- 1Early Investigative Toxicology, Merck Healthcare KGaA, Darmstadt, Germany
- 2Corporate Animal Affairs, Merck KGaA, Darmstadt, Germany
- 3Cell Design Lab, Molecular Biology, Merck KGaA, Darmstadt, Germany
Fetal Bovine Serum (FBS) is an important ingredient in cell culture media and the current standard for most cells in vitro. However, the use of FBS is controversial for several reasons, including ethical concerns, political, and societal pressure, as well as scientific problems due to the undefined and variable nature of FBS. Nevertheless, scientists hesitate to change the paradigm without solid data de-risking the switch of their assays to alternatives. In this study, HepG2 cells, a human hepatoblastoma cell line commonly used to study drug hepatotoxicity, were adapted to serum-free conditions by using different commercially available media and FBS replacements. After transition to these new culture conditions, the success of adaptation was determined based on cell morphology and growth characteristics. Long-term culturing capacity for each medium was defined as the number of passages HepG2 cells could be cultured without any alterations in morphology or growth behavior. Two media (Advanced DMEM/F12 from ThermoFisher and TCM® Serum Replacement from MP Biomedicals) showed a long-term cultivation capacity comparable to media containing FBS and were selected for further analysis. Both media can be characterized as serum-free, however still contain animal-derived components: bovine serum albumin (both media) and bovine transferrin (only TCM® serum replacement). To assess the functionality of the cells cultivated in either of the two media, HepG2 cells were treated with reference compounds, specifically selected for their known hepatotoxicity characteristics in man. Different toxicological assays focusing on viability, mitochondrial toxicity, oxidative stress, and intracellular drug response were performed. Throughout the different assays, response to reference compounds was comparable, with a slightly higher sensitivity of serum-free cultivated HepG2 cells when assessing viability/cell death and a lower sensitivity towards oxidative stress. Taken together, the two selected media were shown to support growth, morphology, and function of serum-free cultivated HepG2 cells in the early preclinical safety space. Therefore, these results can serve as a starting point to further optimize culture conditions with the goal to remove any remaining animal-derived components.
1 Introduction
In pharmaceutical drug development, the use of in vitro cell, tissue and organ culture is an important tool to test drug candidates for efficacy, DMPL and toxicity. With increasing complexity of available cell culture models, great progress has been made to better approximate the human in vivo situation. These models have the potential of reducing the need for animal experiments in research and development (Ma et al., 2021; Wang et al., 2021). However, despite being considered a major alternative for animal experimentation, human in vitro cell culture systems often require animal components for supplementation (Jochems et al., 2002; van Zutphen et al., 2001; Weihe, 1985). Fetal bovine serum (FBS), Matrigel, and other animal derived products, such as growth factors, hormones, adhesion factors are commonly used to maintain and proliferate cells and tissues (Gospodarowicz and Moran, 1976; Hughes et al., 2010; Yao and Asayama, 2017).
FBS is an important ingredient in cell culture media and the current standard for media supplementation. It contains a highly complex mixture of components including proteins and peptides (adhesion molecules, carrier proteins, growth factors, hormones, etc.), vitamins, inorganic salts, amino acids, trace elements, carbohydrates, lipids and is able to support growth and survival of a variety of different cells and tissues (Gstraunthaler and Lindl, 2013; Maurer, 1986). In contrast to other sera, fetal calf serum is richer in growth factors while containing lower levels of γ-globulin, which is considered a cell growth inhibitor. For these reasons, FBS is the preferred cell culture supplement for in vitro experimentation (Gstraunthaler and Lindl, 2013).
However, using FBS in cell culture is highly questionable for several reasons. Ethical concerns arise due to the collection process for fetal calf blood. FBS, as a by-product of the beef industry, is collected when a cow sent for slaughter is discovered to be pregnant. Collection of fetal calf blood is done by cardiac puncture of at least 3-month-old calf fetuses, usually without any form of anesthesia (Jochems et al., 2002; van der Valk et al., 2004). A study conducted by Jochems et al. on the fetal bovine blood collection process drew the conclusion that fetuses are most likely exposed to pain and/or suffering, increasing the necessity to replace FBS in cell culture with an animal free alternative (Jochems et al., 2002).
Additionally, scientific problems arise due to the undefined nature and highly variable composition of FBS. Although FBS was introduced into cell culture already in the 1950s, it has never been fully characterized (Puck et al., 1958). Proteomic studies detected more than 3,000 proteins, with only a fraction of these being identified (Nakamura et al., 2021; Zheng et al., 2006). More studies have been conducted on human serum. Approximately 3,700 distinct proteins were found, of which 1800 proteins were identified (Anderson and Anderson, 2002; Anderson et al., 2004; Pieper et al., 2003). Furthermore, more than 4,000 metabolites are present in human serum (Psychogios et al., 2011).
Apart from the undefined nature, FBS is subject to seasonal and geographical lot-to-lot differences, which increases experimental variability (Baker, 2016; Zheng et al., 2006). Therefore, batch-to-batch differences are thought to play an important part in the well-known reproducibility problems when using serum and therefore force scientist to perform excessive batch-to-batch testing (Baker, 2016; Zheng et al., 2006). Additionally, FBS is also critical from a biosafety aspect, since FBS can be a source for contaminations such as mycoplasma, viruses, prion proteins or endotoxins (Merten, 2002; Nikfarjam and Farzaneh, 2012; Wessman and Levings, 1999).
Despite being able to support growth and maintain viability of different cells, factors present in FBS might interfere with the phenotypic stability of cells or interact with test substances, thus influencing experimental outcome (Khasawneh et al., 2019; Lau and Chang, 2014; Tang et al., 2017). As examples, Shahdadfar et al. demonstrated that FBS induced a more differentiated and less stable transcriptional profile in human bone marrow mesenchymal stem cells (hMSCs) compared to human serum while Bilgen et al. showed that FBS inhibited glycosaminoglycan and type II collagen production in fibroblast-like type-B synoviocytes (Bilgen et al., 2007; Shahdadfar et al., 2005). Despite all these described reasons, scientists hesitate to change the paradigm without solid data de-risking the switch of their assays to FBS-free culture media.
It has become clear that almost every cell type has its own requirements concerning medium supplements, since different cell types have different receptors involved in cell survival, growth and differentiation (van der Valk et al., 2010). Different alternatives to FBS have been suggested, including human platelet lysates (hPL), bovine ocular fluid, pituitary extracts, Earthworm heat inactivated coelomic fluid (HI-CF) or sericin (Bratko et al., 2002; Hammond et al., 1984; Johansson et al., 2003; Patil and Biradar, 2017; Rauch et al., 2011; Subbiahanadar Chelladurai et al., 2021; Terada et al., 2002). However, these alternatives are, like FBS, undefined and therefore scientific problems related to batch-to-batch variabilities are not eliminated. In order to tackle both, scientific and ethical problems in in vitro cell culture, approaches using serum-free media, protein-free media, animal-derived component free media (xeno-free media) and chemically defined media (See Table 1 for definition) are preferable. Implementation of such media is therefore recommended by the ECVAM Scientific Advisory Committee (ESAC) for current and new in vitro methods (Gstraunthaler et al., 2013; van der Valk et al., 2010). Notably, animal-derived component free or xeno-free media should be preferred, while serum-free media still containing animal-derived components should only be used if no suitable animal-derived component free or xeno-free alternative is available.
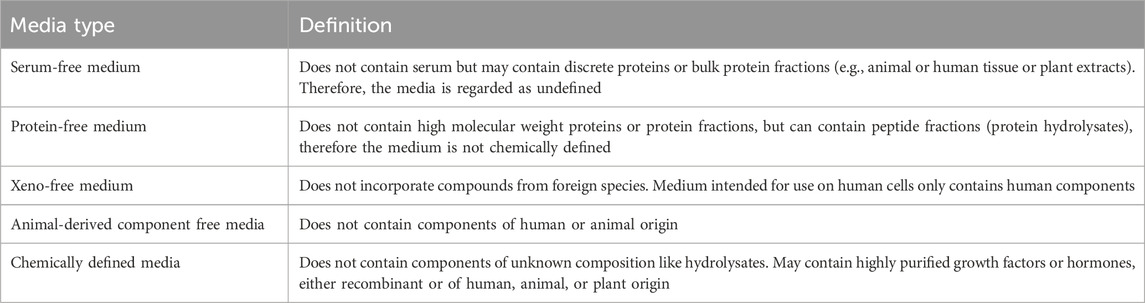
Table 1. Definition of cell culture media adapted from van der Valk et al. (2010).
Since different cells and cell lines rely on different factors for survival or proliferation, the development of a chemically defined medium is not always straight forward and needs to be properly assessed. To simplify the development process, van der Valk et al. proposed a modular approach starting off with a 1:1 mixture of DMEM and Ham-F12 supplemented with insulin, transferrin and sodium selenite (van der Valk et al., 2010). For adherent cells and cell lines, adhesion factors like fibronectin, vitronectin, collagen or laminin are typically used for pre-coating of the culture vessels. Preferably, these adhesion factors should be either human or recombinant in order to avoid animal components in cell culture. Subsequently, specific hormones and growth factors may be supplemented. In the last step, lipids, antioxidants and/or vitamins are added to the medium. With increasing complexity of the serum-free media composition, specificity is increased as well (van der Valk et al., 2010). Therefore, development of cell or cell type specific media have been often preferred over a universal chemical defined medium, especially when considering production costs for growth factors and other proteins (Karnieli et al., 2017; van der Valk et al., 2010; Yao and Asayama, 2017).
Several different FBS free media have been developed, both cell type specific and universal, with a steady growth with progressing scientific progress (Karnieli et al., 2017). Media formulations from literature are collected within the Fetal Calf Serum free Database (RRID:SCR_018769; https://fcs-free.org), while formulations of commercial media are usually proprietary. Finding a suitable media to maintain the desired cells and tissues and support the experimental goals can be challenging, especially when an immediate transfer of cells to serum free conditions is not possible. In this case, cells need to be adapted carefully in a time-consuming process. Different protocols to do this have been proposed to adapt cells to serum-free conditions (see Figure 1).
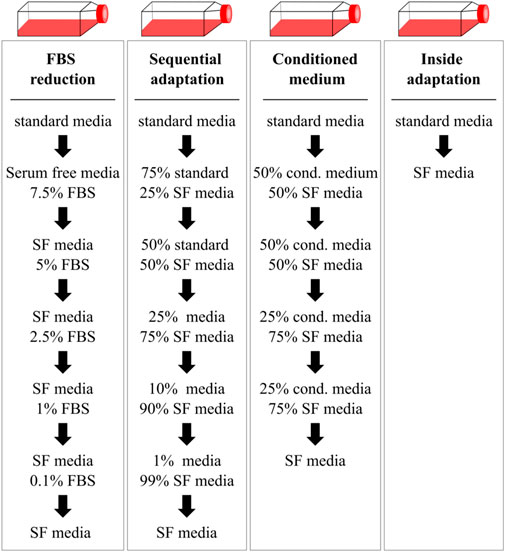
Figure 1. Gradual and immediate adaptation mechanisms as described by Van-der-Valk et al. used to transfer HepG2 cells in serum-free conditions. Cells were transferred to serum-free media (SF media) either by gradually reducing the FBS content (FBS reduction), mixing of the serum-free medium with the standard medium containing FBS (sequential adaptation) or by supplementing the serum-free medium with conditioned medium of the previous passage. Alternatively, cells were immediately transferred to serum-free conditions during the splitting process (inside adaptation). Cells were transferred to the next condition if viability was above 90%.
Dependent on the cell type or cell line, serum-free culture may either be already commonly performed and described frequently in the literature, while for other cell types, fewer resources may be available. Under the scope of liver models for toxicity testing, serum-free culture is routinely used to cultivate primary human hepatocytes, in both 2D or 3D applications (Bell et al., 2016; Klaas et al., 2021; Lübberstedt et al., 2015; Runge et al., 2000). Additionally, serum-free culture was demonstrated for HepaRG cells, an immortalized hepatic cell line, as well as different liver cancer derived cell lines, e.g., Huh7, SK-Hepp-1 and HKB-11 cells (Abe et al., 2007; Akazawa et al., 2011; Biaggio et al., 2015; Klein et al., 2014). However, these serum-free cultivated liver cancer cells have not yet been tested for specific toxicity applications.
The aim of this study was to adapt HepG2 cells to serum-free conditions using different commercially available media and subsequently test serum-free cultivated cells in comparison to cells cultivated in serum containing medium in standard assays which are sued for assessing cytotoxicity and hepatotoxicity.
HepG2 cells are a popular highly proliferative hepatic cell line with epithelial-like morphology that grows as monolayers and form characteristic cell clusters or islands (Vinken and Rogiers, 2015). According to ATCC, this cell line was isolated from a hepatocellular carcinoma (HCC) of a 15-year-old, White, male youth with liver cancer, however more recent findings suggest that the HepG2 cell line is instead a hepatoblastoma (HB) cell line (Arzumanian et al., 2021; Hep G2 [HB-8065] | ATCC). This cell line can be cultured in different media (Dulbecco’s Modified Eagle Medium, Dulbecco’s Modified Eagle Medium/Nutrient Mixture F-12, Eagle’s Minimum Essential medium, or Roswell Park Memorial Institute 1,640 medium) supplemented with 10% FBS, and, dependent on the used media, L-glutamine and/or sodium pyruvate (Hep G2 [HB-8065] | ATCC; Luckert et al., 2016; Naito et al., 2018; Vinken and Rogiers, 2015). So far, serum-free culture of HepG2 cells has been shown mainly for short term applications, especially for 3D spheroids or multi-organ models or very short-term applications under serum-starvation (Gunn et al., 2017; Oleaga et al., 2016; Sefried et al., 2018; Sun et al., 2024; Wrzesinski et al., 2013). Since only a limited number of animal-derived component free or xeno-free media was deemed suitable, serum-free media were included as well, if the percentage of animal-derived components within the media was low. Results on serum-free medium should then be used to further optimize culture conditions towards animal-derived component free culture in future experiments.
Cells adapted to serum-free conditions should maintain morphological characteristics and display similar high proliferation rates–both parameters were being used in this work. With a wide range of applications, the HepG2 cell line is most often used in early drug development, to test for cytotoxicity and hepatotoxicity (Vinken and Rogiers, 2015). Therefore, the key aspect of this study was on the comparability of serum-free cultured HepG2 in common toxicological assays. To compare serum-free HepG2 cells with cells cultured in FBS containing media, cells were treated with a broad range of reference compounds, selected from the IMI project “mechanism-based integrated prediction of drug-induced liver injury in man” (MIP-DILI), internal reference compounds or negative control compounds (Dragovic et al., 2016). These compounds exhibit different mechanisms of DILI including: mitochondrial impairment, reactive metabolites, lysosomal impairment, BSEP inhibition and/or innate/adaptive immune activation.
2 Experimental procedures
2.1 Materials
All reference compounds were purchased from Sigma-Aldrich (St.Louis, MO) unless otherwise noted. Reference compounds are depicted in Table 2.
2.2 Cell culturing (standard procedure with FBS)
HepG2 (HB-8065, ATCC) were cultured in Dulbecco’s Modified Eagle Medium/Nutrient Mixture F-12 (DMEM/F12 with HEPES, 31330) supplemented with 10% fetal bovine serum and 1 mM sodium pyruvate (all Gibco™) at 37°C and 5% CO2. Cells were split twice per week using TrypLE™ (Gibco™, 12604013) for detachment. Subcultivation ratios ranged between 1:3 and 1:5, corresponding to ∼8–10 × 104 cells/cm2. Cells were used for up to 30 passages before disposal.
2.3 Comparison of adaptation procedures
For a comparison of adaptation processes, HepG2 cells were adapted to two different serum-free media, serum-free medium 1 (SF-M1; Advanced DMEM/F12) or serum-free medium 3 (SF-M3), by FBS reduction, sequential adaptation, by using conditioned medium or inside adaptation as described in Figure 1 (van der Valk et al., 2010). Media characterization can be found in Supplementary Table S1. Subcultivation was performed when a confluency of ∼70–80% was reached. Cell media was changed in case of slower growth every 2–3 days. Cells were maintained in one condition for at least two passages before lowering the FBS concentration again. Viability and cell density were taken as parameters to decide whether to maintain cells in one particular condition or continue the adaptation process. Vitronectin coating (using VTN-N, A14700, ThermoFisher, 0.5 μg/cm2) was applied if a reduction in cellular adherence was observed.
2.4 Adaption of HepG2 cells to serum-free conditions
Cells were adapted to serum-free conditions using the inside adaptation strategy proposed by van der Valk et al. (2010) (Figure 1). Different products were tested for cultivation of HepG2 (Supplementary Table S1). Serum-free media (SF media) were supplemented with HEPES, sodium pyruvate (1 mM) or GlutaMax (2 mM) if not present in the medium already.
2.5 Growth curve and doubling time determination
Growth of cells cultivated in standard medium containing FBS, Advanced DMEM/F12 or TCM® Serum Replacement was assessed using the Incucyte® S3 Live-Cell Analysis Instrument from Sartorius. HepG2 were seeded in 6-well plates at 8 × 104 cells/cm2. For serum-free conditions, plates were coated with vitronectin (0.5 μg/cm2). Medium was changed after 3 days for all conditions. Confluency was determined every 8 h using 4 pictures per well. Growth curves were modeled in GraphPad Prism from confluency values using a logistic growth model. Doubling times were calculated on the exponential (log) phase using an exponential (Malthusian) growth model.
2.6 Immunofluorescent staining
HepG2 cells cultivated in standard medium containing FBS, Advanced DMEM/F12 or TCM® Serum Replacement were stained for nuclei (DAPI, D9542-10 MG, Millipore Sigma), actin (A12379, Invitrogen), albumin (sc50535, Santa Cruz Biotechnology) and alpha-1-antitrypsin (PA5-16661, Invitrogen). Cells were cultured in 96-well plates for 3 days. For staining, media was aspirated, and cells were fixed with 4% formaldehyde for 30 min. Afterwards, cells were washed two times with Wash buffer (PBS +/+, 0.2% Triton X-100, 0.04% Tween-20) and incubated for 5 min with permeabilization buffer (PBS +/+, 0.1% Triton X-100). Before staining, blocking buffer (PBS +/+, 5% BSA, 0.2% Triton X-100, 0.04% Tween-20) was added to the cells for 30 min. Buffer was aspirated and cells were incubated with primary antibodies over night at 4°C.
The next day, the primary antibodies were aspirated, the cells were washed twice with wash buffer before the secondary staining antibody (Alexa Fluor 680 goat-anti-rabbit, A21076, Life technologies) was added. Cells were incubated for 1 h at RT, washed twice and subsequently imaged using the high content imager CellInsight CX7 from Thermo Fisher.
Notably, antibodies and BSA are animal-derived components and should therefore be avoided.
2.7 Assessment of urea and albumin secretion
Urea and human albumin secretion was measured in cell culture supernatants of cells cultivated in standard medium containing FBS, Advanced DMEM/F12 or TCM® Serum Replacement. Cells were grown over 96 h and samples were taken after 6 h, 48 h and 96 h. Cells were counted after sample collection. Human albumin concentration was measured using the human albumin ELISA kit (ab179887) from Abcam according to manufacturer’s instructions. Urea concentrations were determined using the urea assay kit (MAK006) from Sigma-Aldrich. Standard curves for both assays were generated using a sigmoidal, four parameter curve fit (4 PL).
2.8 CellTiter-Glo® assay
Cell viability in response to compound treatment was accessed by measuring ATP using the CellTiter-Glo® Luminescent Cell Viability Assay from Promega (G7571) according to manufacturer´s instructions. HepG2 cells cultivated in standard medium containing FBS, Advanced DMEM/F12 or TCM® Serum Replacement were seeded in 384-well plates, incubated for 24 h, and subsequently treated with the MIP-DILI reference compounds, negative controls, or internal controls in a three-fold dilution series performed in triplicates using the Tecan 300e Dispenser (Dragovic et al., 2016). Treatment was performed for 24 h.
CellTiter-Glo® substrate was dissolved in CellTiter-Glo® buffer and equilibrated at room temperature prior to use. Plates were incubated at room temperature for 30 min before adding the CellTiter-Glo® reagent. Subsequently, plates were shaken for 2 min protected from light and incubated for 10 min in the dark. Luminescence was measured using the Varioskan Plate Reader from Thermo Fisher. IC50 values were obtained from dose response curves in GraphPad Prism using a log(inhibitor) vs. response model (variable slope, four parameters, bottom constraint = 0).
2.9 Alamar blue assay
Metabolic activity of cells in response to compound treatment was accessed using the Alamar Blue Assay. HepG2 cells cultivated in standard medium containing FBS, Advanced DMEM/F12 or TCM® Serum Replacement were seeded in 384-well plates, incubated for 24 h, and subsequently treated with the MIP-DILI reference compounds or negative controls in a three-fold dilution series performed in triplicates using the Tecan 300e Dispenser (Dragovic et al., 2016). Treatment was performed for 24 h.
Resazurin (Sigma-Aldrich, R7017-5G) was dissolved in PBS solution at 4.5 mM and added to as 10% of total volume per well. Plates were incubated for 2 h. After incubation, the supernatant was transferred into black, clear bottom 384-well plates and protected from light until measurement. Fluorescence was measured at λ excitation: 571 nm; λ emission: 585 nm using the Varioskan Plate Reader from Thermo Fisher. IC50 values were obtained from dose response curves in GraphPad Prism using a log(inhibitor) vs. response model (variable slope, four parameters, bottom constraint = 0).
2.10 Glutathione (GSH) assay
To assess oxidative stress in cells in response to compound treatment, the GSH-Glo™ Glutathione Assay from Promega was used according to manufacturer’s instructions (V6912). HepG2 cells cultivated in standard medium containing FBS, Advanced DMEM/F12 or TCM® Serum Replacement were seeded in 384-well plates, incubated for 24 h, and subsequently treated with the MIP-DILI reference compounds, negative controls, or internal controls in a three-fold dilution series performed in triplicates using the Tecan 300e Dispenser (Dragovic et al., 2016). Treatment was performed for 24 h.
Assay reagents and plates were first equilibrated to room temperature. GSH-Glo™ Reagent 1x was obtained by diluting Luciferin-NT substrate and Glutathione S-Transferase 1:100 in GSH-Glo™ Reaction Buffer. Luciferin Detection reagent was dissolved in Reconstitution Buffer with Esterase.
The medium was aspirated, GSH-Glo™ Reagent 1x was added to the cells and plates were shaken for 2 min and subsequently incubated for 30 min at room temperature protected from light. Afterwards, reconstituted Luciferin Detection Reagent was added, and cells were again shaken for 2 min before incubation for 15 min at room temperature in the dark. Luminescence was measured using the Varioskan Plate Reader from Thermo Fisher.
2.11 Mitochondrial toxicity assay
To assess mitochondrial toxicity, the Glucose/Galactose Assay was used. HepG2 cells cultivated in standard medium containing FBS, Advanced DMEM/F12 or TCM® Serum Replacement were seeded in 384-well plates and incubated for 48 h. Cells were washed once, and subsequently switched to DMEM (ThermoFisher, 11966025) supplemented with sodium pyruvate, HEPES and either 25 mM D-glucose or 10 mM D-galactose without FBS to avoid residual glucose. Cells were pre-incubated for 4 h before treatment with the MIP-DILI reference compounds, negative controls or internal controls (Dragovic et al., 2016). Treatment was performed in a three-fold dilution series in triplicates using the Tecan 300e Dispenser. Cells were treated for 2 h, and the read-out was performed by measuring cell viability using the CellTiter-Glo® reagent as described previously. IC50 values were obtained from dose response curves in GraphPad Prism using a log(inhibitor) vs. response model (variable slope, four parameters, bottom constraint = 0). Mitochondrial toxicity was assessed by calculating the IC50Glc/IC50Gal ratio and the threshold for risk for mitochondrial toxicity was set at >2.
2.12 Measurement of intracellular drug response markers (cellular fingerprint assay)
To measure intracellular drug response markers, HepG2 cells cultivated in standard medium containing FBS, Advanced DMEM/F12 or TCM® Serum Replacement were seeded in 384-well plates and incubated for 24 h. After incubation, cells were treated with selected MIP-DILI compounds or negative controls in a three-fold dilution series performed in triplicates using the Tecan 300e Dispenser (Dragovic et al., 2016). Additionally, cells were treated with assay specific control substances (see Supplementary Table S2). Treatment was performed for 24 h. After treatment, media was carefully aspirated und cells were lysed by adding 40 µL lysis buffer (Supplementary Table S3) to each well. The plates were incubated for 30 min at 4°C and either stored at −80°C or used immediately.
Intracellular drug response markers were assessed using the Luminex technology. Color-coded beads from NMI specific for one analyte each were used to qualify and quantify p-serin 10 of Histon H3 (cell division marker), LC3B (autophagy marker), PARP (cell death/apoptosis marker), HSP70 (stress response marker), p-serin 2/5 of RNA-Polymerase II (protein transcription marker), p-serin 422 of EIF4B (protein synthesis marker) and HIF1-alpha (oxidative stress marker).
For hybridization, 25 μL cell lysate of each sample was transferred to a fresh 384-well plate. 25 μL prepared bead mix and 25 µL assay buffer were added and the plate was sealed. Plates were incubated for 16–20 h at 4°C and 600 rpm. The TS Pro thermal plate shaker from CellMedia was used for all incubation steps.
After incubation the plates were washed and successively incubated with 15 µL detection antibody (1 h, RT, 650 rpm) and 15 µL streptavidin, R-phycoerythrin conjugate (SAPE; 45 min, RT, 650 rpm). After each step, the plates were washed three times with wash buffer. Before measurement, 90 µL assay buffer was added to each well and the plates were incubated for 10 min at RT and 700 rpm. Measurement was performed using the Luminex™ FlexMAP 3D from ThermoFisher.
2.13 Comparison of freezing media
Different freezing media were tested for their potential to store serum-free cultivated HepG2 as depicted in Table 3. HepG2 cells cultivated in standard medium containing FBS, Advanced DMEM/F12 or TCM® Serum Replacement were detached using TrypLE™ and resuspended in media. After centrifugation, medium was aspirated, and cells were resuspended in freezing media. Commercial media were used according to manufacturer’s instructions. For conditioned medium, 45% fresh medium, 45% conditioned medium and 10% DMSO was used. Cells were transferred to cryogenic storage vials and frozen using NALGENE™ Cryo 1°C Freezing containers (ThermoFisher, 5100–0001).
2.14 Statistical analysis
All results are presented as mean ± standard deviation if not stated otherwise. Analysis was performed using the GraphPad prism software. All statistical test performed, as well as the number of replicates are summarized in Supplementary Table S4. Results were considered significant if adjusted p < 0.05.
2.15 Ethical statement
The materials used for this research do not meet the standard of being completely free from animal derived components.
We are committed to continuous improvement in our sourcing and manufacturing processes to comply with our high ethical standards and growing societal expectations.
3 Results
3.1 Comparison of adaptation protocols for transition of HepG2 to serum-free conditions
The four different adaptation protocols described by Van-der-Valk et al. (FBS reduction, sequential adaptation, conditioned medium and inside adaptation, see Figure 1) were compared for the transitioning of HepG2 to serum-free conditions (van der Valk et al., 2010). Ten different commercially available products (either serum-free media or FBS replacements) were purchased (Supplementary Table S1). Of these products, two media (serum-free medium 1 and serum-free medium 3) were randomly selected and cells were either gradually or immediately transitioned to serum-free conditions (Figure 2). For gradual adaptation of the cells, serum was reduced during passaging, whenever the viability of the culture was higher than 90%. In case that the cells displayed a viability lower than 90%, cells were kept in the same condition for another passage.
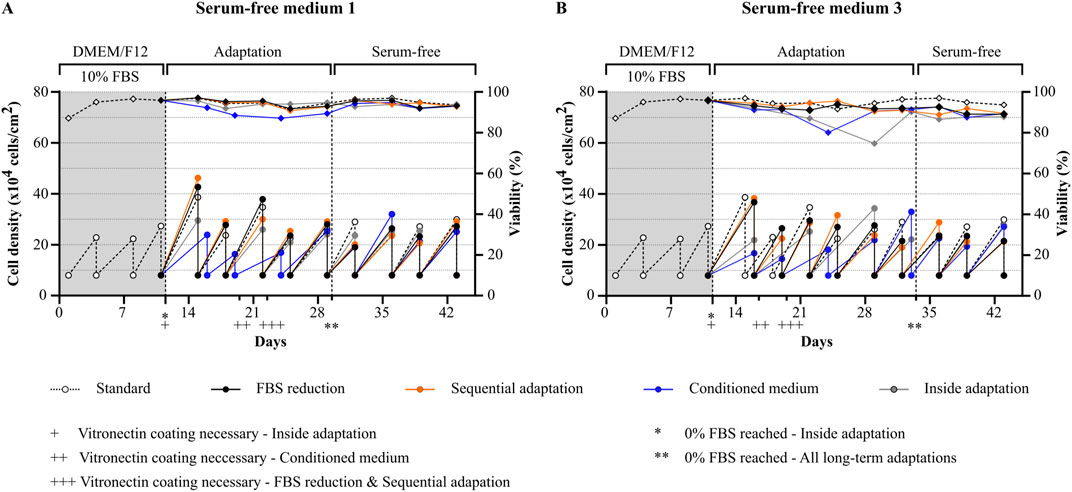
Figure 2. Comparison of adaptation protocols for transitioning of HepG2 cells to serum-free conditions. Viability (rhombuses) and viable cell density (circles) of cells during and after the adaptation process using different protocols (FBS reduction, sequential adaptation, conditioned medium or inside adaptation). Two randomly selected serum-free media were used: (A) serum-free medium 1 and (B) serum-free medium 3. Vitronectin coating was required for successful serum-free culture and was mandatory for all protocols with different starting points as indicated (+: inside adaptation, ++ conditioned medium, +++ FBS reduction and sequential adaptation). FBS-free conditions for the long-term adaptation protocols were reached at day 29 for serum-free medium 1 and Day 32 for serum-free medium 3 as indicated. Cells were splitted to the same cell density during each splitting process (8 × 104 cells/cm2).
In general, the two media showed a different performance. While it was possible to maintain the cells after adaptation in serum-free media 1 with similar growth rates and a high viability comparable to cells cultured under FBS containing conditions, cells cultured in serum-free medium 3 were less viable throughout the whole experiment.
For cells adapted to serum-free medium 1 (Figure 2A), positive results were obtained with all four protocols. No differences were observed between FBS reduction and sequential adaptation, and viability and growth rates remained comparable to the control condition during the whole experiment. The use of conditioned medium during the adaptation process led to a significant reduction in both viability and growth, which normalized again after reaching 0% FBS. Finally, cells transitioned to FBS free medium using the inside adaptation protocol maintained a high viability but showed slightly reduced growth rates in the first three serum-free passages (day 11 till day 22) which subsequently normalized again.
For cells adapted to serum-free medium 3 (Figure 2B), the best results were obtained by using either the FBS reduction or sequential adaptation approach. Nevertheless, a reduction in viability and growth was observed during the adaptation process which did not normalize after reaching 0% FBS. For the conditioned medium approach, and the inside adaptation protocol, viability and growth rates dropped significantly. In both cases, growth and viability improved after several passages, however, as observed for FBS reduction and sequential adaptation, cells were not as viable as cells cultured in the FBS-containing condition.
Taken together, we observed that gradual adaptation using either FBS reduction or sequential adaptation is a gentler approach which allowed cells to maintain high viability and comparable cell growth at the expense of a prolonged time frame. In contrast, inside adaptation allowed for fast transitioning of cells to serum-free conditions at the expense of cell growth and viability, especially if the media formulation is not specifically designed for HepG2 cells. However, as inside adaptation allows for a fast differentiation between suitable and less suitable serum-free media and cells transitioned to serum-free medium 1 showed comparable growth and viability after already 3 passages, follow-up experiments were conducted using this approach.
3.2 Serum-free medium–front-runner selection
Cells were adapted to the different serum-free media or media supplemented with FBS replacements using the inside adaptation approach (Supplementary Table S1). After transition to the new culture conditions, the success of adaptation was determined based on cell morphology and growth characteristics. In addition, the long-term culturing capacity for each medium was defined as the number of passages HepG2 could be cultured without any alterations in these two parameters. If cells displayed significant changes in growth behavior or morphology, the culture was terminated. Since the aim of this experiment was to find the most suitable serum-free growth media, the possibility of cells to recover after multiple passages was not assessed.
Based on these specific criteria, of the ten different products used in this experiment, only two were suitable for long-term serum-free culture of HepG2. These two products, Advanced DMEM/F12, ThermoFisher (serum-free medium 1) and TCM® Serum Replacement, MP Biomedicals (serum replacement 2) were able to maintain HepG2 cells with their usual growth characteristics and no significant changes in morphology for more than 25 passages (Figure 3). The average doubling time of cells cultivated in Advanced DMEM/F12 was comparable to cells cultured in FBS containing medium (47.7 ± 1.7 h vs. 48.2 ± 4.6 h, respectively), while the doubling time for cells cultured in TCM® Serum Replacement was slightly higher (55.5 ± 20.2 h) (Figure 4).
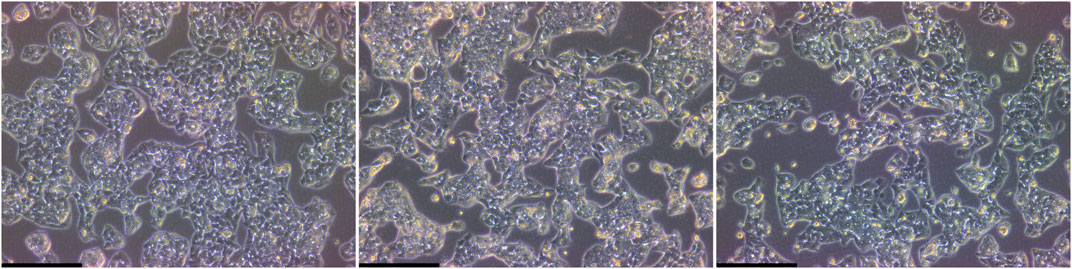
Figure 3. Analysis of morphology of serum-free cultivated HepG2. Microscopic images of cells cultivated under serum containing conditions (left), in Advanced DMEM/F12 (ThermoFisher, middle) or DMEM/F12 supplemented with TCM® defined serum replacement (MP Biomedicals, right). Vitronectin coating was used for serum-free conditions to facilitate attachment. Scalebar: 250 µm.
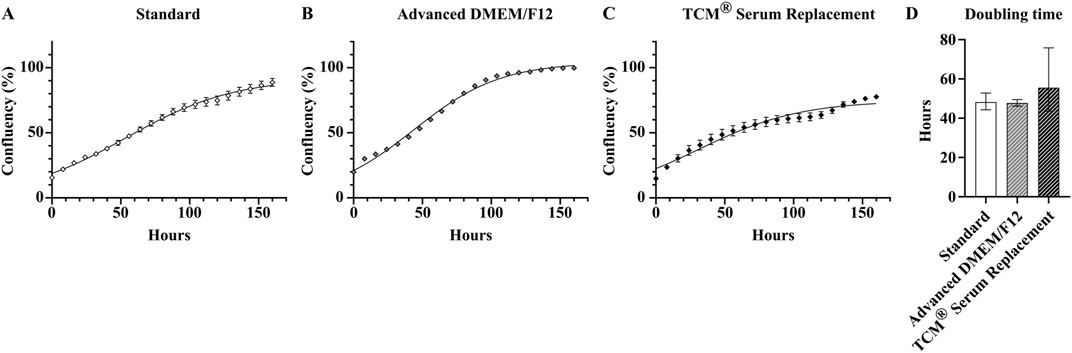
Figure 4. Analysis of growth properties of serum-free cultivated HepG2. Growth curves of cells cultivated either under (A) serum containing conditions, (B) in Advanced DMEM/F12 (ThermoFisher, middle) or (C) DMEM/F12 supplemented with TCM® defined serum replacement (MP Biomedicals, right). (D) Doubling times of serum-free cultivated cells and cells cultivated in standard medium calculated from logarithmic phase (8 h–64 h) of growth curves using an exponential (Malthusian) growth equation (R squared Standard: 0.993, R squared Advanced DMEM/F12: 0.998, R squared TCM® Serum Replacement: 0.935). Data are presented as mean ± range. Ordinary one-way ANOVA with Tukey correction for multiple comparison was used to assess statistical significance (N = 1; n = 4).
Of all other products, two additional products (serum-free medium 2 and serum replacement 4) displayed a limited long-term culturing capacity, with cells surviving without alterations in growth behavior for eleven or eight passages, respectively. All other products were only suitable for short-term culture or not suitable at all. Of these six media, immediate cell death was observed in one condition (serum-free media 4), while death after the first splitting process was observed in another (serum replacement 1). In two conditions (serum-free media 5 and serum-replacement 5) drastic changes in morphology were observed, while in the last two conditions, cell growth was significantly reduced leading to termination of the cultivation of cells in these conditions (see Supplementary Figures S2–S4).
To assess common HepG2 characteristics and functionalities, cells cultured in Advanced DMEM/F12 and TCM® Serum Replacement were stained for two typical HepG2 markers (albumin and alpha 1-antitrypsin) and compared to cells cultivated in FBS containing medium (Hep G2 [HB-8065] | ATCC) (Figure 5A). Both markers were expressed under all conditions, mainly in the cytoplasm as expected.
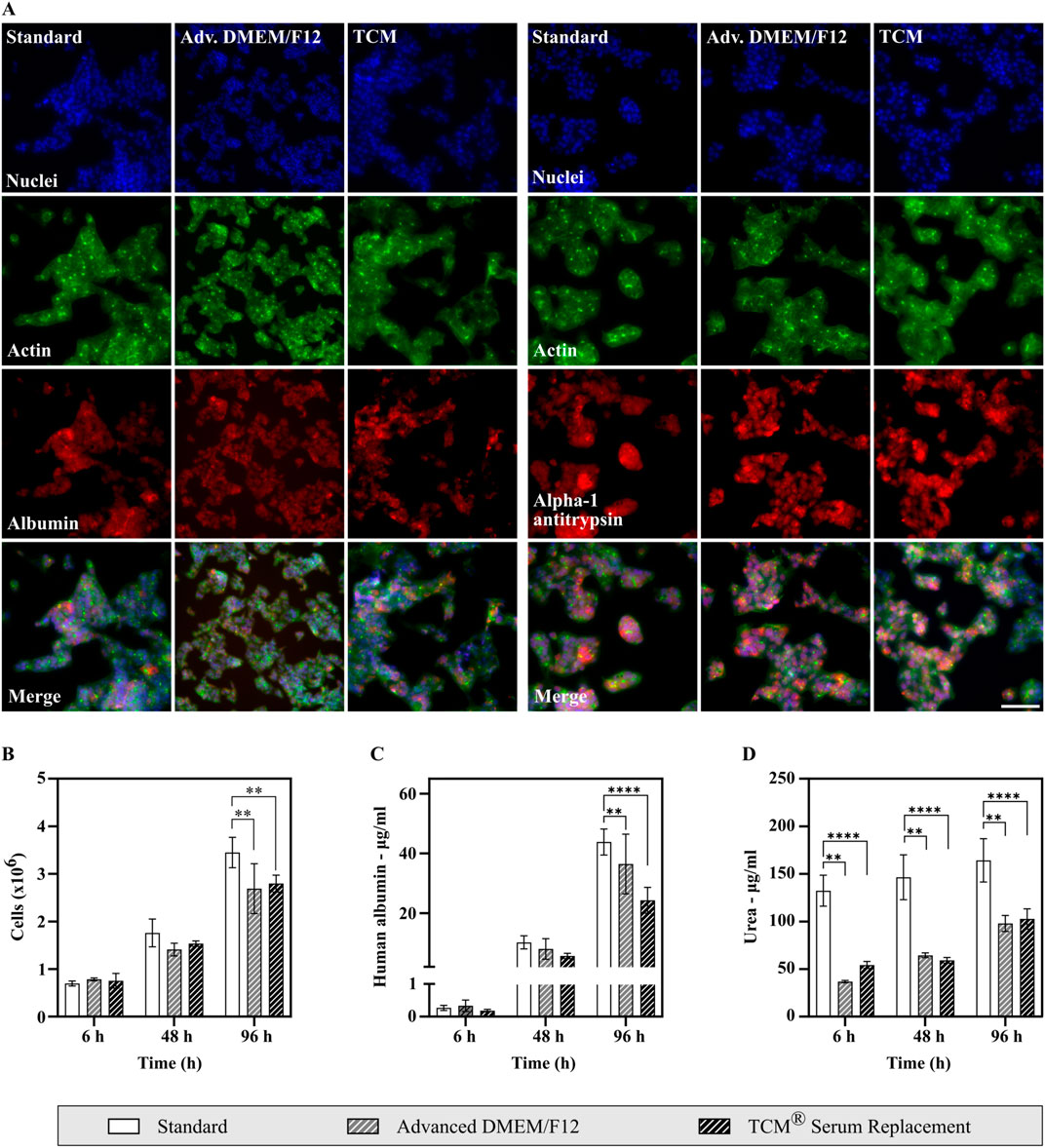
Figure 5. Analysis of HepG2 cell marker expression, albumin production and urea secretion of cells cultivated in either FBS containing medium or serum-free medium. (A) Analysis of HepG2 markers using high content imaging. Cells are shown in ×20 magnification. Scale bar: 100 µm. Cells were fixed using paraformaldehyde and subsequently stained with DAPI (nuclei), Actin Phalloidin Alexa 488 (Actin), and either Rabbit ALB (H126) polyclonal antibody (Albumin, left) or Rabbit IgG Alpha-1-Antitrypsin Polyclonal Antibody (Alpha-1 Antitrypsin, right) in combination with Alexa Fluor 680 goat-anti-rabbit as secondary antibody. (B–D) Assessment of albumin and urea secretion. Supernatant samples were taken, and cells counted after 6 h, 48 h and 96 h (N = 3, n = 2). Statistical significance was assessed using a two-way ANOVA with Dunnett´s correction for multiple comparison. (B) Cell density throughout the experiment. (C) Human albumin concentration measured from cell culture supernatants. Albumin production was shown for all conditions. (D) Urea secretion measured from cell culture supernatants. Cells in all conditions display urea secretion. Higher concentrations of urea in the standard condition can be explained by high urea concentrations present in fetal bovine serum.
Additionally, albumin and urea secretion were analyzed from cell culture supernatants (Figures 5B–D). Samples were taken after 6 h, 48 h and 96 h. Human albumin concentrations increased significantly throughout the experiment in all conditions, showing that HepG2 cells produce and secrete albumin. Albumin concentrations were lower in serum-free conditions, however if corrected for cell density, albumin production was highest in Advanced DMEM/F12 (27.11 µg/106 cells after 96 h) with no significant difference to cells cultivated under FBS containing conditions (25.41 µg/106 cells after 96 h, see Supplementary Figure S5). A lower albumin production was only observed for TCM® Serum Replacement (17.39 µg/106 cells after 96 h).
Similarly, increasing urea concentrations were measured in cell culture supernatant samples from all conditions. A significant difference in concentrations was observed between cells cultivated under FBS containing conditions and serum-free conditions. This can be explained by high urea concentrations present in fetal bovine serum, thus increasing the total concentration of urea in cell culture supernatants. Therefore, to compare conditions independently of the corresponding medium fold changes were calculated (Supplementary Figure S5). Fold changes were highest in Advanced DMEM/F12 with a 2.65-fold increase after 96 h. In cell culture supernatants obtained from cells cultured under FBS containing conditions, urea concentrations increased 1.25-fold, while an increase by 1.90-fold was observed in samples of cells cultivated in TCM® Serum Replacement.
As serum-free cultivated cells display HepG2 typical growth characteristics, morphologies, marker expression as well as albumin and urea secretion, cells in both conditions were used to further assess HepG2 cells in common toxicological assays.
3.3 Functionality assessment in non-clinical safety assays
Functionality of HepG2 cells cultivated in either Advanced DMEM/F12 or TCM® Serum replacement was assessed using different reference compounds in different drug response assays. MIP-DILI compounds (positive and negative) were selected from Dragovic et al. (2016). Additionally, internal reference compounds were used. All compounds are depicted in Table 2. Two assays focusing on overall cell viability were performed using either ATP (CellTiter Glo Assay) or reduction of resazurin to resorufin (Alamar Blue Assay) as a read out. In the CellTiter Glo Assay, cells were treated with 20 different compounds. A slightly higher sensitivity was observed for the serum-free conditions with 6 compounds (standard containing FBS) vs. 7 compounds (Advanced DMEM/F12) vs. 8 compounds (TCM® Serum replacement) displaying an IC50 below 50 μM, the internally set threshold for potential DILI risk (Figures 6A, C). When compared to the human DILI categorization according to the FDA, cells in standard medium identified 4 compounds correctly, missed 8 and identified one negative compound as potentially positive. In the serum-free conditions, one or two compounds were additionally identified as DILI risks, respectively (Figure 6E). Whether the higher sensitivity is due to altered drug response or simply due to less media components binding to the drug, thereby increasing the compound concentration reaching the cells should be addressed in future experiment. For the second assay assessing viability (Alamar Blue Assay), 16 compounds were used. Similar IC50 values were obtained for all tested compounds, and 4 compounds were identified with an IC50 below 50 µM in all conditions (Figure 6B).
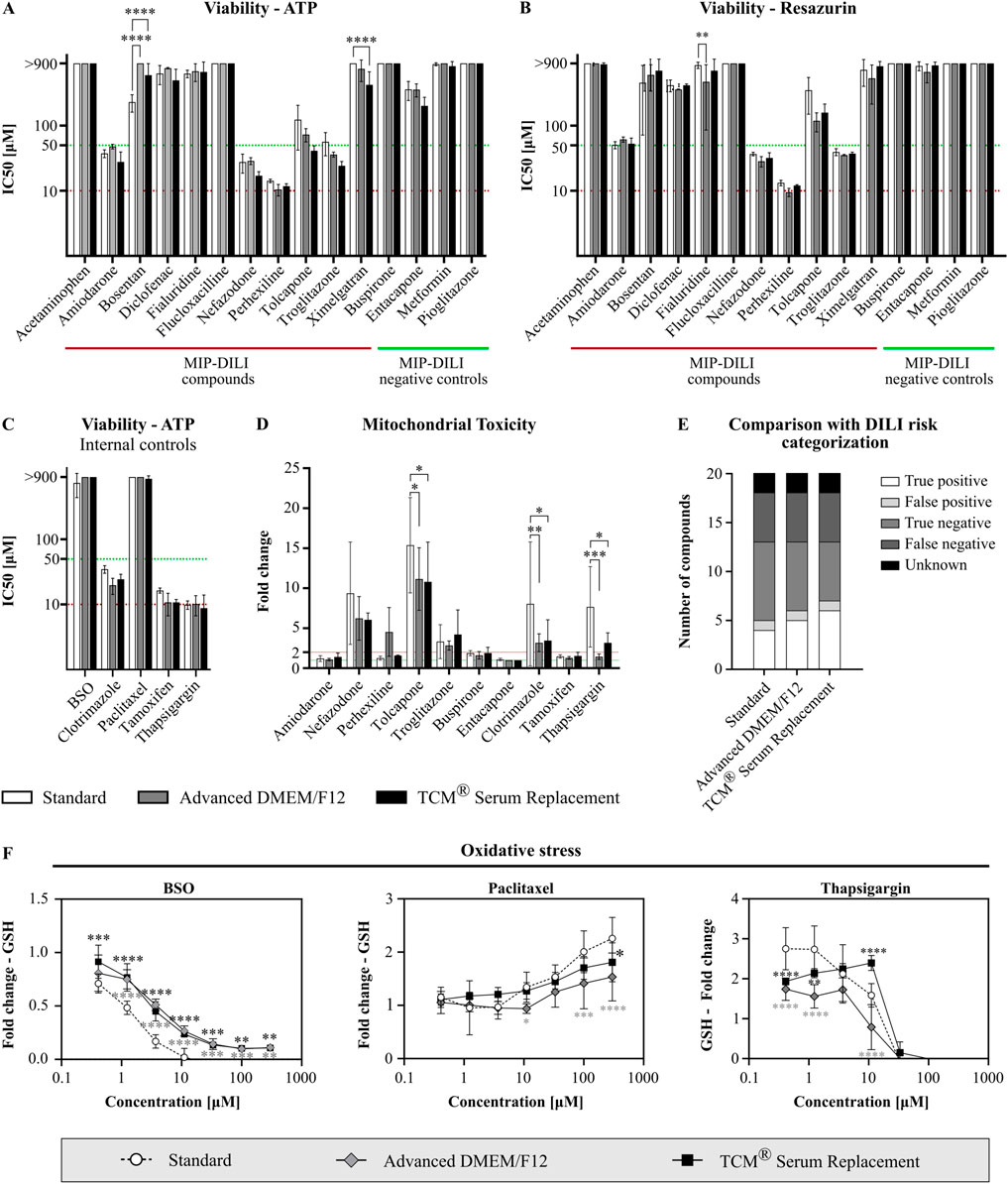
Figure 6. Functionality assessment of serum-free cultivated HepG2. Cells were treated with different reference compounds in a threefold dilution series and subsequently analyzed for viability (N = 3, n = 3), mitochondrial function (N = 3, n = 3), and oxidative stress (N = 2, n = 3). IC50 values were obtained from dose response curves, calculated using a log(inhibitor) vs. response model (variable slope, four parameters, bottom constraint = 0). Statistical significance was assessed using a two-way ANOVA with Dunnett´s correction for multiple comparison for all assays. (A) Viability assay after treatment of HepG2 cells with MIP-DILI compounds using ATP as read-out (CellTiter Glo Assay). (B) Viability assay after treatment with MIP-DILI compounds using the reduction of resazurin to resorufin as read-out (Alamar Blue Assay). (C) Viability assay after treatment of HepG2 cells with additional, internal control compounds using ATP as read-out. (D) Mitochondrial toxicity assay (Glucose Galactose assay). Cells were treated with either MIP-DILI compounds or internal control compounds. Fold changes between the glucose and galactose condition are used as read-out. Only compounds with a fold change >1 are shown. (E) Comparison of in vitro data (CellTiter Glo Assay) with FDA categorization of the used reference compounds. Serum-free cultivated cells identified 1 or 2 compounds as a potential DILI risk, which were missed in the FBS containing condition. (F) Oxidative stress assay for selected reference compounds (BSO, paclitaxel, and thapsigargin). GSH fold changes are used as read-out. For most compounds, GSH fold changes correlated with viability, indicating no oxidative stress. Viability independent changes in GSH content were only observed for BSO, paclitaxel and thapsigargin. Generally, serum-free cultivated cells displayed a lower oxidative stress response.
Two further assays were performed to assess potential mitochondrial toxicity and oxidative stress (Figures 6D, F; Supplementary Figure S6). In the Mitochondrial toxicity assay, comparable read-outs were obtained for cells cultivated in FBS containing medium and TCM® serum replacement with 4 shared compounds displaying a fold change higher than 2 throughout all conditions. Generally, fold changes were higher in the standard FBS condition, however especially when comparing standard cells with TCM® Serum Replacement cultivated cells, the same compounds were identified as potential mitochondrial toxins. Differences for two compounds, perhexiline and thapsigargin, were observed for cells cultured in Advanced DMEM/F12. While perhexiline was only identified as a potential mitochondrial toxin in Advanced DMEM/F12 cultivated cells, thapsigargin was below the two-fold threshold, while above in the other conditions. While for perhexiline, mitotoxicity is not reported as a primary mechanism in vivo, protonated perhexiline was shown to accumulate in the mitochondrial membrane, resulting in uncoupling of mitochondrial oxidative phosphorylation, inhibition of complexes I and II, and subsequent decreased ATP formation (Dragovic et al., 2016). To further address this, more experiments should be conducted to assess whether the observed differences are caused by an altered drug response or are an effect of temporal resolution.
In the oxidative stress assay, changes in GSH caused by most compounds correlated with a decrease in viability, therefore we selected several reference compounds displaying different responses in the oxidative stress assay (GSH changes correlating with viability, GSH increase independent from viability and GSH decrease independent from viability). (Supplementary Figure S6). From these selected compounds, viability dependent changes in GSH were observed for buspirone, diclofenac, nefazodone and perhexiline and comparable results were obtained for all conditions (Supplementary Figure S6). Additionally, treatment with two compounds induced an increase in GSH either at low concentrations (thapsigargin) or at high concentrations (paclitaxel). For both serum-free media, the observed increase was significantly lower compared to cells cultured under serum-containing conditions (Figure 6F). A strong decrease in GSH, independently from viability, was only observed for buthionine sulfoximine (BSO). Again, changes in GSH content were less profound compared to cells cultivated under serum containing conditions (Figure 6F); therefore, an adaption of assay specific thresholds might be necessary when planning to assess oxidative stress in serum-free conditions.
Overall, cells cultured in serum-free conditions displayed a comparable drug response in in vitro toxicology assays assessing viability, mitochondrial toxicity, and oxidative stress response upon compound treatment. Differences observed regarding mitochondrial toxicity and oxidative stress between serum-free cultured cells and cells cultured in FBS containing medium should be addressed in future experiments.
3.4 Assessment of intracellular drug response markers
Additionally to non-clinical safety assays, we assessed the effects of test compounds on a specific set of protein markers relevant for cell health. Seven different markers, involved in cell death/apoptosis (PARP), autophagy (LC3B), stress response (HSP70), oxidative stress (HIF-1-alpha), cell division (Histone H3, pSer10), transcription (RNA Pol II, pSer2) and protein synthesis (EIF4B, pSer422) were compared after treatment with either assay specific control substances (bafilomycin, geldanamycin, actinomycin D or deferoxamine) or three previously tested MIP DILI (negative) controls (amiodarone, perhexiline, and metformin).
When treated with assay specific test substances, cells showed similar response to bafilomycin, with a strong increase in LC3B levels (Figure 7A). After treatment with geldanamycin, cells cultivated in Advanced DMEM/F12 showed a significantly stronger response compared to cells cultivated either in standard medium or TCM (Figure 7B; fold change: 7.4 vs. 20.6 vs. 8.7). Furthermore, cells in serum-free media showed a higher response to actinomycin D (fold change: 3.1 vs. 11.3 vs. 6.6), which was accompanied with a significant decrease in RNA Pol II and EIF4B levels in all conditions (Figure 7C). When treated with deferoxamine, an oxidative stress inducer, only cells in standard medium and TCM showed elevated HIF-1-alpha levels, with standard cells displaying a significantly stronger oxidative stress response, accompanied with increased PARP level, which was not observed in the serum-free media (Figure 7D).
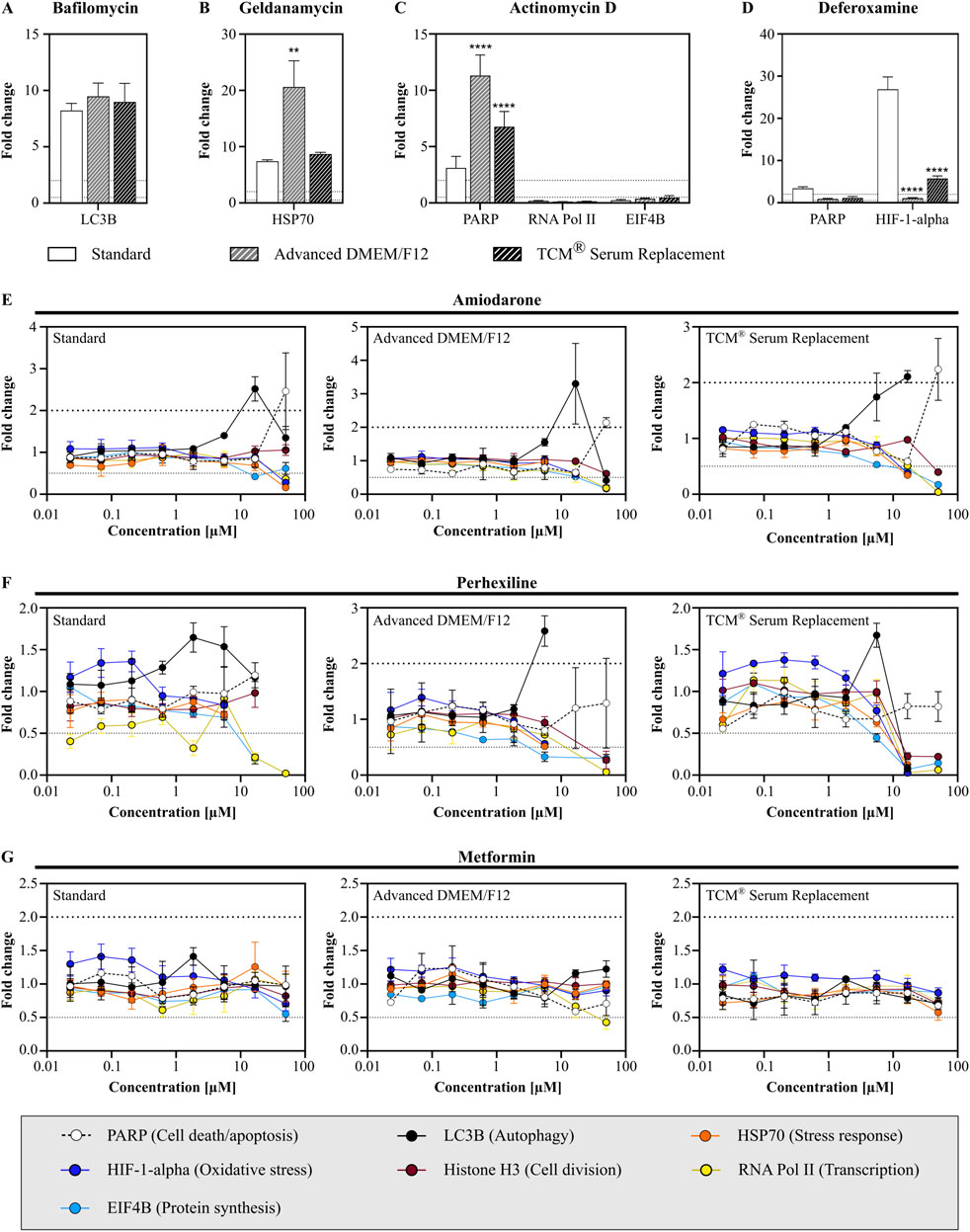
Figure 7. Assessment of intracellular drug response markers after compound treatment. Cells were treated with either assay specific control compounds (A) bafilomycin, (B) geldanamycin, (C) actinomycin D or (D) deferoxamine or MIP-DILI (negative) compounds (E) amiodarone, (F) perhexiline and (G) metformin. Protein markers were measured after 24 h and included PARP (cell death/apoptosis), LC3B (autophagy), HSP70 (stress response), HIF-1-alpha (oxidative stress), p-serine of histone H3 (cell division), p-serin 2/5 RNA Polymerase II (transcription), and p-serin 422 EIF4B (protein synthesis). ANOVA with Dunnett correction for multiple testing was used to assess statistical significance (N = 1, n = 3).
After treatment with the MIP-DILI compounds (amiodarone, perhexiline and metformin), cells displayed a similar drug response across all media. Treatment with amiodarone resulted in elevated PARP levels at the highest concentration, accompanied by a decrease in RNA Pol II, EIF4B, and HSP70 (Figure 7E). A significant decrease in multiple markers indicates that viability was significantly decreased, which given the previously determined IC50 values was expected. Furthermore, elevated LC3B levels were observed in the second highest concentration, similar in all conditions. Both, PARP and LC3B levels did not differ significantly between the different conditions.
After treatment with perhexiline, cell death again occurred at concentrations ≥16.7 µM, resulting in multiple parameters either being drastically reduced or below the detection limit, again in accordance with previous results (Figure 7F). A significant increase in PARP was only observed for cells cultivated in Advanced DMEM/F12, with a significant difference to both standard medium and TCM® Serum Replacement. Treatment with Metformin did not result in elevated markers, only a slight decrease in RNA Pol II levels were observed at the highest concentration tested in cells cultivated in Advanced DMEM/F12 (Figure 7G).
Taken together, cells cultivated in Advanced DMEM/F12 and TCM® Serum Replacement showed stronger drug responses when assessing markers related to cell death/apoptosis and stress response, and dependent on the compound a stronger or similar autophagy response compared to cells cultivated in the standard condition. In contrast, the oxidative stress response was significantly lower in both serum-free media. This was already observed in the previously described oxidative stress assay, measuring GSH content, and should be addressed in further experiments. However, oxidative stress was only observed after treatment with deferoxamine, therefore, more oxidative stress inducers should be tested on HepG2 cells with multiple concentrations and time points, to understand whether cells are indeed less sensitive to oxidative stress or if the oxidative stress response is simply shifted in time and may be observed at different time points.
3.5 Comparison of freezing media
After confirming functionality of serum-free cultivated cells, another important aspect was to evaluate the possibility of cryo-preservation of cells for later use. Standard freezing media usually contain 10%–20% FBS as well as DMSO, glycerin or other substances as anti-freeze agent (Gstraunthaler and Lindl, 2013). In serum-free cell culture, the protective characteristics of the serum are absent, which is why an evaluation of different freezing media should be performed to ensure high cell recovery and viability after thawing. Therefore, four different serum-free freezing media were tested. Three are commercially available (see Table 3), while the fourth medium was a self-made freezing medium, consisting of 45% conditioned medium, 45% fresh medium and 10% DMSO. After thawing of the cells, cell recovery was determined, and assessment of growth and morphology was performed after 24 h and 72 h, respectively.
Cell recovery was highest for Cryo-SFM (77.37% ± 9.37% across all conditions), and conditioned medium (only Standard and TCM® Serum Replacement), while recovery in CryoStore and CryoPan was generally lower (See Figure 8A). Between Standard cells and serum-free cultivated cells, significant differences for recovery were only observed for conditioned medium (Standard: 77.81% ± 2.65%; Advanced DMEM/F12: 38.25% ± 7.43%; TCM Serum Replacement: 48.75% ± 22.27%).
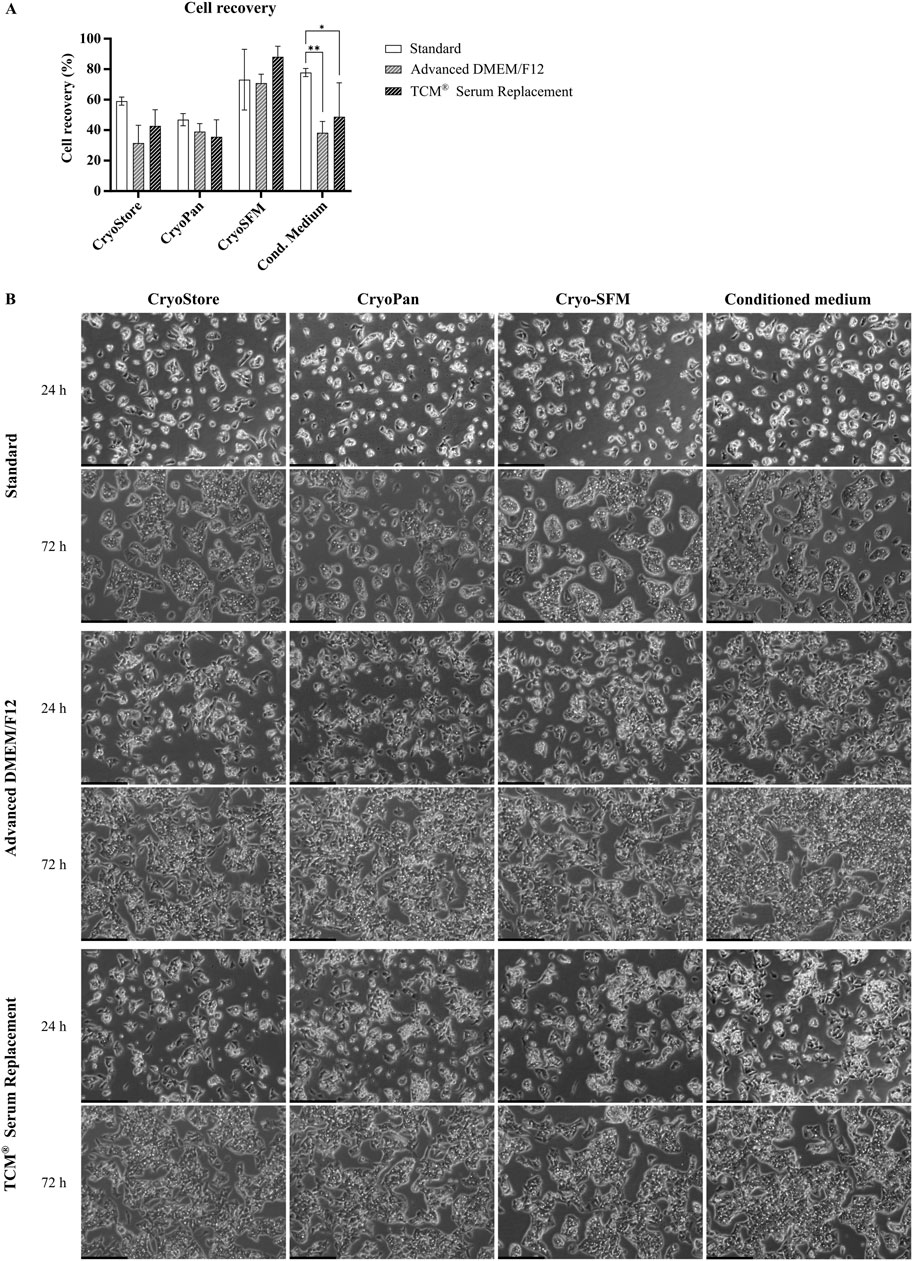
Figure 8. Comparison of freezing media. (A) Recovery rate of cryopreserved cells previously cultivated in serum-containing medium (Standard), Advanced DMEM/F12 or TCM® Serum Replacement. Four different freezing reagents, CryoStore, CryoPan, Cryo-SFM or a self-made freezing medium based on conditioned medium, were compared. Cell recovery was highest for Cryo-SFM (across all conditions), and conditioned medium (only Standard and TCM). Two-way ANOVA with Dunnett correction for multiple testing was used to assessed statistical significance. (B) Microscopic images of thawed cell taken after 24 h and 72 h. Scale bar: 250 µm.
Microscopic images taken after 24 h showed slight differences between serum- and serum-free cultivated cells as already previously observed (Figure 8B). Cells cultured in serum-containing medium showed already small islands, while cells cultivated in serum-free media grew further apart with less cell-cell interactions. No significant differences in cell growth or morphology were observed between the different freezing media, however, particles were observed in all images of cells frozen in CryoPan, which could have originated from the freezing media itself or cell debris.
After 72 h, cells in all condition showed significant cell growth with clear HepG2 typical island formations. Again, islands in serum-free conditions were less dense compared to cells cultivated in serum-containing medium resulting in a higher confluency. Between the different freezing media, no differences were observed.
Taken together, HepG2 cells can easily be cryopreserved using different freezing media resulting in similar growth behavior and similar morphology after thawing. While the recovery rate differed significantly between the different freezing reagents, cell growth after seeding was not impacted.
4 Discussion
As the 3R principles are playing an increasing role in research and drug development, and are gaining increasing attention in society and politics, in vitro technologies are considered a major replacement tool to avoid/reduce animal experimentation (Jochems et al., 2002; van Zutphen et al., 2001; Weihe, 1985). Nevertheless, animal components are still commonly used in cell culture systems to maintain and proliferate cells, causing both scientific and ethical concerns (Gospodarowicz and Moran, 1976; Hughes et al., 2010; Yao and Asayama, 2017). To fully exploit the potential of in vitro technologies as key replacements for animal experimentation, avoiding animal components completely is out of the question. However, since especially FBS is an incredibly complex mixture of nutrients and is until today poorly defined, replacing FBS in cell culture comes with the major task of finding a suitable medium to not only maintain morphology and growth behavior, but cellular functionality (van der Valk et al., 2010).
When deciding to switch out serum containing media with serum-free media, scientists are faced with the question whether designing media themselves or using commercial options. This decision might be influenced by many different aspects and relies heavily on the corresponding situation. Self-made media have the advantage of full control over the formulation (van der Valk et al., 2010). This not only means knowing the full formulation, which is usually not the case for commercial options, but also that media can be easily adapted in case changes are needed, e.g., for a new cell line or in case of shortages in supply (Rafnsdóttir et al., 2023; van der Valk et al., 2010; Weber et al., 2024; Yao and Asayama, 2017). However, designing an animal-component free medium can be highly complex, dependent on the nutritional needs of a certain cell line or cell type as well as assay dependent cellular functions which need to be retained (Cervera et al., 2011; Karnieli et al., 2017; Skrivergaard et al., 2023; van der Valk et al., 2018; Yao and Asayama, 2017). If designing a self-made medium is the preferred option, scientists can significantly benefit from the Fetal Calf Serum free Database (RRID:SCR_018769; https://fcs-free.org), offered by the 3Rs-Centre ULS in collaboration with Animal Free Research United Kingdom.
If setting up a serum-free medium is out of the scope, scientists can rely on commercially available media. In this case, scientists benefit from the usual ready-to-use format, except for few additional supplements, meaning less time and effort needs to be spent (Lindroos et al., 2009; Tan et al., 2015). Furthermore, variability in media composition and costs are significantly decreased due to the tightly regulated and economically effective processes during media production (van der Valk et al., 2018). However, these commercial options have the strong disadvantage of proprietary formulations and the possibility of changes in the formulations without notice (Yao and Asayama, 2017). Furthermore, there is still a significant supply vs. demand problem, with an increasing demand for animal-component free media, while the supply for fully animal-derived component free media however is not sufficient (Brunner et al., 2010). Unfortunately, commercially available media are often designed for one particular cell type or cell line, while media suitable for several cell types are less common.
For this study, designing a media was not the preferred option, therefore different commercially available serum-free media and serum replacements were selected for comparison, using one of the major work horses in early toxicity testing, the HepG2 cell line (Vinken and Rogiers, 2015). During the media selection process, the previously mentioned demand vs. supply chain was highly noticeable with a total number of 5 fully animal-component free or xeno-free products deemed suitable for the planned experiments. Due to the small set of available media, the range was enlarged by including serum-free media as well, if the percentage of animal-derived components within the media was low. Furthermore, since animal components are not restricted to media, TrypLE™, was used to replace the commonly used dissociating agent trypsin and truncated, recombinant vitronectin was used to ensure attachment of cells to the flask under serum-free conditions.
After selecting the inside adaptation protocol as a suitable adaptation strategy by comparing the different protocols described by van der Valk et al., the selected media were compared regarding their long-term culturing capacity. Most media did not support cellular morphology and HepG2 typical growth rates for a prolonged time, with only two media showing long term culturing capacities comparable to the FBS containing condition, indicating missing nutrients in those media that are essential for supporting HepG2 growth. Nevertheless, the used inside adaptation strategy exerted a significant amount of stress on the cells, and therefore cells might not be capable of significantly altering necessary signaling/metabolic pathways based on the new nutritional supply (van der Valk et al., 2010). Therefore, a softer, less challenging long-term adaptation might render more media suitable, however since the goal of this experiment was to find the best media options, this was not further addressed.
Cells adapted to both front-runner media displayed similar growth characteristics, with comparable doubling times and the same epithelial-like morphology, as is typical for HepG2 cells (Arzumanian et al., 2021). Furthermore, the hepatocyte markers albumin and alpha-1-antitrypsin were equally expressed and albumin and urea secretion were shown for all conditions. Since the HepG2 cell line is commonly used to study cell viability, mitochondrial toxicity or oxidative stress upon treatment with new drug candidates, cellular function of these cells cultivated in either one of the two front-runner media was assessed after treatment with either control substances described by the MIP-DILI consortium or internal control compounds (Arzumanian et al., 2021; Dragovic et al., 2016; Vinken and Rogiers, 2015). Throughout the different toxicology assays, serum-free cultivated cells displayed a similar or slightly elevated drug response in the assays focusing on viability/cell death. Comparing our in vitro data with the human DILI categorization according to the FDA, cells cultivated in standard medium identified 4 compounds correctly as having a DILI risk, while 8 DILI-positive compounds were not identified. Serum-free cultivated cells identified one or two additional compounds as DILI risk, showing an improvement of the predictive strength of the HepG2 model. The fact that multiple compounds were falsely identified as DILI negative can be explained by the generally lower expression of drug-metabolizing enzymes and transporters in HepG2 cells in comparison to hepatocytes, as well as the absence of immune cells which are known to mediate toxicity of ximelagatram and flucloxacillin (Arzumanian et al., 2021; Berger et al., 2016; Dragovic et al., 2016; Gerets et al., 2012; Sison-Young et al., 2015; Tyakht et al., 2014; Wiśniewski et al., 2016). For this reason, HepG2 cells are suitable as a comparative early screening model and less suitable to investigate metabolism-mediated toxicity (Ren et al., 2018).
A slightly lower sensitivity of cells cultivated under serum-containing conditions might be explained by the presence of many different factors capable of binding the drug in serum, which might prevent higher concentrations of drugs from reaching the cells. FBS contains between 32–70 g/L total protein, the majority made up by serum albumin, an important carrier protein capable of binding lipids, sterols, trace elements or drugs (Francis, 2010; Gstraunthaler and Lindl, 2013; Mishra and Heath, 2021). If using 10% FBS, this corresponds to 3.2–7.0 g/L (0.32%–0.7% w/v) total protein in the final medium and between 2.0 and 3.6 g/L (0.2%–0.36% w/v) BSA. In both serum-free media, the protein quantity is much lower. Advanced DMEM/F12 contains BSA (AlbuMax® II), human transferrin, and recombinant insulin with a total concentration of ∼0.42 g/L (0.042% w/v) and an albumin concentration of 0.4 g/L. For TCM® Serum Replacement, the formulation is proprietary. However, the manufacturer reports heat treated bovine albumin and transferrin as media components with a final total protein concentration less than 0.1%. The lower protein amount therefore might contribute to the observations made in the assays focusing on viability/cytotoxicity. Nevertheless, changes in sensitivity might also be explained by an altered temporal resolution or even changes in expression of drug metabolizing enzymes. To identify key drivers for these observations, additional experiments should therefore be performed, assessing multiple time points or measuring protein expression of key drug metabolizing enzymes.
More significant differences were observed when assessing oxidative stress. In both serum-free media, response to BSO was less profound when measuring GSH content. When measuring HIF-1-alpha expression, no significant increase in HIF-1-alpha levels was observed in treated vs. untreated cells after treatment with deferoxamine for Advanced DMEM/F12 cultured cells, while cells cultivated in TCM® Serum Replacement showed an increase above the threshold, but still significantly lower compared to cell grown in serum containing medium. This might again be explained by different concentrations of media supplements. Advanced DMEM/F12 contains additional ascorbic acid phosphate, glutathione, and transferrin. While both ascorbic acid and glutathione are antioxidants, transferrin prevents formation of reactive oxygen species by binding free iron (Galaris et al., 2019; Jiang et al., 2020; Mandal et al., 2022; Moritz et al., 2020; Njus et al., 2020; Shen et al., 2021). Furthermore, selen and zinc are supplemented in Advanced DMEM/F12. While not having antioxidant activity themselves, both elements are critical for the activity of antioxidative enzymes, like glutathione reductase and superoxide dismutase (Choi et al., 2018; Kang et al., 2020; Olechnowicz et al., 2018; Tinggi, 2008). Since information regarding the FBS formulation are limited, both concentrations of human serum or FBS were used for comparison with Advanced DMEM/F12 (see Table 4).
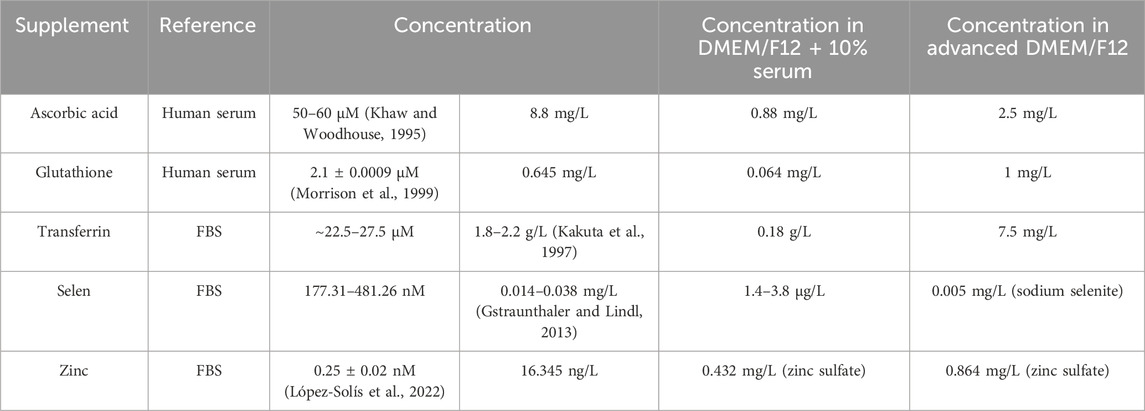
Table 4. Comparison of antioxidative agent concentrations in serum supplemented medium and Advanced DMEM/F12.
Except for transferrin, all other mentioned supplements are present in Advanced DMEM/F12 in higher concentrations compared to DMEM/F12 supplemented with 10% FBS. Therefore, oxidative stress response might indeed be affected. However, to find the definite cause, further experiments are necessary.
Lastly, we want to address the remaining animal-derived components present in both front-runner media. As animal-derived components still compromise the potential of in vitro cell culture models to function as replacement tools for animal experimentation, these compounds should be considered as critical even if present in low concentrations (Messmer et al., 2022; Post et al., 2020). While serum-free media are a better alternative for serum-containing media, any remaining animal-derived components should be removed as well in order to be more ethically acceptable. Additionally, removing animal-derived components is preferential also for scientific reasons, as for instance bovine transferrin was shown to contain a xenoantigen (Neu5Gc) contamination which might impact assay outcomes (Heiskanen et al., 2007). Therefore, next steps should include building a self-made medium based on the formulation on Advanced DMEM/F12 which is publicly available. Advanced DMEM/F12 contains only 10 different supplements, with only albumin as an animal-derived component. Bovine serum albumin is commonly used, due to its lower cost (Keenan et al., 1995). However, studies on functionality of human serum albumin or recombinant human albumin in cell culture already showed that HSA is a suitable alternative (Barbaro et al., 2008; Fanali et al., 2012; Francis, 2010; Keenan et al., 1997; Ng et al., 2008). To find a suitable product, it may be necessary to compare different manufacturers due to different ligand-interactions and in case of recombinant albumin potential alterations in post-translational modifications, dependent on the expression host (Mishra and Heath, 2021). A fully animal-derived component-free medium formulation similar, albeit more complex compared to Advanced DMEM/F12, was already published by Rafnsdóttir et al. and supplements used for this medium should be tried first when comparing different media supplements (Rafnsdóttir et al., 2023).
Additionally, as one important factor necessary for replacing animal-derived components in cell culture are manufacturers, users of cell culture media should communicate the needs and demands in animal-derived component free media (van der Valk et al., 2018). Furthermore, convincing scientists to switch to animal-component free media by publishing and presenting solid data showing comparability of results and advertising the advantages of serum-free culture is key to further drive ambitions to replace animal components in cell culture (Duarte et al., 2023).
Taken together, the obtained data strongly support the use of serum-free media when working with HepG2 cells. Generally, growth characteristics and morphology were maintained, while assay data were comparable with those obtained from cells cultivated under standard, FBS containing conditions.
Data availability statement
The raw data supporting the conclusions of this article will be made available by the authors, without undue reservation.
Ethics statement
Ethical approval was not required for the studies on humans in accordance with the local legislation and institutional requirements because only commercially available established cell lines were used.
Author contributions
LMP: Conceptualization, Data curation, Formal Analysis, Investigation, Methodology, Validation, Visualization, Writing–original draft. JS: Conceptualization, Methodology, Writing–review and editing. FP: Conceptualization, Funding acquisition, Writing–review and editing. DW: Conceptualization, Project administration, Writing–review and editing. PH: Conceptualization, Supervision, Writing–review and editing.
Funding
The author(s) declare that no financial support was received for the research, authorship, and/or publication of this article.
Acknowledgments
The authors thank all members of the Merck FBS phase-out project for constructive discussions and shared enthusiasm, thus driving ambitions to replace animal-derived components in cell and tissue culture. Furthermore, we thank the members of the Early Investigative Toxicology Lab, especially Isabel Koscielski for her support with the Luminex™ FLEXMAP 3D™ platform, as well as Sara Lodholz and Selma Hartlap from the Cell Design Lab for their assistance with image acquisition for growth curve doubling time determinations.
Conflict of interest
Authors LMP, JS, and PH were employed by Merck Healthcare KGaA. Authors FP and DW were employed by Merck KGaA.
Publisher’s note
All claims expressed in this article are solely those of the authors and do not necessarily represent those of their affiliated organizations, or those of the publisher, the editors and the reviewers. Any product that may be evaluated in this article, or claim that may be made by its manufacturer, is not guaranteed or endorsed by the publisher.
Supplementary material
The Supplementary Material for this article can be found online at: https://www.frontiersin.org/articles/10.3389/ftox.2024.1439031/full#supplementary-material
SUPPLEMENTARY FIGURE S1 | Selection of the front-runner media for serum-free culture of HepG2. Long-term culturing capacity of different serum-free media using the inside adaptation procedure (left) and categorization of serum-free media with the reason of termination if a medium was not classified as suitable for long-term culture.
SUPPLEMENTARY FIGURE S2 | Microscopic images of HepG2 cells cultured in different serum-free media or media containing serum replacements on day 3 (serum-free passage 1). Marked conditions were terminated. Scale bar: 100 µm.
SUPPLEMENTARY FIGURE S3 | Microscopic images of HepG2 cells cultured in different serum-free media or media containing serum replacements on day 7 (serum-free passage 2). Marked conditions were terminated. Scale bar: 100 µm.
SUPPLEMENTARY FIGURE S4 | Microscopic images of HepG2 cells cultured in different serum-free media or media containing serum replacements on day 11/12 (serum-free passage 3). Marked conditions were terminated. Scale bar: 100 µm.
SUPPLEMENTARY FIGURE S5 | Analysis of albumin production and urea secretion. Supernatant samples were taken after 6 h, 48 h and 96 h (N = 3, n = 2). Albumin concentrations were normalized to cell count to compare conditions. Urea production was analyzed by calculating the fold change based on samples taken after 6 h in culture. Statistical significance was assessed using a two-way ANOVA with Dunnett´s correction for multiple comparison.
SUPPLEMENTARY FIGURE S6 | Oxidative stress assay using the GSH fold change as read-out. Viability data are shown for comparison. Different responses to compound treatment were observed: viability dependent GSH changes, viability independent GSH increase at either high or low compound concentrations or a viability independent GSH decrease.
References
Abe, K., Ikeda, M., Ariumi, Y., Dansako, H., and Kato, N. (2007). Serum-free cell culture system supplemented with lipid-rich albumin for hepatitis C virus (strain O of genotype 1b) replication. Virus Res. 125 (2), 162–168. doi:10.1016/j.virusres.2006.12.015
Akazawa, D., Morikawa, K., Omi, N., Takahashi, H., Nakamura, N., Mochizuki, H., et al. (2011). Production and characterization of HCV particles from serum-free culture. Vaccine 29 (29), 4821–4828. doi:10.1016/j.vaccine.2011.04.069
Anderson, N. L., and Anderson, N. G. (2002). The human plasma proteome: history, character, and diagnostic prospects. Mol. and Cell. Proteomics 1 (11), 845–867. doi:10.1074/mcp.r200007-mcp200
Anderson, N. L., Polanski, M., Pieper, R., Gatlin, T., Tirumalai, R. S., Conrads, T. P., et al. (2004). The human plasma proteome: a nonredundant list developed by combination of four separate sources. Mol. and Cell. Proteomics 3 (4), 311–326. doi:10.1074/mcp.m300127-mcp200
Arzumanian, V. A., Kiseleva, O. I., and Poverennaya, E. V. (2021). The curious case of the HepG2 cell line: 40 Years of expertise. Int. J. Mol. Sci. 22 (23), 13135. doi:10.3390/ijms222313135
Ashrafian, H., Horowitz, J. D., and Frenneaux, M. P. (2007). Perhexiline. Cardiovasc Drug Rev. 25 (1), 76–97. doi:10.1111/j.1527-3466.2007.00006.x
Barbaro, B., Kuechle, J., Salehi, P., Rodriguez, L., Qi, M., Gangemi, A., et al. (2008). Increased albumin concentration reduces apoptosis and improves functionality of human islets. Artif. Cells Blood Substit. Immobil. Biotechnol. 36 (1), 74–81. doi:10.1080/10731190701857819
Bell, C. C., Hendriks, D. F. G., Moro, S. M. L., Ellis, E., Walsh, J., Renblom, A., et al. (2016). Characterization of primary human hepatocyte spheroids as a model system for drug-induced liver injury, liver function and disease. Sci. Rep. 6 (1), 25187. doi:10.1038/srep25187
Berger, B., Donzelli, M., Maseneni, S., Boess, F., Roth, A., Krähenbühl, S., et al. (2016). Comparison of liver cell models using the basel phenotyping cocktail. Front. Pharmacol. 7, 443. doi:10.3389/fphar.2016.00443
Biaggio, R. T., Abreu-Neto, M. S., Covas, D. T., and Swiech, K. (2015). Serum-free suspension culturing of human cells: adaptation, growth, and cryopreservation. Bioprocess Biosyst. Eng. 38 (8), 1495–1507. doi:10.1007/s00449-015-1392-9
Bilgen, B., Orsini, E., Aaron, R. K., and Ciombor, D. M. K. (2007). FBS suppresses TGF-beta1-induced chondrogenesis in synoviocyte pellet cultures while dexamethasone and dynamic stimuli are beneficial. J. Tissue Eng. Regen. Med. 1 (6), 436–442. doi:10.1002/TERM.56
Bratko, F., Shehata, M., Toth, S., Schwarzmeier, J., and Koren, S. (2002). Novel serum replacement based on bovine ocular fluid: a useful tool for cultivation of different animal cells in vitro. ALTEX - Altern. animal Exp. 19 (1), 15–20. Available at: https://www.altex.org/index.php/altex/article/view/1114.
Brunner, D., Frank, J., Appl, H., Schöffl, H., Pfaller, W., and Gstraunthaler, G. (2010). Serum-free cell culture: the serum-free media interactive online database. ALTEX 27, 53–62. doi:10.14573/altex.2010.1.53
Cervera, L., Gutiérrez, S., Gòdia, F., and Segura, M. M. (2011). Optimization of HEK 293 cell growth by addition of non-animal derived components using design of experiments. BMC Proc. 5 (8), P126. doi:10.1186/1753-6561-5-S8-P126
Choi, S., Liu, X., and Pan, Z. (2018). Zinc deficiency and cellular oxidative stress: prognostic implications in cardiovascular diseases. Acta Pharmacol. Sin. 39 (7), 1120–1132. doi:10.1038/aps.2018.25
Dragovic, S., Vermeulen, N. P. E., Gerets, H. H., Hewitt, P. G., Ingelman-Sundberg, M., Park, B. K., et al. (2016). Evidence-based selection of training compounds for use in the mechanism-based integrated prediction of drug-induced liver injury in man. Arch. Toxicol. 90 (12), 2979–3003. doi:10.1007/s00204-016-1845-1
Duarte, A. C., Costa, E. C., Filipe, H. A. L., Saraiva, S. M., Jacinto, T., Miguel, S. P., et al. (2023). Animal-derived products in science and current alternatives. Biomater. Adv. 151, 213428. doi:10.1016/j.bioadv.2023.213428
Dykens, J. A., Jamieson, J. D., Marroquin, L. D., Nadanaciva, S., Xu, J. J., Dunn, M. C., et al. (2008). In vitro assessment of mitochondrial dysfunction and cytotoxicity of nefazodone, trazodone, and buspirone. Toxicol. Sci. 103 (2), 335–345. doi:10.1093/TOXSCI/KFN056
Fanali, G., di Masi, A., Trezza, V., Marino, M., Fasano, M., and Ascenzi, P. (2012). Human serum albumin: from bench to bedside. Mol. Asp. Med. 33 (3), 209–290. doi:10.1016/j.mam.2011.12.002
Fattinger, K., Funk, C., Pantze, M., Weber, C., Reichen, J., Stieger, B., et al. (2001). The endothelin antagonist bosentan inhibits the canalicular bile salt export pump: a potential mechanism for hepatic adverse reactions. Clin. Pharmacol. and Ther. 69, 223–231. doi:10.1067/MCP.2001.114667
Francis, G. L. (2010). Albumin and mammalian cell culture: implications for biotechnology applications. Cytotechnology 62 (1), 1–16. doi:10.1007/s10616-010-9263-3
Galaris, D., Barbouti, A., and Pantopoulos, K. (2019). Iron homeostasis and oxidative stress: an intimate relationship. Biochimica Biophysica Acta (BBA) - Mol. Cell Res. 1866 (12), 118535. doi:10.1016/j.bbamcr.2019.118535
Gao, F. F., Lv, J. W., Wang, Y., Fan, R., Li, Q., Zhang, Z., et al. (2016). Tamoxifen induces hepatotoxicity and changes to hepatocyte morphology at the early stage of endocrinotherapy in mice. Biomed. Rep. 4 (1), 102–106. doi:10.3892/BR.2015.536
Gerets, H. H., Tilmant, K., Gerin, B., Chanteux, H., Depelchin, B. O., Dhalluin, S., et al. (2012). Characterization of primary human hepatocytes, HepG2 cells, and HepaRG cells at the mRNA level and CYP activity in response to inducers and their predictivity for the detection of human hepatotoxins. Cell Biol. Toxicol. 28 (2), 69–87. doi:10.1007/s10565-011-9208-4
Gómez-Lechón, M. J., Ponsoda, X., O'Connor, E., Donato, T., Castell, J. V., and Jover, R. (2003). Diclofenac induces apoptosis in hepatocytes by alteration of mitochondrial function and generation of ROS. Toxicol. Vitro 66 (11), 2155–2167. doi:10.1016/J.BCP.2003.08.003
Gospodarowicz, D., and Moran, J. S. (1976). Growth factors in mammalian cell culture. Annu. Rev. Biochem. 45, 531–558. doi:10.1146/ANNUREV.BI.45.070176.002531
Gstraunthaler, G., and Lindl, T. (2013). Zell-und Gewebekultur: Allgemeine Grundlagen und spezielle Anwendungen. 7 ed. Heidelberg, Germany: Springer-Verlag. doi:10.1007/978-3-642-35997-2
Gstraunthaler, G., Lindl, T., and van der Valk, J. (2013). A plea to reduce or replace fetal bovine serum in cell culture media. Cytotechnology 65 (5), 791–793. doi:10.1007/S10616-013-9633-8
Gunn, P. J., Green, C. J., Pramfalk, C., and Hodson, L. (2017). In vitro cellular models of human hepatic fatty acid metabolism: differences between Huh7 and HepG2 cell lines in human and fetal bovine culturing serum. Physiol. Rep. 5 (24), e13532. doi:10.14814/phy2.13532
Haasio, K., Koponen, A., Penttilä, K. E., and Nissinen, E. (2002). Effects of entacapone and tolcapone on mitochondrial membrane potential. Eur. J. Pharmacol. 453 (1), 21–26. doi:10.1016/S0014-2999(02)02383-X
Hammond, S. L., Ham, R. G., and Stampfer, M. R. (1984). Serum-free growth of human mammary epithelial cells: rapid clonal growth in defined medium and extended serial passage with pituitary extract. Proc. Natl. Acad. Sci. U. S. A. 81 (17), 5435–5439. doi:10.1073/PNAS.81.17.5435
Heath, M. F., Costa-Jussa, F. R., Jacobs, J. M., and Jacobson, W. (1985). The induction of pulmonary phospholipidosis and the inhibition of lysosomal phospholipases by amiodarone. Br. J. Exp. Pathol. 66 (44), 391–397. Available at: https://www.ncbi.nlm.nih.gov/pmc/articles/pmid/2992568/?tool=EBI.
Heiskanen, A., Satomaa, T., Tiitinen, S., Laitinen, A., Mannelin, S., Impola, U., et al. (2007). N-glycolylneuraminic acid xenoantigen contamination of human embryonic and mesenchymal stem cells is substantially reversible. Stem cells 25 (1), 197–202. doi:10.1634/stemcells.2006-0444
Hep G2 HB-8065 (2024). ATCC. Available at: https://www.atcc.org/products/hb-8065.
Hughes, C. S., Postovit, L. M., and Lajoie, G. A. (2010). Matrigel: a complex protein mixture required for optimal growth of cell culture. Proteomics 10 (9), 1886–1890. doi:10.1002/PMIC.200900758
Jiang, X., Chen, J., Wang, M. C., and Wang, J. (2020). Glutathione quantification in live cells with real-time imaging and flow cytometry. Star. Protoc. 1 (3), 100170. doi:10.1016/j.xpro.2020.100170
Jochems, C. E. A., Van der Valk, J. B. F., Stafleu, F. R., and Baumans, V. (2002). The use of fetal bovine serum: ethical or scientific problem? Altern. Lab. Anim. 30 (2), 219–227. doi:10.1177/026119290203000208
Johansson, L., Klinth, J., Holmqvist, O., and Ohlson, S. (2003). Platelet lysate: a replacement for fetal bovine serum in animal cell culture? Cytotechnology 42 (2), 67–74. doi:10.1023/B:CYTO.0000009820.72920.cf
Kakuta, K., Orino, K., Yamamoto, S., and Watanabe, K. (1997). High levels of ferritin and its iron in fetal bovine serum. Comp. Biochem. Physiol. A Physiol. 118 (1), 165–169. doi:10.1016/s0300-9629(96)00403-3
Kalgutkar, A. S., Vaz, A. D. N., Lame, M. E., Henne, K. R., Soglia, J., Zhao, S. X., et al. (2005). Bioactivation of the nontricyclic antidepressant nefazodone to a reactive quinone-imine species in human liver microsomes and recombinant cytochrome P450 3A4. Drug Metab. Dispos. 33 (2), 243–253. doi:10.1124/DMD.104.001735
Kang, D., Lee, J., Wu, C., Guo, X., Lee, B. J., Chun, J.-S., et al. (2020). The role of selenium metabolism and selenoproteins in cartilage homeostasis and arthropathies. Exp. and Mol. Med. 52 (8), 1198–1208. doi:10.1038/s12276-020-0408-y
Karnieli, O., Friedner, O. M., Allickson, J. G., Zhang, N., Jung, S., Fiorentini, D., et al. (2017). A consensus introduction to serum replacements and serum-free media for cellular therapies. Cytotherapy 19 (2), 155–169. doi:10.1016/j.jcyt.2016.11.011
Kassianides, C., Nussenblatt, R., Palestine, A. G., Mellow, S. D., and Hoofnagle, J. H. (1990). Liver injury from cyclosporine A. Dig. Dis. Sci. 35 (6), 693–697. doi:10.1007/bf01540169
Keenan, J., Doherty, G., and Clynes, M. (1995). Separation of growth-stimulating activity of BSA fraction V from the bulk of albumin using Heparin Sepharose Chromatography. Cytotechnology 19 (1), 63–72. doi:10.1007/bf00749756
Keenan, J., Dooley, M., Pearson, D., and Clynes, M. (1997). Recombinant human albumin in cell culture: evaluation of growth-promoting potential for NRK and SCC-9 cells in vitro. Cytotechnology 24 (3), 243–252. doi:10.1023/a:1007916930200
Khasawneh, R. R., Al Sharie, A. H., Abu-El Rub, E., Serhan, A. O., and Obeidat, H. N. (2019). Addressing the impact of different fetal bovine serum percentages on mesenchymal stem cells biological performance. Mol. Biol. Rep. 46 (4), 4437–4441. doi:10.1007/S11033-019-04898-1
Khaw, K. T., and Woodhouse, P. (1995). Interrelation of vitamin C, infection, haemostatic factors, and cardiovascular disease. BMJ 310 (6994), 1559–1563. doi:10.1136/bmj.310.6994.1559
Kindmark, A., Jawaid, A., Harbron, C. G., Barratt, B. J., Bengtsson, O. F., Andersson, T. B., et al. (2007). Genome-wide pharmacogenetic investigation of a hepatic adverse event without clinical signs of immunopathology suggests an underlying immune pathogenesis. Pharmacogenomics J. 8 (3), 186–195. doi:10.1038/sj.tpj.6500458
Klaas, M., Möll, K., Mäemets-Allas, K., Loog, M., Järvekülg, M., and Jaks, V. (2021). Long-term maintenance of functional primary human hepatocytes in 3D gelatin matrices produced by solution blow spinning. Sci. Rep. 11 (1), 20165. doi:10.1038/s41598-021-99659-1
Klein, S., Mueller, D., Schevchenko, V., and Noor, F. (2014). Long-term maintenance of HepaRG cells in serum-free conditions and application in a repeated dose study. J. Appl. Toxicol. 34 (10), 1078–1086. doi:10.1002/jat.2929
Kleiner, D. E., Gaffey, M. J., Sallie, R., Tsokos, M., Nichols, L., McKenzie, R., et al. (1997). Histopathologic changes associated with fialuridine hepatotoxicity. Mod. Pathol. 10 (3), 192–199. Available at: https://europepmc.org/article/MED/9071726.
Korolczuk, A., Caban, K., Amarowicz, M., Czechowska, G., and Irla-Miduch, J. (2016). Oxidative stress and liver morphology in experimental cyclosporine A-induced hepatotoxicity. BioMed Res. Int. 2016, 5823271–5823279. doi:10.1155/2016/5823271
Kostrubsky, S. E., Strom, S. C., Kalgutkar, A. S., Kulkarni, S., Atherton, J., Mireles, R., et al. (2006). Inhibition of hepatobiliary transport as a predictive method for clinical hepatotoxicity of nefazodone. Toxicol. Sci. 90 (2), 451–459. doi:10.1093/TOXSCI/KFJ095
Lau, A. J., and Chang, T. K. H. (2014). Fetal bovine serum and human constitutive androstane receptor: evidence for activation of the SV23 splice variant by artemisinin, artemether, and arteether in a serum-free cell culture system. Toxicol. Appl. Pharmacol. 277 (2), 221–230. doi:10.1016/J.TAAP.2014.03.023
Lin, Y., Liu, J., Zhang, X., Li, L., Hu, R., Liu, J., et al. (2014). A prospective, randomized study on hepatotoxicity of anastrozole compared with tamoxifen in women with breast cancer. Cancer Sci. 105 (9), 1182–1188. doi:10.1111/CAS.12474
Lindroos, B., Boucher, S., Chase, L., Kuokkanen, H., Huhtala, H., Haataja, R., et al. (2009). Serum-free, xeno-free culture media maintain the proliferation rate and multipotentiality of adipose stem cells in vitro. Cytotherapy 11 (7), 958–972. doi:10.3109/14653240903233081
Longo, D. M., Yang, Y., Watkins, P. B., Howell, B. A., and Siler, S. Q. (2016). Elucidating differences in the hepatotoxic potential of tolcapone and entacapone with DILIsym(®), a mechanistic model of drug-induced liver injury. CPT Pharmacometrics Syst. Pharmacol. 5 (1), 31–39. doi:10.1002/PSP4.12053
López-Solís, L., Companys, E., Puy, J., Blindauer, C. A., and Galceran, J. (2022). Direct determination of free Zn concentration in samples of biological interest. Anal. Chim. Acta 1229, 340195. doi:10.1016/j.aca.2022.340195
Lübberstedt, M., Müller-Vieira, U., Biemel, K. M., Darnell, M., Hoffmann, S. A., Knöspel, F., et al. (2015). Serum-free culture of primary human hepatocytes in a miniaturized hollow-fibre membrane bioreactor for pharmacological in vitro studies. J. Tissue Eng. Regen. Med. 9 (9), 1017–1026. doi:10.1002/term.1652
Luckert, C., Schulz, C., Lehmann, N., Thomas, M., Hofmann, U., Hammad, S., et al. (2016). Comparative analysis of 3D culture methods on human HepG2 cells. Arch. Toxicol. 91 (1), 393–406. doi:10.1007/S00204-016-1677-Z
Ma, C., Peng, Y., Li, H., and Chen, W. (2021). Organ-on-a-Chip: a new paradigm for drug development. Trends Pharmacol. Sci. 42 (2), 119–133. doi:10.1016/J.TIPS.2020.11.009
Mandal, P. K., Roy, R. G., and Samkaria, A. (2022). Oxidative stress: glutathione and its potential to protect methionine-35 of Aβ peptide from oxidation. ACS Omega 7 (31), 27052–27061. doi:10.1021/acsomega.2c02760
Marupudi, N. I., Han, J. E., Li, K. W., Renard, V. M., Tyler, B. M., and Brem, H. (2007). Paclitaxel: a review of adverse toxicities and novel delivery strategies. Expert Opin. Drug Saf. 6 (5), 609–621. doi:10.1517/14740338.6.5.609
Maurer, H. (1986). Towards chemically-defined, serum-free media for mamalian cell culture. Anim. Cell Cult., 13–31.
McClain, C. J., Price, S., Barve, S., Devalarja, R., and Shedlofsky, S. (1999). Acetaminophen hepatotoxicity: an update. Curr. Gastroenterol. Rep. 1 (1), 42–49. doi:10.1007/S11894-999-0086-3
McKee, C., Soeda, J., Asilmaz, E., Sigalla, B., Morgan, M., Sinelli, N., et al. (2013). Propranolol, a β-adrenoceptor antagonist, worsens liver injury in a model of non-alcoholic steatohepatitis. Biochem. Biophys. Res. Commun. 437 (4), 597–602. doi:10.1016/j.bbrc.2013.07.005
Merten, O.-W. (2002). Virus contaminations of cell cultures - a biotechnological view. Cytotechnology 39 (2), 91–116. doi:10.1023/A:1022969101804
Messmer, T., Klevernic, I., Furquim, C., Ovchinnikova, E., Dogan, A., Cruz, H., et al. (2022). A serum-free media formulation for cultured meat production supports bovine satellite cell differentiation in the absence of serum starvation. Nat. Food 3 (1), 74–85. doi:10.1038/s43016-021-00419-1
Mishra, V., and Heath, R. J. (2021). Structural and biochemical features of human serum albumin essential for eukaryotic cell culture. Int. J. Mol. Sci. 22 (16), 8411. doi:10.3390/ijms22168411
Monshi, M. M., Faulkner, L., Gibson, A., Jenkins, R. E., Farrell, J., Earnshaw, C. J., et al. (2013). Human leukocyte antigen (HLA)-B*57:01-restricted activation of drug-specific T cells provides the immunological basis for flucloxacillin-induced liver injury. Hepatology 57 (2), 727–739. doi:10.1002/hep.26077
Moritz, B., Schmitz, A. E., Rodrigues, A. L. S., Dafre, A. L., and Cunha, M. P. (2020). The role of vitamin C in stress-related disorders. J. Nutr. Biochem. 85, 108459. doi:10.1016/j.jnutbio.2020.108459
Morrison, J. A., Jacobsen, D. W., Sprecher, D. L., Robinson, K., Khoury, P., and Daniels, S. R. (1999). Serum glutathione in adolescent males predicts parental coronary heart disease. Circulation 100 (22), 2244–2247. doi:10.1161/01.CIR.100.22.2244
Naito, Y., Hamada-Tsutsumi, S., Yamamoto, Y., Kogure, A., Yoshioka, Y., Watashi, K., et al. (2018). Screening of microRNAs for a repressor of hepatitis B virus replication. Oncotarget 9 (52), 29857–29868. doi:10.18632/ONCOTARGET.25557
Nakamura, R., Nakajima, D., Sato, H., Endo, Y., Ohara, O., and Kawashima, Y. (2021). A simple method for in-depth proteome analysis of mammalian cell culture conditioned media containing fetal bovine serum. Int. J. Mol. Sci. 22 (5), 2565. doi:10.3390/ijms22052565
Ng, E. S., Davis, R., Stanley, E. G., and Elefanty, A. G. (2008). A protocol describing the use of a recombinant protein-based, animal product-free medium (APEL) for human embryonic stem cell differentiation as spin embryoid bodies. Nat. Protoc. 3 (5), 768–776. doi:10.1038/nprot.2008.42
Nikfarjam, L., and Farzaneh, P. (2012). Prevention and detection of mycoplasma contamination in cell culture. Cell J. 13 (4), 203–212. Available at: https://www.ncbi.nlm.nih.gov/pmc/articles/PMC3584481/.
Njus, D., Kelley, P. M., Tu, Y.-J., and Schlegel, H. B. (2020). Ascorbic acid: the chemistry underlying its antioxidant properties. Free Radic. Biol. Med. 159, 37–43. doi:10.1016/j.freeradbiomed.2020.07.013
O'Dwyer, P. J., Hamilton, T. C., LaCreta, F. P., Gallo, J. M., Kilpatrick, D., Halbherr, T., et al. (2016). Phase I trial of buthionine sulfoximine in combination with melphalan in patients with cancer. J. Clin. Oncol. 14 (1), 249–256. doi:10.1200/JCO.1996.14.1.249
Oleaga, C., Bernabini, C., Smith, A. S. T., Srinivasan, B., Jackson, M., McLamb, W., et al. (2016). Multi-Organ toxicity demonstration in a functional human in vitro system composed of four organs. Sci. Rep. 6 (1), 20030. doi:10.1038/srep20030
Olechnowicz, J., Tinkov, A., Skalny, A., and Suliburska, J. (2018). Zinc status is associated with inflammation, oxidative stress, lipid, and glucose metabolism. J. Physiological Sci. 68 (1), 19–31. doi:10.1007/s12576-017-0571-7
Patil, S. R., and Biradar, P. M. (2017). Earthworm’s coelomic fluid: extraction and importance. Int. J. Adv. Sci. Res. 2, 01–04.
Pieper, R., Gatlin, C. L., Makusky, A. J., Russo, P. S., Schatz, C. R., Miller, S. S., et al. (2003). The human serum proteome: display of nearly 3700 chromatographically separated protein spots on two-dimensional electrophoresis gels and identification of 325 distinct proteins. Proteomics 3 (7), 1345–1364. doi:10.1002/pmic.200300449
Pirovino, M., Müller, O., Zysset, T., and Honegger, U. (1988). Amiodarone-induced hepatic phospholipidosis: correlation of morphological and biochemical findings in an animal model. Hepatology 8 (3), 591–598. doi:10.1002/HEP.1840080326
Post, M. J., Levenberg, S., Kaplan, D. L., Genovese, N., Fu, J., Bryant, C. J., et al. (2020). Scientific, sustainability and regulatory challenges of cultured meat. Nat. Food 1 (7), 403–415. doi:10.1038/s43016-020-0112-z
Psychogios, N., Hau, D. D., Peng, J., Guo, A. C., Mandal, R., Bouatra, S., et al. (2011). The human serum metabolome. PLoS One 6 (2), e16957. doi:10.1371/JOURNAL.PONE.0016957
Puck, T., Cieciura, S., and Robinson, A. (1958). Genetics of somatic mammalian cells. III. Long-term cultivation of euploid cells from human and animal subjects. J. Exp. Med. 108 (6), 945–956. doi:10.1084/JEM.108.6.945
Rafnsdóttir, Ó. B., Kiuru, A., Tebäck, M., Friberg, N., Revstedt, P., Zhu, J., et al. (2023). A new animal product free defined medium for 2D and 3D culturing of normal and cancer cells to study cell proliferation and migration as well as dose response to chemical treatment. Toxicol. Rep. 10, 509–520. doi:10.1016/j.toxrep.2023.04.001
Rajagopalan, R., Iyer, S., and Perez, A. (2005). Comparison of pioglitazone with other antidiabetic drugs for associated incidence of liver failure: no evidence of increased risk of liver failure with pioglitazone. Diabetes, Obes. Metababolism 7 (2), 161–169. doi:10.1111/J.1463-1326.2004.00382.X
Rauch, C., Feifel, E., Amann, E. M., Spötl, H. P., Schennach, H., Pfaller, W., et al. (2011). Alternatives to the use of fetal bovine serum: human platelet lysates as a serum substitute in cell culture media. ALTEX 28 (4), 305–316. doi:10.14573/ALTEX.2011.4.305
Ren, Z., Chen, S., Ning, B., and Guo, L. (2018). “Use of liver-derived cell lines for the study of drug-induced liver injury,” in Drug-induced liver toxicity. Editors M. Chen, and Y. Will (New York: Springer), 151–177. doi:10.1007/978-1-4939-7677-5_8
Routledge, P. A., and Shand, D. G. (1979). Clinical pharmacokinetics of propranolol. Clin. Pharmacokinet. 4 (2), 73–90. doi:10.2165/00003088-197904020-00001
Runge, D., Runge, D. M., Jäger, D., Lubecki, K. A., Beer Stolz, D., Karathanasis, S., et al. (2000). Serum-free, long-term cultures of human hepatocytes: maintenance of cell morphology, transcription factors, and liver-specific functions. Biochem. Biophysical Res. Commun. 269 (1), 46–53. doi:10.1006/bbrc.2000.2215
Sefried, S., Häring, H. U., Weigert, C., and Eckstein, S. S. (2018). Suitability of hepatocyte cell lines HepG2, AML12 and THLE-2 for investigation of insulin signalling and hepatokine gene expression. Open Biol. 8 (10), 180147. doi:10.1098/rsob.180147
Shahdadfar, A., Frønsdal, K., Haug, T., Reinholt, F. P., Brinchmann, J. E., and Brinchmann, J. E. (2005). In vitro expansion of human mesenchymal stem cells: choice of serum is a determinant of cell proliferation, differentiation, gene expression, and transcriptome stability. Stem cells 23 (9), 1357–1366. doi:10.1634/STEMCELLS.2005-0094
Shen, J., Griffiths, P. T., Campbell, S. J., Utinger, B., Kalberer, M., and Paulson, S. E. (2021). Ascorbate oxidation by iron, copper and reactive oxygen species: review, model development, and derivation of key rate constants. Sci. Rep. 11 (1), 7417. doi:10.1038/s41598-021-86477-8
Shurrab, N. T., and Arafa, E. S. A. (2020). Metformin: a review of its therapeutic efficacy and adverse effects. Obes. Med. 17, 100186. doi:10.1016/J.OBMED.2020.100186
Sison-Young, R. L. C., Mitsa, D., Jenkins, R. E., Mottram, D., Alexandre, E., Richert, L., et al. (2015). Comparative proteomic characterization of 4 human liver-derived single cell culture models reveals significant variation in the capacity for drug disposition, bioactivation, and detoxication. Toxicol. Sci. 147 (2), 412–424. doi:10.1093/toxsci/kfv136
Skrivergaard, S., Young, J. F., Sahebekhtiari, N., Semper, C., Venkatesan, M., Savchenko, A., et al. (2023). A simple and robust serum-free media for the proliferation of muscle cells. Food Res. Int. 172, 113194. doi:10.1016/j.foodres.2023.113194
Smith, M. T. (2003). Mechanisms of troglitazone hepatotoxicity. Chem. Res. Toxicol. 16 (6), 679–687. doi:10.1021/tx034033e
Subbiahanadar Chelladurai, K., Selvan Christyraj, J. D., Rajagopalan, K., Yesudhason, B. V., Venkatachalam, S., Mohan, M., et al. (2021). Alternative to FBS in animal cell culture - an overview and future perspective. Heliyon 7 (8), e07686. doi:10.1016/J.HELIYON.2021.E07686
Sun, B., Liang, Z., Wang, Y., Yu, Y., Zhou, X., Geng, X., et al. (2024). A 3D spheroid model of quadruple cell co-culture with improved liver functions for hepatotoxicity prediction. Toxicology 505, 153829. doi:10.1016/j.tox.2024.153829
Tan, K. Y., Teo, K. L., Lim, J. F. Y., Chen, A. K. L., Reuveny, S., Oh, S. K. W., et al. (2015). Serum-free media formulations are cell line–specific and require optimization for microcarrier culture. Cytotherapy 17 (8), 1152–1165. doi:10.1016/j.jcyt.2015.05.001
Tang, F., Xie, Y., Cao, H., Yang, H., Chen, X., and Xiao, J. (2017). Fetal bovine serum influences the stability and bioactivity of resveratrol analogues: a polyphenol-protein interaction approach. Food Chem. 219, 321–328. doi:10.1016/J.FOODCHEM.2016.09.154
Tang, W. (2005). The metabolism of diclofenac - enzymology and toxicology perspectives. Curr. Drug Metab. 4 (4), 319–329. doi:10.2174/1389200033489398
Terada, S., Nishimura, T., Sasaki, M., Yamada, H., and Miki, M. (2002). Sericin, a protein derived from silkworms, accelerates the proliferation of several mammalian cell lines including a hybridoma. Cytotechnology 40 (1-3), 3–12. doi:10.1023/A:1023993400608
Thastrup, O., Cullen, P. J., Drobak, B. K., Hanley, M. R., and Dawson, A. P. (1990). Thapsigargin, a tumor promoter, discharges intracellular Ca2+ stores by specific inhibition of the endoplasmic reticulum Ca2(+)-ATPase. Proc. Natl. Acad. Sci. U. S. A. 87 (7), 2466–2470. doi:10.1073/PNAS.87.7.2466
Tinggi, U. (2008). Selenium: its role as antioxidant in human health. Environ. Health Prev. Med. 13 (2), 102–108. doi:10.1007/s12199-007-0019-4
Tyakht, A. V., Ilina, E. N., Alexeev, D. G., Ischenko, D. S., Gorbachev, A. Y., Semashko, T. A., et al. (2014). RNA-Seq gene expression profiling of HepG2 cells: the influence of experimental factors and comparison with liver tissue. BMC Genomics 15 (1), 1108. doi:10.1186/1471-2164-15-1108
van der Valk, J., Bieback, K., Buta, C., Cochrane, B., Dirks, W. G., Fu, J., et al. (2018). Fetal bovine serum (FBS): past – present – future. ALTEX 35 (1), 99–118. doi:10.14573/altex.1705101
van der Valk, J., Brunner, D., De Smet, K., Fex Svenningsen, Å., Honegger, P., Knudsen, L. E., et al. (2010). Optimization of chemically defined cell culture media - replacing fetal bovine serum in mammalian in vitro methods. Toxicol. Vitro 24 (4), 1053–1063. doi:10.1016/j.tiv.2010.03.016
van der Valk, J., Mellor, D., Brands, R., Fischer, R., Gruber, F., Gstraunthaler, G., et al. (2004). The humane collection of fetal bovine serum and possibilities for serum-free cell and tissue culture. Toxicol. Vitro 18 (1), 1–12. doi:10.1016/J.TIV.2003.08.009
van Zutphen, L. F. M., Baumans, V., and Beynen, A. C. (2001). Principles of laboratory animal science. Revised Edition. 2 ed. Amsterdam, Netherlands: Elsevier Science Publishers.
Vinken, M., and Rogiers, V. (2015). Protocols in in vitro hepatocyte research. New York: Springer. doi:10.1007/978-1-4939-2074-7/COVER
Wang, H., Brown, P. C., Chow, E. C. Y., Ewart, L., Ferguson, S. S., Fitzpatrick, S., et al. (2021). 3D cell culture models: drug pharmacokinetics, safety assessment, and regulatory consideration. Clin. Transl. Sci. 14 (5), 1659–1680. doi:10.1111/cts.13066
Weber, T., Bajramovic, J., and Oredsson, S. (2024). Preparation of a universally useable, animal product free, defined medium for 2D and 3D culturing of normal and cancer cells. MethodsX 12, 102592. doi:10.1016/j.mex.2024.102592
Weihe, W. H. (1985). Use and misuse of an imprecise concept: alternative methods in animal experiments. Lab. Anim. 19 (1), 19–26. doi:10.1258/002367785780890758
Wessman, S. J., and Levings, R. L. (1999). Benefits and risks due to animal serum used in cell culture production. Dev. Biol. Stand. 99, 3–8.
Wiśniewski, J. R., Vildhede, A., Norén, A., and Artursson, P. (2016). In-depth quantitative analysis and comparison of the human hepatocyte and hepatoma cell line HepG2 proteomes. J. Proteomics 136, 234–247. doi:10.1016/j.jprot.2016.01.016
Wrzesinski, K., Magnone, M. C., Hansen, L. V., Kruse, M. E., Bergauer, T., Bobadilla, M., et al. (2013). HepG2/C3A 3D spheroids exhibit stable physiological functionality for at least 24 days after recovering from trypsinisation. Toxicol. Res. 2 (3), 163–172. doi:10.1039/c3tx20086h
Yao, T., and Asayama, Y. (2017). Animal-cell culture media: history, characteristics, and current issues. Reproductive Med. Biol. 16 (2), 99–117. doi:10.1002/rmb2.12024
Yong, C. S., Li, D. X., Prabagar, B., Park, B. C., Yi, S. J., Yoo, B. K., et al. (2007). The effect of beta-cyclodextrin complexation on the bioavailability and hepatotoxicity of clotrimazole. Pharmazie 62 (10), 756–759.
Zheng, X., Baker, H., Hancock, W. S., Fawaz, F., McCaman, M., and Pungor, E. (2006). Proteomic analysis for the assessment of different lots of fetal bovine serum as a raw material for cell culture. Part IV. Application of proteomics to the manufacture of biological drugs. Biotechnol. Prog. 22 (5), 1294–1300. doi:10.1021/BP060121O
Keywords: serum-free, in vitro toxicology, sustainability, animal welfare, HepG2
Citation: Pfeifer LM, Sensbach J, Pipp F, Werkmann D and Hewitt P (2024) Increasing sustainability and reproducibility of in vitro toxicology applications: serum-free cultivation of HepG2 cells. Front. Toxicol. 6:1439031. doi: 10.3389/ftox.2024.1439031
Received: 27 May 2024; Accepted: 30 October 2024;
Published: 22 November 2024.
Edited by:
Tilo Michael Weber, Animal Welfare Academy, GermanyReviewed by:
Seiichi Ishida, Sojo University, JapanAditya Reddy Kolli, Philip Morris International, Switzerland
Copyright © 2024 Pfeifer, Sensbach, Pipp, Werkmann and Hewitt. This is an open-access article distributed under the terms of the Creative Commons Attribution License (CC BY). The use, distribution or reproduction in other forums is permitted, provided the original author(s) and the copyright owner(s) are credited and that the original publication in this journal is cited, in accordance with accepted academic practice. No use, distribution or reproduction is permitted which does not comply with these terms.
*Correspondence: Philip Hewitt, cGhpbGlwLmhld2l0dEBtZXJja2dyb3VwLmNvbQ==