- Department of General Surgery, Boston Children’s Hospital, Boston, MA, United States
Successful organ transplantation provides an opportunity to extend the lives of patients with end-stage organ failure. Selectively suppressing the donor-specific alloimmune response, however, remains challenging without the continuous use of non-specific immunosuppressive medications, which have multiple adverse effects including elevated risks of infection, chronic kidney injury, cardiovascular disease, and cancer. Efforts to promote allograft tolerance have focused on manipulating the adaptive immune response, but long-term allograft survival rates remain disappointing. In recent years, the innate immune system has become an attractive therapeutic target for the prevention and treatment of transplant organ rejection. Indeed, contemporary studies demonstrate that innate immune cells participate in both the initial alloimmune response and chronic allograft rejection and undergo non-permanent functional reprogramming in a phenomenon termed “trained immunity.” Several types of innate immune cells are currently under investigation as potential therapeutics in transplantation, including myeloid-derived suppressor cells, dendritic cells, regulatory macrophages, natural killer cells, and innate lymphoid cells. In this review, we discuss the features and functions of these cell types, with a focus on their role in the alloimmune response. We examine their potential application as therapeutics to prevent or treat allograft rejection, as well as challenges in their clinical translation and future directions for investigation.
1. Introduction
Solid organ transplantation is the only curative treatment for end-stage organ failure. While short-term patient and allograft survival have significantly improved over time, long-term allograft survival rates remain stagnant (1, 2). To prevent rejection, patients are reliant on continuous immunosuppression with medications such as calcineurin inhibitors, corticosteroids, and anti-proliferative agents. Though these regimens are critical for allograft survival, they are associated with significant morbidity including greater susceptibility to infections, chronic kidney injury, cardiovascular disease, and cancer (2, 3). New strategies to reduce or eliminate the use of these medications are needed.
Though previous efforts to promote tolerance have focused largely on the adaptive immune response, interest in innate immune cells as therapeutic targets to promote tolerance has grown, especially given recent findings that these cells can undergo non-permanent epigenetic and metabolic changes that prime their future activity in a phenomenon termed “trained immunity” (4–6). While all these cell types have been identified in vivo, they are not present in sufficient numbers during the alloimmune response to exert significant immunosuppressive effects, and thus must be artificially activated in the recipient, engineered ex vivo, or adoptively transferred following ex vivo expansion. In this review, we first describe these innate immune cell populations under investigation, including their endogenous features and functions, with an emphasis on their role in alloimmunity. We then discuss preclinical and clinical studies in which these cell types are modified ex vivo to prevent or treat allograft rejection, supporting their application as cellular therapeutics. Finally, we summarize challenges to their clinical use and future directions for investigation.
2. Tolerogenic innate immune cells and their immunomodulatory functions in vivo
2.1. Myeloid-derived suppressor cells
Myeloid-derived suppressor cells (MDSCs) are leukocytes that develop from immature myeloid cells in response to inflammation (7). They have been divided into two subtypes, namely polymorphonuclear MDSCs (PMN-MDCs) and monocytic MDSCs (M-MDSCs), based on their resemblance in morphology and phenotype to polymorphonuclear cells and monocytes, respectively (8). Murine PMN-MDSCs are identified as CD11b+ Gr-1+ Ly6Clow Ly6Ghigh cells, while M-MDSCs are defined as CD11b+ Gr-1+ Ly6Chigh Ly6G− cells (9, 10). Human M-MDSCs are designated as CD11b+ CD14+ CD15− HLA-DRlow/− cells, while PMN-MDSCs are defined as CD11b+ CD15+ CD14− cells (8, 9). The ratio of these two subtypes varies by inflammatory condition and while the optimal proportions of each to effectively suppress the alloimmune response remains unclear, evidence suggests a more critical role for M-MDSCs in tolerance induction (11–14). MDSCs were first described in cancer biology, in which they play a deleterious role suppressing anti-tumor T cell responses and creating an immunosuppressive milieu for unopposed tumor growth (15–18). Additional research has illustrated their involvement in other inflammatory conditions, including autoimmunity, trauma, sepsis, and allograft rejection (7, 11, 19–23). MDSCs interact with their primary targets, natural killer (NK) cells and effector T cells, through cell-cell interactions and signaling with soluble factors (22, 24, 25). Firstly, MDSCs express Programmed Cell Death Ligand 1 (PD-L1), activating regulatory T cells (Tregs) and suppressing activated T cells by binding their cognate Programmed Cell Death Protein 1 (PD-1), which has been shown to be necessary for their immunosuppressive effect in a murine model of islet transplantation (14, 26–28). Colony stimulating factor 1 receptor (CSF1R) is also critical to MDSC functions, binding colony stimulating factor (CSF) to regulate their expansion and migration (29, 30). CSF/CSF1R signaling has been shown to recruit MDSCs to the tumor microenvironment and promote upregulation of PD-L1, reducing the efficacy of chemotherapy, radiation, and checkpoint immunotherapy in various cancers (31–33).
MDSCs also secrete soluble factors that modulate immune responses. For example, they produce nitric oxide (NO) via inducible nitric oxide synthase (iNOS), which inhibits the expansion, differentiation, and effector functions of NK cells, B cells, and T cells (14, 21, 22, 34–39). The synthesis of NO simultaneously depletes L-arginine, a critical substrate for T cell expansion (38, 39). MDSCs also consume L-arginine through the expression of arginase-1 (Arg-1), producing urea and ornithine (34). Furthermore, MDSCs express heme oxigenase-1 (HO-1), which suppresses T cells and mediates delays in skin graft rejection (40). MDSCs produce transforming growth factor-β (TGF-β) and interleukin (IL)-10 in response to interferon-γ (IFN-γ) signaling, which results in downstream activation of Tregs (18, 36). Finally, in models of kidney and heart transplantation in rats, MDSCs were found to play a unique role in trafficking Tregs to the allograft from secondary lymphoid organs by creating a C-C chemokine ligand 5 (CCL5) gradient between the graft and periphery, as summarized in Figure 1 (41).
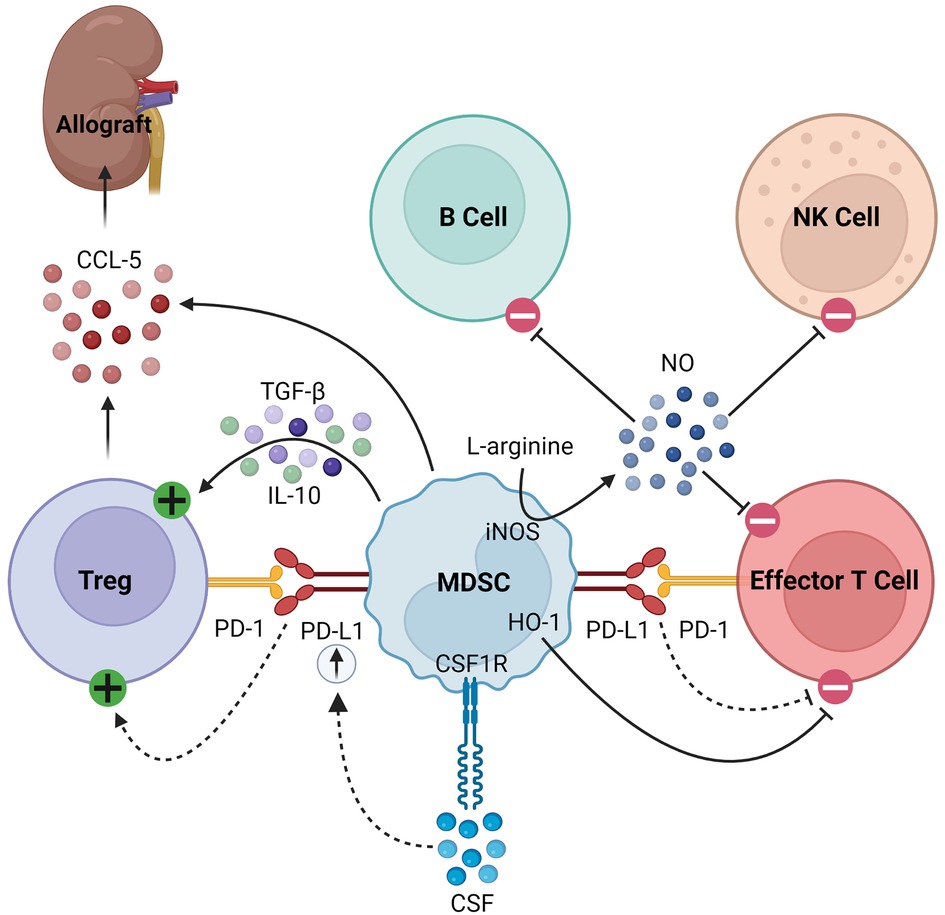
Figure 1. Immunosuppressive mechanisms of MDSCs. MDSCs express PD-L1, which activates Tregs and inhibits effector T cells by binding its cognate PD-1. In response to CSF/CSF1R signaling, MDSCs upregulate PD-L1. They express HO-1, which similarly inhibits effector T cells. MDSCs express iNOS which consumes L-arginine to produce NO, the latter of which then inhibits B cells, NK cells, and effector T cells. Finally, they produce TGF-β and IL-10 to activate Tregs and CCL-5 to recruit Tregs to the allograft.
Correlational studies have demonstrated that MDSCs develop in patients following solid organ transplantation and support a potential role for these cells in promoting tolerance (11, 42–44). MDSCs were shown to expand in the peripheral blood of patients following renal transplantation and correlated with their level of circulating Tregs, but more contemporary studies did not identify them in the peripheral blood or within the allograft of such patients using single-cell cytometry by time-of-flight and single cell RNA sequencing, respectively (11, 44–46). In acute T cell-mediated rejection, greater numbers of circulating MDSCs were associated with superior allograft survival and function (42). Finally, in lung transplant patients with stable allograft function, circulating levels of MDSCs were higher than in those with chronic allograft dysfunction (43). Overall, correlational studies in transplant patients suggest a promising role for MDSCs in preventing or treating allograft rejection, further supported by preclinical studies that will be discussed in the following section.
2.2. Regulatory dendritic cells
Dendritic cells (DCs) are a heterogeneous population of myeloid antigen presenting cells (APCs) that regulate immunity and maintain self-tolerance under homeostatic conditions (47, 48). Human DCs are divided into conventional DCs (defined as CD11c+ HLA-DR+ cells) and plasmocytoid DCs (defined as CD11c− HLA-DR+ CD123+ cells) (49). Regulatory DCs (regDCs or tolerogenic DCs) were first described in 1996 and so named for their tolerogenic properties in vivo, but it remains uncertain whether they represent an alternatively activated population or their own unique lineage (50, 51). Human regDCs are defined as CD11clow CD11bhigh CD14+ HLA-DR+ MHCIIlow CD86low DCs with low expression of co-stimulatory molecules (including CD40, CD80, and OX40L), MHCI, and adhesion proteins, but high expression of co-inhibition ligands (such as PD-L1) and death-inducing proteins [such as Fas ligand (FasL)] (52–55).
Beyond their well-described role as APCs, regDCs induce tolerance through several mechanisms, as summarized in Figure 2. They inhibit effector T cells through direct cell-cell interactions, triggering clonal deletion and anergy (56, 57). They upregulate various pro-apoptotic or immunomodulatory signals, including FasL, PD-L1, and indoleamine 2,3-dioxygenase (IDO), to delete or inhibit the proliferation of naïve and memory T cells (58–60). Like MDSCs, regDCs upregulate HO-1, which suppresses proinflammatory cytokine production and alloreactive T cell expansion (61–63). They drive the differentiation and activation of immunosuppressive immune cells, namely regulatory B cells (Bregs), Tregs, and double negative T cells (64–67). They secrete various anti-inflammatory soluble factors and cytokines such as NO, TGFβ, and IL-10 (60, 68, 69). Finally, dendritic cells release exosomes, or membrane nanovesicles carrying MHC molecules, which were recently shown to induce and sustain peripheral tolerance in various animal models of transplantation (70–73). These donor-derived exosomes are believed to promote tolerance through trogocytosis, creating “cross-dressed” recipient DCs that upregulate inhibitory cell surface markers (such as PD-L1) and cytokines (such as IL-10), suppressing the alloimmune response and prolonging allograft survival (74, 75). Based on these immunoregulatory properties of regDCs, as well as the observation that deletion of DCs induces spontaneous autoimmunity, they have been investigated in the setting of transplantation in both preclinical models and clinical trials, which will be discussed in detail in the next section (48).
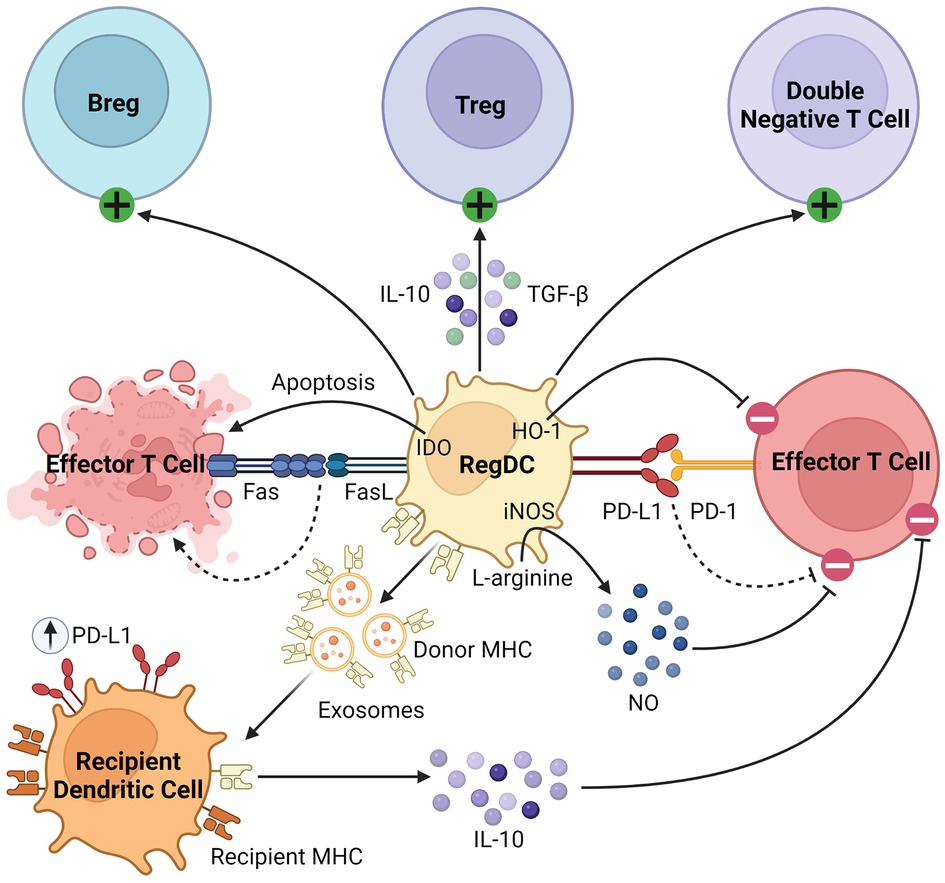
Figure 2. Immunosuppressive mechanisms of regDCs. RegDCs express PD-L1 and FasL to inhibit or delete effector and memory T cells through direct cell-cell contact. They also inhibit effector T cell activation through HO-1 and IDO signaling. They activate and promote the differentiation of Bregs, Tregs, and double negative T cells, and secrete anti-inflammatory cytokines including TGFβ, NO, and IL-10. Finally, donor regDCs release exosomes carrying donor MHC, creating “cross-dressed” recipient DCs via trogocytosis. These recipient DCs then upregulate inhibitory cell surface receptors (such as PD-L1) and secrete anti-inflammatory cytokines (such as IL-10) to further inhibit alloreactive T cells.
2.3. Regulatory macrophages
Macrophages are a heterogenous, highly plastic population of myeloid cells that play diverse roles in health and disease states, including phagocytosis, antigen presentation, tissue repair, and angiogenesis (76, 77). They can be classified into one of three subtypes: classically activated (M1) macrophages, alternatively activated (M2) macrophages, and regulatory macrophages (Mregs) (78). While M1 macrophages exhibit marked proinflammatory and bactericidal properties and M2 macrophages participate in wound healing and angiogenesis, Mregs have garnered particular attention in the field of transplantation for their robust inhibition of T cells (55, 77, 79–82). Human Mregs are defined as CD14−/low CD16− HLA-DR+ CD40−/low CD80−/low CD83− CD163−/low TLR2− TLR4− cells (83–86). Murine Mregs are identified as CD11b+ CD11c+ CD14+ MHCIIint CD40− CD80int CD86− CD169+ CD204+ CD206− TLR2− TLR4− cells (55).
As robust APCs with high expression of costimulatory molecules and anti-inflammatory cytokines, Mregs exert their immunosuppressive effects in various inflammatory conditions (87, 88). Parasites have been shown to promote Mreg induction, leading to impaired anti-parasitic immunity and chronic infection (89, 90). In various tumor models, conventional DCs are converted to Mregs which subsequently suppress inflammation (91). Mregs attenuate inflammation through suppression of activated T cells, stimulation of Tregs, and production of anti-inflammatory soluble factors, such as IL-10 and TGF-β, as illustrated in Figure 3 (92). Following IFN-γ signaling, Mregs produce IDO in humans and NO in mice to block T cell proliferation (84, 86). Secondly, Mregs suppress inflammation by promoting Treg expansion through TGF-β signaling and converting allogenic CD4+ T cells to inhibitory TIGIT+ FoxP3+ Tregs, the latter enhancing IL-10 production (93–95). Furthermore, TIGIT+ Tregs arrest DC maturation, resulting in allogenic T cell anergy or deletion via the indirect allorecognition pathway (55, 93). These findings have been correlated in vivo, with humanized mice demonstrating elevated levels of circulating TIGIT+ Tregs following Mreg administration (93, 94). Overall, this suggests a feed-forward mechanism between Mregs and Tregs to maintain an immunosuppressive environment lasting beyond the lifespan of adoptively transferred Mregs, which is promising for their application in solid organ transplantation (96). They seem to exert lasting effects on allogenic T cells, as any remaining after co-culture have significantly attenuated IFN-γ and IL-2 production on repeat stimulation (83).
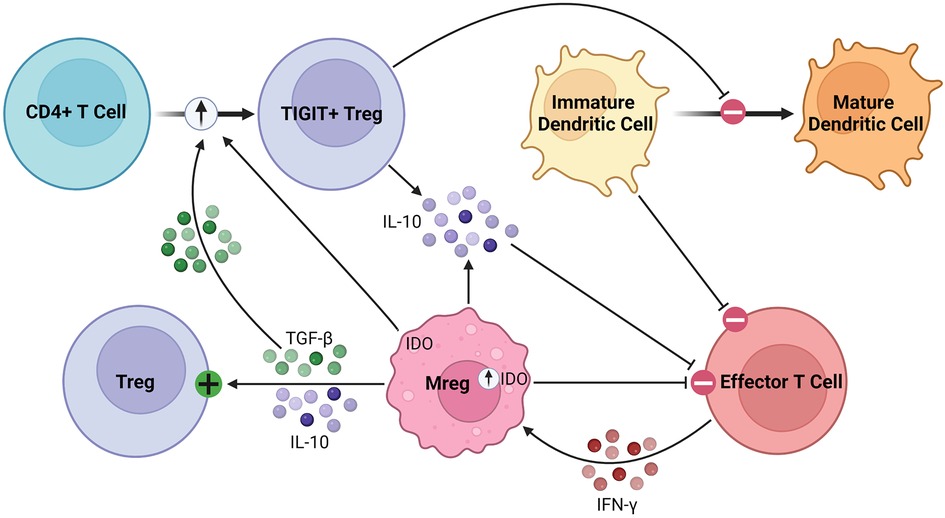
Figure 3. Immunosuppressive mechanisms of Mregs. Mregs express IDO in response to IFN-γ signaling, which then inhibits effector T cell proliferation. They secrete TGF-β and IL-10 to activate and promote the expansion of Tregs. Additionally, Mregs promote the conversion of allogenic CD4+ T cells to inhibitory TIGIT+ Tregs through the TGF-β and IDO signaling pathways, among others. The TIGIT+ Tregs secrete IL-10 and arrest dendritic cell maturation, the latter of which promotes allogenic T cell anergy or deletion through the indirect allorecognition pathway.
Beyond their immunosuppressive effects, Mregs limit fibrosis by suppressing M2 macrophage arginase production and fibroblast proliferation (97). Finally, they stimulate angiogenesis following hypoxia through upregulation of angiogenic proteins, suggesting they may limit allograft fibrosis and ischemia-reperfusion injury (IRI) (97, 98). The robust capacity of Mregs to suppress inflammation and promote tissue repair highlights why they, along with regDCs, have been the most extensively studied and developed as innate immune cellular therapeutics to date. Data regarding their application in preclinical and clinical studies will be discussed in detail in the next section.
2.4. Natural killer cells
NK cells are cytotoxic lymphoid cells known for their roles in anti-tumor and anti-viral immunity (99). Their cytotoxic activity does not require prior sensitization against a target and can be activated by cells lacking self MHC, while their inhibitory receptors recognize self MHC to prevent autologous cell death (100). Murine NK cells are identified as CD3− TCR− cells that express either DX5 or NK1.1 (101, 102). Human NK cells are defined as CD3− CD56+ NKp46+ cells, then can be further divided into subtypes of high density CD56bright cells and low density CD56dim cells (103). CD56dim cells express CD16, exhibit cytotoxic activity, and are found in the circulation, while CD56bright cells lack CD16 expression, localize to secondary lymphoid organs and peripheral tissues, and modulate the immune response through the production of cytokines, including IFN-γ and TNF-α (104, 105).
NK cells were initially shown to play immunoregulatory roles in other disease states such as bacterial infections and tumor immunization, in which they delete immature dendritic cells to prevent excessive inflammation (106, 107). Over the years, various immunoregulatory mechanisms of NK cells have been elucidated, including cytotoxic killing of APCs and effector T cells, activation of Tregs, cell surface receptor signaling, and cytokine production (108, 109). NK cells can inhibit the alloimmune response by killing donor DCs using perforin and granzyme, suppressing downstream activation of host alloimmune CD8+ T cells (110, 111). They have also been shown to delete recipient DCs to prevent presentation of allograft antigens (112). Alternatively, they can target activated allogenic CD4+ and CD8+ effector T cells for cytotoxic killing (113, 114). Beyond their cytotoxic activity, NK cells may shape the immune response via cytokine production in response to damaged or infected cells (115, 116). NK cells can produce IL-10, which promotes Th2 polarization of inflammation and Treg expansion (117). They may also activate Tregs in response to TGF-β signaling in the inflammatory milieu (118, 119). Furthermore, Deniz et al. found that a subset of NK cells in peripheral blood monocytes (PBMCs) isolated from healthy human subjects could suppress CD4+ T cell responses in an antigen-specific manner via IL-10 secretion (120).
Their immunosuppressive capacity can also be mediated by cell surface receptor signaling (121–124). Transplant tolerance induced by costimulatory blockade requires the presence of NK cells and their expression of the NKG2D receptor, which attenuates IFN-γ secretion and degranulation (121, 122). NK1.1+ cells in the liver were found to upregulate NKG2D expression to trigger IL-22 secretion, which then promoted allograft tolerance by limiting inflammation (123). Similar to NKG2D, lower expression of CD16 on CD56dim NK cells was associated with decreased expression of IFN-γ and perforin (124). Finally, NK cells express killer-like immunoglobulin receptors (KIRs) that bind MHCI molecules, and a subset of KIRs trigger downstream inhibitory signaling that suppresses NK cell activity (125). These cell surface receptors are notably expressed by uterine NK cells during pregnancy, which are known to regulate inflammation at the maternal-fetal interface and induce a tolerogenic environment (126, 127). In the presence of fetal HLA-C2+ cells, KIR2DS1+ uterine NK cells have been shown to promote the expansion of inhibitory monocytes expressing IDO, activate Tregs, and target effector T cells for apoptosis (118, 128–130). Furthermore, HLA-E expression on fetal cells has been shown to suppress NK cells via signaling through NKG2A (126).
While there are no clinical trials of NK cells in transplantation to date, correlational data suggests they play a role in allograft tolerance (124, 131–134). Compared to those with rejection, tolerized liver transplant patients have elevated numbers of circulating NK cells (131). In a study of kidney transplant patients with stable allograft function, CD56dim NK cells downregulated NKp46 and perforin compared to healthy controls (124). Patients with operational tolerance no longer requiring immunosuppression also demonstrated lower CD16 expression on these cells, which was associated with reduced IFN-γ secretion and cytotoxic activity (124). Downregulation of CD16 appears to promote tolerance, while upregulation has been associated with antibody-mediated rejection (124, 132). NK expression of KIR2DL1 and KIR3DL1, two subtypes of inhibitory KIRs, was ubiquitous in tolerized deceased donor kidney transplant patients, and the absence of both plus their cognate HLA ligands was associated with a heightened risk of chronic rejection, suggesting they may play an important role in tolerance induction (124, 133). Finally, a subset of these cells termed regulatory NK cells are though to suppress the alloimmune response through similar mechanisms as the uterine NK cells described above (135, 136). These regulatory NK cells express CD16 and/or CD56 and secrete perforin, granzyme, IFN-γ, and IL-10 (134). Indeed, such cells have been detected in the blood of renal transplant patients after achieving stable, durable allograft function (134). Based on the immunosuppressive properties of NK cells and available correlational data in transplant populations, the capacity of NK cells to inhibit alloimmunity has been investigated in preclinical models, which we summarize in the next section.
2.5. Innate lymphoid cells
Innate lymphoid cells (ILCs) are a family of lymphoid cells that play important roles in both homeostasis and disease (137–142). They are found in various tissues throughout the body but are particularly enriched at mucosal barriers (143–150). Following their activation by lipid mediators, alarmins, or neuropeptides, ILCs regulate inflammation and adaptive immunity at these barriers (142, 151). The ILC family encompasses NK cells and three subtypes of ILCs: group 1 (ILC1), group 2 (ILC2), and group 3 (ILC3). ILC1s, ILC2s, and ILC3s can be distinguished based on their transcription factor, cytokine, and cell surface marker expression, and are functionally homologous to the Th subsets of the adaptive immune system (152–154). ILC2s are defined as lineage− CD127+ c-kit+ Sca1+ ST2+ GATA3+ cells (154). They have been extensively studied in allergic airway inflammation and helminth infections, in which they promote a type 2 inflammatory response (143–146, 148, 155). They have garnered particular attention, however, as a potential therapeutic target for preventing or treating allograft rejection based on their newly characterized roles in tissue repair, suppression of damaging type 1 inflammation, and induction of other immunosuppressive cells (146, 154, 156–164).
ILC2s regulate the immune response through the production of soluble proteins and direct cell-cell interactions, as seen in Figure 4. They interact with T cells, firstly through the production of various effector cytokines, including IL-4, IL-5, and IL-13, to promote type 2 inflammation (143–146, 148, 155). As early and potent sources of these cytokines, ILC2s recruit and activate Th2 helper T cells to sites of inflammation, stimulating a positive feedback loop and suppressing more damaging Th1 and Th17 inflammation (165, 166). Like other innate immune cells described in this review, ILC2s can activate Tregs through the production of amphiregulin and direct cell contact, including signaling through inducible co-stimulator (ICOS)/IOCS ligand (ICOSL) and GITR/GITRL binding (160, 163, 167). In various cancers, ILC2s have been found to inhibit the anti-tumor immune response by promoting the infiltration and activation of MDSCs via IL-13 signaling (159, 168, 169). They have also been shown to stimulate and maintain M2 macrophages in inflamed tissues, which are less inflammatory than their M1 counterparts, through IL-5 and IL-13 signaling (157, 161).
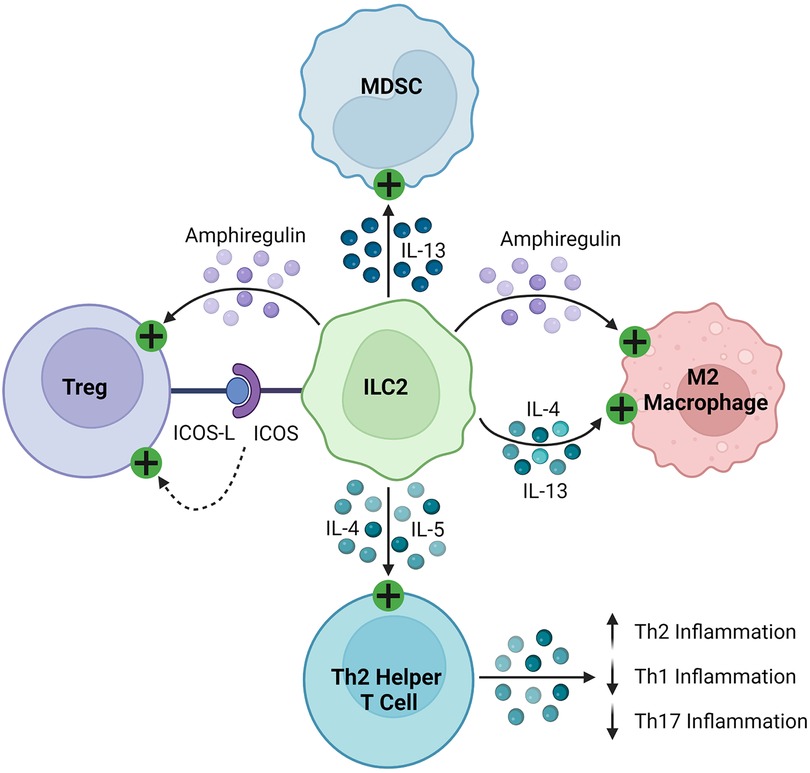
Figure 4. Immunosuppressive mechanisms of ILC2s. ILC2s express ICOS and activate Tregs by binding their cognate ICOS-L. They secrete amphiregulin, which activates Tregs and M2 macrophages. ILC2s produce IL-4, IL-5, and IL-13, which then stimulate MDSCs, M2 macrophages, and Th2 helper T cells, promoting a Th2 polarized inflammatory response.
Finally, ILC2s play a protective role in tissue repair, proliferating in response to alarmins, such as IL-33 and IL-25, released by the damaged epithelium after tissue injury or ischemia (154, 164, 170, 171). ILC2s subsequently upregulate their signature cytokines and amphiregulin, the latter of which controls the expansion and differentiation of various cell types through epidermal growth factor receptor signaling, to promote repair of the damaged epithelium (146, 172). To further support their potential role in solid organ transplantation, recent studies have illustrated that ILC2s attenuate IRI through M2 macrophage activation by IL-4, IL-13, or amphiregulin (164, 173).
There is limited correlational data regarding ILC2s in transplant patients, with one single-center cohort study demonstrating an inverse relationship between the number of ILC2s in lung allografts following reperfusion and the risk of primary graft dysfunction (174). While they are the least extensively studied cell type in this review and will require further investigation to elucidate their role in solid organ transplantation, a few preclinical studies have shown that ILC2s can be expanded in vitro and adoptively transferred to shape the alloimmune response. These studies will be covered in the next section.
3. Engineering tolerance and the development of innate immune cellular therapeutics
Based on the immunosuppressive properties of these innate immune effector cells in vivo, they have been proposed as therapeutic targets either to reduce reliance on immunosuppressive drugs or modulate peripheral tolerance through interactions with other immunoregulatory cells. As they are generally not present in sufficient numbers during the alloimmune response, however, they require stimulation or modulation ex vivo to exert robust immunosuppressive effects. In this section, we summarize preclinical studies applying in vitro-generated innate immune cells to models of transplantation, as well as clinical trials that have been conducted in transplant patients to date.
3.1. Myeloid-derived suppressor cells
MDSCs develop from immature myeloid cells in response to signals of chronic inflammation, including granulocyte colony-stimulating factor (G-CSF), granulocyte-macrophage colony-stimulating factor (GM-CSF), TNF-α, IFN-γ, TGF-β, lipopolysaccharide (LPS), CXCL-1/2, IL-2, and IL-6 (8, 9, 18, 175–179). Previous work has demonstrated that MDSCs can be induced in vitro from human bone marrow precursors cells in the presence of IL-6, GM-CSF, and G-CSF (179). Their subsequent immunosuppressive capacity, however, varies based on the cytokines used, with the greatest effect observed with combination GM-CSF and IL-6 treatment (179).
MDSCs have been shown to delay allograft rejection in multiple animal models, as summarized in Table 1. As mentioned above, activated MDSCs have been generated in culture using cytokines such as IL-6, G-CSF, and GM-CSF or induced in vivo with G-CSF or IL-33, both of which promoted skin allograft tolerance (36, 40, 175, 179–184). MDSCs generated in vitro with GM-CSF and IL-6 successfully delayed islet allograft rejection up to 200 days through CD8+ T cell suppression and NO production, the latter of which promoted antigen-specific Treg expansion and migration to lymphoid organs near the allograft (26, 37, 179). Lastly, MDSCs prolonged the survival of vascularized allografts in rodents following adoptive transfer or induction with agents such as IL-33 and anti-CD40L monoclonal antibody for co-stimulation blockade (14, 185, 186).
There are no clinical trials of MDSCs in transplant patients to date. While it remains unclear why they have not been pursued more aggressively as cellular therapeutics in these patients, the inherent challenges of generating immature immune cells, such the risk of differentiation once removed from artificial culture conditions and difficulties tracking their reconstitution in vivo, are likely contributing factors. Additional studies examining methods to expand these cells in vivo may yield more durable results.
3.2. Human monocyte-derived suppressor cells
Several studies have described the anti-inflammatory effects of human monocyte-derived suppressor cells (HuMoSCs) in murine models of graft-versus-host disease (GVHD) (187–189). HuMoSCs share many features with human M-MDSCs, as they are generated in vitro from PBMCs in the presence of GM-CSF and IL-6 and are defined as CD11b+ CD14+ CD33+ cells, but notably differ based on the former's high expression of HLA-DR (189). Using a xenogenic model of GVHD in which human PBMCs were injected into immunocompromised NSG mice, Janikashvili et al. demonstrated that concurrent infusion of autologous HuMoSCs could prevent GVHD and improve overall survival (189). Interestingly, these HuMoSCs inhibited both autologous and allogenic effector T cells in vitro and promoted expansion of CD8+ Tregs in vivo, the former of which is particularly relevant for targeting donor and recipient T cell contributions to the alloimmune response (189). In a follow up study, HuMoSCs retained their immunosuppressive capacity in a proinflammatory cytokine milieu and demonstrated an enhanced survival benefit in GVHD with the concurrent administration of cyclophosphamide (187). Given difficulties generating large numbers of HuMoSCs in vitro due to limited yield from PBMCs with existing protocols, administration of their culture supernatant as a therapeutic was trialed and found to alleviate xenogenic GVHD in mice, thought to be mediated by various immunosuppressive proteins including IL-1RA, GPNMB, and galectin-3 (188). Together, these studies support the feasibility of generating immunosuppressive myeloid cells from human PBMCs and the efficacy of the cells themselves or their products, which is encouraging for such application of HuMoSCs and MDSCs (187–189).
3.3. Regulatory dendritic cells
While the optimal method for generating stable regDCs remains a subject of debate, they are commonly induced in vitro from bone marrow cells in rodents and PBMCs in human subjects by culturing with GM-CSF with or without IL-4, followed by an anti-inflammatory or immunosuppressive agent to arrest further maturation (49, 69, 190–197). Such agents include anti-inflammatory cytokines (such as TNF-α, TGF-β, or IL-10), immunosuppressive drugs (such as mycophenolate, rapamycin, or corticosteroids), vitamins (such as vitamin D3 or retinoids), or tissue factors (such as vasoactive intestinal peptide or hepatocyte growth factor) (49, 69, 190–197).
The immunoregulatory properties of regDCs were first investigated in other inflammatory conditions, particularly autoimmune disorders following the observation that deletion of DCs induces spontaneous autoimmunity in mice (48, 190, 198). Preclinical studies demonstrated a protective role for in vitro-generated regDCs in such diseases, including rheumatoid arthritis and Crohn's disease, and more recently their short-term safety and efficacy were supported in clinical trials of patients with these disorders, type 1 diabetes mellitus, and multiple sclerosis (199–206). RegDCs also attenuated GVHD following bone marrow transplantation in mice (190). Based on these findings, multiple studies have investigated donor-derived regDCs in preclinical transplant models, as summarized in Table 2 (193, 207–216).
Preoperative or postoperative administration of a single dose of donor-derived regDCs prolonged heart allograft survival beyond 100 days in mice and rats, with these regDCs inhibiting T cell responses both in vitro and in vivo (207, 208). Interestingly, Lan et al. observed that the effects of regDCs could be potentiated with concurrent administration of CTLA-4 immunoglobulin (Ig), further delaying allograft rejection, while Bohnam et al. described similar results after genetically modifying regDCs to express CTLA-4 Ig (209, 210). Additional studies demonstrated a synergistic effect between regDCs and low dose immunosuppression in prolonging cardiac and renal allograft survival (211, 212, 217–219). Furthermore, donor-derived regDCs have successfully induced tolerance of rodent renal and composite tissue (CTA) allografts with standard-of-care immunosuppressants (193, 213, 214, 218). Donor-derived regDCs were then investigated in non-human primates (NHPs), with a single infusion of cells extending allograft survival nearly threefold to a median 113.5 days (from 39.5 days in controls) following MHC-mismatched kidney transplantation in rhesus macaques with minimal immunosuppression (216). Of note, these regDCs were found to have no adverse effects and induced substantial donor-specific memory T cell exhaustion (215, 216).
Given that donor-derived regDCs would be largely limited to use in living donor transplants and could cause sensitization, additional efforts have been dedicated to developing recipient-derived (or autologous) regDCs as cellular therapeutics (220–227). Autologous regDCs pulsed with donor allopeptides were found to delay islet, cardiac, and CTA rejection in rodents, likely through the indirect allorecognition pathway (220–222). As seen with donor-derived regDCs, the effects of autologous regDCs could be potentiated by an adjunct, as Baas et al. described superior islet allograft survival with concurrent anti-CD3 antibody administration (221, 222). Autologous regDCs promote tolerance in an antigen-specific manner by inducing anergy of alloreactive T cells and proliferation of Tregs in vivo in various transplant models, while syngeneic regDCs without alloantigen exposure delay rejection in a non-specific manner through NO production (224–226). Furthermore, allopeptide-pulsed autologous regDCs led to modest delays in MHC-mismatched renal allograft rejection in NHPs to 56 days (from 39.5 days in controls with no infusion and 29 days with naïve regDC infusion) in an HO-1-dependent manner (62, 227).
RegDCs are undergoing investigation in a few transplant clinical trials (228). The ONE Study (NCT02252055) tested multiple regulatory cell products in living donor renal transplant recipients in seven separate study arms, with one arm investigating one-time infusion of non-pulsed autologous regDCs one day prior to transplantation followed by standard-of-care immunosuppression (228). In this phase 1/2 trial, aggregate analysis demonstrated these regulatory immune cell products, including regDCs, were safe, feasible, associated with fewer viral infections, and led to successful weaning of immunosuppression in many participants at one year post-transplant (228). Phase 1/2 clinical trials of donor-derived regDCs in living donor kidney and liver transplant patients are ongoing at the University of Pittsburgh (NCT03726307, NCT03164265). Similar to the ONE Study, investigators will attempt to wean immunosuppression in these patients starting at six months post-transplant in the absence of rejection (228). Additional studies and clinical trials will clearly be needed to compare the efficacy of donor- and recipient-derived regDCs, the optimal dosing and timing of administration, and their long-term effects in transplant patients.
3.4. Regulatory macrophages
Mregs may be generated from bone marrow precursors or PBMCs in vitro following the activation of two signals. The first signal initiates the polarization of monocytes to monocyte macrophages, which then activate Mregs, and can be triggered by agents such as growth factors [including GM-CSF and macrophage colony-stimulating factor (M-CSF)], apoptotic cells, and glucocorticoids (78, 87, 229–232). With the second signal, Mregs are directly activated by TLR ligands and cytokines, which leads to downregulation of inflammatory factors and upregulation of inhibitory factors (95, 233–235). Mregs have been successfully generated from PBMCs in culture using M-CSF, human serum, and a brief 24-h pulse of IFN-γ, and while this approach has been utilized in clinical trials with renal transplant patients, their optimal induction method remains unclear (86, 92).
Mregs have been shown to delay allograft rejection in multiple preclinical models, as demonstrated in Table 3 (55, 83, 181, 236–243). Firstly, CSF1 treatment attenuates GVHD following bone marrow transplantation in mice through the expansion of recipient Mregs that inhibit allogenic donor T cell responses (236). Subsequent adoptive transfer experiments demonstrated preoperative donor-derived Mreg infusion delays rejection of skin, CTA, and heart allografts, the latter in an iNOS-dependent fashion enhanced by concurrent rapamycin administration (83, 181, 240, 243). In contrast to regDCs discussed above, only donor-derived Mregs are effective in delaying rejection, with no benefit observed with autologous Mregs (55, 83). In heart transplant recipients treated with anti-CD40L mAb costimulatory blockade, allograft tolerance was associated with enhanced migration of Ly6Chi monocytes to the graft, which then differentiated to Ly6Clo Mregs that secreted IL-10 in response to DC-SIGN or TLR4 signaling (237–239). In a porcine lung transplant model, donor-derived Mreg infusion did not prolong allograft survival, perhaps due to an insufficient dosage of cells (241). Finally, in vitro studies found that human Mregs can suppress the xenogenic immune response to porcine cells in an IDO-dependent manner, suggesting a potential role for these cells in xenotransplantation (242).
Mregs are the most extensively studied innate immune cells in transplant clinical trials (86, 228, 244–247). They were first investigated in the phase 1 Transplant Acceptance-Inducing Cell trial I (TAIC-I) in which deceased donor kidney transplant patients received one infusion of donor-derived Mregs on postoperative day five (244). No adverse effects were reported and two patients successfully weaned from standard immunosuppression, but the therapeutic benefit of these cells could not be clearly discerned (244, 245). TAIC-I was followed by several small phase 1 trials of preoperative Mreg administration in living donor kidney transplantation at various time points, with six out of eight patients transitioning to tacrolimus monotherapy without eliciting rejection (86, 246, 247). Of the two remaining patients, one was undergoing living-related kidney transplant against which he was already sensitized but was subsequently transitioned to low dose tacrolimus and prednisolone with no rejection episodes (247). Interestingly, at eight weeks postoperatively, he was found to be hyporesponsive to his donor on mixed lymphocyte reaction and had resolved his donor-specific antibodies, which remained absent through the 53 week follow up period (247). As previously mentioned, the ONE Study (NCT02085629) investigated various regulatory immune cells in living donor renal transplant patients, with one arm dedicated to donor-derived Mregs (228). Administration of these cells, including Mregs, led to no adverse events and was associated with a reduction in post-transplant viral infections and successful weaning of immunosuppression (228). Overall, significant preclinical and early clinical trial data suggest donor-derived Mregs may be harnessed to prevent rejection in solid organ transplant patients.
3.5. Natural killer cells
In the setting of transplantation, NK cells have been found to participate in both rejection and tolerance, likely due to the distinct functions of their various subsets or differentiation states (248). Despite these mixed findings, several preclinical studies support a role for NK cells in inducing allograft tolerance (110, 111, 113, 121–123, 249, 250). Firstly, NK cells were shown to attenuate the severity of GVHD in mice by suppressing activated alloreactive T cells, mediated by perforin and FasL signaling (249). As mentioned in the prior section, several studies have found that tolerance induction by costimulatory blockade is dependent on NK cells (121, 122). Various functions of NK cells have been implicated in this phenomenon, including perforin secretion in an islet allograft model and NKG2D receptor signaling in a heart allograft model (121, 122). Additionally, NK cells were shown to delay skin graft rejection through cytotoxic killing of donor-derived APCs, which would otherwise activate host alloimmune T cells (110, 111). NK cells also delayed rejection of murine skin grafts by suppressing alloimmune T cell responses directly, either through competition for shared growth factors or cytotoxic killing (113, 250). Finally, upregulation of the NKG2A receptor in NK cells following islet transplantation stimulated secretion of IL-22, which attenuated inflammation and prolonged allograft survival (123). These studies are summarized in Table 4.
Finally, with recent advances in genetic modification of immune cells, chimeric antigen receptor (CAR)-NK cells have been developed as novel therapeutics for advanced malignancies resistant to standard treatment (251, 252). Unlike CAR-T cells, CAR-NK cells can be generated from individuals other that the recipient without the risk of GVHD and have not been associated with common side effects of the former, such as neurotoxicity or cytokine release syndrome (253–255). These features are promising for their application as “off-the-shelf” cellular products and with greater understanding of the inherent immunosuppressive features of these cells, we imagine such engineered NK cells may be explored as therapeutics to prevent or treat rejection in the coming years. In summary, preclinical studies suggest a subpopulation of NK cells can modulate the alloimmune response to promote allograft tolerance, and recent advances in immunotherapy support the feasibility and efficacy of engineered NK cells as therapeutics.
3.6. Innate lymphoid cells
Preclinical studies investigating ILC2s in transplantation are limited to date, as summarized in Table 5. Bruce et al. found that adoptive transfer of activated ILC2s attenuated the severity and mortality of GVHD in mice, as they migrated to the gastrointestinal tract to improve barrier function and recruited MDSCs to suppress inflammation (256). Perhaps most exciting, Huang et al. demonstrated that systemic IL-33 treatment or infusion of IL-33-primed ILC2s could significantly delay rejection of islet allografts with major antigen mismatch via IL-10 secretion (257). The efficacy of IL-33 treatment was dampened following depletion of Tregs, which themselves are stimulated by IL-33 and have been shown to delay allograft rejection in other transplant models (185, 257–260). Furthermore, IL-33 is also known to activate MDSCs to delay heart allograft rejection in mice, which was not investigated by the authors (185, 257). Donor-derived ILC2s activated by IRI were recently shown to enhance eosinophil recruitment to the allograft following lung transplantation, leading to reduced T cell infiltration and attenuating rejection at seven days post-transplant (261). Of note, this axis was dependent on donor ILC2s, rather than infiltrating recipient ILC2s, suggesting additional work will be required to differentiate the roles of donor and recipient ILC2s in other transplant models (261). These studies are reviewed in Table 5.
Overall, our current understanding of ILC2s suggests they may be capable of inhibiting the alloimmune response and preventing allograft rejection through multiple pathways, including stimulating tissue repair, secreting anti-inflammatory cytokines, and activating other immunosuppressive cells (146, 154, 156–164). Additional investigation, however, is clearly needed to characterize the functions of ILC2s in different tissues, their roles when derived from donor versus recipient, and their long-term effects in human transplant patients.
4. Discussion
Preclinical and clinical studies performed to date investigating the therapeutic potential of innate immune cells to prevent or treat allograft rejection are encouraging. Infusion of MDSCs, HuMoSCs, regDCs, Mregs, NK cells, and ILC2s may eventually reduce or replace the need for non-specific, chronic immunosuppression, with early clinical trials of Mregs and regDCs demonstrating successful weaning in some patients (86, 228, 244, 246). Many questions, however, remain regarding their use, including the most appropriate dosing range, timing, and frequency of administration. Weekly adoptive transfers of MDSCs, for example, led to superior allograft survival than a single administration following skin transplantation in mice (175). Mreg infusion has been trialed both preoperatively and postoperatively in small clinical studies of kidney transplant patients, with no clear conclusion yet as to the best strategy (86, 244, 246, 247).
Furthermore, additional studies are needed to elucidate the optimal source of these cells. While only donor-derived Mregs promote tolerance, both donor- and recipient-derived regDCs have been utilized effectively in animal models (55, 83, 207, 208, 220, 224, 225). Given the breadth of signals that drive the proliferation and maturation of these cells, it will be necessary to determine the optimal conditions for their induction in vitro, as has been investigated for MDSCs and Mregs to some extent (85, 86, 92, 179). Given that these cells are known to interact with each other and other immunosuppressive cells, whether infusion of multiple cell types potentiates their effects in vivo warrants further investigation as well (14, 26, 64–67, 159, 168, 169). Similarly, some studies have demonstrated greater efficacy of these cells when administered with an adjunct, such as CTLA-4 Ig with regDCs and rapamycin with Mregs, which should be explored further (83, 209, 210).
Finally, recent studies suggest that innate immune cells may be targeted in vivo to promote transplant tolerance by stimulating or inhibiting various signaling pathways, including microRNA (miRNA) and purinergic signaling (262–265). Usuelli et al. found that suppression of miRNA-21 prevented allograft rejection and chronic allograft vasculopathy in a murine model of cardiac transplantation by promoting M2 polarization in infiltrating macrophages (262). Conversely, other groups have shown that miRNA-22 is critical for the development of functional MDSCs and M2 macrophages following CSF1R signaling (263, 264). Additional work will be needed to elucidate the roles of these various soluble factors and signaling pathways in the alloimmune response, and to determine which pathways could be selectively targeted with novel therapeutics in these various regulatory innate immune cells to promote tolerance.
Large scale clinical trials will be necessary to answer these questions prior to the widespread implementation of innate immune cells as therapeutics in transplantation. Long-term follow up will be critical to characterize any adverse events or unintended consequences of these cells over time, such as possible fibrosis associated with prolonged ILC2 activation (266, 267). Overall, innate immune cells represent a promising new therapeutic strategy to induce tolerance following solid organ transplantation and we look forward to their translation to clinical practice.
Author contributions
LCO and AGC contributed to the writing of this manuscript. All authors contributed to the article and approved the submitted version.
Funding
AGC is supported by awards from the American Society of Transplant Surgeons, the American Pediatric Surgical Association, and the Translational Research program at Boston Children's Hospital. LCO is supported by National Institutes of Health Grant 2T32DK007754-21.
Acknowledgments
Figures were created with BioRender.com.
Conflict of interest
The authors declare that the research was conducted in the absence of any commercial or financial relationships that could be construed as a potential conflict of interest.
Publisher's note
All claims expressed in this article are solely those of the authors and do not necessarily represent those of their affiliated organizations, or those of the publisher, the editors and the reviewers. Any product that may be evaluated in this article, or claim that may be made by its manufacturer, is not guaranteed or endorsed by the publisher.
References
1. Wekerle T, Segev D, Lechler R, Oberbauer R. Strategies for long-term preservation of kidney graft function. Lancet. (2017) 389(10084):2152–62. doi: 10.1016/S0140-6736(17)31283-7
2. Bamoulid J, Staeck O, Halleck F, Khadzhynov D, Brakemeier S, Dürr M, et al. The need for minimization strategies: current problems of immunosuppression. Transpl Int. (2015) 28(8):891–900. doi: 10.1111/tri.12553
3. Miloh T, Barton A, Wheeler J, Pham Y, Hewitt W, Keegan T, et al. Immunosuppression in pediatric liver transplant recipients: unique aspects. Liver Transpl. (2017) 23(2):244–56. doi: 10.1002/lt.24677
4. Cunningham KT, Mills KHG. Trained innate immunity in hematopoietic stem cell and solid organ transplantation. Transplantation. (2021) 105(8):1666–76. doi: 10.1097/TP.0000000000003673
5. Divangahi M, Aaby P, Khader SA, Barreiro LB, Bekkering S, Chavakis T, et al. Trained immunity, tolerance, priming and differentiation: distinct immunological processes. Nat Immunol. (2021) 22(1):2–6. doi: 10.1038/s41590-020-00845-6
6. Ochando J, Fayad ZA, Madsen JC, Netea MG, Mulder WJM. Trained immunity in organ transplantation. Am J Transplant. (2020) 20(1):10–8. doi: 10.1111/ajt.15620
7. Lee YS, Zhang T, Saxena V, Li L, Piao W, Bromberg JS, et al. Myeloid-derived suppressor cells expand after transplantation and their augmentation increases graft survival. Am J Transplant. (2020) 20(9):2343–55. doi: 10.1111/ajt.15879
8. Bronte V, Brandau S, Chen SH, Colombo MP, Frey AB, Greten TF, et al. Recommendations for myeloid-derived suppressor cell nomenclature and characterization standards. Nat Commun. (2016) 7:12150. doi: 10.1038/ncomms12150
9. Scalea JR, Lee YS, Davila E, Bromberg JS. Myeloid-derived suppressor cells and their potential application in transplantation. Transplantation. (2018) 102(3):359–67. doi: 10.1097/TP.0000000000002022
10. Gabrilovich DI, Nagaraj S. Myeloid-derived suppressor cells as regulators of the immune system. Nat Rev Immunol. (2009) 9(3):162–74. doi: 10.1038/nri2506
11. Luan Y, Mosheir E, Menon MC, Wilson D, Woytovich C, Ochando J, et al. Monocytic myeloid-derived suppressor cells accumulate in renal transplant patients and mediate CD4+ Foxp3+ treg expansion. Am J Transplant. (2013) 13(12):3123–31. doi: 10.1111/ajt.12461
12. Höchst B, Mikulec J, Baccega T, Metzger C, Welz M, Peusquens J, et al. Differential induction of Ly6G and Ly6C positive myeloid derived suppressor cells in chronic kidney and liver inflammation and fibrosis. PLoS One. (2015) 10(3):e0119662. doi: 10.1371/journal.pone.0119662
13. Vendramin A, Gimondi S, Bermema A, Longoni P, Rizzitano S, Corradini P, et al. Graft monocytic myeloid-derived suppressor cell content predicts the risk of acute graft-versus-host disease after allogeneic transplantation of granulocyte colony-stimulating factor-mobilized peripheral blood stem cells. Biol Blood Marrow Transplant. (2014) 20(12):2049–55. doi: 10.1016/j.bbmt.2014.09.011
14. Garcia MR, Ledgerwood L, Yang Y, Xu J, Lal G, Burrell B, et al. Monocytic suppressive cells mediate cardiovascular transplantation tolerance in mice. J Clin Invest. (2010) 120(7):2486–96. doi: 10.1172/JCI41628
15. Bronte V, Apolloni E, Cabrelle A, Ronca R, Serafini P, Zamboni P, et al. Identification of a CD11b+/Gr-1+/CD31+ myeloid progenitor capable of activating or suppressing CD8+ T cells. Blood. (2000) 96(12):3838–46. doi: 10.1182/blood.V96.12.3838
16. Almand B, Clark JI, Nikitina E, van Beynen J, English NR, Knight SC, et al. Increased production of immature myeloid cells in cancer patients: a mechanism of immunosuppression in cancer. J Immunol. (2001) 166(1):678–89. doi: 10.4049/jimmunol.166.1.678
17. Movahedi K, Guilliams M, van den Bossche J, van den Bergh R, Gysemans C, Beschin A, et al. Identification of discrete tumor-induced myeloid-derived suppressor cell subpopulations with distinct T cell suppressive activity. Blood. (2008) 111(8):4233–44. doi: 10.1182/blood-2007-07-099226
18. Huang B, Pan PY, Li Q, Sato AI, Levy DE, Bromberg J, et al. Gr-1+ CD115+ immature myeloid suppressor cells mediate the development of tumor-induced T regulatory cells and T-cell anergy in tumor-bearing host. Cancer Res. (2006) 66(2):1123–31. doi: 10.1158/0008-5472.CAN-05-1299
19. Ost M, Singh A, Peschel A, Mehling R, Rieber N, Hartl D. Myeloid-derived suppressor cells in bacterial infections. Front Cell Infect Microbiol. (2006) 6:37. doi: 10.3389/fcimb.2016.00037
20. He Y, Bei J, Zeng H, Pan Z. The roles of sepsis-induced myeloid derived suppressor cells in mice corneal, skin and combined transplantation. Transpl Immunol. (2016) 34:8–13. doi: 10.1016/j.trim.2015.12.003
21. Wu T, Zhao Y, Wang H, Li Y, Shao L, Wang R, et al. MTOR Masters monocytic myeloid-derived suppressor cells in mice with allografts or tumors. Sci Rep. (2016) 6:20250. doi: 10.1038/srep20250
22. Dugast AS, Haudebourg T, Coulon F, Heslan M, Haspot F, Poirier N, et al. Myeloid-derived suppressor cells accumulate in kidney allograft tolerance and specifically suppress effector T cell expansion. J Immunol. (2008) 180(12):7898–906. doi: 10.4049/jimmunol.180.12.7898
23. Zhang K, Bai X, Li R, Xiao Z, Chen J, Yang F, et al. Endogenous glucocorticoids promote the expansion of myeloid-derived suppressor cells in a murine model of trauma. Int J Mol Med. (2012) 30(2):277–82. doi: 10.3892/ijmm.2012.1014
24. Bruno A, Mortara L, Baci D, Noonan DM, Albini A. Myeloid derived suppressor cells interactions with natural killer cells and pro-angiogenic activities: roles in tumor progression. Front Immunol. (2019) 10:771. doi: 10.3389/fimmu.2019.00771
25. Oberholtzer N, Atkinson C, Nadig SN. Adoptive transfer of regulatory immune cells in organ transplantation. Front Immunol. (2021) 12:631365. doi: 10.3389/fimmu.2021.631365
26. Chou HS, Hsieh CC, Charles R, Wang L, Wagner T, Fung JJ, et al. Myeloid-derived suppressor cells protect islet transplants by B7-H1 mediated enhancement of T regulatory cells. Transplantation. (2012) 93(3):272–82. doi: 10.1097/TP.0b013e31823ffd39
27. Gupta HB, Clark CA, Yuan B, Sareddy G, Pandeswara S, Padron AS, et al. Tumor cell-intrinsic PD-L1 promotes tumor-initiating cell generation and functions in melanoma and ovarian cancer. Signal Transduct Target Ther. (2016) 1:6030. doi: 10.1038/sigtrans.2016.30
28. Butte MJ, Keir ME, Phamduy TB, Sharpe AH, Freeman GJ. Programmed death-1 ligand 1 interacts specifically with the B7-1 costimulatory molecule to inhibit T cell responses. Immunity. (2007) 27(1):111–22. doi: 10.1016/j.immuni.2007.05.016
29. Hamilton JA. Colony-stimulating factors in inflammation and autoimmunity. Nat Rev Immunol. (2008) 8(7):533–44. doi: 10.1038/nri2356
30. Chitu V, Stanley ER. Colony-stimulating factor-1 in immunity and inflammation. Curr Opin Immunol. (2006) 18(1):39–48. doi: 10.1016/j.coi.2005.11.006
31. Zhao T, Liu S, Ding X, Johnson EM, Hanna NH, Singh K, et al. Lysosomal acid lipase, CSF1R and PD-L1 determine functions of CD11c+ myeloid-derived suppressor cells. JCI Insight. (2022) 7(17):e156623. doi: 10.1172/jci.insight.156623
32. Priceman SJ, Sung JL, Shaposhnik Z, Burton JB, Torres-Collado AX, Moughon DL, et al. Targeting distinct tumor-infiltrating myeloid cells by inhibiting CSF-1 receptor: combating tumor evasion of antiangiogenic therapy. Blood. (2010) 115(7):1461–71. doi: 10.1182/blood-2009-08-237412
33. Zhu Y, Knolhoff BL, Meyer MA, Nywening TM, West BL, Luo J, et al. CSF1/CSF1R Blockade reprograms tumor-infiltrating macrophages and improves response to T-cell checkpoint immunotherapy in pancreatic cancer models. Cancer Res. (2014) 74(18):5057–69. doi: 10.1158/0008-5472.CAN-13-3723
34. Lees JR, Azimzadeh AM, Bromberg JS. Myeloid derived suppressor cells in transplantati. Curr Opin Immunol. (2011) 23(5):692–7. doi: 10.1016/j.coi.2011.07.004
35. Han C, Wu T, Na N, Zhao Y, Li W, Zhao Y. The effect of immunosuppressive drug cyclosporine A on myeloid-derived suppressor cells in transplanted mice. Inflammation Res. (2016) 65(9):679–88. doi: 10.1007/s00011-016-0949-7
36. Yang F, Li Y, Zou W, Xu Y, Wang H, Wang W, et al. Adoptive transfer of IFN-γ-induced M-MDSCs promotes immune tolerance to allografts through iNOS pathway. Inflammation Res. (2019) 68(7):679–88. doi: 10.1007/s00011-019-01237-9
37. Arakawa Y, Qin J, Chou HS, Bhatt S, Wang L, Stuehr D, et al. Cotransplantation with myeloid-derived suppressor cells protects cell transplants: a crucial role of inducible nitric oxide synthase. Transplantation. (2014) 97(7):740–7. doi: 10.1097/01.TP.0000442504.23885.f7
38. Liew FY. Regulation of lymphocyte functions by nitric oxide. Curr Opin Immunol. (1995) 7(3):396–9. doi: 10.1016/0952-7915(95)80116-2
39. Rodriguez PC, Quiceno DG, Ochoa AC. L-arginine availability regulates T-lymphocyte cell-cycle progression. Blood. (2007) 109(4):1568–73. doi: 10.1182/blood-2006-06-031856
40. de Wilde V, van Rompaey N, Hill M, Lebrun JF, Lemaître P, Lhommé F, et al. Endotoxin-induced myeloid-derived suppressor cells inhibit alloimmune responses via heme oxygenase-1. Am J Transplant. (2009) 9(9):2034–47. doi: 10.1111/j.1600-6143.2009.02757.x
41. Dilek N, Poirier N, Usal C, Martinet B, Blancho G, Vanhove B. Control of transplant tolerance and intragraft regulatory T cell localization by myeloid-derived suppressor cells and CCL5. J Immunol. (2012) 188(9):4209–16. doi: 10.4049/jimmunol.1101512
42. Meng F, Chen SY, Guo X, Chen Z, Huang X, Lai Y, et al. Clinical significance of myeloid-derived suppressor cells in human renal transplantation with acute T cell-mediated rejection. Inflammation. (2014) 37(5):1799–805. doi: 10.1007/s10753-014-9910-5
43. Heigl T, Singh A, Saez-Gimenez B, Kaes J, van Herck A, Sacreas A, et al. Myeloid-derived suppressor cells in lung transplantation. Front Immunol. (2019) 10:900. doi: 10.3389/fimmu.2019.00900
44. Hock BD, MacKenzie KA, Cross NB, Taylor KG, Currie MJ, Robinson BA, et al. Renal transplant recipients have elevated frequencies of circulating myeloid-derived suppressor cells. Nephrol Dial Transplant. (2012) 27(1):402–10. doi: 10.1093/ndt/gfr264
45. Liu Y, Liu X, Zhou S, Xu R, Hu J, Liao G, et al. Single-cell profiling of kidney transplant recipients with immunosuppressive treatment reveals the dynamic immune characteristics. Front Immunol. (2021) 12:639942. doi: 10.3389/fimmu.2021.639942
46. Malone AF, Wu H, Fronick C, Fulton R, Gaut JP, Humphreys BD. Harnessing expressed single nucleotide variation and single cell RNA sequencing to define immune cell chimerism in the rejecting kidney transplant. J Am Soc Nephrol. (2020) 31(9):1977–86. doi: 10.1681/ASN.2020030326
47. Banchereau J, Steinman RM. Dendritic cells and the control of immunity. Nature. (1998) 392(6673):245–52. doi: 10.1038/32588
48. Ohnmacht C, Pullner A, King SBS, Drexler I, Meier S, Brocker T, et al. Constitutive ablation of dendritic cells breaks self-tolerance of CD4 T cells and results in spontaneous fatal autoimmunity. J Exp Med. (2009) 206(3):549–59. doi: 10.1084/jem.20082394
49. Sato K, Fujita S. Dendritic cells-nature and classification. Allergol Int. (2007) 56(3):183–91. doi: 10.2332/allergolint.R-06-139
50. Takenaka MC, Quintana FJ. Tolerogenic dendritic cells. Semin Immunopathol. (2017) 39(2):113–20. doi: 10.1007/s00281-016-0587-8
51. Steptoe RJ, Thomson AW. Dendritic cells and tolerance induction. Clin Exp Immunol. (1996) 105(3):397–402. doi: 10.1046/j.1365-2249.1996.d01-779.x
52. Raker VK, Domogalla MP, Steinbrink K. Tolerogenic dendritic cells for regulatory T cell induction in man. Front Immunol. (2015) 6:569. doi: 10.3389/fimmu.2015.00569
53. Sato K, Uto T, Fukaya T, Takagi H. Regulatory dendritic cells. Curr Top Microbiol Immunol. (2017) 410:47–71. doi: 10.1007/82_2017_60
54. Wilson HL, Ni K, O’Neill HC. Identification of progenitor cells in long-term spleen stromal cultures that produce immature dendritic cells. Proc Natl Acad Sci USA. (2000) 97(9):4784–9. doi: 10.1073/pnas.080278897
55. Riquelme P, Geissler EK, Hutchinson JA. Alternative approaches to myeloid suppressor cell therapy in transplantation: comparing regulatory macrophages to tolerogenic DCs and MDSCs. Transplant Res. (2012) 1(1):17. doi: 10.1186/2047-1440-1-17
56. Schietinger A, Greenberg PD. Tolerance and exhaustion: defining mechanisms of T cell dysfunction. Trends Immunol. (2014) 35(2):51–60. doi: 10.1016/j.it.2013.10.001
57. Schwartz RH. T cell anergy. Annu Rev Immunol. (2003) 21:305–34. doi: 10.1146/annurev.immunol.21.120601.141110
58. Mellor AL, Baban B, Chandler P, Marshall B, Jhaver K, Hansen A, et al. Cutting edge: induced indoleamine 2,3 dioxygenase expression in dendritic cell subsets suppresses T cell clonal expansion. J Immunol. (2003) 171(4):1652–5. doi: 10.4049/jimmunol.171.4.1652
59. Lu L, Qian S, Hershberger PA, Rudert WA, Lynch DH, Thomson AW. Fas ligand (CD95L) and B7 expression on dendritic cells provide counter-regulatory signals for T cell survival and proliferation. J Immunol. (1997) 158(12):5676–84. doi: 10.4049/jimmunol.158.12.5676
60. Li H, Shi B. Tolerogenic dendritic cells and their applications in transplantation. Cell Mol Immunol. (2015) 12(1):24–30. doi: 10.1038/cmi.2014.52
61. Chauveau C, Rémy S, Royer PJ, Hill M, Tanguy-Royer S, Hubert FX, et al. Heme oxygenase-1 expression inhibits dendritic cell maturation and proinflammatory function but conserves IL-10 expression. Blood. (2005) 106(5):1694–702. doi: 10.1182/blood-2005-02-0494
62. Moreau A, Hill M, Thébault P, Deschamps JY, Chiffoleau E, Chauveau C, et al. Tolerogenic dendritic cells actively inhibit T cells through heme oxygenase-1 in rodents and in nonhuman primates. FASEB J. (2009) 23(9):3070–7. doi: 10.1096/fj.08-128173
63. Rémy S, Blancou P, Tesson L, Tardif V, Brion R, Royer PJ, et al. Carbon monoxide inhibits TLR-induced dendritic cell immunogenicity. J Immunol. (2009) 182(4):1877–84. doi: 10.4049/jimmunol.0802436
64. Qian L, Qian C, Chen Y, Bai Y, Bao Y, Lu L, et al. Regulatory dendritic cells program B cells to differentiate into CD19 hiFcγIIb hi regulatory B cells through IFN-β and CD40l. Blood. (2012) 120(3):581–91. doi: 10.1182/blood-2011-08-377242
65. Hsu SM, Mathew R, Taylor AW, Stein-Streilein J. Ex-vivo tolerogenic F4/80+ antigen-presenting cells (APC) induce efferent CD8+ regulatory T cell-dependent suppression of experimental autoimmune uveitis. Clin Exp Immunol. (2014) 176(1):37–48. doi: 10.1111/cei.12243
66. Huang H, Dawicki W, Zhang X, Town J, Gordon JR. Tolerogenic dendritic cells induce CD4+CD25 hi Foxp3+regulatory T cell differentiation from CD4+CD25 −/lo Foxp3− effector T cells. J Immunol. (2010) 185(9):5003–10. doi: 10.4049/jimmunol.0903446
67. Hill M, Thebault P, Segovia M, Louvet C, Bériou G, Tilly G, et al. Cell therapy with autologous tolerogenic dendritic cells induces allograft tolerance through interferon-gamma and Epstein-Barr virus-induced gene 3. Am J Transplant. (2011) 11(10):2036–45. doi: 10.1111/j.1600-6143.2011.03651.x
68. Ilarregui JM, Croci DO, Bianco GA, Toscano MA, Salatino M, Vermeulen ME, et al. Tolerogenic signals delivered by dendritic cells to T cells through a galectin-1-driven immunoregulatory circuit involving interleukin 27 and interleukin 10. Nat Immunol. (2009) 10(9):981–91. doi: 10.1038/ni.1772
69. Marín E, Cuturi MC, Moreau A. Tolerogenic dendritic cells in solid organ transplantation: where do we stand? Front Immunol. (2018) 9:274. doi: 10.3389/fimmu.2018.00274
70. Pêche H, Renaudin K, Beriou G, Merieau E, Amigorena S, Cuturi MC. Induction of tolerance by exosomes and short-term immunosuppression in a fully MHC-mismatched rat cardiac allograft model. Am J Transplant. (2006) 6(7):1541–50. doi: 10.1111/j.1600-6143.2006.01344.x
71. Pêche H, Heslan M, Usal C, Amigorena S, Cuturi MC. Presentation of donor major histocompatibility complex antigens by bone marrow dendritic cell-derived exosomes modulates allograft rejection. Transplantation. (2003) 76(10):1503–10. doi: 10.1097/01.TP.0000092494.75313.38
72. Song J, Huang J, Chen X, Teng X, Song Z, Xing Y, et al. Donor-derived exosomes induce specific regulatory T cells to suppress immune inflammation in the allograft heart. Sci Rep. (2016) 7:20077. doi: 10.1038/srep20077
73. Ochando J, Ordikhani F, Jordan S, Boros P, Thomson AW. Tolerogenic dendritic cells in organ transplantation. Transpl Int. (2020) 33(2):113–27. doi: 10.1111/tri.13504
74. Ono Y, Perez-Gutierrez A, Nakao T, Dai H, Camirand G, Yoshida O, et al. Graft-infiltrating PD-L1hi cross-dressed dendritic cells regulate antidonor T cell responses in mouse liver transplant tolerance. Hepatology. (2018) 67(4):1499–515. doi: 10.1002/hep.29529
75. Bracamonte-Baran W, Florentin J, Zhou Y, Jankowska-Gan E, Haynes WJ, Zhong W, et al. Modification of host dendritic cells by microchimerism-derived extracellular vesicles generates split tolerance. Proc Natl Acad Sci USA. (2017) 114(5):1099–104. doi: 10.1073/pnas.1618364114
76. Chen T, Cao Q, Wang Y, Harris DCH. M2 macrophages in kidney disease: biology, therapies, and perspectives. Kidney Int. (2019) 95(4):760–73. doi: 10.1016/j.kint.2018.10.041
77. Wynn TA, Vannella KM. Macrophages in tissue repair, regeneration, and fibrosis. Immunity. (2016) 44(3):450–62. doi: 10.1016/j.immuni.2016.02.015
78. Mosser DM, Edwards JP. Exploring the full spectrum of macrophage activation. Nat Rev Immunol. (2008) 8(12):958–69. doi: 10.1038/nri2448
79. Wager CML, Wormley FL. Classical versus alternative macrophage activation: the Ying and the Yang in host defense against pulmonary fungal infections. Mucosal Immunol. (2014) 7(5):1023–35. doi: 10.1038/mi.2014.65
80. Atri C, Guerfali FZ, Laouini D. Role of human macrophage polarization in inflammation during infectious diseases. Int J Mol Sci. (2018) 19(6):1801. doi: 10.3390/ijms19061801
81. Sica A, Mantovani A. Macrophage plasticity and polarization: in vivo veritas. J Clin Invest. (2012) 122(3):787–95. doi: 10.1172/JCI59643
82. Saha S, Shalova IN, Biswas SK. Metabolic regulation of macrophage phenotype and function. Immunol Rev. (2017) 280(1):102–11. doi: 10.1111/imr.12603
83. Riquelme P, Tomiuk S, Kammler A, Fändrich F, Schlitt HJ, Geissler EK, et al. IFN-γ-induced iNOS expression in mouse regulatory macrophages prolongs allograft survival in fully immunocompetent recipients. Mol Ther. (2013) 21(2):409–22. doi: 10.1038/mt.2012.168
84. Riquelme P, Gövert F, Geissler EK, Fändrich F, Hutchinson JA. Human transplant acceptance-inducing cells suppress mitogen-stimulated T cell proliferation. Transpl Immunol. (2009) 21(3):162–5. doi: 10.1016/j.trim.2009.03.004
85. Hutchinson JA, Riquelme P, Geissler EK, Fändrich F. Human regulatory macrophages. Methods Mol Biol. (2011) 677:181–92. doi: 10.1007/978-1-60761-869-0_13
86. Hutchinson JA, Riquelme P, Sawitzki B, Tomiuk S, Miqueu P, Zuhayra M, et al. Cutting edge: immunological consequences and trafficking of human regulatory macrophages administered to renal transplant recipients. J Immunol. (2011) 187(5):2072–8. doi: 10.4049/jimmunol.1100762
87. Mosser DM, Zhang X. Activation of murine macrophages. Curr Protoc Immunol (2008). Chapter 14:14.2.1-14.2.8. doi: 10.1002/0471142735.im1402s83
88. Edwards JP, Zhang X, Frauwirth KA, Mosser DM. Biochemical and functional characterization of three activated macrophage populations. J Leukoc Biol. (2006) 80(6):1298–307. doi: 10.1189/jlb.0406249
89. Narasimhan PB, Akabas L, Tariq S, Huda N, Bennuru S, Sabzevari H, et al. Similarities and differences between helminth parasites and cancer cell lines in shaping human monocytes: insights into parallel mechanisms of immune evasion. PLoS Negl Trop Dis. (2018) 12(4):e0006406. doi: 10.1371/journal.pntd.0006404
90. Christensen SM, Belew AT, El-Sayed NM, Tafuri WL, Silveira FT, Mosser DM. Host and parasite responses in human diffuse cutaneous leishmaniasis caused by L. amazonensis. PLoS Negl Trop Dis. (2018) 13(3):e0007152. doi: 10.1371/journal.pntd.0007152
91. Diao J, Mikhailova A, Tang M, Gu H, Zhao J, Cattral MS. Immunostimulatory conventional dendritic cells evolve into regulatory macrophage-like cells. Blood. (2012) 119(21):4919–27. doi: 10.1182/blood-2011-11-392894
92. Zhang F, Zhang J, Cao P, Sun Z, Wang W. The characteristics of regulatory macrophages and their roles in transplantation. Int Immunopharmacol. (2021) 91:107322. doi: 10.1016/j.intimp.2020.107322
93. Riquelme P, Haarer J, Kammler A, Walter L, Tomiuk S, Ahrens N, et al. TIGIT+ iTregs elicited by human regulatory macrophages control T cell immunity. Nat Commun. (2018) 9(1):2858. doi: 10.1038/s41467-018-05167-8
94. Morelli AE. Promise of regulatory macrophage (Mreg)-induced T-cell immunosuppression. Transplantation. (2019) 103(7):1291–2. doi: 10.1097/TP.0000000000002637
95. Schmidt A, Zhang XM, Joshi RN, Iqbal S, Wahlund C, Gabrielsson S, et al. Human macrophages induce CD4+Foxp3+regulatory T cells via binding and re-release of TGF-β. Immunol Cell Biol. (2016) 94(8):747–62. doi: 10.1038/icb.2016.34
96. Riquelme P, Hutchinson JA. Novel molecules mediate specialized functions of human regulatory macrophages. Curr Opin Organ Transplant. (2018) 23(5):533–7. doi: 10.1097/MOT.0000000000000560
97. Pilling D, Gomer RH. Persistent lung inflammation and fibrosis in serum amyloid P component (Apcs-/-) knockout mice. PLoS One. (2014) 9(4):e93730. doi: 10.1371/journal.pone.0093730
98. Hummitzsch L, Zitta K, Rusch R, Cremer J, Steinfath M, Gross J, et al. Characterization of the angiogenic potential of human regulatory macrophages (MREG) after ischemia/reperfusion injury in vitro. Stem Cells Int. (2019) 2019:3725863. doi: 10.1155/2019/3725863
99. Mbiribindi B, Harden JT, Pena JK, Krams SM. Natural killer cells as modulators of alloimmune responses. Curr Opin Organ Transplant. (2019) 24(1):37–41. doi: 10.1097/MOT.0000000000000590
100. Kiessling R, Klein E, Wigzell H. “Natural” killer cells in the mouse. I. Cytotoxic cells with specificity for mouse Moloney leukemia cells. Specificity and distribution according to genotype. Eur J Immunol. (1975) 5(2):112–7. doi: 10.1002/eji.1830050208
101. Manilay JO, Sykes M. Natural killer cells and their role in graft rejection. Curr Opin Immunol. (1998) 10(5):532–8. doi: 10.1016/S0952-7915(98)80219-7
102. Lanier LL. NK Cell recognition. Annu Rev Immunol. (2005) 23:225–74. doi: 10.1146/annurev.immunol.23.021704.115526
103. Caligiuri MA. Human natural killer cells. Blood. (2008) 112(3):461–9. doi: 10.1182/blood-2007-09-077438
104. Fehniger TA, Shah MH, Turner MJ, VanDeusen JB, Whitman SP, Cooper MA, et al. Differential cytokine and chemokine gene expression by human NK cells following activation with IL-18 or IL-15 in combination with IL-12: implications for the innate immune response. J Immunol. (1999) 162(8):4511–20. doi: 10.4049/jimmunol.162.8.4511
105. Cooper MA, Fehniger TA, Caligiuri MA. The biology of human natural killer-cell subsets. Trends Immunol. (2001) 22(11):633–40. doi: 10.1016/S1471-4906(01)02060-9
106. Ferlazzo G, Morandi B, D’Agostino A, Meazza R, Melioli G, Moretta A, et al. The interaction between NK cells and dendritic cells in bacterial infections results in rapid induction of NK cell activation and in the lysis of uninfected dendritic cells. Eur J Immunol. (2003) 33(2):306–13. doi: 10.1002/immu.200310004
107. Hayakawa Y, Screpanti V, Yagita H, Grandien A, Ljunggren HG, Smyth MJ, et al. NK cell TRAIL eliminates immature dendritic cells in vivo and limits dendritic cell vaccination efficacy. J Immunol. (2004) 172(1):123–9. doi: 10.4049/jimmunol.172.1.123
108. Pontrelli P, Rascio F, Castellano G, Grandaliano G, Gesualdo L, Stallone G. The role of natural killer cells in the immune response in kidney transplantation. Front Immunol. (2020) 11:1454. doi: 10.3389/fimmu.2020.01454
109. Huang H, Lu Y, Zhou T, Gu G, Xia Q. Innate immune cells in immune tolerance after liver transplantation. Front Immunol. (2018) 9:2401. doi: 10.3389/fimmu.2018.02401
110. Laffont S, Seillet C, Ortaldo J, Coudert JD, Guéry JC. Natural killer cells recruited into lymph nodes inhibit alloreactive T-cell activation through perforin-mediated killing of donor allogeneic dendritic cells. Blood. (2008) 112(3):661–71. doi: 10.1182/blood-2007-10-120089
111. Yu G, Xu X, Minh DV, Kilpatrick ED, Xian CL. NK Cells promote transplant tolerance by killing donor antigen-presenting cells. J Exp Med. (2006) 203(8):1851–8. doi: 10.1084/jem.20060603
112. Hadad U, Martinez O, Krams SM. NK Cells after transplantation: friend or foe. Immunol Res. (2014) 58(2–3):259–67. doi: 10.1007/s12026-014-8493-4
113. Bose A, Inoue Y, Kokko KE, Lakkis FG. Cutting edge: perforin down-regulates CD4 and CD8 T cell-mediated immune responses to a transplanted organ. J Immunol. (2003) 170(4):1611–4. doi: 10.4049/jimmunol.170.4.1611
114. Rabinovich BA, Li J, Shannon J, Hurren R, Chalupny J, Cosman D, et al. Activated, but not resting, T cells can be recognized and killed by syngeneic NK cells. J Immunol. (2003) 170(7):3572–6. doi: 10.4049/jimmunol.170.7.3572
115. Djeu JY, Stocks N, Zoon K, Stanton GJ, Timonen T, Herberman RB. Positive self regulation of cytotoxicity in human natural killer cells by production of interferon upon exposure to influenza and herpes viruses. J Exp Med. (1982) 156(4):1222–34. doi: 10.1084/jem.156.4.1222
116. Welsh RM. Cytotoxic cells induced during lymphocytic choriomeningitis virus infection of mice: I. Characterization of natural killer cell induction. J Exp Med. (1978) 148(1):163–81. doi: 10.1084/jem.148.1.163
117. Maroof A, Beattie L, Zubairi S, Svensson M, Stager S, Kaye PM. Posttranscriptional regulation of Il10 gene expression allows natural killer cells to express immunoregulatory function. Immunity. (2008) 29(2):295–305. doi: 10.1016/j.immuni.2008.06.012
118. Vacca P, Cantoni C, Vitale M, Prato C, Canegallo F, Fenoglio D, et al. Crosstalk between decidual NK and CD14+ myelomonocytic cells results in induction of Tregs and immunosuppression. Proc Natl Acad Sci USA. (2010) 107(26):11918–23. doi: 10.1073/pnas.1001749107
119. Pedroza-Pacheco I, Madrigal A, Saudemont A. Interaction between natural killer cells and regulatory T cells: perspectives for immunotherapy. Cell Mol Immunol. (2013) 10(3):222–9. doi: 10.1038/cmi.2013.2
120. Deniz G, Erten G, Kücüksezer UC, Kocacik D, Karagiannidis C, Aktas E, et al. Regulatory NK cells suppress antigen-specific T cell responses. J Immunol. (2008) 180(2):850–7. doi: 10.4049/jimmunol.180.2.850
121. Beilke JN, Kuhl NR, van Kaer L, Gill RG. NK Cells promote islet allograft tolerance via a perforin-dependent mechanism. Nat Med. (2005) 11(10):1059–65. doi: 10.1038/nm1296
122. Fabritius C, Ritschl PV, Resch T, Roth M, Ebner S, Günther J, et al. Deletion of the activating NK cell receptor NKG2D accelerates rejection of cardiac allografts. Am J Transplant. (2017) 17(12):3199–209. doi: 10.1111/ajt.14467
123. Tripathi D, Venkatasubramanian S, Cheekatla SS, Paidipally P, Welch E, Tvinnereim AR, et al. A TLR9 agonist promotes IL-22-dependent pancreatic islet allograft survival in type 1 diabetic mice. Nat Commun. (2016) 7:13896. 10.1038.ncomms13896 27982034
124. Dugast E, David G, Oger R, Danger R, Judor JP, Gagne K, et al. Broad impairment of natural killer cells from operationally tolerant kidney transplanted patients. Front Immunol. (2017) 8:1721. doi: 10.3389/fimmu.2017.01721
125. Fan QR, Long EO, Wiley DC. Crystal structure of the human natural killer cell inhibitory receptor KIR2DL1-HLA-Cw4 complex. Nat Immunol. (2001) 2(5):452–60. doi: 10.1038/87766
126. Rajagopalan S, Bryceson YT, Kuppusamy SP, Geraghty DE, van der Meer A, Joosten I, et al. Activation of NK cells by an endocytosed receptor for soluble HLA-G. PLoS Biol. (2006) 4(1):e9. doi: 10.1371/journal.pbio.0040009
127. Fu B, Wei H. Decidual natural killer cells and the immune microenvironment at the maternal-fetal interface. Sci China Life Sci. (2016) 59(12):1224–31. doi: 10.1007/s11427-016-0337-1
128. Ashkar AA, di Santo JP, Croy BA. Interferon γ contributes to initiation of uterine vascular modification, decidual integrity, and uterine natural killer cell maturation during normal murine pregnancy. J Exp Med. (2000) 192(2):259–70. doi: 10.1084/jem.192.2.259
129. Fu B, Li X, Sun R, Tong X, Ling B, Tian Z, et al. Natural killer cells promote immune tolerance by regulating inflammatory TH17 cells at the human maternal-fetal interface. Proc Natl Acad Sci USA. (2013) 110(3):E231–40. doi: 10.1073/pnas.1206322110
130. Felker AM, Croy BA. Uterine natural killer cell partnerships in early mouse decidua basalis. J Leukoc Biol. (2016) 100(4):645–55. doi: 10.1189/jlb.1HI0515-226R
131. García de la Garza R, Sarobe P, Merino J, Lasarte JJ, D’Avola D, Belsue V, et al. Immune monitoring of immunosuppression withdrawal of liver transplant recipients. Transpl Immunol. (2015) 33(2):110–6. doi: 10.1016/j.trim.2015.07.006
132. Parkes MD, Halloran PF, Hidalgo LG. Evidence for CD16a-mediated NK cell stimulation in antibody-mediated kidney transplant rejection. Transplantation. (2017) 101(4):e102–11. doi: 10.1097/TP.0000000000001568
133. Littera R, Piredda G, Argiolas D, Lai S, Congeddu E, Ragatzu P, et al. KIR And their HLA class I ligands: two more pieces towards completing the puzzle of chronic rejection and graft loss in kidney transplantation. PLoS One. (2017) 12(7):e0180831. doi: 10.1371/journal.pone.0180831
134. Zhu L, Aly M, Wang H, Karakizlis H, Weimer R, Morath C, et al. Decreased NK cell immunity in kidney transplant recipients late post-transplant and increased NK-cell immunity in patients with recurrent miscarriage. PLoS One. (2017) 12(10):e0186349. doi: 10.1371/journal.pone.0186349
135. de Carolis C, Perricone C, Perricone R. NK Cells, autoantibodies, and immunologic infertility: a complex interplay. Clin Rev Allergy Immunol. (2010) 39(3):166–75. doi: 10.1007/s12016-009-8184-4
136. Mori M, Bogdan A, Balassa T, Csabai T, Szekeres-Bartho J. The decidua—the maternal bed embracing the embryo—maintains the pregnancy. Semin Immunopathol. (2016) 38(6):635–49. doi: 10.1007/s00281-016-0574-0
137. Cella M, Miller H, Song C. Beyond NK cells: the expanding universe of innate lymphoid cells. Front Immunol. (2014) 5:282. doi: 10.3389/fimmu.2014.00282
138. Artis D, Spits H. The biology of innate lymphoid cells. Nature. (2015) 517(7534):293–301. doi: 10.1038/nature14189
139. Yagi R, Zhong C, Northrup DL, Yu F, Bouladoux N, Spencer S, et al. The transcription factor GATA3 is critical for the development of all IL-7Rα-expressing innate lymphoid cells. Immunity. (2014) 40(3):378–88. doi: 10.1016/j.immuni.2014.01.012
140. Klose CSN, Flach M, Möhle L, Rogell L, Hoyler T, Ebert K, et al. Differentiation of type 1 ILCs from a common progenitor to all helper-like innate lymphoid cell lineages. Cell. (2014) 157(2):340–56. doi: 10.1016/j.cell.2014.03.030
141. Constantinides MG, McDonald BD, Verhoef PA, Bendelac A. A committed precursor to innate lymphoid cells. Nature. (2014) 508(7496):397–401. doi: 10.1038/nature13047
142. Sonnenberg GF, Hepworth MR. Functional interactions between innate lymphoid cells and adaptive immunity. Nat Rev Immunol. (2019) 19(10):599–613. doi: 10.1038/s41577-019-0194-8
143. Price AE, Liang HE, Sullivan BM, Reinhardt RL, Eisley CJ, Erle DJ, et al. Systemically dispersed innate IL-13-expressing cells in type 2 immunity. Proc Natl Acad Sci USA. (2010) 107(25):11489–94. doi: 10.1073/pnas.1003988107
144. Neill DR, Wong SH, Bellosi A, Flynn RJ, Daly M, Langford TKA, et al. Nuocytes represent a new innate effector leukocyte that mediates type-2 immunity. Nature. (2010) 464(7293):1367–70. doi: 10.1038/nature08900
145. Moro K, Yamada T, Tanabe M, Takeuchi T, Ikawa T, Kawamoto H, et al. Innate production of TH 2 cytokines by adipose tissue-associated c-Kit+ Sca-1 + lymphoid cells. Nature. (2010) 463(7280):540–4. doi: 10.1038/nature08636
146. Monticelli LA, Sonnenberg GF, Abt MC, Alenghat T, Ziegler CGK, Doering TA, et al. Innate lymphoid cells promote lung-tissue homeostasis after infection with influenza virus. Nat Immunol. (2011) 12(11):1045–54. doi: 10.1038/ni.2131
147. Molofsky AB, Nussbaum JC, Liang HE, Dyken SJV, Cheng LE, Mohapatra A, et al. Innate lymphoid type 2 cells sustain visceral adipose tissue eosinophils and alternatively activated macrophages. J Exp Med. (2013) 210(3):535–49. doi: 10.1084/jem.20121964
148. Mjösberg JM, Trifari S, Crellin NK, Peters CP, van Drunen CM, Piet B, et al. Human IL-25-and IL-33-responsive type 2 innate lymphoid cells are defined by expression of CRTH2 and CD161. Nat Immunol. (2011) 12(11):1055–62. doi: 10.1038/ni.2104
149. Kim BS, Siracusa MC, Saenz SA, Noti M, Monticelli LA, Sonnenberg GF, et al. TSLP Elicits IL-33-independent innate lymphoid cell responses to promote skin inflammation. Sci Transl Med. (2013) 5(170):170ra16. doi: 10.1126/scitranslmed.3005374
150. Brestoff JR, Kim BS, Saenz SA, Stine RR, Monticelli LA, Sonnenberg GF, et al. Group 2 innate lymphoid cells promote beiging of white adipose tissue and limit obesity. Nature. (2015) 519(7542):242–6. doi: 10.1038/nature14115
151. Veiga-Fernandes H, Artis D. Neuronal-immune system cross-talk in homeostasis. Science. (2018) 359(6383):1465–6. doi: 10.1126/science.aap9598
152. Spits H, Artis D, Colonna M, Diefenbach A, di Santo JP, Eberl G, et al. Innate lymphoid cells-a proposal for uniform nomenclature. Nat Rev Immunol. (2013) 13(2):145–9. doi: 10.1038/nri3365
153. Spits H, di Santo JP. The expanding family of innate lymphoid cells: regulators and effectors of immunity and tissue remodeling. Nat Immunol. (2011) 12(1):21–7. doi: 10.1038/ni.1962
154. Ignacio A, Breda CNS, Camara NOS. Innate lymphoid cells in tissue homeostasis and diseases. World J Hepatol. (2017) 9(23):979–89. doi: 10.4254/wjh.v9.i23.979
155. Wilhelm C, Hirota K, Stieglitz B, van Snick J, Tolaini M, Lahl K, et al. An IL-9 fate reporter demonstrates the induction of an innate IL-9 response in lung inflammation. Nat Immunol. (2011) 12(11):1071–7. doi: 10.1038/ni.2133
156. Schulz-Kuhnt A, Neurath MF, Wirtz S, Atreya I. Innate lymphoid cells as regulators of epithelial integrity: therapeutic implications for inflammatory bowel diseases. Front Med (Lausanne). (2021) 8:656745. doi: 10.3389/fmed.2021.656745
157. Bouchery T, Kyle R, Camberis M, Shepherd A, Filbey K, Smith A, et al. ILC2s And T cells cooperate to ensure maintenance of M2 macrophages for lung immunity against hookworms. Nat Commun. (2015) 6:6970. doi: 10.1038/ncomms7970
158. Hepworth MR, Monticelli LA, Fung TC, Ziegler CGK, Grunberg S, Sinha R, et al. Innate lymphoid cells regulate CD4+T-cell responses to intestinal commensal bacteria. Nature. (2013) 498(7452):113–7. doi: 10.1038/nature12240
159. Schneider AK, Chevalier MF, Derré L. The multifaceted immune regulation of bladder cancer. Nat Rev Urol. (2019) 16(10):613–30. doi: 10.1038/s41585-019-0226-y
160. Wang S, Zhang Y, Wang Y, Ye P, Li J, Li H, et al. Amphiregulin confers regulatory T cell suppressive function and tumor invasion via the EGFR/GSK-3β/Foxp3 axis. J Biol Chem. (2016) 291(40):21085–95. doi: 10.1074/jbc.M116.717892
161. You Y, Zhang X, Wang X, Yue D, Meng F, Zhu J, et al. ILC2 proliferated by IL-33 stimulation alleviates acute colitis in Rag1-/-mouse through promoting M2 macrophage polarization. J Immunol Res. (2020) 2020:5018975. doi: 10.1155/2020/5018975
162. Zaiss DMW, Gause WC, Osborne LC, Artis D. Emerging functions of amphiregulin in orchestrating immunity, inflammation, and tissue repair. Immunity. (2015) 42(2):216–26. doi: 10.1016/j.immuni.2015.01.020
163. Zaiss DMW, van Loosdregt J, Gorlani A, Bekker CPJ, Gröne A, Sibilia M, et al. Amphiregulin enhances regulatory T cell-suppressive function via the epidermal growth factor receptor. Immunity. (2013) 38(2):275–84. doi: 10.1016/j.immuni.2012.09.023
164. Cao Q, Wang Y, Niu Z, Wang C, Wang R, Zhang Z, et al. Potentiating tissue-resident type 2 innate lymphoid cells by IL-33 to prevent renal ischemia-reperfusion injury. J Am Soc Nephrol. (2018) 29(3):961–76. doi: 10.1681/ASN.2017070774
165. Biedermann T, Röcken M, Carballido JM. TH1 and TH2 lymphocyte development and regulation of TH cell-mediated immune responses of the skin. J Investig Dermatol Symp Proc. (2004) 9(1):5–14. doi: 10.1111/j.1087-0024.2004.00829.x
166. Kaiko GE, Horvat JC, Beagley KW, Hansbro PM. Immunological decision-making: how does the immune system decide to mount a helper T-cell response? Immunology. (2008) 123(3):326–38. doi: 10.1111/j.1365-2567.2007.02719.x
167. Rauber S, Luber M, Weber S, Maul L, Soare A, Wohlfahrt T, et al. Resolution of inflammation by interleukin-9-producing type 2 innate lymphoid cells. Nat Med. (2017) 23(8):938–44. doi: 10.1038/nm.4373
168. Chevalier MF, Trabanelli S, Racle J, Salomé B, Cesson V, Gharbi D, et al. ILC2-modulated T cell-to-MDSC balance is associated with bladder cancer recurrence. J Clin Invest. (2017) 127(8):2916–29. doi: 10.1172/JCI89717
169. Trabanelli S, Chevalier MF, Martinez-Usatorre A, Gomez-Cadena A, Salomé B, Lecciso M, et al. Tumour-derived PGD2 and NKp30-B7H6 engagement drives an immunosuppressive ILC2-MDSC axis. Nat Commun. (2017) 8(1):593. doi: 10.1038/s41467-017-00678-2
170. Klose CSN, Artis D. Innate lymphoid cells as regulators of immunity, inflammation and tissue homeostasis. Nat Immunol. (2016) 17(7):765–74. doi: 10.1038/ni.3489
171. Cording S, Medvedovic J, Aychek T, Eberl G. Innate lymphoid cells in defense, immunopathology and immunotherapy. Nat Immunol. (2016) 17(7):755–7. doi: 10.1038/ni.3448
172. Monticelli LA, Osborne LC, Noti M, Tran SV, Zaiss DMW, Artis D. IL-33 promotes an innate immune pathway of intestinal tissue protection dependent on amphiregulin-EGFR interactions. Proc Natl Acad Sci USA. (2015) 112(34):10762–7. doi: 10.1073/pnas.1509070112
173. Huang Q, Niu Z, Tan J, Yang J, Liu Y, Ma H, et al. IL-25 elicits innate lymphoid cells and multipotent progenitor type 2 cells that reduce renal ischemic/reperfusion injury. J Am Soc Nephrol. (2015) 26(9):2199–211. doi: 10.1681/ASN.2014050479
174. Monticelli LA, Diamond JM, Saenz SA, Tait Wojno ED, Porteous MK, Cantu E, et al. Lung innate lymphoid cell composition is altered in primary graft dysfunction. Am J Respir Crit Care Med. (2020) 201(1):63–72. doi: 10.1164/rccm.201906-1113OC
175. Drujont L, Carretero-Iglesia L, Bouchet-Delbos L, Beriou G, Merieau E, Hill M, et al. Evaluation of the therapeutic potential of bone Marrow-Derived Myeloid Suppressor Cell (MDSC) adoptive transfer in mouse models of autoimmunity and allograft rejection. PLoS One. (2014) 9(6):e100013. doi: 10.1371/journal.pone.0100013
176. Zhang C, Wang S, Li J, Zhang W, Zheng L, Yang C, et al. The mtor signal regulates myeloid-derived suppressor cells differentiation and immunosuppressive function in acute kidney injury. Cell Death Dis. (2017) 8(3):e2695. doi: 10.1038/cddis.2017.86
177. Pinton L, Solito S, Damuzzo V, Francescato S, Pozzuoli A, Berizzi A, et al. Activated T cells sustain myeloid-derived suppressor cell-mediated immune suppression. Oncotarget. (2016) 7(2):1168–84. doi: 10.18632/oncotarget.6662
178. Fillatreau S, Sweenie CH, McGeachy MJ, Gray D, Anderton SM. B cells regulate autoimmunity by provision of IL-10. Nat Immunol. (2002) 3(10):944–50. doi: 10.1038/ni833
179. Marigo I, Bosio E, Solito S, Mesa C, Fernandez A, Dolcetti L, et al. Tumor-induced tolerance and immune suppression depend on the C/EBPβ transcription factor. Immunity. (2010) 32(6):790–802. doi: 10.1016/j.immuni.2010.05.010
180. Yang F, Li Y, Wu T, Na N, Zhao Y, Li W, et al. TNFα-induced M-MDSCs promote transplant immune tolerance via nitric oxide. J Mol Med. (2016) 94(8):911–20. doi: 10.1007/s00109-016-1398-z
181. Wu T, Sun C, Chen Z, Zhen Y, Peng J, Qi Z, et al. Smad3-Deficient CD11b+Gr1+myeloid-derived suppressor cells prevent allograft rejection via the nitric oxide pathway. J Immunol. (2012) 189(10):4989–5000. doi: 10.4049/jimmunol.1200068
182. Carretero-Iglesia L, Bouchet-Delbos L, Louvet C, Drujont L, Segovia M, Merieau E, et al. Comparative study of the immunoregulatory capacity of in vitro generated tolerogenic dendritic cells, suppressor macrophages, and myeloid-derived suppressor cells. Transplantation. (2016) 100(10):2079–89. doi: 10.1097/TP.0000000000001315
183. Adeegbe D, Serafini P, Bronte V, Zoso A, Ricordi C, Inverardi L. In vivo induction of myeloid suppressor cells and CD4 +foxp3+T regulatory cells prolongs skin allograft survival in mice. Cell Transplant. (2011) 20(6):941–54. doi: 10.3727/096368910X540621
184. Gajardo T, Morales RA, Campos-Mora M, Campos-Acuña J, Pino-Lagos K. Exogenous interleukin-33 targets myeloid-derived suppressor cells and generates periphery-induced Foxp3 + regulatory T cells in skin-transplanted mice. Immunology. (2015) 146(1):81–8. doi: 10.1111/imm.12483
185. Turnquist HR, Zhao Z, Rosborough BR, Liu Q, Castellaneta A, Isse K, et al. IL-33 expands suppressive CD11b+ gr-1 int and regulatory T cells, including ST2l+ Foxp3+ cells, and mediates regulatory T cell-dependent promotion of cardiac allograft survival. J Immunol. (2011) 187(9):4598–610. doi: 10.4049/jimmunol.1100519
186. Bryant J, Lerret NM, Wang JJ, Kang HK, Tasch J, Zhang Z, et al. Preemptive donor apoptotic cell infusions induce IFN-γ–producing myeloid-derived suppressor cells for cardiac allograft protection. J Immunol. (2014) 192(12):6092–101. doi: 10.4049/jimmunol.1302771
187. Janikashvili N, Gérard C, Thébault M, Brazdova A, Boibessot C, Cladière C, et al. Efficiency of human monocyte-derived suppressor cell-based treatment in graft-versus-host disease prevention while preserving graft-versus-leukemia effect. Oncoimmunology. (2021) 10(1):1880046. doi: 10.1080/2162402X.2021.1880046
188. Gérard C, Thébault M, Lamarthée B, Genet C, Cattin F, Brazdova A, et al. Human monocyte-derived suppressor cell supernatant induces immunoregulatory effects and mitigates xenoGvHD. Front Immunol. (2022) 13:827712. doi: 10.3389/fimmu.2022.827712
189. Janikashvili N, Trad M, Gautheron A, Samson M, Lamarthée B, Bonnefoy F, et al. Human monocyte-derived suppressor cells control graft-versus-host disease by inducing regulatory forkhead box protein 3-positive CD8+ T lymphocytes. J Allergy Clin Immunol. (2015) 135(6):1614–24. doi: 10.1016/j.jaci.2014.12.1868
190. Sato K, Yamashita N, Yamashita N, Baba M, Matsuyama T. Regulatory dendritic cells protect mice from murine acute graft-versus-host disease and leukemia relapse. Immunity. (2003) 18(3):367–79. doi: 10.1016/S1074-7613(03)00055-4
191. Nestle FO, Banchereau J, Hart D. Dendritic cells: on the move from bench to bedside. Nat Med. (2001) 7(7):761–5. doi: 10.1038/89863
192. Thomson AW, Zahorchak AF, Ezzelarab MB, Butterfield LH, Lakkis FG, Metes DM. Prospective clinical testing of regulatory dendritic cells in organ transplantation. Front Immunol. (2016) 7:15. doi: 10.3389/fimmu.2016.00015
193. Adorini L, Penna G, Giarratana N, Uskokovic M. Tolerogenic dendritic cells induced by vitamin D receptor ligands enhance regulatory T cells inhibiting allograft rejection and autoimmune diseases. J Cell Biochem. (2003) 88(2):227–33. doi: 10.1002/jcb.10340
194. Rutella S, Danese S, Leone G. Tolerogenic dendritic cells: cytokine modulation comes of age. Blood. (2006) 108(5):1435–40. doi: 10.1182/blood-2006-03-006403
195. Morelli AE, Thomson AW. Tolerogenic dendritic cells and the quest for transplant tolerance. Nat Rev Immunol. (2007) 7(8):610–21. doi: 10.1038/nri2132
196. Du X, Chang S, Guo W, Zhang S, Chen ZK. Progress in liver transplant tolerance and tolerance-inducing cellular therapies. Front Immunol. (2020) 11:1326. doi: 10.3389/fimmu.2020.01326
197. ten Brinke A, Martinez-Llordella M, Cools N, Hilkens CMU, van Ham SM, Sawitzki B, et al. Ways forward for tolerance-inducing cellular therapies- an AFACTT perspective. Front Immunol. (2019) 10:181. doi: 10.3389/fimmu.2019.00181
198. Sato K, Yamashita N, Baba M, Matsuyama T. Modified myeloid dendritic cells act as regulatory dendritic cells to induce anergic and regulatory T cells. Blood. (2003) 101(9):3581–9. doi: 10.1182/blood-2002-09-2712
199. Stoop JN, Robinson JH, Hilkens CMU. Developing tolerogenic dendritic cell therapy for rheumatoid arthritis: what can we learn from mouse models? Ann Rheum Dis. (2011) 70(9):1526–33. doi: 10.1136/ard.2011.151654
200. Natarajan S, Thomson AW. Tolerogenic dendritic cells and myeloid-derived suppressor cells: potential for regulation and therapy of liver auto- and alloimmunity. Immunobiology. (2010) 215(9–10):698–703. doi: 10.1016/j.imbio.2010.05.024
201. Thomson AW, Robbins PD. Tolerogenic dendritic cells for autoimmune disease and transplantation. Ann Rheum Dis. (2008) 67(Suppl 3):iii90–6. doi: 10.1136/ard.2008.099176
202. Giannoukakis N, Phillips B, Finegold D, Harnaha J, Trucco M. Phase I (safety) study of autologous tolerogenic dendritic cells in type 1 diabetic patients. Diabetes Care. (2011) 34(9):2026–32. doi: 10.2337/dc11-0472
203. Jauregui-Amezaga A, Cabezón R, Ramírez-Morros A, España C, Rimola J, Bru C, et al. Intraperitoneal administration of autologous tolerogenic dendritic cells for refractory crohn’s disease: a phase I study. J Crohns Colitis. (2015) 9(12):1071–8. doi: 10.1093/ecco-jcc/jjv144
204. ten Brinke A, Hilkens CMU, Cools N, Geissler EK, Hutchinson JA, Lombardi G, et al. Clinical use of tolerogenic dendritic cells-harmonization approach in European collaborative effort. Mediat Inflamm. (2015) 2015:471719. doi: 10.1155/2015/471719
205. Bell GM, Anderson AE, Diboll J, Reece R, Eltherington O, Harry RA, et al. Autologous tolerogenic dendritic cells for rheumatoid and inflammatory arthritis. Ann Rheum Dis. (2017) 76(1):227–34. doi: 10.1136/annrheumdis-2015-208456
206. Willekens B, Presas-Rodríguez S, Mansilla MJ, Derdelinckx J, Lee WP, Nijs G, et al. Tolerogenic dendritic cell-based treatment for multiple sclerosis (MS): a harmonised study protocol for two phase I clinical trials comparing intradermal and intranodal cell administration. BMJ Open. (2019) 9(9):e030309. doi: 10.1136/bmjopen-2019-030309
207. Lutz MB, Suri RM, Niimi M, Ogilvie ALJ, Kukutsch NA, Rößner S, et al. Immature dendritic cells generated with low doses of GM-CSF in the absence of IL-4 are maturation resistant and prolong allograft survival in vivo. Eur J Immunol. (2000) 30(7):1813–22. doi: 10.1002/1521-4141(200007)30:7%3C1813::AID-IMMU1813%3E3.0.CO;2-8
208. Hayamizu K, Huie P, Sibley RK, Strober S. Monocyte-derived dendritic cell precursors facilitate tolerance to heart allografts after total lymphoid irradiation. Transplantation. (1998) 66(10):1285–91. doi: 10.1097/00007890-199811270-00004
209. Lan YY, Wang Z, Raimondi G, Wu W, Colvin BL, de Creus A, et al. “Alternatively activated” dendritic cells preferentially secrete IL-10, expand Foxp3+CD4+T cells, and induce long-term organ allograft survival in combination with CTLA4-ig. J Immunol. (2006) 177(9):5868–77. doi: 10.4049/jimmunol.177.9.5868
210. Bonham CA, Peng L, Liang X, Chen Z, Wang L, Ma L, et al. Marked prolongation of cardiac allograft survival by dendritic cells genetically engineered with NF-κB oligodeoxyribonucleotide decoys and adenoviral vectors encoding CTLA4-ig. J Immunol. (2002) 169(6):3382–91. doi: 10.4049/jimmunol.169.6.3382
211. Lina LU, Wei LI, Fumin FU, Chambers FG, Qian S, Fung JJ, et al. Blockade of the CD40-CD40 ligand pathway potentiates the capacity of donor-derived dendritic cell progenitors to induce long-term cardiac allograft survival. Transplantation. (1997) 64(12):1808–15. doi: 10.1097/00007890-199712270-00031
212. Lu L, Li W, Zhong C, Qian S, Fung JJ, Thomson AW, et al. Increased apoptosis of immunoreactive host cells and augmented donor leukocyte chimerism, not sustained inhibition of B7 molecule expression are associated with prolonged cardiac allograft survival in mice preconditioned with immature donor dendritic cells plus anti-CD40L mAb. Transplantation. (1999) 68(6):747–57. doi: 10.1097/00007890-199909270-00006
213. Ikeguchi R, Sacks JM, Unadkat JV, Solari M, Horibe EK, Thomson AW, et al. Long-term survival of limb allografts induced by pharmacologically conditioned, donor alloantigen-pulsed dendritic cells without maintenance immunosuppression. Transplantation. (2008) 85(2):237–46. doi: 10.1097/TP.0b013e31815e870e
214. Eun SC, Baek RM, Park CG. Prolongation of the rat composite tissue allograft survival by the combination of tolerogenic immature dendritic cells and short-term treatment with FK506. Transplant Proc. (2013) 45(5):1792–6. doi: 10.1016/j.transproceed.2013.01.021
215. Ezzelarab MB, Lu L, Guo H, Zahorchak AF, Shufesky WF, Cooper DKC, et al. Eomesoderminlo CTLA4hi alloreactive CD8+ memory T cells are associated with prolonged renal transplant survival induced by regulatory dendritic cell infusion in CTLA4 immunoglobulin-treated nonhuman primates. Transplantation. (2016) 100(1):91–102. doi: 10.1097/TP.0000000000000871
216. Ezzelarab MB, Zahorchak AF, Lu L, Morelli AE, Chalasani G, Demetris AJ, et al. Regulatory dendritic cell infusion prolongs kidney allograft survival in nonhuman primates. Am J Transplant. (2013) 13(8):1989–2005. doi: 10.1111/ajt.12310
217. DePaz HA, Oluwole OO, Adeyeri AO, Witkowski P, Jin MX, Hardy MA, et al. Immature rat myeloid dendritic cells generated in low-dose granulocyte macrophage-colony stimulating factor prolong donor-specific rat cardiac allograft survival. Transplantation. (2003) 75(4):521–8. doi: 10.1097/01.TP.0000048380.84355.4A
218. Mirenda V, Berton I, Read J, Cook T, Smith J, Dorling A, et al. Modified dendritic cells coexpressing self and allogeneic major histocompatability complex molecules: an efficient way to induce indirect pathway regulation. J Am Soc Nephrol. (2004) 15(4):987–97. doi: 10.1097/01.ASN.0000119575.98696.1D
219. Wang Q, Zhang M, Ding G, Liu Y, Sun Y, Wang J, et al. Anti-ICAM-1 antibody and CTLA-4Ig synergistically enhance immature dendritic cells to induce donor-specific immune tolerance in vivo. Immunol Lett. (2003) 90(1):33–42. doi: 10.1016/S0165-2478(03)00160-3
220. Garrovillo M, Ali A, Oluwole SF. Indirect allorecognition in acquired thymic tolerance: induction of donor-specific tolerance to rat cardiac allografts by allopeptide-pulsed host dendritic cells. Transplantation. (1999) 68(12):1827–34. doi: 10.1097/00007890-199912270-00001
221. Ali A, Garrovillo M, Jin MX, Hardy MA, Oluwole SF. Major histocompatibility complex class I peptide-pulsed host dendritic cells induce antigen-specific acquired thymic tolerance to islet cells. Transplantation. (2000) 69(2):221–6. doi: 10.1097/00007890-200001270-00005
222. Baas MC, Kuhn C, Valette F, Mangez C, Duarte MS, Hill M, et al. Combining autologous dendritic cell therapy with CD3 antibodies promotes regulatory T cells and permanent islet allograft acceptance. J Immunol. (2014) 193(9):4696–703. doi: 10.4049/jimmunol.1401423
223. Oluwole OO, Depaz HA, Gopinathan R, Ali A, Garrovillo M, Jin MX, et al. Indirect allorecognition in acquired thymic tolerance: induction of donor-specific permanent acceptance of rat islets by adoptive transfer of allopeptide-pulsed host myeloid and thymic dendritic cells. Diabetes. (2001) 50(7):1546–52. doi: 10.2337/diabetes.50.7.1546
224. Taner T, Hackstein H, Wang Z, Morelli AE, Thomson AW. Rapamycin-treated, alloantigen-pulsed host dendritic cells induce Ag-specific T cell regulation and prolong graft survival. Am J Transplant. (2005) 5(2):228–36. doi: 10.1046/j.1600-6143.2004.00673.x
225. Pêche H, Trinité B, Martinet B, Cuturi MC. Prolongation of heart allograft survival by immature dendritic cells generated from recipient type bone marrow progenitors. Am J Transplant. (2005) 5(2):255–67. doi: 10.1111/j.1600-6143.2004.00683.x
226. Bériou G, Pêche H, Guillonneau C, Merieau E, Cuturi MC. Donor-specific allograft tolerance by administration of recipient-derived immature dendritic cells and suboptimal immunosuppression. Transplantation. (2005) 79(8):969–72. doi: 10.1097/01.TP.0000158277.50073.35
227. Ezzelarab MB, Raich-Regue D, Lu L, Zahorchak AF, Perez-Gutierrez A, Humar A, et al. Renal allograft survival in nonhuman primates infused with donor antigen-pulsed autologous regulatory dendritic cells. Am J Transplant. (2017) 17(6):1476–89. doi: 10.1111/ajt.14182
228. Sawitzki B, Harden PN, Reinke P, Moreau A, Hutchinson JA, Game DS, et al. Regulatory cell therapy in kidney transplantation (the ONE study): a harmonised design and analysis of seven non-randomised, single-arm, phase 1/2A trials. Lancet. (2020) 395(10237):1627–39. doi: 10.1016/S0140-6736(20)30167-7
229. Fadok VA, Bratton DL, Konowal A, Freed PW, Westcott JY, Henson PM. Macrophages that have ingested apoptotic cells in vitro inhibit proinflammatory cytokine production through autocrine/paracrine mechanisms involving TGF-β, PGE2, and PAF. J Clin Invest. (1998) 101(4):890–8. doi: 10.1172/JCI1112
230. Zizzo G, Cohen PL. IL-17 Stimulates differentiation of human anti-inflammatory macrophages and phagocytosis of apoptotic neutrophils in response to IL-10 and glucocorticoids. J Immunol. (2013) 190(10):5237–46. doi: 10.4049/jimmunol.1203017
231. Mia S, Warnecke A, Zhang XM, Malmström V, Harris RA. An optimized protocol for human M2 macrophages using M-CSF and IL-4/IL-10/TGF-β yields a dominant immunosuppressive phenotype. Scand J Immunol. (2014) 79(5):305–14. doi: 10.1111/sji.12162
232. Elcombe SE, Naqvi S, van den Bosch MWM, MacKenzie KF, Cianfanelli F, Brown GD, et al. Dectin-1 regulates IL-10 production via a MSK1/2 and CREB dependent pathway and promotes the induction of regulatory macrophage markers. PLoS One. (2013) 8(3):e60086. doi: 10.1371/journal.pone.0060086
233. Foucher ED, Blanchard S, Preisser L, Garo E, Ifrah N, Guardiola P, et al. IL-34 Induces the differentiation of human monocytes into immunosuppressive macrophages. Antagonistic effects of GM-CSF and IFNγ. PLoS One. (2013) 8(2):e56045. doi: 10.1371/journal.pone.0056045
234. Meshkibaf S, Martins AJ, Henry GT, Kim SO. Protective role of G-CSF in dextran sulfate sodium-induced acute colitis through generating gut-homing macrophages. Cytokine. (2016) 78:69–78. doi: 10.1016/j.cyto.2015.11.025
235. Baliu-Piqué M, Jusek G, Holzmann B. Neuroimmunological communication via CGRP promotes the development of a regulatory phenotype in TLR4-stimulated macrophages. Eur J Immunol. (2014) 44(12):3708–16. doi: 10.1002/eji.201444553
236. Hashimoto D, Chow A, Greter M, Saenger Y, Kwan WH, Leboeuf M, et al. Pretransplant CSF-1 therapy expands recipient macrophages and ameliorates GVHD after allogeneic hematopoietic cell transplantation. J Exp Med. (2011) 208(5):1069–82. doi: 10.1084/jem.20101709
237. Llaudo I, Fribourg M, Medof ME, Conde P, Ochando J, Heeger PS. C5ar1 regulates migration of suppressive myeloid cells required for costimulatory blockade-induced murine allograft survival. Am J Transplant. (2019) 19(3):633–45. doi: 10.1111/ajt.15072
238. Braza MS, Conde P, Garcia M, Cortegano I, Brahmachary M, Pothula V, et al. Neutrophil derived CSF1 induces macrophage polarization and promotes transplantation tolerance. Am J Transplant. (2018) 18(5):1247–55. doi: 10.1111/ajt.14645
239. Conde P, Rodriguez M, van der Touw W, Jimenez A, Burns M, Miller J, et al. DC-SIGN+ macrophages control the induction of transplantation tolerance. Immunity. (2015) 42(6):1143–58. doi: 10.1016/j.immuni.2015.05.009
240. Radu CA, Horn D, Kiefer J, Rebel M, Gebhard MM, Ryssel H, et al. Donor-derived transplant acceptance-inducing cells in composite tissue allotransplantation. J Plast Reconstr Aesthet Surg. (2012) 65(12):1684–91. doi: 10.1016/j.bjps.2012.07.003
241. Warnecke G, Hutchinson JA, Riquelme P, Kruse B, Thissen S, Avsar M, et al. Postoperative intravenous infusion of donor-derived transplant acceptance-inducing cells as an adjunct immunosuppressive therapy in a porcine pulmonary allograft model. Transpl Int. (2009) 22(3):332–41. doi: 10.1111/j.1432-2277.2008.00778.x
242. Guo F, Hu M, Huang D, Zhao Y, Heng B, Guillemin G, et al. Human regulatory macrophages are potent in suppression of the xenoimmune response via indoleamine-2,3-dioxygenase-involved mechanism(s). Xenotransplantation. (2017) 24(5):e12326. doi: 10.1111/xen.12326
243. Carretero-Iglesia L, Hill M, Cuturi MC. Generation and characterization of mouse regulatory macrophages. Methods Mol Biol. (2016) 1371:89–100. doi: 10.1007/978-1-4939-3139-2_6
244. Hutchinson JA, Riquelme P, Brem-Exner BG, Schulze M, Matthäi M, Renders L, et al. Transplant acceptance-inducing cells as an immune-conditioning therapy in renal transplantation. Transpl Int. (2008) 21(8):728–41. doi: 10.1111/j.1432-2277.2008.00680.x
245. Hutchinson JA, Gövert F, Riquelme P, Bräsen JH, Brem-Exner BG, Matthäi M, et al. Administration of donor-derived transplant acceptance-inducing cells to the recipients of renal transplants from deceased donors is technically feasible. Clin Transplant. (2009) 23(1):140–5. doi: 10.1111/j.1399-0012.2008.00953.x
246. Hutchinson JA, Brem-Exner BG, Riquelme P, Roelen D, Schulze M, Ivens K, et al. A cell-based approach to the minimization of immunosuppression in renal transplantation. Transpl Int. (2008) 21(8):742–54. doi: 10.1111/j.1432-2277.2008.00692.x
247. Hutchinson JA, Roelen D, Riquelme P, Brem-Exner BG, Witzke O, Philipp T, et al. Preoperative treatment of a presensitized kidney transplant recipient with donor-derived transplant acceptance-inducing cells. Transpl Int. (2008) 21(8):808–13. doi: 10.1111/j.1432-2277.2008.00712.x
248. Ochando JC, Homma C, Yang Y, Hidalgo A, Garin A, Tacke F, et al. Alloantigen-presenting plasmacytoid dendritic cells mediate tolerance to vascularized grafts. Nat Immunol. (2006) 7(6):652–62. doi: 10.1038/ni1333
249. Olson JA, Leveson-Gower DB, Gill S, Baker J, Beilhack A, Negrin RS. NK Cells mediate reduction of GVHD by inhibiting activated, alloreactive T cells while retaining GVT effects. Blood. (2010) 115(21):4293–301. doi: 10.1182/blood-2009-05-222190
250. Rodriguez-Barbosa JI, Ferreras MC, Buhler L, Jones ND, Schneider P, Perez-Simon JA, et al. Therapeutic implications of NK cell regulation of allogeneic CD8 T cell-mediated immune responses stimulated through the direct pathway of antigen presentation in transplantation. MAbs. (2018) 10(7):1030–44. doi: 10.1080/19420862.2018.1502127
251. Hu W, Wang G, Huang D, Sui M, Xu Y. Cancer immunotherapy based on natural killer cells: current progress and new opportunities. Front Immunol. (2019) 10:1205. doi: 10.3389/fimmu.2019.01205
252. Melaiu O, Lucarini V, Cifaldi L, Fruci D. Influence of the tumor microenvironment on NK cell function in solid tumors. Front Immunol. (2020) 10:3038. doi: 10.3389/fimmu.2019.03038
253. Liu E, Marin D, Banerjee P, Macapinlac HA, Thompson P, Basar R, et al. Use of CAR-transduced natural killer cells in CD19-positive lymphoid tumors. N Engl J Med. (2020) 382(6):545–53. doi: 10.1056/NEJMoa1910607
254. Daher M, Rezvani K. Next generation natural killer cells for cancer immunotherapy: the promise of genetic engineering. Curr Opin Immunol. (2018) 51:146–53. doi: 10.1016/j.coi.2018.03.013
255. Sivori S, Pende D, Quatrini L., Pietra G, della Chiesa M, Vacca P., et al. NK Cells and ILCs in tumor immunotherapy. Mol Asp Med. (2021) 80:100870. doi: 10.1016/j.mam.2020.100870
256. Bruce DW, Stefanski HE, Vincent BG, Dant TA, Reisdorf S, Bommiasamy H, et al. Type 2 innate lymphoid cells treat and prevent acute gastrointestinal graft-versus-host disease. J Clin Invest. (2017) 127(5):1813–25. doi: 10.1172/JCI91816
257. Huang Q, Ma X, Wang Y, Niu Z, Wang R, Yang F, et al. IL -10 producing type 2 innate lymphoid cells prolong islet allograft survival. EMBO Mol Med. (2020) 12(11):e12305. doi: 10.15252/emmm.202012305
258. Schiering C, Krausgruber T, Chomka A, Fröhlich A, Adelmann K, Wohlfert EA, et al. The alarmin IL-33 promotes regulatory T-cell function in the intestine. Nature. (2014) 513(7519):564–8. doi: 10.1038/nature13577
259. Yi S, Ji M, Wu J, Ma X, Phillips P, Hawthorne WJ, et al. Adoptive transfer with in vitro expanded human regulatory T cells protects against porcine islet xenograft rejection via interleukin-10 in humanized mice. Diabetes. (2012) 61(5):1180–91. doi: 10.2337/db11-1306
260. Issa F, Hester J, Goto R, Nadig SN, Goodacre TE, Wood K. Ex vivo-expanded human regulatory T cells prevent the rejection of skin allografts in a humanized mouse model. Transplantation. (2010) 90(12):1321–7. doi: 10.1097/TP.0b013e3181ff8772
261. Guo Y, Mei Z, Li D, Banerjee A, Khalil MA, Burke A, et al. Ischemia reperfusion injury facilitates lung allograft acceptance through IL-33-mediated activation of donor-derived IL-5 producing group 2 innate lymphoid cells. Am J Transplant. (2022) 22(8):1963–75. doi: 10.1111/ajt.17084
262. Usuelli V, Ben Nasr M, D’Addio F, Liu K, Vergani A, El Essawy B, et al. miR-21 antagonism reprograms macrophage metabolism and abrogates chronic allograft vasculopathy. Am J Transplant. (2021) 21(10):3280–95. doi: 10.1111/ajt.16581
263. Li L, Zhang J, Diao W, Wang D, Wei Y, Zhang C, et al. MicroRNA-155 and microRNA-21 promote the expansion of functional myeloid-derived suppressor cells. J Immunol. (2014) 192(3):1034–43. doi: 10.4049/jimmunol.1301309
264. Caescu C, Guo X, Tesfa L, Bhagat T, Verma A, Zheng D, et al. Colony stimulating factor-1 receptor signaling networks inhibit mouse macrophage inflammatory responses by induction of microRNA-21. Blood. (2015) 125(8):e1–e13. doi: 10.1182/blood-2014-10-608000
265. Usuelli V, Loretelli C, Seelman AJ, Pastore I, D’Addio F, Ben Nasr M, et al. Novel soluble mediators of innate immune system activation in solid allograft rejection. Transplantation. (2022) 106(3):500–9. doi: 10.1097/TP.0000000000003834
266. Gonzalez-Polo V, Pucci-Molineris M, Cervera V, Gambaro S, Yantorno SE, Descalzi V, et al. Group 2 innate lymphoid cells exhibit progressively higher levels of activation during worsening of liver fibrosis. Ann Hepatol. (2019) 18(2):366–72. doi: 10.1016/j.aohep.2018.12.001
Keywords: cellular therapeutics, transplantation, MDSCs (myeloid-derived suppressor cells), regulatory dendritic cells, regulatory macrophages, innate lymphoid cells (ILCs), human monocyte-derived suppressor cells
Citation: Ott LC and Cuenca AG (2023) Innate immune cellular therapeutics in transplantation. Front. Transplant. 2:1067512. doi: 10.3389/frtra.2023.1067512
Received: 2 February 2023; Accepted: 16 March 2023;
Published: 31 March 2023.
Edited by:
Satish N. Nadig, Northwestern University, United StatesReviewed by:
Francesca D’Addio, University of Milan, ItalyBaptiste Lamarthée, INSERM U1098 Interactions Hôte-Greffon-Tumeur & Ingénierie Cellulaire et Génique, France
© 2023 Ott and Cuenca. This is an open-access article distributed under the terms of the Creative Commons Attribution License (CC BY). The use, distribution or reproduction in other forums is permitted, provided the original author(s) and the copyright owner(s) are credited and that the original publication in this journal is cited, in accordance with accepted academic practice. No use, distribution or reproduction is permitted which does not comply with these terms.
*Correspondence: Alex G. Cuenca YWxleC5jdWVuY2FAY2hpbGRyZW5zLmhhcnZhcmQuZWR1
Specialty Section: This article was submitted to Transplantation Immunology, a section of the journal Frontiers in Transplantation