- 1Fraunhofer Institute for Molecular Biology and Applied Ecology, Schmallenberg, Germany
- 2Centre for Fish and Wildlife Health, University of Bern, Bern, Switzerland
- 3Department of Physical & Environmental Sciences, University of Toronto Scarborough, Toronto, ON, Canada
- 4ARC Arnot Research and Consulting, Toronto, ON, Canada
Biotransformation assays using primary hepatocytes from rainbow trout, Oncorhynchus mykiss, were validated as a reliable in vitro tool to predict in vivo bioconcentration factors (BCF) of chemicals in fish. Given the pronounced interspecies differences of chemical biotransformation, the present study aimed to compare biotransformation rate values and BCF predictions obtained with hepatocytes from the cold-water species, rainbow trout, to data obtained with hepatocytes of the warm-water species, common carp (Cyprinus carpio). In a first step, we adapted the protocol for the trout hepatocyte assay, including the cryopreservation method, to carp hepatocytes. The successful adaptation serves as proof of principle that the in vitro hepatocyte biotransformation assays can be technically transferred across fish species. In a second step, we compared the in vitro intrinsic clearance rates (CLin vitro, int) of two model xenobiotics, benzo[a]pyrene (BaP) and methoxychlor (MXC), in trout and carp hepatocytes. The in vitro data were used to predict in vivo biotransformation rate constants (kB) and BCFs, which were then compared to measured in vivo kB and BCF values. The CLin vitro, int values of BaP and MXC did not differ significantly between trout and carp hepatocytes, but the predicted BCF values were significantly higher in trout than in carp. In contrast, the measured in vivo BCF values did not differ significantly between the two species. A possible explanation of this discrepancy is that the existing in vitro-in vivo prediction models are parameterized only for trout but not for carp. Therefore, future research needs to develop species-specific extrapolation models.
Introduction
For the aquatic environment, the most widely used parameter to estimate the bioaccumulation potential of a chemical is the bioconcentration factor (BCF) in fish. The standard approach for regulatory purposes to determine the BCF is the in vivo test according to the OECD Test Guideline (TG) 305 (OECD, 2012). The test is labor-intensive, costly, and requires a high number of animals (>100 per test). With the implementation of new chemical regulations worldwide (e.g., REACH), the need for bioaccumulation testing has increased extensively (Nichols et al., 2007; Gobas et al., 2009). The development of alternative testing strategies to reduce the need for in vivo BCF testing is therefore urgently needed (de Wolf et al., 2007; Lombardo et al., 2014).
A possible component of an alternative to the in vivo bioaccumulation assessment for fish is the use of in silico predictions of BCF values using Quantitative-Structure-Activity-Relationships (QSARs), mass balance models or physiologically based toxicokinetic (PBTK) models (Arnot and Gobas, 2003; Arnot et al., 2008; Nendza et al., 2018). A major uncertainty in the in silico predictions of in vivo BCF values, however, is the role of biotransformation which may substantially reduce the bioaccumulation potential of chemicals (Nichols et al., 2007). In absence of reliable biotransformation rate data, mass balance and PBTK models will overpredict BCFs for chemicals subject to biotransformation (Nichols et al., 2007; Nichols et al., 2009). QSARs for predicting biotransformation rate constants (and associated half-lives) in fish from chemical structure have been developed and validated (Arnot et al., 2009; Brown et al., 2012; Papa et al., 2014; Mansouri et al., 2018) following OECD QSAR guidance for use in regulatory applications (OECD, 2004, 2014). However, there is a need to expand the applicability domain of the QSARs by adding more diversity to the chemical training and testing sets, as well by evaluating inter-species differences in biotransformation rate constants. An approach to broaden the database on chemical biotransformation rates under avoidance of animal testing is the use of in vitro assays based on isolated liver cells or liver subcellular (post-mitochondrial) fractions (hepatic S9 fractions) of fish (Segner and Cravedi, 2001; de Wolf et al., 2007; Han et al., 2007; Nichols et al., 2007; Weisbrod et al., 2009; Kropf et al., 2020). These assays determine the depletion of a test compound over time (substrate depletion approach), and provide in vitro intrinsic clearance rates (CLin vitro, int). In vitro-in vivo extrapolation (IVIVE) models can then be used to estimate in vivo intrinsic clearance rates (CLin vivo, int), tissue clearance, i.e., hepatic clearance (CLH) and “whole body” biotransformation rate constants (kB) (Han et al., 2007; Nichols et al., 2013; Krause and Goss, 2018). For both the hepatocyte and the S9 assay, standard protocols and OECD Test Guidelines are available (Fay et al., 2015; Johanning et al., 2012; OECD, 2018a, b) so that they can be applied in regulatory testing.
The OECD TG 305 for in vivo BCF determination in fish accepts a broad variety of cold and warm water fish as test species, including rainbow trout (Oncorhynchus mykiss), common carp (Cyprinus carpio), fathead minnow (Pimephales promelas), medaka (Oryzias latipes), stickleback (Gasterosteus aculeatus), etc. (OECD, 2012). In contrast, the existing protocols for in vitro biotransformation assays are restricted to a single fish species, the rainbow trout. It is well documented that chemical biotransformation can differ strongly between fish species (Roberts et al., 2011; Bearr et al., 2012). It is thus critical to develop confidence in the inter-species scaling of biotransformation rates obtained from in vitro testing methods (Lin, 1998; Schultz and Hayton, 1999; Hutchinson et al., 2014). The question that needs to be answered is whether the in vitro assays for biotransformation assessment can follow a “one size fits all” approach, i.e., restrict the in vitro testing to a single species like rainbow trout, or whether they have to be expanded to in vitro preparations from diverse species.
The present study aims to compare biotransformation rate data obtained from in vitro assays with hepatocytes of a cold-water fish species, the rainbow trout, and a warm-water fish species, the common carp, Cyprinus carpio. More specifically, the questions addressed in this study are: i) Is it technically feasible to transfer the in vitro method established for rainbow trout hepatocytes to carp hepatocytes?, ii) can the resulting in vitro biotransformation rates be used to predict species-specific in vivo kB and BCF values?, and iii) how do the predicted values compare to the in vivo values as determined by means of the OECD 305 Test Guideline for trout and carp?
Common carp has been selected as a second species since it is frequently used for BCF testing in Asian countries (Weisbrod et al., 2007), and it is the second most common fish species used for laboratory bioaccumulation experiments (Arnot and Gobas, 2006; Arnot and Quinn, 2015). As test compounds, we selected two xenobiotics which show rather different biotransformation rates in rainbow trout hepatocytes, namely benzo[a]pyrene (BaP), and methoxychlor (MXC). BaP shows relatively fast rates of biotransformation and MXC shows relatively slow rates (Fay et al., 2014b; Nichols et al., 2018). Both chemicals are hydrophobic compounds with relatively high log KOW values (5.99 and 5.08, respectively) (Fay et al., 2014b). In addition to the in vitro assays, we performed in vivo studies according to OECD TG 305, since we were not able to find BCF data of comparable quality between rainbow trout and common carp. Finally, the present study also addressed the question whether the cryopreservation protocol as it has been established for rainbow trout hepatocytes (Mingoia et al., 2010), can be applied for carp hepatocytes as well.
Materials and methods
All experiments of the present study were carried out in late summer in the same laboratory. The in vitro experiments were performed with the same batches of fish and identical procedures of sample preparation and analysis were used, minimizing potential technical sources of variability in biotransformation rates.
Standards and reagents
Methoxychlor (MXC, 99.7% purity, used in the in vivo experiments) and benzo[a]pyrene (BaP, ≥ 96% purity) and Florisil®, 30–60 mesh, were obtained from Sigma Aldrich (Schnelldorf, Germany). 14C-MXC (3.96 MBq/mg, 98.4% radiochemical purity, used in the in vitro assays) was ordered from Quotient Bioresearch (Cardiff, UK). Deuterated methoxychlor (MXC-d6, 99 atom % D) and benzo[a]pyrene (BaP-d12, 98.4 atom % D) were purchased from CDN Isotopes (Pointe-Claire, Quebec, Canada) and Labor Dr. Ehrenstorfer-Schäfers (Augsburg, Germany), respectively. Media, reagents, and material used for the in vitro hepatocyte experiments are specified in Bischof et al. (2016). All other reagents and solvents were of the purest grade available and purchased from commercial sources if not stated otherwise.
Animals
Common carp (mirror carp, Cyprinus carpio) and rainbow trout (Oncorhynchus mykiss) used for liver cell isolation were obtained from Westerwälder Fischzucht Stähler (Hadamar-Niederzeuzheim, Germany) and as fertilized eggs from Sauerländer Forellenzucht Rameil (Lennestadt, Germany), respectively. The fish were raised to their experimental size at the Fraunhofer-IME-husbandry (see below). Rainbow trout used in this study were all female and sexually immature with an average gonadosomatic index (GSI) of <0.2 (Fay et al., 2014a) (Table 1). Common carp, in contrast, were from both sexes and their average GSI was 2.47–5.52, indicating sexual maturity. Further information on the animals used for cell isolation is given in Bischof et al. (2016). Rainbow trout (Oncorhynchus mykiss) and common carp (mirror carp, Cyprinus carpio) used in the in vivo bioconcentration tests were purchased as juveniles from Fischzucht Störk (Bad Saulgau, Germany) and Carus-ARF (Wageningen, Netherlands), respectively.
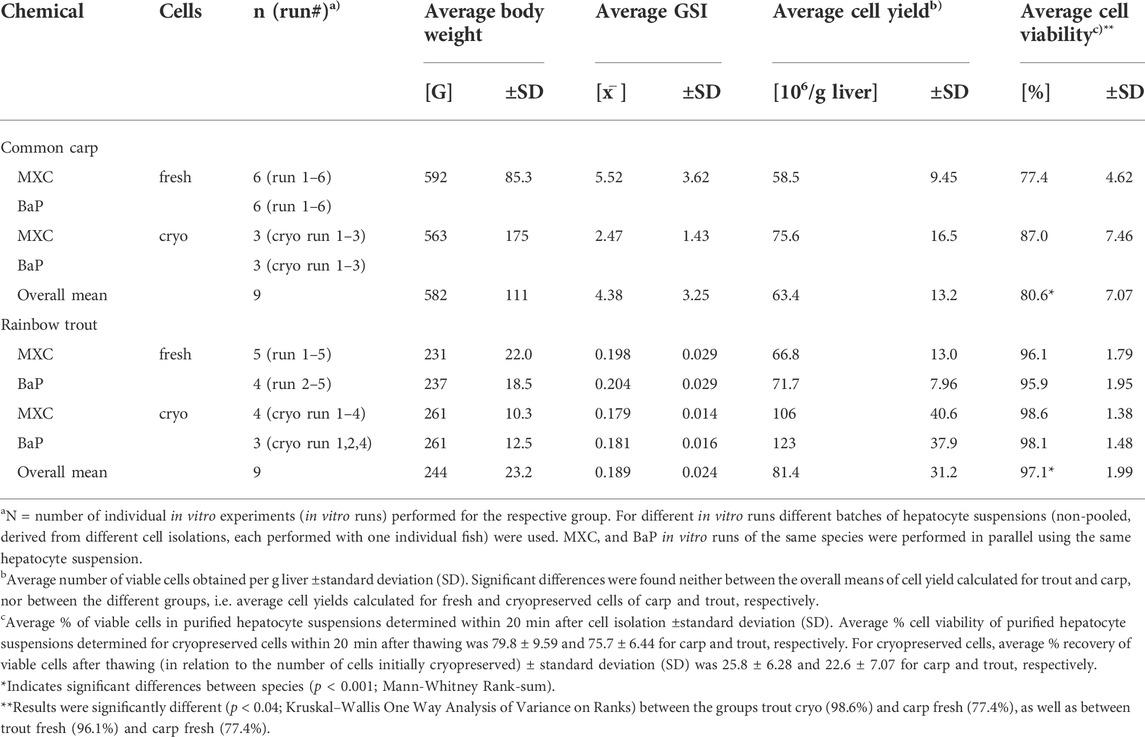
TABLE 1. Overview of the in vitro assays: Characteristics of hepatocyte donor fish and quality of cell isolation.
All fish were held at the Fraunhofer-IME-husbandry in charcoal-filtered and dechlorinated city water at a temperature of 11–16°C (trout) and 18–22°C (carp) and under a 16:8 light/dark cycle for several weeks or months until the study start. The fish were fed once daily with a commercially available extruded diet (Milkivit-type F-2P B40 for trout and Milkivit-type Pro Aqua K18 C3 for carp) at a rate of 1% of the animals’ average weight.
Isolation and cryopreservation of hepatocytes
Isolation of hepatocytes from liver of rainbow trout was performed by a two-step collagenase perfusion procedure which is well established for rainbow trout (Nabb et al., 2006; Fay et al., 2015). Since carp liver (more precisely the hepatopancreas), in contrast to rainbow trout, does not possess a central portal vein, a different approach for liver cell isolation had to be used. In previous studies with common carp, an in vitro digestion method was used to isolate hepatocytes (Cowan-Ellsberry et al., 2008; Dyer et al., 2008). Here, we adopted the method of Segner et al. (1993) who used a two-step collagenase perfusion of carp liver via the Arteria coeliaca for cell isolation. With the perfusion method, the percentage of pancreatic cells in the final cell suspension is typically <5% (Vogt and Segner, 1997). Cryopreservation and thawing procedures applied for hepatocytes of both species were based on the standard protocols for rainbow trout (Fay et al., 2014a; Fay et al., 2014b). The viability of the cells was determined by means of the trypan blue exclusion assay and counting of stained and non-stained cells in a hemocytometer. The carp and trout primary hepatocyte suspensions used in the present study were aliquots of the in vitro preparations used in a previous study investigating the in vitro metabolite patterns of MXC (Bischof et al., 2016). A detailed description of the methods and materials used for the preparation of hepatocyte suspensions including the procedures for cell isolation, cryopreservation, and thawing, as well as cell counting, diluting to the target cell concentration, and heat-inactivating can therefore be taken from Bischof et al. (2016) and from the cited publications.
In vitro hepatocyte clearance assays
The in vitro hepatocyte clearance experiments were performed in a substrate depletion approach according to the methods previously published by Fay et al. (2014b). These methods are in accordance with the procedures provided in the OECD TG 319A on the performance of in vitro hepatocyte clearance assays. In the present study, several in vitro runs with MXC and BaP using suspensions of either freshly isolated or cryopreserved hepatocytes from common carp and rainbow trout were carried out. For the different in vitro runs non-pooled hepatocytes were used, i.e. hepatocytes from different individual fish. For each run, triplicates of live cells (3 × 1 ml at nominal 2 × 106 cells/mL) and heat-inactivated cells as controls were dosed with the test chemical dissolved in acetonitrile (ACN) at the targeted concentration (2 µM for MXC and 0.5 µM for BaP) and incubated for 4 h at 15 °C (trout) or 20 °C (carp). MXC and BaP reactions were run side by side in the same in vitro run using the same hepatocyte suspensions. The reactions were stopped after 5, 15, 20, 30, 60, 120, 180, and 240 min by pipetting aliquots (100 µL) of the cell suspensions into centrifuge tubes containing 400 µl methylene chloride (DCM) spiked with MXC-d6 at 0.4 µM (MXC reactions) or ice-cold ACN (BaP reactions). Samples were vortexed and centrifuged at 20,000 g for 10 min. The organic phase was then transferred to analytical vials and subjected to chemical analyses.
Table 1 provides an overview of the in vitro hepatocyte clearance experiments conducted in this study. In addition, characteristics of the donor fish such as the average body weights and GSI values as a measure of sexual maturity, and relevant information about the success of hepatocyte isolation such as the average cell yields and viabilities are specified for the different in vitro runs.
In vivo bioconcentration tests
In vivo BCF values for MXC and BaP in carp and trout were determined in bioconcentration tests with both species in accordance to OECD TG 305 (OECD, 2012). The studies were approved by the State Agency for Nature, Environment and Consumer Protection (LANUV) North Rhine-Westphalia. The fish were exposed to a single concentration of both test items in a flow-through system for 35 days. No control groups were used. Dosing was performed by a solid-phase desorption system which was integrated in the fresh water supply allowing for a constant delivery of test concentrations to the fish chambers. The procedure of column generated concentrations was described in detail by Schlechtriem et al. (2017). For the present study, two packed columns containing each 500 g Florisil® loaded with 200 mg of either MXC or BaP were used for each fish chamber. The uptake (exposure) phase of the study was followed by a 35 days depuration phase in uncontaminated water. The flow-through rate was maintained at 38.6 L/h for carp and 30.1 L/h for trout throughout the test. On days 0, 7, 14, 21, 28, 31, and 35 of the uptake phase and on days 4, 10, 21, and 35 of the depuration phase each time four fish from each test species were sampled for chemical analysis. Additional 2-4 fish were sampled each, at the start and at the end of the exposure period, as well as at the end of the depuration period for lipid analysis. The total lipid content of the fish was measured individually by gravimetric analysis according to Smedes (1999).
Water samples (400 ml) were taken from the test chambers at least three times per week. Preparation for chemical analysis was performed by liquid-liquid extraction using n-hexane (10 ml) containing deuterated MXC (35 ng). The purified organic phase was subdivided into two equal parts and evaporated to dryness under a stream of nitrogen. One part of the residue was dissolved in 200 µL DCM for MXC analysis. The other part was mixed with 1 ml ACN: water (8:2, v/v) and subjected to BaP analysis. Whole fish samples were prepared for chemical analysis individually. After grinding the tissue with the addition of dry ice in a Thermomix™ 31 (Vorwerk), the finely powdered homogenate was lyophilized using an AdVantage freeze dryer (Ismatec). Aliquots of the dry samples (0.5 g) were spiked with deuterated internal standards (5 ng MXC-d6 and 0.2 ng BaP-d12) and extracted three times with acetone: DCM (1:1, v/v) (10 ml). The pooled extracts were then extracted with ultra-pure water (45 ml) and mixed with sodium chloride (5 g) to remove acetone and matrix. The organic phase was concentrated (0.5 ml) and dissolved in cyclohexane: DCM (1:1, v/v) (4.2 ml). This was subjected to gel permeation chromatography (GPC) (GILSON system equipped with pump 307, syringe pump 402, GX-271 liquid handler, 508 interface module, and Trilution® LC software; column i. d. 1.5 cm, material Bio-Beads S-X3, 200–400 mesh, Bio-Rad, flow rate 2 ml/min). Eluates containing MXC (26–31 min retention time) and BaP (32–38 min retention time) were evaporated to dryness and dissolved in hexane (1 ml) and toluol (50 µL), respectively. The collected MXC extracts were further concentrated by solid-phase extraction (SPE, Sep-Pak® Vac 12cc, 2 g, silica cartridges, Waters) using DCM: n-hexane (50:50, v/v) as eluent. Eluent and hexane were used to equilibrate and condition the column before sample loading and subsequent washing with DCM: n-hexane (30:70, v/v). The eluate containing MXC was dried down and reconstituted in toluol (0.5 ml).
Chemical analysis
MXC in the organic phase of samples from the in vitro incubations and in DCM extracts of water samples from the in vivo bioconcentration tests were analyzed by gas chromatography-mass spectrometry (GC-MS). The GC-MS system consisted of a HP5890 series II GC coupled to a HP5972 mass sensitive detector (Hewlett-Packard). Separations were performed on 5% phenyl polysilphenylene-siloxane capillary columns (BPX-5, 30 m length x 0.25 mm i. d, 0.25 µm df, SGE). Analysis of BaP in ACN extracts of samples from the in vitro incubations and in ACN/water extracts of water samples from the in vivo bioconcentration tests was carried out by liquid chromatography (LC)-fluorescence detection on a HPLC Summit system equipped with an Ultimate 3,000 pump and a RF 2000 fluorescence detector (Dionex). Separations were performed on a Luna C18 100A column (Phenomenex, 250 mm × 4.6 mm, 5 μm particle size). Toluol extracts of fish samples from the in vivo bioconcentration studies were analyzed for MXC and BaP by GC-MS using a Varian Bruker 450-GC coupled to Varian Bruker 320-MS. Separations were performed on 1,4-bis(dimethylsiloxy)phenylene dimethyl polysiloxane capillary columns (Rxi®-5Sil MS, 20 m length x 0.18 mm i. d, 0.18 µm df, Restek). A detailed description of the instrument conditions used for GC-MS and LC-fluorescence analysis is provided in appendices (Supplementary Appendix A.1).
In vitro intrinsic clearance calculation
Measured values of parent chemical concentration were log10-transformed and plotted against time. A linear regression was performed on the substrate depletion data to estimate a first order elimination rate constant (k; equal to −2.3 × slope) with units of inverse time (1/h). In vitro intrinsic clearance values (CLin vitro, int, mL/h/106 cells) were then obtained by dividing k by 2 × 106 cells/mL, the nominal concentration of viable hepatocytes in the in vitro experiment (Fay et al., 2014b).
Extrapolation of kB and prediction of BCF
The IVIVE-BCF model of Nichols et al. (2013) for rainbow trout was applied. In contrast to previous approaches, this model predicts the BCF for a reference rainbow trout (10 g fish held at 15 °C and with a whole body lipid content of 5%), accounting for the scenario in most of the in vivo BCF tests. With algorithms that adjust for user-specified changes, the new model provides flexibility with respect to settings of fish holding temperature, lipid content, and body mass, as well as several other extrapolation factors. The model was used in a study by Fay et al. (2014b) investigating the intra- and interlaboratory reliability of the trout hepatocyte assay, and was shown to deliver improved BCF estimates when in vitro biotransformation rate data were incorporated. A drawback is that the Nichols et al. (2013) model is not parameterized for carp. We introduced some modifications to adopt the trout model for carp. Instead of the specific fractional liver weight of 0.015 g/kg for trout, for carp we used 0.0174 g/kg as assumed in the carp extrapolation model of Cowan-Ellsberry (Cowan-Ellsberry et al., 2008). In the same way, the equation used for estimating fish growth (kG) was adopted for carp. For trout, fish growth was calculated according to the algorithm given in the Nichols model. Furthermore, the fish holding temperature was changed from 15 to 21°C for carp and to 12°C for trout, representing the actual conditions in the in vivo tests carried out as part of this study. The settings for lipid content (9.08% for trout and 9.57% for carp) and body mass (18.5 g for trout and 44.6 g for carp) for the modeled fish were adapted according to the values measured in the experimental fish. A hepatocellularity value of 510 × 106 hepatocytes/g liver was used for both species to extrapolate CLin vitro, int to the whole liver. This hepatocellularity value has been determined empirically for rainbow trout liver (Fay et al., 2014a) and was used in a recent application of the Nichols prediction model (Fay et al., 2014b). However, it should be kept in mind that hepatocellularity can strongly vary with the physiological conditions, as the size of fish hepatocytes depends on the amount of stored energy reserves (Segner and Braunbeck, 1990). An important parameter in the extrapolation model is the unitless fu parameter which accounts for the difference of chemical binding to proteins in vitro and in vivo. While some studies used a default fu of 1, in the present study we used a fu estimated by QSAR. Due to the lack of species-specific information, the default algorithms for calculating fu as given by the Nichols model were also used for carp. All other equations and parameters were used as suggested by Nichols et al. (2013).
In vivo bioconcentration test derived kB and BCF
In addition to the in vitro biotransformation assay data, also the in vivo BCF test data were used to determine kB values for carp and trout. The BCF values were calculated as steady-state bioconcentration factor (BCFSS) according to OECD TG 305 by dividing the mean of measured concentrations in fish (Cf) at steady-state through the time-weighted average (TWA) concentration in water during the uptake phase. The decrease of test substance during the depuration phase was calculated by fitting an exponential function (Cf = Cf,0 × e(−kT×t)), where Cf,0 is the concentration at the start of the depuration period to the concentrations measured in fish, resulting in the depuration rate constant (kT) (OECD, 2012). Due to unexpectedly rapid uptake kinetics for both test substances, it was not possible to calculate the uptake rate constant (k1) based on the measured concentrations in fish during the uptake phase. For in vivo kB estimation, the k1 values were therefore estimated using the kinetic mass balance modelling method of Arnot et al. (2008). The kB values were then calculated as kB = kT−(k2+kE + kG), where k2, kE, and kG are rate constants (1/d) representing chemical elimination from the organism via the respiratory surface, fecal egestion, and growth dilution. The model was parameterized maximizing the available measured physiological (e.g. whole body wet weight, lipid content) and exposure (e.g. temperature, dissolved oxygen concentration) data from the laboratory BCF study (Arnot et al., 2008). Details of the evaluation of the in vivo data for BCF calculation and of the method used for modeling the BCF parameters are given in appendices (Supplementary Appendix A.2). To distinguish the kB and BCF values predicted based on the in vitro biotransformation data, in the following the kB and BCF values derived from measured in vivo data are referred to as experimentally determined or in vivo kB (kB, in vivo estimate) values and experimentally determined or measured BCF (BCF, measured) values.
Statistics
Differences between groups were analyzed as appropriate by Student’s t-test, Mann-Whitney Rank-sum test, One-way ANOVA, or Kruskal–Wallis One-way ANOVA on ranks followed by an all pairwise multiple comparison procedure using SigmaStat® 3.5 Software (Systat).
Results
Yield and viability of hepatocytes
Cell isolations performed by the two-step collagenase perfusion technique were successful for both species. They resulted in comparable average cell yields of 63.4 ± 13.2 and 81.4 ± 31.2 × 106 viable cells per g liver for carp and trout, respectively (Table 1). The average percent cell viability of freshly isolated hepatocyte suspensions was significantly greater for trout compared to carp, with 97.1 ± 1.99% and 80.6 ± 7.07%, respectively. In contrast, the cell viabilities for suspensions of cryopreserved hepatocytes after thawing were comparable between carp and trout, with on average 79.8 ± 9.59 and 75.7 ± 6.44%, respectively. In addition, for both species, a comparable percentage of viable cells could be recovered after thawing in relation to the number of cells initially cryopreserved with 25.8 ± 6.28% and 22.6 ± 7.07% for carp and trout, respectively.
In vitro intrinsic clearance
Figure 1 shows the substrate depletion curves for MXC and BaP in carp and trout hepatocytes. The values were normalized to the first sampling time point for better comparability between the experiments. For both species, significant depletion of MXC and BaP was observed in freshly isolated and in cryopreserved hepatocytes. The depletion rates were comparable between freshly isolated and cryopreserved cells and log-linear, confirming first order kinetics. In cryopreserved carp hepatocytes the biotransformation activity slowed down toward the end of the incubation period. Therefore, for these experiments CLin vitro, int rates were determined excluding data from the 4 h sampling time point using only the linear portion of the curve. Within the heat-inactivated controls (“dead” cells), the loss of parent chemical in no case exceeded 5% of the clearance rate of the live cells. The standard deviation between the three technical replicates of an in vitro run (intra-assay variability, error bars in Figure 1), generally tended to be higher for BaP compared to MXC. This finding might be attributed largely to the absence of an internal standard for BaP, which could compensate for the variability of the measured concentrations resulting from sample preparation and analysis.
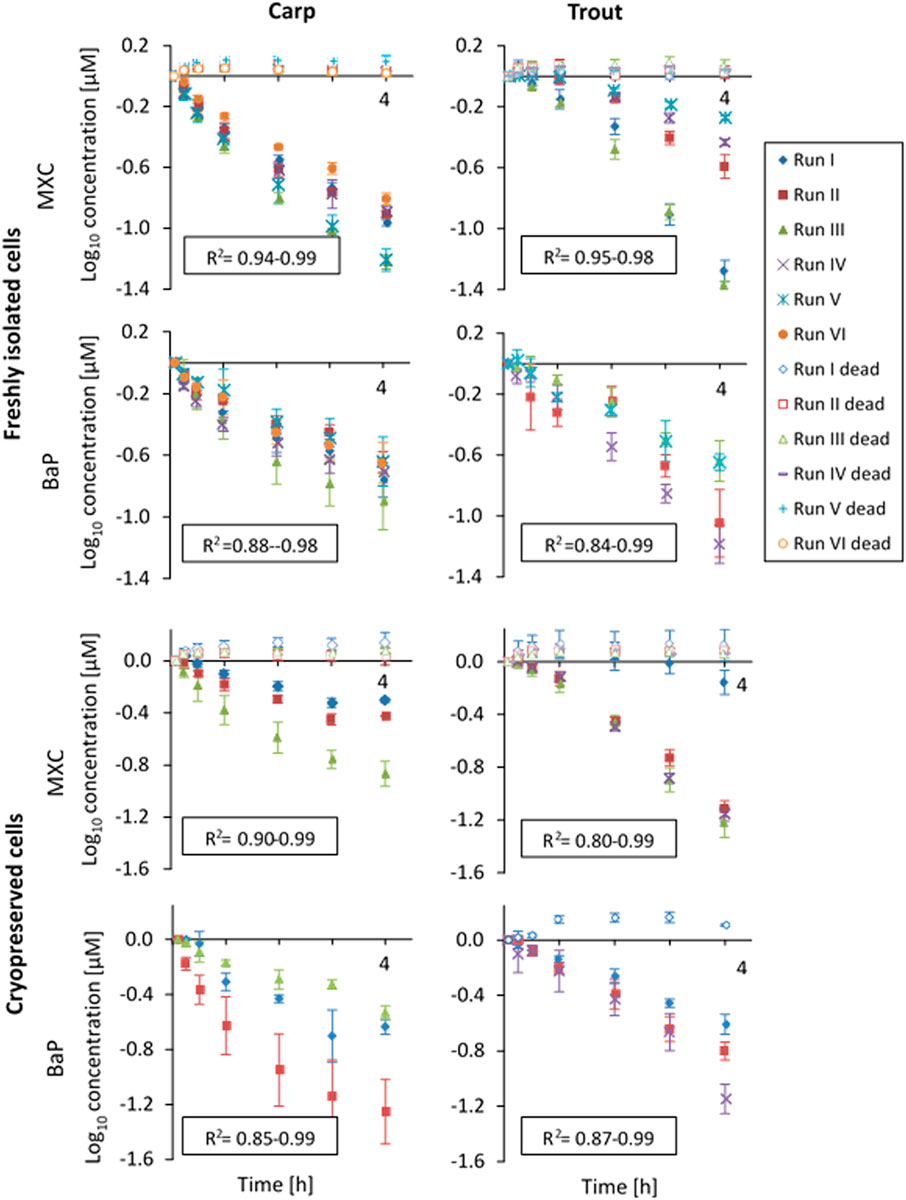
FIGURE 1. In vitro substrate depletion curves (measured concentrations were log10-transformed and normalized to the first sampling time point) from biotransformation rate assays with freshly isolated or cryopreserved common carp and rainbow trout hepatocytes. The cells were incubated over a 4-h-period (x-axis). Data points represent average substrate concentrations for each experimental run over the 4 h incubation period. Error bars indicate the standard deviation between triplicates. R2-values of the linear regressions are given for live replicates as a measure of goodness of fit. “Dead” indicates control values obtained with heat-inactivated hepatocytes.
Average CLin vitro, int values of MXC and BaP calculated for the independent biological replicates of the in vitro runs are plotted in Figure 2. The values obtained for the same fish species and the same chemical varied up to a factor of 5. This variability may confound existing inter-species differences.
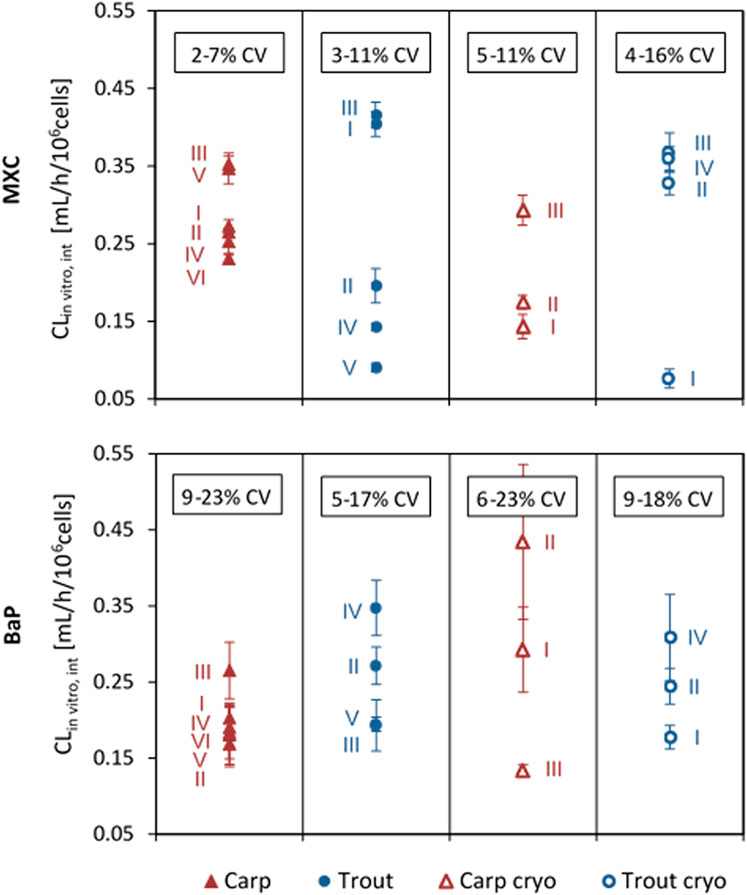
FIGURE 2. In vitro intrinsic clearance (CLin vitro, int) of methoxychlor (MXC) and benzo[a]pyrene (BaP) in common carp and rainbow trout hepatocytes. Values are normalized to 106 cells, using the nominal test concentration of 2 × 106 cells/mL. Values represent the average CLin vitro, int from technical triplicates for each run. Error bars indicate the standard deviation (SD) between triplicates. The range of total coefficient of variation (%CV) values obtained for the triplicates in the different groups is provided in the boxes.
Overall mean CLin vitro, int rates determined for MXC and BaP in carp and trout hepatocytes are summarized in Table 2. Mean measured CLin vitro, int values of hepatocytes of both species were in the range of 0.198 and 0.287 ml/h/106 cells. Significant differences of MXC and BaP clearance rates were found neither between the two fish species nor between freshly isolated and cryopreserved cells. Also the mean CLin vitro, int values of the two test chemicals did not differ significantly, except of freshly isolated hepatocytes of carp (Table 2). The in vitro intrinsic clearance rates differed between carp and trout hepatocytes with respect to the observed variabilities of the mean CLin vitro, int rates: The inter-assay variability (variability between biological replicates) was mostly higher for isolated trout hepatocytes than for carp hepatocytes.
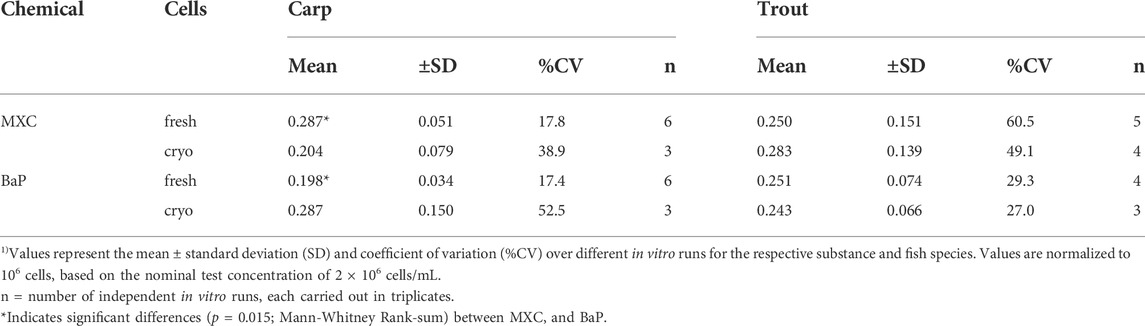
TABLE 2. Mean in vitro intrinsic clearance (CLin vitro, int, mL/h/106 cells) 1) of methoxychlor (MXC) and benzo[a]pyrene (BaP) in freshly isolated and cryopreserved common carp and rainbow trout hepatocytes.
In vivo bioconcentration test
The results of the in vivo bioconcentration studies with rainbow trout and common carp are presented in detail in appendices (Supplementary Appendix A.2). In brief, common carp and rainbow trout were exposed simultaneously to 6.94 ± 1.66 and 11.45 ± 1.48 ng/L MXC, and 1.12 ± 0.34 and 1.62 ± 0.44 ng/L BaP (time-weighted average concentration ±SD, Supplementary Table A.2 6), respectively. Exposure concentrations below the solubility limit of the test items in water could be guaranteed due to the specific character of the column associated dosing system. The observed variabilities in the measured water concentrations (23.8 and 12.8% for MXC and 30.4 and 27.8% for BaP in carp and trout experiments, respectively), however, partly exhibited the allowed range of 20% according to OECD TG 305. This can be attributed to the very low test concentrations in combination with the long exposure period. With respect to water temperature (20.7 ± 0.08 for carp and 12.2 ± 0.07 for trout, average ± SD, Supplementary Figure A.2 1 and Supplementary Table A.2 1), dissolved oxygen concentration (77 ± 12.1% for carp and 91 ± 4.13% for trout, average ± SD, Supplementary Table A.2 2), and mortality (<10% at the end of the test) the test conditions met the OECD requirements for a valid test. At the end of the uptake period, mean lipid contents of 9.57 ± 1.48% and 9.08 ± 1.32% were measured for carp and trout, respectively (Supplementary Table A.2 4). Average body weights of carp and trout determined at the end of uptake were 44.6 ± 13.8 and 18.5 ± 7.10 g, respectively (Supplementary Table A.2 5).
In vivo kB and BCF values measured for MXC and BaP in the two test species are summarized in Tables 3 and 4. Detailed data on the relevant bioconcentration parameters are contained in appendices (Supplementary Appendix A.2, Supplementary Table A.2 7, Supplementary Figure A.2 3, Supplementary Table A.2 8). Experimentally determined kB values for carp compared to trout were 0.48 and 0.40 1/d for BaP as well as 0.21 and 0.10 1/d for MXC, respectively, with calculated uncertainty factors (95% probability confidences factors of in vivo kB values, see Table 3) ranging from 2.2 to 3. Significant species differences of in vivo kB values are thus not indicated for both chemicals. The experimentally determined BCF values differed significantly between the two test species: rainbow trout showed significantly higher BCF values than common carp in the case of MXC (2,686 compared to 1,188 L/kg), whereas the situation was opposite in the case of BaP: trout displayed significantly lower BCF values for BaP than did carp (77.7 compared to 140 L/kg). Measured BCF values differed significantly between MXC and BaP, with MXC showing much higher bioaccumulation than BaP in both species.
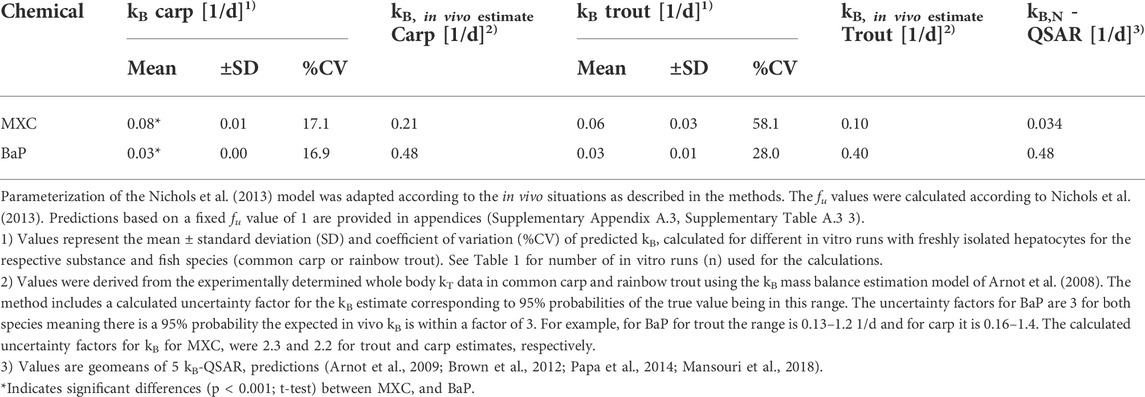
TABLE 3. In vitro − in vivo extrapolated whole body biotransformation rate constants (kB) of methoxychlor (MXC) and benzo[a]pyrene (BaP) vs in vivo derived kB (kB, in vivo estimate) values.
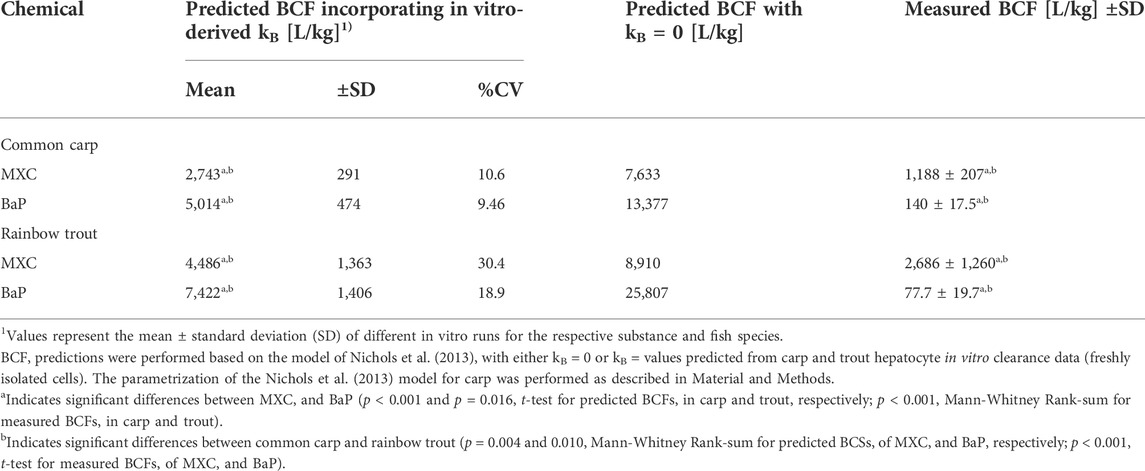
TABLE 4. Comparison of predicted and measured bioconcentration factor (BCF) values of methoxychlor (MXC) and benzo[a]pyrene (BaP) in common carp and rainbow trout.
In vitro-in vivo extrapolation of kB values and comparison to in vivo-derived KB values
The CLin vitro, int values obtained from the in vitro assays with trout and carp hepatocytes were incorporated into the prediction model of Nichols et al. (2013) to predict the in vivo kB values of MXC and BaP. Mean kB values predicted from the clearance rates of freshly isolated cells of both species are presented in Table 3. The values ranged from 0.03 to 0.08 1/d depending on the fish species and the chemical. The variability in the extrapolated kB values was reduced compared to the %CV of CLin vitro, int rates. Overall, predicted kB values were lower than experimentally determined in vivo kB values (Table 3), what probably relates to the fact that the latter values reflect whole body biotransformation rather than liver biotransformation only.
Similar to the measured CLin vitro, int data, the extrapolated kB values of MXC and BaP were not significantly different between carp and trout. This agrees with the findings from the in vivo tests: the kB values of MXC and BaP derived from the in vivo experiment were not significantly different between carp and trout (Table 3).
When comparing the predicted kB values for the two test compounds, the extrapolations based on data of the carp hepatocytes predicted a significantly higher biotransformation rate for MXC than for BaP. Such a compound difference was not predicted for rainbow trout.
In vitro − in vivo extrapolations of kB values were also carried out for CLin vitro, int data derived from experiments with cryopreserved hepatocytes. The estimated kB values were not statistically different from those obtained with freshly isolated cells. The results are therefore provided in appendices (Supplementary Appendix A.3, Supplementary Table A.3 1).
Table 3 also compares the new IVIVE kB values and empirical in vivo kB estimates (obtained from the in vivo depuration experiments) with the geomean of 5 QSAR predictions (Arnot et al., 2009; Brown et al., 2012; Papa et al., 2014; Mansouri et al., 2018). For MXC, the in vitro derived kB estimates (from liver tissue only) are within a factor of about 2-3 of both the in vivo and in silico kB estimates. The in vitro values are slightly slower than the in vivo values and slightly faster than the QSAR predictions. For kB BaP, the in vitro estimates (from liver tissue only) are slower than the in vivo and in silico estimates. Notably the BaP in vitro derived kB estimates are about 1 order of magnitude slower than the in vivo and in silico kB estimates, which are in very good agreement with each other. Collectively the in vitro, in vivo and in silico data demonstrate the utility of a weight-of-evidence approach for characterizing variability and uncertainty in kB that can be considered when parameterizing bioaccumulation models.
In vitro-based prediction of BCF values and comparison to in vivo-derived BCF values
The BCF values for carp and trout resulting from the model predictions are shown in Table 4. Assuming no biotransformation (kB = 0), predicted BCF values for carp were 7,633 L/kg for MXC and 13,377 L/kg for BaP. In the case of trout, predicted BCF values with kB = 0 ranged from 8,910 L/kg for MXC to 25,807 L/kg for BaP. Thus in both species BaP was predicted to have more bioaccumulation potential than MXC reflecting the higher Kow of the former.
When the predicted kB values were incorporated in the BCF calculations, the BCF values were, in all cases, reduced (2–3.5 fold). In contrast to the predicted kB values, the predicted BCF values differed between the two fish species: for both chemicals, the BCF values of carp were significantly lower than those of trout (2,743 and 5,014 compared to 4,486 and 7,422 L/kg for MXC and BaP, respectively). This is in accordance with the findings from the in vivo test for MXC (lower BCF values for carp compared to trout) but not for BaP, where higher BCF values for carp than for trout were observed. Predicted BCF values differed significantly between MXC and BaP, with BaP showing higher bioaccumulation than MXC in both species. This is in contrast to the findings from the in vivo test, where MXC showed significantly higher bioaccumulation than BaP in both fish species.
The BCF predictions obtained for cryopreserved cells yielded comparable results to those for freshly isolated cells and can be found in appendices (Supplementary Appendix A.3 and Supplementary Table A.3 2).
Discussion
The purpose of this study was to explore the inter-species applicability of the in vitro fish hepatocyte assay as a source of biotransformation rate data in alternative testing strategies for bioconcentration assessment in fish. More specifically, we investigated whether the well-established in vitro protocols for a biotransformation assay with hepatocytes of the cold water species, rainbow trout, can be transferred to the warm water species, common carp, and if the in vitro assays are able to predict in vivo species differences of BCF values.
Technical transferability of the in vitro assay protocol
The technical transferability is not a trivial aspect since trout and carp differ substantially in their liver morphology. In contrast to salmonids, the non-capsule liver of carp is diffuse and intertwined with intestines, and there is not a single, large portal vein but many small venous connections between intestine and liver (Segner, 1998). It is therefore not possible to directly transfer the liver perfusion technique as it is routinely used for the isolation of hepatocytes from rainbow trout (Fay et al., 2015). As alternative to perfusion-based cell isolation, previous studies with carp hepatocytes relied on the ex vivo digestion of carp liver tissue with collagenase and trypsin (Cowan-Ellsberry et al., 2008; Dyer et al., 2008). However, the in situ digestion gives lower cell yields and is comparatively stressful for the cells, what may result in lower cell quality (Braunbeck and Segner, 2000). In the present study, we used a carp liver perfusion technique via the Arteria coeliaca according to Segner et al. (1993). This enabled us to isolate carp liver cells under comparable conditions as it is done with trout hepatocytes.
The cell yields obtained in the present study for carp liver (viable cells per g liver on average: 63.4 ± 13.2 × 106) were comparable to the cell yields for rainbow trout liver in the present study (viable cells per g liver on average: 81.4 ± 31.2 ×106) and to the values for trout liver of previous publications (Han et al., 2007; Mingoia et al., 2010; Fay et al., 2014a, 2017; Fay et al., 2014b). The carp hepatocyte yield of our study was also in close agreement to the cell yield for carp reported by Dyer et al. (Dyer et al., 2008, 2009) of 84 ± 21 × 106 hepatocytes per Gram liver, and it corresponds to the data of Smeets et al. (1999) of 150–400 × 106 cells per carp (our study: 402 × 106 viable cells per fish after multiplication with the mean carp liver weight). The in vitro substrate depletion measurements worked well with the isolated carp hepatocytes. Substrate depletion followed first order kinetics in carp hepatocyte suspensions, as it did with trout liver cells, as evident from the R2-values of the log-linear decay curves of 0.85–0.99 for carp and 0.80–0.99 for trout.
A further indicator of the quality of in vitro hepatocyte preparations can be the intra- and inter-assay variability. Intra-assay variabilities of the carp hepatocyte assay were comparable to those of the trout hepatocyte assay (coefficients of variation (CV) between replicates of an in vitro run for MXC: 2–11% carp and 3–16% trout, and for BaP: 6–23% carp and 5–18% trout). In previous studies, for the rainbow trout hepatocyte assay up to 50% variability between cell preparations from different individual fish were reported (Fay et al., 2017). Depending on the test chemical under investigation, an inter-assay variability of up to 30% was determined for rainbow trout (Mingoia et al., 2010; Fay et al., 2014b). The variability of CLin vitro, int across different laboratories was determined with up to 61% in a recent study using the trout hepatocyte assay (Fay et al., 2014b). Using freshly isolated hepatocytes of individual rainbow trout to measure in vitro metabolic rate values of difficult test compounds, Kropf et al. (2020) obtained inter-assay variabilities of 11–59%. In our study, measured total coefficients of variation (CV) of CLin vitro, int ranged from 17.8 to 60.5% for MXC and from 17.4 to 52.5% for BaP. Thus, the inter-assay variabilities observed for the trout and carp hepatocyte assay in the present study were within the range of the results previously reported for trout.
Interestingly, a smaller variability of CLin vitro, int rates between independent biological replicates was observed for hepatocyte suspensions from carp compared to trout, suggesting that inter-individual differences in the biotransformation potential may be less pronounced for carp in comparison to trout. This observation is consistent with the findings from a previous feeding study on common carp and rainbow trout (Bischof et al., 2016) which was performed with the same batches of fish as used for cell isolations in the present study. It was found that compared to trout, the concentration of MXC residues and the proportion of formed MXC metabolites in liver and fillet of carp varied less between different animals. More research is needed to evaluate whether the observed differences between carp and trout are the result of a different homogeneity of the particular fish strain or batch, or truly can be attributed to species-specific differences. If different fish species may be more or less subject to inter-individual differences in the metabolic potential, such inter-species differences could be an important criterion for the choice of fish species used for the hepatocyte assay.
A prerequisite for the commercial application and widespread use of primary fish hepatocytes for bioaccumulation assessment is the availability of a cryopreservation procedure that enables the storing and shipping of well-characterized batches of high quality hepatocytes. As demonstrated earlier, rainbow trout hepatocytes can be successfully cryopreserved without loss of biotransformation activity (Mingoia et al., 2010). The findings from the present study indicate that established protocols for trout hepatocyte cryopreservation can be successfully transferred to carp hepatocytes. Cell recoveries obtained with cryopreserved carp hepatocytes after thawing (percentage of viable cells in relation to the number of cells initially cryopreserved) were similar to those for trout and well in accordance to previously reported recoveries for trout of 22–37% (Fay et al., 2014a; Fay et al., 2014b). Also with respect to the cell viability, no significant differences were found between cryopreserved hepatocytes from carp and trout. Only towards the end of the in vitro incubation period, the biotransformation activity of cryopreserved and thawed carp hepatocytes slowed down. This was not the case with the cryopreserved and thawed trout liver cells.
In conclusion, the results of this study suggest that the standard protocols for the substrate depletion assay and the cryopreservation techniques as established for trout hepatocytes can be transferred to carp hepatocytes without the need for substantial modifications.
Inter-species differences of biotransformation rates: Can they be predicted from in vitro assays?
Biotransformation rates vary between species (Schultz and Hayton, 1999; Han et al., 2007; Connors et al., 2013; Hutchinson et al., 2014). The question is whether in vitro preparations and the related in vitro-in vivo predictions reflect such species differences. Metabolic enzyme activities can differ significantly between mammalian and piscine in vitro systems (Nabb et al., 2006; Han et al., 2009). For fish, it has been shown that in vitro liver preparations are well able to unravel species differences in the patterns of the produced metabolites (Segner and Cravedi, 2001; Bischof et al., 2016). Also differences of biotransformation rates have been found between in vitro hepatic systems of different fish species (Fitzsimmons et al., 2007). Schultz and Hayton (1999) found over 25-fold variation of trifluralin metabolism in liver S9 preparations of rainbow trout, bluegill (Lepomis macrochirus), fathead minnow (Pimephales), lake sturgeon (Acipenser fulvescens), gizzard shad (Dorosoma cepedianum), channel catfish (Ictalurus punctatus) and largemouth bass (Micropterus salmoides). Roberts et al. (2011) investigated the in vitro metabolism of eleven individual polybrominated diphenyl ethers (PBDE) in three fish species and found that metabolite formation rates in carp liver microsomes were in general 10 to 100 times faster than those measured in trout and salmon. Strobel et al. (2015) reported significantly different in vitro biotransformation rates of BaP between Antarctic fish species and rainbow trout. Different in vitro biotransformation rates between rainbow trout and common carp liver microsomes were also determined for two surfactants in a study by Dyer et al. (2008).
While the available evidence suggests that in vitro liver preparations from different fish species metabolize identical chemicals at different rates, the question is whether these differences are predictive of the in vivo differences. A principal limitation in evaluating the predictivity of in vitro data is the availability of reliable in vivo data. For our test chemicals, MXC and BaP, we were not able to find BCFs of comparable quality between carp and trout. Therefore, we decided to perform an in vivo bioconcentration test according to OECD TG 305 for both test compounds and both test species. The results of the in vivo experiment revealed significant species differences of the BCF values: The measured in vivo BCF of MXC was significantly higher in trout than in carp, while the in vivo BCF of BaP showed no significant species difference. Interestingly, the species difference of the in vivo BCF values was not accompanied by a species difference of the kB values derived from the in vivo experiment.
The in vitro assay with trout and carp hepatocytes displayed no significant differences of the intrinsic clearance rates for MXC and BaP. Likewise, the kB values of BaP and MXC predicted from the results of the in vitro assays showed no significant species difference. However, the IVIVE predicted BCF values of MXC and BaP for rainbow trout and common carp differed significantly. These findings may point to a role of systemic toxicokinetic processes in determining the in vivo bioconcentration of chemicals. Therefore, the critical question with respect to the ability of in vitro assays to predict in vivo species differences of chemical bioaccumulation is probably not if the measured in vitro Clin vitro, int values differ between liver preparations of the species but if the overall extrapolation model is able to reflect the toxicokinetically relevant differences between the species. A handicap in answering this question is that the currently available IVIVE prediction models are parameterized for trout, but nor for carp. In our calculations, we tried to populate the Nichols model with carp-specific data, however, this was only partly possible, as the database of basic physiological parameters of carp is limited. This may explain why there was still a partial discrepancy between the measured and the predicted BCF values: while both measured and predicted data indicate a significantly lower bioconcentration of MXC in carp compared to trout, measured and predicted values differed for BaP: The IVIVE method predicted a significantly lower BCF of BaP in carp than in trout, whereas in the in vivo experiment carp show a significantly higher bioconcentration of BaP than trout. In this context, the findings of Laue et al. (2020) are of interest who showed that current IVIVE models tend to overpredict in vivo BCF values, and that this is not improved by changing in vitro assay conditions, by use of alternate fu values or by species matching, but seems to be an inherent problem of the existing extrapolation models. Thus, currently the ability of in vitro assays to reveal species differences in biotransformation rates appears to be related both to characteristics of the in vitro assays and the parametrization of the prediction models.
In conclusion, the findings from this study provide for the first time information on the ability of in vitro biotransformation rate assays to predict in vivo species differences of chemical bioaccumulation. It is evident that the results from this study having tested just two compounds in two species allows no conclusive statement, but the study findings highlight that the key challenge in this field is probably not so much the technical transfer of the in vitro methodology from rainbow trout to other fish species, but the requirement for improved species-specific parameterization of the IVIVE models.
Data availability statement
The original contributions presented in the study are included in the article/Supplementary Material, further inquiries can be directed to the corresponding author.
Ethics statement
The animal study was reviewed and approved by the State Agency for Nature, Environment and Consumer Protection (LANUV) North Rhine-Westphalia.
Author contributions
HJ and GK performed the chemical analysis by gas chromatography-mass spectrometry and liquid chromatography-fluorescence detection, respectively. AS performed the in vivo bioconcentration experiments with trout and carp and contributed to chemical analysis, as well as to the analysis and interpretation of the experimental data. JA performed the kinetic mass balance modelling for estimating in vivo uptake rate constants (k1) and biotransformation rate constants (kB). In addition, JA contributed to the interpretation of the results and revised the article critically for important intellectual content. IB, CS, and HS contributed substantially to the concept and design of the research, the interpretation of the results and to the writing of the manuscript. IB performed the in vitro biotransformation assays and supervised the conduction of the fish bioconcentration study.
Funding
This project was funded by the Fraunhofer-Gesellschaft, Germany and by Stiftung 3R, Munsingen, Switzerland (Project 141–14).
Conflict of interest
JA is employed by the ARC Arnot Research and Consulting, Toronto, ON, Canada.
The remaining authors declare that the research was conducted in the absence of any commercial or financial relationships that could be construed as a potential conflict of interest.
Publisher’s note
All claims expressed in this article are solely those of the authors and do not necessarily represent those of their affiliated organizations, or those of the publisher, the editors and the reviewers. Any product that may be evaluated in this article, or claim that may be made by its manufacturer, is not guaranteed or endorsed by the publisher.
Supplementary material
The Supplementary Material for this article can be found online at: https://www.frontiersin.org/articles/10.3389/ftox.2022.1021880/full#supplementary-material
References
Arnot, J. A., and Gobas, F. A. P. C. (2003). A generic QSAR for assessing the bioaccumulation potential of organic chemicals in aquatic food webs. QSAR Comb. Sci. 22, 337–345. doi:10.1002/qsar.200390023
Arnot, J. A., and Gobas, F. A. P. C. (2006). A review of bioconcentration factor (BCF) and bioaccumulation factor (BAF) assessments for organic chemicals in aquatic organisms. Environ. Rev. 14, 257–297. doi:10.1139/a06-005
Arnot, J. A., Mackay, D., and Bonnell, M. (2008). Estimating metabolic biotransformation rates in fish from laboratory data. Environ. Toxicol. Chem. 27, 341–351. doi:10.1897/07-310R.1
Arnot, J. A., Meylan, W., Tunkel, J., Howard, P. H., Mackay, D., Bonnell, M., et al. (2009). A quantitative structure-activity relationship for predicting metabolic biotransformation rates for organic chemicals in fish. Environ. Toxicol. Chem. 28, 1168–1177. doi:10.1897/08-289.1
Arnot, J. A., and Quinn, C. L. (2015). Development and evaluation of a database of dietary bioaccumulation test data for organic chemicals in fish. Environ. Sci. Technol. 49, 4783–4796. doi:10.1021/es506251q
Bearr, J. S., Mitchelmore, C. L., Roberts, S. C., and Stapleton, H. M. (2012). Species specific differences in the in vitro metabolism of the flame retardant mixture, Firemaster® BZ-54. Aquat. Toxicol. 124-125, 41–47. doi:10.1016/j.aquatox.2012.06.006
Bischof, I., Koster, J., Segner, H., and Schlechtriem, C. (2016). Hepatocytes as in vitro test system to investigate metabolite patterns of pesticides in farmed rainbow trout and common carp: Comparison between in vivo and in vitro and across species. Comp. Biochem. Physiol. C. Toxicol. Pharmacol. 187, 62–73. doi:10.1016/j.cbpc.2016.05.003
Braunbeck, T., and Segner, H. (2000). “Isolation and cultivation of teleost hepatocytes,” in The hepatocyte review. Editors M. N. Berry, and A. M. Edwards (Dordrecht, Netherlands: Kluwer Academic Publishers), 49–72.
Brown, T. N., Arnot, J. A., and Wania, F. (2012). Iterative fragment selection: A group contribution approach to predicting fish biotransformation half-lives. Environ. Sci. Technol. 46, 8253–8260. doi:10.1021/es301182a
Connors, K. A., Du, B., Fitzsimmons, P. N., Hoffman, A. D., Chambliss, C. K., Nichols, J. W., et al. (2013). Comparative pharmaceutical metabolism by rainbow trout (Oncorhynchus mykiss) liver S9 fractions. Environ. Toxicol. Chem. 32, 1810–1818. doi:10.1002/etc.2240
Cowan-Ellsberry, C. E., Dyer, S. D., Erhardt, S., Bernhard, M. J., Roe, A. L., Dowty, M. E., et al. (2008). Approach for extrapolating in vitro metabolism data to refine bioconcentration factor estimates. Chemosphere 70, 1804–1817. doi:10.1016/j.chemosphere.2007.08.030
de Wolf, W., Comber, M., Douben, P., Gimeno, S., Holt, M., Léonard, M., et al. (2007). Animal use replacement, reduction, and refinement: Development of an integrated testing strategy for bioconcentration of chemicals in fish. Integr. Environ. Assess. Manag. 3, 3–17. doi:10.1897/1551-3793(2007)3[3:aurrar]2.0.co;2
Dyer, S. D., Bernhard, M. J., Cowan-Ellsberry, C., Perdu-Durand, E., Demmerle, S., and Cravedi, J. P. (2008). In vitro biotransformation of surfactants in fish. Part I: Linear alkylbenzene sulfonate (C12-LAS) and alcohol ethoxylate (C13EO8). Chemosphere 72, 850–862. doi:10.1016/j.chemosphere.2008.02.019
Dyer, S. D., Bernhard, M. J., Cowan-Ellsberry, C., Perdu-Durand, E., Demmerle, S., and Cravedi, J. P. (2009). In vitro biotransformation of surfactants in fish. Part II--Alcohol ethoxylate (C16EO8) and alcohol ethoxylate sulfate (C14EO2S) to estimate bioconcentration potential. Chemosphere 76, 989–998. doi:10.1016/j.chemosphere.2009.04.011
Fay, K. A., Fitzsimmons, P. N., Hoffman, A. D., and Nichols, J. W. (2017). Comparison of trout hepatocytes and liver S9 fractions as in vitro models for predicting hepatic clearance in fish. Environ. Toxicol. Chem. 36, 463–471. doi:10.1002/etc.3572
Fay, K. A., Fitzsimmons, P. N., Hoffman, A. D., and Nichols, J. W. (2014a). Optimizing the use of rainbow trout hepatocytes for bioaccumulation assessments with fish. Xenobiotica. 44, 345–351. doi:10.3109/00498254.2013.845704
Fay, K. A., Mingoia, R. T., Goeritz, I., Nabb, D. L., Hoffman, A. D., Ferrell, B. D., et al. (2014b). Intra- and interlaboratory reliability of a cryopreserved trout hepatocyte assay for the prediction of chemical bioaccumulation potential. Environ. Sci. Technol. 48, 8170–8178. doi:10.1021/es500952a
Fay, K. A., Nabb, D. L., Mingoia, R. T., Bischof, I., Nichols, J. W., Segner, H., et al. (2015). Determination of metabolic stability using cryopreserved hepatocytes from rainbow trout (Oncorhynchus mykiss). Curr. Protoc. Toxicol. 65, 4. doi:10.1002/0471140856.tx0442s65
Fitzsimmons, P. N., Lien, G. J., and Nichols, J. W. (2007). A compilation of in vitro rate and affinity values for xenobiotic biotransformation in fish, measured under physiological conditions. Comp. Biochem. Physiol. C. Toxicol. Pharmacol. 145, 485–506. doi:10.1016/j.cbpc.2006.12.011
Gobas, F. A. P. C., de Wolf, W., Burkhard, L. P., Verbruggen, E., and Plotzke, K. (2009). Revisiting bioaccumulation criteria for POPs and PBT assessments. Integr. Environ. Assess. Manag. 5, 624–637. doi:10.1897/IEAM_2008-089.1
Han, X., Nabb, D. L., Mingoia, R. T., and Yang, C. H. (2007). Determination of xenobiotic intrinsic clearance in freshly isolated hepatocytes from rainbow trout (Oncorhynchus mykiss) and rat and its application in bioaccumulation assessment. Environ. Sci. Technol. 41, 3269–3276. doi:10.1021/es0626279
Han, X., Nabb, D. L., Yang, C. H., Snajdr, S. I., and Mingoia, R. T. (2009). Liver microsomes and S9 from rainbow trout (Oncorhynchus mykiss): Comparison of basal-level enzyme activities with rat and determination of xenobiotic intrinsic clearance in support of bioaccumulation assessment. Environ. Toxicol. Chem. 28, 481–488. doi:10.1897/08-269.1
Hutchinson, T. H., Madden, J. C., Naidoo, V., and Walker, C. H. (2014). Comparative metabolism as a key driver of wildlife species sensitivity to human and veterinary pharmaceuticals. Philos. Trans. R. Soc. Lond. B Biol. Sci. 369, 20130583. doi:10.1098/rstb.2013.0583
Johanning, K., Hancock, G., Escher, B., Adekola, A., Bernhard, M. J., Cowan-Ellsberry, C., et al. (2012). Assessment of metabolic stability using the rainbow trout (Oncorhynchus mykiss) liver S9 fraction. Curr. Protoc. Toxicol. 14, 1–28. doi:10.1002/0471140856.tx1410s53
Krause, S., and Goss, K. U. (2018). In vitro- in vivo extrapolation of hepatic metabolism for different scenarios - a toolbox. Chem. Res. Toxicol. 31, 1195–1202. doi:10.1021/acs.chemrestox.8b00187
Kropf, C., Begnaud, F., Gimeno, S., Berthaud, F., Debonneville, C., and Segner, H. (2020). In vitro biotransformation assays using liver S9 fractions and hepatocytes from rainbow trout (Oncorhynchus mykiss): Overcoming challenges with difficult to test fragrance chemicals. Environ. Toxicol. Chem. 39, 2396–2408. doi:10.1002/etc.4872
Laue, H., Hostettler, L., Badertscher, R. P., Jenner, K. J., Sanders, G., Arnot, J. A., et al. (2020). Examining uncertainty in in vitro-in vivo extrapolation applied in fish bioconcentration models. Environ. Sci. Technol. 54, 9483–9494. doi:10.1021/acs.est.0c01492
Lin, J. H. (1998). Applications and limitations of interspecies scaling and in vitro extrapolation in pharmacokinetics. Drug Metab. Dispos. 26, 1202–1212.
Lombardo, A., Roncaglioni, A., Benfentati, E., Nendza, M., Segner, H., Fernandez, A., et al. (2014). Integrated testing strategy (ITS) for bioaccumulation assessment under REACH. Environ. Int. 69, 40–50. doi:10.1016/j.envint.2014.04.005
Mansouri, K., Grulke, C. M., Judson, R. S., and Williams, A. J. (2018). OPERA models for predicting physicochemical properties and environmental fate endpoints. J. Cheminform. 10, 10. doi:10.1186/s13321-018-0263-1
Mingoia, R. T., Glover, K. P., Nabb, D. L., Yang, C. H., Snajdr, S. I., and Han, X. (2010). Cryopreserved hepatocytes from rainbow trout (Oncorhynchus mykiss): A validation study to support their application in bioaccumulation assessment. Environ. Sci. Technol. 44, 3052–3058. doi:10.1021/es903909g
Nabb, D. L., Mingoia, R. T., Yang, C. H., and Han, X. (2006). Comparison of basal level metabolic enzyme activities of freshly isolated hepatocytes from rainbow trout (Oncorhynchus mykiss) and rat. Aquat. Toxicol. 80, 52–59. doi:10.1016/j.aquatox.2006.07.012
Nendza, M., Kuhne, R., Lombardo, A., Strempel, S., and Schuurmann, G. (2018). PBT assessment under REACH: Screening for low aquatic bioaccumulation with QSAR classifications based on physicochemical properties to replace BCF in vivo testing on fish. Sci. Total Environ. 616-617, 97–106. doi:10.1016/j.scitotenv.2017.10.317
Nichols, J., Erhardt, S., Dyer, S., James, M., Moore, M., Plotzke, K., et al. (2007). Use of in vitro absorption, distribution, metabolism, and excretion (ADME) data in bioaccumulation assessments for fish. Hum. Ecol. Risk Assess. Int. J. 13, 1164–1191. doi:10.1080/10807030701655897
Nichols, J., Fay, K., Bernhard, M. J., Bischof, I., Davis, J., Halder, M., et al. (2018). Reliability of in vitro methods used to measure intrinsic clearance of hydrophobic organic chemicals by rainbow trout: Results of an international ring trial. Toxicol. Sci. 164, 563–575. doi:10.1093/toxsci/kfy113
Nichols, J. W., Bonnell, M., Dimitrov, S. D., Escher, B. I., Han, X., and Kramer, N. I. (2009). Bioaccumulation assessment using predictive approaches. Integr. Environ. Assess. Manag. 5, 577–597. doi:10.1897/IEAM-2008-088.1
Nichols, J. W., Huggett, D. B., Arnot, J. A., Fitzsimmons, P. N., and Cowan-Ellsberry, C. E. (2013). Toward improved models for predicting bioconcentration of well-metabolized compounds by rainbow trout using measured rates of in vitro intrinsic clearance. Environ. Toxicol. Chem. 32, 1611–1622. doi:10.1002/etc.2219
OECD (2014). Guidance document on the validation of (quantitative) structure-activity relationship [(Q)SAR] models. Paris: Organisation for Economic Cooperation and Development, Environment Directorate. Available at https://read.oecd.org/10.1787/9789264085442-en?format=pdf.
OECD (2004). OECD principles for the validation, for regulatory purposes, of (quantitative) structure-activity relationship models. Available at https://www.oecd.org/chemicalsafety/risk-assessment/37849783.pdf.
OECD (2012). Test No. 305: Bioaccumulation in fish: Aqueous and dietary exposure. in OECD Guidelines for the Testing of Chemicals. Paris: OECD Publishing. Section 3. doi:10.1787/9789264185296-en
OECD (2018a). “Test No. 319A: Determination of in vitro intrinsic clearance using cryopreserved rainbow trout hepatocytes (RT-HEP),” in OECD Guidelines for the testing of chemicals (Paris: OECD Publishing). Section 3. doi:10.1787/9789264303218-en
OECD (2018b). “Test No. 319B: Determination of in vitro intrinsic clearance using rainbow trout liver S9 sub-cellular fraction (RT-S9),” in OECD Guidelines for the testing of chemicals (Paris: OECD Publishing). Section 3. doi:10.1787/9789264303232-en
Papa, E., van der Wal, L., Arnot, J. A., and Gramatica, P. (2014). Metabolic biotransformation half-lives in fish: QSAR modeling and consensus analysis. Sci. Total Environ. 470-471, 1040–1046. doi:10.1016/j.scitotenv.2013.10.068
Roberts, S. C., Noyes, P. D., Gallagher, E. P., and Stapleton, H. M. (2011). Species-specific differences and structure-activity relationships in the debromination of PBDE congeners in three fish species. Environ. Sci. Technol. 45, 1999–2005. doi:10.1021/es103934x
Schlechtriem, C., Bohm, L., Bebon, R., Bruckert, H. J., and During, R. A. (2017). Fish bioconcentration studies with column-generated analyte concentrations of highly hydrophobic organic chemicals. Environ. Toxicol. Chem. 36, 906–916. doi:10.1002/etc.3635
Schultz, I. R., and Hayton, W. L. (1999). Interspecies scaling of the bioaccumulation of lipophilic xenobiotics in fish: An example using trifluralin. Environ. Toxicol. Chem. 18, 1440–1449. doi:10.1002/etc.5620180714
Segner, H., Bohm, R., and Kloas, W. (1993). Binding and bioactivity of insulin in primary cultures of carp (Cyprinus carpio) hepatocytes. Fish. Physiol. Biochem. 11, 411–420. doi:10.1007/BF00004591
Segner, H., and Braunbeck, T. (1990). Adaptive changes of liver composition and structure in golden ide during winter acclimatization. J. Exp. Zool. 255, 171–185. doi:10.1002/jez.1402550206
Segner, H., and Cravedi, J. P. (2001). Metabolic activity in primary cultures of fish hepatocytes. Altern. Lab. Anim. 29, 251–257. doi:10.1177/026119290102900321
Segner, H. (1998). Isolation and primary culture of teleost hepatocytes. Comp. Biochem. Physiology Part A Mol. Integr. Physiology 120, 71–81. doi:10.1016/s1095-6433(98)10012-0
Smedes, F. (1999). Determination of total lipid using non-chlorinated solvents. Analyst 124, 1711–1718. doi:10.1039/a905904k
Smeets, J. M., Rankouhi, T. R., Nichols, K. M., Komen, H., Kaminski, N. E., Giesy, J. P., et al. (1999). In vitro vitellogenin production by carp (Cyprinus carpio) hepatocytes as a screening method for determining (anti)estrogenic activity of xenobiotics. Toxicol. Appl. Pharmacol. 157, 68–76. doi:10.1006/taap.1999.8663
Strobel, A., Burkhardt-Holm, P., Schmid, P., and Segner, H. (2015). Benzo(a)pyrene metabolism and EROD and GST biotransformation activity in the liver of red- and white-blooded antarctic fish. Environ. Sci. Technol. 49, 8022–8032. doi:10.1021/acs.est.5b00176
Vogt, G., and Segner, H. (1997). Spontaneous formation of intercellular bile canaliculi and hybrid biliary-pancreatic canaliculi in co-culture of hepatocytes and exocrine pancreas cells from carp. Cell. Tissue Res. 289, 191–194. doi:10.1007/s004410050865
Weisbrod, A. V., Burkhard, L. P., Arnot, J., Mekenyan, O., Howard, P. H., Russom, C., et al. (2007). Workgroup report: Review of fish bioaccumulation databases used to identify persistent, bioaccumulative, toxic substances. Environ. Health Perspect. 115, 255–261. doi:10.1289/ehp.9424
Keywords: rainbow trout, common carp, bioaccumulation, biotransformation, hepatocytes, in vitro assay
Citation: Bischof I, Arnot JA, Jürling H, Knipschild G, Schlechtriem C, Schauerte A and Segner H (2022) In vitro biotransformation assays using fish liver cells: Comparing rainbow trout and carp hepatocytes. Front. Toxicol. 4:1021880. doi: 10.3389/ftox.2022.1021880
Received: 18 August 2022; Accepted: 05 September 2022;
Published: 23 September 2022.
Edited by:
Gabino Garrido, Catholic University of the North, ChileReviewed by:
Ramon Lavado, Baylor University, United StatesMarkus Brinkmann, University of Saskatchewan, Canada
Copyright © 2022 Bischof, Arnot, Jürling, Knipschild, Schlechtriem, Schauerte and Segner. This is an open-access article distributed under the terms of the Creative Commons Attribution License (CC BY). The use, distribution or reproduction in other forums is permitted, provided the original author(s) and the copyright owner(s) are credited and that the original publication in this journal is cited, in accordance with accepted academic practice. No use, distribution or reproduction is permitted which does not comply with these terms.
*Correspondence: Ina Bischof, aW5hLmJpc2Nob2ZAaW1lLmZyYXVuaG9mZXIuZGU=