- 1Institute of Environmental Health Sciences, Wayne State University, Detroit, MI, United States
- 2Department of Chemistry, Wayne State University, Detroit, MI, United States
- 3Department of Pharmaceutical Sciences, College of Pharmacy, Wayne State University, Detroit, MI, United States
- 4Department of Pharmacology, School of Medicine, Wayne State University, Detroit, MI, United States
Per- and polyfluoroalkyl substances (PFAS) are ubiquitously found in the environment due to their widespread commercial use and high chemical stability. Humans are exposed primarily through ingestion of contaminated water and food and epidemiological studies over the last several decades have shown that PFAS levels are associated with adverse chronic health effects, including cardiometabolic disorders such as hyperlipidemia and non-alcoholic fatty liver disease. Perhaps the most well-established effects, as demonstrated in animal studies and human epidemiological studies, are the metabolic alterations PFAS exposure can lead to, especially on lipid homeostasis and signaling. This altered lipid metabolism has often been linked to conditions such as dyslipidemia, leading to fatty liver disease and steatosis. Western diets enriched in high fat and high cholesterol containing foods may be an important human exposure route of PFAS and may also act as an important modulator of associated toxicities. In fact, the chemical structure of PFAS resemble fatty acids and may activate some of the same signaling cascades critical for endogenous metabolism. In this review we aim to outline known dietary exposure sources of PFAS, describe the detrimental metabolic health effects associated with PFAS exposure, and focus on studies examining emerging interaction of dietary effects with PFAS exposure that further alter the dysregulated metabolic state.
Introduction
Recently, per- and polyfluoroalkyl substances (PFAS) have become a popular component added to numerous industrial and consumer products used in everyday life (Center for Disease Control and Prevention, 2017). The family of PFAS chemicals are carbon-chain molecules that have been fully fluorinated. They are created by replacing all hydrogen bonds with fluorine bonds of at least one carbon atom (poly-) or of all carbon atoms (per-) in a substance, such that they form the perfluoroalkyl moiety CnF2n+1- (Buck et al., 2011). The carbon-fluorine bond is particularly strong and stable, making PFAS highly resistant to degradation. The combination of the chemical and thermal stability of PFAS, along with their lipophobic/hydrophobic properties, has led to the use of PFAS for their excellent surfactant properties in industrial and consumer products such as cookware, clothing, and carpets, as well as in aqueous film-forming foams (AFFs) used in fire-fighting (Clara et al., 2008; Trier et al., 2011; Nickerson et al., 2020). Long-chain PFAS, known as “legacy” PFAS, have been used in products for decades, with the two most studied legacy PFAS being perfluorooctane sulfonic acid (PFOS) and perfluoro octanoic acid (PFOA). Studies screening a wide range of consumer products have determined that PFAS are present at significant levels in the majority of products tested (Kotthoff et al., 2015).
The robust resistance of PFAS to degradation has also led to their bioaccumulation in the environment and in animals and humans (Wang et al., 2015). Legacy PFAS have been increasingly measured in environments across the globe, including water sources and wildlife food webs (Giesy and Kannan, 2001; Domingo and Nadal, 2019). In accordance with the bioaccumulation of PFAS in the environment, the U.S. Centers for Disease Control and Prevention (CDC) reported via data collected from the National Health and Nutrition Examination Survey (NHANES) 2003-2004 that legacy PFAS compounds are detectable in the blood of 98% of adult Americans (Center for Disease Control and Prevention, 2005; Calafat et al., 2007). The biological half-lives are long for many of the legacy PFAS. The elimination half-life from human serum has been calculated around 4 years for PFOA, 5 years for PFOS, and as great as 8.5 years for perfluorohexane sulfonate (PFHxS) (Olsen and Zobel, 2007). Due to the persistence of these chemicals, the growing amount of evidence that PFAS exposure can lead to adverse health effects in humans is alarming. Toxicity studies over the past decade have shown an association of PFAS exposure with immunotoxicity and chronic diseases including hepatic steatosis, cardiometabolic diseases, and cancer (DeWitt et al., 2012; Grandjean et al., 2012; Barry et al., 2013; Vieira et al., 2013; Liu H. S. et al., 2018). Because of the growing concern over the detrimental effects of legacy PFAS on the environment and on humans, legacy PFAS are being phased out and substituted by alternative, emerging PFAS. Emerging PFAS often feature shorter carbon chains or fluoroether replacements, such as perfluorobutane sulfonate (PFBS), ammonium perfluoro(2-methyl-3-oxahexanoate) GenX, and 4,8-dioxa-3H-perfluorononanoate (ADONA) (Lindstrom et al., 2011; Dodds et al., 2020). However, these emerging PFAS remain an area of active research and ongoing concern over their health impact on the environment and human population as well (Wang et al., 2015; Gomis et al., 2018).
Exposure Routes of PFAS
The U.S. Environmental Protection Agency (EPA) has published health advisories for PFOA and PFOS levels in drinking water set at 70 parts per trillion (ppt) (U.S. Environmental Protection Agency, 2016). In February 2020, the EPA announced its proposition to regulate PFOS and PFOA, which would establish official regulations on PFAS contaminant levels (U.S. Environmental Protection Agency, 2020). In Canada, the Canadian Federal-Provincial-Territorial Committee on Health and the Environment has set drinking water maximum acceptable concentrations of 600 and 200 ppt for PFOS and PFOA, respectively (Health-Canada, 2020). In other regions of the globe, the Heads of EPAs Australia and New Zealand (HEPA), as part of their PFAS National Environmental Management Plan (NEMP), have instated drinking water regulations at 70 ppt for PFOS and PFHxS and 560 ppt for PFOA (Heads of EPAs Australia and New Zealand, 2020). There are a variety of routes through which human exposure to PFAS are known to occur, including indoor dust and air, drinking water, and diet (Domingo and Nadal, 2017, 2019; Sunderland et al., 2019). These PFAS exposure routes are outlined in Figure 1. Although not as significant as drinking water and diet, studies have detected PFAS ingestion in indoor environments as a potential contributing factor to total body burden (Ericson Jogsten et al., 2012). PFAS are used in the manufacture of a wide variety of consumer products commonly found in households and offices, including carpets, upholstery, food packaging, and clothing (Guo et al., 2009; Zabaleta et al., 2016; Favreau et al., 2017; Robel et al., 2017). PFAS can migrate from said products and permeate into the indoor environment, collecting in air and dust that can be ingested by the environment's inhabitants. Inhalation of PFAS from these environments account for a detectable level of total human PFAS intake that can lead to detrimental health effects (Trudel et al., 2008; Harrad et al., 2010; Haug et al., 2011; Winkens et al., 2018). However, the average indoor environmental exposure is estimated to comprise only around 1% of total PFAS intake, whereas the main route of exposure to PFAS is through dietary intake (Ericson Jogsten et al., 2012). Numerous studies have shown that the consumption of drinking water is a robust exposure route for PFAS. PFAS have been detected in the surface water and drinking water in countries across the globe (Skutlarek et al., 2006; Takagi et al., 2008; Appleman et al., 2014; Schwanz et al., 2016). The exposure to PFAS is especially high in areas surrounding industrial and chemical manufacturing facilities, where multiple PFAS have been discovered contaminating nearby river and drinking water resources (Bach et al., 2017; Herrick et al., 2017). Populations exposed to PFAS-contaminated water sources have been associated with increased measured serum concentrations of PFAS (Emmett et al., 2006; Steenland et al., 2009; Frisbee et al., 2010; Seals et al., 2011). Further studies have established associations between PFAS exposure and adverse health effects of those exposed populations (Lopez-Espinosa et al., 2012; Barry et al., 2013; Darrow et al., 2013; Steenland et al., 2013). Although the focus of this review is on the association of PFAS exposure with hyperlipidemia and steatosis, there are other adverse outcomes that have been linked to PFAS. For example, in a 2008-2011 study of Mid-Ohio Valley adults exposed to PFAS through drinking water contamination, PFOA exposure was found to be associated with kidney and testicular cancer, as well as ulcerative colitis (Barry et al., 2013; Steenland et al., 2013). Another study of pregnant women from 2005 to 2010 exposed to PFAS through drinking water contamination in the same region found PFOA and PFOS levels positively associated with pregnancy-induced hypertension (Darrow et al., 2013). Moreover, in 2005-2006, as part of the C8 Health Project, it was found that in children exposed to PFAS through contaminated drinking water, PFOS, PFOA, and PFNA were associated with thyroid function impairment (Lopez-Espinosa et al., 2012). Of note, the use of granular activated carbon in water filtration systems has shown to be effective in lowering serum PFAS concentrations of the exposed populations (Bartell et al., 2010). However, continual exposure to even relatively low amounts of PFAS can still result in elevated body burdens and health risks (Post et al., 2012).
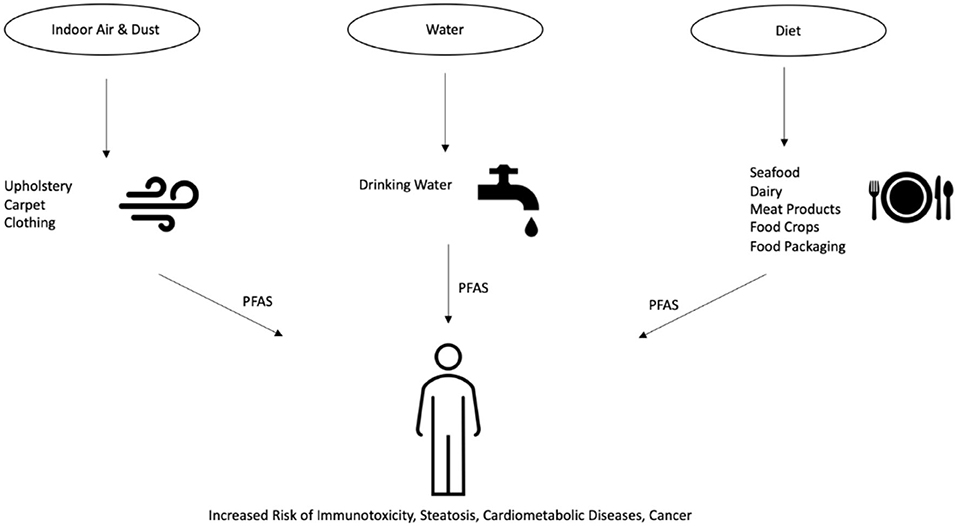
Figure 1. An overview of the various routes through which human exposure to PFAS can occur. First, PFAS can migrate from products such as carpet and clothing into the surrounding indoor air and dust. Second, PFAS enter the environment through production or waste streams and into the drinking water supplies. Lastly, PFAS present in animals, food crops, and food packaging can enter humans through their food and diets.
In addition to indoor environments and water, the greatest source of PFAS exposure is estimated to be through diet via the intake of contaminated foods (Ericson et al., 2008; Domingo and Nadal, 2017). Seafood consumption has been shown to be a key source of dietary PFAS. In 2018, the European Food and Safety Authority (EFSA) estimated that up to 86% of dietary exposure to PFAS from food comes from “fish and other seafood” (EFSA, 2018). Furthermore, PFAS have been measured in U.S. rivers and lakes, and data collected from the 2007–2014 U.S. NHANES found that higher serum PFAS levels are associated with greater fish and shellfish consumption (Stahl et al., 2014; Christensen et al., 2017). Although to a lesser extent than seafood, the EFSA also identified meat products and dairy products as contributors to dietary exposure to PFAS (EFSA, 2018). Studies across Europe and Asia have found contamination of dairy and meat products with PFAS are frequently detected but at low concentrations (Hlouskova et al., 2013; Barbarossa et al., 2014; Heo et al., 2014). In the U.S. FDA's Total Diet Study, which is an ongoing program monitoring levels of contaminants in the average U.S. diet, low levels of PFAS were detected in milk products, the highest being PFOA at 42 parts per trillion and perfluorononanoic acid (PFNA) at 39 parts per trillion (U.S. Food and Drug Administration, 2019). PFAS levels in cheeses were also measured at no < 200 ppt, with perfluorodecanoic acid (PFDA). In addition, PFAS have also been shown to enter and bioaccumulate in food crops after being taken up from the soil or water (Lechner and Knapp, 2011; Herzke et al., 2013; Blaine et al., 2014). Lastly, food contamination can occur indirectly through migration of PFAS from food packaging or cookware (Susmann et al., 2019). Studies have detected up to 46 PFAS in food packaging materials, such as microwave popcorn bags, at levels between 3.5 and 750 ng/g (Moreta and Tena, 2014; Zabaleta et al., 2017). However, no PFAS were detected in the popcorn after cooking, and whether or not this route of dietary exposure contributes significantly to the overall body burden remains inconclusive (Tittlemier et al., 2006; Vestergren et al., 2008; Jogsten et al., 2009; D'Hollander et al., 2010; Moreta and Tena, 2014).
Impacts of PFAS on Metabolism and Metabolic Diseases
Lipid Metabolism and Dyslipidemia
PFAS structurally resemble fatty acids and are known to activate peroxisome proliferator-activated receptors (PPARs), which elicit pathways that play key roles in lipid metabolism and adipogenesis (Vanden Heuvel et al., 2006; Wolf et al., 2012). Therefore, the ability of PFAS to disrupt lipid metabolism has been an active area of research. Studies over the past decades have revealed that dyslipidemia is the most evident metabolic outcome associated with exposure to PFAS. Epidemiological studies have shown that humans exposed to very high levels of PFAS, most frequently PFOS and PFOA, are positively associated with increased cholesterol and other serum lipids (Olsen and Zobel, 2007; Sakr et al., 2007; Costa et al., 2009; Steenland et al., 2009; Frisbee et al., 2010). Table 1 outlines many of these most recent studies on PFAS exposure in human epidemiological studies and the resulting endpoints related to metabolic disease. However, there has been variability in the classes of lipids seen to increase. For example, in a study of employees occupationally exposed to PFAS at the DuPont Washington Works manufacturing site in the Mid-Ohio Valley between 1979 and 2004, where employees had serum PFOA concentrations ranging from 0 to 22.66 ug/mL, an association was found between one ppm increase in serum PFOA and a 1.06 mg/dL increased total cholesterol (P = 0.011) (Sakr et al., 2007). However, no association was found with serum triglycerides. Additionally, in another study of DuPont workers, where measured PFOA serum levels ranged from 0.20 to 47.04 ug/mL, PFOA exposure was reported to significantly increase mean total cholesterol (P = 0.005) (Costa et al., 2009). On the contrary, in another study of employees exposed to PFAS at 3M plant locations in Antwerp, Belgium, in Decatur, Alabama, and in Cottage Grove, Minnesota, where serum PFOA levels ranged from 0.007 to 92.03 μg/mL (mean 2.21 μg/mL), PFOA was reported as positively associated with triglycerides (P < 0.0001), but not with total cholesterol (P > 0.05) (Olsen and Zobel, 2007). In residential communities exposed to environmental PFOA contamination from a chemical plant in West Virginia, Steenland et al. reported a positive correlation between PFOA and total cholesterol, with the odds of high cholesterol (>240 mg/dL) increasing 40–50% from the lowest quartile of PFOA and PFOS serum concentrations to the highest quartile (Steenland et al., 2009). When children and adolescents also exposed to PFAS drinking water contaminated from the DuPont chemical plant in West Virginia were evaluated as part of the C8 Health Project, it was reported that they also demonstrated elevated total cholesterol associated with both PFOA and PFOS (p < 0.0001), with a 4.6 and 8.5 mg/dL increase in total cholesterol between the lowest and highest quintiles of PFOA and PFOS, respectively (Frisbee et al., 2010). However, the significance of the positive association between PFAS and lipid levels for the general population has been disputed. The median serum concentration of PFOA in NHANES (2003-2004) participants was 4 ng/mL, which is around 1 to upwards of 3 orders of magnitude lower than those exposed occupationally or by contaminated environments (Calafat et al., 2007; Sakr et al., 2007; Costa et al., 2009; Nelson et al., 2010). Yet, when analyzing NHANES (2003-2004) participants, Nelson et al. found a positive association with total and LDL cholesterol despite the lower “background” PFAS exposure levels of the general U.S. population (Nelson et al., 2010). Another study of NHANES (1999-2008) participants observed PFAS exposure significantly associated with increased total and LDL cholesterol in adolescents in the U.S. at all exposure levels, including the lowest “background” exposure (Geiger et al., 2014). Although many studies support the correlation between PFAS exposure and lipid levels, the specific mechanisms of how PFAS alter lipid metabolism have not been completely elucidated. PFAS in serum are mainly proteinophilic as opposed to lipophilic, and studies have shown that albumin is the major carrier protein for PFAS with over 90% of PFAS bound to serum albumin in human blood (Han et al., 2003; Forsthuber et al., 2020). However, PFOA has not been shown to effect serum albumin levels, although it participates in the transportation of PFAS and fatty acids (Wang et al., 2013). Studies have shown that PFAS activate nuclear receptors like PPARs, and have been associated with changes in the expression of genes involved in lipid metabolism (Wolf et al., 2012; Fletcher et al., 2013). Furthermore, in a study by Liu et al. using samples from the 2-year Prevention of Obesity Using Novel Dietary Strategies (POUNDS) Lost study, plasma PFAS concentrations were primarily associated with blood lipids of specific subfractions that contain apoC-III, a known risk factor for coronary disease, suggesting that mechanistic research should focus on specific instead of total lipid levels (Mendivil et al., 2011; Liu G. et al., 2020).
Although exposure of humans to PFAS is commonly associated with hyperlipidemia, the literature surrounding animal studies on PFAS exposure conversely report symptoms of hypolipidemia. Studies with rodents have generally reported that animals exposed to PFAS orally or through diet demonstrate serum cholesterol and triglyceride levels that are either decreased or not significantly altered (Haughom and Spydevold, 1992; Loveless et al., 2006; Martin et al., 2007; Qazi et al., 2010; Butenhoff et al., 2012). Table 2 outlines many of these important studies on PFAS exposure in animal models and the resulting endpoints related to metabolic disease. One study has reported, however, that while mice exposed to high levels of PFOA (30 mg/kg) displayed decreased serum triglyceride levels, lower levels of PFOA (0.3–10 mg/kg) demonstrated increased triglyceride levels (Loveless et al., 2006). In another study, Sprague-Dawley rats exposed to 20 mg/kg PFOA or 10 mg/kg PFOS daily for up to 5 days were reported to have decreased total serum cholesterol, downregulated cholesterol biosynthesis genes, and increased hepatocellular hypertrophy (Martin et al., 2007). In 2010, Qazi et al. exposed mice to dietary PFOA and PFOS for 10 days and similarly reported decreased total cholesterol, decreased serum triglycerides, and increased hepatocellular hypertrophy (Qazi et al., 2010). In wild-type mice receiving 1 or 5 mg/kd/day PFOA for 6 weeks, Nakagawa et al. reported decreased hepatic triglyceride levels and increased steatosis (Nakagawa et al., 2012). Further reports of hypolipidemic effects have been reported in studies of monkeys, where cynomolgus monkeys given 0.75 mg/kg/day PFOS (corresponding to >100 ppm serum PFOS) PFOS or PFOA orally for 6 months had decreased total serum cholesterol (Seacat et al., 2002). Some recent studies have begun to evaluate the effect of emerging, short-chain PFAS on metabolic disease and hepatic injury in mice (Wang J. et al., 2017; Chappell et al., 2020). In 2017, Wang et al. reported that daily administration of 1 mg/kg GenX for 3 weeks resulted in hepatomegaly, altered hepatic lipid metabolism gene levels, and hepatic injury (Wang J. et al., 2017). In early 2020, mice exposed to GenX at 0.5 and 5 mg/kg daily for 90 days demonstrated hepatic hypertrophy and hepatic lesions, upregulated fatty acid metabolism genes, and induced hepatic PPARα signaling (Chappell et al., 2020). These results are consistent with one of the well-established toxicodynamic mechanisms of PFAS, by which they can activate signaling pathways via PPARα (Intrasuksri et al., 1998; Takacs and Abbott, 2007; Rosen et al., 2008). Takacs et al. demonstrated the ability of PFAS, using transient transfection cell assays, to differentially bind and activate PPARα, β/δ, and γ (Takacs and Abbott, 2007). Activation of PPARα-signaling pathways leads to increased fatty acid oxidation and inhibition of the secretion of cholesterol and other lipids from the liver, resulting in a decrease of the overall levels of circulating lipids (Kennedy et al., 2004; Buhrke et al., 2015). The inconsistencies between the hyperlipidemia reported in human studies and the hypolipidemia observed in animal studies may be explained by interspecies differences or genetic influences. For example, Nakamura et. al. demonstrated that PFAS activate PPARα to a greater degree in mice than in humans (Nakamura et al., 2009). In these studies, wild-type mice (mPPARα), Pparα-null mice and humanized PPARα (hPPARα) mice were exposed to control, 0.1, or 0.3 mg/kg PFOA for two weeks. At the high dose, hepatic triglyceride levels, cholesterol levels, and PPARα-target genes were increased only in the mPPARα group, suggesting human PPARα to be less responsive to PFAS than mouse PPARα. In addition, expression of PPARα in humans is thought to be 1/10 that expressed by rodents (Palmer et al., 1998). Differences in lipoprotein metabolism also hinder extrapolation of data between rodents and humans, such as the absence of cholesteryl ester transfer protein (CETP) and a faster clearance of Apolipoprotein B (ApoB) from the liver, resulting in a higher proportion of HDL-C to LDL-C compared to humans (Princen et al., 2016). Because of these differences, some studies have been conducted using genetically engineered animal models that more closely mimic humans (Nakagawa et al., 2012; Pouwer et al., 2019).
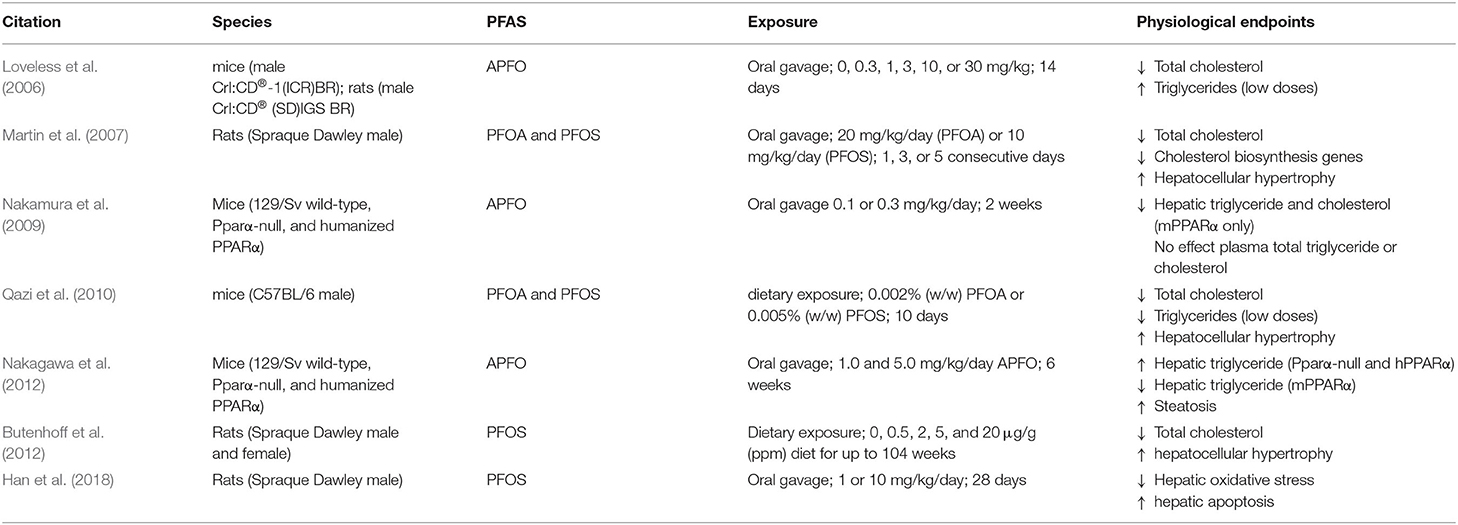
Table 2. Key physiological endpoints from studies on PFAS exposure in rodent models related to metabolic disease.
Non-alcoholic Fatty Liver Disease
Epidemiological studies in humans have linked PFAS exposure to fatty liver disease. The liver provides the principal line of defense against harmful xenobiotics, making it the organ most frequently affected by industrially- and commercially- manufactured chemicals. After entrance into the body through dietary exposure, PFAS proceeds to the liver. Due to structural similarities between fatty acids and PFAS, PFAS are able to bind to liver-fatty acid binding protein (L-FABP) for transport into hepatocytes and their nucleus (Luebker et al., 2002; Sheng et al., 2018). Once in the nucleus, PFAS are able to interfere with the DNA and transcriptional pathways. PFAS can further proceed to be a component of bile (Genuis et al., 2010). In the bile, it remains within the enterohepatic circulation pathway and eventually accumulates in the liver. Chronic exposure to PFAS may lead to injuries to the liver cells and increase risks for non-alcoholic fatty liver disease (NAFLD). NAFLD is characterized by excessive fat accumulation in the liver unrelated to significant alcohol consumption. NAFLD has been more specifically defined as macrovesicular or microvesicular accumulation of triglycerides in at least 5% of hepatocytes (Aly and Kleiner, 2011; Kawano and Cohen, 2013). NAFLD is the most predominant liver disease in humans, with a prevalence rate of 30% in the U.S. adult population (Estes et al., 2018). Fatty liver disease incorporates a spectrum of liver pathologies that can progress from simple fatty liver to steatohepatitis (NASH) which is characterized by increased fibrosis and inflammation. NASH can sometimes end in cirrhosis and hepatocellular carcinoma (Cohen et al., 2011; Kleiner and Brunt, 2012). Studies involving populations exposed to PFAS-contaminated water sources revealed strong associations between serum concentrations of PFOS and PFOA with elevated ALT levels, a surrogate marker for fatty liver disease (Gallo et al., 2012; Darrow et al., 2016). Analysis of the general U.S. adult population, based on NHANES data, also established an association between elevated ALT levels and elevated serum PFAS concentrations (Lin et al., 2010; Gleason et al., 2015; Jain and Ducatman, 2019b). However, there have been some studies debating the nuances of this association. A U.S. pregnancy cohort study reported that children exposed to higher levels of PFAS had lower ALT levels, revealing the need for further studies and analysis (Mora et al., 2018). Additional markers of liver injury, such as the liver apoptosis marker cytokeratin 18, were reported by Bassler et al. to be positively associated with PFAS levels as well (Bassler et al., 2019). Interestingly, this study also found PFAS exposure to be associated with decreased inflammation. In addition, a recent study by Jin et al. revealed that higher exposure to PFAS was associated with more severe fatty liver disease in children 7–19 years old based on liver biopsy histology scored for steatosis (Jin et al., 2020). In this study, PFAS exposure was associated with increased risk of steatohepatitis, fibrosis, lobular inflammation, and increased NAFLD score. Furthermore, this study found higher concentration of plasma PFAS to be associated with alterations in key metabolic pathways, including glycerophospholipid and tyrosine metabolism, that may be impacting fatty liver disease severity. Both dysregulated tyrosine metabolism and dysregulated glycerophospholipid metabolism have been previously associated with the severity of NAFLD progression (Gorden et al., 2015; Jin et al., 2016). In addition, cell and animal-based studies have reported an increased incidence of oxidative stress characterized by increase production of reactive oxygen species (ROS) after exposure to PFAS through dietary pathways (Wielsøe et al., 2015; Wang X. et al., 2017; Han et al., 2018), which can lead to mitochondrial dysfunction and consequently induce cell apoptosis. Excessive ROS production and apoptosis can increase the susceptibility to NAFLD. Although the specific mechanisms leading to the association of PFAS levels with NAFLD remain unknown, other human cross-sectional studies have reported that PFAS exposure was associated with dysregulation of fatty acid metabolism in children, adult, and elderly populations, even at low levels of PFAS exposure (Wang J. et al., 2017; Alderete et al., 2019; Kingsley et al., 2019; Salihovic et al., 2019).
It should be noted that genetic variants play an important role in the development of NAFLD/NASH in humans. A few single nucleotide polymorphisms and their associated genes have been identified in genome-wide association studies of NAFLD, e.g., PNPLA3, TM6SF2, HSD17B13, MBOA7, PPP1R3B, LYPLAL1, and GCKR (Liu Z. et al., 2020). Therefore, the inter-individual differences as well as the inter-study discrepancies in the impact of PFAS on NAFLD risk may be attributed to these genetic variations. More studies are needed to further clarify this PFAS-genetic interaction in the development of NAFLD.
Dietary Modulation of PFAS Toxicity
Studies over the past several decades in animals and humans have demonstrated the range of health effects linked to PFAS exposure. Because of this, it is important to gain a better understanding of the mechanisms involved in PFAS-induced manipulation of lipid metabolism. Studies using rodents are key to learning these mechanisms and how they can be translated to humans. However, in most published rodent studies, the animals have been fed a standard diet, containing low fat and negligible cholesterol. Only a few studies have examined the effects of PFAS exposure in combination with a diet similar to one consumed by a majority of humans in western societies that is high in fat and cholesterol. Table 3 outlines some of these important studies on PFAS exposure in animal models and the resulting endpoints related to the interactions between diet and metabolic disease. Previous studies in humans have suggested that plasma cholesterol and PFAS serum concentrations are directly related, while rodent studies suggest an inverse relationship when fed a standard diet. To examine the effects of dietary modulation of PFAS toxicity in a mouse model, Rebholz et al. fed mice a PFOA-laced Western diet (0.25% cholesterol, 32% fat) for 6 weeks (Rebholz et al., 2016). PFOA exposure led to hypercholesteremia in both male and female mice compared to control, in contrast to previous studies using a standard diet. Plasma cholesterol levels in mice exposed to PFOA increased by 70% in females and a smaller 35% increase in males. Liver weights was increased in mice fed PFOA, but hepatic tissue cholesterol levels were largely unchanged. Of note, the increase in plasma cholesterol was determined to occur in the HDL fraction, while previous studies in humans have shown that PFOA-induced hypercholesteremia is usually the result of higher LDL and lower HDL cholesterol levels (Olsen and Zobel, 2007; Steenland et al., 2009; Frisbee et al., 2010; Geiger et al., 2014). However, several high fat diet studies have reported that exposure to PFAS in mice fed a high fat diet generally resulted in increased hepatic steatosis and hypolipidemia. Male BALB/c or C57BL/6 mice exposed to PFOS or PFOA on a standard or high fat diet had reduced serum cholesterol and triglycerides, as well as increased hepatic injury and steatosis (Tan et al., 2013; Wang et al., 2014). These changes were found to occur mainly through inhibited excretion of low-density lipoproteins. A recent study in 2019 by Pouwer et al. explored the effects of PFOA on plasma cholesterol and triglyceride metabolism using genetically engineered APOE*3-Leiden.CETP mice, which exhibit human-like lipoprotein metabolism through cholesteryl ester transfer protein expression and delayed apoB clearance (Pouwer et al., 2019). In this study, mice fed a Western type diet for 4 weeks were then exposed to varying concentrations of PFOA incorporated into the Western diet (0.25% cholesterol, 14% fat) for 4 or 6 weeks. Mice exposed to PFOA had decreased plasma triglycerides, decreased plasma total cholesterol, and decreased non-HDL cholesterol, whereas HDL cholesterol was increased (Pouwer et al., 2019). These changes were mediated mainly through activation of PPARα. Although no difference from control was seen in hepatic free cholesterol or triglycerides, PFOA exposure did induce hepatic hypertrophy and microvesicular steatosis. Similar decreases in plasma lipoproteins were reported in mouse experiments using APOE*3-Leiden.CETP mice were fed a Western-type diet and exposure to PFBS, PFHxS, or PFOS for 4–6 weeks (Bijland et al., 2011). In this study, exposure to PFAS resulted in reduced plasma triglycerides, total cholesterol, non-HDL-C and HDL-C. These reductions were largely the result of impaired lipoprotein production via activation of PPARα and pregnane X receptor. PFAS exposure also led to increased hepatic steatosis, displaying increased liver weight and hepatic triglyceride content. A recent study by Marques et al. demonstrated that PFOS exacerbates hepatic steatosis in mice fed a high-fat diet (Marques et al., 2020). Further mRNA and proteomic analysis revealed PFOS-induced lipid and xenobiotic transporters and metabolism pathways that interfered with lipid loss when mice were switched from a high-fat diet to a standard diet and exacerbated the lipid accumulation in mice on a high-fat diet. In addition, other studies have shown that exposure to PFOS in conjunction with a high fat diet increases atrophy and accumulation of adipocytes in immune organs such as the thymus and spleen (Wang et al., 2011). Thus, PFAS can lead to dysfunction of immune organs via interference with lipid metabolism, leading to senescence and the attenuation of humoral immune responses that play a role in systemic metabolic homeostasis (Zmora et al., 2017).
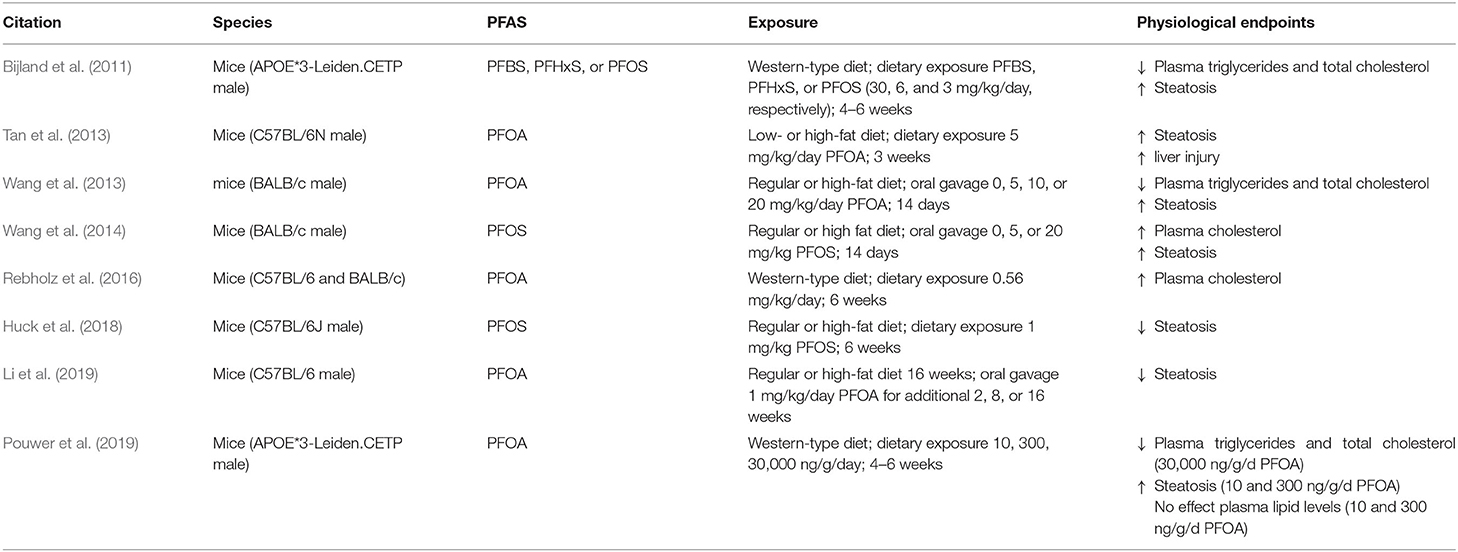
Table 3. Key physiological endpoints from studies on PFAS exposure in rodent models related to dietary-modulation of metabolic disease.
Nevertheless, the ability of PFAS exposure simultaneous with a high-fat diet to cause hypolipidemia and increase hepatic steatosis remains a disputed topic. A study conducted by Wang et al. looked at male BALB/c mice exposed to 5–20 mg/kg PFOA via oral gavage for 14 days while being fed either a regular or high-fat diet (Wang et al., 2013). In this study, it was reported that the decreased levels of plasma lipoproteins and changes in hepatic histology were not significantly different in high fat diet vs. regular diet. Furthermore, other recent studies have shown that PFAS actually have a protective effect against high-fat diet-induced hepatic steatosis. Huck et al. reported that although a high-fat diet alone or 1 mg/kg daily PFOS treatment alone for 6 weeks led to increased hepatic steatosis, this increase was prevented through a combined high-fat diet and PFOS treatment (Huck et al., 2018). Furthermore, when mice were fed a high-fat diet for 16 weeks to induce steatosis, subsequent administration of 1 mg/kg PFOA daily by oral gavage for an additional 2–16 weeks was shown to decrease the severity of hepatic steatosis (Li et al., 2019). Transcriptomic analysis revealed that the preexisting steatosis led to enhanced PFOA-related lipid oxidation pathways in the mice fed a high fat diet. Although further studies are needed, this model takes into consideration the effects of PFAS on preexisting and naturally progressing NAFLD and may be more relevant to actual human exposure conditions, specifically in overweight individuals. To date, very few human studies of PFAS toxicity have examined the role of diet as a modulatory factor. In 2018, Liu et al. analyzed the ability of PFAS to interfere with body weight control in humans via data from the 2-year POUNDS Lost randomized clinical trial (Liu G. et al., 2018). In the POUNDS Lost trial, 621 overweight and obese participants went through a 6-month weight loss period, followed by a 6–24 month weight regain period. Higher plasma PFAS concentrations were found to be associated with greater weight regain, especially in women. The higher plasma PFAS levels and greater weight regain were also associated with a slower regression of resting metabolic rate during the weight regain period. These data illustrate an interaction between PFAS and dietary regulation in humans. Furthermore, in a 2019 study analyzing data from NHANES for 2005–2014, it was demonstrated that obesity in both males and females altered the cross-sectional associations of different PFAS with plasma lipid concentrations (Jain and Ducatman, 2019a). In this study, both PFOA and PFNA were positively associated with total cholesterol levels for obese males, but not for non-obese males. Additionally, PFDA and PFNA were positively associated with total cholesterol levels for obese females, but not for non-obese females. Overall, these findings suggest a greater vulnerability in obese humans to higher cholesterol levels, with some variances between men and women. However, the impact of dietary modulation on PFAS toxicity in humans remains a largely unexplored area of research and warrants further investigation to better understand the interactive effects of diet and PFAS toxicity.
Future Directions and Conclusions
Although current animal studies highlight a relationship between diet and PFAS exposure, inconsistencies exist among the studies on the changes in lipid metabolism and cardiometabolic profiles that are produced. Numerous studies have highlighted the association between PFAS exposure in humans to hyperlipidemia and fatty liver disease, while studies in rodents conversely report hypolipidemia. When diet is further introduced as a variable, most rodent studies investigating mice exposed to PFAS and a high fat diet reported an exacerbation of results found in standard diet studies, namely increased hepatic steatosis and hypolipidemia. These studies varied across the mouse strains and models used (Tan et al., 2013; Wang et al., 2014; Pouwer et al., 2019). However, a few studies have shown that PFAS actually have a protective effect against high fat diet-induced hepatic steatosis (Huck et al., 2018; Li et al., 2019). Although the exact cause of these inconsistencies is not known, important differences between these studies include whether steatosis was preexisting before PFAS exposure as well as the length of the study. Furthermore, there are many limitations in the effort to extrapolate health effects observed in laboratory animals to humans. Many animal studies report that PFAS, which are structurally similar to fatty acids, act in major part through PPARα, a key transcription factor regulating lipid metabolism (Rosen et al., 2008; Wolf et al., 2008; Bjork et al., 2011). Several studies reporting on the exacerbation of PFAS exposure effects by high fat diet also attribute the effects primarily to mechanisms involving PPARα activation (Tan et al., 2013; Li et al., 2019; Pouwer et al., 2019). The role of both dietary PFAS and the consumption of a high fat diet on PPARα activation and the downstream effects on hepatic lipid accumulation and secretion are illustrated in Figure 2. However, important species differences exist between PPARα expression and its induced effects in rodents compared to humans that are problematic for the extrapolation of results from animal studies to humans (Palmer et al., 1998; Cattley, 2004; Lake, 2009). Moreover, human PPARα transgenic mice have been generated and studies report differences in fenofibrate activation of hepatic lipid metabolism and proliferation responses between human PPARα transgenic mice and wild-type mice (Yang et al., 2008). Further investigations utilizing human PPARα transgenic mice are needed to better exploit the utility of animal studies in exploring the role of PPARα in human-relevant metabolic syndrome and NAFLD models. However, although PPARα activation is often identified as a major pathway, PFAS exposure has also been shown to produce PPARα-independent effects through receptors such as the pregnane X receptor and the farnesoid X receptor (Rosen et al., 2010; Bjork et al., 2011). Further studies are required to examine the multiplicity and relative effects these different pathways induced by PFAS exposure. In addition, in a comprehensive review of the literature surrounding PFAS toxicokinetics in both animals and humans, interspecies differences have been identified, including species-specific tissue distribution, half-life, and maternal transfer. For example, humans may be less susceptible to hepatic effects from PFAS exposure than rodents since most PFAS (aside from PFOA) preferentially distribute to the kidneys in humans as opposed to preferential distribution to the liver in rodents (Pizzurro et al., 2019). Furthermore, there are also important differences between specific PFAS, such as varying chain lengths and toxicological modes of action, that must be studied at greater depth in order to more confidently establish human-relevant findings from animal study data (Pizzurro et al., 2019). Since the 2000's, long-chain PFAS are being replaced by shorter-chain, emerging PFAS, such as GenX (Brendel et al., 2018). The main rationale for the transition from long-chain, legacy PFAS to the shorter-chain PFAS was that shorter-chain PFAS are considered less toxic and less bioaccumulative. However, recent findings have brought into question the actual toxicity of these emerging PFAS as well as environmental and regulatory concerns (Gebbink et al., 2017; Brendel et al., 2018; Gomis et al., 2018). Overall, few studies exist for these new, emerging PFAS, or PFAS mixtures that include both legacy and emerging contaminants of concern, especially those examining human-relevant health ramifications. The toxicity of these emerging PFAS, and the possible dietary modulation of that toxicity, remain largely unexplored and calls for additional studies to provide greater insight.
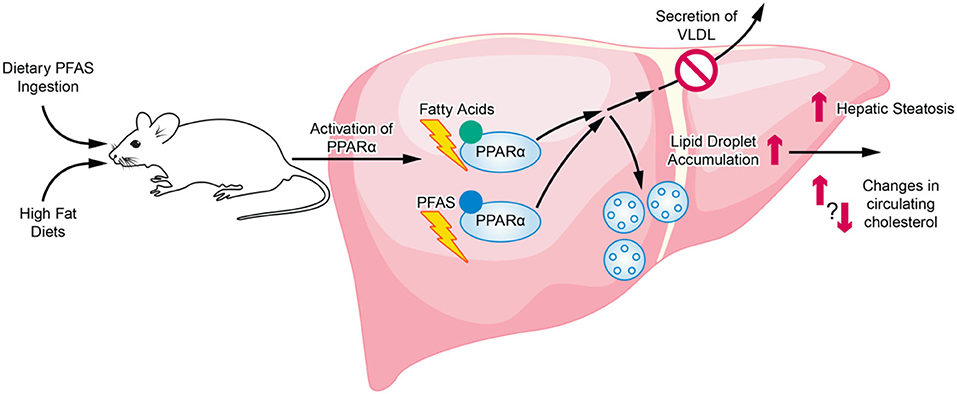
Figure 2. Overview of PFAS and HFD interaction in relation to activation of PPARα and regulation of hepatic lipid accumulation and secretion. The structurally similar PFAS chemicals and dietary fatty acids can both activation PPARα. Sustained PPARα activation eventually can lead to the inhibition of lipid secretion into the blood as well as an increase in lipid droplet accumulation in the liver.
Summary and Concluding Remarks
The PFAS family of synthetic chemicals are pervasive throughout the environment and exposure is known to occur via multiple routes, including indoor dust and air, drinking water, and diet. PFAS exposure is associated with hyperlipidemia in humans, while animal studies commonly report hypolipidemia. Furthermore, epidemiological studies in humans have linked PFAS exposure to fatty liver disease. Several recent studies exploring dietary modulation of PFAS toxicity report an exacerbation of hepatic steatosis and hypolipidemia. However, the specific interactions of diet with PFAS toxicity remains unclear, and some studies have shown PFASs to have a protective effect against high-fat diet-induced hepatic steatosis, especially when the condition is pre-existing. The manufacture of long-chain PFAS has been halted because of substantial public and media attention due to their documented water pollution and toxicity in the U.S., European Union, and Australia. However, regulations on PFAS substances in other areas of the world are absent, and information regarding PFAS contamination and exposure levels in many nations is lacking. Furthermore, PFAS contamination can negatively impacts humans in more ways than simply direct human exposure. The ubiquitous nature of PFAS in the environment has also been shown to disturb natural ecosystems by disrupting aspects of animal development, life cycles, and reproduction. While companies have halted the manufacture of long-chain PFAS, short-chain emerging PFAS, such as GenX, are being produced in their place. Toxicities of short-chain PFAS are not well-understood and more studies are required in order to evaluate the risks associated with them. Despite increased awareness and documentation, both long- and short-chain PFAS remain present in drinking water sources worldwide, and regulators in nations across the globe may need to reexamine risk assessment and toxicity studies in order to accurately evaluate the NOAEL and LOAEL for human populations.
Author Contributions
KR and ZI wrote and edited the manuscript. MP and WL wrote, edited, and oversaw the completion of the review. All authors have read and approved of the manuscript.
Funding
This work was supported by the National Institute of Environmental Health Sciences [P30ES02095, R00ES028734] and the National Institute of Diabetes and Digestive and Kidney [R01DK106540] at the National Institutes of Health and the Office of the Vice President for Research at Wayne State University. The content is solely the responsibility of the authors and does not necessarily represent the official views of the National Institutes of Health.
Conflict of Interest
The authors declare that the research was conducted in the absence of any commercial or financial relationships that could be construed as a potential conflict of interest.
Acknowledgments
We thank Matthew Garin at the Wayne State University School of Medicine's BioMedical Communications group for artistic support related to Figure 2.
References
Alderete, T. L., Jin, R., Walker, D. I., Valvi, D., Chen, Z., Jones, D. P., et al. (2019). Perfluoroalkyl substances, metabolomic profiling, and alterations in glucose homeostasis among overweight and obese Hispanic children: A proof-of-concept analysis. Environ. Int. 126, 445–453. doi: 10.1016/j.envint.2019.02.047
Aly, F. Z., and Kleiner, D. E. (2011). Update on fatty liver disease and steatohepatitis. Adv. Anat. Pathol. 18, 294–300. doi: 10.1097/PAP.0b013e318220f59b
Appleman, T. D., Higgins, C. P., Quinones, O., Vanderford, B. J., Kolstad, C., Zeigler-Holady, J. C., et al. (2014). Treatment of poly- and perfluoroalkyl substances in U.S. full-scale water treatment systems. Water Res. 51, 246–255. doi: 10.1016/j.watres.2013.10.067
Bach, C., Dauchy, X., Boiteux, V., Colin, A., Hemard, J., Sagres, V., et al. (2017). The impact of two fluoropolymer manufacturing facilities on downstream contamination of a river and drinking water resources with per- and polyfluoroalkyl substances. Environ. Sci. Pollut. Res. Int. 24, 4916–4925. doi: 10.1007/s11356-016-8243-3
Barbarossa, A., Gazzotti, T., Zironi, E., Serraino, A., and Pagliuca, G. (2014). Short communication: monitoring the presence of perfluoroalkyl substances in Italian cow milk. J. Dairy Sci. 97, 3339–3343. doi: 10.3168/jds.2014-8005
Barry, V., Winquist, A., and Steenland, K. (2013). Perfluorooctanoic acid (PFOA) exposures and incident cancers among adults living near a chemical plant. Environ. Health Perspect. 121, 1313–1318. doi: 10.1289/ehp.1306615
Bartell, S. M., Calafat, A. M., Lyu, C., Kato, K., Ryan, P. B., and Steenland, K. (2010). Rate of decline in serum PFOA concentrations after granular activated carbon filtration at two public water systems in Ohio and West Virginia. Environ. Health Perspect. 118, 222–228. doi: 10.1289/ehp.0901252
Bassler, J., Ducatman, A., Elliott, M., Wen, S., Wahlang, B., Barnett, J., et al. (2019). Environmental perfluoroalkyl acid exposures are associated with liver disease characterized by apoptosis and altered serum adipocytokines. Environ. Pollut. 247, 1055–1063. doi: 10.1016/j.envpol.2019.01.064
Bijland, S., Rensen, P. C., Pieterman, E. J., Maas, A. C., van der Hoorn, J. W., van Erk, M. J., et al. (2011). Perfluoroalkyl sulfonates cause alkyl chain length-dependent hepatic steatosis and hypolipidemia mainly by impairing lipoprotein production in APOE*3-Leiden CETP mice. Toxicol. Sci. 123, 290–303. doi: 10.1093/toxsci/kfr142
Bjork, J. A., Butenhoff, J. L., and Wallace, K. B. (2011). Multiplicity of nuclear receptor activation by PFOA and PFOS in primary human and rodent hepatocytes. Toxicology 288, 8–17. doi: 10.1016/j.tox.2011.06.012
Blaine, A. C., Rich, C. D., Sedlacko, E. M., Hyland, K. C., Stushnoff, C., Dickenson, E. R., et al. (2014). Perfluoroalkyl acid uptake in lettuce (Lactuca sativa) and strawberry (Fragaria ananassa) irrigated with reclaimed water. Environ. Sci. Technol. 48, 14361–14368. doi: 10.1021/es504150h
Brendel, S., Fetter, É., Staude, C., Vierke, L., and Biegel-Engler, A. (2018). Short-chain perfluoroalkyl acids: environmental concerns and a regulatory strategy under REACH. Environ. Sci. Eur. 30:9. doi: 10.1186/s12302-018-0134-4
Buck, R. C., Franklin, J., Berger, U., Conder, J. M., Cousins, I. T., de Voogt, P., et al. (2011). Perfluoroalkyl and polyfluoroalkyl substances in the environment: terminology, classification, and origins. Integr. Environ. Assess. Manag. 7, 513–541. doi: 10.1002/ieam.258
Buhrke, T., Kruger, E., Pevny, S., Rossler, M., Bitter, K., and Lampen, A. (2015). Perfluorooctanoic acid (PFOA) affects distinct molecular signalling pathways in human primary hepatocytes. Toxicology 333, 53–62. doi: 10.1016/j.tox.2015.04.004
Butenhoff, J. L., Chang, S. C., Olsen, G. W., and Thomford, P. J. (2012). Chronic dietary toxicity and carcinogenicity study with potassium perfluorooctanesulfonate in sprague dawley rats. Toxicology 293, 1–15. doi: 10.1016/j.tox.2012.01.003
Calafat, A. M., Wong, L. Y., Kuklenyik, Z., Reidy, J. A., and Needham, L. L. (2007). Polyfluoroalkyl chemicals in the U.S. population: data from the National Health and Nutrition Examination Survey (NHANES) 2003-2004 and comparisons with NHANES 1999–2000. Environ. Health Perspect. 115, 1596–1602. doi: 10.1289/ehp.10598
Cattley, R. C. (2004). Peroxisome proliferators and receptor-mediated hepatic carcinogenesis. Toxicol. Pathol. 32(Suppl. 2), 6–11. doi: 10.1080/01926230490451680
Center for Disease Control Prevention. (2005). NHANES 2003-2004 Public Data General Release File Documentation. Available online at: https://www.cdc.gov/nchs/data/nhanes/nhanes_03_04/general_data_release_doc_03-04.pdf
Center for Disease Control Prevention. (2017). PFAS in the U.S. Population. Available online at: https://www.atsdr.cdc.gov/pfas/health-effects/us-population.html
Chappell, G. A., Thompson, C. M., Wolf, J. C., Cullen, J. M., Klaunig, J. E., and Haws, L. C. (2020). Assessment of the mode of action underlying the effects of GenX in mouse liver and implications for assessing human health risks. Toxicol. Pathol. 48, 494–508. doi: 10.1177/0192623320905803
Christensen, K. Y., Raymond, M., Blackowicz, M., Liu, Y., Thompson, B. A., Anderson, H. A., et al. (2017). Perfluoroalkyl substances and fish consumption. Environ. Re.s 154, 145–151. doi: 10.1016/j.envres.2016.12.032
Clara, M., Scheffknecht, C., Scharf, S., Weiss, S., and Gans, O. (2008). Emissions of perfluorinated alkylated substances (PFAS) from point sources–identification of relevant branches. Water Sci. Technol. 58, 59–66. doi: 10.2166/wst.2008.641
Cohen, J. C., Horton, J. D., and Hobbs, H. H. (2011). Human fatty liver disease: old questions and new insights. Science 332, 1519–1523. doi: 10.1126/science.1204265
Costa, G., Sartori, S., and Consonni, D. (2009). Thirty years of medical surveillance in perfluooctanoic acid production workers. J. Occup. Environ. Med. 51, 364–372. doi: 10.1097/JOM.0b013e3181965d80
Darrow, L. A., Groth, A. C., Winquist, A., Shin, H. M., Bartell, S. M., and Steenland, K. (2016). Modeled perfluorooctanoic acid (PFOA) exposure and liver function in a mid-ohio valley community. Environ. Health Perspect. 124, 1227–1233. doi: 10.1289/ehp.1510391
Darrow, L. A., Stein, C. R., and Steenland, K. (2013). Serum perfluorooctanoic acid and perfluorooctane sulfonate concentrations in relation to birth outcomes in the Mid-Ohio Valley, 2005–2010. Environ. Health Perspect. 121, 1207–1213. doi: 10.1289/ehp.1206372
DeWitt, J. C., Peden-Adams, M. M., Keller, J. M., and Germolec, D. R. (2012). Immunotoxicity of perfluorinated compounds: recent developments. Toxicol. Pathol. 40, 300–311. doi: 10.1177/0192623311428473
D'Hollander, W., de Voogt, P., De Coen, W., and Bervoets, L. (2010). Perfluorinated substances in human food and other sources of human exposure. Rev. Environ. Contam. Toxicol. 208, 179–215. doi: 10.1007/978-1-4419-6880-7_4
Dodds, J. N., Hopkins, Z. R., Knappe, D. R. U., and Baker, E. S. (2020). Rapid characterization of per- and polyfluoroalkyl substances (PFAS) by ion mobility spectrometry-mass spectrometry (IMS-MS). Anal. Chem. 92, 4427–4435. doi: 10.1021/acs.analchem.9b05364
Domingo, J. L., and Nadal, M. (2017). Per- and polyfluoroalkyl substances (PFASs) in food and human dietary intake: a review of the recent scientific literature. J. Agric. Food Chem. 65, 533–543. doi: 10.1021/acs.jafc.6b04683
Domingo, J. L., and Nadal, M. (2019). Human exposure to per- and polyfluoroalkyl substances (PFAS) through drinking water: a review of the recent scientific literature. Environ. Res. 177:108648. doi: 10.1016/j.envres.2019.108648
EFSA. (2018). Risk to human health related to the presence of perfluorooctane sulfonic acid and perfluorooctanoic acid in food. EFSA J. 16, 1–293. doi: 10.2903/j.efsa.2018.5194
Emmett, E. A., Shofer, F. S., Zhang, H., Freeman, D., Desai, C., and Shaw, L. M. (2006). Community exposure to perfluorooctanoate: relationships between serum concentrations and exposure sources. J. Occup. Environ. Med. 48, 759–770. doi: 10.1097/01.jom.0000232486.07658.74
Ericson Jogsten, I., Nadal, M., van Bavel, B., Lindstrom, G., and Domingo, J. L. (2012). Per- and polyfluorinated compounds (PFCs) in house dust and indoor air in Catalonia, Spain: implications for human exposure. Environ. Int. 39, 172–180. doi: 10.1016/j.envint.2011.09.004
Ericson, I., Marti-Cid, R., Nadal, M., Van Bavel, B., Lindstrom, G., and Domingo, J. L. (2008). Human exposure to perfluorinated chemicals through the diet: intake of perfluorinated compounds in foods from the Catalan (Spain) market. J. Agric. Food Chem. 56, 1787–1794. doi: 10.1021/jf0732408
Estes, C., Razavi, H., Loomba, R., Younossi, Z., and Sanyal, A. J. (2018). Modeling the epidemic of nonalcoholic fatty liver disease demonstrates an exponential increase in burden of disease. Hepatology 67, 123–133. doi: 10.1002/hep.29466
Favreau, P., Poncioni-Rothlisberger, C., Place, B. J., Bouchex-Bellomie, H., Weber, A., Tremp, J., et al. (2017). Multianalyte profiling of per- and polyfluoroalkyl substances (PFASs) in liquid commercial products. Chemosphere 171, 491–501. doi: 10.1016/j.chemosphere.2016.11.127
Fletcher, T., Galloway, T. S., Melzer, D., Holcroft, P., Cipelli, R., Pilling, L. C., et al. (2013). Associations between PFOA, PFOS and changes in the expression of genes involved in cholesterol metabolism in humans. Environ. Int. 57–58, 2–10. doi: 10.1016/j.envint.2013.03.008
Forsthuber, M., Kaiser, A. M., Granitzer, S., Hassl, I., Hengstschläger, M., Stangl, H., et al. (2020). Albumin is the major carrier protein for PFOS, PFOA, PFHxS, PFNA and PFDA in human plasma. Environ. Int. 137:105324. doi: 10.1016/j.envint.2019.105324
Frisbee, S. J., Shankar, A., Knox, S. S., Steenland, K., Savitz, D. A., Fletcher, T., et al. (2010). Perfluorooctanoic acid, perfluorooctanesulfonate, and serum lipids in children and adolescents: results from the C8 health project. Arch. Pediatr. Adolesc. Med. 164, 860–869. doi: 10.1001/archpediatrics.2010.163
Gallo, V., Leonardi, G., Genser, B., Lopez-Espinosa, M. J., Frisbee, S. J., Karlsson, L., et al. (2012). Serum perfluorooctanoate (PFOA) and perfluorooctane sulfonate (PFOS) concentrations and liver function biomarkers in a population with elevated PFOA exposure. Environ. Health Perspect. 120, 655–660. doi: 10.1289/ehp.1104436
Gebbink, W. A., van Asseldonk, L., and van Leeuwen, S. P. J. (2017). Presence of emerging per- and polyfluoroalkyl substances (PFASs) in river and drinking water near a fluorochemical production plant in the Netherlands. Environ. Sci. Technol. 51, 11057–11065. doi: 10.1021/acs.est.7b02488
Geiger, S. D., Xiao, J., Ducatman, A., Frisbee, S., Innes, K., and Shankar, A. (2014). The association between PFOA, PFOS and serum lipid levels in adolescents. Chemosphere 98, 78–83. doi: 10.1016/j.chemosphere.2013.10.005
Genuis, S. J., Birkholz, D., Ralitsch, M., and Thibault, N. (2010). Human detoxification of perfluorinated compounds. Public Health 124, 367–375. doi: 10.1016/j.puhe.2010.03.002
Giesy, J. P., and Kannan, K. (2001). Global distribution of perfluorooctane sulfonate in wildlife. Environ. Sci. Technol. 35, 1339–1342. doi: 10.1021/es001834k
Gleason, J. A., Post, G. B., and Fagliano, J. A. (2015). Associations of perfluorinated chemical serum concentrations and biomarkers of liver function and uric acid in the US population (NHANES), 2007–2010. Environ. Res. 136, 8–14. doi: 10.1016/j.envres.2014.10.004
Gomis, M. I., Vestergren, R., Borg, D., and Cousins, I. T. (2018). Comparing the toxic potency in vivo of long-chain perfluoroalkyl acids and fluorinated alternatives. Environ. Int. 113, 1–9. doi: 10.1016/j.envint.2018.01.011
Gorden, D. L., Myers, D. S., Ivanova, P. T., Fahy, E., Maurya, M. R., Gupta, S., et al. (2015). Biomarkers of NAFLD progression: a lipidomics approach to an epidemic. J. Lipid Res. 56, 722–736. doi: 10.1194/jlr.P056002
Grandjean, P., Andersen, E. W., Budtz-Jorgensen, E., Nielsen, F., Molbak, K., Weihe, P., et al. (2012). Serum vaccine antibody concentrations in children exposed to perfluorinated compounds. JAMA 307, 391–397. doi: 10.1001/jama.2011.2034
Guo, Z., Liu, X., and Krebs, A. K. (2009). Perfluorocarboxylic acid Content in 116 Articles of Commerce: EPA?600/R-09/−33; Environmental Protections Agency.
Han, R., Hu, M., Zhong, Q., Wan, C., Liu, L., Li, F., et al. (2018). Perfluorooctane sulphonate induces oxidative hepatic damage via mitochondria-dependent and NF-κB/TNF-α-mediated pathway. Chemosphere 191, 1056–1064. doi: 10.1016/j.chemosphere.2017.08.070
Han, X., Snow, T. A., Kemper, R. A., and Jepson, G. W. (2003). Binding of perfluorooctanoic acid to rat and human plasma proteins. Chem. Res. Toxicol. 16, 775–781. doi: 10.1021/tx034005w
Harrad, S. d. W. C., Abdallah, M. A.-E., Bergh, C., Björklund, J. A., Covaci, A., Darnerud, P. O., et al. (2010). Indoor contamination with hexabromocyclododecanes, polybrominated diphenyl ethers, and perfluoroalkyl compounds: an important exposure pathway for people? Environ. Sci. Technol. 44, 3221–3231. doi: 10.1021/es903476t
Haug, L. S., Huber, S., Becher, G., and Thomsen, C. (2011). Characterisation of human exposure pathways to perfluorinated compounds–comparing exposure estimates with biomarkers of exposure. Environ. Int. 37, 687–693. doi: 10.1016/j.envint.2011.01.011
Haughom, B., and Spydevold, O. (1992). The mechanism underlying the hypolipemic effect of perfluorooctanoic acid (PFOA), perfluorooctane sulphonic acid (PFOSA) and clofibric acid. Biochim. Biophys. Acta 1128, 65–72. doi: 10.1016/0005-2760(92)90258-W
Heads of EPAs Australia New Zealand. (2020). PFAS National Environmental Management Plan. Available online at: https://www.environment.gov.au/system/files/resources/2fadf1bc-b0b6-44cb-a192-78c522d5ec3f/files/pfas-nemp-2.pdf
Health Canada. (2020). Guidelines for Canadian Drinking Water Quality. Available online at: https://www.canada.ca/en/health-canada/services/environmental-workplace-health/reports-publications/water-quality/guidelines-canadian-drinking-water-quality-summary-table.html
Heo, J. J., Lee, J. W., Kim, S. K., and Oh, J. E. (2014). Foodstuff analyses show that seafood and water are major perfluoroalkyl acids (PFAAs) sources to humans in Korea. J. Hazard. Mater. 279, 402–409. doi: 10.1016/j.jhazmat.2014.07.004
Herrick, R. L., Buckholz, J., Biro, F. M., Calafat, A. M., Ye, X., Xie, C., et al. (2017). Polyfluoroalkyl substance exposure in the Mid-Ohio River Valley, 1991–2012. Environ. Pollut. 228, 50–60. doi: 10.1016/j.envpol.2017.04.092
Herzke, D., Huber, S., Bervoets, L., D'Hollander, W., Hajslova, J., Pulkrabova, J., et al. (2013). Perfluorinated alkylated substances in vegetables collected in four European countries; occurrence and human exposure estimations. Environ. Sci. Pollut. Res. Int. 20, 7930–7939. doi: 10.1007/s11356-013-1777-8
Hlouskova, V., Hradkova, P., Poustka, J., Brambilla, G., De Filipps, S. P., D'Hollander, W., et al. (2013). Occurrence of perfluoroalkyl substances (PFASs) in various food items of animal origin collected in four European countries. Food Addit. Contam. Part A Chem. Anal. Control Expo. Risk Assess. 30, 1918–1932. doi: 10.1080/19440049.2013.837585
Huck, I., Beggs, K., and Apte, U. (2018). Paradoxical protective effect of perfluorooctanesulfonic acid against high-fat diet-induced hepatic steatosis in mice. Int. J. Toxicol. 37, 383–392. doi: 10.1177/1091581818790934
Intrasuksri, U., Rangwala, S. M., O'Brien, M., Noonan, D. J., and Feller, D. R. (1998). Mechanisms of peroxisome proliferation by perfluorooctanoic acid and endogenous fatty acids. Gen. Pharmacol. 31, 187–197. doi: 10.1016/S0306-3623(98)00029-9
Jain, R. B., and Ducatman, A. (2019a). Roles of gender and obesity in defining correlations between perfluoroalkyl substances and lipid/lipoproteins. Sci. Total. Environ. 653, 74–81. doi: 10.1016/j.scitotenv.2018.10.362
Jain, R. B., and Ducatman, A. (2019b). Selective Associations of recent low concentrations of perfluoroalkyl substances with liver function biomarkers: NHANES 2011 to 2014 data on US adults aged ≥20 years. J. Occup. Environ. Med. 61, 293–302. doi: 10.1097/JOM.0000000000001532
Jin, R., Banton, S., Tran, V. T., Konomi, J. V., Li, S., Jones, D. P., et al. (2016). Amino acid metabolism is altered in adolescents with non-alcoholic fatty liver disease-an untargeted, high resolution metabolomics study. J. Pediatr. 172, 14–19.e15. doi: 10.1016/j.jpeds.2016.01.026
Jin, R., McConnell, R., Catherine, C., Xu, S., Walker, D. I., Stratakis, N., et al. (2020). Perfluoroalkyl substances and severity of nonalcoholic fatty liver in children: an untargeted metabolomics approach. Environ. Int. 134:105220. doi: 10.1016/j.envint.2019.105220
Jogsten, I. E., Perello, G., Llebaria, X., Bigas, E., Marti-Cid, R., Karrman, A., et al. (2009). Exposure to perfluorinated compounds in Catalonia, Spain, through consumption of various raw and cooked foodstuffs, including packaged food. Food Chem. Toxicol. 47, 1577–1583. doi: 10.1016/j.fct.2009.04.004
Kawano, Y., and Cohen, D. E. (2013). Mechanisms of hepatic triglyceride accumulation in non-alcoholic fatty liver disease. J. Gastroenterol. 48, 434–441. doi: 10.1007/s00535-013-0758-5
Kennedy, G. L. Jr, Butenhoff, J. L., Olsen, G. W., O'Connor, J. C., Seacat, A. M., Perkins, R. G., et al. (2004). The toxicology of perfluorooctanoate. Crit. Rev. Toxicol. 34, 351–384. doi: 10.1080/10408440490464705
Kingsley, S. L., Walker, D. I., Calafat, A. M., Chen, A., Papandonatos, G. D., Xu, Y., et al. (2019). Metabolomics of childhood exposure to perfluoroalkyl substances: a cross-sectional study. Metabolomics 15:95. doi: 10.1007/s11306-019-1560-z
Kleiner, D. E., and Brunt, E. M. (2012). Nonalcoholic fatty liver disease: pathologic patterns and biopsy evaluation in clinical research. Semin. Liver Dis. 32, 3–13. doi: 10.1055/s-0032-1306421
Kotthoff, M., Muller, J., Jurling, H., Schlummer, M., and Fiedler, D. (2015). Perfluoroalkyl and polyfluoroalkyl substances in consumer products. Environ. Sci. Pollut. Res. Int. 22, 14546–14559. doi: 10.1007/s11356-015-4202-7
Lake, B. G. (2009). Species differences in the hepatic effects of inducers of CYP2B and CYP4A subfamily forms: relationship to rodent liver tumour formation. Xenobiotica 39, 582–596. doi: 10.1080/00498250903098184
Lechner, M., and Knapp, H. (2011). Carryover of perfluorooctanoic acid (PFOA) and perfluorooctane sulfonate (PFOS) from soil to plant and distribution to the different plant compartments studied in cultures of carrots (Daucus carota ssp. Sativus), potatoes (Solanum tuberosum), and cucumbers (Cucumis Sativus). J Agric Food Chem. 59, 11011–11018. doi: 10.1021/jf201355y
Li, X., Wang, Z., and Klaunig, J. E. (2019). The effects of perfluorooctanoate on high fat diet induced non-alcoholic fatty liver disease in mice. Toxicology 416, 1–14. doi: 10.1016/j.tox.2019.01.017
Lin, C. Y., Lin, L. Y., Chiang, C. K., Wang, W. J., Su, Y. N., Hung, K. Y., et al. (2010). Investigation of the associations between low-dose serum perfluorinated chemicals and liver enzymes in US adults. Am. J. Gastroenterol. 105, 1354–1363. doi: 10.1038/ajg.2009.707
Lindstrom, A. B., Strynar, M. J., and Libelo, E. L. (2011). Polyfluorinated compounds: past, present, and future. Environ. Sci. Technol. 45, 7954–7961. doi: 10.1021/es2011622
Liu, G., Dhana, K., Furtado, J. D., Rood, J., Zong, G., Liang, L., et al. (2018). Perfluoroalkyl substances and changes in body weight and resting metabolic rate in response to weight-loss diets: a prospective study. PLoS Med. 15:e1002502. doi: 10.1371/journal.pmed.1002502
Liu, G., Zhang, B., Hu, Y., Rood, J., Liang, L., Qi, L., et al. (2020). Associations of Perfluoroalkyl substances with blood lipids and Apolipoproteins in lipoprotein subspecies: the POUNDS-lost study. Environ. Health 19:5. doi: 10.1186/s12940-020-0561-8
Liu, H. S., Wen, L. L., Chu, P. L., and Lin, C. Y. (2018). Association among total serum isomers of perfluorinated chemicals, glucose homeostasis, lipid profiles, serum protein and metabolic syndrome in adults: NHANES, 2013–2014. Environ. Pollut. 232, 73–79. doi: 10.1016/j.envpol.2017.09.019
Liu, Z., Zhang, Y., Graham, S., Wang, X., Cai, D., Huang, M., et al. (2020). Causal relationships between NAFLD, T2D and obesity have implications for disease subphenotyping. J. Hepatol. 73, 263–276. doi: 10.1016/j.jhep.2020.03.006
Lopez-Espinosa, M. J., Mondal, D., Armstrong, B., Bloom, M. S., and Fletcher, T. (2012). Thyroid function and perfluoroalkyl acids in children living near a chemical plant. Environ. Health Perspect. 120, 1036–1041. doi: 10.1289/ehp.1104370
Loveless, S. E., Finlay, C., Everds, N. E., Frame, S. R., Gillies, P. J., O'Connor, J. C., et al. (2006). Comparative responses of rats and mice exposed to linear/branched, linear, or branched ammonium perfluorooctanoate (APFO). Toxicology 220, 203–217. doi: 10.1016/j.tox.2006.01.003
Luebker, D. J., Hansen, K. J., Bass, N. M., Butenhoff, J. L., and Seacat, A. M. (2002). Interactions of fluorochemicals with rat liver fatty acid-binding protein. Toxicology 176, 175–185. doi: 10.1016/S0300-483X(02)00081-1
Marques, E., Pfohl, M., Auclair, A., Jamwal, R., Barlock, B. J., Sammoura, F. M., et al. (2020). Perfluorooctanesulfonic acid (PFOS) administration shifts the hepatic proteome and augments dietary outcomes related to hepatic steatosis in mice. Toxicol. Appl. Pharmacol. 408:115250. doi: 10.1016/j.taap.2020.115250
Martin, M. T., Brennan, R. J., Hu, W., Ayanoglu, E., Lau, C., Ren, H., et al. (2007). Toxicogenomic study of triazole fungicides and perfluoroalkyl acids in rat livers predicts toxicity and categorizes chemicals based on mechanisms of toxicity. Toxicol. Sci. 97, 595–613. doi: 10.1093/toxsci/kfm065
Mendivil, C. O., Rimm, E. B., Furtado, J., Chiuve, S. E., and Sacks, F. M. (2011). Low-density lipoproteins containing apolipoprotein C-III and the risk of coronary heart disease. Circulation 124, 2065–2072. doi: 10.1161/CIRCULATIONAHA.111.056986
Mora, A. M., Fleisch, A. F., Rifas-Shiman, S. L., Woo Baidal, J. A., Pardo, L., Webster, T. F., et al. (2018). Early life exposure to per- and polyfluoroalkyl substances and mid-childhood lipid and alanine aminotransferase levels. Environ. Int. 111, 1–13. doi: 10.1016/j.envint.2017.11.008
Moreta, C., and Tena, M. T. (2014). Determination of perfluorinated alkyl acids in corn, popcorn and popcorn bags before and after cooking by focused ultrasound solid-liquid extraction, liquid chromatography and quadrupole-time of flight mass spectrometry. J. Chromatogr. A 1355, 211–218. doi: 10.1016/j.chroma.2014.06.018
Nakagawa, T., Ramdhan, D. H., Tanaka, N., Naito, H., Tamada, H., Ito, Y., et al. (2012). Modulation of ammonium perfluorooctanoate-induced hepatic damage by genetically different PPARα in mice. Arch. Toxicol. 86, 63–74. doi: 10.1007/s00204-011-0704-3
Nakamura, T., Ito, Y., Yanagiba, Y., Ramdhan, D. H., Kono, Y., Naito, H., et al. (2009). Microgram-order ammonium perfluorooctanoate may activate mouse peroxisome proliferator-activated receptor alpha, but not human PPARalpha. Toxicology 265, 27–33. doi: 10.1016/j.tox.2009.09.004
Nelson, J. W., Hatch, E. E., and Webster, T. F. (2010). Exposure to polyfluoroalkyl chemicals and cholesterol, body weight, and insulin resistance in the general U.S. population. Environ. Health Perspect. 118, 197–202. doi: 10.1289/ehp.0901165
Nickerson, A., Maizel, A. C., Kulkarni, P. R., Adamson, D. T., Kornuc, J. J., and Higgins, C. P. (2020). Enhanced extraction of AFFF-associated PFASs from source zone soils. Environ. Sci. Technol. 54, 4952–4962. doi: 10.1021/acs.est.0c00792
Olsen, G. W., and Zobel, L. R. (2007). Assessment of lipid, hepatic, and thyroid parameters with serum perfluorooctanoate (PFOA) concentrations in fluorochemical production workers. Int. Arch. Occup. Environ. Health 81, 231–246. doi: 10.1007/s00420-007-0213-0
Palmer, C. N., Hsu, M. H., Griffin, K. J., Raucy, J. L., and Johnson, E. F. (1998). Peroxisome proliferator activated receptor-alpha expression in human liver. Mol. Pharmacol. 53, 14–22. doi: 10.1124/mol.53.1.14
Pizzurro, D. M., Seeley, M., Kerper, L. E., and Beck, B. D. (2019). Interspecies differences in perfluoroalkyl substances (PFAS) toxicokinetics and application to health-based criteria. Regul. Toxicol. Pharmacol. 106, 239–250. doi: 10.1016/j.yrtph.2019.05.008
Post, G. B., Cohn, P. D., and Cooper, K. R. (2012). Perfluorooctanoic acid (PFOA), an emerging drinking water contaminant: a critical review of recent literature. Environ. Res. 116, 93–117. doi: 10.1016/j.envres.2012.03.007
Pouwer, M. G., Pieterman, E. J., Chang, S. C., Olsen, G. W., Caspers, M. P. M., Verschuren, L., et al. (2019). Dose effects of ammonium perfluorooctanoate on lipoprotein metabolism in APOE*3-leiden.CETP mice. Toxicol. Sci. 168, 519–534. doi: 10.1093/toxsci/kfz015
Princen, H. M. G., Pouwer, M. G., and Pieterman, E. J. (2016). Comment on hypercholesterolemia with consumption of PFOA-laced Western diets is dependent on strain and sex of mice. Toxicol. Rep. 3, 306–309. doi: 10.1016/j.toxrep.2016.02.002
Qazi, M. R., Abedi, M. R., Nelson, B. D., DePierre, J. W., and Abedi-Valugerdi, M. (2010). Dietary exposure to perfluorooctanoate or perfluorooctane sulfonate induces hypertrophy in centrilobular hepatocytes and alters the hepatic immune status in mice. Int. Immunopharmacol. 10, 1420–1427. doi: 10.1016/j.intimp.2010.08.009
Rebholz, S. L., Jones, T., Herrick, R. L., Xie, C., Calafat, A. M., Pinney, S. M., et al. (2016). Hypercholesterolemia with consumption of PFOA-laced Western diets is dependent on strain and sex of mice. Toxicol. Rep. 3, 46–54. doi: 10.1016/j.toxrep.2015.11.004
Robel, A. E., Marshall, K., Dickinson, M., Lunderberg, D., Butt, C., Peaslee, G., et al. (2017). Closing the mass balance on fluorine on papers and textiles. Environ. Sci. Technol. 51, 9022–9032. doi: 10.1021/acs.est.7b02080
Rosen, M. B., Lee, J. S., Ren, H., Vallanat, B., Liu, J., Waalkes, M. P., et al. (2008). Toxicogenomic dissection of the perfluorooctanoic acid transcript profile in mouse liver: evidence for the involvement of nuclear receptors PPAR alpha and CAR. Toxicol. Sci. 103, 46–56. doi: 10.1093/toxsci/kfn025
Rosen, M. B., Schmid, J. R., Corton, J. C., Zehr, R. D., Das, K. P., Abbott, B. D., et al. (2010). Gene expression profiling in wild-type and PPARalpha-Null mice exposed to perfluorooctane sulfonate reveals PPARalpha-independent effects. PPAR Res. 2010:794739. doi: 10.1155/2010/794739
Sakr, C. J., Leonard, R. C., Kreckmann, K. H., Slade, M. D., and Cullen, M. R. (2007). Longitudinal study of serum lipids and liver enzymes in workers with occupational exposure to ammonium perfluorooctanoate. J. Occup. Environ. Med. 49, 872–879. doi: 10.1097/JOM.0b013e318124a93f
Salihovic, S., Fall, T., Ganna, A., Broeckling, C. D., Prenni, J. E., Hyötyläinen, T., et al. (2019). Identification of metabolic profiles associated with human exposure to perfluoroalkyl substances. J. Expo. Sci. Environ. Epidemiol. 29, 196–205. doi: 10.1038/s41370-018-0060-y
Schwanz, T. G., Llorca, M., Farre, M., and Barcelo, D. (2016). Perfluoroalkyl substances assessment in drinking waters from Brazil, France and Spain. Sci. Total Environ. 539, 143–152. doi: 10.1016/j.scitotenv.2015.08.034
Seacat, A. M., Thomford, P. J., Hansen, K. J., Olsen, G. W., Case, M. T., and Butenhoff, J. L. (2002). Subchronic toxicity studies on perfluorooctanesulfonate potassium salt in cynomolgus monkeys. Toxicol. Sci. 68, 249–264. doi: 10.1093/toxsci/68.1.249
Seals, R., Bartell, S. M., and Steenland, K. (2011). Accumulation and clearance of perfluorooctanoic acid (PFOA) in current and former residents of an exposed community. Environ. Health Perspect. 119, 119–124. doi: 10.1289/ehp.1002346
Sheng, N., Cui, R., Wang, J., Guo, Y., Wang, J., and Dai, J. (2018). Cytotoxicity of novel fluorinated alternatives to long-chain perfluoroalkyl substances to human liver cell line and their binding capacity to human liver fatty acid binding protein. Arch. Toxicol. 92, 359–369. doi: 10.1007/s00204-017-2055-1
Skutlarek, D., Exner, M., and Farber, H. (2006). Perfluorinated surfactants in surface and drinking waters. Environ. Sci. Pollut. Res. Int. 13, 299–307. doi: 10.1065/espr2006.07.326
Stahl, L. L., Snyder, B. D., Olsen, A. R., Kincaid, T. M., Wathen, J. B., and McCarty, H. B. (2014). Perfluorinated compounds in fish from U.S. urban rivers and the Great Lakes. Sci. Total Environ. 499, 185–195. doi: 10.1016/j.scitotenv.2014.07.126
Steenland, K., Tinker, S., Frisbee, S., Ducatman, A., and Vaccarino, V. (2009). Association of perfluorooctanoic acid and perfluorooctane sulfonate with serum lipids among adults living near a chemical plant. Am. J. Epidemiol. 170, 1268–1278. doi: 10.1093/aje/kwp279
Steenland, K., Zhao, L., Winquist, A., and Parks, C. (2013). Ulcerative colitis and perfluorooctanoic acid (PFOA) in a highly exposed population of community residents and workers in the mid-Ohio valley. Environ. Health Perspect. 121, 900–905. doi: 10.1289/ehp.1206449
Sunderland, E. M., Hu, X. C., Dassuncao, C., Tokranov, A. K., Wagner, C. C., and Allen, J. G. (2019). A review of the pathways of human exposure to poly- and perfluoroalkyl substances (PFASs) and present understanding of health effects. J. Expo. Sci. Environ. Epidemiol. 29, 131–147. doi: 10.1038/s41370-018-0094-1
Susmann, H. P., Schaider, L. A., Rodgers, K. M., and Rudel, R. A. (2019). Dietary habits related to food packaging and population exposure to PFASs. Environ. Health Perspect. 127:107003. doi: 10.1289/EHP4092
Takacs, M. L., and Abbott, B. D. (2007). Activation of mouse and human peroxisome proliferator-activated receptors (alpha, beta/delta, gamma) by perfluorooctanoic acid and perfluorooctane sulfonate. Toxicol. Sci. 95, 108–117. doi: 10.1093/toxsci/kfl135
Takagi, S., Adachi, F., Miyano, K., Koizumi, Y., Tanaka, H., Mimura, M., et al. (2008). Perfluorooctanesulfonate and perfluorooctanoate in raw and treated tap water from Osaka, Japan. Chemosphere 72, 1409–1412. doi: 10.1016/j.chemosphere.2008.05.034
Tan, X., Xie, G., Sun, X., Li, Q., Zhong, W., Qiao, P., et al. (2013). High fat diet feeding exaggerates perfluorooctanoic acid-induced liver injury in mice via modulating multiple metabolic pathways. PLoS ONE 8:e61409. doi: 10.1371/journal.pone.0061409
Tittlemier, S. A., Pepper, K., and Edwards, L. (2006). Concentrations of perfluorooctanesulfonamides in Canadian total diet study composite food samples collected between 1992 and 2004. J. Agric. Food Chem. 54, 8385–8389. doi: 10.1021/jf061713p
Trier, X., Granby, K., and Christensen, J. H. (2011). Polyfluorinated surfactants (PFS) in paper and board coatings for food packaging. Environ. Sci. Pollut. Res. Int. 18, 1108–1120. doi: 10.1007/s11356-010-0439-3
Trudel, D., Horowitz, L., Wormuth, M., Scheringer, M., Cousins, I. T., and Hungerbuhler, K. (2008). Estimating consumer exposure to PFOS and PFOA. Risk Anal. 28, 251–269. doi: 10.1111/j.1539–6924.2008.01017.x
U.S. Environmental Protection Agency. (2016). PFOA & PFOS Drinking Water Health Advisories. Available online at: https://www.epa.gov/ground-water-and-drinking-water/drinking-water-health-advisories-pfoa-and-pfos
U.S. Environmental Protection Agency. (2020). PFAS Action Plan: Program Update. Available online at: https://www.epa.gov/sites/production/files/2020-01/documents/pfas_action_plan_feb2020.pdf
U.S. Food Drug Administration. (2019). Analytical Results for PFAS in Total Diet Study Sampling (Parts Per Trillion). Available online at: https://www.fda.gov/media/127852/download
Vanden Heuvel, J. P., Thompson, J. T., Frame, S. R., and Gillies, P. J. (2006). Differential activation of nuclear receptors by perfluorinated fatty acid analogs and natural fatty acids: a comparison of human, mouse, and rat peroxisome proliferator-activated receptor-alpha, -beta, and -gamma, liver X receptor-beta, and retinoid X receptor-alpha. Toxicol. Sci. 92, 476–489. doi: 10.1093/toxsci/kfl014
Vestergren, R., Cousins, I. T., Trudel, D., Wormuth, M., and Scheringer, M. (2008). Estimating the contribution of precursor compounds in consumer exposure to PFOS and PFOA. Chemosphere 73, 1617–1624. doi: 10.1016/j.chemosphere.2008.08.011
Vieira, V. M., Hoffman, K., Shin, H. M., Weinberg, J. M., Webster, T. F., and Fletcher, T. (2013). Perfluorooctanoic acid exposure and cancer outcomes in a contaminated community: a geographic analysis. Environ. Health Perspect. 121, 318–323. doi: 10.1289/ehp.1205829
Wang, J., Wang, X., Sheng, N., Zhou, X., Cui, R., Zhang, H., et al. (2017). RNA-sequencing analysis reveals the hepatotoxic mechanism of perfluoroalkyl alternatives, HFPO2 and HFPO4, following exposure in mice. J. Appl. Toxicol. 37, 436–444. doi: 10.1002/jat.3376
Wang, L., Wang, Y., Liang, Y., Li, J., Liu, Y., Zhang, J., et al. (2013). Specific accumulation of lipid droplets in hepatocyte nuclei of PFOA-exposed BALB/c mice. Sci. Rep. 3:2174. doi: 10.1038/srep02174
Wang, L., Wang, Y., Liang, Y., Li, J., Liu, Y., Zhang, J., et al. (2014). PFOS induced lipid metabolism disturbances in BALB/c mice through inhibition of low density lipoproteins excretion. Sci. Rep. 4:4582. doi: 10.1038/srep04582
Wang, X., Liu, L., Zhang, W., Zhang, J., Du, X., Huang, Q., et al. (2017). Serum metabolome biomarkers associate low-level environmental perfluorinated compound exposure with oxidative/nitrosative stress in humans. Environ. Pollut. 229, 168–176. doi: 10.1016/j.envpol.2017.04.086
Wang, Y., Wang, L., Liang, Y., Qiu, W., Zhang, J., Zhou, Q., et al. (2011). Modulation of dietary fat on the toxicological effects in thymus and spleen in BALB/c mice exposed to perfluorooctane sulfonate. Toxicol. Lett. 204, 174–182. doi: 10.1016/j.toxlet.2011.04.029
Wang, Z., Cousins, I. T., Scheringer, M., and Hungerbuehler, K. (2015). Hazard assessment of fluorinated alternatives to long-chain perfluoroalkyl acids (PFAAs) and their precursors: status quo, ongoing challenges and possible solutions. Environ. Int. 75, 172–179. doi: 10.1016/j.envint.2014.11.013
Wielsøe, M., Long, M., Ghisari, M., and Bonefeld-Jørgensen, E. C. (2015). Perfluoroalkylated substances (PFAS) affect oxidative stress biomarkers in vitro. Chemosphere 129, 239–245. doi: 10.1016/j.chemosphere.2014.10.014
Winkens, K., Giovanoulis, G., Koponen, J., Vestergren, R., Berger, U., Karvonen, A. M., et al. (2018). Perfluoroalkyl acids and their precursors in floor dust of children's bedrooms - Implications for indoor exposure. Environ. Int. 119, 493–502. doi: 10.1016/j.envint.2018.06.009
Wolf, C. J., Schmid, J. E., Lau, C., and Abbott, B. D. (2012). Activation of mouse and human peroxisome proliferator-activated receptor-alpha (PPARalpha) by perfluoroalkyl acids (PFAAs): further investigation of C4-C12 compounds. Reprod. Toxicol. 33, 546–551. doi: 10.1016/j.reprotox.2011.09.009
Wolf, C. J., Takacs, M. L., Schmid, J. E., Lau, C., and Abbott, B. D. (2008). Activation of mouse and human peroxisome proliferator-activated receptor alpha by perfluoroalkyl acids of different functional groups and chain lengths. Toxicol. Sci. 106, 162–171. doi: 10.1093/toxsci/kfn166
Yang, Q., Nagano, T., Shah, Y., Cheung, C., Ito, S., and Gonzalez, F. J. (2008). The PPAR alpha-humanized mouse: a model to investigate species differences in liver toxicity mediated by PPAR alpha. Toxicol. Sci. 101, 132–139. doi: 10.1093/toxsci/kfm206
Zabaleta, I., Bizkarguenaga, E., Bilbao, D., Etxebarria, N., Prieto, A., and Zuloaga, O. (2016). Fast and simple determination of perfluorinated compounds and their potential precursors in different packaging materials. Talanta 152, 353–363. doi: 10.1016/j.talanta.2016.02.022
Zabaleta, I., Negreira, N., Bizkarguenaga, E., Prieto, A., Covaci, A., and Zuloaga, O. (2017). Screening and identification of per- and polyfluoroalkyl substances in microwave popcorn bags. Food Chem. 230, 497–506. doi: 10.1016/j.foodchem.2017.03.074
Keywords: NAFLD, PPAR, diet, PFAS, hyperlipidemia
Citation: Roth K, Imran Z, Liu W and Petriello MC (2020) Diet as an Exposure Source and Mediator of Per- and Polyfluoroalkyl Substance (PFAS) Toxicity. Front. Toxicol. 2:601149. doi: 10.3389/ftox.2020.601149
Received: 31 August 2020; Accepted: 06 November 2020;
Published: 04 December 2020.
Edited by:
Pradyumna Kumar Mishra, ICMR-National Institute for Research in Environmental Health, IndiaReviewed by:
Dr Ravindra M. Samartha, Bhopal Memorial Hospital & Research Center, IndiaGyanendra Singh, National Institute of Occupational Health (ICMR), India
Siddhartha Kumar Mishra, Dr. Hari Singh Gour University, India
Copyright © 2020 Roth, Imran, Liu and Petriello. This is an open-access article distributed under the terms of the Creative Commons Attribution License (CC BY). The use, distribution or reproduction in other forums is permitted, provided the original author(s) and the copyright owner(s) are credited and that the original publication in this journal is cited, in accordance with accepted academic practice. No use, distribution or reproduction is permitted which does not comply with these terms.
*Correspondence: Michael C. Petriello, bWljaGFlbC5wZXRyaWVsbG9Ad2F5bmUuZWR1