- 1Department of Pharmaceutical Sciences, College of Pharmacy, University of New Mexico Health Sciences Center, Albuquerque, NM, United States
- 2Department of Biochemistry and Molecular Biology, School of Medicine, University of New Mexico Health Sciences Center, Albuquerque, NM, United States
- 3Department of Neurosciences, School of Medicine, University of New Mexico Health Sciences Center, Albuquerque, NM, United States
Aging is a complex biological process involving multiple interacting mechanisms and is being increasingly linked to environmental exposures such as wildfire smoke. In this review, we detail the hallmarks of aging, emphasizing the role of telomere attrition, cellular senescence, epigenetic alterations, proteostasis, genomic instability, and mitochondrial dysfunction, while also exploring integrative hallmarks - altered intercellular communication and stem cell exhaustion. Within each hallmark of aging, our review explores how environmental disasters like wildfires, and their resultant inhaled toxicants, interact with these aging mechanisms. The intersection between aging and environmental exposures, especially high-concentration insults from wildfires, remains under-studied. Preliminary evidence, from our group and others, suggests that inhaled wildfire smoke can accelerate markers of neurological aging and reduce learning capabilities. This is likely mediated by the augmentation of circulatory factors that compromise vascular and blood-brain barrier integrity, induce chronic neuroinflammation, and promote age-associated proteinopathy-related outcomes. Moreover, wildfire smoke may induce a reduced metabolic, senescent cellular phenotype. Future interventions could potentially leverage combined anti-inflammatory and NAD + boosting compounds to counter these effects. This review underscores the critical need to study the intricate interplay between environmental factors and the biological mechanisms of aging to pave the way for effective interventions.
1 Introduction
You are going to die (>95% confidence). Odds are strong that the cause of your death will be age-related. Ample scientific evidence suggests that contaminants in our environment, including air pollution (Pope et al., 2009), can not only contribute to the cause of death, but also as a result shorten lifespan. However, there is also common thought that environmental challenges (for instance caloric restriction and fasting) may enhance cellular and organismal efficiency to promote longevity. A growing number of scientists believe this mortal fate can be altered, and death might not be a guarantee, as certain organisms and cells have been effectively “immortalized”. “Why humans age” is a timeless question, with recorded answers dating back to Aristotle’s theory of aging. His comprehension of biology was understandably limited, and he was tethered with terminology that was more romanticized and metaphorical. Yet, he surmised that the aging process coincides with the growth and decay of lungs, the exhaustion of heat in the heart, and a general cooling as we become geriatric (Aristotle, 2023). Unfortunately, the question of “why we age” was largely disregarded by the scientific community for nearly 1,000 years thereafter.
During the Darwinian era of science, researchers believed that aging was a natural aspect of evolution, with intervention being an impossibility. Furthermore, most humans during this period of history died of infectious diseases, deeming scientific investigation in aging as unnecessary. These feelings of indifference would change in the early 1900’s after scientists realized that mortality rate of diseases increased in concert with age. Through intervention studies, the caloric restriction theory showed promise in slowing down the process of aging and extending lifespan (Holehan and Merry, 1986).
Fortunately, humans have significantly advanced our ability to both investigate biological mechanisms and alter the natural outcomes of life. In the early 1970’s and 1980’s, nematodes were employed by Dr. Michael R. Klass and others to determine genetic variations that could prolong lifespan. To date, the list of longevity-associated genes has risen to 115 in total, spanning 25 species (Yu et al., 2021; Bin-Jumah et al., 2022). Aging research sprawled outward in all directions, looking for any and every way possible to increase longevity. One group even found that the removal of gonads will increase lifespan (Hsin and Kenyon, 1999).
Some trends (thankfully) never made it to the clinic. But, through a massive undertaking, consistencies emerged, mechanisms of aging were theorized, and some have been popularized. We now understand that human longevity is modestly heritable - somewhere between 12% and 25% (Herskind et al., 1996; Kaplanis et al., 2018; Ruby et al., 2018; van den Berg et al., 2017; van den Berg et al., 2019)- with family clusters showing a further increase in longevity incidence (Pedersen et al., 2017). Enhancements for these families include insulin sensitivity (Wijsman et al., 2011) and lipid metabolism, leading to healthy lipid levels in the circulatory system (Vaarhorst et al., 2011). Additionally, some families are observed to have more robust immune systems and metabolisms, leading to extensions in longevity (Andersen et al., 2012; Ash et al., 2015). However, the question of “why humans age” still persists, with our current best guesses revolving around the López-Otín et al. (2013) publication of the 9 hallmarks of aging in 2013; updated to 15 hallmarks in 2022 (Schmauck-Medina et al., 2022) (Figure 1).
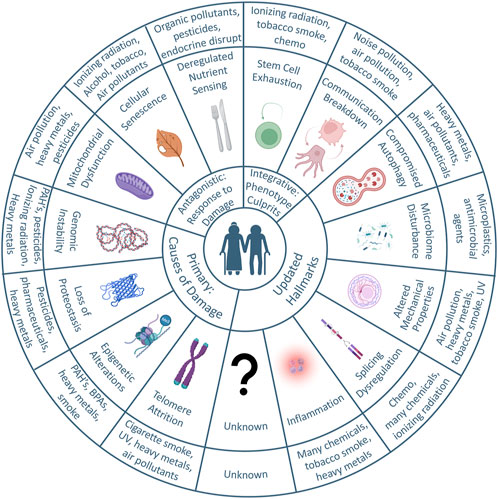
FIGURE 1. Graphical abstract: the hallmarks of aging and environmental contributors. Adapted from the original and updated hallmarks of aging, the circular plot illustrates the concepts known to contribute to aging thus far and the potential influences of environmental toxicants. The circle immediately out from the center illustrates the main categories. Primary hallmarks are the causes of damage, and include telomere attrition, epigenetic alterations, loss of proteostasis, and genomic instability. Antagonistic hallmarks are responses to damage, and include mitochondrial dysfunction, cellular senescence, and deregulated nutrient sensing. Integrative hallmarks manifest via the aging phenotype and include stem cell exhaustion and communication breakdown. The Hallmarks were updated after a 2022 Aging Research and Drug Discovery meeting. The most outer circle illustrates the environmental toxicants that are known to affect each of the primary, antagonistic, integrative, or updated hallmarks.
The original hallmarks fall into 3 main categories of damage-causing (primary), response to damage (antagonistic), and those that manifest via the aging phenotype (integrative). Primary hallmarks that cause damage include genomic instability, telomere attrition, epigenetic alterations, and loss of proteostasis. From antagonistic damaging stimuli, our bodies can be subjected to deregulated nutrient sensing, mitochondrial dysfunction, and cellular senescence. With accumulated damage, we manifest the aging phenotype through stem cell exhaustion and altered intercellular communication. Strangely, the hallmarks themselves are not guaranteed as “causes of aging” but could also be viewed as “the reason for mortality.” To illustrate, we will frame this argument through the lens of common model organisms and their “reason for mortality.” Typically, cancer is the leading cause of mortality across mouse strains (70%–90%) (Leibiger and Berggren, 2006; Brady and Attardi, 2010; Feng et al., 2011; Ng et al., 2015; Zhao et al., 2018b).
A meta-analysis was performed on the putative longevity interventions in mouse models, which revealed >80% of interventions are linked to cancer inhibition (Keshavarz et al., 2022). As such, those longevity interventions linked to cancer incidence prevention could be viewed as “mortality reduction” rather than “aging interventions”. Just as the cause of death in mice is typically cancer, reductions in intestinal stem cell function can be linked to the cause of death in Drosophila melanogaster (Rera et al., 2012). From genetic manipulation studies, several longevity genes have been targeted to reduce intestinal stem cell hyperproliferation, which resulted in an extended lifespan (Waters and Kaye, 2002; Mahesh and Kaskel, 2008; van Heemst, 2010; Xing, 2010; Vijayakumar et al., 2011; López-Otín et al., 2016; Bartke, 2019). In yeast, increases to extrachromosomal circular DNA elements (eccDNA) have been linked with mortality (Sinclair and Guarente, 1997). This was reversed through sirtuin activation, resulting in lifespan extension. For C. elegans, the natural cause of death is not fully understood. Studies have recently pointed toward a positive correlation between active pharyngeal pumping with longevity (de Zelicourt et al., 2016). These results were expanded by observing two types of death. Namely, an infected pharynx leading to early mortality, and pharyngeal atrophy leading to longevity (Zhu et al., 2002). Regardless, with recent advances in Caenorhabditis elegans lifespan study automation (Felker et al., 2020) and high throughput data analysis pipelines (Small et al., 2020), the “reason for mortality” could soon come into focus.
While a great deal of effort–and progress–has been made in recent years understanding the basic molecular and cellular pathways that promote aging, the role of environmental stressors has not yet entered into the “hallmarks of aging” lexicon. When examining aging from a bottom-up approach, it is a complicated, multidimensional issue with contributions from all levels of cellular health. To fully grasp this, one would need to understand the aging-associated input and interactions from the molecular level of each cell, build that understanding in a stepwise manner to the level of tissue organization, and deconvolute the crosstalk from an individual layer before interlayer dependencies could be understood. Adding the implications of xenobiotic agents/chemicals to this mix is an exponential increase in complexity. Fortunately, scientific advancements are not restricted to an all-or-nothing approach. From the top-down perspective, research has shown that chemicals in our environment exist that can impact each and every hallmark of aging.
For example, exposure to heavy metals can induce oxidative stress, linking exposure to the aging hallmarks of mitochondrial dysfunction and DNA damage. These same metals can directly inhibit DNA repair. Therefore, these metals can be implicated in the hallmarks of epigenetic alteration, genomic instabilty, loss of proteostasis, and compromised autophagy. There have also been direct epidemiological links between air pollution and a decrease in life expectancies. Notably, C. Adren Pope et al. and others found that a reduction in air pollution of 10 μg/m3 was associated with an increased mean life expectancy of 0.17 years (Pope et al., 2009; Correia et al., 2013). For context, wildfires consistently generate air pollution levels well in excess of 100 μg/m3 for weeks at a time. Others have sought to determine mechanistic understandings, and found DNA damage events in the lung (Kaur et al., 2021), vascular endothelial activation (Aragon et al., 2016) and neuroinflammatory effects in the brain (Haghani et al., 2020; Scieszka et al., 2022). This inexorable linkage between the environment and each hallmark of aging is explored in more detail below.
2 Primary hallmarks of aging: genomic instability, telomere attrition, epigenetic alterations and clocks, and loss of Proteostasis
2.1 Genomic instability
Genomic instability is defined as an increased susceptibility to mutational frequency and other genetic alterations during cellular division. Genomic instability is a prerequisite for many cancers, is modestly heritable, clearly vulnerable to environmental toxicants, and increases with age (Vijg and Suh, 2013; Vijg and Montagna, 2017). Regarding cancer, the systems in charge of genomic PMCRS (preventative maintenance, checks, repair, and service) have failed, causing dysregulated cellular division. The heritability of genomic instability mainly arises from conferred mutations in DNA repair enzymes themselves, or other molecules that stabilize cellular processes during division (NCI Dictionary of Cancer Terms - NCI, 2011). However, genomic instability can also arise from environmental toxicant exposures, including heavy metals like chromium, cadmium, and arsenic (Langie et al., 2015; Balali-Mood et al., 2021; Medina et al., 2022). Specifically, arsenic is known to cause epigenetic changes, disruptions to DNA base excision repair and nonhomologous end joining, increases to oxidative stress, abnormal apoptotic signaling, impaired lineage commitment of hematopoietic progenitors, and immunosuppression through autophagy alteration (Bolt et al., 2010a; Bolt et al., 2010b; Bolt et al., 2012; Bolt and Klimecki, 2012; Medina et al., 2017; Medina et al., 2020; Medina et al., 2021; Parvez et al., 2017; Zhou et al., 2020). Mechanistically, arsenic biotransformation can cause s-adenosylmethionine depletion leading to epigenetic alterations, while different forms of downstream monomethylarsonic acid and dimethylarsinic acid cause increases to ROS generation, leading to DNA damage and mitochondrial dysfunction (Minatel et al., 2018). Biotransformation products of arsenic are also known to compete with zinc finger domains in transcription factors and DNA repair enzymes, such as such as GATA-1, GATA-2, PARP-1, XPC, APE1, OGG-1, XRCC, and ERCC (Minatel et al., 2018; Medina et al., 2022). The simultaneous damage of DNA and inhibition of repair mechanisms can lead to pathogenic outcomes like cancer, or cell-cycle inhibiting results that lead to senescence or apoptosis, among others.
Somewhat paradoxically, genomic instability can also confer cellular stability. Of note, extrachromosomal circular DNA (eccDNA) is a type of circular, double-stranded DNA element within the nucleus. Studies have found that these exist in healthy (Møller et al., 2018), cancerous (Fan et al., 2011), and aged cells (Hull et al., 2019), with many concluding that these elements drive tumor formation (Turner et al., 2017) due to enhanced chromatin accessibility (Wu et al., 2019). However, Shoura et al. (2017) found eccDNA in worm germ line cells, which begs the question of heritability. In humans, some eccDNAs originate from telomeres (t-circles) and from centromeres (Zuo et al., 2022). The consistency between these two are that they both consist of tandem repeats, but the functionality of tandem repeats remains unknown, with recent work suggesting translational possibilities (Al-Turki and Griffith, 2023). In cell culture, t-circles were required for healthy telomere maintenance (Neumann et al., 2013), and excreted eccDNAs have been found in vitro. Here, they are reported as messengers that mousee and human cells recognize (Wang et al., 2021b). Based on the accepted eccDNA formation mechanisms, one could speculate that lucky cells might acquire gain-of-function eccDNAs that confer longevity. This is based on the recently found gain of function enhancement to transcription factor repair fidelity (Xu et al., 2021). Alternative to genomic instability, the heritability of genomic stability is woefully understudied. In fact, the majority of studies were only able to identify a small number of genes with variations. Namely, variants for APOE and FOXO3A (Nygaard et al., 2014; Flachsbart et al., 2017) that could confer longevity in individuals across generations. The variants in APOE and FOXO3A genes have been shown to play a role in regulating DNA repair and oxidative stress response, respectively. However, the complexity of the human genome and the interactions between different genetic and environmental factors make it challenging to fully understand the heritability of genomic stability.
2.2 Telomere attrition
Perhaps one of the best-studied hallmarks of aging, telomere attrition has been linked to somatic aging (Vaiserman and Krasnienkov, 2021) and artificially extending telomeres has been found to reverse signs of aging in rodents (Jaskelioff et al., 2011). However, the predictive framework and ability to reverse telomere-related aging effects have seen mixed results. Succinctly put, the replicative ability of cells has a limit, and this limit has been eponymously named the Hayflick Limit (Hayflick and Moorhead, 1961) after a series of experiments performed by Leonard Hayflick in 1961. Embryonic stem cells activate the gene for telomerase reverse transcriptase, an enzyme that extends the end-caps of chromosomes, called telomeres. However, most adult stem cells lack telomerase expression.
After each cellular replication, telomeric DNA shortens by 50–200 base pairs due to incomplete lagging strand synthesis (Srinivas et al., 2020). DNA polymerases are unable to fully replicate the 3′ DNA sequence, leading to “the end-replication problem” (Watson, 1972; Olovnikov, 1973). Telomere length is highly heterogeneous between tissue types. It has also been shown that telomeres are highly susceptible to oxidative damage (Oikawa and Kawanishi, 1999), meaning lifestyle choices and environmental exposures can directly affect the length of cellular telomeres. Regardless, successive cellular divisions by stem cells will eventually lead to full telomere attrition, resulting in cell cycle arrest. As such, the length of telomeres was thought to be associated with the biological somatic age of humans, and has been proposed as a key driver of aging (von Zglinicki et al., 2021). These studies reached a fever pitch when the CEO of BioViva, Elizabeth Parrish, traveled to Columbia for a gene therapy injection designed to activate her cells’ telomerase gene. BioViva claimed that her immune cells showed a reduction in “telomeric age” of 20 years in some cases. Unfortunately, this study lacked US government approval, had an n = 1, and was not widely promoted within the academic community.
Regarding environmental exposures, air pollution has been shown to shorten telomere length in several cell types, including leukocytes, placenta, and lung epithelial cells (Zhao et al., 2018a; Chang-Chien et al., 2021), which directly correlates with accelerated aging (Pieters et al., 2016), and cognitive decline in the elderly (Colicino et al., 2017). Alarmingly, prenatal exposure to air pollution resulted in shorter leukocyte telomere length at birth (Lee et al., 2020; Durham et al., 2022). One follow-up study showed these prenatally exposed children had a significant increase in repeated wheeze tests 4 years later. How the lungs of unborn children are subject to leukocyte telomere effects was not discussed. However, these results are a stark reminder of the interconnectedness of organ systems and the continued linkage of environmental exposures with aging.
2.3 Epigenetic alterations and clocks
By root definition, an epigenetic alteration is an alteration that is above the genetic code. These alterations do not change the genetic code itself but alter the expression of genes through modifications to bases in heterochromatin and euchromatin, leading to increased or decreased accessibility. Histone tails can be rapidly modified before, during, and after the cell cycle via methylation, acetylation, ubiquitination, and phosphorylation on different amino acid residues. Conversely, the DNA has a relatively stable presence of methyl groups, noncoding RNAs (ncRNA) bound to methyl groups, or ncRNAs bound to the DNA sequence itself (Figure 2). Histones can also be methylated, but it is much less common. All of these alterations serve to change the effects of transcription, DNA accessibility, and mitotic bookmarks.
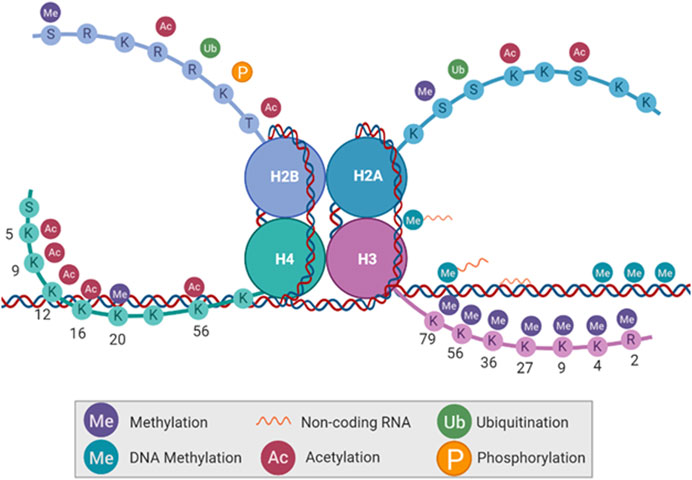
FIGURE 2. Types of epigenetic modifications. DNA base pairs and histone tail amino acid residues can be altered without changing the DNA code itself. Histone tails can be methylated, acetylated, ubiquitinated, and phosphorylated. DNA can be methylated as well, with the absence or addition of non-coding RNA.
For a brief history, evidence of transcriptional changes based on epigenetic alterations began in the 1960’s through direct DNA and RNA comparisons at different timepoints during whale pregnancy (Berdyshev et al., 1967). However, the ability to directly measure the methylation status of particular DNA loci was not popularized until the 1980s through the use of methylation-sensitive restriction enzymes. These loci are often referred to as CpG islands, which are defined as clusters of Cytosine-phosphate-Guanine dinucleotides and are the target of DNA methyltransferases.
Due to the DNA methylome being relatively stable, methyl group additions play a critical role in gene silencing. These can be additions in gene promoter regions, which affect transcription factor binding, or alterations to histones and their tails, resulting in heterochromatin (Esteller, 2008). Regarding histone tails, >60 post-translational modifications have been identified (Sterner and Berger, 2000; Cuthbert et al., 2004; Nowak and Corces, 2004; Nathan et al., 2006; Nelson et al., 2006; Shilatifard, 2006; Berger, 2007; Goldberg et al., 2007). Regarding ncRNAs, they can act independently or in addition to other epigenetic modifications to silence genes or maintain gene silencing. For an in-depth review of these topics, there are a plethora of publications available (Sterner and Berger, 2000; Cuthbert et al., 2004; Nowak and Corces, 2004; Nelson et al., 2006; Shilatifard, 2006; Trojer and Reinberg, 2006; Berger, 2007; Goldberg et al., 2007; Jones and Baylin, 2007; Ruthenburg et al., 2007; Gal-Yam et al., 2008).
Regarding environmental exposures, air pollution has been shown to modulate the epigenetic landscape differentially throughout lifespan based on the onset and duration of exposure (Ferrari et al., 2019). Broadly, prenatal exposures have been linked with global alterations, with specific focus on long interspaced nuclear elements 1 (LINE-1) and its function of telomere reprogramming during embryo development (Wang et al., 2021a; Kohlrausch et al., 2022); childhood alterations are varied and are increased with asthma; and adult alterations are fewer than those seen during childhood but are greatly increased by disease states. For an overview adapted from Ferrari et al. (2019), see Table 1. With respect to functional outcomes, air pollution-induced epigenetic changes have been associated with lung cancer (Li et al., 2017), COPD (Leclercq et al., 2017), cardiovascular disease (Chen et al., 2018), atherosclerosis (Chi et al., 2016), Alzheimer’s disease (Calderón-Garcidueñas et al., 2020), accelerated biological aging (Ward-Caviness et al., 2016) and many others (Wilhelm-Benartzi et al., 2011; Janssen et al., 2013; Liu et al., 2015; Breton et al., 2016; Byun et al., 2016; Motta et al., 2016; Ding et al., 2017; Solaimani et al., 2017; Li et al., 2018; Maghbooli et al., 2018; Miller et al., 2018; Rodosthenous et al., 2018; Callahan et al., 2019; Goodson et al., 2019; Gruzieva et al., 2019; Cantone et al., 2020; Eze et al., 2020; Ma et al., 2020; Tsamou et al., 2020; Liang et al., 2021; Mukherjee et al., 2021).
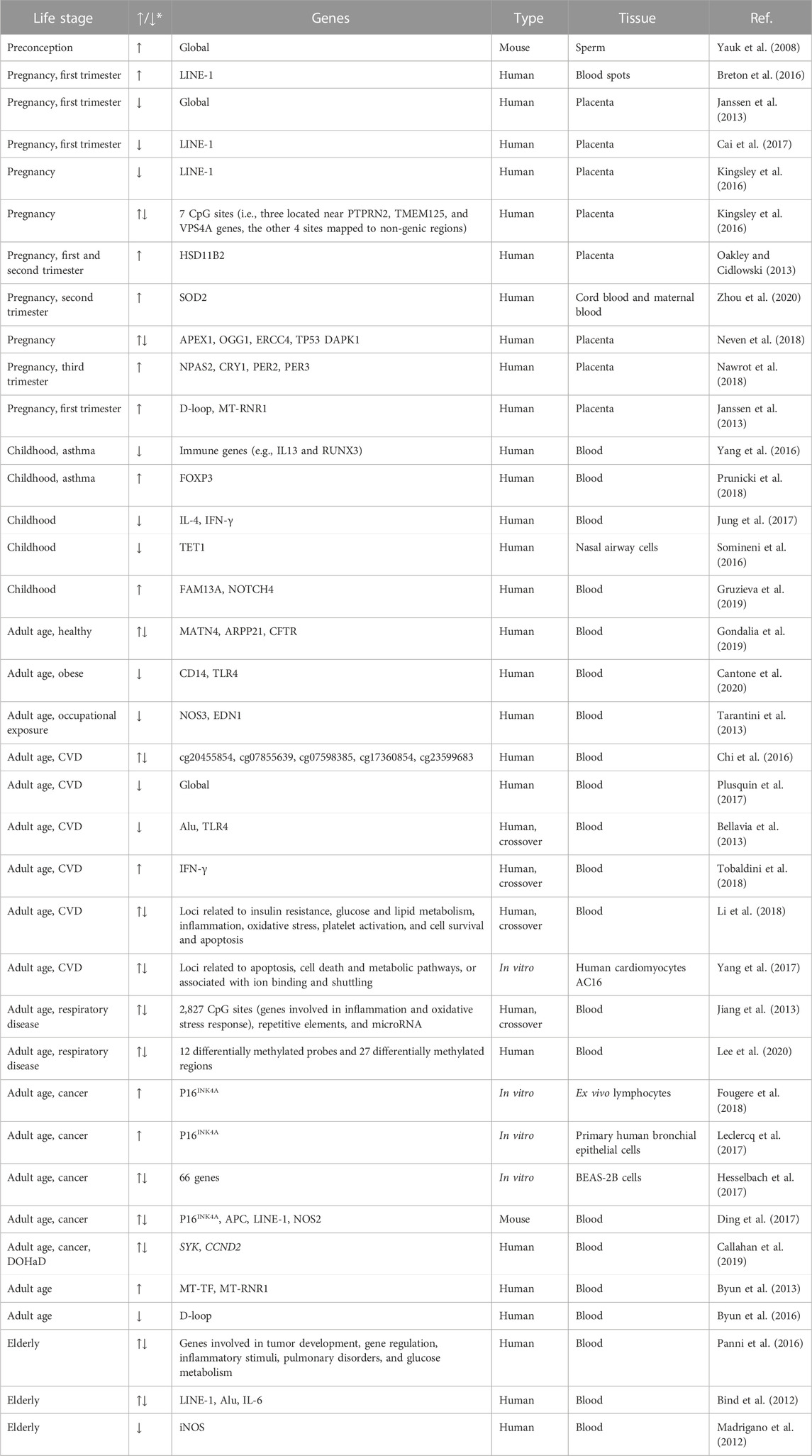
TABLE 1. Effects on methylation changes from pollution exposure at different stages of lifespan. Adapted from Ferrari et al. (2019).
Some of the contemporary excitement in epigenetics involves epigenetic clocks. These clocks measure DNA methylation sites to correlate a “biological” or “phenotypic” age to the “chronological” age and are prized for their ability to measure longevity rejuvenation therapeutic effectiveness. Although the field has developed many aging clocks (Figure 3), their nuances have increased, and the causal nature of these methylation sites is still incompletely understood. Meaning, they originally sought to measure the biological age and correlate it to chronological age to determine aging trajectories. However, people have developed clocks for children, clocks for women/men exclusively, clocks for cell culture, clocks to measure physical fitness, clocks for blood, clocks for skin, and many have repurposed clocks to measure exposome-based age accelerations. These clocks will give you a snapshot of the current phenotypic age. But they will not tell you whether these methylation sites are causing a change in phenotypic age relative to chronological, or whether these sites are the result of the change. Unsurprisingly, air pollution particulate matter content, size, and location of population (high or lower pollution) have altered clock results. For example, one group sampled non-Hispanic white women aged 35–47 in the United States (n = 2,764) and found age acceleration associated with particulate matter <10 microns in diameter (PM10), with no correlation between age and NO2, and heterogeneity per cluster when measuring PM2.5 correlations (White et al., 2019b). Contrarily, the same group separated the US by multi-state regions and subdivided further by county level. They found PM2.5 to be associated with epigenetic age, while NO2 was inversely related, and PM10 had no correlation (White et al., 2019a). These studies offer intriguing findings, but also indicate the novelty of air pollution within the aging research space. Other groups have investigated the interaction of differential effects of environmental toxicants with epigenetic outcomes, such as microplastics, cadmium, and arsenic (Meakin et al., 2019; Zhang et al., 2020).
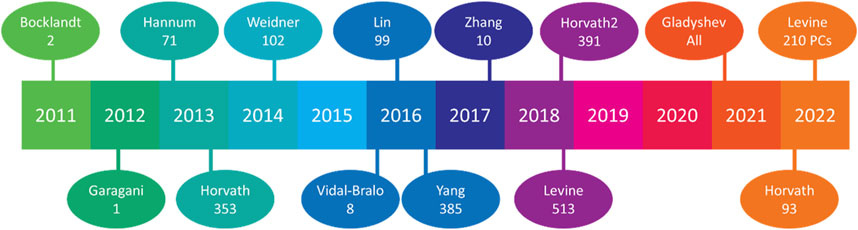
FIGURE 3. Aging clocks released by year. Since 2011, 14 aging clocks have been widely publicized, with their respective methylation sites beneath the lead author’s name. Horvath’s 2013 release featured the first multi-tissue clock, Vidal-Bralo’s utilized a validation cohort, Yang’s sought to clock CD4 monocytes, Zhang attempted to predict ACM (Arrhythmogenic cardiomyopathy), Gladyshev used all methylation sites found from scRNA-seq analysis, and Levine’s 2022 release utilized different principal components (PCs) from methylation sites rather than methylation sites themselves.
2.4 Loss of proteostasis
This hallmark describes the breakdown of protein building machinery, an accumulation of misfolded proteins, and the link to age-related diseases, like Alzheimer’s disease and related dementias (ADRD). The loss protein homeostasis has subcategories which can be divided into dysregulation/breakdown of ribosomal pathways, chaperones, and protein degradation.
Regarding ribosomes, extrachromosomal ribosomal circular DNA (ecrDNA) appears to be a key factor in the aging process (Hull and Houseley, 2020). Within yeast, ecrDNA molecules were discovered by Guarente’s group at MIT in 1997 (Sinclair and Guarente, 1997). These consisted of ribosomal DNA repeats, and titration of ecrDNA abundance was further proposed to limit the lifespan of yeast mothers. This belief is widely regarded in the literature as correct, however many studies have found no correlation with ecrDNA abundance and lifespan (Heo et al., 1999; Kim et al., 1999; Mays Hoopes et al., 2002; Merker and Klein, 2002; Borghouts et al., 2004; Kaeberlein et al., 2005; Ganley et al., 2009; Kwan et al., 2013). A second theory regarding ecrDNA maintenance has been proposed as the main driver of this yeast aging phenotype (Sinclair and Guarente, 1997). Ribosomal DNA is repeated a large number of times in most eukaryotes, with rDNA representing two of the largest repeats within humans (Warmerdam and Wolthuis, 2019). However, the nature of repeated DNA segments makes their replication more prone to errors during recombination events (Carvalho and Lupski, 2016; Warmerdam and Wolthuis, 2019), and these repeats have been shown as the most unstable region within the yeast genome (Kobayashi, 2006). Being housed in the nucleolus, double-strand breaks (DSBs) will activate special DNA repair machinery, reduce rRNA transcription, and induce nucleolar cap formation (Korsholm et al., 2019). Although these steps can be successful, recombination events often lead to copy number reductions or mutations. As previously stated in this chapter, genomic instability is linked to organismal aging, and ribosomal DNA repeats are the most susceptible to instability. Hence, the aging hallmarks of protein homeostasis and genome instability are inherently linked.
Of course, the loss of proteostasis is linked to protein synthesis as well, and aberrations in this process can increase in frequency over time (Taylor and Dillin, 2011). Protein synthesis must occur slowly enough for secondary structures to form. Without these, the proper tertiary and quaternary structures could be disallowed. Additionally, the number and type of proteins synthesized over the lifetime has been linked to aging (Selman et al., 2009), partially through results obtained from intermittent fasting which activates autophagy (Chung and Chung, 2019) and has been shown to improve proteostasis (Matai et al., 2019). If initial folding occurs correctly, and the ribosomes are operating at optimal levels, then chaperones and co-chaperones typically assist in forming the tertiary and quaternary protein structures. Co-chaperones do not directly interact with the protein folding itself but assist the chaperones in guiding proteins to their folding sites. Co-chaperones, like heat shock proteins, help chaperones during times of stress, such as anaerobic conditions, acidic conditions, and heat. It has been shown that age-related declines in co-chaperone effectiveness can lead to endoplasmic reticulum stress and chondrocyte apoptosis (Tan et al., 2020).
In addition to protein translation and folding, protein degradation processes become less efficient with age (Vernace et al., 2007). There are several aspects of protein degradation, including lysosomal, proteasomal, and autophagic, with the latter also able to degrade whole organelles (Zhao et al., 2022). Lysosomes are typically highly acidic, at a pH of 4.5, and contain digestive enzymes that optimally function at this pH (Cooper, 2000). During homeostatic processes, both aspects of acid and enzymes are used for protein/organelle degradation, surface receptor recycling, and pathogen sequestration. Ubiquitination is an additional process that targets proteins for degradation through chaperone machinery. Regardless of the molecules being degraded, the internal lysosomal contents require strict regulation to prevent spillage into the cytosol. For reasons still unknown, lysosomal permeability increases in frequency with age (Guerrero-Navarro et al., 2022).
Additionally, the field of autophagy has strong links to aging (Barbosa et al., 2019). Specifically, the ability of cells to undergo accurate and effective degradation of organelles and proteins via autophagic mechanisms declines with age. Coupled with lysosomal permeability, autophagy dysfunction can lead to the accumulation of misfolded proteins and defunct organelles. In fact, during the natural course of aging, any number of these proteostatic processes can breakdown or become less efficient, resulting in a multitude of aging-related phenotypes, including mitochondrial dysfunction and senescent cell accumulation (see below).
Regarding environmental exposures, air pollution has been shown to promote cellular oxidative stress (Lodovici and Bigagli, 2011; Gangwar et al., 2020), protein misfolding (Devi et al., 2021; 2022; Jankowska-Kieltyka et al., 2021), post-translational protein modifications (Watterson et al., 2009; Lodovici and Bigagli, 2011), and aberrant protein degradation (lysosomal (Watts et al., 2022) and autophagy (Park et al., 2018)). Many papers also point to air pollution-associated post-transcriptional modifications to RNAs (Rider and Carlsten, 2019; Gonzalez-Rivera et al., 2020), which would affect downstream protein translation. These result in any number of disease modalities, but particular attention is paid to lung fibrosis (Majewski and Piotrowski, 2020), cardiovascular disease (Gangwar et al., 2020), and ADRD (Kikis, 2020; Calderón-Garcidueñas et al., 2021).
Given that air pollution is inhaled, the initial site of oxidative stress is typically the airway and lungs. As such, the resident populations of macrophages and neutrophils are first-responders to this insult (Becker et al., 2005; Valderrama et al., 2022) and sound the alarm for a more systemic and adaptive immune response (Miyata and van Eeden, 2011). Regardless, endogenous ROS production will increase (Rao et al., 2018) which can affect proteins, and can cause neutrophils to release their cellular contents into the extracellular space—a dramatic event whose end result has been termed a neutrophil extracellular trap (NET). These NETs are known to cause additional oxidative stress and protein damage in the surrounding cells (Beavers and Skaar, 2016). As protein folding mechanisms rely on redox homeostasis (Chong et al., 2017), an abundance of ROS can cause misfolded proteins. In turn, this can cause additional ER stress and activate the unfolded protein response, resulting in additional cellular stress (Grootjans et al., 2016; Plaisance et al., 2016; Chong et al., 2017; Bhattarai et al., 2021). Additionally, protein misfolding can be recognized and sequestered for refolding by chaperones (Hervás and Oroz, 2020) or transferred to protein degradation machinery with the help of co-chaperones (Sontag et al., 2017). After exposure to air pollution, proteosomal activity is decreased (Kipen et al., 2011; Devi et al., 2021) leaving the chaperone and co-chaperone with nowhere to shuttle the misfolded protein. In some cases, the lysosomal and autophagic routes of misfolded protein degradation could be alternatively activated, and have been shown to be increased after air pollution exposure on skin fibroblasts (Park et al., 2018). Moreover, the mechanistic alterations to protein homeostasis after air pollution are coming into focus. However, the individual organ responses (e.g., heart, lung, gut, brain, etc.) could differ and their thorough investigation will eventually be required for a complete comprehension of the role of air pollution in protein homeostasis.
3 Antagonistic hallmarks of aging–mitochondrial dysfunction, deregulated nutrient sensing, and cellular senescence
3.1 Mitochondrial dysfunction
Although the “Loss of Protein Homeostasis” category does include protein degradation, the lysosomal and proteasomal machinery are linked with damage response so intimately that the aging field has designated an entire category to the mitochondrial-lysosomal axis theory of aging (Brunk and Terman, 2002). This axis of aging revolves around the idea that long-lived post-mitotic cells often exist without a stock of stem cells for replenishment. Moreover, these cells will be the most susceptible to age-related phenomena. Cell types in this category include cardiomyocytes and neurons, but any cell that cannot be replaced easily will fall under this classification. To put it lightly, these cells have problems: 1) with age, they cannot degrade protein adducts and organelles; 2) this results in an accumulation of ROS via mitochondrial production; and then 3) these cells cannot be replaced since reinforcements do not exist.
One outcome from these problems is the unintentional formation of lipofuscin through the oxidization of unsaturated fatty acids from mitochondrial ROS production, which have been called “age pigment” and are linked to aging in neurons (Gray and Woulfe, 2005), and cardiomyocytes (Terman and Brunk, 1998). Broadly, lipofuscins are yellow-brown granules that contain biproducts of lysosomal degradation. Moreover, lipofuscin granules appear to be comprised of oxidized proteins and lipids (Double et al., 2008) but can also contain sugar and metals like mercury, aluminum, iron, copper, and/or zinc. Strangely, a comprehensive understanding of lipofuscin composition, distribution, and formation is still sorely lacking. Within the brain, it appears that a decrease in the iron-storing ferritin protein can cause lipofuscin production via the inhibition of the MCOLN1 metal transport channel (König et al., 2017; Boudewyn and Walkley, 2019; Stepien et al., 2020). Although this could be caused by dysfunctions in MCOLN1 rather than a decrease in ferritin production, the end result is the same. Namely, free ferrous ions can interact with lipids and proteins within lysosomes transforming them into lipofuscin. In turn, this impairs the ability of affected lysosomes to perform normal functions and can result in multi-lysosome fusion (Milward et al., 2012).
Indeed, there are many known mitochondrial dysfunctions known to be associated with particulate matter exposure, and some speculate that the olfactory system is the causeway to deteriorating brain health (Chew et al., 2020b). Specifically, decreased mitochondrial oxygen consumption rate (Breton et al., 2019; Chew et al., 2020b), increased mtDNA oxidization (Byun et al., 2016; Grevendonk et al., 2016), altered methylation patterns (Byun et al., 2013; Breton et al., 2019), outer membrane permeabilization (Chew et al., 2020a), and fusion/fission events (Li et al., 2015). Although the large breadth of mitochondrial studies utilized in vitro methodologies, the persistence of in vivo results reveals a diverse landscape of health effects that should be heeded. A recent review (Reddam et al., 2022) attempted to subdivide the literature from 2012–2022 into chemical exposures of polycyclic aromatic hydrocarbons, air pollutants, heavy metals, endocrine-disrupting compounds, pesticides, and nanomaterials. They applied these classifications to population studies and revealed consistent mitochondrial dysfunction biomarkers, including mtDNA copy number, oxidative damage, outer membrane potential, calcium levels, and ATP levels.
3.2 Deregulated nutrient sensing
There are four key pathways affecting aging-associated nutrient sensing, with their component proteins of insulin-like growth factor 1 (IGF-1), mammalian/mechanistic target of rapamycin (mTOR), sirtuins, and AMP (adenosine monophosphate) kinase (AMPK) (López-Otín et al., 2013). Together, insulin and IGF-1 make up the insulin and insulin-like growth factor (IIS) pathway. Attenuation of this pathway appears to increase lifespan in mice, worms, and flies, which implicates the caloric restriction model of aging (López-Otín et al., 2013). When overactive, the IIS pathway can increase cancer risk, metabolic load, and cellular proliferation (Milman et al., 2016). As such, when we reduce IIS early in life, it promotes longevity. However, it appears that the body naturally reduces this pathway during aging and diminishes IIS to the point of detriment once we reach a geriatric state (Milman et al., 2016). Metformin is a drug developed for diabetic patients, which can increase insulin sensitivity (decreases blood glucose levels) and decreases glucose liver production and absorption. As a putative antiaging drug, it was discovered to reduce mitochondrial oxidative stress (partially through sirtuin 3), which increased lifespans in model organisms. Unfortunately, long-term use can lead to vitamin B12 deficiency, liver problems, lactic acidosis, and has been linked to neuropathy (Metformin, 2012; Aroda et al., 2016). The search for a silver bullet continues.
The mTORC1 and mTORC2 protein complexes result in different outcomes (Figure 4). The mTORC1 complex responds to amino acids and growth factors by signaling the cell to grow, divide, synthesize proteins, and other inflammation-inducing cellular processes. These processes have been linked to senescent cell accumulation based on cellular division leading to a Hayflick limit, stem cell exhaustion, and accumulation of errors during DNA replication. The mTORC1 complex activation has also been shown to halt autophagy, which is likely an on/off gate that is used to determine which energy source best suits the cellular needs (Rabanal-Ruiz et al., 2017). Namely, if there are nutrients in the environment, then we should not cannibalize excess organelles and lipids via autophagy as an energy source.
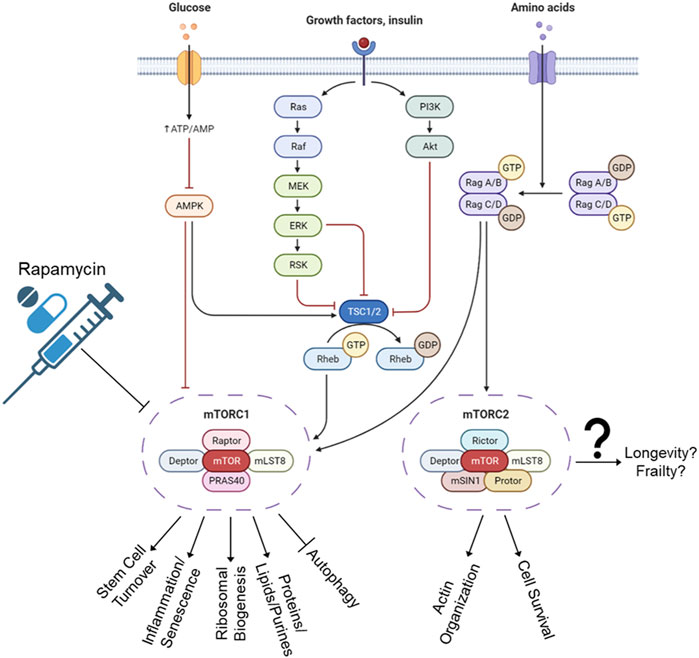
FIGURE 4. mTORC1 and mTORC2 pathways. The C1 complex regulates cellular processes such as growth, division, and protein synthesis. This activation has been linked to senescent cell accumulation and a decrease in lifespan. Autophagy, a process of energy source selection, is also impacted by C1 activation. The effects of C2 complex are less clear and still debated in the scientific community, with some studies showing it promoting lifespan and others reducing it. The outcomes of C2 can also vary depending on its regulation of factors such as FOXO3a and mTORC2. Rapamycin was developed for immunosuppressive properties and was found to extend lifespan through blocking of mTORC1 complex. Unfortunately, long-term use also inactivates mTORC2, increases blood pressure, elevates cholesterol, and increases risk of blood clots, stroke, and pulmonary embolisms.
The current understanding of mTORC2 complex outcomes will be a shorter list than mTORC1, and whether mTORC2 promotes or reduces lifespan is still debated. Some studies say that mTORC2 also blocks autophagy (Ballesteros-Álvarez and Andersen, 2021), which decreases lifespan. Some publications say it blocks FOXO3a, thereby decreasing proliferation and increasing lifespan (Johnson et al., 2013). While other studies have shown that FOXO3a downregulation leads to age-associated pathologies (Akasaki et al., 2014). Confounding still, murine hypothalamic mTORC2 was shown to increase with age, which prevented obesity, frailty, inactivity, and age (Ballesteros-Álvarez and Andersen, 2021).
Regarding drug interventions, rapamycin was originally discovered on Rapa Nui (Easter Island), and developed initially as an antifungal (Vézina et al., 1975). Later, it was developed for its immunosuppressive—and hence, organ rejection suppression—abilities (Baroja-Mazo et al., 2016). It was later discovered that rapamycin extended lifespans in model organisms, and humans jumped at the opportunity to fill their bodies with the unknown. Unfortunately, long-term rapamycin will also inactivate mTORC2 (Sarbassov et al., 2006). It also could increase arterial pressure, elevate cholesterol and/or other lipids (Sacks, 1999; Kumar et al., 2019), and does increase risk of thrombosis, venous thromboembolism, and clotting problems (Camici et al., 2010; Ahya et al., 2011). The search for a silver bullet continues.
Sirtuins are a particularly fascinating category of enzymes, which also have a deep connection with aging. Originally discovered in yeast, these are currently classified as Class III histone deacetylases (HDACs): a group of histone deacetylases that have the ability to remove acetyl groups from histones, proteins, and transcription factors in an NAD+-dependent manner (Braunstein et al., 1993). However, sirtuin functions have been expanded to NAD+-dependent mono-ADP ribosyltransferases, lipoamidase, desuccinylase, demalonylase, and deacylase activity (Chang et al., 2020). The HDAC classification comes under further fire based on publications showing that sirtuins can remove moieties other than acetyl groups, and that their catalytic activity increases proportionally to the size of aliphatic tail being removed (Gertler and Cohen, 2013). Meaning, some sirtuins will have a preference for butyryl, palmitoyl, and myristoyl over acetyl groups. As such, Jiang et al. (2013), are proposing to rename the sirtuins to deacylases. Arguably, this recategorization would be misleading, as not all sirtuins have deacylase ability. To authentically recategorize sirtuins, it would be more reasonable to categorize sirtuins based on cellular compartment localization, or we could recategorize sirtuins by their individual functions. Regardless, each sirtuin will be partially explained below and their known functions are summarized in Chang et al. (2020).
Unfortunately, the expression levels and effect sizes of sirtuins varies wildly in our current model organisms (López-Otín et al., 2013), so we will be forced to focus on the most important animal. Humans have 7 sirtuins (named SIRT1-SIRT7). In young and middle-aged humans, low cellular energy reserves cause an increase in NAD+ levels, a mechanism which is impaired in older adults (McReynolds et al., 2020). Sirtuins sense this increase, and some can control catabolic metabolism. More specifically, SIRTs 1 and 2 can translocate between cytoplasm and nucleus; SIRTs 6 and 7 are said to exclusively reside in the nucleus; and SIRTs 3, 4, and 5 localize to the mitochondria (Liszt et al., 2005; Ahuja et al., 2007; Buler et al., 2016). Notably, SIRT2 is somewhat of a nomadic enzyme, able to translocate between the cytoplasm, nucleus, and mitochondria, but is most often found in the cytoplasmic compartment (Liu et al., 2017). All SIRTs have deacetylase abilities and others, but SIRT 4 and 6 are the ADP-ribosyltransferases (Liszt et al., 2005; Ahuja et al., 2007; Buler et al., 2016).
SIRT1 is an ortholog of the gene for SIRT2, and is involved in neural development through axonal elongation, neurite growth, and dendrite branching; synaptic plasticity for memory formation; hypothalamic functions affecting feeding behaviors, endocrine functions, and circadian rhythm; protects against Alzheimer’s, Parkinson’s, and motor neuron disease; and confers stress resistance, metabolic homeostasis, and genomic stability (Herskovits and Guarente, 2014). SIRT1 has secondary activation effects, including activation of PARP for DNA repair, TFAM for mitochondrial biogenesis, FOXO3a for antioxidant effects, and NRF2 which blocks NFκB and activates SOD for downstream secondary activation of antioxidant genes.
With the nomadic behavior of SIRT2, the ability to perform intercompartmental tasks presents itself. Specifically, SIRT2 is associated with senescence (Anwar et al., 2016), cytoskeletal stabilization (North et al., 2003; Harting and Knöll, 2010; Maxwell et al., 2011; Silva et al., 2017; Esteves et al., 2019), myelin formation (Beirowski et al., 2011), oligodendrocyte differentiation (Tang and Chua, 2008), autophagy (Gal et al., 2012; Inoue et al., 2014; Liu et al., 2017; Silva et al., 2017; Esteves et al., 2019), inflammation (Mendes et al., 2017), upregulation during G2/M mitotic transition (Sola-Sevilla et al., 2021), and is required for proper cytokinesis (Tang and Chua, 2008). Among the sirtuins, SIRT2 has the strongest neurological presence, with expression seen in neurons, oligodendrocytes, astrocytes, and microglia (Jayasena et al., 2016). Based on the neurological potential, autophagy and senescence implications, and wide localization for a multitude of histone deacetylations, SIRT2 has been proposed as central to human aging. Unfortunately, the increase/decrease in SIRT2 abundance within different brain compartments is riddled with conflicting studies (Sola-Sevilla et al., 2021). Namely, three splice variants have been discovered, but mRNA and proteins levels are seen to increase, decrease, or stay the same with age (Maxwell et al., 2011; Kireev et al., 2013; Braidy et al., 2015; Garg et al., 2017; Diaz-Perdigon et al., 2020). When examining the breadth of data available, a clearer picture can be resolved after removing conflicting data in rats and mice. In humans, the studies show an age-related increase in plasma SIRT2 mRNA (Yudoh et al., 2015), with a decrease in peripheral blood mononuclear cell (PBMC) protein abundance (Wongchitrat et al., 2019). Unfortunately, the neurological implications remain unclear, and this does not speak to regional abundances.
Pharmacologically, few studies have upregulated SIRT1 or SIRT2 after air pollution exposure, but some have performed correlation studies with SIRTs and air pollution. A longitudinal study from China saw a significant correlation with hazard ratios from particulate matter exposure and levels of SIRT1 polymorphisms (Yao et al., 2021). Another study revealed pre-conception air pollution exposure increased SIRT1 and SIRT2 mRNA in offspring after birth. These data indicate a potential linkage in anti-oxidant effects of these sirtuins, or the epigenetic modification capabilities (Tanwar et al., 2018).
SIRT3 is one of the 3 mitochondrial sirtuins and has been implicated in neurological and cardiovascular diseases (Silaghi et al., 2021) as well as having protective effects after urban air pollution exposure (Chen et al., 2016). These findings are largely based on the age-related decline in activity leading to increased susceptibility of endothelial dysfunction, hypertension, and heart failure. Furthermore, proteomic data shows that it regulates 100s of proteins (Rardin et al., 2013; Yang et al., 2016; Carrico et al., 2018), involving β-oxidation, antioxidant proteins, glutathione production, metabolism of amino acids, and mitochondrial permeability (Hazelton et al., 2009; Cheng et al., 2016). As the primary mitochondrial deacetylase, it removes the acyl group from most mitochondrial proteins. In fact, it is the largest contributor to the mitochondrial “acetylome”, whereby deletion of SIRT3 (but not 4 or 5) will result in protein hyperacetylation (Lombard et al., 2007). In humans, polymorphisms that upregulate SIRT3 increased longevity (Albani et al., 2014), and increased expression in populations have been shown to extend human lifespan (Rose et al., 2003; Halaschek-Wiener et al., 2009). Based on its antioxidant effects, a clear link to aging can be seen. Through the complexing of SIRT3 with FOXO3a, SOD2 upregulations occur, which reduce ROS (Silaghi et al., 2021). But, if SIRT3 is exclusively found in the mitochondria, and the SOD gene is found in the nucleus, a question can be proposed of whether SIRT3 has a nomadic tendency as well. Independently of these musings, SIRT3 decreases mitochondrial membrane potential and ROS production while increasing cellular respiration. Conversely, downregulation of SIRT3 was shown to induce TGF-β synthesis, resulting in activation of NFATc and beta-catenin (pro-fibrosis). This mechanism is largely known, whereby SIRT3 indirectly downregulates TGF-β by inhibiting the transcription factor c-Jun (Sundaresan et al., 2015). Within the heart, TGF-β differentiates cardiac fibroblasts to myofibroblasts for the deposition of extracellular matrix, and the formation of fibrotic tissue. Indeed, patients with end-stage heart failure showed a significant decrease in SIRT3, resulting in an increase of TGF-β production (Sundaresan et al., 2015). Furthermore, hyperlipidemia has been shown to induce macrophage-based atherosclerosis. These monocytes downregulate SIRT3, leading to overabundance of acetylated ATG5 which decreases autophagic processes. In turn, this increases NLRP3 inflammasome production, and IL-1β secretion for plaque formation. Taken together, SIRT3 is an exciting target for therapeutic intervention, but the sheer number of outcomes associated with SIRT3 requires careful investigation.
Although we know that SIRT4 is localized within the mitochondria, its activities are still somewhat mysterious (Min et al., 2019). This can partially be explained by the tissue- and cell-specific activities already observed. For example, pancreatic SIRT4 has been shown to inhibit insulin secretion. Within the muscles, adipose, and liver, it suppresses fatty acid oxidization. However, the oxidization mechanism appears to be different from tissue to tissue as well. In muscles and fat, SIRT4 appears to block the malonyl-CoA decarboxylase, resulting in fatty acid synthesis rather than oxidation (Nasrin et al., 2010; Acs et al., 2014; Zeng et al., 2018). In the liver, SIRT4 has been shown to block the interaction between SIRT1 and PPARα, leading to a decrease in fatty acid oxidization (Nasrin et al., 2010). Unfortunately, these studies were performed in mice, which disallows human pathway translations or conclusions.
The first review of SIRT5 came out in 2016 (Yang et al., 2017) after a string of discoveries showed weak deacetylase activity, but strong desuccinylase, demalonylase and deglutarylase activities (Du et al., 2011), among others. After preliminary links were formed between SIRT5 abundance and incidence of Alzheimer’s, cancer, and Parkinson’s disease, the door of excitement blew open for a rash of new peptide and small-molecule inhibitors. Unlike every other SIRT, SIRT5 activation and overexpression was initially believed to be deleterious. Studies showed that overexpression led to accelerated aging and damage, rather than increasing longevity. For example, SIRT5 was amplified by 30% in ovarian carcinoma (Bell et al., 2011), was overexpressed in non-small cell lung cancer (Lu et al., 2014), and protein levels were shown to increase in tandem with Alzheimer’s progression (Lutz et al., 2014). Furthermore, a SNP in SIRT5 increased protein abundance, and appeared to contribute to mitochondrial dysfunction. The authors related these changes to Parkinson’s and other age-associated disease states through gene activation profiles (Glorioso et al., 2011). However, more recent studies have found counter evidence showing that SIRT5 depletion causes mitochondrial dysfunction, expression does not increase with Alzheimer’s progression, and can only be linked to Parkinson’s via an autophagic degradation of SIRT5 itself (Baeken et al., 2021; Haschler et al., 2021; Wu et al., 2021). Arguably, a beneficial effect of SIRT5 seems more likely based on the positive effects of SIRTs 1, 2, 3, 4, 6, and 7.
But rather than attempting to fully understand the functionality of SIRT5, the field is sprinting ahead with inhibition strategies in mice through small molecules and peptides. As of 2017, there were 6 peptide inhibitors, and 7 small molecule inhibitors. Through genetic engineering, a SIRT5 knockout group showed an increase in lifespan and decrease in tumor incidence within a mouse breast cancer model (Abril et al., 2021). However, these findings of SIRT5 knockouts and deleterious outcomes should be taken with a huge grain of salt until studies are repeated and mechanisms of action are elucidated.
Unlike SIRT5, the field believes SIRT6 to have antitumor properties through its roles in regulating gene expression, metabolism, and DNA repair. However, these effects can be lost after air pollution exposure, evidenced by an in vivo inhibition of SIRT6 mRNA in the lungs (Ribeiro et al., 2017) As stated earlier, SIRT 6 is proposed as a permanent resident of the nucleus. One outcome is the potential to directly impact DNA repair. This is achieved through ADP-ribosylation of K521 residue on PARP1, which engages the dsDNA break repair (Mao et al., 2011). Further, SIRT6 has a high binding affinity for NAD+, which allowed a systematic evaluation of binding partners. Results from these experiments include the deacetylation of H3K9 and H3K56 in gene promoter sites (Kugel and Mostoslavsky, 2014), co-repression of nuclear factor κB (NFκB) and hypoxia-inducible factor 1α (HIF-1α) (Kawahara et al., 2009; Zhong et al., 2010), telomere maintenance (Michishita et al., 2008), and co-repression of transcription factor c-MYC (Sebastián et al., 2012), among many others. Interestingly, the diacylation activity of SIRT6 is wide-ranging as well, and includes modifying the inflammatory master regulator TNF-α (Jiang et al., 2013). More specifically, the secretion of TNF-α requires SIRT6 to remove the fatty acyl groups from K19 and K20 (Jiang et al., 2013). Without this diacylation, TNF-α is relegated to the lysosomes for degradation. More recently, SIRT6 has been shown to deacylate R-Ras2, which prevents the complexing to PI3K and thereby inhibits proliferation (Zhang et al., 2017). Thus, SIRT6 has a plethora of therapeutic potential, but this widespread activity also increases the potential for off-target effects through pharmacological modulation (Chang et al., 2020). As an opportunity for researchers investigating mitotic bookmarks, lower levels of SIRT6 correlate with mitotic defects (Tasselli et al., 2016), indicating the potential for SIRT6 as a yet-uninvestigated mitotic bookmark.
SIRT7 is known to localize within the nucleus and nucleolus and interacts with RNA polymerase I (Lombard et al., 2007). It is currently believed that SIRT7 has a role in DNA repair, whereby it accumulates to the site of dsDNA breaks and deacetylates H3K18ac (Barber et al., 2012; Vazquez et al., 2016; Zhang et al., 2016). This results in the binding of double-strand break repair protein-1 for nonhomologous end joining. Additionally, SIRT7 has been implicated in cardiomyocyte stress resistance (Vakhrusheva et al., 2008), and reductions in liver endoplasmic reticulum stress through suppression of Myc activity (Shin et al., 2013).
Since these molecules are NAD-dependent, a small review of NAD+ should be endeavored. NAD+ is generated in vivo through the de novo pathway from amino acids, or the salvage pathway from nicotinamide. At last count on Pathbank, there are 6 main branching pathways that lead back to mitochondrial production of NAD+ via nicotinamide mononucleotide adenylyltransferase1 (NMNAT3), with further production available in the nucleus, and Golgi via NMNAT1 and NMNAT1, respectively. Increasing these branches to a point of convolution, hundreds of compounds are known to feed into the de novo and salvage pathways. From convolution to simplicity, we know that the de novo pathway is regulated by a rate-limiting enzyme, nicotinamide phosphoribosyltransferase (NAMPT). The NAMPT enzyme has its own circadian regulator complex consisting of a circadian locomotor output cycles kaput (CLOCK) and a brain and muscle aryl hydrocarbon receptor nuclear translocator-like 1 (BMAL1) (Nakagawa and Guarente, 2011; Grabowska et al., 2017). Without the proper abundance of feeder molecules, circadian time of day, and amount of rate-limiting enzyme, NAD+ metabolism can be skewed. In turn, this could cause an unintended decrease in sirtuin activity.
With regard to air pollution, these essential factors may be altered, but few studies have examined the link between sirtuins two to seven with air pollution/particulate matter. As of writing, the following results were found from a PubMed query for “(‘sirtuin [x]’ OR ‘SIRT [x]’ OR ‘sirtuin-[x]’) and (‘air pollution’ OR ‘particulate matter’)” where x represents the numerical sirtuin in question. SIRT1: 28 publications; SIRT2: 4; SIRT3: 5; SIRT4: 0; SIRT5: 0; SIRT6: 1; SIRT7: 0.
Of note, a longitudinal human cohort study (n = 7,083 participants) correlated air pollution levels with all-cause mortality and revealed a significantly higher hazard ration in women carrying two SIRT1_391 alleles (Yao et al., 2021). In mice, air pollution exposure in utero caused reduced cardiac expression levels of SIRT1 and SIRT2, with physiological outcomes of adult heart failure, remodeling, and epigenetic changes. SIRT3 also appears to have a cardioprotective effect in mice based on a SIRT3 KO model compared to WT controls (Song et al., 2021), and melatonin supplementation could offset some of the cardiac fibrosis effects from PM2.5 by regulation SIR3-mediated SOD2 deacetylaton (Jiang et al., 2021). For SIRT6, young (2 months) and old animals (15 months) were exposed to diesel exhaust for 30 days. In young animals, lung SIRT6 gene expression was decreased, while protein expression increased. No effects in SIRT6 were observed in old animals (Ribeiro Júnior et al., 2019).
Finally, AMP kinase is thought to extend lifespan through regulation of metabolic homeostasis and stress resistance (Salminen and Kaarniranta, 2012). Studies have shown increased in AMPK activity stimulate the signaling pathways of FoxO, Nrf2, and SIRT1, as well as downregulation of NF-κB, which reduces inflammatory responses. Furthermore, this responsiveness and activity declines with age, allowing for chronic, unresolved, low-grade inflammation. In turn, this inflammation has been linked to senescence, metabolic disorders, and age-related diseases like cancer and Alzheimer’s.
With regard to environmental stressors, heavy metal exposure from cadmium, lead, and mercury (among others) have been implicated with metabolic syndrome (Planchart et al., 2018), possibly through an increased insulin resistance (Su et al., 2021) and islet cell damage (Chen et al., 2009). Metabolic syndrome is characterized as having a minimum of 3/5 of the following: abdominal obesity, high blood pressure, impaired fasting glucose, high triglyceride levels, and low HDL cholesterol levels. Overall, metabolic syndrome has been partially linked to accelerated cellular aging (Kong et al., 2013), but more evidence is necessary before solid conclusions to be made.
3.3 Cellular senescence
Senescence is an irreversible state of proinflammatory cell cycle arrest. During natural aging, mammals accumulate senescent cells. Upon senescing, cells alter their secretome (Coppé et al., 2008), which has been characterized as the senescence-associated secretory phenotype (SASP). These factors (like IL-6, IL-8, and TNFα) can cause paracrine senescence (Borghesan et al., 2019), which can feed forward senescent cell accumulation (Teo et al., 2019). Studies have shown SASPs to be cell-type specific (Hudgins et al., 2018; Basisty et al., 2020), species-dependent (Hudgins et al., 2018), stimulus-specific (Martínez-Zamudio et al., 2017), and age-dependent (Basisty et al., 2020), but broadly link senescence with age-associated pathologies.
Environmental stressors can promote neurological senescence, but the mechanisms underlying this relationship are unclear (Chinta et al., 2018) and the ability of post-mitotic neurons to undergo senescence is still debated (Chinta et al., 2013). However, neuronal progenitors can undergo senescence (Rachmian and Krizhanovsky, 2023), as well as oligodendrocyte progenitors (Ogrodnik et al., 2021), astrocytes (Simmnacher et al., 2020), microglia (Hu et al., 2022), and neuro-associated endothelial cells and pericytes (Yamazaki et al., 2016). Additionally, the herbicide paraquat is associated with idiopathic Parkinson’s disease, potentially through a skewing of neurological astrocytes towards the senescent phenotype (Chinta et al., 2013; Chinta et al., 2018; Scott and Williams-Gray, 2018). Within other tissues, air pollution has been shown to increase senescence in the lung (Mora and Rojas, 2013; Chang-Chien et al., 2021; Eckhardt and Wu, 2021). However, any organ susceptible to the immediate and downstream effects of particulate matter insult (Schraufnagel et al., 2019) will also have an increased risk for senescence, including eyes (Sreekumar et al., 2020), heart (Chen et al., 2022), stomach (Penfield et al., 2013), bone (Pignolo et al., 2021), mouth and jaw (Parkinson and Prime, 2022), and reproductive health (Lemaître and Gaillard, 2017). These senescent cells can contribute to pathology, meaning inhaled toxicants may indirectly or directly augment those outcomes.
4 Integrative hallmarks of aging–intercellular communication and stem cell exhaustion
4.1 Intercellular communication
Cellular communication and hormone balance alters as we age. This results in a skewing towards a more inflammatory state, resulting in osteoporosis, sarcopenia, cognitive decline, stem cell exhaustion (see below), and others (López-Otín et al., 2013). This hallmark has been modulated through dietary caloric restriction (Lee et al., 2006; Pifferi et al., 2018), parabiosis (connecting the circulation of old mice to young), and apheresis (removal of blood), but the human lifespan effects are difficult to determine (Mattison et al., 2017). Mechanistically, the increase in NFκB within the hypothalamus causes a reduction in gonadotropin-releasing hormone (GnRH), leading to some of the aforementioned effects. Additionally, SASP factors have been implicated in this breakdown of altered communication through their consistent recruitment of immune cells, lack of clearance, and proinflammatory state (Palmer et al., 2015; Childs et al., 2016; Xu et al., 2017).
Regarding environmental toxicants, a longstanding body of research has linked exposures with altered intercellular communication (Peters et al., 2021). The alterations are typically thought of in the context of SASP alterations through exposures like ozone (Feng et al., 2016), and alterations to extracellular vesicle payloads through exposures like air pollution (Bollati et al., 2015). However, environmental toxicant exposure can alter the ligandome (Tian et al., 2020), and chemical mixtures are being employed to determine receptor changes that would alter endocrine signaling (Vinggaard et al., 2021). Summarily, EVs and SASP release are factors that should be brought into context with the receptor/ligand alterations that occur from multiple environmental toxicants.
4.2 Stem cell exhaustion
Stem cell exhaustion is directly responsible for age-related problems of frailty and a weakened immune system. However, there are multiple causes of this phenotypic outcome. To this point, we have observed aging phenotypes arising from accumulation of ROS, toxic metabolites, DNA damage, epigenetic alterations, damaged proteins, and mitochondrial dysfunction. All of these can contribute to stem cell exhaustion in addition to many of the other hallmarks listed in Figure 1. For example, the pro-inflammatory cytokines released from senescent cells will suppress the proliferation of stem cells, which contributes to the age-related reductions in tissue regeneration and cellular turnover. Another aspect of exhaustion occurs from telomere shortening (Hiyama and Hiyama, 2007) which can result in a replicative senescence within the stem cell population. The existence of senescent cells within signaling distance of other stem cells will promote further reductions in stem cell activity through SASP factor release. Additionally, both white and red blood cell production occurs through the proliferative ability of stem cells. Neutrophils are short-lived and are known to return to the bone marrow niche after several hours in circulation. They are welcomed home by macrophages who cannibalize them. This triggers macrophages to express a liver x receptor (LXR), which blocks CXCL12 production in the hematopoietic bone marrow niche. Through this blockage, stem cells begin differentiation and are released as hematopoietic progenitor cells in the blood (Casanova-Acebes et al., 2013).
Over the natural course of aging, the abundance of immune cells declines. This reduction in available immune cells decreases the body’s ability to fight off pathogenic insults as we age, leading to multiple diseases. Although science has been unable to reverse this effect, many researchers are examining the blood and its contents. Seminal work from the R.A. Lambert in 1911 revealed animals could have their circulatory systems surgically conjoined (parabiosis) (Lambert, 1911). For over a half-century, parabiosis studies were performed based on age-matched exposure and control animals (Binhammer et al., 1953; Warren et al., 1960; Carroll and Kimeldorf, 1967; Carroll and Kimeldorf, 1969). In 1957, the circulatory systems of young and old mice were surgically integrated (heterochronic parabiosis), and an observable lifespan increase was achieved (Mccay et al., 1957; Hrůza, 1971; Ludwig and Elashoff, 1972). Frankensteinian parabiosis has been deemed immoral and unethical in humans. As such, we must examine the blood factors themselves and attempt intervention. Pioneering work by the Wyss-Coray lab illustrated beneficial neurological effects from young plasma transfusions in old mice (Villeda et al., 2014). Similarly, researchers injected a blood plasma cocktail meant to mimic the effects of parabiosis, and reverted the epigenetic age of liver by 73.4%, blood and heart by 52%, and hypothalamus by 11% (Yousef et al., 2015), and plasma dilution (rather than transfusion) has been shown to be effective in mice (Mehdipour et al., 2020; Mehdipour et al., 2021). Not wanting to wait for government approval, a group of Russian biohackers took it upon themselves to undergo the first human tests. Their results showed an improvement in liver markers, increase in naïve T-cells, and the myelocyte/lymphocyte ratios improved. Unfortunately, the sample size of 2 called these results into question until a clinical trial performed plasma dilution and achieved similar results (Kim et al., 2022). Namely, reductions in DNA damage marker 8-OHdG and cellular senescence marker p16 in PBMCs, and a rejuvenation of the myelocyte/lymphocyte ratio. Finally, proteomic comparative analyses revealed the potential aging biomarkers of uPAR, TRAIL R1, IL-16, TIMP-1, IL-15R alpha, CD27, APJ, TNFRSF27, CCL25, and TGFBR2.
Regarding air pollution, blood is the highway between organ systems, and is the current best guess as to why non-outward-facing organs are affected after exposure. Ongoing research is attempting to determine whether inhaled smoke particles themselves are entering the blood stream, or if fragmented peptides from lung responses are causing peripheral organ effects. Later this year, Andrew Ottens at Virginia Commonwealth will be publishing proteomics results from mouse hippocampi after wildfire exposure. He was unable to directly determine whether the wildfire particles were reaching the brain. However, pathway analysis revealed neurological damage and aggrephagy–a process of selective autophagic degradation of protein aggregates. Although these results do not preclude particulate matter escaping lungs and entering circulation, this is strong evidence that fragmented peptides are reaching the brain and being cleared.
Another study showed that firefighters who donated blood reduced the circulating levels of “forever chemicals” like perfluoroalkyl and polyfluoroalkyl substances (Gasiorowski et al., 2022). Somewhat gloomily, EPA scientists now say that it is no longer safe to drink rainwater anywhere on the face of this planet due to the presence of these forever chemicals (Cousins et al., 2022). So, the take-away could be to donate more blood, but then the recipient of that blood will receive those chemicals. Broadly, it could be assumed that the biohackers’ results were due to a similar reduction in harmful circulating substances. Regardless, both studies could reduce the amount of stem cell exhaustion by way of removing inflammatory markers that prevent stem cell functions from occurring.
5 The interaction between wildfire smoke and the hallmarks of aging
Environmental disasters such as wildfires pose an increasing threat to a growing and aging global population (WHO, 2017). Wildfires produce complex mixtures of inhaled toxicants that can not only damage the lungs but also promote systemic health effects. Short-term pulmonary, cardiovascular and neurological outcomes from inhaled toxicants are well studied, as are some of the implications for chronic diseases such as atherosclerosis. However, relatively little is known about the impact of inhaled toxicants from wildfires on aging—and the further implications for priming age-related disease sequelae, particularly in the brain.
Striking parallels exist between molecular changes within the blood induced by aging and inhaled toxicants. Circulating proteins, such as MMPs, serpins, and inflammatory factors, have been documented to increase with aging and directly promote age-related neurological pathologies (Lehallier et al., 2019; Pluvinage and Wyss-Coray, 2020). Similarly, we have documented that inhaled pollutants (ozone, particulates, complex emissions) cause overlapping compositional and functional alterations to the circulation, and those changes promote vascular and neurological inflammation, which in turn prime age-related pathologies (Channell et al., 2012; Aragon et al., 2017; Mostovenko et al., 2019). Downstream consequences of the circulatory changes following inhalation of particulates and gases include breakdown of the blood-brain barrier (BBB) and activation of microglia and astrocytes (Scieszka et al., 2022). Recent studies of aging note a clear role for vascular cell adhesion molecule-1 (VCAM-1) as a vital intermediate in aging-related BBB breakdown and neurological sequelae (Yousef et al., 2019), and serum obtained after exposure to particulates and gases (in both humans and animals) directly upregulate endothelial VCAM-1 (Channell et al., 2012; Aragon et al., 2016; Tyler et al., 2016). Furthermore, while age-related changes in the circulating proteome provide fundamental revelations, there is another unstudied set of bioactive factors induced within the blood - the peptidomic fraction. We have demonstrated how circulating peptide fragments arise from activated proteases in the lung following inhalation of particulate and gaseous toxicants, retain 2° structure and act through ligand-receptor endothelial interactions to compromise BBB integrity and promote inflammation (Mostovenko et al., 2019). Our laboratory and others have demonstrated that advanced age confers vulnerability to many of the neuroinflammatory and vascular outcomes of inhaled toxicants (Mumaw et al., 2016; Tyler et al., 2018). However, gaps remain in our understanding of the underlying mechanisms of aging and environmental interactions that compromise the neurological and cardiovascular healthspan. Furthermore, how environmental exposures promote aging processes remains understudied, especially in terms of high-concentration insults as seen in wildfire scenarios. Preliminary evidence shows that inhaled wildfire smoke (WFS) accelerate markers of and neurological aging (Scieszka et al., 2022) and reduce learning capability (Cleland et al., 2022). This is likely occurring through the augmentation of circulatory factors that 1) compromise vascular and BBB integrity to induce chronic neuroinflammation and age-associated proteinopathy-related outcomes and 2) induce a reduced metabolic, senescent cellular phenotype that may be treatable with combined anti-inflammatory and NAD + boosting compounds.
6 Discussion
The intricate interplay between aging and environmental exposures, as explored in this review, underscores the need for future research on multiple fronts. A critical area of inquiry lies in the further examination of the molecular and cellular mechanisms underpinning the interactions between aging hallmarks and environmental stressors, e.g., inhaled toxicants from wildfires. Although the repercussions on circulatory alterations, neuroinflammation, and BBB integrity have been established and are related to ADRD, specific pollutant effects on cellular/molecular aging pathways involved remain largely elusive. Therefore, uncovering these could lead to targeted interventions and potential therapeutic approaches.
One such prospect is the exploration of specific compounds that could counter the adverse effects induced by wildfire smoke and other inhaled pollutants. In this context, our preliminary findings point towards the potential of combined anti-inflammatory and NAD + boosting compounds. These could act by mitigating inflammation and restoring the reduced metabolic activity in senescent cells induced by wildfire smoke. Therefore, rigorous testing of these compounds in preclinical and clinical settings could help establish their therapeutic efficacy and safety.
Moreover, the intriguing concept of circulating peptide fragments arising from activated proteases in the lung and their subsequent role in promoting inflammation and BBB compromise deserves more in-depth investigation. This could entail studying the peptide fragments’ structure, their interactions with endothelial receptors, and their potential modulation to mitigate their harmful effects.
Beyond mechanistic inquiries, our findings also call for policy action. Given the growing and aging global population and the increasing prevalence of wildfires and other environmental disasters, addressing the health implications of environmental exposures is a public health imperative. Regulatory bodies should consider implementing stringent measures to manage these disasters and reduce public exposure to their harmful consequences. Furthermore, health education campaigns can raise awareness about these health risks and strategies to minimize exposure.
Finally, the role of lifestyle interventions, such as dietary caloric restriction, in modulating the aging hallmarks affected by environmental exposures, warrants further exploration. Long-term, population-level studies could provide valuable insights into their potential benefits.
In conclusion, our review highlights the need for a multidisciplinary approach to tackling the complex problem of environmental exposure-induced acceleration of aging mechanisms. Through concerted efforts spanning basic research, therapeutic development, and policy action, we can aspire to mitigate the detrimental health consequences of such exposures and ensure healthy aging in our society.
7 Caveats
While striving to be comprehensive through literature searches, this review is not intended as a systematic review and has likely overlooked some information that could enrich the discussion. In focusing primarily on woodsmoke, we acknowledge that many emerging environmental toxicants warrant their own reviews. This targeted scope allowed a deeper exploration of woodsmoke’s impacts but means the effects of other pollutants require additional attention in future work. Overall, we aimed to advance the conversation on woodsmoke’s effects while recognizing this review’s limitations in breadth. Further research across the spectrum of environmental exposures will continue illuminating their influences on human and ecological health.
Author contributions
DS: Writing–original draft, Writing–review and editing. AB: Writing–review and editing. MM: Writing–review and editing. JB: Writing–review and editing. MC: Writing–review and editing.
Funding
The authors declare financial support was received for the research, authorship, and/or publication of this article. National Institutes of Health/National Institute of Aging R01 AG070776.
Conflict of interest
The authors declare that the research was conducted in the absence of any commercial or financial relationships that could be construed as a potential conflict of interest.
The authors declared that they were an editorial board member of Frontiers, at the time of submission. This had no impact on the peer review process and the final decision.
Publisher’s note
All claims expressed in this article are solely those of the authors and do not necessarily represent those of their affiliated organizations, or those of the publisher, the editors and the reviewers. Any product that may be evaluated in this article, or claim that may be made by its manufacturer, is not guaranteed or endorsed by the publisher.
References
Abril, Y. L. N., Fernandez, I. R., Hong, J. Y., Chiang, Y.-L., Kutateladze, D. A., Zhao, Q., et al. (2021). Pharmacological and genetic perturbation establish SIRT5 as a promising target in breast cancer. Oncogene 40, 1644–1658. doi:10.1038/s41388-020-01637-w
Acs, Z., Bori, Z., Takeda, M., Osvath, P., Berkes, I., Taylor, A. W., et al. (2014). High altitude exposure alters gene expression levels of DNA repair enzymes, and modulates fatty acid metabolism by SIRT4 induction in human skeletal muscle. Respir. Physiol. Neurobiol. 196, 33–37. doi:10.1016/j.resp.2014.02.006
Ahuja, N., Schwer, B., Carobbio, S., Waltregny, D., North, B. J., Castronovo, V., et al. (2007). Regulation of insulin secretion by SIRT4, a mitochondrial ADP-ribosyltransferase. J. Biol. Chem. 282, 33583–33592. doi:10.1074/jbc.M705488200
Ahya, V. N., McShane, P. J., Baz, M. A., Valentine, V. G., Arcasoy, S. M., Love, R. B., et al. (2011). Increased risk of venous thromboembolism with a sirolimus-based immunosuppression regimen in lung transplantation. J. Heart Lung Transpl. Off. Publ. Int. Soc. Heart Transpl. 30, 175–181. doi:10.1016/j.healun.2010.08.010
Akasaki, Y., Alvarez-Garcia, O., Saito, M., Caramés, B., Iwamoto, Y., and Lotz, M. K. (2014). FoxO transcription factors support oxidative stress resistance in human chondrocytes. Arthritis Rheumatol. Hob. N. J. 66, 3349–3358. doi:10.1002/art.38868
Al-Turki, T. M., and Griffith, J. D. (2023). Mammalian telomeric RNA (TERRA) can be translated to produce valine–arginine and glycine–leucine dipeptide repeat proteins. Proc. Natl. Acad. Sci. 120, e2221529120. doi:10.1073/pnas.2221529120
Albani, D., Ateri, E., Mazzuco, S., Ghilardi, A., Rodilossi, S., Biella, G., et al. (2014). Modulation of human longevity by SIRT3 single nucleotide polymorphisms in the prospective study Treviso Longeva (TRELONG). Age Dordr. Neth. 36, 469–478. doi:10.1007/s11357-013-9559-2
Andersen, S. L., Sebastiani, P., Dworkis, D. A., Feldman, L., and Perls, T. T. (2012). Health span approximates life span among many supercentenarians: compression of morbidity at the approximate limit of life span. J. Gerontol. A. Biol. Sci. Med. Sci. 67, 395–405. doi:10.1093/gerona/glr223
Anwar, T., Khosla, S., and Ramakrishna, G. (2016). Increased expression of SIRT2 is a novel marker of cellular senescence and is dependent on wild type p53 status. Cell Cycle georget. Tex 15, 1883. doi:10.1080/15384101.2016.1189041
Aragon, M. J., Chrobak, I., Brower, J., Roldan, L., Fredenburgh, L. E., McDonald, J. D., et al. (2016). Inflammatory and vasoactive effects of serum following inhalation of varied complex mixtures. Cardiovasc. Toxicol. 16, 163–171. doi:10.1007/s12012-015-9325-z
Aragon, M. J., Topper, L., Tyler, C. R., Sanchez, B., Zychowski, K., Young, T., et al. (2017). Serum-borne bioactivity caused by pulmonary multiwalled carbon nanotubes induces neuroinflammation via blood-brain barrier impairment. Proc. Natl. Acad. Sci. U. S. A. 114, E1968. doi:10.1073/pnas.1616070114
Aristotle (2023). Aristotle’s parva naturalia. Available at: http://sqapo.com/completetext-aristotlesparvanaturalia.htm (Accessed July 25, 2023).
Aroda, V. R., Edelstein, S. L., Goldberg, R. B., Knowler, W. C., Marcovina, S. M., Orchard, T. J., et al. (2016). Long-term metformin use and vitamin B12 deficiency in the diabetes prevention program outcomes study. J. Clin. Endocrinol. Metab. 101, 1754–1761. doi:10.1210/jc.2015-3754
Ash, A. S., Kroll-Desrosiers, A. R., Hoaglin, D. C., Christensen, K., Fang, H., and Perls, T. T. (2015). Are members of long-lived families healthier than their equally long-lived peers? Evidence from the long life family study. J. Gerontol. A. Biol. Sci. Med. Sci. 70, 971–976. doi:10.1093/gerona/glv015
Baeken, M. W., Schwarz, M., Kern, A., Moosmann, B., Hajieva, P., and Behl, C. (2021). The selective degradation of sirtuins via macroautophagy in the MPP+ model of Parkinson’s disease is promoted by conserved oxidation sites. Cell Death Discov. 7, 1–11. doi:10.1038/s41420-021-00683-x
Balali-Mood, M., Naseri, K., Tahergorabi, Z., Khazdair, M. R., and Sadeghi, M. (2021). Toxic mechanisms of five heavy metals: mercury, lead, chromium, cadmium, and arsenic. Front. Pharmacol. 12, 643972. doi:10.3389/fphar.2021.643972
Ballesteros-Álvarez, J., and Andersen, J. K. (2021). mTORC2: the other mTOR in autophagy regulation. Aging Cell 20, e13431. doi:10.1111/acel.13431
Barber, M. F., Michishita-Kioi, E., Xi, Y., Tasselli, L., Kioi, M., Moqtaderi, Z., et al. (2012). SIRT7 links H3K18 deacetylation to maintenance of oncogenic transformation. Nature 487, 114–118. doi:10.1038/nature11043
Barbosa, M. C., Grosso, R. A., and Fader, C. M. (2019). Hallmarks of aging: an autophagic perspective. Front. Endocrinol. 9, 790. doi:10.3389/fendo.2018.00790
Baroja-Mazo, A., Revilla-Nuin, B., Ramírez, P., and Pons, J. A. (2016). Immunosuppressive potency of mechanistic target of rapamycin inhibitors in solid-organ transplantation. World J. Transpl. 6, 183–192. doi:10.5500/wjt.v6.i1.183
Bartke, A. (2019). Growth hormone and aging: updated review. World J. Mens. Health 37, 19–30. doi:10.5534/wjmh.180018
Basisty, N., Kale, A., Jeon, O. H., Kuehnemann, C., Payne, T., Rao, C., et al. (2020). A proteomic atlas of senescence-associated secretomes for aging biomarker development. PLoS Biol. 18, e3000599. doi:10.1371/journal.pbio.3000599
Beavers, W. N., and Skaar, E. P. (2016). Neutrophil-generated oxidative stress and protein damage in Staphylococcus aureus. Pathog. Dis. 74, ftw060. doi:10.1093/femspd/ftw060
Becker, S., Mundandhara, S., Devlin, R. B., and Madden, M. (2005). Regulation of cytokine production in human alveolar macrophages and airway epithelial cells in response to ambient air pollution particles: further mechanistic studies. Toxicol. Appl. Pharmacol. 207, 269–275. doi:10.1016/j.taap.2005.01.023
Beirowski, B., Gustin, J., Armour, S. M., Yamamoto, H., Viader, A., North, B. J., et al. (2011). Sir-two-homolog 2 (Sirt2) modulates peripheral myelination through polarity protein Par-3/atypical protein kinase C (aPKC) signaling. Proc. Natl. Acad. Sci. U. S. A. 108, E952–E961. doi:10.1073/pnas.1104969108
Bell, D., Berchuck, A., Birrer, M., Chien, J., Cramer, D. W., Dao, F., et al. (2011). Integrated genomic analyses of ovarian carcinoma. Nature 474, 609–615. doi:10.1038/nature10166
Bellavia, A., Urch, B., Speck, M., Brook, R. D., Scott, J. A., Albetti, B., et al. (2013). DNA hypomethylation, ambient particulate matter, and increased blood pressure: findings from controlled human exposure experiments. J. Am. Heart Assoc. 2 (3), e000212. doi:10.1161/JAHA.113.000212
Berdyshev, G. D., Korotaev, G. K., Boiarskikh, G. V., and Vaniushin, B. F. (1967). Nucleotide composition of DNA and RNA from somatic tissues of humpback and its changes during spawning. Biokhimiia Mosc. Russ. 32, 988–993.
Berger, S. L. (2007). The complex language of chromatin regulation during transcription. Nature 447, 407–412. doi:10.1038/nature05915
Bhattarai, K. R., Riaz, T. A., Kim, H.-R., and Chae, H.-J. (2021). The aftermath of the interplay between the endoplasmic reticulum stress response and redox signaling. Exp. Mol. Med. 53, 151–167. doi:10.1038/s12276-021-00560-8
Bin-Jumah, M. N., Nadeem, M. S., Gilani, S. J., Al-Abbasi, F. A., Ullah, I., Alzarea, S. I., et al. (2022). Genes and longevity of lifespan. Int. J. Mol. Sci. 23, 1499. doi:10.3390/ijms23031499
Bind, M. A., Baccarelli, A., Zanobetti, A., Tarantini, L., Suh, H., Vokonas, P., et al. (2012). Air pollution and markers of coagulation, inflammation, and endothelial function: associations and epigene-environment interactions in an elderly cohort. Epidemiology 23 (2), 332–340. doi:10.1097/EDE.0b013e31824523f0
Binhammer, R. T., Schneider, M., and Finerty, J. C. (1953). Time as a factor in postirradiation protection by parabiosis. Am. J. Physiol. 175, 440–442. doi:10.1152/ajplegacy.1953.175.3.440
Bollati, V., Angelici, L., Rizzo, G., Pergoli, L., Rota, F., Hoxha, M., et al. (2015). Microvesicle-associated microRNA expression is altered upon particulate matter exposure in healthy workers and in A549 cells. J. Appl. Toxicol. 35, 59–67. doi:10.1002/jat.2987
Bolt, A. M., Byrd, R. M., and Klimecki, W. T. (2010a). Autophagy is the predominant process induced by arsenite in human lymphoblastoid cell lines. Toxicol. Appl. Pharmacol. 244, 366–373. doi:10.1016/j.taap.2010.01.019
Bolt, A. M., Douglas, R. M., and Klimecki, W. T. (2010b). Arsenite exposure in human lymphoblastoid cell lines induces autophagy and coordinated induction of lysosomal genes. Toxicol. Lett. 199, 153–159. doi:10.1016/j.toxlet.2010.08.017
Bolt, A. M., and Klimecki, W. T. (2012). Autophagy in toxicology: self-consumption in times of stress and plenty. J. Appl. Toxicol. JAT 32, 465–479. doi:10.1002/jat.1787
Bolt, A. M., Zhao, F., Pacheco, S., and Klimecki, W. T. (2012). Arsenite-induced autophagy is associated with proteotoxicity in human lymphoblastoid cells. Toxicol. Appl. Pharmacol. 264, 255–261. doi:10.1016/j.taap.2012.08.006
Borghesan, M., Fafián-Labora, J., Eleftheriadou, O., Carpintero-Fernández, P., Paez-Ribes, M., Vizcay-Barrena, G., et al. (2019). Small extracellular vesicles are key regulators of non-cell autonomous intercellular communication in senescence via the interferon protein IFITM3. Cell Rep. 27, 3956–3971.e6. doi:10.1016/j.celrep.2019.05.095
Borghouts, C., Benguria, A., Wawryn, J., and Jazwinski, S. M. (2004). Rtg2 protein links metabolism and genome stability in yeast longevity. Genetics 166, 765–777. doi:10.1534/genetics.166.2.765
Boudewyn, L. C., and Walkley, S. U. (2019). Current concepts in the neuropathogenesis of mucolipidosis type IV. J. Neurochem. 148, 669–689. doi:10.1111/jnc.14462
Brady, C. A., and Attardi, L. D. (2010). p53 at a glance. J. Cell Sci. 123, 2527–2532. doi:10.1242/jcs.064501
Braidy, N., Poljak, A., Grant, R., Jayasena, T., Mansour, H., Chan-Ling, T., et al. (2015). Differential expression of sirtuins in the aging rat brain. Front. Cell. Neurosci. 9, 167. doi:10.3389/fncel.2015.00167
Braunstein, M., Rose, A. B., Holmes, S. G., Allis, C. D., and Broach, J. R. (1993). Transcriptional silencing in yeast is associated with reduced nucleosome acetylation. Genes Dev. 7, 592–604. doi:10.1101/gad.7.4.592
Breton, C. V., Gao, L., Yao, J., Siegmund, K. D., Lurmann, F., and Gilliland, F. (2016). Particulate matter, the newborn methylome, and cardio-respiratory health outcomes in childhood. Environ. Epigenetics 2, dvw005. doi:10.1093/eep/dvw005
Breton, C. V., Song, A. Y., Xiao, J., Kim, S.-J., Mehta, H. H., Wan, J., et al. (2019). Effects of air pollution on mitochondrial function, mitochondrial DNA methylation, and mitochondrial peptide expression. Mitochondrion 46, 22–29. doi:10.1016/j.mito.2019.04.001
Brunk, U. T., and Terman, A. (2002). The mitochondrial-lysosomal axis theory of aging. Eur. J. Biochem. 269, 1996–2002. doi:10.1046/j.1432-1033.2002.02869.x
Buler, M., Andersson, U., and Hakkola, J. (2016). Who watches the watchmen? Regulation of the expression and activity of sirtuins. FASEB J. Off. Publ. Fed. Am. Soc. Exp. Biol. 30, 3942–3960. doi:10.1096/fj.201600410RR
Byun, H.-M., Colicino, E., Trevisi, L., Fan, T., Christiani, D. C., and Baccarelli, A. A. (2016). Effects of air pollution and blood mitochondrial DNA methylation on markers of heart rate variability. J. Am. Heart Assoc. 5, e003218. doi:10.1161/JAHA.116.003218
Byun, H.-M., Panni, T., Motta, V., Hou, L., Nordio, F., Apostoli, P., et al. (2013). Effects of airborne pollutants on mitochondrial DNA methylation. Part. Fibre Toxicol. 10, 18. doi:10.1186/1743-8977-10-18
Cai, J., Zhao, Y., Liu, P., Xia, B., Zhu, Q., Wang, X., et al. (2017). Exposure to particulate air pollution during early pregnancy is associated with placental DNA methylation. Sci. Total Environ. 607-608, 1103–1108. doi:10.1016/j.scitotenv.2017.07.029
Calderón-Garcidueñas, L., Herrera-Soto, A., Jury, N., Maher, B. A., González-Maciel, A., Reynoso-Robles, R., et al. (2020). Reduced repressive epigenetic marks, increased DNA damage and Alzheimer’s disease hallmarks in the brain of humans and mice exposed to particulate urban air pollution. Environ. Res. 183, 109226. doi:10.1016/j.envres.2020.109226
Calderón-Garcidueñas, L., Stommel, E. W., Rajkumar, R. P., Mukherjee, P. S., and Ayala, A. (2021). Particulate air pollution and risk of neuropsychiatric outcomes. What we breathe, swallow, and put on our skin matters. Int. J. Environ. Res. Public. Health 18, 11568. doi:10.3390/ijerph182111568
Callahan, C. L., Bonner, M. R., Nie, J., Wang, Y., Tao, M.-H., Shields, P. G., et al. (2019). Active and secondhand smoke exposure throughout life and DNA methylation in breast tumors. Cancer Causes Control CCC 30, 53–62. doi:10.1007/s10552-018-1102-4
Camici, G. G., Steffel, J., Amanovic, I., Breitenstein, A., Baldinger, J., Keller, S., et al. (2010). Rapamycin promotes arterial thrombosis in vivo: implications for everolimus and zotarolimus eluting stents. Eur. Heart J. 31, 236–242. doi:10.1093/eurheartj/ehp259
Cantone, L., Tobaldini, E., Favero, C., Albetti, B., Sacco, R. M., Torgano, G., et al. (2020). Particulate air pollution, clock gene methylation, and stroke: effects on stroke severity and disability. Int. J. Mol. Sci. 21, 3090. doi:10.3390/ijms21093090
Carrico, C., Meyer, J. G., He, W., Gibson, B. W., and Verdin, E. (2018). The mitochondrial acylome emerges: proteomics, regulation by sirtuins, and metabolic and disease implications. Cell Metab. 27, 497–512. doi:10.1016/j.cmet.2018.01.016
Carroll, H. W., and Kimeldorf, D. J. (1969). Mechanisms of protection against gastrointestinal and hematopoietic radiation lethality by parabiosis. Radiat. Res. 39, 770–776.
Carroll, H. W., and Kimeldorf, D. J. (1967). Protection through parabiosis against the lethal effects of exposure to large doses of x-rays. Science 156, 954–955. doi:10.1126/science.156.3777.954
Carvalho, C. M. B., and Lupski, J. R. (2016). Mechanisms underlying structural variant formation in genomic disorders. Nat. Rev. Genet. 17, 224–238. doi:10.1038/nrg.2015.25
Casanova-Acebes, M., Pitaval, C., Weiss, L. A., Nombela-Arrieta, C., Chèvre, R., A-González, N., et al. (2013). Rhythmic modulation of the hematopoietic niche through neutrophil clearance. Cell 153, 1025–1035. doi:10.1016/j.cell.2013.04.040
Chang, A. R., Ferrer, C. M., and Mostoslavsky, R. (2020). SIRT6, a mammalian deacylase with multitasking abilities. Physiol. Rev. 100, 145–169. doi:10.1152/physrev.00030.2018
Chang-Chien, J., Huang, J.-L., Tsai, H.-J., Wang, S.-L., Kuo, M.-L., and Yao, T.-C. (2021). Particulate matter causes telomere shortening and increase in cellular senescence markers in human lung epithelial cells. Ecotoxicol. Environ. Saf. 222, 112484. doi:10.1016/j.ecoenv.2021.112484
Channell, M. M., Paffett, M. L., Devlin, R. B., Madden, M. C., and Campen, M. J. (2012). Circulating factors induce coronary endothelial cell activation following exposure to inhaled diesel exhaust and nitrogen dioxide in humans: evidence from a novel translational in vitro model. Toxicol. Sci. Off. J. Soc. Toxicol. 127, 179–186. doi:10.1093/toxsci/kfs084
Chen, I.-C., Huang, H.-H., Chen, P.-F., and Chiang, H.-C. (2016). Sirtuin 3 protects against urban particulate matter-induced autophagy in human bronchial epithelial cells. Toxicol. Sci. 152, 113–127. doi:10.1093/toxsci/kfw073
Chen, M. S., Lee, R. T., and Garbern, J. C. (2022). Senescence mechanisms and targets in the heart. Cardiovasc. Res. 118, 1173–1187. doi:10.1093/cvr/cvab161
Chen, R., Li, H., Cai, J., Wang, C., Lin, Z., Liu, C., et al. (2018). Fine particulate air pollution and the expression of microRNAs and circulating cytokines relevant to inflammation, coagulation, and vasoconstriction. Environ. Health Perspect. 126, 017007. doi:10.1289/EHP1447
Chen, Y. W., Yang, C. Y., Huang, C. F., Hung, D. Z., Leung, Y. M., and Liu, S. H. (2009). Heavy metals, islet function and diabetes development. Islets 1, 169–176. doi:10.4161/isl.1.3.9262
Cheng, A., Yang, Y., Zhou, Y., Maharana, C., Lu, D., Peng, W., et al. (2016). Mitochondrial SIRT3 mediates adaptive responses of neurons to exercise and metabolic and excitatory challenges. Cell Metab. 23, 128–142. doi:10.1016/j.cmet.2015.10.013
Chew, S., Kolosowska, N., Saveleva, L., Malm, T., and Kanninen, K. M. (2020a). Impairment of mitochondrial function by particulate matter: implications for the brain. Neurochem. Int. 135, 104694. doi:10.1016/j.neuint.2020.104694
Chew, S., Lampinen, R., Saveleva, L., Korhonen, P., Mikhailov, N., Grubman, A., et al. (2020b). Urban air particulate matter induces mitochondrial dysfunction in human olfactory mucosal cells. Part. Fibre Toxicol. 17, 18. doi:10.1186/s12989-020-00352-4
Chi, G. C., Liu, Y., MacDonald, J. W., Barr, R. G., Donohue, K. M., Hensley, M. D., et al. (2016). Long-term outdoor air pollution and DNA methylation in circulating monocytes: results from the multi-ethnic study of atherosclerosis (MESA). Environ. Health Glob. Access Sci. Source 15, 119. doi:10.1186/s12940-016-0202-4
Childs, B. G., Baker, D. J., Wijshake, T., Conover, C. A., Campisi, J., and van Deursen, J. M. (2016). Senescent intimal foam cells are deleterious at all stages of atherosclerosis. Science 354, 472–477. doi:10.1126/science.aaf6659
Chinta, S. J., Lieu, C. A., Demaria, M., Laberge, R.-M., Campisi, J., and Andersen, J. K. (2013). Environmental stress, ageing and glial cell senescence: a novel mechanistic link to Parkinson’s disease? J. Intern. Med. 273, 429–436. doi:10.1111/joim.12029
Chinta, S. J., Woods, G., Demaria, M., Rane, A., Zou, Y., McQuade, A., et al. (2018). Cellular senescence is induced by the environmental neurotoxin paraquat and contributes to neuropathology linked to Parkinson’s disease. Cell Rep. 22, 930–940. doi:10.1016/j.celrep.2017.12.092
Chong, W. C., Shastri, M. D., and Eri, R. (2017). Endoplasmic reticulum stress and oxidative stress: A vicious nexus implicated in bowel disease pathophysiology. Int. J. Mol. Sci. 18, 771. doi:10.3390/ijms18040771
Chung, K. W., and Chung, H. Y. (2019). The effects of calorie restriction on autophagy: role on aging intervention. Nutrients 11, 2923. doi:10.3390/nu11122923
Cleland, S. E., Wyatt, L. H., Wei, L., Paul, N., Serre, M. L., West, J. J., et al. (2022). Short-term exposure to wildfire smoke and PM2.5 and cognitive performance in a brain-training game: A longitudinal study of U.S. Adults. Environ. Health Perspect. 130, 67005. doi:10.1289/EHP10498
Colicino, E., Wilson, A., Frisardi, M. C., Prada, D., Power, M. C., Hoxha, M., et al. (2017). Telomere length, long-term black carbon exposure, and cognitive function in a cohort of older men: the VA normative aging study. Environ. Health Perspect. 125, 76–81. doi:10.1289/EHP241
Cooper, G. M. (2000). “Lysosomes,” in The cell: A molecular approach. 2nd edition (United States: Sinauer Associates).
Coppé, J.-P., Patil, C. K., Rodier, F., Sun, Y., Muñoz, D. P., Goldstein, J., et al. (2008). Senescence-associated secretory phenotypes reveal cell-nonautonomous functions of oncogenic RAS and the p53 tumor suppressor. PLOS Biol. 6, e301. doi:10.1371/journal.pbio.0060301
Correia, A. W., Pope, C. A., Dockery, D. W., Wang, Y., Ezzati, M., and Dominici, F. (2013). The effect of air pollution control on life expectancy in the United States: an analysis of 545 US counties for the period 2000 to 2007. Epidemiol. Camb. Mass 24, 23–31. doi:10.1097/EDE.0b013e3182770237
Cousins, I. T., Johansson, J. H., Salter, M. E., Sha, B., and Scheringer, M. (2022). Outside the safe operating space of a new planetary boundary for per- and polyfluoroalkyl substances (PFAS). Environ. Sci. Technol. 56, 11172–11179. doi:10.1021/acs.est.2c02765
Cuthbert, G. L., Daujat, S., Snowden, A. W., Erdjument-Bromage, H., Hagiwara, T., Yamada, M., et al. (2004). Histone deimination antagonizes arginine methylation. Cell 118, 545–553. doi:10.1016/j.cell.2004.08.020
de Zelicourt, A., Colcombet, J., and Hirt, H. (2016). The role of MAPK modules and ABA during abiotic stress signaling. Trends Plant Sci. 21, 677–685. doi:10.1016/j.tplants.2016.04.004
Devi, S., Chaturvedi, M., Fatima, S., and Priya, S. (2022). Environmental factors modulating protein conformations and their role in protein aggregation diseases. Toxicology 465, 153049. doi:10.1016/j.tox.2021.153049
Devi, S., Kim, J.-J., Singh, A. P., Kumar, S., Dubey, A. K., Singh, S. K., et al. (2021). Proteotoxicity: A fatal consequence of environmental pollutants-induced impairments in protein clearance machinery. J. Pers. Med. 11, 69. doi:10.3390/jpm11020069
Diaz-Perdigon, T., Belloch, F. B., Ricobaraza, A., Elboray, E. E., Suzuki, T., Tordera, R. M., et al. (2020). Early sirtuin 2 inhibition prevents age-related cognitive decline in a senescence-accelerated mouse model. Neuropsychopharmacol. Off. Publ. Am. Coll. Neuropsychopharmacol. 45, 347–357. doi:10.1038/s41386-019-0503-8
Ding, R., Jin, Y., Liu, X., Ye, H., Zhu, Z., Zhang, Y., et al. (2017). Dose- and time-effect responses of DNA methylation and histone H3K9 acetylation changes induced by traffic-related air pollution. Sci. Rep. 7, 43737. doi:10.1038/srep43737
Double, K. L., Dedov, V. N., Fedorow, H., Kettle, E., Halliday, G. M., Garner, B., et al. (2008). The comparative biology of neuromelanin and lipofuscin in the human brain. Cell. Mol. Life Sci. 65, 1669–1682. doi:10.1007/s00018-008-7581-9
Du, J., Zhou, Y., Su, X., Yu, J. J., Khan, S., Jiang, H., et al. (2011). Sirt5 is a NAD-dependent protein lysine demalonylase and desuccinylase. Science 334, 806–809. doi:10.1126/science.1207861
Durham, T., Guo, J., Cowell, W., Riley, K. W., Wang, S., Tang, D., et al. (2022). Prenatal PM2.5 exposure in relation to maternal and newborn telomere length at delivery. Toxics 10, 13. doi:10.3390/toxics10010013
Eckhardt, C. M., and Wu, H. (2021). Environmental exposures and lung aging: molecular mechanisms and implications for improving respiratory health. Curr. Environ. Health Rep. 8, 281–293. doi:10.1007/s40572-021-00328-2
Esteller, M. (2008). Epigenetics in cancer. N. Engl. J. Med. 358, 1148–1159. doi:10.1056/NEJMra072067
Esteves, A. R., Palma, A. M., Gomes, R., Santos, D., Silva, D. F., and Cardoso, S. M. (2019). Acetylation as a major determinant to microtubule-dependent autophagy: relevance to Alzheimer’s and Parkinson disease pathology. Biochim. Biophys. Acta Mol. Basis Dis. 1865, 2008–2023. doi:10.1016/j.bbadis.2018.11.014
Eze, I. C., Jeong, A., Schaffner, E., Rezwan, F. I., Ghantous, A., Foraster, M., et al. (2020). Genome-wide DNA methylation in peripheral blood and long-term exposure to source-specific transportation noise and air pollution: the SAPALDIA study. Environ. Health Perspect. 128, 67003. doi:10.1289/EHP6174
Fan, Y., Mao, R., Lv, H., Xu, J., Yan, L., Liu, Y., et al. (2011). Frequency of double minute chromosomes and combined cytogenetic abnormalities and their characteristics. J. Appl. Genet. 52, 53–59. doi:10.1007/s13353-010-0007-z
Felker, D. P., Robbins, C. E., and McCormick, M. A. (2020). Automation of C. elegans lifespan measurement. Transl. Med. Aging 4, 1–10. doi:10.1016/j.tma.2019.12.001
Feng, F., Jin, Y., Duan, L., Yan, Z., Wang, S., Li, F., et al. (2016). Regulation of ozone-induced lung inflammation by the epidermal growth factor receptor in mice. Environ. Toxicol. 31, 2016–2027. doi:10.1002/tox.22202
Feng, Z., Lin, M., and Wu, R. (2011). The regulation of aging and longevity: A new and complex role of p53. Genes Cancer 2, 443–452. doi:10.1177/1947601911410223
Ferrari, L., Carugno, M., and Bollati, V. (2019). Particulate matter exposure shapes DNA methylation through the lifespan. Clin. Epigenetics 11, 1–14. doi:10.1186/s13148-019-0726-x
Flachsbart, F., Dose, J., Gentschew, L., Geismann, C., Caliebe, A., Knecht, C., et al. (2017). Identification and characterization of two functional variants in the human longevity gene FOXO3. Nat. Commun. 8, 2063. doi:10.1038/s41467-017-02183-y
Fougere, B., Landkocz, Y., Lepers, C., Martin, P. J., Armand, L., Grossin, N., et al. (2018). Influence of aging in the modulation of epigenetic biomarkers of carcinogenesis after exposure to air pollution. Exp. Gerontol. 110, 125–132. doi:10.1016/j.exger.2018.05.018
Gal, J., Bang, Y., and Choi, H. J. (2012). SIRT2 interferes with autophagy-mediated degradation of protein aggregates in neuronal cells under proteasome inhibition. Neurochem. Int. 61, 992–1000. doi:10.1016/j.neuint.2012.07.010
Gal-Yam, E. N., Saito, Y., Egger, G., and Jones, P. A. (2008). Cancer epigenetics: modifications, screening, and therapy. Annu. Rev. Med. 59, 267–280. doi:10.1146/annurev.med.59.061606.095816
Gangwar, R. S., Bevan, G. H., Palanivel, R., Das, L., and Rajagopalan, S. (2020). Oxidative stress pathways of air pollution mediated toxicity: recent insights. Redox Biol. 34, 101545. doi:10.1016/j.redox.2020.101545
Ganley, A. R. D., Ide, S., Saka, K., and Kobayashi, T. (2009). The effect of replication initiation on gene amplification in the rDNA and its relationship to aging. Mol. Cell 35, 683–693. doi:10.1016/j.molcel.2009.07.012
Garg, G., Singh, S., Singh, A. K., and Rizvi, S. I. (2017). Antiaging effect of metformin on brain in naturally aged and accelerated senescence model of rat. Rejuvenation Res. 20, 173–182. doi:10.1089/rej.2016.1883
Gasiorowski, R., Forbes, M. K., Silver, G., Krastev, Y., Hamdorf, B., Lewis, B., et al. (2022). Effect of plasma and blood donations on levels of perfluoroalkyl and polyfluoroalkyl substances in firefighters in Australia: A randomized clinical trial. JAMA Netw. Open 5, e226257. doi:10.1001/jamanetworkopen.2022.6257
Gertler, A. A., and Cohen, H. Y. (2013). SIRT6, a protein with many faces. Biogerontology 14, 629–639. doi:10.1007/s10522-013-9478-8
Glorioso, C., Oh, S., Douillard, G. G., and Sibille, E. (2011). Brain molecular aging, promotion of neurological disease and modulation by Sirtuin5 longevity gene polymorphism. Neurobiol. Dis. 41, 279–290. doi:10.1016/j.nbd.2010.09.016
Goldberg, A. D., Allis, C. D., and Bernstein, E. (2007). Epigenetics: a landscape takes shape. Cell 128, 635–638. doi:10.1016/j.cell.2007.02.006
Gondalia, R., Baldassari, A., Holliday, K. M., Justice, A. E., Méndez-Giráldez, R., Stewart, J. D., et al. (2019). Methylome-wide association study provides evidence of particulate matter air pollution-associated DNA methylation. Environ. Int. 132, 104723. doi:10.1016/j.envint.2019.03.071
Gonzalez-Rivera, J. C., Baldridge, K. C., Wang, D. S., Patel, K., Chuvalo-Abraham, J. C. L., Hildebrandt Ruiz, L., et al. (2020). Post-transcriptional air pollution oxidation to the cholesterol biosynthesis pathway promotes pulmonary stress phenotypes. Commun. Biol. 3, 1–16. doi:10.1038/s42003-020-01118-6
Goodson, J. M., MacDonald, J. W., Bammler, T. K., Chien, W.-M., and Chin, M. T. (2019). In utero exposure to diesel exhaust is associated with alterations in neonatal cardiomyocyte transcription, DNA methylation and metabolic perturbation. Part. Fibre Toxicol. 16, 17. doi:10.1186/s12989-019-0301-9
Grabowska, W., Sikora, E., and Bielak-Zmijewska, A. (2017). Sirtuins, a promising target in slowing down the ageing process. Biogerontology 18, 447–476. doi:10.1007/s10522-017-9685-9
Gray, D. A., and Woulfe, J. (2005). Lipofuscin and aging: A matter of toxic waste. Sci. Aging Knowl. Environ. 2005, re1. doi:10.1126/sageke.2005.5.re1
Grevendonk, L., Janssen, B. G., Vanpoucke, C., Lefebvre, W., Hoxha, M., Bollati, V., et al. (2016). Mitochondrial oxidative DNA damage and exposure to particulate air pollution in mother-newborn pairs. Environ. Health 15, 10. doi:10.1186/s12940-016-0095-2
Grootjans, J., Kaser, A., Kaufman, R. J., and Blumberg, R. S. (2016). The unfolded protein response in immunity and inflammation. Nat. Rev. Immunol. 16, 469–484. doi:10.1038/nri.2016.62
Gruzieva, O., Xu, C.-J., Yousefi, P., Relton, C., Merid, S. K., Breton, C. V., et al. (2019). Prenatal particulate air pollution and DNA methylation in newborns: an epigenome-wide meta-analysis. Environ. Health Perspect. 127, 57012. doi:10.1289/EHP4522
Guerrero-Navarro, L., Jansen-Dürr, P., and Cavinato, M. (2022). Age-related lysosomal dysfunctions. Cells 11, 1977. doi:10.3390/cells11121977
Haghani, A., Morgan, T. E., Forman, H. J., and Finch, C. E. (2020). Air pollution neurotoxicity in the adult brain: emerging concepts from experimental findings. J. Alzheimers Dis. Jad. 76, 773–797. doi:10.3233/JAD-200377
Halaschek-Wiener, J., Amirabbasi-Beik, M., Monfared, N., Pieczyk, M., Sailer, C., Kollar, A., et al. (2009). Genetic variation in healthy oldest-old. PloS One 4, e6641. doi:10.1371/journal.pone.0006641
Harting, K., and Knöll, B. (2010). SIRT2-mediated protein deacetylation: an emerging key regulator in brain physiology and pathology. Eur. J. Cell Biol. 89, 262–269. doi:10.1016/j.ejcb.2009.11.006
Haschler, T. N., Horsley, H., Balys, M., Anderson, G., Taanman, J.-W., Unwin, R. J., et al. (2021). Sirtuin 5 depletion impairs mitochondrial function in human proximal tubular epithelial cells. Sci. Rep. 11, 15510. doi:10.1038/s41598-021-94185-6
Hayflick, L., and Moorhead, P. S. (1961). The serial cultivation of human diploid cell strains. Exp. Cell Res. 25, 585–621. doi:10.1016/0014-4827(61)90192-6
Hazelton, J. L., Petrasheuskaya, M., Fiskum, G., and Kristián, T. (2009). Cyclophilin D is expressed predominantly in mitochondria of gamma-aminobutyric acidergic interneurons. J. Neurosci. Res. 87, 1250–1259. doi:10.1002/jnr.21921
Heo, S. J., Tatebayashi, K., Ohsugi, I., Shimamoto, A., Furuichi, Y., and Ikeda, H. (1999). Bloom’s syndrome gene suppresses premature ageing caused by Sgs1 deficiency in yeast. Genes Cells Devoted Mol. Cell. Mech. 4, 619–625. doi:10.1046/j.1365-2443.1999.00288.x
Herskind, A. M., McGue, M., Holm, N. V., Sørensen, T. I., Harvald, B., and Vaupel, J. W. (1996). The heritability of human longevity: a population-based study of 2872 Danish twin pairs born 1870-1900. Hum. Genet. 97, 319–323. doi:10.1007/BF02185763
Herskovits, A. Z., and Guarente, L. (2014). SIRT1 in neurodevelopment and brain senescence. Neuron 81, 471–483. doi:10.1016/j.neuron.2014.01.028
Hervás, R., and Oroz, J. (2020). Mechanistic insights into the role of molecular chaperones in protein misfolding diseases: from molecular recognition to amyloid disassembly. Int. J. Mol. Sci. 21, 9186. doi:10.3390/ijms21239186
Hesselbach, K., Kim, G. J., Flemming, S., Häupl, T., Bonin, M., Dornhof, R., et al. (2017). Disease relevant modifications of the methylome and transcriptome by particulate matter (PM2.5) from biomass combustion. Epigenetics 12 (9), 779–792. doi:10.1080/15592294.2017.1356555
Hiyama, E., and Hiyama, K. (2007). Telomere and telomerase in stem cells. Br. J. Cancer 96, 1020–1024. doi:10.1038/sj.bjc.6603671
Holehan, A. M., and Merry, B. J. (1986). The experimental manipulation of ageing by diet. Biol. Rev. Camb. Philos. Soc. 61, 329–368. doi:10.1111/j.1469-185x.1986.tb00658.x
Hrůza, Z. (1971). Increase of cholesterol turnover of old rats connected by parabiosis with young rats. Exp. Gerontol. 6, 103–107. doi:10.1016/0531-5565(71)90054-4
Hsin, H., and Kenyon, C. (1999). Signals from the reproductive system regulate the lifespan of C. elegans. Nature 399, 362–366. doi:10.1038/20694
Hu, Y., Huang, Y., Xing, S., Chen, C., Shen, D., and Chen, J. (2022). Aβ promotes CD38 expression in senescent microglia in Alzheimer’s disease. Biol. Res. 55, 10. doi:10.1186/s40659-022-00379-1
Hudgins, A. D., Tazearslan, C., Tare, A., Zhu, Y., Huffman, D., and Suh, Y. (2018). Age- and tissue-specific expression of senescence biomarkers in mice. Front. Genet. 9, 59. doi:10.3389/fgene.2018.00059
Hull, R. M., and Houseley, J. (2020). The adaptive potential of circular DNA accumulation in ageing cells. Curr. Genet. 66, 889–894. doi:10.1007/s00294-020-01069-9
Hull, R. M., King, M., Pizza, G., Krueger, F., Vergara, X., and Houseley, J. (2019). Transcription-induced formation of extrachromosomal DNA during yeast ageing. PLoS Biol. 17, e3000471. doi:10.1371/journal.pbio.3000471
Inoue, T., Nakayama, Y., Li, Y., Matsumori, H., Takahashi, H., Kojima, H., et al. (2014). SIRT2 knockdown increases basal autophagy and prevents postslippage death by abnormally prolonging the mitotic arrest that is induced by microtubule inhibitors. FEBS J. 281, 2623–2637. doi:10.1111/febs.12810
Jankowska-Kieltyka, M., Roman, A., and Nalepa, I. (2021). The air we breathe: air pollution as a prevalent proinflammatory stimulus contributing to neurodegeneration. Front. Cell. Neurosci. 15, 647643. doi:10.3389/fncel.2021.647643
Janssen, B. G., Godderis, L., Pieters, N., Poels, K., Kiciński, M., Cuypers, A., et al. (2013). Placental DNA hypomethylation in association with particulate air pollution in early life. Part. Fibre Toxicol. 10, 22. doi:10.1186/1743-8977-10-22
Jaskelioff, M., Muller, F. L., Paik, J.-H., Thomas, E., Jiang, S., Adams, A. C., et al. (2011). Telomerase reactivation reverses tissue degeneration in aged telomerase-deficient mice. Nature 469, 102–106. doi:10.1038/nature09603
Jayasena, T., Poljak, A., Braidy, N., Zhong, L., Rowlands, B., Muenchhoff, J., et al. (2016). Application of targeted mass spectrometry for the quantification of sirtuins in the central nervous system. Sci. Rep. 6, 35391. doi:10.1038/srep35391
Jiang, H., Khan, S., Wang, Y., Charron, G., He, B., Sebastian, C., et al. (2013). SIRT6 regulates TNF-α secretion through hydrolysis of long-chain fatty acyl lysine. Nature 496, 110–113. doi:10.1038/nature12038
Jiang, J., Liang, S., Zhang, J., Du, Z., Xu, Q., Duan, J., et al. (2021). Melatonin ameliorates PM2.5 -induced cardiac perivascular fibrosis through regulating mitochondrial redox homeostasis. J. Pineal Res. 70, e12686. doi:10.1111/jpi.12686
Johnson, S. C., Rabinovitch, P. S., and Kaeberlein, M. (2013). mTOR is a key modulator of ageing and age-related disease. Nature 493, 338–345. doi:10.1038/nature11861
Jones, P. A., and Baylin, S. B. (2007). The epigenomics of cancer. Cell 128, 683–692. doi:10.1016/j.cell.2007.01.029
Jung, K. H., Torrone, D., Lovinsky-Desir, S., Perzanowski, M., Bautista, J., Jezioro, J. R., et al. (2017). Short-term exposure to PM2.5 and vanadium and changes in asthma gene DNA methylation and lung function decrements among urban children. Respir. Res. 18 (1), 63. doi:10.1186/s12931-017-0550-9
Kaeberlein, M., Kirkland, K. T., Fields, S., and Kennedy, B. K. (2005). Genes determining yeast replicative life span in a long-lived genetic background. Mech. Ageing Dev. 126, 491–504. doi:10.1016/j.mad.2004.10.007
Kaplanis, J., Gordon, A., Shor, T., Weissbrod, O., Geiger, D., Wahl, M., et al. (2018). Quantitative analysis of population-scale family trees with millions of relatives. Science 360, 171–175. doi:10.1126/science.aam9309
Kaur, G., Sundar, I. K., and Rahman, I. (2021). p16-3MR: A novel model to study cellular senescence in cigarette smoke-induced lung injuries. Int. J. Mol. Sci. 22, 4834. doi:10.3390/ijms22094834
Kawahara, T. L. A., Michishita, E., Adler, A. S., Damian, M., Berber, E., Lin, M., et al. (2009). SIRT6 links histone H3 lysine 9 deacetylation to NF-kappaB-dependent gene expression and organismal life span. Cell 136, 62–74. doi:10.1016/j.cell.2008.10.052
Keshavarz, M., Xie, K., Schaaf, K., Bano, D., and Ehninger, D. (2022). Targeting the “hallmarks of aging” to slow aging and treat age-related disease: fact or fiction? Mol. Psychiatry 2022, 1–14. doi:10.1038/s41380-022-01680-x
Kikis, E. A. (2020). The proteostatic effects of traffic-derived air pollution on Alzheimer’s disease risk. Open Biol. 10, 200146. doi:10.1098/rsob.200146
Kim, D., Kiprov, D. D., Luellen, C., Lieb, M., Liu, C., Watanabe, E., et al. (2022). Old plasma dilution reduces human biological age: a clinical study. GeroScience 44, 2701–2720. doi:10.1007/s11357-022-00645-w
Kim, S., Benguria, A., Lai, C.-Y., and Jazwinski, S. M. (1999). Modulation of life-span by histone deacetylase genes in Saccharomyces cerevisiae. Mol. Biol. Cell 10, 3125–3136.
Kingsley, S. L., Eliot, M. N., Whitsel, E. A., Huang, Y. T., Kelsey, K. T., Marsit, C. J., et al. (2016). Maternal residential proximity to major roadways, birth weight, and placental DNA methylation. Environ. Int. 92-93, 43–49. doi:10.1016/j.envint.2016.03.020
Kipen, H. M., Gandhi, S., Rich, D. Q., Ohman-Strickland, P., Laumbach, R., Fan, Z.-H., et al. (2011). Acute decreases in proteasome pathway activity after inhalation of fresh diesel exhaust or secondary organic aerosol. Environ. Health Perspect. 119, 658–663. doi:10.1289/ehp.1002784
Kireev, R. A., Vara, E., and Tresguerres, J. a. F. (2013). Growth hormone and melatonin prevent age-related alteration in apoptosis processes in the dentate gyrus of male rats. Biogerontology 14, 431–442. doi:10.1007/s10522-013-9443-6
Kobayashi, T. (2006). Strategies to maintain the stability of the ribosomal RNA gene repeats. Genes Genet. Syst. 81, 155–161. doi:10.1266/ggs.81.155
Kohlrausch, F. B., Berteli, T. S., Wang, F., Navarro, P. A., and Keefe, D. L. (2022). Control of LINE-1 expression maintains genome integrity in germline and early embryo development. Reprod. Sci. 29, 328–340. doi:10.1007/s43032-021-00461-1
Kong, C. M., Lee, X. W., and Wang, X. (2013). Telomere shortening in human diseases. FEBS J. 280, 3180–3193. doi:10.1111/febs.12326
König, J., Ott, C., Hugo, M., Jung, T., Bulteau, A.-L., Grune, T., et al. (2017). Mitochondrial contribution to lipofuscin formation. Redox Biol. 11, 673–681. doi:10.1016/j.redox.2017.01.017
Korsholm, L. M., Gál, Z., Lin, L., Quevedo, O., Ahmad, D. A., Dulina, E., et al. (2019). Double-strand breaks in ribosomal RNA genes activate a distinct signaling and chromatin response to facilitate nucleolar restructuring and repair. Nucleic Acids Res. 47, 8019–8035. doi:10.1093/nar/gkz518
Kugel, S., and Mostoslavsky, R. (2014). Chromatin and beyond: the multitasking roles for SIRT6. Trends biochem. Sci. 39, 72–81. doi:10.1016/j.tibs.2013.12.002
Kumar, V., Evans, L. C., Kurth, T., Yang, C., Wollner, C., Nasci, V., et al. (2019). Therapeutic suppression of mTOR (mammalian target of rapamycin) signaling prevents and reverses salt-induced hypertension and kidney injury in dahl salt-sensitive rats. Hypertension 73, 630–639. doi:10.1161/HYPERTENSIONAHA.118.12378
Kwan, E. X., Foss, E. J., Tsuchiyama, S., Alvino, G. M., Kruglyak, L., Kaeberlein, M., et al. (2013). A natural polymorphism in rDNA replication origins links origin activation with calorie restriction and lifespan. PLoS Genet. 9, e1003329. doi:10.1371/journal.pgen.1003329
Lambert, R. A. (1911). The influence of mouse-rat parabiosis on the growth in rats of A transplantable mouse sarcoma. J. Exp. Med. 13, 257–262. doi:10.1084/jem.13.2.257
Langie, S. A. S., Koppen, G., Desaulniers, D., Al-Mulla, F., Al-Temaimi, R., Amedei, A., et al. (2015). Causes of genome instability: the effect of low dose chemical exposures in modern society. Carcinogenesis 36, S61–S88. doi:10.1093/carcin/bgv031
Leclercq, B., Platel, A., Antherieu, S., Alleman, L. Y., Hardy, E. M., Perdrix, E., et al. (2017). Genetic and epigenetic alterations in normal and sensitive COPD-diseased human bronchial epithelial cells repeatedly exposed to air pollution-derived PM2.5. Environ. Pollut. Barking Essex 230, 163–177. doi:10.1016/j.envpol.2017.06.028
Lee, A. G., Cowell, W., Kannan, S., Ganguri, H. B., Nentin, F., Wilson, A., et al. (2020). Prenatal particulate air pollution and newborn telomere length: effect modification by maternal antioxidant intakes and infant sex. Environ. Res. 187, 109707. doi:10.1016/j.envres.2020.109707
Lee, G. D., Wilson, M. A., Zhu, M., Wolkow, C. A., de Cabo, R., Ingram, D. K., et al. (2006). Dietary deprivation extends lifespan in Caenorhabditis elegans. Aging Cell 5, 515–524. doi:10.1111/j.1474-9726.2006.00241.x
Lehallier, B., Gate, D., Schaum, N., Nanasi, T., Lee, S. E., Yousef, H., et al. (2019). Undulating changes in human plasma proteome profiles across the lifespan. Nat. Med. 25, 1843–1850. doi:10.1038/s41591-019-0673-2
Leibiger, I. B., and Berggren, P.-O. (2006). Sirt1: a metabolic master switch that modulates lifespan. Nat. Med. 12, 34–36. doi:10.1038/nm0106-34
Lemaître, J.-F., and Gaillard, J.-M. (2017). Reproductive senescence: new perspectives in the wild. Biol. Rev. Camb. Philos. Soc. 92, 2182–2199. doi:10.1111/brv.12328
Li, H., Chen, R., Cai, J., Cui, X., Huang, N., and Kan, H. (2018). Short-term exposure to fine particulate air pollution and genome-wide DNA methylation: A randomized, double-blind, crossover trial. Environ. Int. 120, 130–136. doi:10.1016/j.envint.2018.07.041
Li, J., Li, W. X., Bai, C., and Song, Y. (2017). Particulate matter-induced epigenetic changes and lung cancer. Clin. Respir. J. 11, 539–546. doi:10.1111/crj.12389
Li, R., Kou, X., Geng, H., Xie, J., Yang, Z., Zhang, Y., et al. (2015). Effect of ambient PM2.5 on lung mitochondrial damage and fusion/fission gene expression in rats. Chem. Res. Toxicol. 28, 408–418. doi:10.1021/tx5003723
Liang, Y., Hu, L., Li, J., Liu, F., Jones, K. C., Li, D., et al. (2021). Short-term personal PM2.5 exposure and change in DNA methylation of imprinted genes: panel study of healthy young adults in guangzhou city, China. Environ. Pollut. Barking Essex 275, 116601. doi:10.1016/j.envpol.2021.116601
Liszt, G., Ford, E., Kurtev, M., and Guarente, L. (2005). Mouse Sir2 homolog SIRT6 is a nuclear ADP-ribosyltransferase. J. Biol. Chem. 280, 21313–21320. doi:10.1074/jbc.M413296200
Liu, C., Xu, J., Chen, Y., Guo, X., Zheng, Y., Wang, Q., et al. (2015). Characterization of genome-wide H3K27ac profiles reveals a distinct PM2.5-associated histone modification signature. Environ. Health Glob. Access Sci. Source 14, 65. doi:10.1186/s12940-015-0052-5
Liu, G., Park, S.-H., Imbesi, M., Nathan, W. J., Zou, X., Zhu, Y., et al. (2017). Loss of NAD-dependent protein deacetylase sirtuin-2 alters mitochondrial protein acetylation and dysregulates mitophagy. Antioxid. Redox Signal. 26, 849–863. doi:10.1089/ars.2016.6662
Lodovici, M., and Bigagli, E. (2011). Oxidative stress and air pollution exposure. J. Toxicol. 2011, 487074. doi:10.1155/2011/487074
Lombard, D. B., Alt, F. W., Cheng, H.-L., Bunkenborg, J., Streeper, R. S., Mostoslavsky, R., et al. (2007). Mammalian Sir2 homolog SIRT3 regulates global mitochondrial lysine acetylation. Mol. Cell. Biol. 27, 8807–8814. doi:10.1128/MCB.01636-07
López-Otín, C., Blasco, M. A., Partridge, L., Serrano, M., and Kroemer, G. (2013). The hallmarks of aging. Cell 153, 1194–1217. doi:10.1016/j.cell.2013.05.039
López-Otín, C., Galluzzi, L., Freije, J. M. P., Madeo, F., and Kroemer, G. (2016). Metabolic control of longevity. Cell 166, 802–821. doi:10.1016/j.cell.2016.07.031
Lu, W., Zuo, Y., Feng, Y., and Zhang, M. (2014). SIRT5 facilitates cancer cell growth and drug resistance in non-small cell lung cancer. Tumour Biol. J. Int. Soc. Oncodevelopmental Biol. Med. 35, 10699–10705. doi:10.1007/s13277-014-2372-4
Ludwig, F. C., and Elashoff, R. M. (1972). Mortality in syngeneic rat parabionts of different chronological age*†. Trans. N. Y. Acad. Sci. 34, 582–587. doi:10.1111/j.2164-0947.1972.tb02712.x
Lutz, M. I., Milenkovic, I., Regelsberger, G., and Kovacs, G. G. (2014). Distinct patterns of sirtuin expression during progression of Alzheimer’s disease. Neuromolecular Med. 16, 405–414. doi:10.1007/s12017-014-8288-8
Ma, Y., Li, J., Xu, Y., Wang, Y., Yao, Y., Liu, Q., et al. (2020). Identification of 34 genes conferring genetic and pharmacological risk for the comorbidity of schizophrenia and smoking behaviors. Aging 12, 2169–2225. doi:10.18632/aging.102735
Madrigano, J., Baccarelli, A., Mittleman, M. A., Sparrow, D., Spiro, A., Vokonas, P. S., et al. (2012). Air pollution and DNA methylation: interaction by psychological factors in the VA Normative Aging Study. Am. J. Epidemiol. 176 (3), 224–232. doi:10.1093/aje/kwr523
Maghbooli, Z., Hossein-Nezhad, A., Adabi, E., Asadollah-Pour, E., Sadeghi, M., Mohammad-Nabi, S., et al. (2018). Air pollution during pregnancy and placental adaptation in the levels of global DNA methylation. PloS One 13, e0199772. doi:10.1371/journal.pone.0199772
Mahesh, S., and Kaskel, F. (2008). Growth hormone axis in chronic kidney disease. Pediatr. Nephrol. Berl. Ger. 23, 41–48. doi:10.1007/s00467-007-0527-x
Majewski, S., and Piotrowski, W. J. (2020). Air pollution—an overlooked risk factor for idiopathic pulmonary fibrosis. J. Clin. Med. 10, 77. doi:10.3390/jcm10010077
Mao, Z., Hine, C., Tian, X., Van Meter, M., Au, M., Vaidya, A., et al. (2011). SIRT6 promotes DNA repair under stress by activating PARP1. Science 332, 1443–1446. doi:10.1126/science.1202723
Martínez-Zamudio, R. I., Robinson, L., Roux, P.-F., and Bischof, O. (2017). SnapShot: cellular senescence pathways. Cell 170, 816–816.e1. doi:10.1016/j.cell.2017.07.049
Matai, L., Sarkar, G. C., Chamoli, M., Malik, Y., Kumar, S. S., Rautela, U., et al. (2019). Dietary restriction improves proteostasis and increases life span through endoplasmic reticulum hormesis. Proc. Natl. Acad. Sci. 116, 17383–17392. doi:10.1073/pnas.1900055116
Mattison, J. A., Colman, R. J., Beasley, T. M., Allison, D. B., Kemnitz, J. W., Roth, G. S., et al. (2017). Caloric restriction improves health and survival of rhesus monkeys. Nat. Commun. 8, 14063. doi:10.1038/ncomms14063
Maxwell, M. M., Tomkinson, E. M., Nobles, J., Wizeman, J. W., Amore, A. M., Quinti, L., et al. (2011). The Sirtuin 2 microtubule deacetylase is an abundant neuronal protein that accumulates in the aging CNS. Hum. Mol. Genet. 20, 3986–3996. doi:10.1093/hmg/ddr326
Mays Hoopes, L. L., Budd, M., Choe, W., Weitao, T., and Campbell, J. L. (2002). Mutations in DNA replication genes reduce yeast life span. Mol. Cell. Biol. 22, 4136–4146. doi:10.1128/MCB.22.12.4136-4146.2002
Mccay, C. M., Pope, F., Lunsford, W., Sperling, G., and Sambhavaphol, P. (1957). Parabiosis between old and young rats. Gerontologia 1, 7–17. doi:10.1159/000210677
McReynolds, M. R., Chellappa, K., and Baur, J. A. (2020). Age-related NAD+ decline. Exp. Gerontol. 134, 110888. doi:10.1016/j.exger.2020.110888
Meakin, C. J., Martin, E. M., Szilagyi, J. T., Nylander-French, L. A., and Fry, R. C. (2019). Inorganic arsenic as an endocrine disruptor: modulation of the glucocorticoid receptor pathway in placental cells via CpG methylation. Chem. Res. Toxicol. 32, 493–499. doi:10.1021/acs.chemrestox.8b00352
Medina, S., Bolt, A. M., Zhou, X., Wan, G., Xu, H., Lauer, F. T., et al. (2021). Arsenite and monomethylarsonous acid disrupt erythropoiesis through combined effects on differentiation and survival pathways in early erythroid progenitors. Toxicol. Lett. 350, 111–120. doi:10.1016/j.toxlet.2021.07.008
Medina, S., Lauer, F. T., Castillo, E. F., Bolt, A. M., Ali, A.-M. S., Liu, K. J., et al. (2020). Exposures to uranium and arsenic alter intraepithelial and innate immune cells in the small intestine of male and female mice. Toxicol. Appl. Pharmacol. 403, 115155. doi:10.1016/j.taap.2020.115155
Medina, S., Xu, H., Wang, S. C., Lauer, F. T., Liu, K. J., and Burchiel, S. W. (2017). Low level arsenite exposures suppress the development of bone marrow erythroid progenitors and result in anemia in adult male mice. Toxicol. Lett. 273, 106–111. doi:10.1016/j.toxlet.2017.03.021
Medina, S., Zhang, H., Santos-Medina, L. V., Wan, G., Bolt, A. M., Zhou, X., et al. (2022). Arsenic impairs the lineage commitment of hematopoietic progenitor cells through the attenuation of GATA-2 DNA binding activity. Toxicol. Appl. Pharmacol. 452, 116193. doi:10.1016/j.taap.2022.116193
Mehdipour, M., Mehdipour, T., Skinner, C. M., Wong, N., Liu, C., Chen, C.-C., et al. (2021). Plasma dilution improves cognition and attenuates neuroinflammation in old mice. GeroScience 43, 1–18. doi:10.1007/s11357-020-00297-8
Mehdipour, M., Skinner, C., Wong, N., Lieb, M., Liu, C., Etienne, J., et al. (2020). Rejuvenation of three germ layers tissues by exchanging old blood plasma with saline-albumin. Aging 12, 8790–8819. doi:10.18632/aging.103418
Mendes, K. L., Lelis, D. de F., and Santos, S. H. S. (2017). Nuclear sirtuins and inflammatory signaling pathways. Cytokine Growth Factor Rev. 38, 98–105. doi:10.1016/j.cytogfr.2017.11.001
Merker, R. J., and Klein, H. L. (2002). hpr1Δ affects ribosomal DNA recombination and cell life span in Saccharomyces cerevisiae. Mol. Cell. Biol. 22, 421–429. doi:10.1128/MCB.22.2.421-429.2002
Metformin (2012). LiverTox: Clinical and research information on drug-induced liver injury. Bethesda (MD): National Institute of Diabetes and Digestive and Kidney Diseases.
Michishita, E., McCord, R. A., Berber, E., Kioi, M., Padilla-Nash, H., Damian, M., et al. (2008). SIRT6 is a histone H3 lysine 9 deacetylase that modulates telomeric chromatin. Nature 452, 492–496. doi:10.1038/nature06736
Miller, C. N., Dye, J. A., Schladweiler, M. C., Richards, J. H., Ledbetter, A. D., Stewart, E. J., et al. (2018). Acute inhalation of ozone induces DNA methylation of apelin in lungs of Long-Evans rats. Inhal. Toxicol. 30, 178–186. doi:10.1080/08958378.2018.1483984
Milman, S., Huffman, D. M., and Barzilai, N. (2016). The somatotropic Axis in human aging: framework for the current state of knowledge and future research. Cell Metab. 23, 980–989. doi:10.1016/j.cmet.2016.05.014
Milward, E., Acikyol, B., Bassett, B., Williams, E., Graham, R., Delima, R., et al. (2012). “Brain changes in iron loading disorders,” in Metal ions in neurological systems. Editors W. Linert, and H. Kozlowski (Vienna: Springer), 17–29. doi:10.1007/978-3-7091-1001-0_2
Min, Z., Gao, J., and Yu, Y. (2019). The roles of mitochondrial SIRT4 in cellular metabolism. Front. Endocrinol. 9, 783. doi:10.3389/fendo.2018.00783
Minatel, B. C., Sage, A. P., Anderson, C., Hubaux, R., Marshall, E. A., Lam, W. L., et al. (2018). Environmental arsenic exposure: from genetic susceptibility to pathogenesis. Environ. Int. 112, 183–197. doi:10.1016/j.envint.2017.12.017
Miyata, R., and van Eeden, S. F. (2011). The innate and adaptive immune response induced by alveolar macrophages exposed to ambient particulate matter. Toxicol. Appl. Pharmacol. 257, 209–226. doi:10.1016/j.taap.2011.09.007
Møller, H. D., Mohiyuddin, M., Prada-Luengo, I., Sailani, M. R., Halling, J. F., Plomgaard, P., et al. (2018). Circular DNA elements of chromosomal origin are common in healthy human somatic tissue. Nat. Commun. 9, 1069. doi:10.1038/s41467-018-03369-8
Mora, A. L., and Rojas, M. (2013). Adult stem cells for chronic lung diseases. Respirol. Carlt. Vic. 18, 1041–1046. doi:10.1111/resp.12112
Mostovenko, E., Young, T., Muldoon, P. P., Bishop, L., Canal, C. G., Vucetic, A., et al. (2019). Nanoparticle exposure driven circulating bioactive peptidome causes systemic inflammation and vascular dysfunction. Part. Fibre Toxicol. 16, 20. doi:10.1186/s12989-019-0304-6
Motta, V., Favero, C., Dioni, L., Iodice, S., Battaglia, C., Angelici, L., et al. (2016). MicroRNAs are associated with blood-pressure effects of exposure to particulate matter: results from a mediated moderation analysis. Environ. Res. 146, 274–281. doi:10.1016/j.envres.2016.01.010
Mukherjee, S., Dasgupta, S., Mishra, P. K., and Chaudhury, K. (2021). Air pollution-induced epigenetic changes: disease development and a possible link with hypersensitivity pneumonitis. Environ. Sci. Pollut. Res. Int. 28, 55981–56002. doi:10.1007/s11356-021-16056-x
Mumaw, C. L., Levesque, S., McGraw, C., Robertson, S., Lucas, S., Stafflinger, J. E., et al. (2016). Microglial priming through the lung-brain axis: the role of air pollution-induced circulating factors. FASEB J. Off. Publ. Fed. Am. Soc. Exp. Biol. 30, 1880–1891. doi:10.1096/fj.201500047
Nakagawa, T., and Guarente, L. (2011). Sirtuins at a glance. J. Cell Sci. 124, 833–838. doi:10.1242/jcs.081067
Nasrin, N., Wu, X., Fortier, E., Feng, Y., Bare’, O. C., Chen, S., et al. (2010). SIRT4 regulates fatty acid oxidation and mitochondrial gene expression in liver and muscle cells. J. Biol. Chem. 285, 31995–32002. doi:10.1074/jbc.M110.124164
Nathan, D., Ingvarsdottir, K., Sterner, D. E., Bylebyl, G. R., Dokmanovic, M., Dorsey, J. A., et al. (2006). Histone sumoylation is a negative regulator in Saccharomyces cerevisiae and shows dynamic interplay with positive-acting histone modifications. Genes Dev. 20, 966–976. doi:10.1101/gad.1404206
Nawrot, T. S., Saenen, N. D., Schenk, J., Janssen, B. G., Motta, V., Tarantini, L., et al. (2018). Placental circadian pathway methylation and in utero exposure to fine particle air pollution. Environ. Int. 114, 231–241. doi:10.1016/j.envint.2018.02.034
NCI Dictionary of Cancer Terms - NCI (2011). Definition of genomic instability - NCI dictionary of cancer terms - NCI. Available at: https://www.cancer.gov/publications/dictionaries/cancer-terms/def/genomic-instability (Accessed February 26, 2023).
Nelson, C. J., Santos-Rosa, H., and Kouzarides, T. (2006). Proline isomerization of histone H3 regulates lysine methylation and gene expression. Cell 126, 905–916. doi:10.1016/j.cell.2006.07.026
Neumann, A. A., Watson, C. M., Noble, J. R., Pickett, H. A., Tam, P. P. L., and Reddel, R. R. (2013). Alternative lengthening of telomeres in normal mammalian somatic cells. Genes Dev. 27, 18–23. doi:10.1101/gad.205062.112
Neven, K. Y., Saenen, N. D., Tarantini, L., Janssen, B. G., Lefebvre, W., Vanpoucke, C., et al. (2018). Placental promoter methylation of DNA repair genes and prenatal exposure to particulate air pollution: an ENVIRONAGE cohort study. Lancet Planet Health 2 (4), e174–e183. doi:10.1016/S2542-5196(18)30049-4
Ng, F., Wijaya, L., and Tang, B. L. (2015). SIRT1 in the brain—Connections with aging-associated disorders and lifespan. Front. Cell. Neurosci. 9. doi:10.3389/fncel.2015.00064
North, B. J., Marshall, B. L., Borra, M. T., Denu, J. M., and Verdin, E. (2003). The human Sir2 ortholog, SIRT2, is an NAD+-dependent tubulin deacetylase. Mol. Cell 11, 437–444. doi:10.1016/s1097-2765(03)00038-8
Nowak, S. J., and Corces, V. G. (2004). Phosphorylation of histone H3: a balancing act between chromosome condensation and transcriptional activation. Trends Genet. TIG 20, 214–220. doi:10.1016/j.tig.2004.02.007
Nygaard, M., Lindahl-Jacobsen, R., Soerensen, M., Mengel-From, J., Andersen-Ranberg, K., Jeune, B., et al. (2014). Birth cohort differences in the prevalence of longevity-associated variants in APOE and FOXO3A in Danish long-lived individuals. Exp. Gerontol. 0, 41–46. doi:10.1016/j.exger.2014.04.018
Oakley, R. H., and Cidlowski, J. A. (2013). The biology of the glucocorticoid receptor: new signaling mechanisms in health and disease. J. Allergy Clin. Immunol. 132 (5), 1033–1044. doi:10.1016/j.jaci.2013.09.007
Ogrodnik, M., Evans, S. A., Fielder, E., Victorelli, S., Kruger, P., Salmonowicz, H., et al. (2021). Whole-body senescent cell clearance alleviates age-related brain inflammation and cognitive impairment in mice. Aging Cell 20, e13296. doi:10.1111/acel.13296
Oikawa, S., and Kawanishi, S. (1999). Site-specific DNA damage at GGG sequence by oxidative stress may accelerate telomere shortening. FEBS Lett. 453, 365–368. doi:10.1016/S0014-5793(99)00748-6
Olovnikov, A. M. (1973). A theory of marginotomy: the incomplete copying of template margin in enzymic synthesis of polynucleotides and biological significance of the phenomenon. J. Theor. Biol. 41, 181–190. doi:10.1016/0022-5193(73)90198-7
Palmer, A. K., Tchkonia, T., LeBrasseur, N. K., Chini, E. N., Xu, M., and Kirkland, J. L. (2015). Cellular senescence in type 2 diabetes: A therapeutic opportunity. Diabetes 64, 2289–2298. doi:10.2337/db14-1820
Panni, T., Mehta, A. J., Schwartz, J. D., Baccarelli, A. A., Just, A. C., Wolf, K., et al. (2016). Genome-wide analysis of DNA methylation and fine particulate matter air pollution in three study populations: KORA F3, KORA F4, and the Normative Aging Study. Environ. Health Perspect. 124 (7), 983–990. doi:10.1289/ehp.1509966
Park, S.-Y., Byun, E. J., Lee, J. D., Kim, S., and Kim, H. S. (2018). Air pollution, autophagy, and skin aging: impact of particulate matter (PM10) on human dermal fibroblasts. Int. J. Mol. Sci. 19, 2727. doi:10.3390/ijms19092727
Parkinson, E. K., and Prime, S. S. (2022). Oral senescence: from molecular biology to clinical research. Front. Dent. Med. 3. doi:10.3389/fdmed.2022.822397
Parvez, F., Medina, S., Santella, R. M., Islam, T., Lauer, F. T., Alam, N., et al. (2017). Arsenic exposures alter clinical indicators of anemia in a male population of smokers and non-smokers in Bangladesh. Toxicol. Appl. Pharmacol. 331, 62–68. doi:10.1016/j.taap.2017.05.014
Pedersen, J. K., Elo, I. T., Schupf, N., Perls, T. T., Stallard, E., Yashin, A. I., et al. (2017). The survival of spouses marrying into longevity-enriched families. J. Gerontol. A. Biol. Sci. Med. Sci. 72, 109–114. doi:10.1093/gerona/glw159
Penfield, J. D., Anderson, M., Lutzke, L., and Wang, K. K. (2013). The role of cellular senescence in the gastrointestinal mucosa. Gut Liver 7, 270–277. doi:10.5009/gnl.2013.7.3.270
Peters, A., Nawrot, T. S., and Baccarelli, A. A. (2021). Hallmarks of environmental insults. Cell 184, 1455–1468. doi:10.1016/j.cell.2021.01.043
Pieters, N., Janssen, B. G., Dewitte, H., Cox, B., Cuypers, A., Lefebvre, W., et al. (2016). Biomolecular markers within the core Axis of aging and particulate air pollution exposure in the elderly: A cross-sectional study. Environ. Health Perspect. 124, 943–950. doi:10.1289/ehp.1509728
Pifferi, F., Terrien, J., Marchal, J., Dal-Pan, A., Djelti, F., Hardy, I., et al. (2018). Caloric restriction increases lifespan but affects brain integrity in grey mouse lemur primates. Commun. Biol. 1, 30. doi:10.1038/s42003-018-0024-8
Pignolo, R. J., Law, S. F., and Chandra, A. (2021). Bone aging, cellular senescence, and osteoporosis. JBMR Plus 5, e10488. doi:10.1002/jbm4.10488
Plaisance, V., Brajkovic, S., Tenenbaum, M., Favre, D., Ezanno, H., Bonnefond, A., et al. (2016). Endoplasmic reticulum stress links oxidative stress to impaired pancreatic beta-cell function caused by human oxidized LDL. PloS One 11, e0163046. doi:10.1371/journal.pone.0163046
Planchart, A., Green, A., Hoyo, C., and Mattingly, C. J. (2018). Heavy metal exposure and metabolic syndrome: evidence from human and model system studies. Curr. Environ. Health Rep. 5, 110–124. doi:10.1007/s40572-018-0182-3
Plusquin, M., Guida, F., Polidoro, S., Vermeulen, R., Raaschou-Nielsen, O., Campanella, G., et al. (2017). DNA methylation and exposure to ambient air pollution in two prospective cohorts. Environ. Int. 108, 127–136. doi:10.1016/j.envint.2017.08.006
Pluvinage, J. V., and Wyss-Coray, T. (2020). Systemic factors as mediators of brain homeostasis, ageing and neurodegeneration. Nat. Rev. Neurosci. 21, 93–102. doi:10.1038/s41583-019-0255-9
Pope, C. A., Ezzati, M., and Dockery, D. W. (2009). Fine particulate air pollution and US county life expectancies. N. Engl. J. Med. 360, 376–386. doi:10.1056/NEJMsa0805646
Prunicki, M., Stell, L., Dinakarpandian, D., de Planell-Saguer, M., Lucas, R. W., Hammond, S. K., et al. (2018). Exposure to NO2, CO, and PM2.5 is linked to regional DNA methylation differences in asthma. Clin. Epigenetics 10, 2. doi:10.1186/s13148-017-0433-4
Rabanal-Ruiz, Y., Otten, E. G., and Korolchuk, V. I. (2017). mTORC1 as the main gateway to autophagy. Essays Biochem. 61, 565–584. doi:10.1042/EBC20170027
Rachmian, N., and Krizhanovsky, V. (2023). Senescent cells in the brain and where to find them. FEBS J. 290, 1256–1266. doi:10.1111/febs.16649
Rao, X., Zhong, J., Brook, R. D., and Rajagopalan, S. (2018). Effect of particulate matter air pollution on cardiovascular oxidative stress pathways. Antioxid. Redox Signal. 28, 797–818. doi:10.1089/ars.2017.7394
Rardin, M. J., Newman, J. C., Held, J. M., Cusack, M. P., Sorensen, D. J., Li, B., et al. (2013). Label-free quantitative proteomics of the lysine acetylome in mitochondria identifies substrates of SIRT3 in metabolic pathways. Proc. Natl. Acad. Sci. U. S. A. 110, 6601–6606. doi:10.1073/pnas.1302961110
Reddam, A., McLarnan, S., and Kupsco, A. (2022). Environmental chemical exposures and mitochondrial dysfunction: a review of recent literature. Curr. Environ. Health Rep. 9, 631–649. doi:10.1007/s40572-022-00371-7
Rera, M., Clark, R. I., and Walker, D. W. (2012). Intestinal barrier dysfunction links metabolic and inflammatory markers of aging to death in Drosophila. Proc. Natl. Acad. Sci. U. S. A. 109, 21528–21533. doi:10.1073/pnas.1215849110
Ribeiro, G., Costa, N. de S. X., Belotti, L., Alemany, A., Cunha, P. G., Duro, S. de O., et al. (2017). Diesel exhaust exposure and sirtuins gene expression in old mice. Eur. Respir. J. 50. doi:10.1183/1393003.congress-2017.PA381
Ribeiro Júnior, G., de Souza Xavier Costa, N., Belotti, L., Dos Santos Alemany, A. A., Amato-Lourenço, L. F., da Cunha, P. G., et al. (2019). Diesel exhaust exposure intensifies inflammatory and structural changes associated with lung aging in mice. Ecotoxicol. Environ. Saf. 170, 314–323. doi:10.1016/j.ecoenv.2018.11.139
Rider, C. F., and Carlsten, C. (2019). Air pollution and DNA methylation: effects of exposure in humans. Clin. Epigenetics 11, 131. doi:10.1186/s13148-019-0713-2
Rodosthenous, R. S., Kloog, I., Colicino, E., Zhong, J., Herrera, L. A., Vokonas, P., et al. (2018). Extracellular vesicle-enriched microRNAs interact in the association between long-term particulate matter and blood pressure in elderly men. Environ. Res. 167, 640–649. doi:10.1016/j.envres.2018.09.002
Rose, G., Dato, S., Altomare, K., Bellizzi, D., Garasto, S., Greco, V., et al. (2003). Variability of the SIRT3 gene, human silent information regulator Sir2 homologue, and survivorship in the elderly. Exp. Gerontol. 38, 1065–1070. doi:10.1016/s0531-5565(03)00209-2
Ruby, J. G., Wright, K. M., Rand, K. A., Kermany, A., Noto, K., Curtis, D., et al. (2018). Estimates of the heritability of human longevity are substantially inflated due to assortative mating. Genetics 210, 1109–1124. doi:10.1534/genetics.118.301613
Ruthenburg, A. J., Li, H., Patel, D. J., and David Allis, C. (2007). Multivalent engagement of chromatin modifications by linked binding modules. Nat. Rev. Mol. Cell Biol. 8, 983–994. doi:10.1038/nrm2298
Sacks, S. H. (1999). Rapamycin on trial. Nephrol. Dial. Transpl. 14, 2087–2089. doi:10.1093/ndt/14.9.2087
Salminen, A., and Kaarniranta, K. (2012). AMP-activated protein kinase (AMPK) controls the aging process via an integrated signaling network. Ageing Res. Rev. 11, 230–241. doi:10.1016/j.arr.2011.12.005
Sarbassov, D. D., Ali, S. M., Sengupta, S., Sheen, J.-H., Hsu, P. P., Bagley, A. F., et al. (2006). Prolonged rapamycin treatment inhibits mTORC2 assembly and Akt/PKB. Mol. Cell 22, 159–168. doi:10.1016/j.molcel.2006.03.029
Schmauck-Medina, T., Molière, A., Lautrup, S., Zhang, J., Chlopicki, S., Madsen, H. B., et al. (2022). New hallmarks of ageing: a 2022 Copenhagen ageing meeting summary. Aging 14, 6829–6839. doi:10.18632/aging.204248
Schraufnagel, D. E., Balmes, J. R., Cowl, C. T., De Matteis, S., Jung, S.-H., Mortimer, K., et al. (2019). Air pollution and noncommunicable diseases: a review by the forum of international respiratory societies’ environmental committee, Part 2: air pollution and organ systems. Chest 155, 417–426. doi:10.1016/j.chest.2018.10.041
Scieszka, D., Hunter, R., Begay, J., Bitsui, M., Lin, Y., Galewsky, J., et al. (2022). Neuroinflammatory and neurometabolomic consequences from inhaled wildfire smoke-derived particulate matter in the western United States. Toxicol. Sci. Off. J. Soc. Toxicol. 186, 149–162. doi:10.1093/toxsci/kfab147
Scott, K. M., and Williams-Gray, C. H. (2018). Targeting aged astrocytes may Be a new therapeutic strategy in Parkinson’s disease. Mov. Disord. Off. J. Mov. Disord. Soc. 33, 758–759. doi:10.1002/mds.27387
Sebastián, C., Zwaans, B. M. M., Silberman, D. M., Gymrek, M., Goren, A., Zhong, L., et al. (2012). The histone deacetylase SIRT6 is a tumor suppressor that controls cancer metabolism. Cell 151, 1185–1199. doi:10.1016/j.cell.2012.10.047
Selman, C., Tullet, J. M. A., Wieser, D., Irvine, E., Lingard, S. J., Choudhury, A. I., et al. (2009). Ribosomal protein S6 kinase 1 signaling regulates mammalian life span. Science 326, 140–144. doi:10.1126/science.1177221
Shilatifard, A. (2006). Chromatin modifications by methylation and ubiquitination: implications in the regulation of gene expression. Annu. Rev. Biochem. 75, 243–269. doi:10.1146/annurev.biochem.75.103004.142422
Shin, J., He, M., Liu, Y., Paredes, S., Villanova, L., Brown, K., et al. (2013). SIRT7 represses Myc activity to suppress ER stress and prevent fatty liver disease. Cell Rep. 5, 654–665. doi:10.1016/j.celrep.2013.10.007
Shoura, M. J., Gabdank, I., Hansen, L., Merker, J., Gotlib, J., Levene, S. D., et al. (2017). Intricate and cell type-specific populations of endogenous circular DNA (eccDNA) in Caenorhabditis elegans and Homo sapiens. G3 Bethesda Md 7, 3295–3303. doi:10.1534/g3.117.300141
Silaghi, C. N., Farcaș, M., and Crăciun, A. M. (2021). Sirtuin 3 (SIRT3) pathways in age-related cardiovascular and neurodegenerative diseases. Biomedicines 9, 1574. doi:10.3390/biomedicines9111574
Silva, D. F., Esteves, A. R., Oliveira, C. R., and Cardoso, S. M. (2017). Mitochondrial metabolism power SIRT2-dependent deficient traffic causing alzheimer’s-disease related pathology. Mol. Neurobiol. 54, 4021–4040. doi:10.1007/s12035-016-9951-x
Simmnacher, K., Krach, F., Schneider, Y., Alecu, J. E., Mautner, L., Klein, P., et al. (2020). Unique signatures of stress-induced senescent human astrocytes. Exp. Neurol. 334, 113466. doi:10.1016/j.expneurol.2020.113466
Sinclair, D. A., and Guarente, L. (1997). Extrachromosomal rDNA circles-a cause of aging in yeast. Cell 91, 1033–1042. doi:10.1016/s0092-8674(00)80493-6
Small, E. M., Felker, D. P., Heath, O. C., Cantergiani, R. J., Osley, M. A., and McCormick, M. A. (2020). SPOCK, an R based package for high-throughput analysis of growth rate, survival, and chronological lifespan in yeast. Transl. Med. Aging 4, 141–148. doi:10.1016/j.tma.2020.08.003
Sola-Sevilla, N., Ricobaraza, A., Hernandez-Alcoceba, R., Aymerich, M. S., Tordera, R. M., and Puerta, E. (2021). Understanding the potential role of sirtuin 2 on aging: consequences of SIRT2.3 overexpression in senescence. Int. J. Mol. Sci. 22, 3107. doi:10.3390/ijms22063107
Solaimani, P., Saffari, A., Sioutas, C., Bondy, S. C., and Campbell, A. (2017). Exposure to ambient ultrafine particulate matter alters the expression of genes in primary human neurons. Neurotoxicology 58, 50–57. doi:10.1016/j.neuro.2016.11.001
Somineni, H. K., Zhang, X., Biagini Myers, J. M., Kovacic, M. B., Ulm, A., Jurcak, N., et al. (2016). Ten-eleven translocation 1 (TET1) methylation is associated with childhood asthma and traffic-related air pollution. J. Allergy Clin. Immunol. 137 (3), 797–805. doi:10.1016/j.jaci.2015.10.021
Song, Y., Zhao, L., Qi, Z., Zhang, Y., Cao, G., Li, R., et al. (2021). Application of a real-ambient fine particulate matter exposure system on different animal models. J. Environ. Sci. China 105, 64–70. doi:10.1016/j.jes.2020.12.007
Sontag, E. M., Samant, R. S., and Frydman, J. (2017). Mechanisms and functions of spatial protein quality control. Annu. Rev. Biochem. 86, 97–122. doi:10.1146/annurev-biochem-060815-014616
Sreekumar, P. G., Hinton, D. R., and Kannan, R. (2020). The emerging role of senescence in ocular disease. Oxid. Med. Cell. Longev. 2020, 2583601. doi:10.1155/2020/2583601
Srinivas, N., Rachakonda, S., and Kumar, R. (2020). Telomeres and telomere length: A general overview. Cancers 12, 558. doi:10.3390/cancers12030558
Stepien, K. M., Roncaroli, F., Turton, N., Hendriksz, C. J., Roberts, M., Heaton, R. A., et al. (2020). Mechanisms of mitochondrial dysfunction in lysosomal storage disorders: A review. J. Clin. Med. 9, 2596. doi:10.3390/jcm9082596
Sterner, D. E., and Berger, S. L. (2000). Acetylation of histones and transcription-related factors. Microbiol. Mol. Biol. Rev. MMBR 64, 435–459. doi:10.1128/MMBR.64.2.435-459.2000
Su, T.-Y., Chuang, K.-J., Jeng, H., Hsu, Y.-T., Chuang, H.-C., Lai, C.-H., et al. (2021). Mixture effects of heavy metals on insulin resistance in shipyard welders. Preprint. doi:10.20944/preprints202101.0004.v1
Sundaresan, N. R., Bindu, S., Pillai, V. B., Samant, S., Pan, Y., Huang, J.-Y., et al. (2015). SIRT3 blocks aging-associated tissue fibrosis in mice by deacetylating and activating glycogen synthase kinase 3β. Mol. Cell. Biol. 36, 678–692. doi:10.1128/MCB.00586-15
Tan, L., Register, T. C., and Yammani, R. R. (2020). Age-related decline in expression of molecular chaperones induces endoplasmic reticulum stress and chondrocyte apoptosis in articular cartilage. Aging Dis. 11, 1091–1102. doi:10.14336/AD.2019.1130
Tang, B. L., and Chua, C. E. L. (2008). SIRT2, tubulin deacetylation, and oligodendroglia differentiation. Cell Motil. Cytoskelet. 65, 179–182. doi:10.1002/cm.20253
Tanwar, V., Adelstein, J. M., Grimmer, J. A., Youtz, D. J., Katapadi, A., Sugar, B. P., et al. (2018). Preconception exposure to fine particulate matter leads to cardiac dysfunction in adult male offspring. J. Am. Heart Assoc. Cardiovasc. Cerebrovasc. Dis. 7, e010797. doi:10.1161/JAHA.118.010797
Tarantini, L., Bonzini, M., Tripodi, A., Angelici, L., Nordio, F., Cantone, L., et al. (2013). Blood hypomethylation of inflammatory genes mediates the effects of metal-rich airborne pollutants on blood coagulation. Occup. Environ. Med. 70 (6), 418–425. doi:10.1136/oemed-2012-101079
Tasselli, L., Xi, Y., Zheng, W., Tennen, R. I., Odrowaz, Z., Simeoni, F., et al. (2016). SIRT6 deacetylates H3K18ac at pericentric chromatin to prevent mitotic errors and cellular senescence. Nat. Struct. Mol. Biol. 23, 434–440. doi:10.1038/nsmb.3202
Taylor, R. C., and Dillin, A. (2011). Aging as an event of proteostasis collapse. Cold Spring Harb. Perspect. Biol. 3, a004440. doi:10.1101/cshperspect.a004440
Teo, Y. V., Rattanavirotkul, N., Olova, N., Salzano, A., Quintanilla, A., Tarrats, N., et al. (2019). Notch signaling mediates secondary senescence. Cell Rep. 27, 997–1007.e5. doi:10.1016/j.celrep.2019.03.104
Terman, A., and Brunk, U. T. (1998). Lipofuscin: mechanisms of formation and increase with age. APMIS Acta Pathol. Microbiol. Immunol. Scand. 106, 265–276. doi:10.1111/j.1699-0463.1998.tb01346.x
Tian, H., Shakya, A., Wang, F., Wu, W. D., and Li, W. (2020). Comparative ligandomic analysis of human lung epithelial cells exposed to PM 2.5. Biomed. Environ. Sci. Bes. 33, 165–173. doi:10.3967/bes2020.023
Tobaldini, E., Bollati, V., Prado, M., Fiorelli, E. M., Pecis, M., Bissolotti, G., et al. (2018). Acute particulate matter affects cardiovascular autonomic modulation and IFN-gamma methylation in healthy volunteers. Environ. Res. 161, 97–103. doi:10.1016/j.envres.2017.10.036
Trojer, P., and Reinberg, D. (2006). Histone lysine demethylases and their impact on epigenetics. Cell 125, 213–217. doi:10.1016/j.cell.2006.04.003
Tsamou, M., Nawrot, T. S., Carollo, R. M., Trippas, A.-J., Lefebvre, W., Vanpoucke, C., et al. (2020). Prenatal particulate air pollution exposure and expression of the miR-17/92 cluster in cord blood: findings from the ENVIRONAGE birth cohort. Environ. Int. 142, 105860. doi:10.1016/j.envint.2020.105860
Turner, K. M., Deshpande, V., Beyter, D., Koga, T., Rusert, J., Lee, C., et al. (2017). Extrachromosomal oncogene amplification drives tumour evolution and genetic heterogeneity. Nature 543, 122–125. doi:10.1038/nature21356
Tyler, C. R., Noor, S., Young, T. L., Rivero, V., Sanchez, B., Lucas, S., et al. (2018). Aging exacerbates neuroinflammatory outcomes induced by acute ozone exposure. Toxicol. Sci. Off. J. Soc. Toxicol. 163, 123–139. doi:10.1093/toxsci/kfy014
Tyler, C. R., Zychowski, K. E., Sanchez, B. N., Rivero, V., Lucas, S., Herbert, G., et al. (2016). Surface area-dependence of gas-particle interactions influences pulmonary and neuroinflammatory outcomes. Part. Fibre Toxicol. 13, 64. doi:10.1186/s12989-016-0177-x
Vaarhorst, A. A. M., Beekman, M., Suchiman, E. H. D., van Heemst, D., Houwing-Duistermaat, J. J., Westendorp, R. G. J., et al. (2011). Lipid metabolism in long-lived families: the leiden longevity study. Age Dordr. Neth. 33, 219–227. doi:10.1007/s11357-010-9172-6
Vaiserman, A., and Krasnienkov, D. (2021). Telomere length as a marker of biological age: state-of-the-art, open issues, and future perspectives. Front. Genet. 11. doi:10.3389/fgene.2020.630186
Vakhrusheva, O., Smolka, C., Gajawada, P., Kostin, S., Boettger, T., Kubin, T., et al. (2008). Sirt7 increases stress resistance of cardiomyocytes and prevents apoptosis and inflammatory cardiomyopathy in mice. Circ. Res. 102, 703–710. doi:10.1161/CIRCRESAHA.107.164558
Valderrama, A., Zapata, M. I., Hernandez, J. C., and Cardona-Arias, J. A. (2022). Systematic review of preclinical studies on the neutrophil-mediated immune response to air pollutants, 1980–2020. Heliyon 8, e08778. doi:10.1016/j.heliyon.2022.e08778
van den Berg, N., Beekman, M., Smith, K. R., Janssens, A., and Slagboom, P. E. (2017). Historical demography and longevity genetics: back to the future. Ageing Res. Rev. 38, 28–39. doi:10.1016/j.arr.2017.06.005
van den Berg, N., Rodríguez-Girondo, M., van Dijk, I. K., Mourits, R. J., Mandemakers, K., Janssens, A. A. P. O., et al. (2019). Longevity defined as top 10% survivors and beyond is transmitted as a quantitative genetic trait. Nat. Commun. 10, 35. doi:10.1038/s41467-018-07925-0
Vazquez, B. N., Thackray, J. K., Simonet, N. G., Kane-Goldsmith, N., Martinez-Redondo, P., Nguyen, T., et al. (2016). SIRT7 promotes genome integrity and modulates non-homologous end joining DNA repair. EMBO J. 35, 1488–1503. doi:10.15252/embj.201593499
Vernace, V. A., Schmidt-Glenewinkel, T., and Figueiredo-Pereira, M. E. (2007). Aging and regulated protein degradation: who has the UPPer hand? Aging Cell 6, 599–606. doi:10.1111/j.1474-9726.2007.00329.x
Vézina, C., Kudelski, A., and Sehgal, S. N. (1975). Rapamycin (AY-22,989), a new antifungal antibiotic. I. Taxonomy of the producing streptomycete and isolation of the active principle. J. Antibiot. (Tokyo) 28, 721–726. doi:10.7164/antibiotics.28.721
Vijayakumar, A., Yakar, S., and LeRoith, D. (2011). The intricate role of growth hormone in metabolism. Front. Endocrinol. 2, 32. doi:10.3389/fendo.2011.00032
Vijg, J., and Montagna, C. (2017). Genome instability and aging: cause or effect? Transl. Med. Aging 1, 5–11. doi:10.1016/j.tma.2017.09.003
Vijg, J., and Suh, Y. (2013). Genome instability and aging. Annu. Rev. Physiol. 75, 645–668. doi:10.1146/annurev-physiol-030212-183715
Villeda, S. A., Plambeck, K. E., Middeldorp, J., Castellano, J. M., Mosher, K. I., Luo, J., et al. (2014). Young blood reverses age-related impairments in cognitive function and synaptic plasticity in mice. Nat. Med. 20, 659–663. doi:10.1038/nm.3569
Vinggaard, A. M., Bonefeld-Jørgensen, E. C., Jensen, T. K., Fernandez, M. F., Rosenmai, A. K., Taxvig, C., et al. (2021). Receptor-based in vitro activities to assess human exposure to chemical mixtures and related health impacts. Environ. Int. 146, 106191. doi:10.1016/j.envint.2020.106191
von Zglinicki, T., Wan, T., and Miwa, S. (2021). Senescence in post-mitotic cells: A driver of aging? Antioxid. Redox Signal. 34, 308–323. doi:10.1089/ars.2020.8048
Wang, F., Chamani, I. J., Luo, D., Chan, K., Navarro, P. A., and Keefe, D. L. (2021a). Inhibition of LINE-1 retrotransposition represses telomere reprogramming during mouse 2-cell embryo development. J. Assist. Reprod. Genet. 38, 3145–3153. doi:10.1007/s10815-021-02331-w
Wang, T., Zhang, H., Zhou, Y., and Shi, J. (2021b). Extrachromosomal circular DNA: a new potential role in cancer progression. J. Transl. Med. 19, 257. doi:10.1186/s12967-021-02927-x
Ward-Caviness, C. K., Nwanaji-Enwerem, J. C., Wolf, K., Wahl, S., Colicino, E., Trevisi, L., et al. (2016). Long-term exposure to air pollution is associated with biological aging. Oncotarget 7, 74510–74525. doi:10.18632/oncotarget.12903
Warmerdam, D. O., and Wolthuis, R. M. F. (2019). Keeping ribosomal DNA intact: a repeating challenge. Chromosome Res. 27, 57–72. doi:10.1007/s10577-018-9594-z
Warren, S., Chute, R. N., and Farrington, E. M. (1960). Protection of the hematopoietic system by parabiosis. Lab. Investig. J. Tech. Methods Pathol. 9, 191–198.
Waters, M. J., and Kaye, P. L. (2002). The role of growth hormone in fetal development. Growth Horm. IGF Res. Off. J. Growth Horm. Res. Soc. Int. IGF Res. Soc. 12, 137–146. doi:10.1016/s1096-6374(02)00018-7
Watson, J. D. (1972). Origin of concatemeric T7DNA. Nat. New Biol. 239, 197–201. doi:10.1038/newbio239197a0
Watterson, T. L., Hamilton, B., Martin, R., and Coulombe, R. A. (2009). Urban particulate matter causes ER stress and the unfolded protein response in human lung cells. Toxicol. Sci. Off. J. Soc. Toxicol. 112, 111–122. doi:10.1093/toxsci/kfp186
Watts, E., Huff, R., Rider, C., Whyte, M., Walmsley, S., and Carlsten, C. (2022). Exposure to particulate matter alters neutrophil lysosomal function and activation status. Eur. Respir. J. 60. doi:10.1183/13993003.congress-2022.4686
White, A. J., Keller, J. P., Zhao, S., Carroll, R., Kaufman, J. D., and Sandler, D. P. (2019a). Air pollution, clustering of particulate matter components, and breast cancer in the sister study: A U.S.-Wide cohort. Environ. Health Perspect. 127, 107002. doi:10.1289/EHP5131
White, A. J., Kresovich, J. K., Keller, J. P., Xu, Z., Kaufman, J. D., Weinberg, C. R., et al. (2019b). Air pollution, particulate matter composition and methylation-based biologic age. Environ. Int. 132, 105071. doi:10.1016/j.envint.2019.105071
WHO (2017). Wildfires. Available at: https://www.who.int/health-topics/wildfires (Accessed March 7, 2023).
Wijsman, C. A., Rozing, M. P., Streefland, T. C. M., le Cessie, S., Mooijaart, S. P., Slagboom, P. E., et al. (2011). Familial longevity is marked by enhanced insulin sensitivity. Aging Cell 10, 114–121. doi:10.1111/j.1474-9726.2010.00650.x
Wilhelm-Benartzi, C. S., Christensen, B. C., Koestler, D. C., Houseman, E. A., Schned, A. R., Karagas, M. R., et al. (2011). Association of secondhand smoke exposures with DNA methylation in bladder carcinomas. Cancer Causes Control CCC 22, 1205–1213. doi:10.1007/s10552-011-9788-6
Wongchitrat, P., Pakpian, N., Kitidee, K., Phopin, K., Dharmasaroja, P. A., and Govitrapong, P. (2019). Alterations in the expression of amyloid precursor protein cleaving enzymes mRNA in alzheimer peripheral blood. Curr. Alzheimer Res. 16, 29–38. doi:10.2174/1567205015666181109103742
Wu, S., Turner, K. M., Nguyen, N., Raviram, R., Erb, M., Santini, J., et al. (2019). Circular ecDNA promotes accessible chromatin and high oncogene expression. Nature 575, 699–703. doi:10.1038/s41586-019-1763-5
Wu, S., Wei, Y., Li, J., Bai, Y., Yin, P., and Wang, S. (2021). SIRT5 represses neurotrophic pathways and aβ production in Alzheimer’s disease by targeting autophagy. ACS Chem. Neurosci. 12, 4428–4437. doi:10.1021/acschemneuro.1c00468
Xing, M. (2010). Genetic alterations in the phosphatidylinositol-3 kinase/Akt pathway in thyroid cancer. Thyroid. Off. J. Am. Thyroid. Assoc. 20, 697–706. doi:10.1089/thy.2010.1646
Xu, M., Bradley, E. W., Weivoda, M. M., Hwang, S. M., Pirtskhalava, T., Decklever, T., et al. (2017). Transplanted senescent cells induce an osteoarthritis-like condition in mice. J. Gerontol. A. Biol. Sci. Med. Sci. 72, 780–785. doi:10.1093/gerona/glw154
Xu, X., Zhang, L., Chu, J. T. S., Wang, Y., Chin, A. W. H., Chong, T. H., et al. (2021). A novel mechanism of enhanced transcription activity and fidelity for influenza A viral RNA-dependent RNA polymerase. Nucleic Acids Res. 49, 8796–8810. doi:10.1093/nar/gkab660
Yamazaki, Y., Baker, D. J., Tachibana, M., Liu, C.-C., van Deursen, J. M., Brott, T. G., et al. (2016). Vascular cell senescence contributes to blood-brain barrier breakdown. Stroke 47, 1068–1077. doi:10.1161/STROKEAHA.115.010835
Yang, L., Ma, X., He, Y., Yuan, C., Chen, Q., Li, G., et al. (2017). Sirtuin 5: a review of structure, known inhibitors and clues for developing new inhibitors. Sci. China Life Sci. 60, 249–256. doi:10.1007/s11427-016-0060-7
Yang, W., Nagasawa, K., Münch, C., Xu, Y., Satterstrom, K., Jeong, S., et al. (2016). Mitochondrial sirtuin network reveals dynamic SIRT3-dependent deacetylation in response to membrane depolarization. Cell 167, 985–1000.e21. doi:10.1016/j.cell.2016.10.016
Yao, Y., Liu, L., Guo, G., Zeng, Y., and Ji, J. S. (2021). Interaction of sirtuin 1 (SIRT1) candidate longevity gene and particulate matter (PM2.5) on all-cause mortality: a longitudinal cohort study in China. Environ. Health 20, 25. doi:10.1186/s12940-021-00718-x
Yauk, C., Polyzos, A., Rowan-Carroll, A., Somers, C. M., Godschalk, R. W., Van Schooten, F. J., et al. (2008). Germ-line mutations, DNA damage, and global hypermethylation in mice exposed to particulate air pollution in an urban/industrial location. Proc. Natl. Acad. Sci. U. S. A. 105 (2), 605–610. doi:10.1073/pnas.0705896105
Yousef, H., Conboy, M. J., Morgenthaler, A., Schlesinger, C., Bugaj, L., Paliwal, P., et al. (2015). Systemic attenuation of the TGF-β pathway by a single drug simultaneously rejuvenates hippocampal neurogenesis and myogenesis in the same old mammal. Oncotarget 6, 11959–11978. doi:10.18632/oncotarget.3851
Yousef, H., Czupalla, C. J., Lee, D., Chen, M. B., Burke, A. N., Zera, K. A., et al. (2019). Aged blood impairs hippocampal neural precursor activity and activates microglia via brain endothelial cell VCAM1. Nat. Med. 25, 988–1000. doi:10.1038/s41591-019-0440-4
Yu, Z., Seim, I., Yin, M., Tian, R., Sun, D., Ren, W., et al. (2021). Comparative analyses of aging-related genes in long-lived mammals provide insights into natural longevity. Innov. Camb. Mass 2, 100108. doi:10.1016/j.xinn.2021.100108
Yudoh, K., Karasawa, R., and Ishikawa, J. (2015). Age-related decrease of sirtuin 2 protein in human peripheral blood mononuclear cells. Curr. Aging Sci. 8, 256–258. doi:10.2174/1874609808999150831112939
Zeng, G., Liu, H., and Wang, H. (2018). Amelioration of myocardial ischemia-reperfusion injury by SIRT4 involves mitochondrial protection and reduced apoptosis. Biochem. Biophys. Res. Commun. 502, 15–21. doi:10.1016/j.bbrc.2018.05.113
Zhang, P.-Y., Li, G., Deng, Z.-J., Liu, L.-Y., Chen, L., Tang, J.-Z., et al. (2016). Dicer interacts with SIRT7 and regulates H3K18 deacetylation in response to DNA damaging agents. Nucleic Acids Res. 44, 3629–3642. doi:10.1093/nar/gkv1504
Zhang, X., Spiegelman, N. A., Nelson, O. D., Jing, H., and Lin, H. (2017). SIRT6 regulates Ras-related protein R-Ras2 by lysine defatty-acylation. eLife 6, e25158. doi:10.7554/eLife.25158
Zhang, Y., Wolosker, M. B., Zhao, Y., Ren, H., and Lemos, B. (2020). Exposure to microplastics cause gut damage, locomotor dysfunction, epigenetic silencing, and aggravate cadmium (Cd) toxicity in Drosophila. Sci. Total Environ. 744, 140979. doi:10.1016/j.scitotenv.2020.140979
Zhao, B., Vo, H. Q., Johnston, F. H., and Negishi, K. (2018a). Air pollution and telomere length: a systematic review of 12,058 subjects. Cardiovasc. Diagn. Ther. 8, 48092–48492. doi:10.21037/cdt.2018.06.05
Zhao, L., Zhao, J., Zhong, K., Tong, A., and Jia, D. (2022). Targeted protein degradation: mechanisms, strategies and application. Signal Transduct. Target. Ther. 7, 1–13. doi:10.1038/s41392-022-00966-4
Zhao, Y., Wu, L., Yue, X., Zhang, C., Wang, J., Li, J., et al. (2018b). A polymorphism in the tumor suppressor p53 affects aging and longevity in mouse models. eLife 7, e34701. doi:10.7554/eLife.34701
Zhong, L., D’Urso, A., Toiber, D., Sebastian, C., Henry, R. E., Vadysirisack, D. D., et al. (2010). The histone deacetylase Sirt6 regulates glucose homeostasis via Hif1alpha. Cell 140, 280–293. doi:10.1016/j.cell.2009.12.041
Zhou, X., Medina, S., Bolt, A. M., Zhang, H., Wan, G., Xu, H., et al. (2020). Inhibition of red blood cell development by arsenic-induced disruption of GATA-1. Sci. Rep. 10, 19055. doi:10.1038/s41598-020-76118-x
Zhu, X., Lee, H., Raina, A. K., Perry, G., and Smith, M. A. (2002). The role of mitogen-activated protein kinase pathways in Alzheimer’s disease. Neurosignals 11, 270–281. doi:10.1159/000067426
Keywords: aging, environmental exposures, environmental stressors, longevity, wildfires, wildfire smoke, air quality
Citation: Scieszka D, Bolt AM, McCormick MA, Brigman JL and Campen MJ (2023) Aging, longevity, and the role of environmental stressors: a focus on wildfire smoke and air quality. Front. Toxicol. 5:1267667. doi: 10.3389/ftox.2023.1267667
Received: 26 July 2023; Accepted: 25 September 2023;
Published: 11 October 2023.
Edited by:
Johnny Wise, University of Louisville, United StatesReviewed by:
Shreesh Raj Sammi, Michigan State University, United StatesCraig Slattery, University College Dublin, Ireland
Copyright © 2023 Scieszka, Bolt, McCormick, Brigman and Campen. This is an open-access article distributed under the terms of the Creative Commons Attribution License (CC BY). The use, distribution or reproduction in other forums is permitted, provided the original author(s) and the copyright owner(s) are credited and that the original publication in this journal is cited, in accordance with accepted academic practice. No use, distribution or reproduction is permitted which does not comply with these terms.
*Correspondence: David Scieszka, ZHNjaWVzemthQHNhbHVkLnVubS5lZHU=; Matthew J. Campen, bWNhbXBlbkBzYWx1ZC51bm0uZWR1