et al. HLA engineering of human pluripotent stem cells. Mol Ther. (2013) 21:1232–41. doi: 10.1038/mt.2013.59
246. Rong Z, Wang M, Hu Z, Stradner M, Zhu S, Kong H, et al. An effective approach to prevent immune rejection of human ESC-derived allografts. Cell Stem Cell. (2014) 14:121–30. doi: 10.1016/j.stem.2013.11.014
247. Gornalusse GG, Hirata RK, Funk SE, Riolobos L, Lopes VS, Manske G, et al. HLA-E-expressing pluripotent stem cells escape allogeneic responses and lysis by NK cells. Nat Biotechnol. (2017) 35:765–72. doi: 10.1038/nbt.3860
248. Deuse T, Hu X, Gravina A, Wang D, Tediashvili G, De C, et al. Hypoimmunogenic derivatives of induced pluripotent stem cells evade immune rejection in fully immunocompetent allogeneic recipients. Nat Biotechnol. (2019) 37:252–8. doi: 10.1038/s41587-019-0016-3
249. Turner M, Leslie S, Martin NG, Peschanski M, Rao M, Taylor CJ, et al. Toward the development of a global induced pluripotent stem cell library. Cell Stem Cell. (2013) 13:382–4. doi: 10.1016/j.stem.2013.08.003
250. Nakatsuji N, Nakajima F, Tokunaga K. HLA-haplotype banking and iPS cells. Nat Biotechnol. (2008) 26:739–40. doi: 10.1038/nbt0708-739
251. Taylor CJ, Peacock S, Chaudhry AN, Bradley JA, Bolton EM. Generating an iPSC bank for HLA-matched tissue transplantation based on known donor and recipient HLA types. Cell Stem Cell. (2012) 11:147–52. doi: 10.1016/j.stem.2012.07.014
252. Pappas DJ, Gourraud P-A, Gall CL, Laurent J, Trounson A, DeWitt N, et al. Proceedings: human leukocyte antigen haplo-homozygous induced pluripotent stem cell haplobank modeled after the California population: evaluating matching in a multiethnic and admixed population. Stem Cells Transl Med. (2015) 4:413–8. doi: 10.5966/sctm.2015-0052
Keywords: retinal degeneration, regenerative medicine, human pluripotent stem cells, photoreceptors, cell therapy, retinal organoids
Citation: Beaver D and Limnios IJ (2023) A treatment within sight: challenges in the development of stem cell-derived photoreceptor therapies for retinal degenerative diseases. Front. Transplant. 2:1130086. doi: 10.3389/frtra.2023.1130086
Received: 22 December 2022; Accepted: 7 September 2023;
Published: 29 September 2023.
Edited by:
Ragai Mitry, King’s College London, United KingdomReviewed by:
Vikram Sabapathy, University of Virginia, United StatesHwee Goon Tay, Duke-NUS Medical School, Singapore
© 2023 Beaver and Limnios. This is an open-access article distributed under the terms of the Creative Commons Attribution License (CC BY). The use, distribution or reproduction in other forums is permitted, provided the original author(s) and the copyright owner(s) are credited and that the original publication in this journal is cited, in accordance with accepted academic practice. No use, distribution or reproduction is permitted which does not comply with these terms.
*Correspondence: Ioannis Jason Limnios aWxpbW5pb3NAYm9uZC5lZHUuYXU=